- 1Department of Sport Rehabilitation, Shanghai University of Sport, Shanghai, China
- 2School of Rehabilitation Science, Shanghai University of Traditional Chinese Medicine, Shanghai, China
- 3Department of Rehabilitation Medicine, Shanghai Shangti Orthopaedic Hospital, Shanghai, China
Neuropathic pain (NP) is a frequent condition caused by a lesion in, or disease of, the central or peripheral somatosensory nervous system and is associated with excessive inflammation in the central and peripheral nervous systems. Repetitive transcranial magnetic stimulation (rTMS) is a supplementary treatment for NP. In clinical research, rTMS of 5–10 Hz is widely placed in the primary motor cortex (M1) area, mostly at 80%–90% RMT, and 5–10 treatment sessions could produce an optimal analgesic effect. The degree of pain relief increases greatly when stimulation duration is greater than 10 days. Analgesia induced by rTMS appears to be related to reestablishing the neuroinflammation system. This article discussed the influences of rTMS on the nervous system inflammatory responses, including the brain, spinal cord, dorsal root ganglia (DRG), and peripheral nerve involved in the maintenance and exacerbation of NP. rTMS has shown an anti-inflammation effect by decreasing pro-inflammatory cytokines, including IL-1β, IL-6, and TNF-α, and increasing anti-inflammatory cytokines, including IL-10 and BDNF, in cortical and subcortical tissues. In addition, rTMS reduces the expression of glutamate receptors (mGluR5 and NMDAR2B) and microglia and astrocyte markers (Iba1 and GFAP). Furthermore, rTMS decreases nNOS expression in ipsilateral DRGs and peripheral nerve metabolism and regulates neuroinflammation.
1 Introduction
Neuropathic pain (NP) is a common chronic pain condition that substantially influence patients’ quality of life and is caused by a disease or lesion affecting the somatosensory nervous system (1, 2). According to epidemiological research, the frequency of NP can be as high as 7% to 8% in the general population, accounting for 20% to 25% of those who experience chronic pain (3, 4). The basic characteristics of NP include sensory abnormalities, spontaneous pain, and hyperalgesia (5). NP management focuses on treating symptoms. In addition to using recommended first-line antidepressants and antiepileptic drugs, repetitive transcranial magnetic stimulation (rTMS) has demonstrated effectiveness in subjects who have not responded to conventional medical treatment (1, 6).
The central and peripheral nervous systems exhibit excessive inflammation in neuropathic pain, which may contribute in initiating and maintaining chronic pain. NP state is associated with infiltrating various immune cells near or within peripheral nerves. Macrophages, cytokines, T-lymphocytes, and chemokines are key players in neuroinflammation and initially used to promote healing and regeneration (7). At the early stage of NP, macrophages and neutrophils sensitize sensory neurons of the dorsal root ganglia (DRG) via mediators such as tumor necrosis factor-alpha (TNF-α) and diverse interleukins(ILs), such as interleukin-10 (IL-10), interleukin-1β (IL-1β), interleukin-4 (IL-4) and interleukin-17 (IL-17), and interferon-γ (8–11). Sensitized afferents activate microglia by releasing ATP, colony stimulating factor 1 (CSF1), chemokines (CCL2, CX3CL1), and proteases, resulting in up-regulation of microglial markers such as ionized calcium-binding adapter molecule 1 (Iba-1) and CD11b in the spinal cord (8, 12, 13). According to a recent explosion in microglial research, numerous signaling molecules are changed in microglia and play a key role in the pathophysiology of NP (14). Microglia proliferation and activation elicit astrocytes, which release pro-inflammatory agents and promote neuronal activity. In addition to microglia and astrocytes, the primary afferent neurons and bone marrow-derived invading and tissue-resident macrophages cooperate to regulate pain signals in peripheral tissues (15). Molecular and cellular imaging of NP helps locate nerve injury or neuroinflammation with accuracy and confidence (16). MicroRNAs and other noncoding RNAs are recently explored as potential master switches that could connect nerve damage, pain, and inflammation (7). In a recently published systematic review and meta-analysis of 38 studies, the outcome demonstrated that rTMS has analgesic effects on NP. According to a subgroup analysis, the key determinants of treatment efficacy include treatment site, stimulation frequency, and interaction between frequency and lesion of the stimulation site (17).
Studies on the mechanisms of rTMS in NP are ongoing. rTMS-induced analgesia is based on the remodeling of the endogenous opioid system and the restoration of normal cortical excitability (18, 19). rTMS also produces analgesic effects by inhibiting the transmission of nociceptive signals (20, 21). The activated motor cortex induced by rTMS may alter the activity of the nearby motor cortex and the thalamic nuclei. Increasing studies have been conducted on how rTMS interact with the nociceptive system by inducing anti-inflammatory cytokines. This review discussed the interrelationships between rTMS and neuroinflammation of NP models and patients.
2 Effect of rTMS on neuropathic pain
European rTMS has wide clinical applications and continuously distributes multiple pulses at a fixed frequency compared with chronic implant therapy (22). The clinical application of rTMS in managing NP involves many central and peripheral nervous diseases, including stroke, Parkinson’s disease, spinal cord injury, and sciatica (23). Guidelines on neuromodulation for chronic pain published in the European Academy of Neurology provided a weak recommendation for using rTMS targeting the primary motor cortex (M1) in NP (24). Low-frequency (1 Hz) rTMS can inhibit nerve cell metabolism and reduce cortex excitability, and high-frequency (≥1 Hz) rTMS can boost nerve cell metabolism and cerebral cortex’s excitability (25). The International Union of Clinical Neurophysiology guideline states that high-frequency rTMS stimulation of the contralateral M1 area has a definite analgesic effect on NP, and a long and continuous course of treatment is highly beneficial and recommended for Grade A (26, 27). In addition to M1, patients with severe pain or depression may benefit from treatment in the left dorsolateral prefrontal cortex (PFC) (28). The concentrated stimulation site of rTMS, such as the “8” coil, is superior to the circular coil and has high stimulation frequency, large stimulation pulse, and many stimulation times, making it an effective method to improve pain. Stimulating the M1 area near the representative pain area can produce a potent analgesic effect (29, 30).
