- 1Laboratory of Pharmaceutical Microbiology, Ghent University, Ghent, Belgium
- 2Cystic Fibrosis Reference Centre, Department of Respiratory Medicine, Ghent University Hospital, Ghent, Belgium
- 3Lung Research Lab, Department of Respiratory Medicine, Ghent University Hospital, Ghent, Belgium
Patients with chronic lung disease suffer from persistent inflammation and are typically colonized by pro-inflammatory pathogenic bacteria. Besides these pathogens, a wide variety of commensal species is present in the lower airways but their role in inflammation is unclear. Here, we show that the lung microbiota contains several species able to inhibit activation of the pro-inflammatory NF-κB pathway and production of interleukin 8 (IL-8), triggered by lipopolysaccharide (LPS) or H2O2, in a physiologically relevant three-dimensional (3D) lung epithelial cell model. We demonstrate that the minimal dose needed for anti-inflammatory activity differs between species (with the lowest dose needed for Rothia mucilaginosa), and depends on the type of pro-inflammatory stimulus and read out. Furthermore, we evaluated synergistic activity between pairs of anti-inflammatory bacteria on the inhibition of the NF-κB pathway and IL-8 secretion. Synergistic anti-inflammatory activity was observed for 4/10 tested consortia. These findings indicate that various microbiota members can influence lung inflammation either alone or as a consortium. This information can contribute to a better understanding of the lung microbiota in chronic lung disease development and process, and could open up new avenues for treatment.
Introduction
Chronic lung inflammation is the major pathophysiological trait in airway diseases such as cystic fibrosis (CF) and chronic obstructive pulmonary disease (COPD). In people living with chronic lung disease, exposure to pro-inflammatory stimuli (such as pathogenic microorganisms, pollutants, cigarette smoke, and allergens) triggers the release of cytokines by the lung mucosa, which in turn results in an excessive immune response dominated by neutrophils (1–4). Due to the persistent inflammation and impeded mucociliary clearance, an imbalance is generated between microbial migration from the upper respiratory tract and microbial elimination through host defense mechanisms, leading to a net higher bacterial load in the lungs of patients with chronic lung disease compared to healthy individuals (5–7). The diseased lower airways are typically colonized by pathogenic bacteria such as Pseudomonas aeruginosa, Staphylococcus aureus, Haemophilus influenzae, Moraxella catarrhalis and Achromobacter xylosoxidans and their role in airway inflammation is widely recognized (8–10). Besides these common pathogens, various other lung microbiota members are present in the airways of patients with chronic lung disease (8, 11). In recent years, an association was reported between the diversity and composition of the lung microbiota and disease exacerbations (5, 12–14), inflammation (15), infection susceptibility (16), and mortality (17). Nevertheless, little is known about the role of specific microbiota members in the disease process (18). In this regard, we recently reported that a non-pathogenic commensal bacterium present in the lung microbiota, i.e. Rothia mucilaginosa, inhibits inflammation by interfering with NF-κB pathway activation in in vitro and in vivo models of lung inflammation (19). In addition, recent reports described that other members of the lung microbiota were able to inhibit inflammation caused by P. aeruginosa (20, 21).
Given the central role of the NF-κB pathway in mediating the pro-inflammatory response to a broad spectrum of inflammatory stimuli (including microorganisms, pollutants, and reactive oxygen species (ROS) (22, 23)), we aimed to understand whether the lung microbiota contains other commensal bacteria that inhibit NF-κB pathway activation, and whether species with anti-inflammatory properties may act synergistically to inhibit inflammation. To this end, we isolated a collection of commensal bacterial species from the sputum of people with cystic fibrosis (pwCF) to identify which species are able to reduce inflammation triggered by pro-inflammatory stimuli. Next, consortia of the most potent anti-inflammatory bacteria were evaluated for synergistic anti-inflammatory activity. In the present study, pro-inflammatory stimuli were selected to evaluate a host-directed anti-inflammatory effect. In that way, potential growth inhibitory effects of commensal species could be excluded, which has been described previously in co-culture studies with P. aeruginosa (24, 25). Knowledge on how different members of the lung microbiota simultaneously interact with the host to influence inflammation can contribute to a better understanding of the function of the lung microbiota in chronic lung disease, not only in CF but also in bronchiectasis and COPD.
Materials and methods
Isolation of commensal bacteria from sputum, and bacterial culture conditions
Sputum samples were collected from 5 pwCF attending the CF Reference Center of Ghent University Hospital for routine follow up, including sputum cultures. The local ethics committee of the Ghent University Hospital approved the study (registration number B670201836204). Inclusion criteria were that subjects had to be 12 years or older, clinically stable, and chronically colonized with P. aeruginosa (26). Before collection, saliva was removed from sputum samples by washing with sterile physiological saline. Next, Sputasol (Oxoid, Basingstoke, UK) was added to decrease viscosity and the samples were shaken for 30-60 min at 250 rpm, 37°C for liquification.
Isolation of bacterial species was done by plating samples on solid media in different oxygen conditions (27). The solid media used in this study were: Colombia agar base (Neogen, Lansing, MI, US) supplemented with 5% sheep blood (Biotrading, Mijdrecht, The Netherlands), Fastidious Anaerobe Agar (Neogen), tryptone soy agar (TSA) (Neogen) supplemented with 1.5% yeast extract (Neogen), 10 µg/mL colistin sulfate (TCI), 0.5 mg/mL L-cysteine (Sigma-Aldrich, St. Louis, MO, US), 1 ng/mL vitamin K1 (Sigma-Aldrich) and 10 ng/mL hemin (Sigma-Aldrich), and kanamycin vancomycin laked blood agar (KVLB), consisting of tryptone soy agar (TSA, Neogen) with 0.1 μg/ml, kanamycin (TCI, Tokyo, Japan), 7.5 μg/ml, vancomycin (Sigma-Aldrich), 10 μg/ml, vitamin K1 (Sigma-Aldrich), 0.05 ng/ml, hemin (Sigma-Aldrich) and 5% laked horse blood (Biotrading). Three different oxygen conditions were used for 3-5 days incubation, i.e. aerobic (37°C), microaerobic (3% O2, 5% CO2 and 92% N2, 37°C) using a hypoxia chamber (Bactrox; Sheldon manufacturing Inc., Cornelius, Oregon, US) and anaerobic (5% CO2, 5% H2, 90% N2, 37°C) using an anaerobic chamber (Bactronez; Sheldon manufacturing Inc.).
Rapid identification of bacterial isolates was done using matrix assisted laser desorption/ionization time-of-flight mass spectrometry (MALDI-TOF MS) (Bruker Microflex, Bruker Daltonics, Bremen, Germany). For isolates that could not be identified at the species level through MALDI-TOF MS, Sanger sequencing of the 16S rRNA gene was performed. Following extraction of the genomic DNA using the glass-bead method (28), amplification of the variable regions of the 16S rRNA gene was done using 27F and 1522R primers. The PCR reaction contained 0.2 µM primer mix, 25 ng template DNA, 0.2 mM deoxynucleoside triphosphates and 0.65 U Taq polymerase and the PCR protocol was carried out as follows; 95°C for 2 minutes, followed by 39 cycles of 94°C for 30 seconds, 57°C for 30 seconds and 72°C for 90 seconds, with a final temperature of 72°C for 5 min. The PCR product was verified by electrophoresis (1% agarose gel), purified using the NucleoSpin Gel and PCR Clean-up kit (Macherey-Nagel, Germany) and sequenced at GATC Biotech (Eurofins Genomics, Germany). Afterwards, sequences were analysed using the Chromas software program (Technelysium Pty Ltd, Australia). Query rRNA sequences were compared to reference sequences deposited in the NCBI 16S rRNA sequences database and in the RDP (Ribosomal Database Project) database. The BLASTn tool of the NCBI database and the Sequence Match tool (SeqMatch) of the RDP database were used for this purpose. The reference sequence with the highest similarity was determined for each query sequence, and the final identity of the isolates was established based on the consistency of the results obtained with the two databases.
All species that were evaluated for anti-inflammatory activity were cultured for 16 to 24 h at 37°C at 250 rpm in suitable broth media and oxygen levels until stationary phase was reached (Table 1).
Three-dimensional lung epithelial cell culture model
The alveolar epithelial cell line A549 (ATCC CCL185) was cultured as an organotypic three-dimensional (3D) cell culture model using the rotating wall vessel (RWV) bioreactor technology, which mimics in vivo phenotypic and functional properties of the parental tissue (29–31). This previously established 3D lung epithelial model was applied for all experiments except for the evaluation of anti-inflammatory properties of lung microbiota members based on inhibition of NF-κB pathway activation (as described below) for which a 3D model of NF-κB–luciferase-transfected A549 cells (BPS Bioscience, San Diego, CA, US) was used (19). Briefly, 3D lung epithelial cultures were generated by growing monolayers in T75 flasks containing GTSF-2 medium (HyClone, Logan, UT, US) supplemented with 1.5 g/L sodium bicarbonate (Sigma-Aldrich), 10% fetal bovine serum (FBS) (Life Technologies, Carlsbad, CA, US), 2.5 mg/L insulin transferrin sodium selenite (Lonza, Basel, Switzerland) and 1% penicillin-streptomycin (Sigma-Aldrich) at 37°C, 5% CO2. When confluency was reached, cells were trypsinized with 0.25% trypsin-EDTA solution (Life Technologies), counted in a Hemocytometer after viability staining using 0.4% Trypan Blue solution (Sigma-Aldrich), and 2 x 106 viable cells were transferred to a RWV together with 0.25 g collagen I-coated microcarrier beads (Cytodex-3 microcarrier beads, Sigma-Aldrich) in the above-described supplemented GTSF-2 medium. After 11-14 days, cell medium was changed to GTSF-2 medium without FBS and antibiotics, and a small volume was trypsinized and counted as described above to determine aggregate density. Finally, 3D cell aggregates were transferred to a 96 well plate at a density of 2.5 x 105 cells per well for host-microbe interaction experiments.
In vitro host-microbe interaction studies
Bacterial cultures were centrifuged (5000 rpm, 8 minutes) and resuspended in GTSF-2 without FBS before bringing them in contact with host cells. 3D cells were exposed to lung microbiota members at various targeted multiplicity of infection (MOI) ranging from MOI 50:1 to MOI 0.003:1 with or without pro-inflammatory stimulus (100 µg/mL lipopolysaccharide (LPS) (Sigma-Aldrich) or 1 mM H2O2 (Sigma-Aldrich)). For example, 1.25 x 107 bacterial CFU was added to 2.5 x 105 lung epithelial cells per well to obtain an MOI of 50:1, and 7.5 x 102 bacterial CFU were added for an MOI of 0.003:1. Plates were incubated for 4 h at 37°C under microaerobic conditions (3% O2, 5% CO2 and 92% N2) in a hypoxia chamber (Bactrox; Sheldon manufacturing Inc.). After incubation, NF-κB pathway activation, cytokine measurement, cytotoxicity and bacterial association to epithelial cells were assessed as described below.
NF-κB luciferase assay
Following incubation of 3D NF-κB–luciferase-transfected A549 cells with bacteria and/or pro-inflammatory stimuli (LPS, H2O2), the One-Step Luciferase Assay System (BPS Bioscience) was used to determine the activation of the NF-κB-pathway, according to the manufacturer’s instructions. Luminescence was measured with an EnVision microplate reader (Perkin Elmer, Waltham, MA, US). Afterwards, values were expressed as a percentage of the signal obtained when cells were incubated with the pro-inflammatory stimulus alone (LPS or H2O2).
Cytokine measurement
Cell supernatant was collected after incubation of 3D A549 cells with lung commensals and/or pro-inflammatory stimuli (LPS, H2O2) to quantify the concentration of secreted interleukin (IL)-8. The cytokine concentration was determined using the Human IL-8 ELISA MAX Standard assay (BioLegend, San Diego, CA, US) according to the manufacturer’s protocol. Afterwards, values were expressed as a percentage of the signal obtained when cells were incubated with the pro-inflammatory stimulus alone (LPS or H2O2).
