- 1Department of Parasitology, Faculty of Science, Charles University, Prague, Czechia
- 2Laboratório de Biologia Molecular de Parasitas e Vetores, Instituto Oswaldo Cruz - Fiocruz, Rio de Janeiro, RJ, Brazil
- 3Department of Microbiology and Immunology, Chicago Medical School, Rosalind Franklin University of Medicine and Science, North Chicago, IL, United States
Introduction: Sand flies (Diptera: Phlebotominae) belonging to the Lutzomyia genus transmit Leishmania infantum parasites. To understand the complex interaction between the vector and the parasite, we have been investigating the sand fly immune responses during the Leishmania infection. Our previous studies showed that genes involved in the IMD, Toll, and Jak-STAT immunity pathways are regulated upon Leishmania and bacterial challenges. Nevertheless, the parasite can thrive in the vectors’ gut, indicating the existence of mechanisms capable of modulating the vector defenses, as was already seen in mammalian Leishmania infections.
Methods, results, and discussion: In this study, we investigated the expression of Lutzomyia longipalpis genes involved in regulating the Toll pathway under parasitic infection. Leishmania infantum infection upregulated the expression of two L. longipalpis genes coding for the putative repressors cactus and protein tyrosine phosphatase SHP. These findings suggest that the parasite can modulate the vectors’ immune response. In mammalian infections, the Leishmania surface glycoprotein GP63 is one of the inducers of host immune depression, and one of the known effectors is SHP. In L. longipalpis we found a similar effect: a genetically modified strain of Leishmania amazonensis over-expressing the metalloprotease GP63 induced a higher expression of the sand fly SHP indicating that the L. longipalpis SHP and parasite GP63 increased expressions are connected. Immuno-stained microscopy of L. longipalpis LL5 embryonic cells cultured with Leishmania strains or parasite conditioned medium showed cells internalization of parasite GP63. A similar internalization of GP63 was observed in the sand fly gut tissue after feeding on parasites, parasite exosomes, or parasite conditioned medium, indicating that GP63 can travel through cells in vitro or in vivo. When the sand fly SHP gene was silenced by RNAi and females infected by L. infantum, parasite loads decreased in the early phase of infection as expected, although no significant differences were seen in late infections of the stomodeal valve.
Conclusions: Our findings show the possible role of a pathway repressor involved in regulating the L. longipalpis immune response during Leishmania infections inside the insect. In addition, they point out a conserved immunosuppressive effect of GP63 between mammals and sand flies in the early stage of parasite infection.
Introduction
Insects have several immune-related mechanisms to control commensal or potentially harmful microorganisms. Similar to mammals, their innate immunity involves cellular and humoral responses that are often tuned by different signaling pathways (1). The Toll and Janus kinase/signal transducer and activator of transcription (Jak-STAT) are among the most studied pathways in insects (2–4). They are activated by pathogen-associated molecular patterns (PAMPs) that bind to the host cell membrane receptors (5, 6). The Toll pathway activation requires first the binding PAMPs to the Spätzle ligand, and this complex subsequently binds to the Toll receptor (5, 7). The Jak-STAT pathway is activated when the unpaired (upd) family of ligands bind to the receptor domeless (dome) (2, 8). When these receptors are activated, a cascade of intracellular molecular events involving regulatory proteases and kinases results in the translocation of transcription factors to the nucleus. These events culminate with the transcription of genes associated with pathogen-killing effectors such as antimicrobial peptides (AMPs) and cytokines (2–4). Such activation can occur within minutes after an injury or microbial challenge.
Besides the pathway activation steps, other regulatory molecules can repress the downstream events of the immune response. These molecules can inhibit the pathway activity by blocking key molecular processes (9). For example, in Drosophila, the Toll pathway repressor cactus binds to nuclear factor kappa B (NF-κB) transcription factors preventing their translocation to the cell nucleus (10). For the Jak-STAT pathway, protein tyrosine phosphatases (PTPs) such as PTP16F (11) and sarcoma homology 2 (SH2)-domain-containing PTP (SHP) (12) repress Jak activity by dephosphorylation (13, 14). These regulatory mechanisms are crucial to prevent an uncontrolled response that may pose a high physiological cost (15).