Hasan (31) used 10 Hz rTMS for five sessions as an intervention for patients with PSP to explore the effect of rTMS on pain sensitivity and somatic sensory function of patients with NP. The results showed that the affected body’s sensory defect to temperature change was significantly improved, and the pain score was greatly relieved, indicating that rTMS might improve the neural pathway shared by noxious stimulus and temperature signals. Concerning the persistence of the therapeutic effect of NP caused by refractory SCI managed by rTMS, Yilmaz et al. (32) adopted a 10 Hz rTMS treatment program for 10 consecutive days and followed up with the subjects for 10 days, 6 weeks, and 6 months to evaluate their pain. The results showed that rTMS could relieve pain for up to 6 weeks. Hosomi et al. (33) conducted four weekly sessions of 5 Hz rTMS in the hand area of M1 patients with upper extremity NP and the foot region of lower extremity pain, and the subjects showed significant analgesic effects after the treatment cycle. However, Hodaj H et al. (34) applied 10Hz rTMS to stimulate the motor cortex of 57 patients with chronic NP in different regions for 12 daily sessions in 3 weeks, and all the patients experienced significant pain relief compared with baseline. A guideline on the safety, ethical considerations, and clinical application of rTMS revealed that 20 Hz stimulation for the treatment of central NP could also provide effective pain relief by stimulating the M1 area on the opposite side of the patient’s pain area (35). Jin Y et al. (36) found regardless of the intensity (5, 10, or 20 Hz), rTMS had an effect on the relief of chronic NP and the pain could be relieved to the maximum extent after the fifth stimulus. High-frequency rTMS therapy for NP is mostly more than 80% of the resting motor threshold, and the total pulse number is more than 1000 (37). rTMS therapy is usually administered once a day for 10–15 consecutive days. The pain relief degree of patients with a stimulation duration greater than 10 days is higher than that of patients with a stimulation duration less than 10 days. The shorter the treatment duration, the shorter the duration of the analgesic effect (38). Currently, no explicit therapeutic parameter of rTMS for NP caused by different diseases has been established in the academic circle. The main site of rTMS is the M1 region for stimulation, and high-frequency stimulation at above 5 Hz is mainly used for analgesia. More research information about the effect of rTMS on NP is shown in Table 1. High-frequency rTMS has demonstrated a good therapeutic effect on NP, and the stimulation site is often on the contrary side of the pain cortex for central stimulation. In clinical practice, 5–10 Hz rTMS is frequently applied in the M1 area contralaterally of NP, typically at an RMT of 80%–90%; 5–10 treatments are usually sufficient to produce a noticeable analgesic effect (39).
3 rTMS regulates neuroinflammation in neuropathic pain
Infections in the CNS rarely occur. The majority of infections and tissue damage occur at the periphery, and neuroinflammatory responses are triggered by peripheral inflammation, which involves the neurons, glia, and blood brain barrier. This neuroinflammatory response leads to synaptic dysfunction, neuronal damage, and worsening of several pathologies in the brain, spinal cord, dorsal root ganglia, and peripheral nerve (40–43). Immune cells can produce pro- or anti-nociceptive mediators, modulating pain in various ways. Pro-inflammatory cytokines such as TNF-α, interleukin-6 (IL-6) and IL-1β are mediators of inflammatory and neuropathic pain (44). Anti-inflammatory cytokines such as IL-10 and IL-4 act to regulate the inflammatory process, limiting tissue damage and restoring homeostasis (45). The interactions between inflammation and NP are bidirectional. These cytokines can adjust the inhibitory and excitatory synaptic transmission, ultimately improving pain signals’ transmission to the brain. rTMS increases the expression of anti-inflammatory and decreases that of pro-inflammatory cytokines (46–48), indicating that it plays a significant role in neuroinflammation (49) (see Figure1).
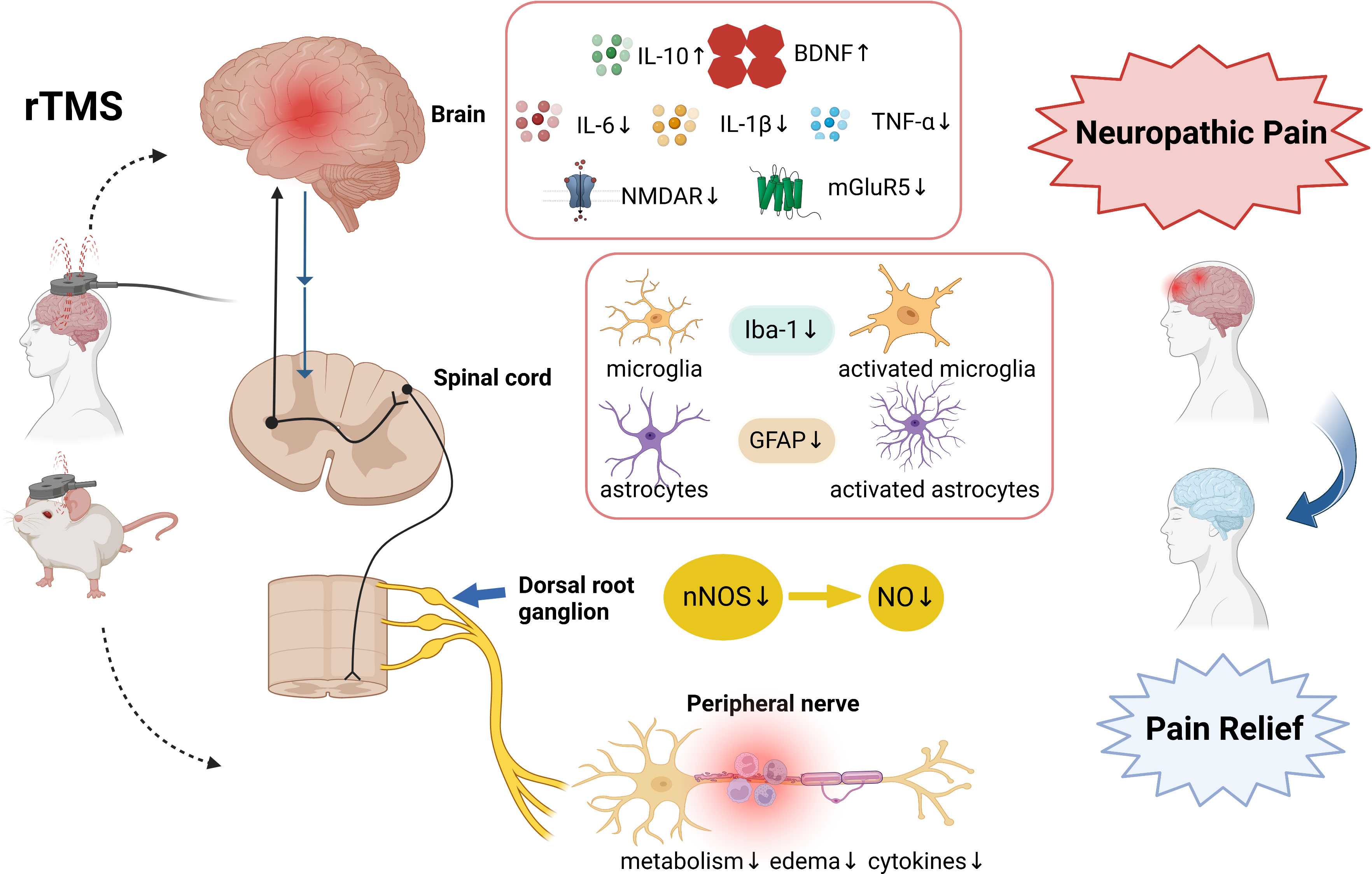
Figure 1 The involved mechanisms in rTMS on neuropathic pain relief. The analgesic mechanism involves neuroinflammatory n cytokines, glutamate receptors, microglia and astrocyte markers, nNOS, and peripheral nerve inflammation. Created with BioRender.com. rTMS, Repetitive Transcranial magnetic stimulation; IL-10, interleukin -10; IL-6, interleukin -6; IL-1β, interleukin -1β; BDNF, brain-derived neurotrophic factor; TNF-α, tumour necrosis factor-alpha; mGluR5, metabotropic glutamate receptors 5; NMDAR2B, N-Methyl-D-Aspartic acid receptor type 2B; Iba-1, Ionized calcium-binding adapter molecule 1; GFAP, glial fibrillary acidic protein nNOS, nitric oxide synthase; NO, Nitric Oxide.