Cytotoxicity assay
Cell viability was assessed using the lactate dehydrogenase (LDH) detection kit (Sigma-Aldrich). To avoid potential interference of bacteria with LDH activity, the intracellular LDH assay was applied (32). Briefly, 3D A549 cells were washed twice with Hank’s Balanced Salt Solution (HBSS, Life Technologies) and lysed with 1% Triton X-100 (Sigma-Aldrich) by vigorous pipetting. LDH activity was further determined as described in the manufacturer’s protocol. Afterwards, cell viability was expressed as a percentage of the uninfected control. When viability was lower than 80% and a statistically significant difference was obtained compared to the uninfected control (p< 0.05), the commensal bacterium was considered as cytotoxic (33, 34).
Host association assay
Bacterial association with the host cells was evaluated in the presence and absence of the pro-inflammatory stimuli (LPS, H2O2) (31). After incubation, 3D A549 cells were transferred to a new plate to avoid inclusion of possible biofilm formation in the wells. Cells were washed twice with HBSS (Life Technologies) to remove bacteria that were not associated with host cells. Next, cells were treated with 1% Triton X-100 (Sigma-Aldrich) and pipetted vigorously to lyse host cells. The obtained lysate containing bacteria associated with the host cells and intracellular bacteria were quantified by plating on solid media as described in Table 1.
Synergism testing
The experimental set-up for synergism testing is presented in Figure 1. Synergistic effects on the inhibition of NF-κB pathway activation in 3D NF-κB–luciferase-transfected A549 cells were evaluated by testing 10 different consortia consisting of 2 species with anti-inflammatory properties (i.e. Actinomyces naeslundii, Gemella haemolysans, Prevotella nigrescens, Rothia mucilaginosa and Streptococcus sanguinis). To this end, a modified checkerboard assay was developed, based on Stein et al., 2015 (35). Species were mixed at varying MOI (range of MOIs: 12.5 to 0.003) by applying 1:4 serial dilutions four times in a two dimensional array. Dilutions started at the mMOINF-κB of each species, which is defined as the lowest MOI with anti-inflammatory activity (i.e.< 50% NF-κB pathway activation compared to LPS-stimulated control, p< 0.05). In that way, 16 different MOI ratios were evaluated for each tested consortium. Lung epithelial cells were exposed for 4 h to 100 µg/mL LPS with or without each of the 10 consortia at varying MOI ratios, and NF-κB-pathway activation was evaluated as mentioned above. For all the MOI ratios, the fractional inhibitory concentration index (FICI) was calculated (35). This method is often used for antimicrobial drugs to evaluate if combinations of antimicrobial drugs can have a stronger effect than the sum of their effect when used alone (36). In the present study, the same principle was applied for each 2-species consortium. Hence, the following formula was used to determine the FICI:
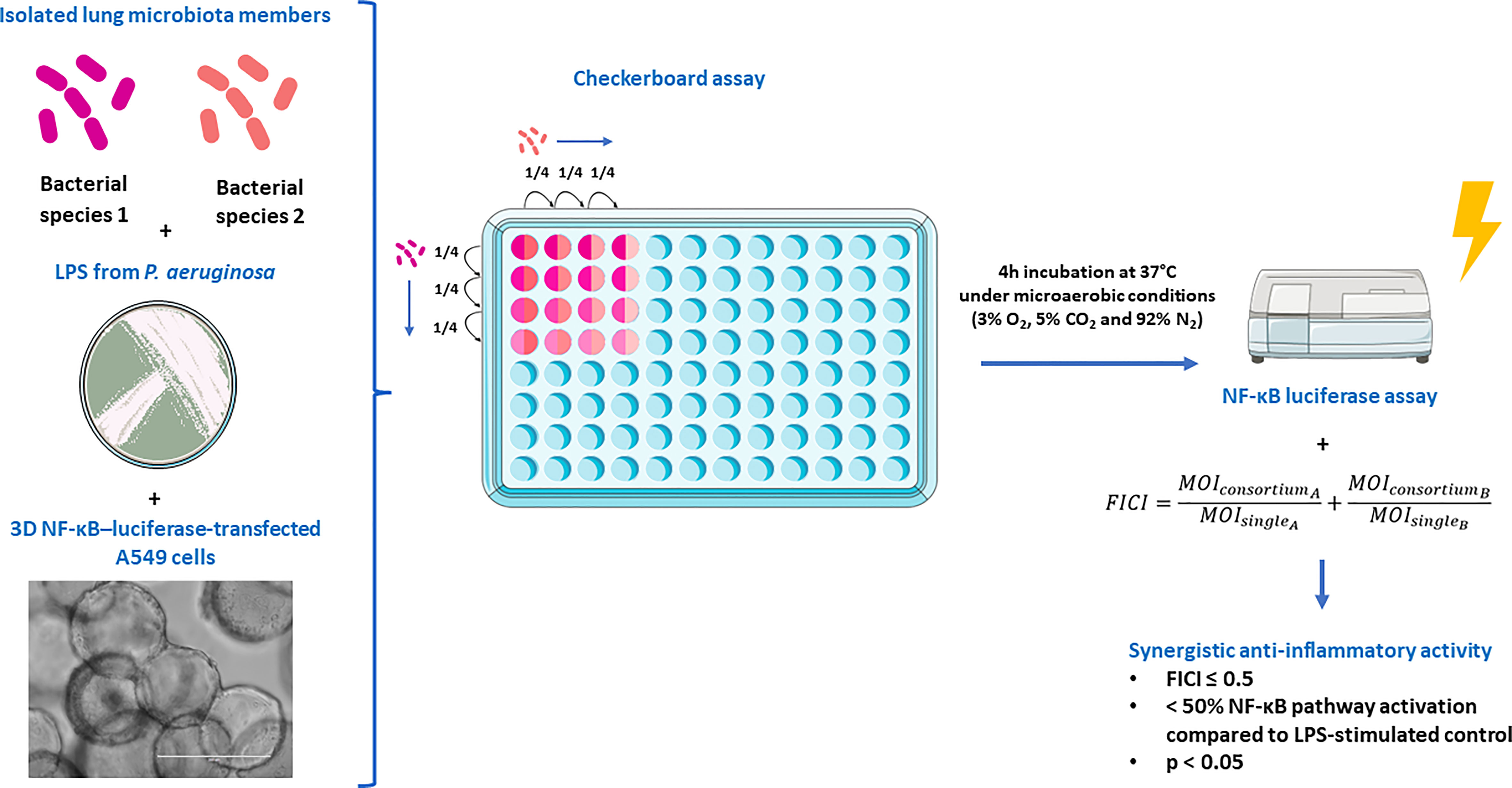
Figure 1 Experimental set-up to evaluate synergistic effects on the inhibition of NF-κB pathway activation in 3D NF-κB–luciferase-transfected A549 cells. The figure was partly created using the Servier Medical Art, licensed under a Creative Commons Attribution 3.0 unported license.
The MOIsingle represents the mMOINF-κB for a single species and the MOIconsortium is the MOI of that same species when used in the consortium. FICI values were interpreted as described by Odds 2003 (36) with modifications, i.e. FICI ≤ 0.5 indicates synergism when NF-kB pathway inactivation of > 50% is observed compared to the LPS-stimulated control (p< 0.05) (as described above); 0.5 > FICI< 4 indicates additivity or no interaction, and FICI ≥ 4 indicates antagonism. Therefore, a 2-species consortium was defined as synergistic when at least one MOI ratio fulfilled the criteria for synergism (i.e. FICI ≤ 0.5,< 50% NF-κB pathway activation compared to LPS-stimulated control, p< 0.05).
Data analysis
All experiments were done at least in biological triplicate. Statistical analysis was performed using SPSS Statistics 27. Normal distribution was assessed with the Shapiro-Wilk test. When data was normally distributed, a one-sample t-test (NF-κB luciferase assay, cytokine measurement and LDH assay) or an independent sample t-test (bacterial host association assay) was performed. Non-normally distributed data was analyzed by a Wilcoxon signed-rank test (NF-κB luciferase assay, cytokine measurement and LDH assay) or a Mann-Whitney U test (bacterial host association assay). When multiple conditions were compared to one control, Benjamini-Hochberg correction was applied to control for multiple testing (37). Statistical significance was considered at p< 0.05.
Results
Isolation of lung microbiota members from sputum samples of people with CF
From 5 sputum samples of adult pwCF, 33 bacterial species from 13 different genera were isolated and identified by MALDI-TOF MS or Sanger sequencing of the 16S rRNA gene (Figure 2). These included 3 pathogenic genera (i.e. Achromobacter, Pseudomonas, Staphylococcus) which were excluded for further analysis. In addition, Megasphaera sp. were not included for subsequent experiments since the growth media wherein all other microbiota members were cultured (Table 1), was not suitable for this bacterial species. This resulted in a collection of 9 non-pathogenic genera consisting of 29 species in total, from which we selected at least one species per genus to evaluate anti-inflammatory activity (15 species in total) (Table 1).
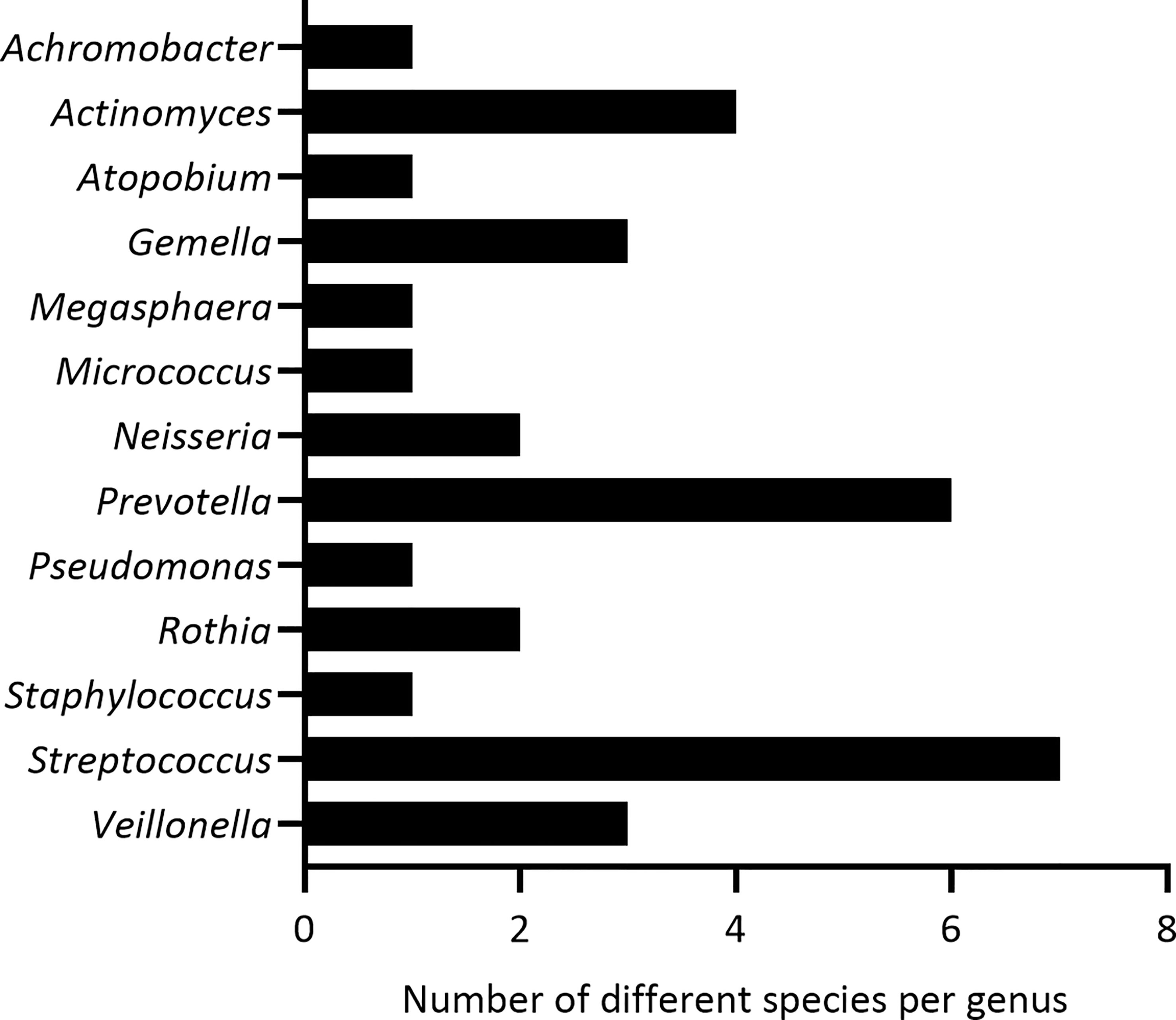
Figure 2 Identified genera and the number of bacterial species per genus, isolated from 5 sputum samples of pwCF.