This complex regulation of the innate immunity in insect vectors of public health importance gained significant attention (16–18). In Aedes aegypti, besides the response against bacteria and fungi, the Toll pathway was shown to be involved in the antiviral response against dengue virus (DENV) (19) and sindbis virus (20). Also, in Anopheles mosquitoes, Toll regulates the expression of the AMP gambicin against Plasmodium parasite (21). Moreover, the Jak-STAT pathway mediates resistance to DENV and ZIKV in the vector A. aegypti. Interestingly, the activation of this pathway through the knockdown of PIAS, one of the negative regulators, decreased the DENV infection (22, 23). The Jak-STAT pathway was also involved in responses to Plasmodium by Anopheles aquasalis (24, 25) and, in the case of Anopheles gambiae, with the transcriptional activation of nitric oxide synthase (NOS) (24).
In vectors of trypanosomatids such as tsetse flies, antimicrobial peptides were identified in the Glossina morsitans hemolymph after bacterial intrathoracic injection and after Trypanosoma brucei brucei oral infection (26). In triatomine kissing bugs, the successful infection of Rhodnius prolixus by Trypanosoma cruzi Dm 28c strain increased the expression of a defensin. In contrast, the T. cruzi Y strain did not complete the infection cycle in the insect and did not elicit AMPs expression (27). These examples show that trypanosome infections trigger their vectors’ immune response. Some components of the main pathways involved in the innate immune response have already been identified in sand flies. Toll, Jak-STAT and immune deficiency (IMD) gene expression in L. longipalpis Lulo and LL5 cell lines indicated that repressors of these pathways (cactus, caspar, and PIAS), as well as their transcription factors (dorsal, relish, and STAT), are differentially modulated depending on the microbial challenges (28–30).
In female L. longipalpis, activating the IMD pathway through the silencing of the repressor caspar favors Leishmania mexicana infection (31). Analogously, suppressing this pathway through the knockout of the pathway transcription factor Relish favored Leishmania major and bacteria growth in Phlebotomus papatasi (32). On the other hand, the Jak-STAT pathway regulators, such as PIAS and STAT showed no significant modulation when sand flies were infected by L. infantum (syn. Leishmania infantum chagasi). Moreover, the silencing of STAT caused the downregulation of an inducible NOS, and this effect was associated with increased L. infantum detection (30). These studies show that sand fly immunity affects the outcome of the parasite infection.
Although transcriptomic analysis indicated that Leishmania infection resulted in small overall transcriptional changes (33, 34), our previous results indicated that Leishmania experimental infection causes a broad spectrum of immune responses. For instance, the L. mexicana infection downregulated the expression of a L. longipalpis defensin gene in late phases of parasitic infection (35). On the other hand, when this sand fly was infected by L. infantum, there was an upregulation of three other AMPs in an earlier phase of infection (36). In addition, a gut-specific defensin was increased in P. papatasi depleted of gut bacteria and infected with L. major (37). Moreover, L. i. chagasi infection of L. longipalpis increased the expression of an activin-like gene that belongs to the Transforming Growth Factor-beta (TGF-β) family. Its suppression by RNAi caused a decline in parasite survival (38), suggesting that the parasite benefits from the sand fly expression of the TGF-β pathway.
There is evidence that Leishmania can modulate gene expression of immune regulatory molecules in L. longipalpis embryonic cells. Co-culture of L. infantum and LL5 cells caused the upregulation of PIAS and PTP61F genes associated with the Jak-STAT pathway’s repression (30). Lessons learned from Leishmania-vertebrate host studies show that parasite virulence factors can be responsible for modulating the host immune response. For example, glycosylinositol phospholipids (GIPLs), lipophosphoglycans (LPGs), proteophosphoglycans (PPGs), and the glycoprotein GP63 are among the Leishmania molecules involved in the host cell invasion and maintenance of chronic infection (39, 40). In fact, Leishmania GP63, a metalloprotease, activates the murine SHP-1, which represses the Jak-STAT (41) and Toll (42) pathways.
