- 1Laboratorio di Immunologia dei Tumori del Sangue (LITS), Centro Interdipartimentale di Biotecnologie Molecolari “Guido Tarone”, Dipartimento di Biotecnologie Molecolari e Scienze per la Salute, Università Degli Studi di Torino, Torino, Italy
- 2Struttura Complessa (SC) Ematologia, Azienda Ospedaliera (AO) S. Croce e Carle, Cuneo, Italy
In the last years, the tumor microenvironment (TME) has emerged as a promising target for therapeutic interventions in cancer. Cancer cells are highly dependent on the TME to growth and evade the immune system. Three major cell subpopulations are facing each other in the TME: cancer cells, immune suppressor cells, and immune effector cells. These interactions are influenced by the tumor stroma which is composed of extracellular matrix, bystander cells, cytokines, and soluble factors. The TME can be very different depending on the tissue where cancer arises as in solid tumors vs blood cancers. Several studies have shown correlations between the clinical outcome and specific patterns of TME immune cell infiltration. In the recent years, a growing body of evidence suggests that unconventional T cells like natural killer T (NKT) cells, mucosal-associated invariant T (MAIT) cells, and γδ T cells are key players in the protumor or antitumor TME commitment in solid tumors and blood cancers. In this review, we will focus on γδ T cells, especially Vγ9Vδ2 T cells, to discuss their peculiarities, pros, and cons as potential targets of therapeutic interventions in blood cancers.
Introduction
γδ T cells are equipped with a T-cell Receptor (TCR) composed of a γ-chain (TRG) and a δ-chain (TRD). The genes encoding TRG and TRD undergo somatic DNA recombination of variable (V), diversity (D, only in TRD) and joining (J) elements during γδ T cell maturation in the thymus (1). γδ TCR and αβ TCR are structurally similar and associated with the same subunits of the CD3 complex which, however, are arranged differently and characterized by unique glycosylation patterns and other minor peculiarities (2, 3). One major difference are the antigens recognized by αβ and γδ T cells and the modality of antigen recognition which is not dependent on the major histocompatibility complex (MHC) in γδ T cells (2, 4). This feature is particularly exciting from the perspective of using γδ T cells as a source for adoptive cell transfer (ACT) or chimeric antigen receptor (CAR)-T cells because MHC-independency reduces the risk of graft-versus-host disease (GvHD) and helps the development of “off-the shelf” cellular products (5).
In humans, γδ T cells represent 1-5% of blood circulating cells (6). Their development begins early during gestation (5-7 weeks), initially in the liver, and after 8 weeks of gestation also in the thymus (7). Later on, γδ T cells colonize predetermined mucosal and epithelial locations to contribute to tissue homeostasis and immune responses against pathogens (8).
Human γδ T cells can be divided in three main subsets: Vδ1+ cells, Vδ2+ cells and Vδ3+ cells (2). Vδ2+ T cells are the predominant γδ T-cell population in the PB of adult humans (9, 10). They are characterized by the expression of the semi-invariant Vγ9Vδ2 TCR made up of a public germline CDR3γ sequence and a more diverse CDR3δ sequence (11–14). Vδ1+ and Vδ3+ cells are commonly found in mucosal epithelial tissues, and in the liver, even if small amounts can also be detected in PB (2). In vitro, CD8+ Vδ1+ T cells which can recognize tumor-associate antigens in an MHC-dependent manner have been generated from human cord blood hematopoietic stem/progenitor cells (HSPC) using the OP9-DL system (15). The importance of γδ T cells in the clearance of pathogens (8, 16, 17) and cancer immunosurveillance (18–20) is very well acknowledged. However, γδ T cells can also negatively affect the outcome of immune responses to pathogens and tumor cells depending on the tissue microenvironment that they have colonized, the cytokines and soluble factors they are exposed to, and the multifaceted interactions engaged with bystander cells and the extracellular matrix (21–23). This functional plasticity can lead to the acquisition of regulatory functions in the tumor microenvironment (TME) leading to immune suppression and protumor functions. Accumulation of CD39+ γδ T cells has been reported in colorectal cancer (23), and interleukin (IL)-17 producing Vδ1+ T cells have been identified as major promoters of tumor progression and metastatization in humans (24–26). Regulatory γδ T cells have also been reported in blood cancers and associated with poor overall survival (27, 28). Fewer data are available about Vγ9Vδ2 T cells and other Vδ2- cells (29). Recently, we have reported that bone marrow (BM) Vγ9Vδ2 T cells in multiple myeloma (MM) patients are dysfunctional, but they do not exert suppressor functions and do not produce IL-17 (30), whereas Lo Presti et al. have reported IL-17 producing Vγ9Vδ2 T cells in the TME of patients with squamous cell carcinoma (31).
In this review, we will discuss the peculiarities and vulnerabilities of Vγ9Vδ2 T cells to behave as antitumor immune effector cells, and the pros and cons to build autologous or allogenic immune-based interventions on these cells.
Activation and functional characteristics of Vγ9Vδ2 T cells
Vγ9Vδ2 T cells can recognize supraphysiological concentrations of phosphoantigens (pAgs) produced by pathogens or eukaryotic cells via the mevalonate pathway (Mev) or Mev-independent pathways of isoprenoid biosynthesis (32). The Mev-independent pathways (MEP/DOXP or Rohmer pathway) are restricted to eubacteria, cyanobacteria, plants, and apicomplexan protozoa (33). The prototype pAg generated in the Mev pathway is isopentenyl pyrophosphate (IPP). IPP is over-produced by stressed cells and cancer cells and promotes the selective activation of Vγ9Vδ2 T cells (34). The mechanisms of pAgs recognition by Vγ9Vδ2 T cells are very different from the canonical MHC-antigen complex recognition by αβ T cells and not yet fully resolved. Three immunoglobulin superfamily members, butyrophilin 3A1 (BTN3A1), butyrophilin 3A2 (BTN3A2), and butyrophilin 2A1 (BTN2A1) are involved in pAgs presentation and Vγ9Vδ2 T-cell activation (8, 35–38). The intracellular 30.2 domain of BTN3A1 senses pAg accumulation in antigen presenting cells (APCs) or target cells (8, 36) and promotes an inside-out modification of the extracellular domains. Once modified, BTN3A1 is stabilized by BTN3A2 and binds to the Vδ2 and γ-chain TCR regions of Vγ9Vδ2 T cells. At the same time, BTN2A1 provides a costimulatory signal via interactions with BTN3A1 and the germline-encoded regions of the Vγ9 chain on the opposite TCR side (37–39).
BTN3A1 and BTN2A1 are also expressed on the cell surface of Vγ9Vδ2 T cells. This implies that Vγ9Vδ2 T cells can self-activate each other without the intervention of APCs or target cells if there are sufficient pAgs in the extracellular space that can be internalized by the ATP-binding cassette transporter A1 (ABCA1). Self-activation is associated with CD107a upregulation and increased interferon-γ (IFNγ) production, potentially leading to Vγ9Vδ2 T-cell fratricide (40, 41). This undesired side-effect can partially explain why Vγ9Vδ2 T-cell based immune interventions have fallen short of expectations in the clinical setting (41).
Aminobisphosphonates (NBP) like zoledronic acid (ZA), and alkylamines enhance the ability of APCs and cancer cells to activate Vγ9Vδ2 T cells by increasing the intracellular production and extracellular release of IPP via inhibition of the farnesyl diphosphate synthase in the Mev pathway (42–45). Vγ9Vδ2 cells can also be activated by natural killer (NK) receptors like the natural killer 2D receptor (NKG2D) and the DNA X accessory molecule 1 (DNAM-1). The former interacts with MICA, MICB, and ULBP1-4, while the latter interacts with Nectin-2 and PVR. These interactions contribute to the induction of cytotoxic responses and cytokine production (25). Vγ9Vδ2 T cells can also express NKp44 which is involved in cytotoxicity against myeloma cells lacking NKG2D ligands (46, 47). Other NK receptors, such as NKp30, NKp40 and NKp46 can also contribute to the antitumor functions of Vδ1 and Vδ2 T cells (32). Upon activation, Vγ9Vδ2 T cells can exert a wide range of functions typical of both adaptive and natural immunity, including cytolytic functions, chemokines and cytokines production. In addition, they can behave as cellular adjuvants to support antigen-specific immune responses mediated by B cells and MHC-restricted αβ T cells (2, 45, 48–52).
Vγ9Vδ2 T cells can also exert regulatory functions to terminate immune reactions and prevent autoimmunity via IL-10 production and the immune checkpoint (ICP) - immune checkpoint ligands (ICP-L) axes (43, 53).
Based on their maturation status, four distinct subsets of Vγ9Vδ2 T cells have been identified after pAgs stimulation (43). Naïve CD45RA+CD27+ Vγ9Vδ2 T cells produce low amount of IFNγ, and they can differentiate into CD45RA-CD27+ central memory (CM) Vγ9Vδ2 T cells with higher proliferation capacity after pAgs stimulation. CM cells can further differentiate into CD45RA-CD27- effector memory (EM) cells that produce high levels of IFNγ and tumor necrosis factor-α (TNFα) (54). EM cells or, alternatively, CM cells in the presence of IL-15, can differentiate into late effector memory CD45RA+CD27- T cells (TEMRA) characterized by high cytotoxic activity, low proliferative capacity, and modest IFNγ production (43, 54). TEMRA cells can be further divided in two subsets based on CD45RA expression levels: CD27-CD45RAhi and CD27-CD45RAint cells. The former are reminiscent of functionally exhausted cells, while the latter are the “classical” TEMRA cells mentioned above (55). The maturation process of Vγ9Vδ2 T cells is highly influenced by the microenvironment in which they are resident and the stimuli they are exposed to. In the presence of tumor cells, the maturation pathway can be redirected to immune senescence and/or functional exhaustion which are tumor permissive conditions (30).
Vγ9Vδ2 T cells in cancer: A delicate balance between antitumor and protumor functions
The antitumor activity of Vγ9Vδ2 T cells encompasses: 1) direct killing of cancer cells through granzyme B (GzmB) and perforin (Prf) secretion; 2) antibody-dependent cellular cytotoxicity (ADCC) dependent on CD16 expression; 3) Fas/FasL-mediated cell death; 4) production of cytokines like IFNγ and TNFα; 5) interactions with other TME-resident immune cells (25, 48, 56, 57). Vγ9Vδ2 T cells, can cross-present tumor antigens to αβ CD8+ T cells to boost antigen-specific IFNγ production and increase antitumor T-cell response (58). Vγ9Vδ2 T cells can also upregulate MHC and co-stimulatory molecules after in vitro IPP stimulation. This APCs-like phenotype allows Vγ9Vδ2 T cells to prime CD4+ T cells, shifting their polarization towards a Th1 antitumor profile (49). We and others have shown that Vγ9Vδ2 T cells can deliver co-stimulatory signals to dendritic cells (DCs) after in vitro ZA stimulation that increase the frequency of antigen-specific CD8+ αβ T cells and concurrently restrain the expansion of IL-2-dependent regulatory T cells (Tregs). Altogether, these data indicate that Vγ9Vδ2 T cells can behave as cellular adjuvants to rally a wide range of immune reactions against cancer cells (52, 59, 60) mediated by innate and adaptive immune effector cells, including B cells, neutrophils, and NK cells (57). Vγ9Vδ2 T cells can provide B-cell help to promote antibody production and immunoglobulin class switching (57, 61). IL-21 in combination with (E)-4-Hydroxy-3-methyl-but-2-enyl pyrophosphate (HMB-PP) can induce a TFH-like Vγ9Vδ2 T-cell differentiation leading to increased IgM and IgG production by B cells (61). Soluble factors released by activated Vγ9Vδ2 T cells trigger neutrophil migration, phagocytic ability and α-defensin release which can exert antitumor activity in the TME (62). IPP-activated Vγ9Vδ2 T cells upregulate CD137L that can engage CD137 on the surface of NK cells and enhance the cytotoxic antitumor activity against squamous cell carcinoma of head and neck and lymphoma cell lines (63).