The lung microbiota contains anti-inflammatory bacterial species
To evaluate whether lung microbiota members have anti-inflammatory properties, 3D cells were exposed to each commensal species in the absence or presence of LPS. This evaluation was initially done at two different MOIs (i.e. 50 and 12.5). Bacterial species were considered as anti-inflammatory when they significantly reduced LPS-triggered NF-κB-pathway activation by at least 50% at MOI 50 and/or MOI 12.5, without activating the NF-κB-pathway by itself (i.e. no significant difference between the uninfected control and cells co-cultured with commensals alone at MOI 50 or MOI 12.5). In addition, commensal species were evaluated for cytotoxicity in the 3D lung epithelial cell model.
Among the 15 commensal species that were evaluated, 12/15 species reduced the activation of the NF-κB pathway at a MOI 50, whereas 8/15 species reduced the NF-κB pathway activation at both MOIs (Figure 3A). For 3/15 species (i.e. Actinomyces oris, Neisseria subflava and Streptococcus salivarius), cytotoxicity was observed at a MOI of 50 (i.e. A. oris) or at both tested MOIs (i.e. N. subflava, S. salivarius) (Figure 3B). These commensals also exhibited inactivation of the NF-κB pathway, most likely as a consequence of cytotoxicity, and were excluded from this study. Hence, 9/15 species were defined as non-cytotoxic and anti-inflammatory (at MOI 12.5 and/or 50) and were evaluated further.
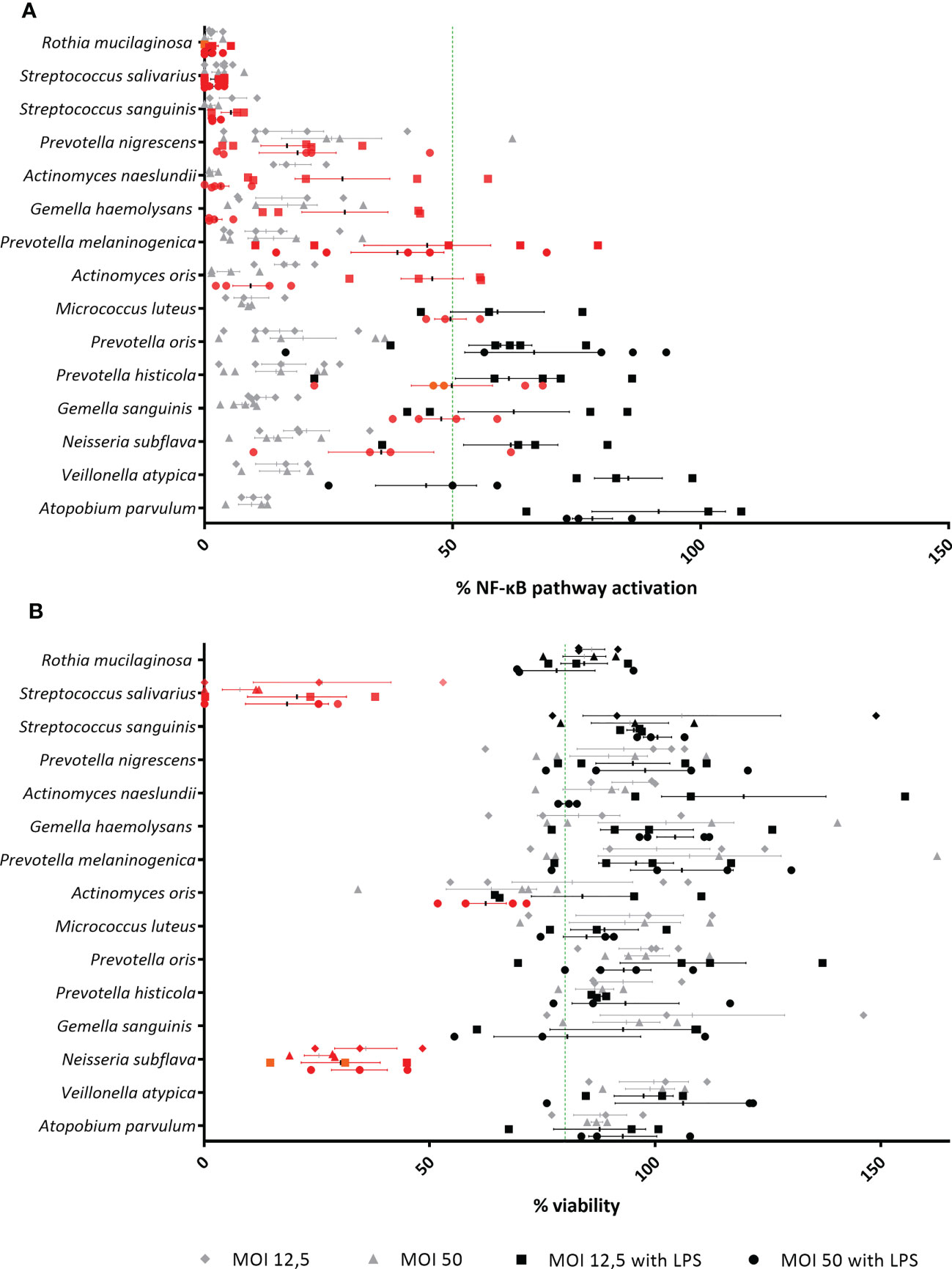
Figure 3 Evaluation of lung microbiota members for identification of anti-inflammatory and non-cytotoxic species in (3D) A549 (luciferase NF-κB-reporter) cells. (A) Isolated lung commensals at MOI 50 and MOI 12.5, with or without LPS, were screened for anti-inflammatory activity by a NF-κB luciferase assay. On the vertical axis, screened lung commensals are depicted, on the horizontal axis, the % NF-κB pathway activation compared to LPS-stimulated cells is shown. Anti-inflammatory effects were defined as;< 50% NF-κB pathway activation compared to LPS-stimulated cells as visualized by a green line, p< 0.05. Anti-inflammatory commensals are marked in red on the graph. N ≥ 3. (B) Isolated lung commensals at MOI 50 and MOI 12.5, with or without LPS, were screened for cytotoxicity by a LDH assay. On the vertical axis, screened lung commensals are depicted, on the horizontal axis, the % viability compared to cells cultured alone is shown. Cytotoxic effects were defined as;< 80% viability compared to cells cultured alone as visualized by a green line, p< 0.05. Cytotoxic commensals are marked in red on the graph. N ≥ 3.
Finally, bacterial association with 3D cells was evaluated. Here, no significant differences were found when commensals were added to the 3D cells with or without LPS (Supplementary Figure S1).
The minimal effective MOI differs between anti-inflammatory species
Next, anti-inflammatory and non-cytotoxic lung microbiota members were further investigated to determine their minimal effective MOI (mMOINF-κB), i.e. the lowest MOI that is able to significantly decrease the LPS-triggered NF-κB-pathway activation by at least 50%. The dose that is necessary for an anti-inflammatory effect was found to differ between genera and even between species from the same genus (Figure 4). For example, a MOI of at least 12.5 was needed to obtain an anti-inflammatory effect for P. melaninogenica, whereas P. nigrescens was already anti-inflammatory at a MOI of 0.78. From all evaluated species and under the tested conditions, Rothia mucilaginosa was the most potent anti-inflammatory bacterium with a mMOINF-κB of 0.2.
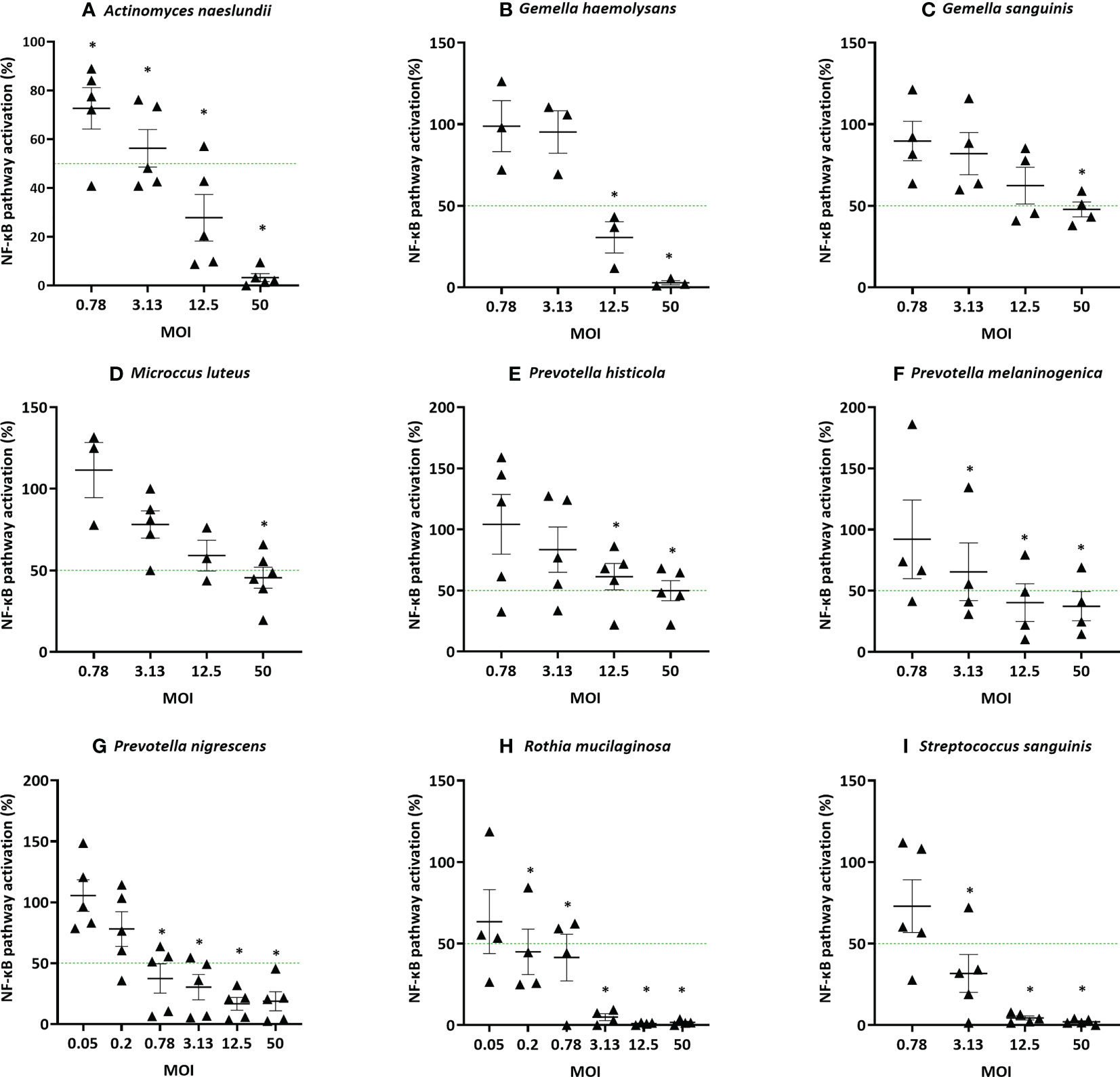
Figure 4 Determination of the minimal effective MOI (mMOINF-κB) of commensal bacteria based on NF-κB pathway activation in (3D) A549 luciferase NF-κB-reporter cells. Selected lung commensals at different MOI were cultured with LPS and the mMOINF-κB was determined by a NF-κB luciferase assay, with (A) A. naeslundii, (B) G. haemolysans, (C) G. sanguinis, (D) M. luteus, (E) P. histicola, (F) P. melaninogenica, (G) P. nigrescens, (H) R. mucilaginosa, (I) S. sanguinis. On the vertical axis % NF-κB pathway activation compared to LPS-stimulated cells is shown, on the horizontal axis the tested MOI is depicted. The mMOINF-κB was defined as the lowest MOI with anti-inflammatory activity (i.e.< 50% NF-κB pathway activation compared to LPS-stimulated cells as visualized by a green line, p< 0,05). *: p< 0.05, n ≥ 3.