We hypothesized that, similar to the mammalian infection model, a sand fly immunity pathway repressor could be the target of a Leishmania virulence factor such as GP63. To address this possibility, we focused on Toll-related pathway genes. We selected two L. longipalpis genes, cactus and SHP-2, coding for repressors involved in the Toll pathway and explored their expression under Leishmania infection conditions. We used RNAi-mediated gene silencing to test the role of SHP-2. In addition, we tested whether the parasite GP63 interfered with the sand fly immunity and whether it could be internalized by L. longipalpis cells. In the present study, we report on the sand fly expression of SHP-2 and its possible connection with a parasite virulence factor.
Materials and methods
Gene identification
L. longipalpis partial coding sequences for the Toll pathway repressor cactus were previously identified (28). Partial coding sequences for an additional repressor associated with the Toll pathway SHP-2 were identified from the National Center for Biotechnology Information (NCBI) (Bethesda, MD, USA) dbESTs database (43) and the L. longipalpis genome available at VectorBase database (44). Similar sequences from Drosophila, Aedes, and Culex were used as a query on blastx and tblastx search against these databases. The obtained sand fly sequences were reversely checked using the same programs against the NCBI non-redundant (nr) protein sequences database (45). The OrthoMCL search tool (46) was used for homology confirmation. The InterPro (47) and the NCBI Conserved Domain Database (48) were used to identify conserved domains to support the sequences’ identity.
Similarities of L. longipalpis cactus and SHP-2 amino acid sequences shared with other insects were assessed by MUSCLE multiple sequence alignments (49) built-in Geneious 7.1.9 software (Biomatters, New Zealand). Cladograms were created using MEGA-X software (50) with the maximum likelihood method. According to the lowest Bayesian Information Criterion (BIC) score, the best substitution model was estimated using MEGA X software. Cactus analysis was done using the Jones-Taylor-Thorton (51), while SHP-2 analysis was done using the Le-Gascuel (52) substitution models. Evolutionary rates among sites were modeled using a Gamma distribution with invariable sites for the phylogeny analyses. A bootstrap of 400 replications was used to model evolutionary rate differences among sites.
Sand fly and parasite cell cultures
L. longipalpis embryonic LL5 cells (53) were grown in L-15 medium (Sigma, USA) supplemented with 10% heat-inactivated fetal bovine serum (HI-FBS) (Cultilab, Brazil), 10% tryptose phosphate broth (TPB) (Sigma), and 1% antibiotics (penicillin 100 U/mL and streptomycin 100 mg/mL) (Sigma).
Leishmania amazonensis (MPRO/BR/72/M1845/LV78) transfected with P6.5/1.9R and P6.5/1.9 for knockdown and over-expression of GP63 metalloprotease, respectively (54) were maintained at 25°C in medium 199 (Thermo Fisher Scientific, USA), pH 7.0, supplemented with 10% HI-FBS under the selective pressure of tunicamycin (10 µg/mL) (Sigma). Wild-type L. amazonensis (BMVirWT) promastigotes were maintained in the same medium without tunicamycin.
L. infantum (syn. L. i. chagasi) (MHOM/BR/1974/PP75) obtained from the Leishmania collection of Instituto Oswaldo Cruz (CLIOC - Fiocruz/IOC, Brazil) was maintained at 25°C in medium 199, pH 7.0, supplemented with 10% HI-FBS. Promastigotes of L. (Viannia) braziliensis (MHOM/BR/75/M2904) were grown at 26°C in Schneider’s Drosophila medium (Sigma) supplemented with 10% heat-inactivated fetal bovine serum (FBS), 1% glutamine, 100 U/ml penicillin, and 100 mg/ml streptomycin (55).