Despite this wide array of direct and indirect antitumor properties, Vγ9Vδ2 T cells are very early targeted and neutralized by cancer cells, especially in the TME. In MM, BM Vγ9Vδ2 T cells are PD-1+ TIM-3+, and anergic to pAgs stimulation (30, 64). These dysfunctions are long-lasting and already detectable in monoclonal gammopathy of undetermined significance (MGUS) (64). PD-1+ BM MM Vγ9Vδ2 T cells combine phenotypic, functional, and TCR-associated alterations consistent with chronic exhaustion and immune senescence, not easily reversible by single or even by dual ICP blockade (30). Interestingly, ICP+ Vγ9Vδ2 T cells maintain the ability to produce IFNγ and to secrete GzmB and Prf in MM, acute myeloid leukemia (AML), and other cancers (30, 65, 66). It is unclear whether these cells are still able to provide some kind of immune surveillance in the TME, but the partial retention of immune effector functions suggests that their immunocompetence is not irreversibly lost, and hopefully recoverable by appropriate manipulation.
The functional plasticity of Vγ9Vδ2 T cells implies a constant risk of switching from antitumor to protumor function (25, 48). Depending on the cytokines they are exposed after activation, Vγ9Vδ2 T cells can polarize into Th1-like, Th2-like, Th17-like, TFH-like, Treg-like, TAPCS-like phenotypes (43, 67–69). The input to undertake one way of differentiation rather than another is also influenced by the tissue environment, including cancer cells. Similarly to what has been reported on total γδ T cells in breast, colon, and pancreatic cancer (21, 26, 56, 70), Th17-like Vγ9Vδ2 T cells with protumor functions have been identified in the TME and associated with a negative outcome in squamous cell carcinoma (31). In the presence of IL-21, Vγ9Vδ2 T cells can become CD73+ and suppress the antitumor activity of conventional T cells via the adenosine suppressive circuitry (67). PD-L1 upregulation in the presence of IPP and IL-15 is another potent immune suppressor mechanism operated by Vγ9Vδ2 T cells against αβ T cells (71, 72). CD86 can also be used by Vγ9Vδ2 T cells to suppress αβ T cells via CTLA-4 and restrain their antitumor activity (72).
Vγ9Vδ2 T cells, in turn, can become easy targets of immune suppressor cells like myeloid-derived suppressors cells (MDSC) or bone marrow stromal cells (BMSC) that are often increased in the TME and are PD-L1+, as we have recently shown in MM (64, 73). The supraphysiological IPP production and release by BMSC via ABCA-1 can also contribute to the functional exhaustion of Vγ9Vδ2 T cells in the TME of MM (30, 40).
Antitumor and protumor functions of Vγ9Vδ2 T cells are represented in Figure 1.
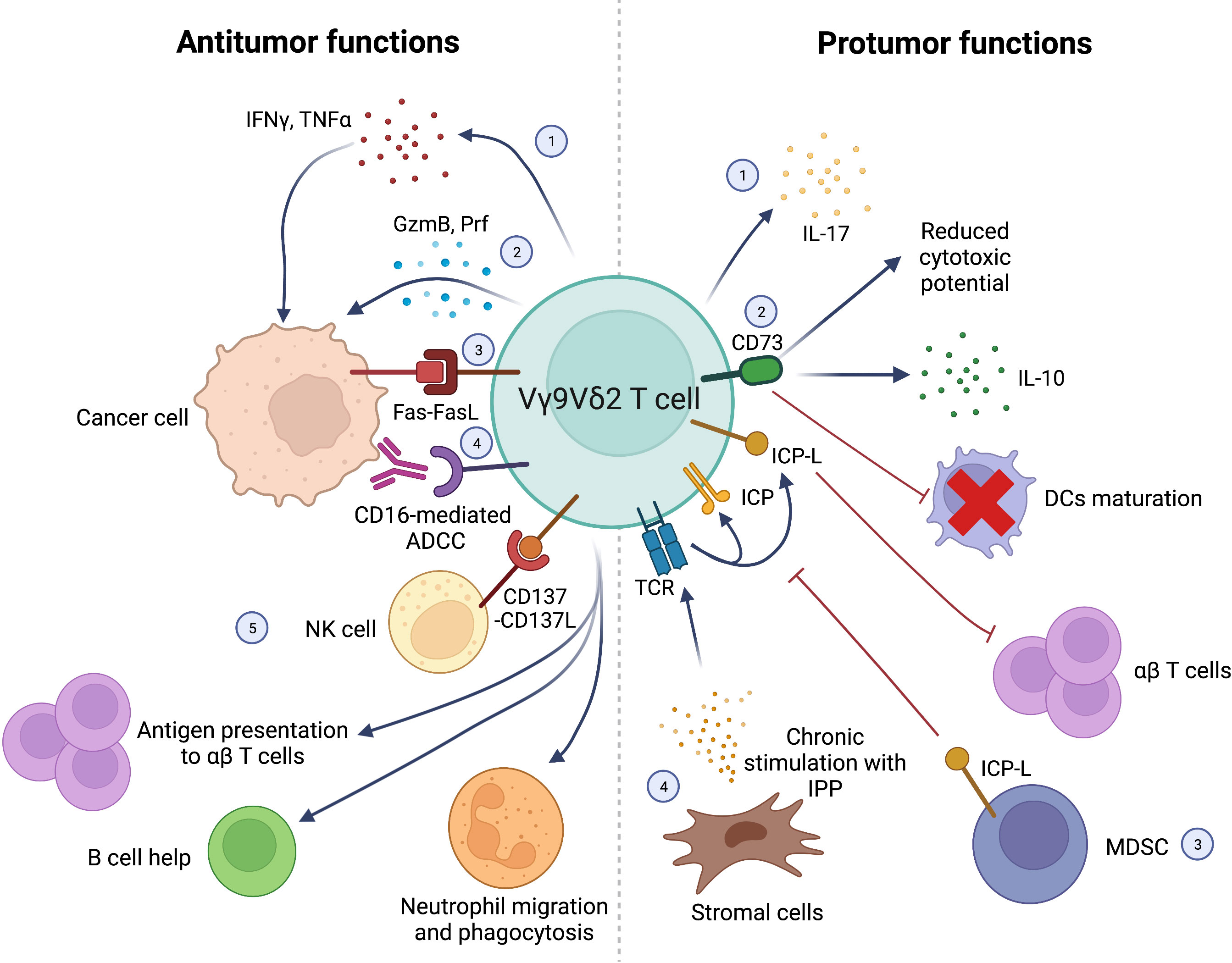
Figure 1 Schematic representation of antitumor (left) and protumor (right) functions of Vγ9Vδ2 T cells. Antitumor functions: 1) IFNγ and TNFα production; 2) direct killing of cancer cells via GzmB and Prf production; 3) cancer cell killing via Fas-FasL interactions; 4) CD16-mediated ADCC; 5) synergistic interactions with other immune cells in the TME: NK cells stimulation via CD137L expression; Ag presentation to αβ T cells; B cell help; stimulation of neutrophils’ migration, phagocytosis and α-defensin release. Protumor functions: 1) IL-17 production; 2) CD73 expression leading to IL-10 production, decreased Vγ9Vδ2 T-cell cytotoxic activity and impaired DCs maturation; 3) Negative regulation of Vγ9Vδ2 by T-cells MDSC expressing ICP-L (i.e. PD-L1); 4) Chronic stimulation of Vγ9Vδ2 TCR with IPP produced by stromal cells leading to exhaustion and suppression of αβ T cells’ function through ICP/ICP-L axis. Interferon γ (IFNγ), Tumor Necrosis Factor α (TNFα), Granzyme B (GzmB), Perforin (Prf), Antibody-dependent cell cytotoxicity (ADCC), Antigen (Ag), Interleukin-17 (IL-17), Interleukin-10 (IL-10), Dendritic cells (DCs), Myeloid-derived suppressor cells (MDSC), Immune Checkpont-Ligands (ICP-L), Programmed Death-Ligand 1 (PD-L1), Isopentenyl pyrophosphate (IPP), Immune Checkpoint (ICP). Created with BioRender.com.
Interestingly, blood cancer cells are more susceptible to the antitumor activity of Vγ9Vδ2 T cells than solid tumors (34, 56). Possible mechanisms are the enhanced Mev pathway activity and the increased expression of stress-induced self-ligands (34). Another major role is played by the TME which is very different in solid and blood cancer. The emergence of protumor Vγ9Vδ2 T cells has more often been reported in the former, whereas in the latter Vγ9Vδ2 T cells are mainly dysfunctional and chronically exhausted, but not fully differentiated into Vγ9Vδ2 T cells with protumor functions (30, 74–76).
Vγ9Vδ2 T cells as candidates for immunotherapy: A failed promise or inappropriate engagement?
The unique properties of Vγ9Vδ2 T cells have raised a great interest as potential candidates for immune-based interventions in solid tumors and blood cancers. Vγ9Vδ2 T-cell activation can be induced by a wide array of ligands making possible to target cancer cells devoid of specific tumor-associated antigens (TAA) or tumors with a limited mutational burden. Moreover, a broad antitumor reactivity could prevent the emergence of tumor variants leading to immune escape and tumor relapse (77).
MHC independency is another major feature making Vγ9Vδ2 T cells safer effector cells than αβ T cells in the context of allogenic hematopoietic stem-cell transplantation (allo-HCT) or other mismatched adoptive immunotherapy approaches. Vγ9Vδ2 T cells can exert effective graft-versus-tumor (GvT) activity with minimal GvHD activity which still is a major cause of early and late morbidity and mortality after allo-HCT (78). MHC-independent recognition of TAA should also limit the ability of cancer cells to evade immune recognition via MHC down-regulation (79).
The frequency of Vγ9Vδ2 T cells in the PB is low, but still significantly higher than any other MHC-restricted TAA-specific αβ T cells, and pAgs stimulation is a polyclonal stimulation recruiting all Vγ9Vδ2 T cells and not only selected clonal or subclonal populations. MHC-independency gives the possibility to develop off-the-shelf cell products from healthy donors bypassing both the time-consuming and expensive manufacturing of personalized cell products, and the immune dysfunctions affecting Vγ9Vδ2 T cells from cancer patients. Allogeneic and haploidentical Vγ9Vδ2 T cells have already been used in solid tumors and hematological malignancies without major adverse effects (80–84). Burnham et al. have shown that Vγ9Vδ2 T cells from multiple donors can be mixed and stimulated with ZA and IL-2 after αβ T-cell depletion without inducing fratricide or affecting their expansion and functional activation (85). Multidonor preparations could circumvent the risk to produce inadequate numbers of activated Vγ9Vδ2 T cells from healthy donors who are poor responders to pAg stimulation (approximately 5-10%). However, safety of multidonor infusions has not been tested in the immunotherapy setting, with the exception of cord blood cells, and the risk of uncontrolled alloreactivity remains a major concern (85). Lastly, pAgs-activated Vγ9Vδ2 T cells have been shown in vitro to behave as cellular adjuvants with the ability to engage immune effector cells of adaptive immunity and boost their antitumor responses (52, 57, 58, 60).
Despite these excellent premises, Vγ9Vδ2 T-cell based immune interventions have not hit the target. Early approaches have used NPB like pamidronate and ZA to induce Vγ9Vδ2 T-cell activation in vivo followed by IL-2 to support proliferation and expansion. Synthetic pAgs like bromohydrin pyrophosphate (BrHPP) and 2-methyl-3-butenyl-1-pyrophosphate (2M3B1PP) have been produced to increase the affinity for Vγ9Vδ2 T cells and extend their half-life after in vivo injection. Synthetic pAgs have been associated in vivo with monoclonal antibodies (mAbs) like rituximab, alemtuzumab, and obinutuzumab to boost ADCC in B-cell malignancies, based on the in vitro findings that pAgs-activated Vγ9Vδ2 T cells upregulate FcγR expression (86–88).
Early approaches of adoptive immunotherapy have also relied on the combination of pAgs and IL-2 to induce the ex-vivo activation of autologous Vγ9Vδ2 T cells. This approach has been tested in MM showing minimal toxicity, but unsatisfactory clinical results (89). The adjuvant properties of Vγ9Vδ2 T cells and their capacity to promote the activation of tumor-specific MHC-restricted αβ T cells has been investigated in a small number of elderly AML patients. These patients have been treated with DCs co-pulsed with WT1 peptide and ZA with some evidences of clinical benefit (90–92).
In conclusion, Vγ9Vδ2 T-cell based immunotherapy has proven safe and well tolerated in blood cancers, but unable to achieve deep and long-lasting responses (32, 93, 94). Failing clinical expectations has stimulated further research to understand the mechanisms exploited by tumor cells to escape Vγ9Vδ2 T-cell recognition and killing, especially in the TME (95, 96), and which strategies are worth investigating to empower their antitumor activity.