3.1 Effects of rTMS on cortical neuroinflammation
NP is associated with excessive cortical inflammation, leading to persistent pain (50). More severe brain inflammation can be observed in patients with NP compared with that in pain-free patients. The inflamed brain can induce oxidative stress and the release of pro-inflammatory factors, such as IL-1β, TNF-α, and nitric oxide (NO) (51, 52). The pain-related symptoms can be relieved by reducing neuroinflammation activation in the cortical areas and vice versa. Inflammatory changes in different brain regions are different. According to a study, NP decreases the complexity of excitatory synaptic connections and brain-derived neurotrophic factor (BDNF) expression in the hippocampus, and the opposite trends are dependent on TNF-α (53). BDNF is down-regulated in the PFC after NP (54). In the anterior cingulate cortex, which is the first response region to painful stimuli, NP increases the expression of TNF-α, chemokine CX3CL1, and IL-6 (55).
rTMS focuses on a particular cortical site to reduce neuroinflammation and has an analgesic effect. Toledo et al. (49, 56) demonstrated that 1 Hz rTMS treatment for 8 days could alter IL-10 levels in the hippocampus and PFC in NP rats. IL-10 plays a significant role in NP conditions. IL-10 reverses memory and learning deficits in NP models by preventing the negative effects of IL-1β or lipopolysaccharide on long-term potentiation (57–59). IL-6 and TNF-α dose-dependently induce the release of IL-10 by microglia. Hu et al. (60) found that rTMS at 10 Hz could noticeably reduce inflammatory factors in NP rats, including TNF-α, lL-6, and IL-1β in anterior agranular insular (AId). In NP rats, IL-1β levels rose in the brain’s prefrontal cortex, brainstem, hippocampus, and peripheral areas (61, 62). IL-1β and TNF-αprimarily mediate the neuroinflammation and stress response. Hu et al. also found that the left AId of the animals with NP caused by CCI exhibited rising levels of metabotropic glutamate receptors 5 (mGluR5) and N-methyl-D-aspartic acid receptor type 2B (NMDAR2B), and 10 Hz-rTMS intervention applied on the left AId could reverse the expression. NMDAR and mGluR5 are key receptors for pain processing and mental changes (63, 64). When NP is present, pro-inflammatory substances such as TNF-α and IL-1β enhance NMDAR-mediated current frequency and spontaneous postsynaptic current, stimulate glutamatergic neurotransmission, and result in mechanical hypersensitivity (65, 66). In addition, rTMS-treated NP rats presented highs level of BDNF in the PFC and hippocampus (49, 56). BDNF is a crucial neuromodulator in the nervous system with alternating persistent pain states and inflammation conditions (54). It is also related to nociceptive hypersensitivity. According to a report, rTMS improves the signaling between BDNF and its receptor TrkB in the cortex (67). rTMS alters the activation of cortical and subcortical structures, such as orbitofrontal cortices, anterior cingulate, medial thalamus, and periaqueductal gray matter (68). Overall, rTMS treatment has an essential impact on the nociceptive responses of NP and a beneficial effect on the levels of neurotrophin and anti-neuroinflammatory cytokines.
3.2 Effects of rTMS on spinal cord neuroinflammation
NP induces sustained inflammatory reactions in the spinal cord and NP rats to increase the level of cytokines, including IL-1β and TNF-α, and the activation level of microglia/macrophages in the dorsal horn (69). rTMS exhibits an inhibition effect on spinal cord inflammation, mainly related to the augmentation of anti-nociceptive effect in NP rats. Kim et al. (70)presented that rTMS applied on the motor cortex reduced the expression of Iba-1 and glial fibrillary acidic protein (GFAP) in spinal dorsal and ventral horns at the L4–L5 levels in NP rats (70). Pro-inflammatory cytokines, chemokines, and their receptors are constantly upregulated by activated microglia and astrocytes, resulting in chronic allodynia development (71–76). In another animal study, Luo et al. (77) stated that rTMS stimulation could control inflammation, suppress apoptosis, and promote neurogenesis. rTMS promotes the anti-inflammatory polarization of microglia. Through rTMS stimulation on microglia, anti-inflammatory cytokine production is promoted in vivo and in vitro. In general, rTMS can stimulate neurogenesis, enhance brain function recovery, and modify microglia polarization toward an anti‐inflammatory phenotype.
In addition to microglia activation and leukocyte infiltration, reactive astrogliosis is a crucial component of inflammation. High-frequency rTMS (50 Hz) can stimulate IL-6 release, boost Ca2+ inflow, and encourage astrocyte proliferation in vitro (78–80). In the spinal cord injury model, rTMS promoted reactive white matter astrocytes’ migration to a CNS lesion (81). In rats with regional brain and spinal cord injury, high-frequency rTMS could reduce glial activation and related neuroinflammation (70, 82). These findings suggested that astrocytic activation may be influenced by rTMS to decrease neuroinflammation. Yang et al. (83) also found that GFAP and 5-bromo-2-deoxyuridine (BrdU) in the dorsal horn were reduced after 20 Hz rTMS treatment for 10 days on M1 in NP rats induced by sciatic nerve ligation. GFAP and BrdU are often used to observe astrocyte proliferation and activation. Inflammation-associated GFAP abnormalities and astrocytic plasticity problems also impact neural tissue growth and repair, degeneration, and death. Overall, rTMS reverses mechanical hyperalgesia, reduces pain via suppressing the activations of astrocytes and microglia, and decreases pro-inflammatory cytokine staining in the NP model, especially central nerve pain after SCI.
3.3 Effects of rTMS on dorsal root ganglia neuroinflammation
NP is closely associated with the hyperresponsiveness of sensory neurons from DRG (84). Glial cells in the DRG produce inflammatory markers and contribute to the development of NP. Neurotrophic factors change NP inflammation in DRG neurons, activate inflammatory agents, and induce the expression of different ion channels (85–87). Inflammation drives molecular alterations in the DRG where IL-1β and purinoceptors are upregulated (88). TNF-α elevates phosphorylation (pERK) in small and medium-sized DRG neurons with NP (89). Yang et al. (83) presented that 20 Hz high frequency rTMS could attenuate brush-evoked and spontaneous pain symptoms and reduce neuronal nitric oxide synthase (nNOS) expression in ipsilateral DRGs in rats with peripheral NP. NO is an extremely unstable free radical gas related to spinal cord hyperalgesia and neurosensitization (90–92). A crucial enzyme in NO production is nitric oxide synthase (NOS). NOS isoform inhibition has recently been promoted as a potential treatment for NP conditions (93–95). Accordingly, rTMS alleviates NP conditions by suppressing nNOS overexpression in ipsilateral DRGs.