The 5 most potent microbiota members (based on the lowest mMOINF-κB, Figure 4), each belonging to different genera, were further validated using an alternative measure of anti-inflammatory activity, i.e. based on the inhibition of IL-8 production. This inflammatory cytokine is upregulated by NF-κB-pathway activation and induces inflammation by attracting neutrophils to the inflammatory site in patients with chronic lung disease (38, 39). These species were A. naeslundii (mMOINF-κB = 12.5), G. haemolysans (mMOINF-κB = 12.5), P. nigrescens (mMOINF-κB = 0.78), R. mucilaginosa (mMOINF-κB = 0.2), and S. sanguinis (mMOINF-κB = 3.13). Similarly to the NF-kB-based mMOI determination, the mMOIIL-8 was defined as the lowest dose that significantly reduced LPS-triggered inflammation (i.e. IL-8 production) by at least 50%. The mMOIIL-8 was 50 for A. naeslundii, 50 for G. haemolysans, 3.12 for P. nigrescens, 0.2 for R. mucilaginosa and 50 for S. sanguinis (Figures 5A–E). Hence, for all species, except R. mucilaginosa, a higher MOI was needed to reduce IL-8 production compared to inhibition of the NF-κB-pathway activation (Figure 5F).
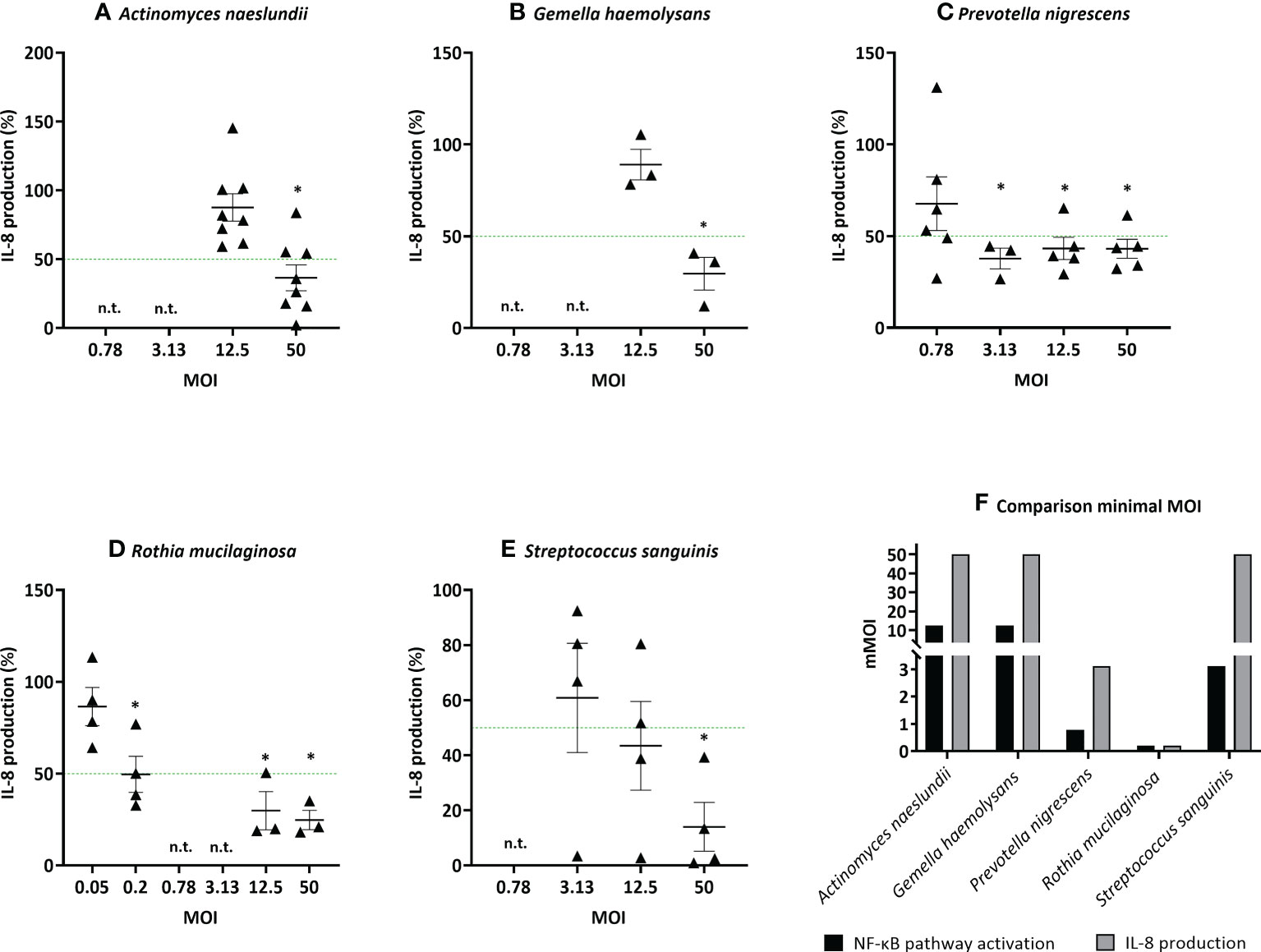
Figure 5 Determination of the minimal effective MOI (mMOIIL-8) of commensal bacteria in (3D) A549 cells based on IL-8 secretion. Selected lung commensals at different MOI were cultured with LPS and the mMOIIL-8 was determined using an ELISA for IL-8 quantification, with (A) A. naeslundii, (B) G. haemolysans, (C) P. nigrescens, (D) R. mucilaginosa, (E) S. sanguinis. On the vertical axis % IL-8 production compared to LPS-stimulated cells is shown, on the horizontal axis the tested MOI is depicted. The mMOIIL-8 was defined as the lowest MOI with anti-inflammatory activity (i.e.< 50% IL-8 production compared to LPS-stimulated cells as visualized by a green line, p< 0.05). *: p< 0.05, n ≥ 3, n.t.: not tested. (F) Comparison of the minimal MOI for inhibition of NF-κB pathway activation and IL-8 production. On the vertical axis the minimal MOI is shown, on the horizontal axis the selected microbiota members are depicted. Anti-inflammatory activity was defined as:< 50% NF-κB pathway activation/IL-8 secretion compared to LPS-stimulated cells, p< 0.05.
Anti-inflammatory species can reduce IL-8 production triggered by H2O2
Next, we evaluated whether the most potent anti-inflammatory bacteria (A. naeslundii, G. haemolysans, P. nigrescens, R. mucilaginosa, S. sanguinis) were able to inhibit inflammation in 3D A549 lung cells caused by another pro-inflammatory stimulus, i.e. H2O2, which is relevant for COPD (40–42). Hydrogen peroxide is a ROS, which is elevated in people living with COPD (pwCOPD) due to exogenous pollutants and inflammation leading to an oxidative imbalance (43, 44). In this study, hydrogen peroxide did not give a measurable signal when activation of the NF-κB pathway was used as read out (Supplementary Figure S2). Most likely, the NF-κB luciferase assay was not sensitive enough to detect H2O2-induced NF-κB pathway activation at the tested concentration or the chemical interfered with the read-out of the assay. Therefore, anti-inflammatory activity was evaluated by measuring IL-8 production after 3D lung cells were exposed to 1 mM H2O2 with or without single species at their mMOIIL-8.
All five anti-inflammatory bacteria significantly reduced IL-8 production triggered by H2O2 (Figure 6). Nevertheless, only A. naeslundii and S. sanguinis reduced inflammation by more than 50%. No significant cytotoxicity was observed for all five single species (Supplementary Figure S3A). However, the viability in presence of A. naeslundii or G. haemolysans was lower than 80% but not significantly different from the control. In addition, H2O2 had no influence on bacterial association with 3D lung epithelial cells (Supplementary Figure S3B).
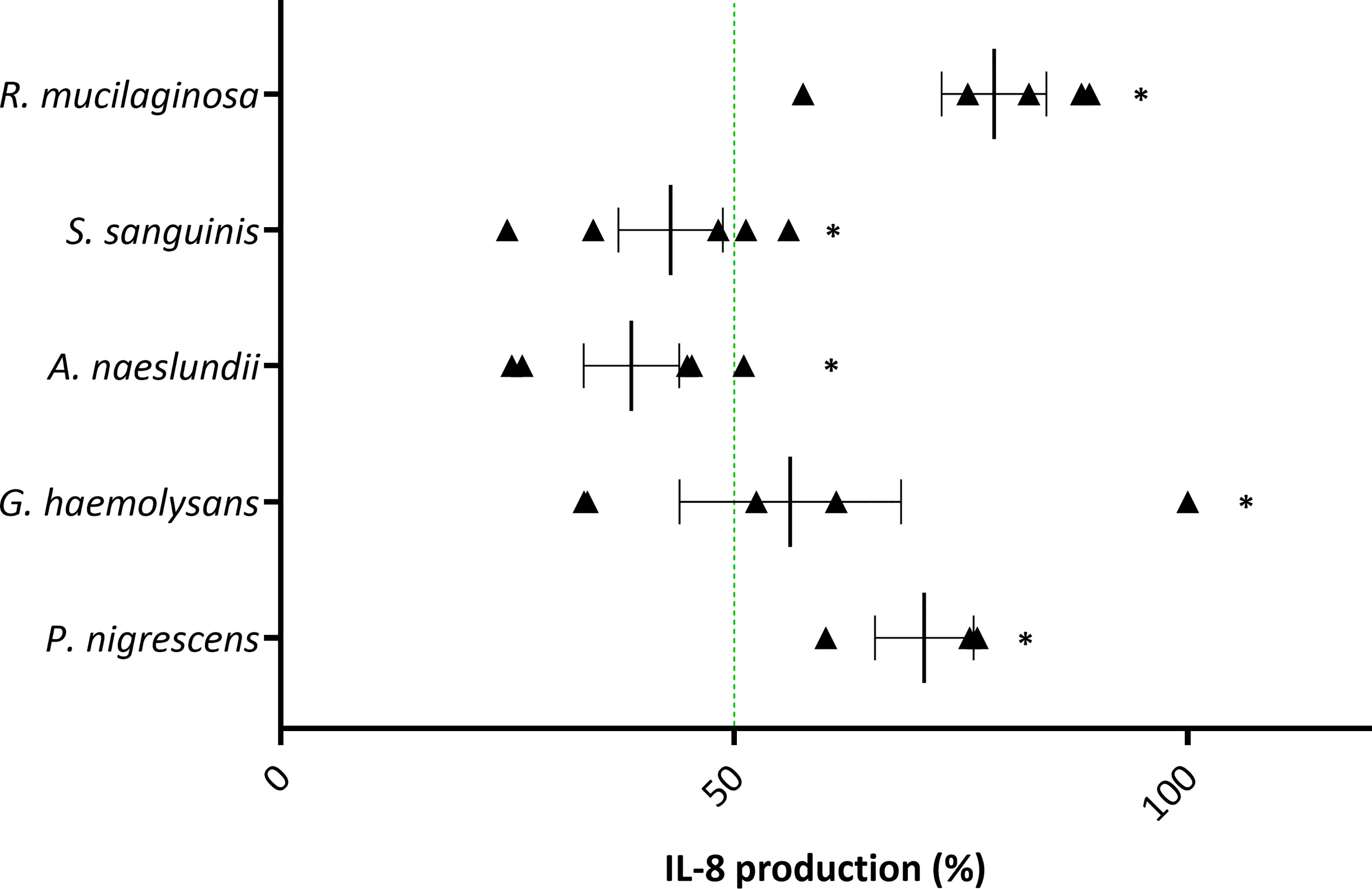
Figure 6 Anti-inflammatory activity of selected lung commensals with 1 mM H2O2 as pro-inflammatory stimulus in (3D) A549 cells based on IL-8 secretion. Selected lung commensals at their mMOIIL-8, in presence of H2O2, were evaluated for anti-inflammatory activity using an ELISA for IL-8 quantification. On the vertical axis the tested species are shown. On the horizontal axis the % IL-8 production compared to H2O2-stimulated cells is shown. Anti-inflammatory activity was defined as:< 50% IL-8 production compared to H2O2-stimulated cells as visualized by a green line, p< 0.05. *: p< 0.05, n ≥ 3.