A critical point is the immune fitness of Vγ9Vδ2 T cells in cancer patients. We and others have shown that about 50% of PB Vγ9Vδ2 T cells from Chronic Lymphocytic Leukemia (CLL), MM, and other blood cancer patients are unable to respond to pAgs stimulation (97, 98). Naïve/CM/EM/TEMRA subset redistribution, ICP upregulation, immune senescence, and functional exhaustion due to chronic stimulation are some of the mechanisms responsible for Vγ9Vδ2 T-cell dysfunctions (30, 64). Unique to Vγ9Vδ2 T cells is the chronic stimulation operated by the supra-physiological IPP concentrations that are released in the TME by BMSC, and to a lower extent by myeloma cells (40). At the same time, the supra-physiological IPP concentrations can license the suppressor activity of Vγ9Vδ2 T cells restraining the antitumor activity of conventional αβ T cells via the PD-1/PD-L1 axis (71).
Interestingly, we have shown that Vγ9Vδ2 T-cell dysfunctions in the TME of MM patients are highly persistent and not reverted even in the remission phase when myeloma cells have disappeared (64). One reason is that the TME remains strongly committed to immune suppression as shown by the persistence of high numbers of PD-L1+ MDSC, PD-L1+ BMSC, and PD-L1+ endothelial cells (EC). Moreover, the disease status strongly influences the reactivity of BM MM Vγ9Vδ2 T cells to pAgs stimulation and the response to ICP blockade. At diagnosis, the combination of PD-1 and TIM-3 blockade allows a partial recovery of Vγ9Vδ2 T-cell immune effector functions; in the remission phase, single PD-1 blockade is moderately effective, whereas PD-1 and LAG-3 blockade is the only combination to be minimally effective in relapsed MM (30).
These data indicate that TME-resident Vγ9Vδ2 T cells are probably not the better targets for cell-based immune interventions in the absence of appropriate ex-vivo or in vivo manipulation correcting their dysfunctions. This is an interesting difference with tumor-infiltrating lymphocytes (TIL) which have been deemed to be very well-suitable for cellular immunotherapy. The assumption is that, at least in solid tumors, tumor-reactive clones have already been primed in the TME and they can be recruited more effectively against cancer cells (99). Moreover, frequency of TIL is much higher than that of γδ T cells in the TME facilitating their selective isolation and expansion (100).
A possible alternative to TME-resident Vγ9Vδ2 T cells is the in vivo or ex-vivo recruitment of circulating Vγ9Vδ2 T cells. Side-by-side comparison of PB and BM Vγ9Vδ2 T cells in MM patients has shown that the former are functionally preserved slightly better than the latter. We and others have shown that approximately 50% of MM and CLL patients retain PB Vγ9Vδ2 T cells that can be stimulated by pAgs (97, 98). Interestingly, in the others the anergy can be reverted with ZA-stimulated DCs that provide huge quantities of IPP and costimulatory signals (45, 97, 101). In CLL, pretreatment of PB Vγ9Vδ2 T cells with ibrutinib promotes a Th1 differentiation with enhanced antitumor activity, probably mediated by ITK inhibition as previously reported in conventional αβ T cells (101).
The use of PB Vγ9Vδ2 T cells is not devoid of drawbacks. One is the progressive decline in the capacity to respond to reiterated ZA stimulations as shown in MM patients after autologous stem cell transplantation (102), and pediatric acute leukemia patients receiving haploidentical αβ T-cell depleted stem cell transplantation (103). Another critical aspect is the inadvertent expansion of CD4+ T cells with a regulatory phenotype, as shown in neuroblastoma patients treated with ZA+IL-2 to intentionally activate Vγ9Vδ2 T cells in vivo (104).
Vγ9Vδ2 T-cell MHC independency gives the possibility to use allogeneic cells from the PB of healthy donors (105). Haploidentical γδ T cells have been infused in 4 patients with refractory hematological malignancies followed by in vivo stimulation with ZA and IL-2. None of the patients suffered from acute or chronic GvHD providing the proof in principle that allogeneic Vγ9Vδ2 T cells can safely be transferred and stimulated in vivo without inducing any undesired alloreactivity (82). These preliminary data have been validated in a large series of patients with advanced stage liver and lung cancer patients who received allogeneic Vγ9Vδ2 T cells without any significant adverse effects (e.g., immune rejection, cytokine storm, or GvHD effects) (81).
Although very exciting, also the use of Vγ9Vδ2 T cells from healthy donors is not exempt from disadvantages and pitfalls. One is the unexpected induction of immune suppressive activity against conventional αβ T cells after repeated pAgs stimulation (71). Another pitfalls are the unpredictable consequences of transferring Vγ9Vδ2 T cells which have been forced to respond to pAgs via noncanonical stimulation. For example, IL-21 has been reported to promote the expansion of Vγ9Vδ2 T cells from non-responder donors after ZA stimulation (85). Unfortunately, IL-21 can also induce Vγ9Vδ2 T cells with immune suppressive and protumor functions exerted via the CD73/adenosine-dependent circuit (67).
Altogether, these data indicate that both TME-resident and PB Vγ9Vδ2 T cells are very sensitive to stimuli delivered by TME, cytokines, and pAgs. Their functional plasticity is a great plus, but at the same time a great risk to inadvertently induce an undesired protumor activity if not properly managed (30, 106).
Strategies to bring Vγ9Vδ2 T-cell immune interventions to prime time
Over the last few years, we have seen an enormous acceleration in the knowledge of immune escape mechanisms together with great advances in the design of therapeutic mAbs, and the development of genetically engineered immune effector cells. These very exciting progresses are revolutionizing cancer immunotherapy including Vδ1 and Vγ9Vδ2 T-cell based approaches (94, 107).
Several approaches are under preclinical or clinical investigation to rescue the immune fitness of Vγ9Vδ2 T cells in cancer patients. Anti-ICP/ICP-L mAbs have been used in vitro to improve pAgs reactivity and immune effector functions of TME-resident Vγ9Vδ2 T cells in MM (30, 64), AML (65) and follicular lymphoma (FL) (108). The agonistic humanized anti-BTN3A mAb ICT01 is under investigation in advanced-stage solid tumors and hematological malignancies (109). Bispecific T-cell engagers antibodies (BiTe) are also under investigation to redirect cytotoxic Vγ9Vδ2 T-cell activity against cancer cells. The bispecific Vγ9/CD123 antibody has been shown to recruit and redirect Vγ9Vδ2 T cells against autologous AML blasts in vitro and in a xenograft mouse model (110). Similar results have been reproduced in vitro and in a xenograft mouse model with the bispecific Vγ9Vδ2/CD40 antibody in CLL and MM patients (111). CD1d is another tumor-associated antigen which can be targeted in CLL with a CD1d-specific Vγ9Vδ2-T cell engager made by single-domain antibodies (VHH). Interestingly, this bispecific VHH does not affect pAg reactivity giving the possibility to boost the antitumor activity of Vγ9Vδ2 T cells with ZA (112). Van Diest et al. have developed a bispecific molecule which exploits the natural predisposition of Vγ9Vδ2 T cells to recognize cancer cells by linking the extracellular domains of tumor reactive Vγ9Vδ2 TCR to a CD3-binding moiety. This bispecific molecule confers to conventional αβ T cells the capacity to recognize cancer cells via pAgs without the limitations imposed by MHC restriction and/or MHC downregulation (113).
A great effort is also ongoing to optimize the use of Vγ9Vδ2 T cells from healthy donors. In this case, strategies are dedicated to improve the efficacy of in vitro expansion protocols and to reinforce the capacity of Vγ9Vδ2 T cells to survive in vivo and to exert a prolonged antitumor activity. One area of research is focused on the discovery of novel NBP and synergistic interactions with other compounds. Tetrakis-pivaloyloxymethyl 2-(thiazole-2-ylamino) ethylidene-1,1-bisphosphonate (PTA) is a novel bisphosphonate prodrug which activates Vγ9Vδ2 T cells more efficiently than ZA (114), while vitamin C and its derivatives can enhance the activation and differentiation of human Vγ9Vδ2 T cells (115). A wise and careful selection of cytokines is also critical to promote the expansion of antitumor Vγ9Vδ2 T cells, and not the undesired expansion of Vγ9Vδ2 T cells with protumor or immune suppressor functions (85, 116).
The use of feeder cells is another workable tool to improve the efficacy of in vitro Vγ9Vδ2 T-cell expansion protocols (117–120). Side-by-side comparison of ZA + IL-2 versus K562-based artificial antigen-presenting cells (aAPCs) has shown in mouse models that the latter induces Vγ9Vδ2 T cells with stronger antitumor activity and enhanced capacity to survive in vivo (118). However, the superiority of aAPCs is challenged by the risk to induce an excessive IL-17A release leading to the differentiation of protumor Vγ9Vδ2 T cells (118, 121). Costimulation with ZA + IL-2 in addition to aAPCs can overcome this undesired bias and support the expansion of large numbers of memory Vγ9Vδ2T cells with low ICP expression that are prone to persist in vivo after infusion (119). This approach has been improved by introducing an intermediate step to remove αβ T cells in between the first stimulation with ZA + IL-2 and the second one with aAPCs and ZA + IL-2. This strategy allows the manufacturing and expansion from healthy donors of huge numbers of highly pure Vγ9Vδ2 T cells (117). The cytotoxic activity of adoptively transferred Vγ9Vδ2 T cells can be strengthened with mAbs to relieve ICP/ICP-L-dependent immune suppression (122, 123), and/or with agonistic anti-BTN3A 20.1 mAb or BiTes to boost antitumor immune effector functions (124).
Alternative strategies to potentiate antitumor effector functions of Vγ9Vδ2 T cells take advantage of their ability to recognize stress-induced self-ligands via killer activating receptors (KAR) like NKG2D. This ability is counterbalanced by the expression of killer inhibitory receptors (KIR) (34), highlighting the importance to develop strategies that upregulate KAR and/or downregulate KIR in Vγ9Vδ2 T cells. Attempts to tilt the balance in favor of KAR range from nanobiomaterial-based strategy to conventional drugs. Lin et al. have shown in vitro that chitosan nanoparticles enhance Vγ9Vδ2 T-cell antitumor functions by upregulating NKG2D, CD56, FasL, and Prf secretion (125). Upregulation of NKG2D-ligands (NKG2D-L) in cancer cells can be a complementary strategy. Conventional drugs like temozolomide, doxorubicin, and 5-fluorouracyl can sensitize cancer cells from solid tumors to Vγ9Vδ2 T cells by inducing the upregulation of Fas, TRAIL-R1, and TRAL-R2 that are recognized by Vγ9Vδ2 T cells via NKG2D and TRAIL (126, 127). These results have been reproduced with bortezomib in AML and acute T-cell lymphoblastic leukemia. Story et al. have shown that bortezomib enhances the recognition and killing of leukemia cells by ex-vivo activated Vγ9Vδ2 T cells from healthy donors by increasing NKG2D/NKG2D-L interactions (128). Unfortunately, these drugs can also be toxic to Vγ9Vδ2 T cells. The easiest way to skip this inconvenience is to give chemotherapy before Vγ9Vδ2 T-cell activation in vivo or before infusion of ex-vivo activated Vγ9Vδ2 T cells (127). A more cumbersome approach is to genetically engineer Vγ9Vδ2 T cells to confer resistance to cytotoxic drug (126). The extracellular release of NKG2D-L is another mechanism exploited by cancer cells to elude NKG2D-dependent immune surveillance, especially after exposure to cytotoxic drugs. Prevention of NKG2D-L shedding is another strategy that can be used to improve the efficacy of combinations with cytotoxic drugs (129).
The immune adjuvant properties of Vγ9Vδ2 T cells are also of renewed interest. Early studies have focused on their ability to boost MHC-restricted antitumor immune responses mediated by conventional CD8+ T cells (92). More recently, tumor cell/Vγ9Vδ2 T-cell fusions have been developed to mimic tumor cell/DC fusions already tested in MM and AML (130–132). In this approach, DCs are replaced by pAg-activated Vγ9Vδ2 T cells to combine their abilities to support adaptive immune responses and to exert antitumor activity, a plus compared with DCs which lack any direct antitumor activity. Wang et al. have validated this approach in vitro by generating osteosarcoma/Vγ9Vδ2 T-cell fusions that induce cytokines production and support antitumor immune responses mediated by conventional αβ T cells (133).