3.4 Effects of rTMS on peripheral nerve neuroinflammation
NP can be produced by inflamed but otherwise uninjured axons (96). Elevated levels of local and serum TNF-α, IL-6, IL-1β, and IL-2 are associated with neuroinflammation and peripheral inflammation in NP (7, 61). Molecules responsible for anti-inflammation have been linked to NP recovery, highlighting the association between inflammation and pain (97–99). “Remote effect” is the term used to describe how rTMS may impact the area of the brain related to the stimulation location and the stimulation site itself (100, 101). rTMS reduces sciatic nerve metabolism and raises the sciatic nerve function index in CCI rats. Increased spontaneous activity and metabolic alterations following nerve damage contribute to the symptoms of NP. Glucose metabolism is necessary for neuronal function, and PET imaging can identify areas with high metabolic activity using modified glucose molecules (16, 102). Following nerve damage, inflammation lowers the amount of glucose in the injured nerve tissue, elevating the phosphorylation of FDG and the relative SUV (103). In one study, the degenerative alterations of the sciatic nerve tissue were less severe in the rTMS groups than in the CCI group. Under light microscope observation, the 10 Hz rTMS treatment group presented complete and distinct sciatic nerve tissues. The inflammatory cells invaded the spaces between the nerve fibers, and the lymphocytes’ nuclei were rounded and heavily stained. In the 1 Hz rTMS-treated group, sciatic nerve pathological alterations were considerably more severe than those in the 10 Hz-rTMS group. Therefore, neuromodulation via rTMS may provide an accessible, practical, low-cost, and noninvasive approach to alleviate symptoms associated with NP (104).
4 Conclusion
This review aimed to summarize the neuroinflammation changes in NP induced by rTMS. In clinical research, rTMS of 5–10 Hz is widely applied in the primary motor cortex (M1) area, mostly at 80%–90% RMT, and 5–10 treatment sessions could produce an optimal analgesic effect. rTMS shows the anti-inflammation effect by decreasing pro-inflammatory cytokines, including TNF-α, IL-1β, and IL-6, and increasing anti-inflammatory cytokines, including IL-10 and BDNF, in cortical and subcortical tissue. rTMS also reduces mGluR5 and NMDAR2B expression levels, regulates neuroinflammation, down-regulates microglia and astrocytes markers Iba1 and GFAP, and decreases the expression of nNOS in DRGs and ipsilateral and peripheral nerve metabolism. In the future, additional in vitro and in vivo studies and clinical trials are required to investigate the mechanism of rTMS on NP and establish the foundation for the clinical treatment.
Author contributions
X-QW conceived the review. Y-WB and Q-HY drafted the manuscript and searched the literature to identify eligible trials. Y-WB and Q-HY extracted and analyzed data. X-QW and P-JC revised the tables in the drafted manuscript. All authors contributed to the article and approved the submitted version.
Funding
The authors disclosed receipt of financial support from the following for the research, authorship, and/or publication of this article: the Scientific and Technological Research Program of the Shanghai Science and Technology Committee (fund number:19080503100; 21S31902400), and the Shanghai Key Lab of Human Performance (Shanghai University of Sport, fund number: 11DZ2261100); Shanghai Frontiers Science Research Base of Exercise and Metabolic Health; Talent Development Fund of Shanghai Municipal (2021081); Shanghai Clinical Research Center for Rehabilitation Medicine (21MC1930200).
Acknowledgments
The authors thank all the participants and clinical researchers involved in the publications cited in this review and peer reviewers who contributed to the continuous improvement of this article.
Conflict of interest
The authors declare that the research was conducted in the absence of any commercial or financial relationships that could be construed as a potential conflict of interest.
Publisher’s note
All claims expressed in this article are solely those of the authors and do not necessarily represent those of their affiliated organizations, or those of the publisher, the editors and the reviewers. Any product that may be evaluated in this article, or claim that may be made by its manufacturer, is not guaranteed or endorsed by the publisher.
References
1. Finnerup NB, Kuner R, Jensen TS. Neuropathic pain: from mechanisms to treatment. Physiol Rev (2021) 101(1):259–301. doi: 10.1152/physrev.00045.2019
2. Bouhassira D. Neuropathic pain: definition, assessment and epidemiology. Rev Neurol (Paris). (2019) 175(1-2):16–25. doi: 10.1016/j.neurol.2018.09.016
3. Torrance N, Smith BH, Bennett MI, Lee AJ. The epidemiology of chronic pain of predominantly neuropathic origin. Results Gen population survey. J Pain. (2006) 7(4):281–9. doi: 10.1016/j.jpain.2005.11.008
4. Bouhassira D, Lantéri-Minet M, Attal N, Laurent B, Touboul C. Prevalence of chronic pain with neuropathic characteristics in the general population. Pain. (2008) 136(3):380–7. doi: 10.1016/j.pain.2007.08.013
5. Baron R, Binder A, Wasner G. Neuropathic pain: diagnosis, pathophysiological mechanisms, and treatment. Lancet Neurol (2010) 9(8):807–19. doi: 10.1016/S1474-4422(10)70143-5
6. Cavalli E, Mammana S, Nicoletti F, Bramanti P, Mazzon E. The neuropathic pain: an overview of the current treatment and future therapeutic approaches. Int J Immunopathol Pharmacol (2019) 33:2058738419838383. doi: 10.1177/2058738419838383
7. Sommer C, Leinders M, Üçeyler N. Inflammation in the pathophysiology of neuropathic pain. Pain. (2018) 159(3):595–602. doi: 10.1097/j.pain.0000000000001122
8. Ji RR, Chamessian A, Zhang YQ. Pain regulation by non-neuronal cells and inflammation. Science. (2016) 354(6312):572–7. doi: 10.1126/science.aaf8924
9. Moehring F, Halder P, Seal RP, Stucky CL. Uncovering the cells and circuits of touch in normal and pathological settings. Neuron. (2018) 100(2):349–60. doi: 10.1016/j.neuron.2018.10.019