Leishmania exosome purification
L. infantum culture was initiated with 106 parasites/mL and grown for three days. Parasites were pelleted by centrifugation at 1,500 x g for 10 min at 4 °C and washed three times with non-supplemented medium 199. Parasites were resuspended in fresh medium 199 supplemented with TPB instead of FBS and grown for 24 h at 26 °C.
For exosome recovery, cultures were submitted to differential centrifugation at 4 °C as follows: 300 x g for 10 min to remove live parasites, 2,000 x g for 10 min to remove dead parasites, 10,000 x g for 30 min to remove cellular debris, and finally, ultracentrifugation at 100,000 x g for 60 min to pellet exosomes. Exosome pellets were resuspended in ice-cold phosphate buffered saline (PBS, pH7.4), washed, and submitted to additional ultracentrifugation (56). The final exosome pellets were resuspended in PBS and used in incubation with LL5 cells or sand fly feeding procedures. Protein quantification of exosomes was performed using the Pierce 600nm colorimetric assay. To note, in our previous proteomic analysis of L. infantum exosomes prepared using this protocol (56), 50 out of 53 exosome markers that have homologues to the ExoCarta database were revealed (57).
Incubation of LL5 cells with Leishmania
LL5 cells were cultured in a 24-well flat-bottom plate at a density of 5 x 105/well in 500 μL of L-15 media supplemented with 10% TPB and 1% antibiotics (penicillin 100 U/mL and streptomycin 100 mg/mL) (Sigma) for 24 h at 30 °C to allow cell attachment to the bottom of the wells. Cells were washed 3x with fresh L-15 medium, L-15 medium containing Leishmania parasites at a 10 to 1 parasite/LL5 cell ratio was added to the wells, and plates were incubated for one hour at 30 °C. LL5 cells cultured under the same conditions without parasites were used as control. Plates were subsequently used for visualization of Leishmania GP63 by immunofluorescent microscopy.
Incubation of LL5 cells with Leishmania conditioned medium
Leishmania conditioned medium was obtained from 7 days cultures after clearance by centrifugation at 2,000 x g for 10 min. LL5 cells were cultured in a 24-well flat-bottom plate as described above. After the cell wash, Leishmania conditioned medium was added to the LL5 cells-containing wells and incubated for one hour at 30 °C. Plates were subsequently used for Leishmania GP63 immunofluorescent microscopy. Fresh 199 medium was used instead of Leishmania conditioned medium as the control.
Lutzomyia longipalpis colony
Sand fly colony was previously established from L. longipalpis originally collected in Jacobina, BA, Brazil, and kept at temperatures between 24-28 °C and 70-80% relative humidity under standard insectary conditions (58). For daily colony maintenance, adult insects were allowed to feed on 50-70% sucrose solution ad libitum, and females were blood-fed once a week on anesthetized hamsters or mice. The use of all animals was reviewed and approved by the Committee on the Ethics for the use of Animals in the Institute Oswaldo Cruz (CEUA-IOC) under permission No. CEUA/IOC-005/2019 and the Committee on the Ethics of Laboratory Experiments of Charles University under permission No. MSMT-8604/2019-6.
Lutzomyia longipalpis artificial feeding with Leishmania exosomes
Three to 6 days old female sand flies were fed through a chickskin membrane, using a Hemotek system, with heat-inactivated rabbit blood (under permission No. CEUA/IOC-005/2019) seeded with Leishmania exosomes at 40 μg/mL. Sand flies fed on blood without exosomes were used as control groups. Only fully engorged females from both experimental and control groups were used.
Lutzomyia longipalpis artificial infection with Leishmania
Sand flies were artificially fed as described on heat-inactivated rabbit blood seeded with L. infantum or L. amazonensis (107 parasites/mL of blood) obtained from exponential growth culture. Sand flies fed on blood without parasites were used as control groups. Fully engorged females were separated and collected at different times post-infection for RNA extraction and microscopy analysis. A 70% sucrose meal was offered ad libitum after blood feeding. Refer to figure legends for more details.