Sharing innate-like and adaptive-like immune functions makes Vγ9Vδ2 T cells very attractive candidates for genetic engineering (134). Vγ9Vδ2 T cells have successfully been armed with CAR to target the B-cell Maturation Antigen (BCMA) in MM and CD123 in AML (135, 136). Interestingly, in vitro data and in vivo mouse models have shown that, unlike conventional anti-CD19 CAR-T cells, ZA-stimulated anti-CD19 Vγ9Vδ2 CAR-T cells from healthy donors can target both CD19+ and CD19- allogeneic leukemia cells via the non-specific MHC-independent cytotoxic activity elicited by pAgs stimulation (137). It is worth investigating whether the retained ability to target CD19- leukemic cells can be exploited to prevent the disease relapse observed in patients treated with conventional anti-CD19 CAR-T cells. In addition, CAR-transduced Vδ2 T cells do not lose their property to behave as professional APCs and to cross-present processed peptides to αβ T cells (138).
ZA-stimulated Vγ9Vδ2 T cells are also excellent candidates for subsequent RNA-transfection with tumor-specific TCRs or CARs (139). Likewise, αβ T cells can be engineered to express γδ TCRs with high capacity to sense BTN3A1 and other conformational changes induced by intracellular pAgs accumulation in tumor cells (140). γδ TCR chains are very strong competitors of αβ TCR chains for the assembly of the TCR/CD3 complex (141) preventing the formation of αβ/γδ heterodimers and limiting the expression of endogenous αβ TCRs (142). The availability of GMP-grade anti-αβ TCR beads gives the possibility to deplete non- and poorly-engineered T cells yielding to a population of untouched engineered immune cells with high purity and substantially reduced “off-target” effects (143, 144). These T cells engineered to express a defined γδ T cell receptor (TEGs) have been shown to limit leukemic cell growth in vitro (140) and to recognize and kill myeloma cells in a 3D model (145). In addition, CD4+ Vγ9Vδ2 TCR-transduced αβ T cells retained the ability to induce DC maturation (140). The high affinity γ9δ2TCR clone 5 has demonstrated to be effective against AML blasts in PD-X models (146) and has been selected within the TEG format as a clinical candidate (TEG001) for a phase I clinical trial in patients with relapsed and refractory AML and MM (NTR https://www.trialregister.nl/trial/6357).
A side-by-side comparison of conventional αβ T cells and Vγ9Vδ2 T cells transduced with TCRs or CARs to target melanoma cells has shown similar antigen-specific cytotoxic activity, but the latter retain also their intrinsic ability to lyse MHC-deficient cells. Moreover, the cytokines pattern released by transduced Vγ9Vδ2 T cells predicts a lower risk of cytokine release syndrome and autoimmunity compared with transduced αβ T cells (139). Lastly, Vγ9Vδ2 T cells have been transfected with NKT cell-derived TCR to create bi-potential innate lymphocytes combining NKT and Vγ9Vδ2 effector functions including cytotoxicity against glycolipid-expressing target cells and K562 cells (147). Saura-Esteller et al. and Mensurado et al. have recently reviewed the clinical studies exploiting BiTes and engineered Vγ9Vδ2 T cells in cancer immunotherapy (94, 148).
Vγ9Vδ2 T-cell-based immunotherapy, like any other immunotherapy, can benefit from interventions shaping the TME to meet the metabolic requirements of immune effector cells at the expense of immune suppressor cells and cancer cells. In mouse cancer models, Lopes et al. have shown that protumor (IL-17+) and antitumor (IFNγ) γδ T cells are characterized by distinct metabolic profiles: the former require mitochondrial metabolism, whereas the latter are almost exclusively glycolytic. As a consequence, antitumor activity of IFNγ+ γδ T cells can be boosted by glucose, whereas protumor activity of IL-17+ γδ T cells can be reinforced or weakened by regulating lipid metabolism (149). Indoleamine 2,3-dioxygenase 1 (IDO1) inhibition is another metabolic approach promoting Vγ9Vδ2 T-cell cytotoxicity against human breast cancer cells and pancreatic ductal adenocarcinoma (PDAC) cells by enhancing perforin production (150), degranulation, and cytokine production (151). The cytotoxic activity promoted by IDO inhibition can be further enhanced with bispecific antibodies targeting Vγ9Vδ2 T cells and PDAC cells (151).
Hypoxia is a metabolic TME alteration compromising the cytotoxic activity Vγ9Vδ2 T cells and promoting IL-17 production, and CD8+ T-cell inhibition via the PD-1/PD-L1 axis (152). In brain tumors, it has been shown that metformin alleviates tumor hypoxia and reinvigorates the antitumor function of γδ T cells by inducing NKG2D upregulation (20). Arginase I inhibition is another metabolic approach that can indirectly promote the antitumor activity of Vγ9Vδ2 T cells by restraining the suppressor activity of MDSC (73, 153). We have recently reviewed the role of metabolic checkpoints compromising the immune competence of Vγ9Vδ2 T cells in MM, and the possible interventions to recover their antitumor activity (154).
Vγ9Vδ2 T cell-based immunotherapy can also be enhanced by increasing tumor sensitivity and immunogenicity. Chemotherapeutic compounds (i.e. doxorubicin and oxaliplatin), proteasome inhibitors and immunomodulatory drugs (IMiDs) can induce immunogenic cell death (ICD) triggering adaptive immune responses through a set of danger signals (155). Combinatorial approaches with ICD-inducers can facilitate Vγ9Vδ2 T-cell recruitment and cytotoxic activity (127, 156). Since accelerated Mev-pathway affects the translocation on the cell surface of Calreticulin (CRT), an hallmark of ICD, NBP-mediated interruption of Mev-pathway could be also an effective strategy to promote the sensitivity of cancer cells to ICD (157).
Figure 2 summarizes the in vivo and ex-vivo strategies currently under investigation to recover and fully exploit the antitumor activity of autologous and/or allogeneic Vγ9Vδ2 T cells.
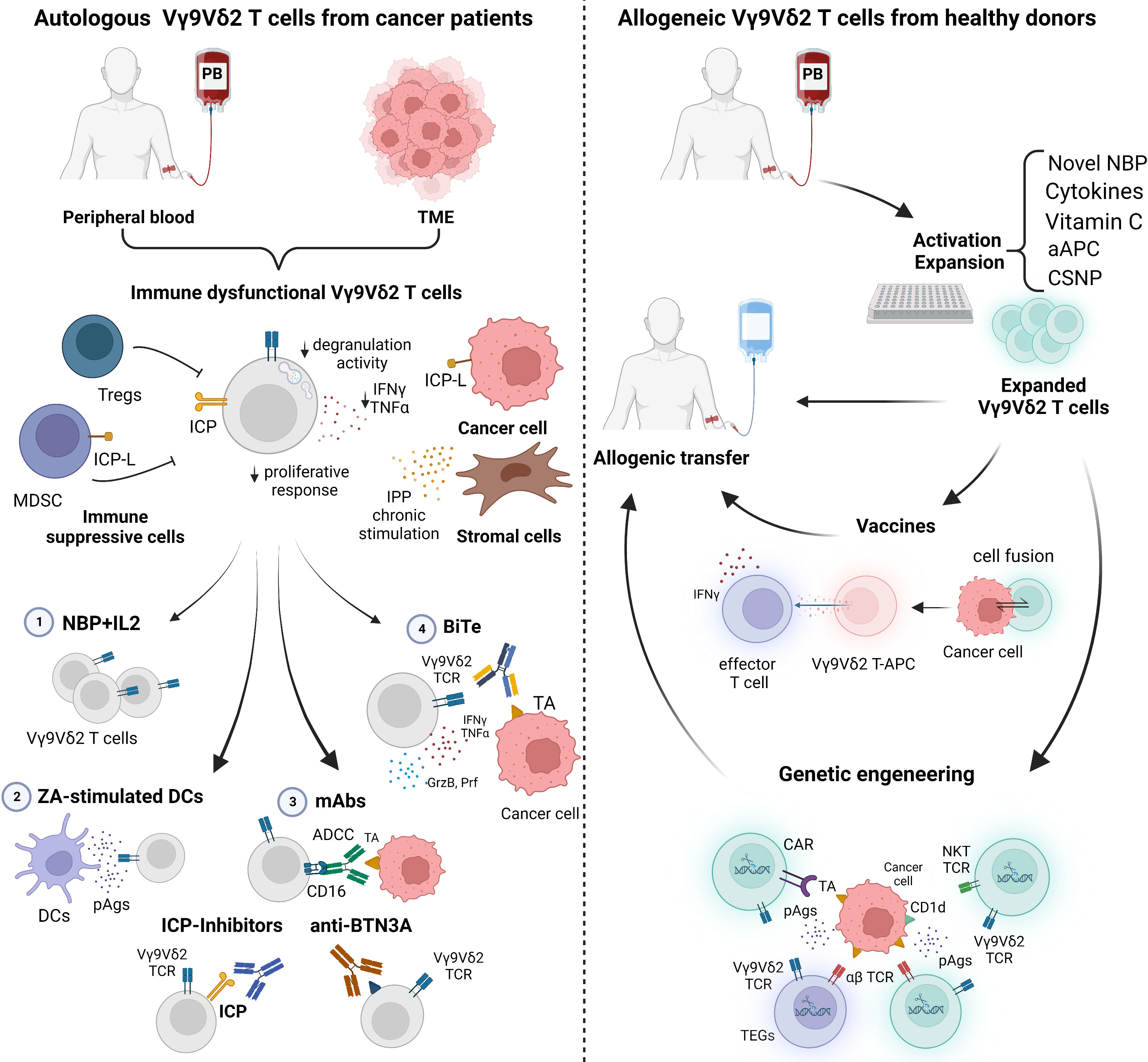
Figure 2 Current strategies to manipulate autologous and allogeneic Vγ9Vδ2 T cells for immunotherapy. Left panel: The immune fitness of patient-derived Vγ9Vδ2 T cells is compromised. Tumor microenvironment (TME)-resident and peripheral blood (PB) Vγ9Vδ2 T cells are characterized by immune checkpoint (ICP) expression, low proliferative response, decreased cytokine production (IFNγ and TNFα), and degranulation activity. Conventional approaches to rescue Vγ9Vδ2 T cells with 1) NBP+IL2 administration can be implemented with 2) ZA-stimulated dendritic cells (DCs) to enhance the amount of phosphoantigens (pAgs) locally available; 3) monoclonal antibodies (mAbs) to boost ADCC, to block ICP/ICP-L interactions, or to target BTN3A; 4) bispecific antibodies (BiTes). Right panel: Vγ9Vδ2 T cells from PB of healthy donors (Ctrl) can be manipulated in vitro for allogenic use. Novel NBP, selected cytokines, vitamin C, artificial antigen presenting cells (aAPCs) or chitosan nanoparticles (CSNP) can be used to improve Vγ9Vδ2 T-cell expansion and activation. Expanded Vγ9Vδ2 T cells can be used for vaccination or genetic engineering. Created with BioRender.com.
Conclusions
In conclusion, Vγ9Vδ2 T cells are very attractive candidates for cell-based immunotherapy in blood cancers. However, Vγ9Vδ2 T cells are also very sensitive to the TME and very easily reprogrammable to exert protumor functions or to undergo functional exhaustion and/or immune senescence. To fully exploit their unique antitumor properties, it is mandatory to protect Vγ9Vδ2 T cells from the pernicious influence operated by the TME and to fully recover their immune competence status.
Author contributions
CG, FA and MM contributed to the writing of the manuscript, CG and FA designed the figures, MM revised the manuscript. All authors contributed to the article and approved the submitted version.
Funding
This study received funding from the Italian Association for Cancer Research (AIRC) (IG21744 to MM), Associazione Italiana contro le Leucemie-Linfomi e Mielomi ONLUS (AIL) (Sezione Paolo Rubino di Cuneo) (MM, FA), and Sanofi (Research-to-Care OncoHematology). The funders had no role in the study design, data collection and analysis, decision to publish, or preparation of the manuscript.
Conflict of interest
MM reports advisory boards for AbbVie, Janssen-Cilag, Sanofi, and research funding from Sanofi.
The remaining authors declare that the research was conducted in the absence of any commercial or financial relationships that could be constructed as a potential conflict of interest.