10. Thacker MA, Clark AK, Marchand F, McMahon SB. Pathophysiology of peripheral neuropathic pain: immune cells and molecules. Anesth Analg. (2007) 105(3):838–47. doi: 10.1213/01.ane.0000275190.42912.37
11. Totsch SK, Sorge RE. Immune system involvement in specific pain conditions. Mol Pain. (2017) 13:1744806917724559. doi: 10.1177/1744806917724559
12. Guan Z, Kuhn JA, Wang X, Colquitt B, Solorzano C, Vaman S, et al. Injured sensory neuron-derived CSF1 induces microglial proliferation and DAP12-dependent pain. Nat Neurosci (2016) 19(1):94–101. doi: 10.1038/nn.4189
13. Grace PM, Hutchinson MR, Maier SF, Watkins LR. Pathological pain and the neuroimmune interface. Nat Rev Immunol (2014) 14(4):217–31. doi: 10.1038/nri3621
14. Chen G, Zhang YQ, Qadri YJ, Serhan CN, Ji RR. Microglia in pain: detrimental and protective roles in pathogenesis and resolution of pain. Neuron. (2018) 100(6):1292–311. doi: 10.1016/j.neuron.2018.11.009
15. Domoto R, Sekiguchi F, Tsubota M, Kawabata A. Macrophage as a peripheral pain regulator. Cells. (2021) 10(8):1881. doi: 10.3390/cells10081881
16. Tung KW, Behera D, Biswal S. Neuropathic pain mechanisms and imaging. Semin Musculoskelet Radiol (2015) 19(2):103–11. doi: 10.1055/s-0035-1547371
17. Jiang X, Yan W, Wan R, Lin Y, Zhu X, Song G, et al. Effects of repetitive transcranial magnetic stimulation on neuropathic pain: a systematic review and meta-analysis. Neurosci Biobehav Rev (2022) 132:130–41. doi: 10.1016/j.neubiorev.2021.11.037
18. Leo RJ, Latif T. Repetitive transcranial magnetic stimulation (rTMS) in experimentally induced and chronic neuropathic pain: a review. J Pain. (2007) 8(6):453–9. doi: 10.1016/j.jpain.2007.01.009
19. Moisset X, de Andrade DC, Bouhassira D. From pulses to pain relief: an update on the mechanisms of rTMS-induced analgesic effects. Eur J Pain. (2016) 20(5):689–700. doi: 10.1002/ejp.811
20. Bestmann S, Baudewig J, Siebner HR, Rothwell JC, Frahm J. Functional MRI of the immediate impact of transcranial magnetic stimulation on cortical and subcortical motor circuits. Eur J Neurosci (2004) 19(7):1950–62. doi: 10.1111/j.1460-9568.2004.03277.x
21. Pleger B, Janssen F, Schwenkreis P, Völker B, Maier C, Tegenthoff M. Repetitive transcranial magnetic stimulation of the motor cortex attenuates pain perception in complex regional pain syndrome type I. Neurosci Lett (2004) 356(2):87–90. doi: 10.1016/j.neulet.2003.11.037
22. Choi GS, Chang MC. Effects of high-frequency repetitive transcranial magnetic stimulation on reducing hemiplegic shoulder pain in patients with chronic stoke: a randomized controlled trial. Int J Neurosci (2018) 128(2):110–6. doi: 10.1080/00207454.2017.1367682
23. Yang QH, Zhang YH, Du SH, Wang YC, Fang Y, Wang XQ. Non-invasive brain stimulation for central neuropathic pain. Front Mol Neurosci (2022) 15:879909. doi: 10.3389/fnmol.2022.879909
24. Cruccu G, Garcia-Larrea L, Hansson P, Keindl M, Lefaucheur JP, Paulus W, et al. EAN guidelines on central neurostimulation therapy in chronic pain conditions. Eur J Neurol (2016) 23(10):1489–99. doi: 10.1111/ene.13103
25. Di Lazzaro V, Rothwell J, Capogna M. Noninvasive stimulation of the human brain: activation of multiple cortical circuits. Neuroscientist. (2018) 24(3):246–60. doi: 10.1177/1073858417717660
26. Nurmikko T, MacIver K, Bresnahan R, Hird E, Nelson A, Sacco P. Motor cortex reorganization and repetitive transcranial magnetic stimulation for pain-a methodological study. Neuromodulation. (2016) 19(7):669–78. doi: 10.1111/ner.12444
27. Lefaucheur JP, André-Obadia N, Antal A, Ayache SS, Baeken C, Benninger DH, et al. Evidence-based guidelines on the therapeutic use of repetitive transcranial magnetic stimulation (rTMS). Clin Neurophysiol (2014) 125(11):2150–206. doi: 10.1016/j.clinph.2014.05.021
28. Knotkova H, Hamani C, Sivanesan E, Le Beuffe MFE, Moon JY, Cohen SP, et al. Neuromodulation for chronic pain. Lancet. (2021) 397(10289):2111–24. doi: 10.1016/S0140-6736(21)00794-7
29. Cruccu G, Aziz TZ, Garcia-Larrea L, Hansson P, Jensen TS, Lefaucheur JP, et al. EFNS guidelines on neurostimulation therapy for neuropathic pain. Eur J Neurol (2007) 14(9):952–70. doi: 10.1111/j.1468-1331.2007.01916.x
30. Lefaucheur JP, Hatem S, Nineb A, Ménard-Lefaucheur I, Wendling S, Keravel Y, et al. Somatotopic organization of the analgesic effects of motor cortex rTMS in neuropathic pain. Neurology. (2006) 67(11):1998–2004. doi: 10.1212/01.wnl.0000247138.85330.88
31. Hasan M, Whiteley J, Bresnahan R, MacIver K, Sacco P, Das K, et al. Somatosensory change and pain relief induced by repetitive transcranial magnetic stimulation in patients with central poststroke pain. Neuromodulation. (2014) 17(8):731–6. doi: 10.1111/ner.12198
32. Yılmaz B, Kesikburun S, Yaşar E, Tan AK. The effect of repetitive transcranial magnetic stimulation on refractory neuropathic pain in spinal cord injury. J Spinal Cord Med (2014) 37(4):397–400. doi: 10.1179/2045772313Y.0000000172
33. Hosomi K, Sugiyama K, Nakamura Y, Shimokawa T, Oshino S, Goto Y, et al. A randomized controlled trial of 5 daily sessions and continuous trial of 4 weekly sessions of repetitive transcranial magnetic stimulation for neuropathic pain. Pain. (2020) 161(2):351–60. doi: 10.1097/j.pain.0000000000001712
34. Hodaj H, Payen JF, Hodaj E, Dumolard A, Maindet C, Cracowski JL, et al. Long-term treatment of chronic orofacial, pudendal, and central neuropathic limb pain with repetitive transcranial magnetic stimulation of the motor cortex. Clin neurophysiology: Off J Int Fed Clin Neurophysiology. (2020) 131(7):1423–32. doi: 10.1016/j.clinph.2020.03.022
35. Rossi S, Hallett M, Rossini PM, Pascual-Leone A. Safety, ethical considerations, and application guidelines for the use of transcranial magnetic stimulation in clinical practice and research. Clin neurophysiology: Off J Int Fed Clin Neurophysiology. (2009) 120(12):2008–39. doi: 10.1016/j.clinph.2009.08.016
36. Jin Y, Xing G, Li G, Wang A, Feng S, Tang Q, et al. High frequency repetitive transcranial magnetic stimulation therapy for chronic neuropathic pain: a meta-analysis. Pain physician. (2015) 18(6):E1029–46.