Synergistic activity is observed between anti-inflammatory species
To evaluate synergistic activity between anti-inflammatory bacteria, the 5 most potent anti-inflammatory microbiota members (based on the lowest MOINF-κB, Figure 4) belonging to different genera were used. We hypothesized that when two bacterial species are added simultaneously as a consortium, a lower total dose is needed to obtain an anti-inflammatory effect as compared to the dose needed using single species. To experimentally test this hypothesis, a checkerboard assay was done for each consortium comprised of two anti-inflammatory species. Each checkerboard contained 16 different MOI ratios, and FICI values were calculated for all MOI ratios. These FICI values were graphically visualized with an isobologram (Figure 7). The isobologram method is commonly used for assessing drug synergism and is similar to the FICI formula (45). In the present study, the axes on each isobologram represent the tested MOI of species A and species B. Hence, data points in the isobolograms represent the tested MOI ratios (i.e. 16 in total), while the line represents the FICI cut-off value for synergism (i.e. FICI ≤ 0.5) (46). When FICI ≤ 0.5, dots are located below or on the FICI cut-off, while values are displayed above the FICI cut-off for FICI > 0.5. For each consortium, the effect of each MOI ratio was evaluated based on LPS-triggered NF-κB pathway activation. When the MOI ratio was situated below or on the FICI cut-off and at least 50% inactivation of the NF-κB pathway was observed, a synergistic anti-inflammatory activity was exerted by that specific consortium at that particular MOI ratio, as compared to single bacterial species.
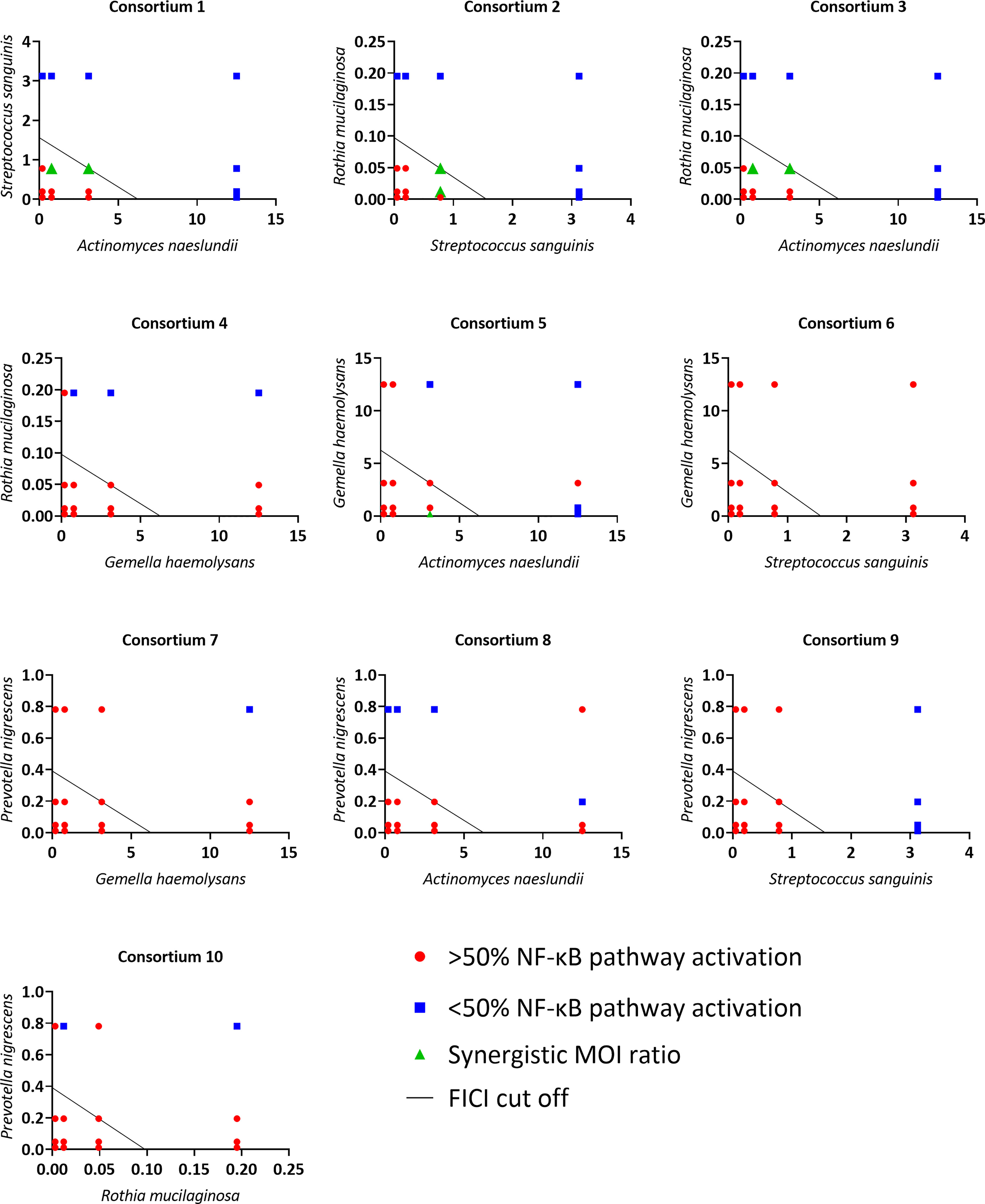
Figure 7 Isoboles of all tested consortia to visualize synergistic anti-inflammatory activity based on NF-κB pathway activation. On the vertical axis the tested MOI of one commensal species is shown, on the horizontal axis the tested MOI of the other commensal species is shown. MOI ratios were defined as synergistic if:< 50% NF-κB pathway activation compared to LPS-stimulated cells, p< 0.05 and FICI ≤ 0.5. n ≥ 3.
Synergistic MOI ratios within the consortium were labeled in green (Figure 7). Four out of ten tested consortia were synergistic as at least one MOI ratio had synergistic anti-inflammatory activity. For these synergistic consortia, FICI values and percentage of NF-κB pathway activation of the synergistic MOI ratios are shown in Table 2. These consortia were: A. naeslundii with S. sanguinis (consortium 1), S. sanguinis with R. mucilaginosa (consortium 2), A. naeslundii with R. mucilaginosa (consortium 3) and A. naeslundii with G. haemolysans (consortium 5).
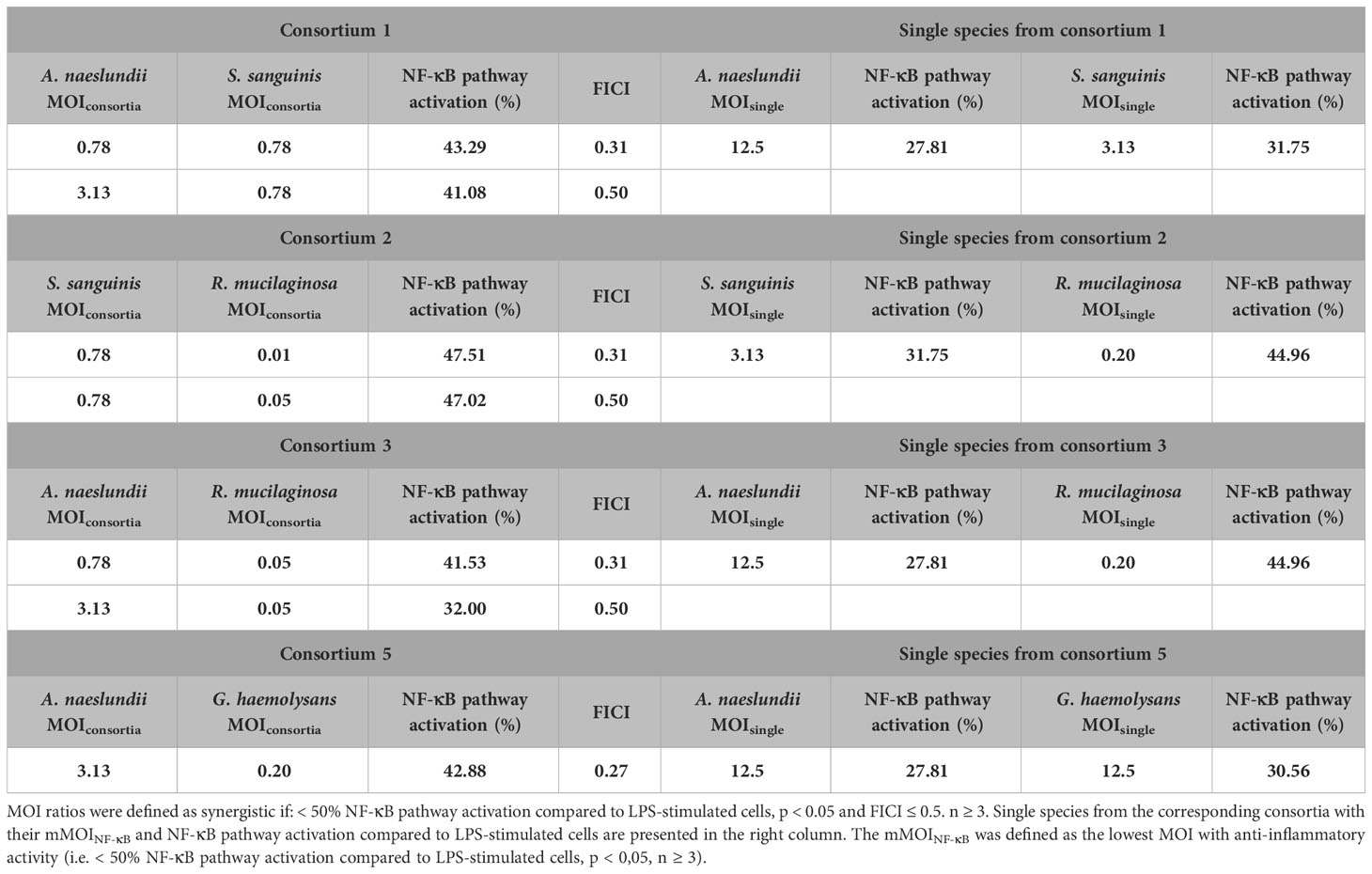
Table 2 Synergistic consortia and single species with corresponding MOI ratios, NF-κB pathway activation compared to LPS-stimulated cells and FICI values.