Publisher’s note
All claims expressed in this article are solely those of the authors and do not necessarily represent those of their affiliated organizations, or those of the publisher, the editors and the reviewers. Any product that may be evaluated in this article, or claim that may be made by its manufacturer, is not guaranteed or endorsed by the publisher.
Abbreviations
ACT, Adoptive Cell Transfer; ABCA1, ATP-binding cassette transporter A1; ADCC, Antibody-dependent cellular cytotoxicity; Allo-HCT, allogenic hematopoietic stem-cell transplantation; AML, Acute myeloid leukemia; APC, Antigen presenting cells; BCMA, B-cell Maturation Antigen; BiTe, Bispecific T-cell engagers antibodies; BM, Bone marrow; BMSC, BM-derived stromal cells; Bregs, Regulatory B cells; BrHPP, Bromohydrin pyrophosphate; BTN, butyrophilin; CAR, Chimeric antigen receptor; CLL, Chronic lymphocytic leukemia; CM, Central memory cells; DCs, Dendritic cells; DNAM-1, DNA X accessory molecule 1; EC, Endothelial cells; EM, Effector memory; FL, Follicular lymphoma; Gr, Granzyme; GvHD, Graft-versus-host disease; GvT, Graft-versus-tumor; HSPC, stem/progenitor cells; HMB-PP, (E)-4-Hydroxy-3-methyl-but-2-enyl pyrophosphate; ICP/ICP-L, Immune checkpoint/immune checkpoint-ligand; IDO1, Indoleamine 2,3-dioxygenase 1; IMiDs, immunomodulatory drugs; IL, Interleukin; IPP, Isopentenyl pyrophosphate; IFNγ, Interferon-γ; KAR, Killer activating receptors; KIR, killer activating receptors; mAbs, monoclonal antibodies; MAIT, mucosal-associated invariant T cells; MDSC, myeloid-derived suppressor cells; Mev, Mevalonate; MGUS, Monoclonal gammopathy of undetermined significance; MHC, Major histocompatibility complex; MM, multiple myeloma; NBP, Aminobisphosphonates; NKG2D, Natural killer 2D receptor; NKT, Natural killer T cells; pAgs, phosphoantigens; PB, Peripheral Blood; PDAC, Pancreatic ductal adenocarcinoma; Pr, Perforin; TAA, Tumor-associated antigens; TCR, T-cell Receptor; TIL, Tumor-infiltrating lymphocytes; TME, Tumor microenvironment; TNFα, Tumor necrosis factor-α; Tregs, Regulatory T cells; ZA, Zoledronic acid; 2M3B1PP, 2-methyl-3-butenyl-1-pyrophosphate.
References
1. Chien YH, Konigshofer Y. Antigen recognition by gammadelta T cells. Immunol Rev (2007) 215:46–58. doi: 10.1111/J.1600-065X.2006.00470.X
2. Chen D, Guo Y, Jiang J, Wu P, Zhang T, Wei Q, et al. γδ T cell exhaustion: Opportunities for intervention. J Leukoc Biol (2022) 112(6):1669–76. doi: 10.1002/JLB.5MR0722-777R
3. Morath A, Schamel WW. αβ and γδ T cell receptors: Similar but different. J Leukoc Biol (2020) 107:1045–55. doi: 10.1002/JLB.2MR1219-233R
4. Silva-Santos B, Mensurado S, Coffelt SB. γδ T cells: pleiotropic immune effectors with therapeutic potential in cancer. Nat Rev Cancer (2019) 19:392–404. doi: 10.1038/s41568-019-0153-5
5. Deng J, Yin H. Gamma delta (γδ) T cells in cancer immunotherapy; where it comes from, where it will go? Eur J Pharmacol (2022) 919:174803. doi: 10.1016/j.ejphar.2022.174803
6. Godfrey DI, le Nours J, Andrews DM, Uldrich AP, Rossjohn J. Unconventional T cell targets for cancer immunotherapy. Immunity (2018) 48:453–73. doi: 10.1016/J.IMMUNI.2018.03.009
7. Pellicci DG, Koay HF, Berzins SP. Thymic development of unconventional T cells: how NKT cells, MAIT cells and γδ T cells emerge. Nat Rev Immunol (2020) 20:756–70. doi: 10.1038/S41577-020-0345-Y
8. Ribot JC, Lopes N, Silva-Santos B. γδ T cells in tissue physiology and surveillance. Nat Rev Immunol (2020) 21:221–32. doi: 10.1038/s41577-020-00452-4
9. De Libero G, Casorati G, Giachino C, Carbonara C, Migone N, Matzinger P, et al. Selection by two powerful antigens may account for the presence of the major population of human peripheral gamma/delta T cells. J Exp Med (1991) 173:1311–22. doi: 10.1084/JEM.173.6.1311
10. Parker CM, Groh V, Band H, Porcelli SA, Morita C, Fabbi M, et al. Evidence for extrathymic changes in the T cell receptor gamma/delta repertoire. J Exp Med (1990) 171:1597–612. doi: 10.1084/JEM.171.5.1597
11. Dimova T, Brouwer M, Gosselin F, Tassignon J, Leo O, Donner C, et al. Effector Vγ9Vδ2 T cells dominate the human fetal γδ T-cell repertoire. Proc Natl Acad Sci USA (2015) 112:E556–65. doi: 10.1073/PNAS.1412058112
12. Pauza CD, Cairo C. Evolution and function of the TCR Vgamma9 chain repertoire: It’s good to be public. Cell Immunol (2015) 296:22–30. doi: 10.1016/J.CELLIMM.2015.02.010
13. Davey MS, Willcox CR, Hunter S, Kasatskaya SA, Remmerswaal EBM, Salim M, et al. The human Vδ2+ T-cell compartment comprises distinct innate-like Vγ9+ and adaptive Vγ9- subsets. Nat Commun (2018) 9(1):1760. doi: 10.1038/S41467-018-04076-0
14. Davey MS, Willcox CR, Joyce SP, Ladell K, Kasatskaya SA, McLaren JE, et al. Clonal selection in the human Vδ1 T cell repertoire indicates γδ TCR-dependent adaptive immune surveillance. Nat Commun (2017) 8:14760. doi: 10.1038/NCOMMS14760
15. Benveniste PM, Roy S, Nakatsugawa M, Chen ELY, Nguyen L, Millar DG, et al. Generation and molecular recognition of melanoma-associated antigen-specific human γδ T cells. Sci Immunol (2018) 3(30):eaav4036. doi: 10.1126/SCIIMMUNOL.AAV4036
16. Liu J, Qu H, Li Q, Ye L, Ma G, Wan H. The responses of γδ T-cells against acute pseudomonas aeruginosa pulmonary infection in mice via interleukin-17. Pathog Dis (2013) 68:44–51. doi: 10.1111/2049-632X.12043
17. Sabbaghi A, Miri SM, Keshavarz M, Mahooti M, Zebardast A, Ghaemi A. Role of γδ T cells in controlling viral infections with a focus on influenza virus: implications for designing novel therapeutic approaches. Virol J (2020) 17(1):174. doi: 10.1186/S12985-020-01449-0
18. Saitoh A, Narita M, Watanabe N, Tochiki N, Satoh N, Takizawa J, et al. Anti-tumor cytotoxicity of gammadelta T cells expanded from peripheral blood cells of patients with myeloma and lymphoma. Med Oncol (2008) 25:137–47. doi: 10.1007/S12032-007-9004-4
19. Cazzetta V, Bruni E, Terzoli S, Carenza C, Franzese S, Piazza R, et al. NKG2A expression identifies a subset of human Vδ2 T cells exerting the highest antitumor effector functions. Cell Rep (2021) 37(3):109871. doi: 10.1016/J.CELREP.2021.109871
20. Park JH, Kim HJ, Kim CW, Kim HC, Jung Y, Lee HS, et al. Tumor hypoxia represses γδ T cell-mediated antitumor immunity against brain tumors. Nat Immunol (2021) 22:336–46. doi: 10.1038/s41590-020-00860-7
21. Coffelt SB, Kersten K, Doornebal CW, Weiden J, Vrijland K, Hau CS, et al. IL-17-producing γδ T cells and neutrophils conspire to promote breast cancer metastasis. Nature (2015) 522:345–8. doi: 10.1038/NATURE14282
22. Ma S, Cheng Q, Cai Y, Gong H, Wu Y, Yu X, et al. IL-17A produced by γδ T cells promotes tumor growth in hepatocellular carcinoma. Cancer Res (2014) 74:1969–82. doi: 10.1158/0008-5472.CAN-13-2534
23. Zhan Y, Zheng L, Liu J, Hu D, Wang J, Liu K, et al. PLA2G4A promotes right-sided colorectal cancer progression by inducing CD39+γδ treg polarization. JCI Insight (2021) 6(16):e148028. doi: 10.1172/JCI.INSIGHT.148028
24. Fleming C, Morrissey S, Cai Y, Yan J. γδ T cells: Unexpected regulators of cancer development and progression. Trends Cancer (2017) 3:561–70. doi: 10.1016/J.TRECAN.2017.06.003
25. Li Y, Li G, Zhang J, Wu X, Chen X. The dual roles of human γδ T cells: Anti-tumor or tumor-promoting. Front Immunol (2021) 11:619954. doi: 10.3389/fimmu.2020.619954
26. Wu P, Wu D, Ni C, Ye J, Chen W, Hu G, et al. γδT17 cells promote the accumulation and expansion of myeloid-derived suppressor cells in human colorectal cancer. Immunity (2014) 40:785–800. doi: 10.1016/J.IMMUNI.2014.03.013
27. Ma Y, Lei H, Tan J, Xuan L, Wu X, Liu Q. Characterization of γδ regulatory T cells from peripheral blood in patients with multiple myeloma. Biochem Biophys Res Commun (2016) 480:594–601. doi: 10.1016/j.bbrc.2016.10.098
28. Jin Z, Ye W, Lan T, Zhao Y, Liu X, Chen J, et al. Characteristic of TIGIT and DNAM-1 expression on Foxp3+ γδ T cells in AML patients. (2020) 2020:4612952. doi: 10.1155/2020/4612952
29. Khairallah C, Chu TH, Sheridan BS. Tissue adaptations of memory and tissue-resident gamma delta T cells. Front Immunol (2018) 9:2636. doi: 10.3389/FIMMU.2018.02636
30. Giannotta C, Castella B, Tripoli E, Grimaldi D, Avonto I, D’Agostino M, et al. Immune dysfunctions affecting bone marrow Vγ9Vδ2 T cells in multiple myeloma: Role of immune checkpoints and disease status. Front Immunol (2022) 13:1073227. doi: 10.3389/FIMMU.2022.10732275
31. Lo Presti E, Toia F, Oieni S, Buccheri S, Turdo A, Mangiapane LR, et al. Squamous cell tumors recruit γδ T cells producing either IL17 or IFNγ depending on the tumor stage. Cancer Immunol Res (2017) 5:397–407. doi: 10.1158/2326-6066.CIR-16-0348
32. Kabelitz D, Serrano R, Kouakanou L, Peters C, Kalyan S. Cancer immunotherapy with γδ T cells: many paths ahead of us. Cell Mol Immunol (2020) 17:925–39. doi: 10.1038/s41423-020-0504-x
33. Gräwert T, Groll M, Rohdich F, Bacher A, Eisenreich W. Biochemistry of the non-mevalonate isoprenoid pathway. Cell Mol Life Sci (2011) 68:3797–814. doi: 10.1007/s00018-011-0753-z
34. Castella B, Vitale C, Coscia M, Massaia M. Vγ9Vδ2 T cell-based immunotherapy in hematological malignancies: from bench to bedside. Cell Mol Life Sci (2011) 68:2419–32. doi: 10.1007/S00018-011-0704-8
35. Vantourout P, Laing A, Woodward MJ, Zlatareva I, Apolonia L, Jones AW, et al. Heteromeric interactions regulate butyrophilin (BTN) and BTN-like molecules governing γδ T cell biology. Proc Natl Acad Sci USA (2018) 115:1039–44. doi: 10.1073/pnas.1701237115
36. Gu S, Borowska MT, Boughter CT, Adams EJ. Butyrophilin3A proteins and Vγ9Vδ2 T cell activation. Semin Cell Dev Biol (2018) 84:65. doi: 10.1016/J.SEMCDB.2018.02.007
37. Rigau M, Ostrouska S, Fulford TS, Johnson DN, Woods K, Ruan Z, et al. Butyrophilin 2A1 is essential for phosphoantigen reactivity by γδ T cells. Science (2020) 367(6478):eaay5516. doi: 10.1126/SCIENCE.AAY5516