37. Quesada C, Pommier B, Fauchon C, Bradley C, Créac’h C, Murat M, et al. New procedure of high-frequency repetitive transcranial magnetic stimulation for central neuropathic pain: a placebo-controlled randomized crossover study. Pain. (2020) 161(4):718–28. doi: 10.1097/j.pain.0000000000001760
38. Hodaj H, Alibeu JP, Payen JF, Lefaucheur JP. Treatment of chronic facial pain including cluster headache by repetitive transcranial magnetic stimulation of the motor cortex with maintenance sessions: a naturalistic study. Brain stimulation. (2015) 8(4):801–7. doi: 10.1016/j.brs.2015.01.416
39. Lefaucheur JP, Aleman A, Baeken C, Benninger DH, Brunelin J, Di Lazzaro V, et al. Evidence-based guidelines on the therapeutic use of repetitive transcranial magnetic stimulation (rTMS): an update (2014-2018). Clin Neurophysiol (2020) 131(2):474–528. doi: 10.1016/j.clinph.2019.11.002
40. Cunningham AJ, Murray CA, O’Neill LA, Lynch MA, O’Connor JJ. Interleukin-1 beta (IL-1 beta) and tumour necrosis factor (TNF) inhibit long-term potentiation in the rat dentate gyrus in vitro. Neurosci Lett (1996) 203(1):17–20. doi: 10.1016/0304-3940(95)12252-4
41. Kitazawa M, Oddo S, Yamasaki TR, Green KN, LaFerla FM. Lipopolysaccharide-induced inflammation exacerbates tau pathology by a cyclin-dependent kinase 5-mediated pathway in a transgenic model of alzheimer’s disease. J Neurosci (2005) 25(39):8843–53. doi: 10.1523/JNEUROSCI.2868-05.2005
42. Micheau O, Tschopp J. Induction of TNF receptor I-mediated apoptosis via two sequential signaling complexes. Cell. (2003) 114(2):181–90. doi: 10.1016/S0092-8674(03)00521-X
43. Lyman M, Lloyd DG, Ji X, Vizcaychipi MP, Ma D. Neuroinflammation: the role and consequences. Neurosci Res (2014) 79:1–12. doi: 10.1016/j.neures.2013.10.004
44. Zelenka M, Schäfers M, Sommer C. Intraneural injection of interleukin-1beta and tumor necrosis factor-alpha into rat sciatic nerve at physiological doses induces signs of neuropathic pain. Pain. (2005) 116(3):257–63. doi: 10.1016/j.pain.2005.04.018
45. Clark AK, Old EA, Malcangio M. Neuropathic pain and cytokines: current perspectives. J Pain Res (2013) 6:803–14. doi: 10.2147/JPR.S53660
46. Zhao X, Li Y, Tian Q, Zhu B, Zhao Z. Repetitive transcranial magnetic stimulation increases serum brain-derived neurotrophic factor and decreases interleukin-1β and tumor necrosis factor-α in elderly patients with refractory depression. J Int Med Res (2019) 47(5):1848–55. doi: 10.1177/0300060518817417
47. Lee JY, Kim HS, Kim SH, Kim HS, Cho BP. Combination of human mesenchymal stem cells and repetitive transcranial magnetic stimulation enhances neurological recovery of 6-hydroxydopamine model of parkinsonian’s disease. Tissue Eng Regener Med (2020) 17(1):67–80. doi: 10.1007/s13770-019-00233-8
48. Yang L, Su Y, Guo F, Zhang H, Zhao Y, Huang Q, et al. Deep rTMS mitigates behavioral and neuropathologic anomalies in cuprizone-exposed mice through reducing microglial proinflammatory cytokines. Front Integr Neurosci (2020) 14:556839. doi: 10.3389/fnint.2020.556839
49. Toledo RS, Stein DJ, Sanches PRS, da Silva LS, Medeiros HR, Fregni F, et al. rTMS induces analgesia and modulates neuroinflammation and neuroplasticity in neuropathic pain model rats. Brain Res (2021) 1762:147427. doi: 10.1016/j.brainres.2021.147427
50. Li J, Wei GH, Huang H, Lan YP, Liu B, Liu H, et al. Nerve injury-related autoimmunity activation leads to chronic inflammation and chronic neuropathic pain. Anesthesiology. (2013) 118(2):416–29. doi: 10.1097/ALN.0b013e31827d4b82
51. Hanisch UK. Proteins in microglial activation–inputs and outputs by subsets. Curr Protein Pept Sci (2013) 14(1):3–15. doi: 10.2174/1389203711314010003
52. Ardalan M, Rafati AH, Nyengaard JR, Wegener G. Rapid antidepressant effect of ketamine correlates with astroglial plasticity in the hippocampus. Br J Pharmacol (2017) 174(6):483–92. doi: 10.1111/bph.13714
53. Liu Y, Zhou LJ, Wang J, Li D, Ren WJ, Peng J, et al. TNF-α differentially regulates synaptic plasticity in the hippocampus and spinal cord by microglia-dependent mechanisms after peripheral nerve injury. J Neurosci (2017) 37(4):871–81. doi: 10.1523/JNEUROSCI.2235-16.2016
54. Vanelderen P, Rouwette T, Kozicz T, Roubos E, Van Zundert J, Heylen R, et al. The role of brain-derived neurotrophic factor in different animal models of neuropathic pain. Eur J Pain. (2010) 14(5):473.e1–9. doi: 10.1016/j.ejpain.2009.09.006
55. Li QY, Chen SX, Liu JY, Yao PW, Duan YW, Li YY, et al. Neuroinflammation in the anterior cingulate cortex: the potential supraspinal mechanism underlying the mirror-image pain following motor fiber injury. J Neuroinflammation. (2022) 19(1):162. doi: 10.1186/s12974-022-02525-8
56. Toledo RS, Stein DJ, Stefani Sanches PR, de Souza A, da Silva LS, Medeiros HR, et al. Repetitive transcranial magnetic stimulation (rTMS) reverses the long-term memory impairment and the decrease of hippocampal interleukin-10 levels, both induced by neuropathic pain in rats. Neuroscience. (2021) 472:51–9. doi: 10.1016/j.neuroscience.2021.07.030
57. Kelly A, Lynch A, Vereker E, Nolan Y, Queenan P, Whittaker E, et al. The anti-inflammatory cytokine, interleukin (IL)-10, blocks the inhibitory effect of IL-1 beta on long term potentiation. A role JNK. J Biol Chem (2001) 276(49):45564–72. doi: 10.1074/jbc.M108757200
58. Lynch AM, Walsh C, Delaney A, Nolan Y, Campbell VA, Lynch MA. Lipopolysaccharide-induced increase in signalling in hippocampus is abrogated by IL-10–a role for IL-1 beta? J Neurochem (2004) 88(3):635–46. doi: 10.1046/j.1471-4159.2003.02157.x
59. Kiyota T, Ingraham KL, Swan RJ, Jacobsen MT, Andrews SJ, Ikezu T. AAV serotype 2/1-mediated gene delivery of anti-inflammatory interleukin-10 enhances neurogenesis and cognitive function in APP+PS1 mice. Gene Ther (2012) 19(7):724–33. doi: 10.1038/gt.2011.126
60. Hu Y, Zhu Y, Wen X, Zeng F, Feng Y, Xu Z, et al. Repetitive transcranial magnetic stimulation regulates neuroinflammation, relieves hyperalgesia and reverses despair-like behaviour in chronic constriction injury rats. Eur J Neurosci (2022) 56(6):4930–47. doi: 10.1111/ejn.15779
61. Hung AL, Lim M, Doshi TL. Targeting cytokines for treatment of neuropathic pain. Scand J Pain. (2017) 17:287–93. doi: 10.1016/j.sjpain.2017.08.002