Since we made dilutions starting from the mMOINF-κB of each microbiota member, a maximum of 9/16 MOI ratios could in theory be synergistic according to the FICI formula. However, only 2/9 MOI ratios were defined as synergistic for consortium 1, 2 and 3, and only one MOI ratio for consortium 5. The cut-off (i.e. 50% reduction in NF-κB pathway activation) that we defined as criterium for anti-inflammatory activity could be an explanation for this observation. Indeed, many other synergistic MOI ratios (i.e. FICI ≤ 0,5) from consortium 1, 2, 3 and 5 also had a significant reduction in NF-κB pathway activation but this was less than 50% (Supplementary Table 1).
To validate if synergistic consortia, which were selected based on NF-κB pathway inactivation as a read out, could also reduce LPS-triggered IL-8 production in 3D A549 lung epithelial cells, three consortia were selected, i.e. A. naeslundii with R. mucilaginosa, A. naeslundii with G. haemolysans and A. naeslundii with S. sanguinis. As mentioned above, we observed that the mMOI differs when NF-κB pathway activation (mMOINF-κB) or IL-8 production (mMOIIL-8) are measured as an outcome (Figure 5F). Hence, we could not use synergistic MOI ratios as determined by the inhibition of the NF-κB pathway (Table 2) for validation purposes. Therefore, based on the definition by Odds et al. (2003) (36), we tested whether the mMOIIL-8/4 of both species is anti-inflammatory in a consortium but not in single species. In this case, a FICI ≤ 0,5 and hence synergism is achieved. As expected, all single species showed a significant reduction of LPS-triggered IL-8 production at their mMOIIL-8, which was not observed at an mMOIIL-8/4 (Figure 8). When the mMOIIL-8/4 of two species was combined in a consortium, more than 50% reduction in IL-8 production was observed compared to LPS-stimulated cells, for 2/3 consortia (i.e. consortium 1 and 3), while the consortium consisting of A. naeslundii and G. haemolysans (consortium 5) significantly reduced IL-8 production by 44% (p< 0.05). In addition, cytotoxicity of these three consortia was tested, and no significant LDH release was observed (Supplementary Figure S4).
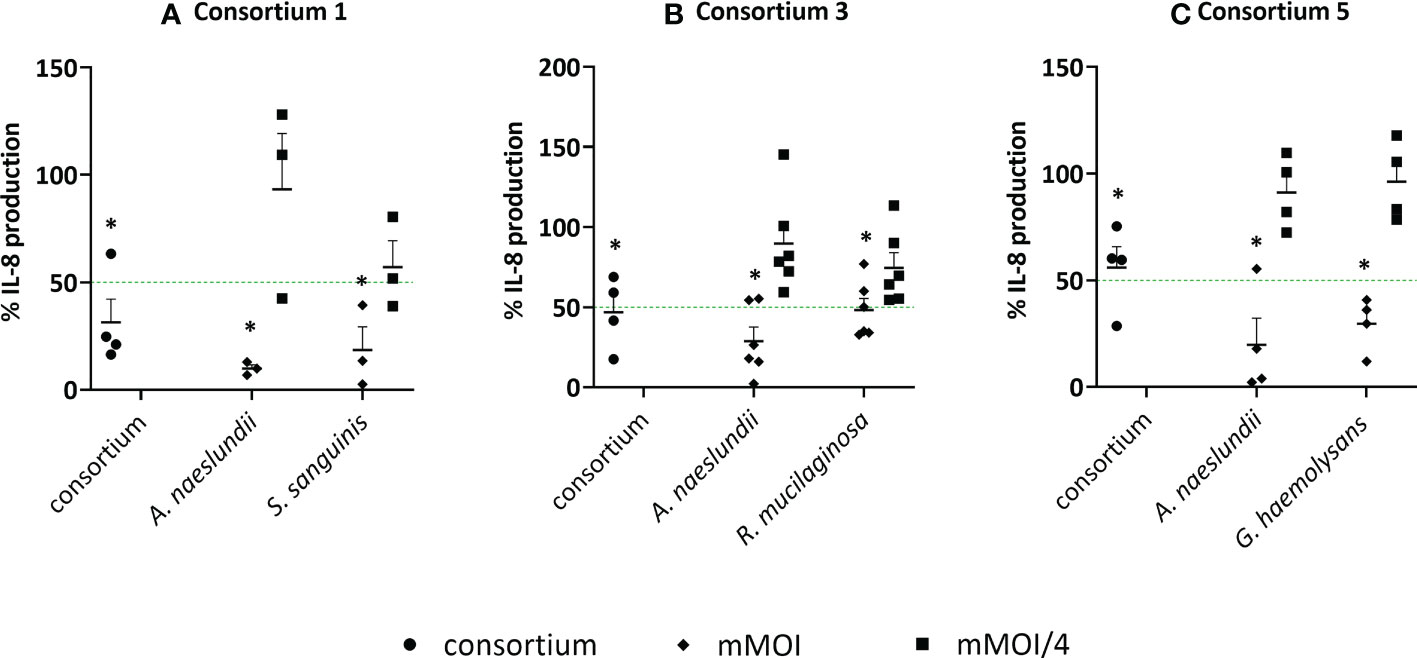
Figure 8 Anti-inflammatory activity of synergistic consortia and single species in (3D) A549 cells based on IL-8 secretion. Single species at their mMOIIL-8 and mMOIIL-8/4 and synergistic consortia at defined MOI ratios, in the presence of LPS, were evaluated for anti-inflammatory activity by using an ELISA for IL-8 quantification with (A) consortium 1; A. naeslundii with S. sanguinis, (B) consortium 3; A. naeslundii with R. mucilaginosa, (C) consortium 5; A. naeslundii with G. haemolysans. On the vertical axis, the % IL-8 production compared to LPS-stimulated cells is shown, on the horizontal axis the consortium and single species at selected MOI (i.e. mMOIIL-8 and mMOIIL-8/4) are presented. Anti-inflammatory (synergistic) activity was defined as:< 50% IL-8 production compared to LPS-stimulated cells as visualized by a green line, p< 0.05, and (FICI ≤ 0.5). *: p<0.05, n ≥ 3.
Discussion
In this study, we demonstrate that several non-pathogenic members of the lung microbiota have anti-inflammatory properties, based on their ability to inhibit NF-κB pathway activation and IL-8 secretion, induced by the pro-inflammatory triggers LPS or H2O2 in a physiologically relevant 3D lung epithelial cell model. In addition, synergistic anti-inflammatory activity was observed between species of the lung microbiota.
The bacterial species that were evaluated for anti-inflammatory activity in this study were isolated from sputum samples of pwCF. While bronchoalveolar lavage (BAL) is the golden standard to sample the lower airways, less invasive methods (such as spontaneously expectorated sputum) are often preferred (47). Nevertheless, the used sampling method may imply the inclusion of some commensals of the oral cavity, which was minimized by rinsing sputum samples with physiological saline prior to bacterial isolation to remove saliva. Interestingly, the species that are part of the CF lung microbiota are highly comparable with those found in other chronic lung diseases, with some differences such as a higher Streptococcus abundance in pwCOPD (48). In addition, interpatient variability in the relative abundance of many genera of the lung microbiota has been reported for patients with different chronic lung diseases (8, 20, 49–51). In this study, we isolated 29 species from 9 non-pathogenic genera (i.e. Actinomyces, Atopobium, Gemella, Micrococcus, Neisseria, Prevotella, Rothia, Streptococcus and Veillonella). Similarly, another study on sputum samples of pwCF, isolated 21 commensal species from 7 genera, and the genus Streptococcus was widely represented with 10 different species present (20). In the present study, 7 different Streptococcus species were identified. The identified genera and selected species represent a significant part of the lung microbiota in chronic lung diseases.
We observed that none of the tested species induced inflammation by itself, while 9/15 non-cytotoxic lung commensals could inhibit NF-κB pathway activation after stimulation with pro-inflammatory stimuli relevant for chronic lung diseases (LPS or H2O2). Recent studies have described beneficial properties of some microbiota members that were identified as anti-inflammatory in the present study. Specifically, anti-inflammatory activity was previously described for Prevotella, Rothia and Streptococcus species (19–21, 52). Recently, P. nigrescens and P. histicola were reported to diminish NF-κB (p65) signaling and levels of cytokines, such as IL-6 and IL-8, induced by P. aeruginosa in CF airway epithelial cells (CFBE41o- cells) by reducing P. aeruginosa growth (53). For the genus Streptococcus, various species, such as S. mitis, S. oralis and S. cristatus, reduced inflammation triggered by P. aeruginosa in BEAS-2B cells (20). Another study found that the majority of oral streptococci was able to reduce CXCL8 secretion through inhibition of NF-κB pathway activation in 16HBE14o- epithelial cells, stimulated with flagellin (54). As mentioned above, Rothia species, incubated under aerobic or microaerobic conditions (3% O2, 5% CO2 and 92% N2, 37°C), were found to interfere with NF-κB pathway activation in vitro, and showed anti-inflammatory activity in vivo as well (19). In the present study, R. mucilaginosa was found to be the most potent anti-inflammatory species since a very low dose (i.e. MOI 0.2) reduced NF-κB pathway activation and IL-8 production when 3D lung cells were triggered with both LPS or H2O2. Moreover, many studies have linked the relative abundance of Rothia spp. in the airways with lower levels of inflammatory markers (i.e. IL-8, IL-6 and IL-1β) in chronic lung diseases (55, 56). Even the relative abundance of Rothia in the gut was shown to have an influence on asthma predisposition (57, 58). For Gemella spp., a study investigating the microbiome profiles of the different inflammatory phenotypes in severe asthma patients, found that the abundance of Gemella spp. was negatively associated with the percentage of sputum neutrophils in patients with neutrophilic asthma (59). While for Actinomyces spp. no studies were found on anti-inflammatory properties, other beneficial characteristics were reported, including inhibition of biofilm formation, virulence and proliferation of Candida albicans (60).
Interestingly, the minimal dose where anti-inflammatory activity was observed differed between genera but also between species of the same genus. Possible reasons for this divergence might be a difference in the type and amount of effector molecules produced by the different bacterial species. Further research, including metabolomic analysis, is now needed to narrow this knowledge gap as anti-inflammatory mediators of the lung microbiota have not been reported yet. In addition, when comparing the mMOI necessary to reduce IL-8 secretion or NF-kB pathway activation in 3D lung epithelial cells, a higher mMOIIL-8 was typically needed, with the exception of R. mucilaginosa where an equal mMOIIL-8 and mMOINF-κB was obtained. A possible explanation for this finding is that a higher net bacterial load may be needed to reduce IL-8 secretion since cytokine production is located downstream in the LPS-triggered inflammatory process compared to activation of the NF-κB pathway (38, 61). Additionally, IL-8 production triggered by LPS stimulation may result from activation of other inflammatory pathways besides the NF-κB pathway, such as mitogen-activated protein kinase (MAPK) (62–64), extracellular signal-regulated kinase (ERK) (65) and c-Jun N-terminal kinase (JNK) (66). The involvement of different inflammatory pathways could also explain the lower reduction of IL-8 secretion when H2O2 was used as pro-inflammatory stimulus compared to LPS. For example, ROS levels can activate the ROS–NLRP3–caspase-1 pathway in A549 cells, in addition to the NF-κB pathway (67).
Another finding in this study is that differences in cytotoxicity were observed between different genera of the lung microbiota but also within a single genus. Specifically, S. sanguinis did not exhibit cytotoxicity, while S. salivarius was strongly cytotoxic. Controversially, S. salivarius is often described in literature as an anti-inflammatory commensal, and has been studied as a probiotic against otitis media in several studies (68, 69). For example, S. salivarius K12 was tested in vitro for its anti-inflammatory effects in 16HBE14o- cells and showed no cytotoxicity using the LDH assay (70). To our knowledge, the present study is the first describing cytotoxic effects of S. salivarius. However, cytotoxicity was reported for other oral streptococci, such as S. mitis and S. cristatus, which caused moderate LDH release when cultured with 16HBE14o- cells for 24h (54). Differences between previous studies and the present study can potentially be explained by the use of different S. salivarius strains since most probiotic studies investigated S. salivarius strain K12 (69). In addition, other host cell types, growth conditions and the type of LDH assay could have an influence (32, 54). In this study, microaerobic conditions (3% O2, 5% CO2 and 92% N2) were used during incubation, which resembles the diseased lung environment more closely since low oxygen levels have been reported in the viscous mucus (71). However, most studies use aerobic conditions (54, 70) for in vitro co-culture experiments. Moreover, previous research demonstrated that S. maltophilia and S. pneumoniae interfered with the outcome of the LDH assay by either protease activity or acidification of cell supernatant (32). For that reason, an alternative LDH assay was used in the present study, which avoids potential underestimation of LDH activity by bacterial interference (32).