38. Karunakaran MM, Willcox CR, Salim M, Paletta D, Fichtner AS, Noll A, et al. Butyrophilin-2A1 directly binds germline-encoded regions of the Vγ9Vδ2 TCR and is essential for phosphoantigen sensing. Immunity (2020) 52:487–498.e6. doi: 10.1016/J.IMMUNI.2020.02.014
39. Eberl M. Antigen recognition by human γδ T cells: one step closer to knowing. Immunol Cell Biol (2020) 98:351. doi: 10.1111/IMCB.12334
40. Castella B, Kopecka J, Sciancalepore P, Mandili G, Foglietta M, Mitro N, et al. The ATP-binding cassette transporter A1 regulates phosphoantigen release and Vγ39Vδ2 T cell activation by dendritic cells. Nat Commun (2017) 8:1–14. doi: 10.1038/ncomms15663
41. Laplagne C, Ligat L, Foote J, Lopez F, Fournié JJ, Laurent C, et al. Self-activation of Vγ9Vδ2 T cells by exogenous phosphoantigens involves TCR and butyrophilins. Cell Mol Immunol (2021) 18:1861–70. doi: 10.1038/S41423-021-00720-W
42. Castella B, Foglietta M, Riganti C, Massaia M. Vγ9Vδ2 T cells in the bone marrow of myeloma patients: A paradigm of microenvironment-induced immune suppression. Front Immunol (2018) 9:1492. doi: 10.3389/FIMMU.2018.01492
43. Pang DJ, Neves JF, Sumaria N, Pennington DJ. Understanding the complexity of γδ T-cell subsets in mouse and human. Immunology (2012) 136:283–90. doi: 10.1111/J.1365-2567.2012.03582.X
44. Thompson K, Rojas-Navea J, Rogers MJ. Alkylamines cause Vgamma9Vdelta2 T-cell activation and proliferation by inhibiting the mevalonate pathway. Blood (2006) 107:651–4. doi: 10.1182/BLOOD-2005-03-1025
45. Fiore F, Castella B, Nuschak B, Bertieri R, Mariani S, Bruno B, et al. Enhanced ability of dendritic cells to stimulate innate and adaptive immunity on short-term incubation with zoledronic acid. Blood (2007) 110:921–7. doi: 10.1182/BLOOD-2006-09-044321
46. Nedellec S, Bonneville M, Scotet E. Human Vγ9Vδ2 T cells: From signals to functions. Semin Immunol (2010) 22:199–206. doi: 10.1016/J.SMIM.2010.04.004
47. Von Lilienfeld-Toal M, Nattermann J, Feldmann G, Sievers E, Frank S, Strehl J, et al. Activated γδ T cells express the natural cytotoxicity receptor natural killer p44 and show cytotoxic activity against myeloma cells. Clin Exp Immunol (2006) 144:528. doi: 10.1111/J.1365-2249.2006.03078.X
48. Xiang Z, Tu W. Dual face of Vγ9Vδ2-T cells in tumor immunology: Anti- versus pro-tumoral activities. Front Immunol (2017) 8:1041. doi: 10.3389/FIMMU.2017.01041
49. Brandes M, Willimann K, Moser B. Professional antigen-presentation function by human gammadelta T cells. Science (2005) 309:264–8. doi: 10.1126/SCIENCE.1110267
50. Beetz S, Wesch D, Marischen L, Welte S, Oberg HH, Kabelitz D. Innate immune functions of human gammadelta T cells. Immunobiology (2008) 213:173–82. doi: 10.1016/J.IMBIO.2007.10.006
51. Bonneville M, Scotet E. Human Vgamma9Vdelta2 T cells: promising new leads for immunotherapy of infections and tumors. Curr Opin Immunol (2006) 18:539–46. doi: 10.1016/J.COI.2006.07.002
52. Castella B, Riganti C, Fiore F, Pantaleoni F, Canepari ME, Peola S, et al. Immune modulation by zoledronic acid in human myeloma: an advantageous cross-talk between Vγ9Vδ2 T cells, αβ CD8+ T cells, regulatory T cells, and dendritic cells. J Immunol (2011) 187:1578–90. doi: 10.4049/JIMMUNOL.1002514
53. Peters C, Kabelitz D, Wesch D. Regulatory functions of γδ T cells. Cell Mol Life Sci (2018) 75:2125–35. doi: 10.1007/S00018-018-2788-X
54. Dieli F, Poccia F, Lipp M, Sireci G, Caccamo N, di Sano C, et al. Differentiation of effector/memory Vdelta2 T cells and migratory routes in lymph nodes or inflammatory sites. J Exp Med (2003) 198:391–7. doi: 10.1084/JEM.20030235
55. Odaira K, Kimura SN, Fujieda N, Kobayashi Y, Kambara K, Takahashi T, et al. CD27(-)CD45(+) γδ T cells can be divided into two populations, CD27(-)CD45(int) and CD27(-)CD45(hi) with little proliferation potential. Biochem Biophys Res Commun (2016) 478:1298–303. doi: 10.1016/J.BBRC.2016.08.115
56. Schönefeldt S, Wais T, Herling M, Mustjoki S, Bekiaris V, Moriggl R, et al. The diverse roles of γδ T cells in cancer: From rapid immunity to aggressive lymphoma. Cancers (Basel) (2021) 13:6212. doi: 10.3390/CANCERS13246212
57. Chan KF, Duarte JDG, Ostrouska S, Behren A. γδ T cells in the tumor microenvironment–interactions with other immune cells. Front Immunol (2022) 13:894315. doi: 10.3389/fimmu.2022.894315
58. Holmen Olofsson G, Idorn M, Carnaz Simões AM, Aehnlich P, Skadborg SK, Noessner E, et al. Vγ9Vδ2 T cells concurrently kill cancer cells and cross-present tumor antigens. Front Immunol (2021) 12:645131. doi: 10.3389/FIMMU.2021.645131
59. Takahara M, Miyai M, Tomiyama M, Mutou M, Nicol AJ, Nieda M. Copulsing tumor antigen-pulsed dendritic cells with zoledronate efficiently enhance the expansion of tumor antigen-specific CD8+ T cells via Vgamma9gammadelta T cell activation. J Leukoc Biol (2008) 83:742–54. doi: 10.1189/JLB.0307185
60. Altvater B, Pscherer S, Landmeier S, Kailayangiri S, Savoldo B, Juergens H, et al. Activated human γδ T cells induce peptide-specific CD8+ T-cell responses to tumor-associated self-antigens. Cancer Immunol Immunother (2012) 61:385–96. doi: 10.1007/S00262-011-1111-6
61. Bansal RR, Mackay CR, Moser B, Eberl M. IL-21 enhances the potential of human γδ T cells to provide b-cell help. Eur J Immunol (2012) 42:110–9. doi: 10.1002/EJI.201142017
62. Agrati C, Cimini E, Sacchi A, Bordoni V, Gioia C, Casetti R, et al. Activated V gamma 9V delta 2 T cells trigger granulocyte functions via MCP-2 release. J Immunol (2009) 182:522–9. doi: 10.4049/JIMMUNOL.182.1.522
63. Maniar A, Zhang X, Lin W, Gastman BR, Pauza CD, Strome SE, et al. Human gammadelta T lymphocytes induce robust NK cell-mediated antitumor cytotoxicity through CD137 engagement. Blood (2010) 116:1726–33. doi: 10.1182/BLOOD-2009-07-234211
64. Castella B, Foglietta M, Sciancalepore P, Rigoni M, Coscia M, Griggio V, et al. Anergic bone marrow Vγ9Vδ2 T cells as early and long-lasting markers of PD-1-targetable microenvironment-induced immune suppression in human myeloma. Oncoimmunology (2015) 4(11):e1047580. doi: 10.1080/2162402X.2015.1047580
65. Wu K, Feng J, Xiu Y, Li Z, Lin Z, Zhao H, et al. Vδ2 T cell subsets, defined by PD-1 and TIM-3 expression, present varied cytokine responses in acute myeloid leukemia patients. Int Immunopharmacol (2020) 80:106122. doi: 10.1016/J.INTIMP.2019.106122
66. He W, Hu Y, Chen D, Li Y, Ye D, Zhao Q, et al. Hepatocellular carcinoma-infiltrating γδ T cells are functionally defected and allogenic Vδ2 + γδ T cell can be a promising complement. Clin Transl Med (2022) 12(4):e800. doi: 10.1002/ctm2.800
67. Barjon C, Michaud HA, Fages A, Dejou C, Zampieri A, They L, et al. IL-21 promotes the development of a CD73-positive Vγ9Vδ2 T cell regulatory population. Oncoimmunology (2017) 7(1):e1379642. doi: 10.1080/2162402X.2017.1379642
68. Dunne MR, Mangan BA, Madrigal-Estebas L, Doherty DG. Preferential Th1 cytokine profile of phosphoantigen-stimulated human Vγ9Vδ2 T cells. Mediators Inflammation (2010) 2010:704941. doi: 10.1155/2010/704941
69. Caccamo N, La Mendola C, Orlando V, Meraviglia S, Todaro M, Stassi G, et al. Differentiation, phenotype, and function of interleukin-17-producing human Vγ9Vδ2 T cells. Blood (2011) 118:129–38. doi: 10.1182/BLOOD-2011-01-331298
70. McAllister F, Bailey JM, Alsina J, Nirschl CJ, Sharma R, Fan H, et al. Oncogenic kras activates a hematopoietic-to-epithelial IL-17 signaling axis in preinvasive pancreatic neoplasia. Cancer Cell (2014) 25:621. doi: 10.1016/J.CCR.2014.03.014
71. Schilbach K, Krickeberg N, Kaißer C, Mingram S, Kind J, Siegers GM, et al. Suppressive activity of Vδ2+ γδ T cells on αβ T cells is licensed by TCR signaling and correlates with signal strength. Cancer Immunology Immunother (2020) 69:593. doi: 10.1007/S00262-019-02469-8
72. Peters C, Oberg HH, Kabelitz D, Wesch D. Phenotype and regulation of immunosuppressive Vδ2-expressing γδ T cells. Cell Mol Life Sci (2014) 71:1943. doi: 10.1007/S00018-013-1467-1
73. Giannotta C, Autino F, Massaia M. The immune suppressive tumor microenvironment in multiple myeloma: The contribution of myeloid-derived suppressor cells. Front Immunol (2023) 13:1102471. doi: 10.3389/fimmu.2022.1102471
74. Wu K, Zhao H, Xiu Y, Li Z, Zhao J, Xie S, et al. IL-21-mediated expansion of Vγ9Vδ2 T cells is limited by the Tim-3 pathway. Int Immunopharmacol (2019) 69:136–42. doi: 10.1016/J.INTIMP.2019.01.027
75. Tirier SM, Mallm JP, Steiger S, Poos AM, Awwad MHS, Giesen N, et al. Subclone-specific microenvironmental impact and drug response in refractory multiple myeloma revealed by single-cell transcriptomics. Nat Commun (2021) 12(1):6960. doi: 10.1038/S41467-021-26951-Z
76. Noviello M, Manfredi F, Ruggiero E, Perini T, Oliveira G, Cortesi F, et al. Bone marrow central memory and memory stem T-cell exhaustion in AML patients relapsing after HSCT. Nat Commun (2019) 10(1):1065. doi: 10.1038/s41467-019-08871-1
77. Liu Y, Yan X, Zhang F, Zhang X, Tang F, Han Z, et al. TCR-T immunotherapy: The challenges and solutions. Front Oncol (2022) 11:794183. doi: 10.3389/fonc.2021.794183
78. Jiang H, Fu D, Bidgoli A, Paczesny S. T Cell subsets in graft versus host disease and graft versus tumor. Front Immunol (2021) 12:761448. doi: 10.3389/fimmu.2021.761448
79. Cornel AM, Mimpen IL, Nierkens S. MHC class I downregulation in cancer: Underlying mechanisms and potential targets for cancer immunotherapy. Cancers (Basel) (2020) 12:1–33. doi: 10.3390/cancers12071760
80. Alnaggar M, Xu Y, Li J, He J, Chen J, Li M, et al. Allogenic Vγ9Vδ2 T cell as new potential immunotherapy drug for solid tumor: a case study for cholangiocarcinoma. J Immunother Cancer (2019) 7(1):36. doi: 10.1186/S40425-019-0501-8
81. Xu Y, Xiang Z, Alnaggar M, Kouakanou L, Li J, He J, et al. Allogeneic Vγ9Vδ2 T-cell immunotherapy exhibits promising clinical safety and prolongs the survival of patients with late-stage lung or liver cancer. Cell Mol Immunol (2021) 18:427–39. doi: 10.1038/S41423-020-0515-7