62. Li JX. Pain and depression comorbidity: a preclinical perspective. Behav Brain Res (2015) 276:92–8. doi: 10.1016/j.bbr.2014.04.042
63. Chopra K, Arora V. An intricate relationship between pain and depression: clinical correlates, coactivation factors and therapeutic targets. Expert Opin Ther Targets. (2014) 18(2):159–76. doi: 10.1517/14728222.2014.855720
64. Larsson M, Broman J. Synaptic plasticity and pain: role of ionotropic glutamate receptors. Neuroscientist. (2011) 17(3):256–73. doi: 10.1177/1073858409349913
65. Castany S, Codony X, Zamanillo D, Merlos M, Verdú E, Boadas-Vaello P. Repeated sigma-1 receptor antagonist MR309 administration modulates central neuropathic pain development after spinal cord injury in mice. Front Pharmacol (2019) 10:222. doi: 10.3389/fphar.2019.00222
66. Norman GJ, Karelina K, Zhang N, Walton JC, Morris JS, Devries AC. Stress and IL-1beta contribute to the development of depressive-like behavior following peripheral nerve injury. Mol Psychiatry (2010) 15(4):404–14. doi: 10.1038/mp.2009.91
67. Wang HY, Crupi D, Liu J, Stucky A, Cruciata G, Di Rocco A, et al. Repetitive transcranial magnetic stimulation enhances BDNF-TrkB signaling in both brain and lymphocyte. J Neurosci (2011) 31(30):11044–54. doi: 10.1523/JNEUROSCI.2125-11.2011
68. Yang S, Chang MC. Effect of repetitive transcranial magnetic stimulation on pain management: a systematic narrative review. Front Neurol (2020) 11:114. doi: 10.3389/fneur.2020.00114
69. Silva GD, Lopes PS, Fonoff ET, Pagano RL. The spinal anti-inflammatory mechanism of motor cortex stimulation: cause of success and refractoriness in neuropathic pain? J Neuroinflamm (2015) 12:10. doi: 10.1186/s12974-014-0216-1
70. Kim JY, Choi GS, Cho YW, Cho H, Hwang SJ, Ahn SH. Attenuation of spinal cord injury-induced astroglial and microglial activation by repetitive transcranial magnetic stimulation in rats. J Korean Med Sci (2013) 28(2):295–9. doi: 10.3346/jkms.2013.28.2.295
71. Hains BC, Waxman SG. Activated microglia contribute to the maintenance of chronic pain after spinal cord injury. J Neurosci (2006) 26(16):4308–17. doi: 10.1523/JNEUROSCI.0003-06.2006
72. Colburn RW, Rickman AJ, DeLeo JA. The effect of site and type of nerve injury on spinal glial activation and neuropathic pain behavior. Exp Neurol (1999) 157(2):289–304. doi: 10.1006/exnr.1999.7065
73. Kim SJ, Park SM, Cho YW, Jung YJ, Lee DG, Jang SH, et al. Changes in expression of mRNA for interleukin-8 and effects of interleukin-8 receptor inhibitor in the spinal dorsal horn in a rat model of lumbar disc herniation. Spine (Phila Pa 1976). (2011) 36(25):2139–46. doi: 10.1097/BRS.0b013e31821945a3
74. Lindia JA, McGowan E, Jochnowitz N, Abbadie C. Induction of CX3CL1 expression in astrocytes and CX3CR1 in microglia in the spinal cord of a rat model of neuropathic pain. J Pain. (2005) 6(7):434–8. doi: 10.1016/j.jpain.2005.02.001
75. Park HW, Ahn SH, Kim SJ, Seo JM, Cho YW, Jang SH, et al. Changes in spinal cord expression of fractalkine and its receptor in a rat model of disc herniation by autologous nucleus pulposus. Spine (Phila Pa 1976). (2011) 36(12):E753–60. doi: 10.1097/BRS.0b013e3181ef610b
76. Sweitzer SM, Colburn RW, Rutkowski M, DeLeo JA. Acute peripheral inflammation induces moderate glial activation and spinal IL-1beta expression that correlates with pain behavior in the rat. Brain Res (1999) 829(1-2):209–21. doi: 10.1016/S0006-8993(99)01326-8
77. Luo J, Feng Y, Li M, Yin M, Qin F, Hu X. Repetitive transcranial magnetic stimulation improves neurological function and promotes the anti-inflammatory polarization of microglia in ischemic rats. Front Cell Neurosci (2022) 16:878345. doi: 10.3389/fncel.2022.878345
78. Hong Y, Liu Q, Peng M, Bai M, Li J, Sun R, et al. High-frequency repetitive transcranial magnetic stimulation improves functional recovery by inhibiting neurotoxic polarization of astrocytes in ischemic rats. J Neuroinflammation. (2020) 17(1):150. doi: 10.1186/s12974-020-01747-y
79. Aldinucci C, Palmi M, Sgaragli G, Benocci A, Meini A, Pessina F, et al. The effect of pulsed electromagnetic fields on the physiologic behaviour of a human astrocytoma cell line. Biochim Biophys Acta (2000) 1499(1-2):101–8. doi: 10.1016/S0167-4889(00)00111-7
80. Pessina GP, Aldinucci C, Palmi M, Sgaragli G, Benocci A, Meini A, et al. Pulsed electromagnetic fields affect the intracellular calcium concentrations in human astrocytoma cells. Bioelectromagnetics. (2001) 22(7):503–10. doi: 10.1002/bem.79
81. Fang ZY, Li Z, Xiong L, Huang J, Huang XL. Magnetic stimulation influences injury-induced migration of white matter astrocytes. Electromagn Biol Med (2010) 29(3):113–21. doi: 10.3109/15368378.2010.500568
82. Sasso V, Bisicchia E, Latini L, Ghiglieri V, Cacace F, Carola V, et al. Repetitive transcranial magnetic stimulation reduces remote apoptotic cell death and inflammation after focal brain injury. J Neuroinflammation. (2016) 13(1):150. doi: 10.1186/s12974-016-0616-5
83. Yang L, Wang SH, Hu Y, Sui YF, Peng T, Guo TC. Effects of repetitive transcranial magnetic stimulation on astrocytes proliferation and nNOS expression in neuropathic pain rats. Curr Med Sci (2018) 38(3):482–90. doi: 10.1007/s11596-018-1904-3
84. Tian JJ, Tan CY, Chen QY, Zhou Y, Qu ZW, Zhang M, et al. Upregulation of Nav1.7 by endogenous hydrogen sulfide contributes to maintenance of neuropathic pain. Int J Mol Med (2020) 46(2):782–94. doi: 10.3892/ijmm.2020.4611
85. Zhang Y, Wang K, Lin M, Li Q, Hong Y. Inhibition of morphine tolerance by MrgC receptor via modulation of interleukin-1β and matrix metalloproteinase 9 in dorsal root ganglia in rats. Eur J Pharmacol (2017) 815:10–7. doi: 10.1016/j.ejphar.2017.10.011
86. Sun J, Li N, Duan G, Liu Y, Guo S, Wang C, et al. Increased Na(v)1.7 expression in the dorsal root ganglion contributes to pain hypersensitivity after plantar incision in rats. Mol Pain (2018) 14:1744806918782323. doi: 10.1177/1744806918782323
87. Kawai H, Asaoka N, Miyake T, Nagayasu K, Nakagawa T, Shirakawa H, et al. Neurotropin inhibits neuronal activity through potentiation of sustained k(v) currents in primary cultured DRG neurons. J Pharmacol Sci (2018) 137(3):313–6. doi: 10.1016/j.jphs.2018.05.005