Next, we demonstrated that lung commensals can synergistically interact with each other to influence host inflammation. The data in this study reveal that synergism can occur but that it is not common. Here, 4/10 studied consortia were found to have synergistic anti-inflammatory activity for inhibition of NF-κB pathway activation. In addition, inhibition of IL-8 production in LPS-stimulated 3D A549 lung epithelial cells was also demonstrated for the three evaluated synergistic consortia. It was noted that three out of four synergistic consortia contained Actinomyces. We hypothesize that the target of Actinomyces in the NF-κB pathway might be different than that of the other four commensals, which may result in a more pronounced inactivation of NF-κB signaling when combining Actinomyces with any one of the other tested species.
In conclusion, our data demonstrate that the lung microbiota contains various anti-inflammatory species and that the minimal dose to exert an effect in vitro may differ between species. Furthermore, synergism between anti-inflammatory species was found, which brings forward a possible role of interspecies interactions in the net inflammatory response of the host.
Data availability statement
The original contributions presented in the study are publicly available. This data can be found here: Genbank [SUB12991115 P1_AE4 OQ693609; SUB12991115 P4_MA3 OQ693610].
Ethics statement
The local ethics committee of the Ghent University Hospital approved the study (registration number B670201836204). Written informed consent to participate in this study was provided by the participants’ legal guardian/next of kin.
Author contributions
AC, EG and CR conceptualized the study and designed the experimental set-up. EB and YV provided the sputum samples of pwCF. LG and SB isolated and identified the lung microbiota members. EG performed the experiments and data analysis. TM and KB provided guidance in the experimental set-up. EG and AC wrote the manuscript, with input from all authors. All authors contributed to the article and approved the submitted version.
Funding
This work was funded by a research grant (G010119N) and an Odysseus grant (G.0.E53.14N) of the Research Foundation Flanders (FWO), and by the Industrial Research Fund of Ghent University (F2020/IOF-StarTT/103). EG is a recipient of an FWO-Strategic Basic Research (SB) fellowship (1S34622N).
Acknowledgments
We thank the Laboratory of Medical Microbiology at Ghent University Hospital for assistance with the identification of lung microbiota members using MALDI TOF analysis.
Conflict of interest
The authors declare that the research was conducted in the absence of any commercial or financial relationships that could be construed as a potential conflict of interest.
Publisher’s note
All claims expressed in this article are solely those of the authors and do not necessarily represent those of their affiliated organizations, or those of the publisher, the editors and the reviewers. Any product that may be evaluated in this article, or claim that may be made by its manufacturer, is not guaranteed or endorsed by the publisher.
Supplementary material
The Supplementary Material for this article can be found online at: https://www.frontiersin.org/articles/10.3389/fimmu.2023.1176044/full#supplementary-material
References
1. Zemanick ET, Hoffman LR. Cystic fibrosis: Microbiology and host response. Pediatr Clin North Am (2016) 63:617–36. doi: 10.1016/j.pcl.2016.04.003
2. Cantin AM, Hartl D, Konstan MW, Chmiel JF. Inflammation in cystic fibrosis lung disease: Pathogenesis and therapy. J Cyst Fibros (2015) 14:419–30. doi: 10.1016/J.JCF.2015.03.003
3. Hartl D, Gaggar A, Bruscia E, Hector A, Marcos V, Jung A, et al. Innate immunity in cystic fibrosis lung disease. J Cyst Fibros (2012) 11:363–82. doi: 10.1016/j.jcf.2012.07.003
4. Sherrard LJ, Bell SC, Tunney MM. The role of anaerobic bacteria in the cystic fibrosis airway. Curr Opin Pulm Med (2016) 22:637–43. doi: 10.1097/MCP.0000000000000299
5. Huffnagle GB, Dickson RP. The bacterial microbiota in inflammatory lung diseases. Clin Immunol (2015) 159:177–82. doi: 10.1016/j.clim.2015.05.022
6. Mathieu E, Escribano-Vazquez U, Descamps D, Cherbuy C, Langella P, Riffault S, et al. Paradigms of lung microbiota functions in health and disease, particularly, in asthma. Front Physiol (2018) 9:1168. doi: 10.3389/fphys.2018.01168
7. Huffnagle GB, Dickson RP, Lukacs NW. The respiratory tract microbiome and lung inflammation: A two-way street. Mucosal Immunol (2017) 10:299–306. doi: 10.1038/mi.2016.108
8. Wypych TP, Wickramasinghe LC, Marsland BJ. The influence of the microbiome on respiratory health. Nat Immunol (2019) 20:1279–90. doi: 10.1038/s41590-019-0451-9
9. N’Guessan PD, Haarmann H, Steiner T, Heyl K, Schreiber F, Heinrich A, et al. The moraxella catarrhalis-induced pro-inflammatory immune response is enhanced by the activation of the epidermal growth factor receptor in human pulmonary epithelial cells. Biochem Biophys Res Commun (2014) 450:1038–44. doi: 10.1016/j.bbrc.2014.06.102
10. Hansen CR, Pressler T, Nielsen KG, Jensen P, Bjarnsholt T, Høiby N. Inflammation in achromobacter xylosoxidans infected cystic fibrosis patients. J Cyst Fibros (2010) 9:51–8. doi: 10.1016/j.jcf.2009.10.005
11. Dickson RP, Huffnagle GB. The lung microbiome: New principles for respiratory bacteriology in health and disease. PloS Pathog (2015) 11:e1004923. doi: 10.1371/journal.ppat.1004923
12. Rogers GB, Masirah N, Zain M, Bruce KD, Burr LD, Chen AC, et al. A novel microbiota stratification system predicts future exacerbations in bronchiectasis. Ann Am Thorac Soc (2014) 11:496–503. doi: 10.1513/AnnalsATS.201310-335OC
13. Madapoosi SS, Cruickshank-Quin C, Opron K, Erb-Downward JR, Begley LA, Li G, et al. Lung microbiota and metabolites collectively associate with clinical outcomes in milder stage chronic obstructive pulmonary disease. Am J Respir Crit Care Med (2022) 206:427–39. doi: 10.1164/RCCM.202110-2241OC
14. Dicker AJ, Lonergan M, Keir HR, Smith AH, Pollock J, Finch S, et al. The sputum microbiome and clinical outcomes in patients with bronchiectasis: a prospective observational study. Lancet Respir Med (2021) 9:885–96. doi: 10.1016/S2213-2600(20)30557-9
15. Man WH, De Steenhuijsen Piters WAA, Bogaert D. The microbiota of the respiratory tract: Gatekeeper to respiratory health. Nat Rev Microbiol (2017) 15:259–70. doi: 10.1038/nrmicro.2017.14
16. Vissers M, De Groot R, Ferwerda G. Severe viral respiratory infections: Are bugs bugging? Mucosal Immunol (2014) 7:227–38. doi: 10.1038/mi.2013.93
17. Molyneaux PL, Cox MJ, Willis-Owen SAG, Mallia P, Russell KE, Russell AM, et al. The role of bacteria in the pathogenesis and progression of idiopathic pulmonary fibrosis. Am J Respir Crit Care Med (2014) 190:906–13. doi: 10.1164/rccm.201403-0541OC
18. Viennois E, Gewirtz AT, Chassaing B. Chronic inflammatory diseases: Are we ready for microbiota-based dietary intervention? Cell Mol Gastroenterol Hepatol (2019) 8:61–71. doi: 10.1016/J.JCMGH.2019.02.008
19. Rigauts C, Aizawa J, Taylor S, Rogers GB, Govaerts M, Cos P, et al. Rothia mucilaginosa is an anti-inflammatory bacterium in the respiratory tract of patients with chronic lung disease. Eur Respir J (2021) 59:2101293. doi: 10.1183/13993003.01293-2021
20. Tony-Odigie A, Wilke L, Boutin S, Dalpke AH, Yi B. Commensal bacteria in the cystic fibrosis airway microbiome reduce p. aeruginosa induced inflammation. Front Cell Infect Microbiol (2022) 12:824101/BIBTEX. doi: 10.3389/FCIMB.2022.824101/BIBTEX
21. Bertelsen A, Elborn SJ, Schock BC. Toll like receptor signalling by prevotella histicola activates alternative NF-κB signalling in cystic fibrosis bronchial epithelial cells compared to p. aeruginosa. PloS One (2020) 15:e0235803. doi: 10.1371/JOURNAL.PONE.0235803
22. Schuliga M. NF-kappaB signaling in chronic inflammatory airway disease. Biomolecules (2015) 5:1266–83. doi: 10.3390/BIOM5031266
23. Rico-Rosillo G, Vega-Robledo GB. The involvement of NF-κB transcription factor in asthma. Rev Alerg México (2011) 58:107–11.