82. Wilhelm M, Smetak M, Schaefer-Eckart K, Kimmel B, Birkmann J, Einsele H, et al. Successful adoptive transfer and in vivo expansion of haploidentical γδ T cells. J Transl Med (2014) 12:45. doi: 10.1186/1479-5876-12-45
83. Vydra J, Cosimo E, Lesný P, Wanless RS, Anderson J, Clark AG, et al. A phase I trial of allogeneic γδ T lymphocytes from haploidentical donors in patients with refractory or relapsed acute myeloid leukemia. Clin Lymphoma Myeloma Leuk (2023), S2152-2650(23)00038-1. doi: 10.1016/J.CLML.2023.02.003
84. Bold A, Gaertner J, Bott A, Mordstein V, Schaefer-Eckart K, Wilhelm M. Haploidentical γδ T cells induce complete remission in chemorefractory b-cell non-Hodgkin lymphoma. J Immunother (2023) 46:56–8. doi: 10.1097/CJI.0000000000000450
85. Burnham RE, Zoine JT, Story JY, Garimalla SN, Gibson G, Rae A, et al. Characterization of donor variability for γδ T cell ex vivo expansion and development of an allogeneic γδ T cell immunotherapy. Front Med (Lausanne) (2020) 7:588453. doi: 10.3389/fmed.2020.588453
86. Braza MS, Klein B, Fiol G, Rossi JF. γδ T-cell killing of primary follicular lymphoma cells is dramatically potentiated by GA101, a type II glycoengineered anti-CD20 monoclonal antibody. Haematologica (2011) 96:400–7. doi: 10.3324/HAEMATOL.2010.029520
87. Tokuyama H, Hagi T, Mattarollo SR, Morley J, Wang Q, Fai-So H, et al. V Gamma 9 V delta 2 T cell cytotoxicity against tumor cells is enhanced by monoclonal antibody drugs–rituximab and trastuzumab. Int J Cancer (2008) 122:2526–34. doi: 10.1002/IJC.23365
88. Gertner-Dardenne J, Bonnafous C, Bezombes C, Capietto AH, Scaglione V, Ingoure S, et al. Bromohydrin pyrophosphate enhances antibody-dependent cell-mediated cytotoxicity induced by therapeutic antibodies. Blood (2009) 113:4875–84. doi: 10.1182/BLOOD-2008-08-172296
89. Abe Y, Muto M, Nieda M, Nakagawa Y, Nicol A, Kaneko T, et al. Clinical and immunological evaluation of zoledronate-activated Vgamma9gammadelta T-cell-based immunotherapy for patients with multiple myeloma. Exp Hematol (2009) 37:956–68. doi: 10.1016/J.EXPHEM.2009.04.008
90. Rezvani K, Yong ASM, Mielke S, Jafarpour B, Savani BN, Le RQ, et al. Repeated PR1 and WT1 peptide vaccination in montanide-adjuvant fails to induce sustained high-avidity, epitope-specific CD8+ T cells in myeloid malignancies. Haematologica (2011) 96:432–40. doi: 10.3324/HAEMATOL.2010.031674
91. Kitawaki T, Kadowaki N, Fukunaga K, Kasai Y, Maekawa T, Ohmori K, et al. A phase I/IIa clinical trial of immunotherapy for elderly patients with acute myeloid leukaemia using dendritic cells co-pulsed with WT1 peptide and zoledronate. Br J Haematol (2011) 153:796–9. doi: 10.1111/J.1365-2141.2010.08490.X
92. Khan MWA, Eberl M, Moser B. Potential use of γδ T cell-based vaccines in cancer immunotherapy. Front Immunol (2014) 5:512. doi: 10.3389/FIMMU.2014.00512
93. Kunzmann V, Smetak M, Kimmel B, Weigang-Koehler K, Goebeler M, Birkmann J, et al. Tumor-promoting versus tumor-antagonizing roles of γδ T cells in cancer immunotherapy: Results from a prospective phase I/II trial. J Immunother (2012) 35:205–13. doi: 10.1097/CJI.0b013e318245bb1e
94. Saura-Esteller J, de Jong M, King LA, Ensing E, Winograd B, de Gruijl TD, et al. Gamma delta T-cell based cancer immunotherapy: Past-Present-Future. Front Immunol (2022) 13:915837. doi: 10.3389/fimmu.2022.915837
95. Presti EL, Pizzolato G, Corsale AM, Caccamo N, Sireci G, Dieli F, et al. γδ T cells and tumor microenvironment: From immunosurveillance to tumor evasion. Front Immunol (2018) 9:1395. doi: 10.3389/FIMMU.2018.01395
96. Capietto AH, Martinet L, Fournie JJ. How tumors might withstand γδ T-cell attack. Cell Mol Life Sci (2011) 68:2433–42. doi: 10.1007/S00018-011-0705-7
97. Mariani S, Muraro M, Pantaleoni F, Fiore F, Nuschak B, Peola S, et al. Effector γδ T cells and tumor cells as immune targets of zoledronic acid in multiple myeloma. Leukemia (2005) 19:664–70. doi: 10.1038/sj.leu.2403693
98. Coscia M, Vitale C, Peola S, Foglietta M, Rigoni M, Griggio V, et al. Dysfunctional Vγ9Vδ2 T cells are negative prognosticators and markers of dysregulated mevalonate pathway activity in chronic lymphocytic leukemia cells. Blood (2012) 120:3271–9. doi: 10.1182/blood-2012-03-417519
99. Tas L, Jedema I, Haanen JBAG. Novel strategies to improve efficacy of treatment with tumor-infiltrating lymphocytes (TILs) for patients with solid cancers. Curr Opin Oncol (2023) 35(2):107–13. doi: 10.1097/CCO.0000000000000925
100. Pizzolato G, Kaminski H, Tosolini M, Franchini D-M, Pont F, Martins F, et al. Single-cell RNA sequencing unveils the shared and the distinct cytotoxic hallmarks of human TCRVδ1 and TCRVδ2 γδ T lymphocytes. PNAS (2019) 116:11906–15. doi: 10.1073/pnas.1818488116
101. de Weerdt I, Hofland T, Lameris R, Endstra S, Jongejan A, Moerland PD, et al. Improving CLL Vγ9Vδ2-t-cell fitness for cellular therapy by ex vivo activation and ibrutinib. Blood (2018) 132:2260–72. doi: 10.1182/blood-2017-12-822569
102. Fazzi R, Petrini I, Giuliani N, Morganti R, Carulli G, Dalla Palma B, et al. Phase II trial of maintenance treatment with IL2 and zoledronate in multiple myeloma after bone marrow transplantation: Biological and clinical results. Front Immunol (2021) 11:573156. doi: 10.3389/fimmu.2020.573156
103. Merli P, Algeri M, Galaverna F, Milano GM, Bertaina V, Biagini S, et al. Immune modulation properties of zoledronic acid on TcRγδ T-lymphocytes after TcRαβ/CD19-depleted haploidentical stem cell transplantation: An analysis on 46 pediatric patients affected by acute leukemia. Front Immunol (2020) 11:699. doi: 10.3389/fimmu.2020.00699
104. Pressey JG, Adams J, Harkins L, Kelly D, You Z, Lamb LS. In vivo expansion and activation of gd T cells as immunotherapy for refractory neuroblastoma a phase 1 study. Med (United States) (2016) 95(39):e4909. doi: 10.1097/MD.0000000000004909
105. Jhita N, Raikar SS. Allogeneic gamma delta T cells as adoptive cellular therapy for hematologic malignancies. Explor Immunol (2022) 2:334–50. doi: 10.37349/EI.2022.00054
106. Chabab G, Barjon C, Bonnefoy N, Lafont V. Pro-tumor γδ T cells in human cancer: Polarization, mechanisms of action, and implications for therapy. Front Immunol (2020) 11:2186. doi: 10.3389/fimmu.2020.02186
107. Miyashita M, Shimizu T, Ashihara E, Ukimura O. Strategies to improve the antitumor effect of γδ T cell immunotherapy for clinical application. Int J Mol Sci (2021) 22(16):8910. doi: 10.3390/ijms22168910
108. Rossi C, Gravelle P, Decaup E, Bordenave J, Poupot M, Tosolini M, et al. Boosting γδ T cell-mediated antibody-dependent cellular cytotoxicity by PD-1 blockade in follicular lymphoma. Oncoimmunology (2019) 8:1554175. doi: 10.1080/2162402X.2018.1554175
109. De Gassart A, Le KS, Brune P, Agaugué S, Sims J, Goubard A, et al. Development of ICT01, a first-in-class, anti-BTN3A antibody for activating Vγ9Vδ2 T cell-mediated antitumor immune response. Sci Transl Med (2021) 13:835. doi: 10.1126/SCITRANSLMED.ABJ0835
110. Ganesan R, Chennupati V, Ramachandran B, Hansen MR, Singh S, Grewal IS. Selective recruitment of γδ T cells by a bispecific antibody for the treatment of acute myeloid leukemia. Leukemia (2021) 35:2274–84. doi: 10.1038/s41375-021-01122-7
111. de Weerdt I, Lameris R, Scheffer GL, Vree J, de Boer R, Stam AG, et al. A bispecific antibody antagonizes prosurvival CD40 signaling and promotes Vγ9Vδ2 T cell-mediated antitumor responses in human b-cell malignancies. Cancer Immunol Res (2021) 9:50–61. doi: 10.1158/2326-6066.CIR-20-0138
112. de Weerdt I, Lameris R, Ruben JM, de Boer R, Kloosterman J, King LA, et al. A bispecific single-domain antibody boosts autologous Vγ9Vδ2-T cell responses toward CD1d in chronic lymphocytic leukemia. Clin Cancer Res (2021) 27:1744–55. doi: 10.1158/1078-0432.CCR-20-4576
113. van Diest E, Hernández López P, Meringa AD, Vyborova A, Karaiskaki F, Heijhuurs S, et al. Gamma delta TCR anti-CD3 bispecific molecules (GABs) as novel immunotherapeutic compounds. J Immunother Cancer (2021) 9:3850. doi: 10.1136/jitc-2021-003850
114. Okuno D, Sugiura Y, Sakamoto N, Tagod MSO, Iwasaki M, Noda S, et al. Comparison of a novel bisphosphonate prodrug and zoledronic acid in the induction of cytotoxicity in human Vγ2Vδ2 T cells. Front Immunol (2020) 11:1405. doi: 10.3389/fimmu.2020.01405
115. Kouakanou L, Xu Y, Peters C, He J, Wu Y, Yin Z, et al. Vitamin c promotes the proliferation and effector functions of human γδ T cells. Cell Mol Immunol (2020) 17:462–73. doi: 10.1038/s41423-019-0247-8
116. van Acker HH, Anguille S, Willemen Y, van den Bergh JM, Berneman ZN, Lion E, et al. Interleukin-15 enhances the proliferation, stimulatory phenotype, and antitumor effector functions of human gamma delta T cells. J Hematol Oncol (2016) 9:1–13. doi: 10.1186/s13045-016-0329-3
117. Landin AM, Cox C, Yu B, Bejanyan N, Davila M, Kelley L. Expansion and enrichment of gamma-delta (γδ) t cells from apheresed human product. J Visualized Experiments (2021) (175). doi: 10.3791/62622
118. Choi H, Lee Y, Hur G, Lee SE, Il CH, HJ S, et al. γδ T cells cultured with artificial antigen-presenting cells and IL-2 show long-term proliferation and enhanced effector functions compared with γδ T cells cultured with only IL-2 after stimulation with zoledronic acid. Cytotherapy (2021) 23:908–17. doi: 10.1016/j.jcyt.2021.06.002
119. Boucher JC, Yu B, Li G, Shrestha B, Sallman D, Landin AM, et al. Large Scale ex vivo expansion of γδ T cells using artificial antigen-presenting cells. J Immunother (2023) 46:5–13. doi: 10.1097/CJI.0000000000000445
120. Hernandez Tejada FN, Jawed J, Olivares S, Mahadeo KM, Singh H. Gamma delta T cells for acute myeloid leukemia. Blood (2022) 140:12696–6. doi: 10.1182/BLOOD-2022-162635