88. Neves AF, Farias FH, de Magalhães SF, Araldi D, Pagliusi M Jr., Tambeli CH, et al. Peripheral inflammatory hyperalgesia depends on P2X7 receptors in satellite glial cells. Front Physiol (2020) 11:473. doi: 10.3389/fphys.2020.00473
89. Takahashi N, Kikuchi S, Shubayev VI, Campana WM, Myers RR. TNF-alpha and phosphorylation of ERK in DRG and spinal cord: insights into mechanisms of sciatica. Spine (Phila Pa 1976). (2006) 31(5):523–9. doi: 10.1097/01.brs.0000201305.01522.17
90. Qian Y, Chao DS, Santillano DR, Cornwell TL, Nairn AC, Greengard P, et al. cGMP-dependent protein kinase in dorsal root ganglion: relationship with nitric oxide synthase and nociceptive neurons. J Neurosci (1996) 16(10):3130–8. doi: 10.1523/JNEUROSCI.16-10-03130.1996
91. Schmidt H, Werner M, Heppenstall PA, Henning M, Moré MI, Kühbandner S, et al. cGMP-mediated signaling via cGKIalpha is required for the guidance and connectivity of sensory axons. J Cell Biol (2002) 159(3):489–98. doi: 10.1083/jcb.200207058
92. Tegeder I, Del Turco D, Schmidtko A, Sausbier M, Feil R, Hofmann F, et al. Reduced inflammatory hyperalgesia with preservation of acute thermal nociception in mice lacking cGMP-dependent protein kinase I. Proc Natl Acad Sci U S A. (2004) 101(9):3253–7. doi: 10.1073/pnas.0304076101
93. Choi SR, Beitz AJ, Lee JH. Spinal nitric oxide synthase type II increases neurosteroid-metabolizing cytochrome P450c17 expression in a rodent model of neuropathic pain. Exp Neurobiol (2019) 28(4):516–28. doi: 10.5607/en.2019.28.4.516
94. Demir IE, Heinrich T, Carty DG, Saricaoglu ÖC, Klauss S, Teller S, et al. Targeting nNOS ameliorates the severe neuropathic pain due to chronic pancreatitis. EBioMedicine. (2019) 46:431–43. doi: 10.1016/j.ebiom.2019.07.055
95. Tolou-Dabbaghian B, Delphi L, Rezayof A. Blockade of NMDA receptors and nitric oxide synthesis potentiated morphine-induced anti-allodynia via attenuating pain-related amygdala pCREB/CREB signaling pathway. J Pain. (2019) 20(8):885–97. doi: 10.1016/j.jpain.2019.01.329
96. Dilley A, Richards N, Pulman KG, Bove GM. Disruption of fast axonal transport in the rat induces behavioral changes consistent with neuropathic pain. J Pain. (2013) 14(11):1437–49. doi: 10.1016/j.jpain.2013.07.005
97. Campana WM, Li X, Shubayev VI, Angert M, Cai K, Myers RR. Erythropoietin reduces schwann cell TNF-alpha, wallerian degeneration and pain-related behaviors after peripheral nerve injury. Eur J Neurosci (2006) 23(3):617–26. doi: 10.1111/j.1460-9568.2006.04606.x
98. Uçeyler N, Sommer C. Cytokine regulation in animal models of neuropathic pain and in human diseases. Neurosci Lett (2008) 437(3):194–8. doi: 10.1016/j.neulet.2008.03.050
99. Martini R, Willison H. Neuroinflammation in the peripheral nerve: cause, modulator, or bystander in peripheral neuropathies? Glia (2016) 64(4):475–86. doi: 10.1002/glia.22899
100. Hallett M, Di Iorio R, Rossini PM, Park JE, Chen R, Celnik P, et al. Contribution of transcranial magnetic stimulation to assessment of brain connectivity and networks. Clin Neurophysiol (2017) 128(11):2125–39. doi: 10.1016/j.clinph.2017.08.007
101. Kumru H, Albu S, Vidal J, Tormos JM. Effectiveness of repetitive trancranial or peripheral magnetic stimulation in neuropathic pain. Disabil Rehabil. (2017) 39(9):856–66. doi: 10.3109/09638288.2016.1170213
102. Behera D, Jacobs KE, Behera S, Rosenberg J, Biswal S. (18)F-FDG PET/MRI can be used to identify injured peripheral nerves in a model of neuropathic pain. J Nucl Med (2011) 52(8):1308–12. doi: 10.2967/jnumed.110.084731
103. Nam JW, Lee MJ, Kim HJ. Diagnostic efficacy of (18)F-FDG PET/MRI in peripheral nerve injury models. Neurochem Res (2019) 44(9):2092–102. doi: 10.1007/s11064-019-02846-w
104. Cywiak C, Ashbaugh RC, Metto AC, Udpa L, Qian C, Gilad AA, et al. Non-invasive neuromodulation using rTMS and the electromagnetic-perceptive gene (EPG) facilitates plasticity after nerve injury. Brain Stimul. (2020) 13(6):1774–83. doi: 10.1016/j.brs.2020.10.006
105. Hosomi K, Kishima H, Oshino S, Hirata M, Tani N, Maruo T, et al. Cortical excitability changes after high-frequency repetitive transcranial magnetic stimulation for central poststroke pain. Pain. (2013) 154(8):1352–7. doi: 10.1016/j.pain.2013.04.017
106. Matsumura Y, Hirayama T, Yamamoto T. Comparison between pharmacologic evaluation and repetitive transcranial magnetic stimulation-induced analgesia in poststroke pain patients. Neuromodulation. (2013) 16(4):349–54. doi: 10.1111/ner.12019
107. Kobayashi M, Fujimaki T, Mihara B, Ohira T. Repetitive transcranial magnetic stimulation once a week induces sustainable long-term relief of central poststroke pain. Neuromodulation. (2015) 18(4):249–54. doi: 10.1111/ner.12301
108. Sun X, Long H, Zhao C, Duan Q, Zhu H, Chen C, et al. Analgesia-enhancing effects of repetitive transcranial magnetic stimulation on neuropathic pain after spinal cord injury:An fNIRS study. Restor Neurol Neurosci (2019) 37(5):497–507. doi: 10.3233/RNN-190934
Keywords: neuropathic pain, repetitive transcranial magnetic stimulation, neuroinflammation, analgesic effect, analgesic mechanism
Citation: Bai Y-W, Yang Q-H, Chen P-J and Wang X-Q (2023) Repetitive transcranial magnetic stimulation regulates neuroinflammation in neuropathic pain. Front. Immunol. 14:1172293. doi: 10.3389/fimmu.2023.1172293
Received: 23 February 2023; Accepted: 12 April 2023;
Published: 25 April 2023.
Edited by:
Hideaki Obata, Saitama Medical University, JapanReviewed by:
Norikazu Kiguchi, Wakayama Medical University, JapanCopyright © 2023 Bai, Yang, Chen and Wang. This is an open-access article distributed under the terms of the Creative Commons Attribution License (CC BY). The use, distribution or reproduction in other forums is permitted, provided the original author(s) and the copyright owner(s) are credited and that the original publication in this journal is cited, in accordance with accepted academic practice. No use, distribution or reproduction is permitted which does not comply with these terms.
*Correspondence: Pei-Jie Chen, Y2hlbnBlaWppZUBzdXMuZXVkLmNu
†These authors have contributed equally to this work and share first authorship