24. Tony-Odigie A, Dalpke AH, Boutin S, Yi B. Commensal bacteria can inhibit the growth of p. aeruginosa in cystic fibrosis airway infections through a released metabolite. bioRxiv (2023), 526996. doi: 10.1101/2023.02.03.526996
25. Scoffield JA, Wu H. Oral streptococci and nitrite-mediated interference of pseudomonas aeruginosa. Infect Immun (2015) 83:101–7. doi: 10.1128/IAI.02396-14
26. Lee TWR, Brownlee KG, Conway SP, Denton M, Littlewood JM. Evaluation of a new definition for chronic pseudomonas aeruginosa infection in cystic fibrosis patients. J Cyst Fibros (2003) 2:29–34. doi: 10.1016/S1569-1993(02)00141-8
27. Whelan FJ, Waddell B, Syed SA, Shekarriz S, Rabin HR, Parkins MD, et al. Culture-enriched metagenomic sequencing enables in-depth profiling of the cystic fibrosis lung microbiota. Nat Microbiol (2020) 5:379–90. doi: 10.1038/s41564-019-0643-y
28. Vandeplassche E, Sass A, Lemarcq A, Dandekar AA, Coenye T, Crabbé A. In vitro evolution of pseudomonas aeruginosa AA2 biofilms in the presence of cystic fibrosis lung microbiome members. Sci Rep (2019) 9. doi: 10.1038/s41598-019-49371-y
29. Barrila J, Radtke AL, Crabbé A, Sarker SF, Herbst-Kralovetz MM, Ott CM, et al. Organotypic 3D cell culture models: Using the rotating wall vessel to study host-pathogen interactions. Nat Rev Microbiol (2010) 8:791–801. doi: 10.1038/nrmicro2423
30. Carterson AJ, Höner Zu Bentrup K, Ott CM, Clarke MS, Pierson DL, Vanderburg CR, et al. A549 lung epithelial cells grown as three-dimensional aggregates: Alternative tissue culture model for pseudomonas aeruginosa pathogenesis. Infect Immun (2005) 73:1129–40. doi: 10.1128/IAI.73.2.1129-1140.2005
31. Crabbé A, Liu Y, Matthijs N, Rigole P, de la Fuente-Nùñez C, Davis R, et al. Antimicrobial efficacy against pseudomonas aeruginosa biofilm formation in a three-dimensional lung epithelial model and the influence of fetal bovine serum. Sci Rep (2017) 7:1–13. doi: 10.1038/srep43321
32. Van den Bossche S, Vandeplassche E, Ostyn L, Coenye T, Crabbé A. Bacterial interference with lactate dehydrogenase assay leads to an underestimation of cytotoxicity. Front Cell Infect Microbiol (2020) 10:494. doi: 10.3389/fcimb.2020.00494
33. Maes M, Vanhaecke T, Cogliati B, Yanguas SC, Willebrords J, Rogiers V, et al. Measurement of apoptotic and necrotic cell death in primary hepatocyte cultures. In: Methods in molecular biology. Humana Press Inc (2015). 1250:349–61. doi: 10.1007/978-1-4939-2074-7_27
34. Vinken M, Blaauboer BJ. In vitro testing of basal cytotoxicity: Establishment of an adverse outcome pathway from chemical insult to cell death. Toxicol Vitr (2017) 39:104–10. doi: 10.1016/j.tiv.2016.12.004
35. Stein C, Makarewicz O, Bohnert JA, Pfeifer Y, Kesselmeier M, Hagel S, et al. Three dimensional checkerboard synergy analysis of colistin, meropenem, tigecycline against multidrug-resistant clinical klebsiella pneumonia isolates. PloS One (2015) 10:e0126479. doi: 10.1371/journal.pone.0126479
36. Odds FC. Synergy, antagonism, and what the chequerboard puts between them. J Antimicrob Chemother (2003) 52:1–1. doi: 10.1093/JAC/DKG301
37. Jafari M, Ansari-Pour N. Why, when and how to adjust your p values? Cell J (2019) 20:604–7. doi: 10.22074/cellj.2019.5992
38. Liu T, Zhang L, Joo D, Sun SC. NF-κB signaling in inflammation. Signal Transduct Target Ther (2017) 2:1–9. doi: 10.1038/sigtrans.2017.23
39. Nichols DP, Chmiel JF. Inflammation and its genesis in cystic fibrosis. Pediatr Pulmonol (2015) 50:S39–56. doi: 10.1002/ppul.23242
40. Mata M, Pallardo F, Morcillo EJ, Cortijo J. Piclamilast inhibits the pro-apoptotic and anti-proliferative responses of A549 cells exposed to H2O2 via mechanisms involving AP-1 activation. Free Radic Res (2012) 46:690–9. doi: 10.3109/10715762.2012.669040
41. Wang W, Zheng JP, Zhu SX, Guan WJ, Chen M, Zhong NS. Carbocisteine attenuates hydrogen peroxide-induced inflammatory injury in A549 cells via NF-κB and ERK1/2 MAPK pathways. Int Immunopharmacol (2015) 24:306–13. doi: 10.1016/J.INTIMP.2014.12.018
42. Hsu JY, Chu JJ, Chou MC, Chen YW. Dioscorin pre-treatment protects A549 human airway epithelial cells from hydrogen peroxide-induced oxidative stress. Inflammation (2013) 36:1013–9. doi: 10.1007/s10753-013-9633-z
43. Boukhenouna S, Wilson MA, Bahmed K, Kosmider B. Reactive oxygen species in chronic obstructive pulmonary disease. Oxid Med Cell Longev (2018) 2018:5730395. doi: 10.1155/2018/5730395
44. Barnes PJ. Inflammatory mechanisms in patients with chronic obstructive pulmonary disease. J Allergy Clin Immunol (2016) 138:16–27. doi: 10.1016/j.jaci.2016.05.011
45. Huang RY, Pei L, Liu Q, Chen S, Dou H, Shu G, et al. Isobologram analysis: A comprehensive review of methodology and current research. Front Pharmacol (2019) 10:1222. doi: 10.3389/fphar.2019.01222
46. Zhao L, Au JLS, Wientjes MG. Comparison of methods for evaluating drug-drug interaction. Front Biosci - Elit (2010) 2:241–9. doi: 10.2741/e86
47. Brennan S, Gangell C, Wainwright C, Sly PD. Disease surveillance using bronchoalveolar lavage. Paediatr Respir Rev (2008) 9:151–9. doi: 10.1016/J.PRRV.2008.01.002
48. Marsland BJ, Gollwitzer ES. Host-microorganism interactions in lung diseases. Nat Rev Immunol (2014) 14:827–35. doi: 10.1038/nri3769
49. Acosta N, Heirali A, Somayaji R, Surette MG, Workentine ML, Sibley CD, et al. Sputum microbiota is predictive of long-term clinical outcomes in young adults with cystic fibrosis. Thorax (2018) 73:1016–25. doi: 10.1136/THORAXJNL-2018-211510
50. Wu BG, Segal LN. Lung microbiota and its impact on the mucosal immune phenotype. Microbiol Spectr (2017) 5. doi: 10.1128/microbiolspec.bad-0005-2016
51. Gupta S, Shariff M, Chaturvedi G, Sharma A, Goel N, Yadav M, et al. Comparative analysis of the alveolar microbiome in COPD, ECOPD, sarcoidosis, and ILD patients to identify respiratory illnesses specific microbial signatures. Sci Rep (2021) 11:1–11. doi: 10.1038/s41598-021-83524-2
52. Marietta EV, Murray JA, Luckey DH, Jeraldo PR, Lamba A, Patel R, et al. Suppression of inflammatory arthritis by human gut-derived prevotella histicola in humanized mice. Arthritis Rheumatol (2016) 68:2878–88. doi: 10.1002/art.39785
53. Bertelsen A, Elborn JS, Schock BC. Microbial interaction: Prevotella spp. reduce p. aeruginosa induced inflammation in cystic fibrosis bronchial epithelial cells. J Cyst Fibros (2021) 20:682–91. doi: 10.1016/j.jcf.2021.04.012
54. Myers S, Do T, Meade JL, Tugnait A, Vernon JJ, Pistolic J, et al. Immunomodulatory streptococci that inhibit CXCL8 secretion and NFκB activation are common members of the oral microbiota. J Med Microbiol (2021) 70:1329. doi: 10.1099/jmm.0.001329
55. Zemanick ET, Harris JK, Wagner BD, Robertson CE, Sagel SD, Stevens MJ, et al. Inflammation and airway microbiota during cystic fibrosis pulmonary exacerbations. PloS One (2013) 8:e62917. doi: 10.1371/JOURNAL.PONE.0062917
56. Zemanick ET, Wagner BD, Robertson CE, Stevens MJ, Szefler SJ, Accurso FJ, et al. Assessment of airway microbiota and inflammation in cystic fibrosis using multiple sampling methods. Ann Am Thorac Soc (2015) 12:221–9. doi: 10.1513/AnnalsATS.201407-310OC
57. Arrieta MC, Sadarangani M, Brown EM, Russell SL, Nimmo M, Dean J, et al. A humanized microbiota mouse model of ovalbumin-induced lung inflammation. Gut Microbes (2016) 7:342–52. doi: 10.1080/19490976.2016.1182293
58. Arrieta MC, Stiemsma LT, Dimitriu PA, Thorson L, Russell S, Yurist-Doutsch S, et al. Early infancy microbial and metabolic alterations affect risk of childhood asthma. Sci Transl Med (2015) 7:307ra152. doi: 10.1126/scitranslmed.aab2271
59. Taylor SL, Leong LEX, Choo JM, Wesselingh S, Yang IA, Upham JW, et al. Inflammatory phenotypes in patients with severe asthma are associated with distinct airway microbiology. J Allergy Clin Immunol (2018) 141:94–103.e15. doi: 10.1016/j.jaci.2017.03.044
60. Guo Y, Wei C, Liu C, Li D, Sun J, Huang H, et al. Inhibitory effects of oral actinomyces on the proliferation, virulence and biofilm formation of candida albicans. Arch Oral Biol (2015) 60:1368–74. doi: 10.1016/J.ARCHORALBIO.2015.06.015
61. Chen R, Xie F, Zhao J, Yue B. Suppressed nuclear factor-kappa b alleviates lipopolysaccharide-induced acute lung injury through downregulation of CXCR4 mediated by microRNA-194. Respir Res (2020) 21:144. doi: 10.1186/s12931-020-01391-3
62. Bhattacharyya S, Gutti U, Mercado J, Moore C, Pollard HB, Biswas R. MAPK signaling pathways regulate IL-8 mRNA stability and IL-8 protein expression in cystic fibrosis lung epithelial cell lines. Am J Physiol - Lung Cell Mol Physiol (2011) 300:81–7. doi: 10.1152/ajplung.00051.2010
63. He W, Qu T, Yu Q, Wang Z, Lv H, Zhang J, et al. LPS induces IL-8 expression through TLR4, MyD88, NF-kappaB and MAPK pathways in human dental pulp stem cells. Int Endod J (2013) 46:128–36. doi: 10.1111/J.1365-2591.2012.02096.X
64. Wu YL, Kou YR, Ou HL, Chien HY, Chuang KH, Liu HH, et al. Glucosamine regulation of LPS-mediated inflammation in human bronchial epithelial cells. Eur J Pharmacol (2010) 635:219–26. doi: 10.1016/J.EJPHAR.2010.02.044
65. Azghani AO, Baker JW, Shetty S, Miller EJ, Bhat GJ. Pseudomonas aeruginosa elastase stimulates ERK signaling pathway and enhances IL- 8 production by alveolar epithelial cells in culture. Inflammation Res (2002) 51:506–10. doi: 10.1007/PL00012420
66. Zhang LL, Chen B, Fan XY, Wu SS, Zhang SQ, Wu HM. LPS cooperates with poly-L-Arginine to promote IL-6 and IL-8 release via the JNK signaling pathway in NCI-H292 cells. J Immunol Res (2016) 2016. doi: 10.1155/2016/3421060
67. Tsai YG, Wen YS, Wang JY, Yang KD, Sun HL, Liou JH, et al. Complement regulatory protein CD46 induces autophagy against oxidative stress-mediated apoptosis in normal and asthmatic airway epithelium. Sci Rep (2018) 8:1–11. doi: 10.1038/s41598-018-31317-5
68. Kaci G, Goudercourt D, Dennin V, Pot B, Doré J, Ehrlich SD, et al. Anti-inflammatory properties of streptococcus salivarius, a commensal bacterium of the oral cavity and digestive tract. Appl Environ Microbiol (2014) 80:928–34. doi: 10.1128/AEM.03133-13
69. Wescombe PA, Hale JD, Heng NC, Tagg JR. Developing oral probiotics from streptococcus salivarius. Future Microbiol (2012) 7:1355–71. doi: 10.2217/fmb.12.113
70. Cosseau C, Devine DA, Dullaghan E, Gardy JL, Chikatamarla A, Gellatly S, et al. The commensal streptococcus salivarius K12 downregulates the innate immune responses of human epithelial cells and promotes host-microbe homeostasis. Infect Immun (2008) 76:4163–75. doi: 10.1128/IAI.00188-08
Keywords: lung microbiome, beneficial commensal, chronic lung disease (CLD), host-microbe interaction, inflammation
Citation: Goeteyn E, Grassi L, Van den Bossche S, Rigauts C, Vande Weygaerde Y, Van Braeckel E, Maes T, Bracke KR and Crabbé A (2023) Commensal bacteria of the lung microbiota synergistically inhibit inflammation in a three-dimensional epithelial cell model. Front. Immunol. 14:1176044. doi: 10.3389/fimmu.2023.1176044
Received: 28 February 2023; Accepted: 30 March 2023;
Published: 21 April 2023.
Edited by:
Alice Prince, Columbia University, United StatesReviewed by:
Shuo Zheng, Virginia Commonwealth University, United StatesLindsay Caverly, Michigan Medicine, University of Michigan, United States
Benjamin G. Wu, New York University, United States
Copyright © 2023 Goeteyn, Grassi, Van den Bossche, Rigauts, Vande Weygaerde, Van Braeckel, Maes, Bracke and Crabbé. This is an open-access article distributed under the terms of the Creative Commons Attribution License (CC BY). The use, distribution or reproduction in other forums is permitted, provided the original author(s) and the copyright owner(s) are credited and that the original publication in this journal is cited, in accordance with accepted academic practice. No use, distribution or reproduction is permitted which does not comply with these terms.
*Correspondence: Aurélie Crabbé, YXVyZWxpZS5jcmFiYmVAdWdlbnQuYmU=