121. Lawrence M, Wiesheu R, Coffelt SB. The duplexity of unconventional T cells in cancer. Int J Biochem Cell Biol (2022) 146:106213. doi: 10.1016/j.biocel.2022.106213
122. Yang R, He Q, Zhou H, Gong C, Wang X, Song X, et al. Vg2 x PD-L1, a bispecific antibody targeting both the Vg2 TCR and PD-L1, improves the anti-tumor response of Vg2Vd2 T cell. Front Immunol (2022) 13:923969. doi: 10.3389/fimmu.2022.923969
123. Nada MH, Wang H, Hussein AJ, Tanaka Y, Morita CT. PD-1 checkpoint blockade enhances adoptive immunotherapy by human Vγ2Vδ2 T cells against human prostate cancer. Oncoimmunology (2021) 10(1):1989789. doi: 10.1080/2162402X.2021.1989789
124. Benyamine A, Le Roy A, Mamessier E, Gertner-Dardenne J, Castanier C, Orlanducci F, et al. BTN3A molecules considerably improve Vγ9Vδ2T cells-based immunotherapy in acute myeloid leukemia. Oncoimmunology (2016) 5(10):e1146843. doi: 10.1080/2162402X.2016.1146843
125. Lin L, He J, Li J, Xu Y, Li J, Wu Y. Chitosan nanoparticles strengthen Vγ9Vδ2 T-cell cytotoxicity through upregulation of killing molecules and cytoskeleton polarization. Int J Nanomed (2019) 14:9325–36. doi: 10.2147/IJN.S212898
126. Lamb LS, Bowersock J, Dasgupta A, Gillespie GY, Su Y, Johnson A, et al. Engineered drug resistant γδ T cells kill glioblastoma cell lines during a chemotherapy challenge: a strategy for combining chemo- and immunotherapy. PloS One (2013) 8(1):e51805. doi: 10.1371/JOURNAL.PONE.0051805
127. Todaro M, Orlando V, Cicero G, Caccamo N, Meraviglia S, Stassi G, et al. Chemotherapy sensitizes colon cancer initiating cells to Vγ9Vδ2 T cell-mediated cytotoxicity. PLoS One (2013) 8(6):e65145. doi: 10.1371/journal.pone.0065145
128. Story JY, Zoine JT, Burnham RE, Hamilton JAG, Spencer HT, Doering CB, et al. Bortezomib enhances cytotoxicity of ex vivo-expanded gamma delta T cells against acute myeloid leukemia and T-cell acute lymphoblastic leukemia. Cytotherapy (2021) 23:12–24. doi: 10.1016/J.JCYT.2020.09.010
129. Alves da Silva PH, Xing S, Kotini AG, Papapetrou EP, Song X, Wucherpfennig KW, et al. MICA/B antibody induces macrophage-mediated immunity against acute myeloid leukemia. Blood (2022) 139:205. doi: 10.1182/BLOOD.2021011619
130. Raje N, Hideshima T, Davies FE, Chauhan D, Treon SP, Young G, et al. Tumour cell/dendritic cell fusions as a vaccination strategy for multiple myeloma. Br J Haematol (2004) 125:343–52. doi: 10.1111/J.1365-2141.2004.04929.X
131. Rosenblatt J, Avivi I, Vasir B, Uhl L, Munshi NC, Katz T, et al. Vaccination with dendritic cell/tumor fusions following autologous stem cell transplant induces immunologic and clinical responses in multiple myeloma patients. Clin Cancer Res (2013) 19:3640–8. doi: 10.1158/1078-0432.CCR-13-0282
132. Gong J, Koido S, Kato Y, Tanaka Y, Chen D, Jonas A, et al. Induction of anti-leukemic cytotoxic T lymphocytes by fusion of patient-derived dendritic cells with autologous myeloblasts. Leuk Res (2004) 28:1303–12. doi: 10.1016/j.leukres.2004.03.018
133. Wang Y, Zhu J, Yu W, Wang J, Xia K, Liang C, et al. Allogenic γδ T cell and tumor cell fused vaccine for enhanced immunotherapeutic efficacy of osteosarcoma. J Bone Oncol (2020) 21:100214. doi: 10.1016/J.JBO.2018.100214
134. Willcox CR, Mohammed F, Willcox BE. The distinct MHC-unrestricted immunobiology of innate-like and adaptive-like human γδ T cell subsets–nature’s CAR-T cells. Immunol Rev (2020) 298:25–46. doi: 10.1111/imr.12928
135. Zhang X, Ng YY, Du Z, Li Z, Chen C, Xiao L, et al. Vγ9Vδ2 T cells expressing a BCMA–specific chimeric antigen receptor inhibit multiple myeloma xenograft growth. PloS One (2022) 17(6):e0267475. doi: 10.1371/journal.pone.0267475
136. Zhang X, Ang WX, Du Z, Ng YY, Zha S, Chen C, et al. A CD123-specific chimeric antigen receptor augments anti-acute myeloid leukemia activity of Vγ9Vδ2 T cells. Immunotherapy (2022) 14:321–36. doi: 10.2217/imt-2021-0143
137. Rozenbaum M, Meir A, Aharony Y, Itzhaki O, Schachter J, Bank I, et al. Gamma-delta CAR-T cells show CAR-directed and independent activity against leukemia. Front Immunol (2020) 11:1347. doi: 10.3389/fimmu.2020.01347
138. Capsomidis A, Benthall G, Van Acker HH, Fisher J, Kramer AM, Abeln Z, et al. Chimeric antigen receptor-engineered human gamma delta T cells: Enhanced cytotoxicity with retention of cross presentation. Mol Ther (2018) 26:354–65. doi: 10.1016/j.ymthe.2017.12.001
139. Harrer DC, Simon B, Fujii Si, Shimizu K, Uslu U, Schuler G, et al. RNA-Transfection of γ/δ T cells with a chimeric antigen receptor or an α/β T-cell receptor: a safer alternative to genetically engineered α/β T cells for the immunotherapy of melanoma. BMC Cancer (2017) 17(1):551. doi: 10.1186/S12885-017-3539-3
140. Marcu-Malina V, Heijhuurs S, van Buuren M, Hartkamp L, Strand S, Sebestyen Z, et al. Redirecting αβT cells against cancer cells by transfer of a broadly tumor-reactive γδT-cell receptor. Blood (2011) 118:50–9. doi: 10.1182/blood-2010-12-325993
141. Kuball J, Dossett ML, Wolfl M, Ho WY, Voss RH, Fowler C, et al. Facilitating matched pairing and expression of TCR chains introduced into human T cells. Blood (2007) 109:2331–8. doi: 10.1182/BLOOD-2006-05-023069
142. van der Veken LT, Coccoris M, Swart E, Falkenburg JHF, Schumacher TN, Heemskerk MHM. Alpha beta T cell receptor transfer to gamma delta T cells generates functional effector cells without mixed TCR dimers. vivo. J Immunol (2009) 182:164–70. doi: 10.4049/JIMMUNOL.182.1.164
143. Straetemans T, Gründer C, Heijhuurs S, Hol S, Slaper-Cortenbach I, Bönig H, et al. Untouched GMP-ready purified engineered immune cells to treat cancer. Clin Cancer Res (2015) 21:3957–68. doi: 10.1158/1078-0432.CCR-14-2860
144. Straetemans T, Kierkels GJJ, Doorn R, Jansen K, Heijhuurs S, dos Santos JM, et al. GMP-grade manufacturing of T cells engineered to express a defined γδTCR. Front Immunol (2018) 9:1062. doi: 10.3389/fimmu.2018.01062
145. Braham MVJ, Minnema MC, Aarts T, Sebestyen Z, Straetemans T, Vyborova A, et al. Cellular immunotherapy on primary multiple myeloma expanded in a 3D bone marrow niche model. Oncoimmunology (2018) 7(6):e1434465. doi: 10.1080/2162402X.2018.1434465
146. Johanna I, Straetemans T, Heijhuurs S, Aarts-Riemens T, Norell H, Bongiovanni L, et al. Evaluating in vivo efficacy-toxicity profile of TEG001 in humanized mice xenografts against primary human AML disease and healthy hematopoietic cells. J Immunother Cancer (2019) 7(1):69. doi: 10.1186/s40425-019-0558-4
147. Shimizu K, Shinga J, Yamasaki S, Kawamura M, Dörrie J, Schaft N, et al. Transfer of mRNA encoding invariant NKT cell receptors imparts glycolipid specific responses to T cells and γδT cells. PloS One (2015) 10(6):e0131477. doi: 10.1371/journal.pone.0131477
148. Mensurado S, Blanco-Domínguez R, Silva-Santos B. The emerging roles of γδ T cells in cancer immunotherapy. Nat Rev Clin Oncol (2023) 20:178–91. doi: 10.1038/S41571-022-00722-1
149. Lopes N, McIntyre C, Martin S, Raverdeau M, Sumaria N, Kohlgruber AC, et al. Distinct metabolic programs established in the thymus control effector functions of γδ T cell subsets in tumor microenvironments. Nat Immunol (2021) 22:179–92. doi: 10.1038/s41590-020-00848-3
150. Li P, Wu R, Li K, Yuan W, Zeng C, Zhang Y, et al. IDO inhibition facilitates antitumor immunity of Vγ9Vδ2 T cells in triple-negative breast cancer. Front Oncol (2021) 11:679517. doi: 10.3389/fonc.2021.679517
151. Jonescheit H, Oberg HH, Gonnermann D, Hermes M, Sulaj V, Peters C, et al. Influence of indoleamine-2,3-Dioxygenase and its metabolite kynurenine on γδ T cell cytotoxicity against ductal pancreatic adenocarcinoma cells. Cells (2020) 9(5):1140. doi: 10.3390/CELLS9051140
152. Sureshbabu SK, Chaukar D, Chiplunkar SV. Hypoxia regulates the differentiation and anti-tumor effector functions of γδT cells in oral cancer. Clin Exp Immunol (2020) 201(1):40–57. doi: 10.1111/cei.13436
153. Dieli F, Zoeller M, Carson WE, Agrati C, Sacchi A, Tumino N, et al. Myeloid-derived suppressor cells specifically suppress IFN-γ production and antitumor cytotoxic activity of Vδ2 T cells. Immunol (2018) 9:1271. doi: 10.3389/fimmu.2018.01271
154. Castella B, Riganti C, Massaia M. Metabolic approaches to rescue antitumor Vγ9Vδ2 T-cell functions in myeloma. Front Biosci - Landmark (2020) 25:69–105. doi: 10.2741/4795
155. Liu Z, Xu X, Liu K, Zhang J, Ding D, Fu R. Immunogenic cell death in hematological malignancy therapy. Advanced Sci (2023) e2207475. doi: 10.1002/ADVS.202207475
156. Mattarollo SR, Kenna T, Nieda M, Nicol AJ. Chemotherapy and zoledronate sensitize solid tumour cells to Vgamma9Vdelta2 T cell cytotoxicity. Cancer Immunol Immunother (2007) 56:1285–97. doi: 10.1007/S00262-007-0279-2
Keywords: Vγ9Vδ2 T cells, immunotherapy, adoptive cell transfer, unconventional T cells, blood cancers
Citation: Giannotta C, Autino F and Massaia M (2023) Vγ9Vδ2 T-cell immunotherapy in blood cancers: ready for prime time? Front. Immunol. 14:1167443. doi: 10.3389/fimmu.2023.1167443
Received: 16 February 2023; Accepted: 31 March 2023;
Published: 18 April 2023.
Edited by:
Paolo Dellabona, San Raffaele Scientific Institute (IRCCS), ItalyReviewed by:
Karin Schilbach, University of Tübingen, GermanyMaciej Zieliński, Medical University of Gdansk, Poland
Copyright © 2023 Giannotta, Autino and Massaia. This is an open-access article distributed under the terms of the Creative Commons Attribution License (CC BY). The use, distribution or reproduction in other forums is permitted, provided the original author(s) and the copyright owner(s) are credited and that the original publication in this journal is cited, in accordance with accepted academic practice. No use, distribution or reproduction is permitted which does not comply with these terms.
*Correspondence: Massimo Massaia, bWFzc2ltby5tYXNzYWlhQHVuaXRvLml0
†These authors have contributed equally to this work and share first authorship