RNA extraction and cDNA synthesis
RNA was extracted from pools of 10 females by homogenization per manufacturer’s instructions in TRIzol reagent (Thermo Fisher Scientific) and stored at -80 °C until used. RNase-free DNase I (Thermo Fisher Scientific) digestion step was added to remove DNA. The cDNA synthesis was done using SuperScript III Reverse Transcriptase (Thermo Fisher Scientific) using up to 1 μg of total RNA as template following the manufacturer’s instructions.
RNAi-mediated gene silencing
The L. longipalpis SHP-2 gene was submitted to RNAi-mediated gene silencing experiments. Gene-specific primers (dsSHP2-F and dsSHP2-R) coupled to T7 promotor sequence (Table 1) were designed to amplify DNA templates for dsRNA synthesis reactions. A DNA template was amplified from p-GEM-T Easy plasmid (Promega, USA) with dsLacZ-F and dsLacZ-R primers (Table 1) to produce a control dsRNA. The templates were amplified by a touchdown PCR under the following cycling conditions: 95 °C for 3 min; 16 cycles of 95 °C for 45 sec, 68 to 50 °C (progressively decreasing 1°C per cycle) for 45 sec, and 72°C for 45 sec; 26 cycles of 95 °C for 45 sec, 50 °C for 45 sec, and 72 °C for 45 sec; 72 °C for 3 min.
SHP-2 and LacZ templates were used in dsRNA synthesis reaction by MEGAscript RNAi kit (Thermo Fisher Scientific) following the manufacturer’s instructions. The produced dsRNA was concentrated to 4.5 μg/μL, and 32.2 nL were microinjected intrathoracically into L. longipalpis females using Nanoject II microinjector (Drummond, USA) (62). The dsRNA injected flies were kept under colony maintenance conditions with sucrose feeding and used on the following day for Leishmania infection experiments. Refer to figure legends for more details.
Relative gene expression
The relative expression of selected genes was assessed by qPCR using L. longipalpis cDNA samples from various samples as described under different experimental settings. Gene-specific primer sets used for qPCR are listed in Table 1. Cycling conditions followed manufacturer’s standard procedures using SYBR Green PCR Master Mix in a 7500 Real-Time PCR System (Applied Biosystems, USA). Specific gene expression was calculated relative to a ribosomal protein (RP49) reference gene (28) and expressed in fold-change values compared to a control group defined according to each experimental design. Refer to figure legends for more details.
Immunofluorescence detection of Leishmania GP63
Midguts from artificially blood-fed L. longipalpis females were dissected in PBS at 2 h and 24 h post-feeding, and the ingested bloodmeal content was removed. Midguts were incubated in 4% paraformaldehyde (PFA) for 30 min, transferred to 0.5% triton X-100 for 20 min, and to 3% BSA for 40 min. The midguts were then washed 3x in PBS for 10 min and incubated with primary anti-GP63 antibody (1:5,000 dilution) in a 1% BSA, 0.25% triton X-100 solution overnight at 4 °C. Midguts were subsequently washed 3x in PBS for 10 min and incubated with goat anti-rabbit IgG (H+L) Cross-Adsorbed Secondary Antibody, Alexa Fluor™ 546 (Thermo Fisher Scientific) diluted in 1% BSA, 0.25% triton X-100 solution for 1 hour. After additional 2x wash steps in PBS for 10 min samples were incubated with DAPI for 10 min followed by a final 1x wash in PBS for 10 min. Midguts were transferred to glass slides for subsequent confocal microscope analysis using a Leica DMi8 confocal microscope (Leica, Germany).
Leishmania development in sand fly guts
Eight days post-infection, Leishmania loads, location, and development were assessed by light microscopy in L. longipalpis females silenced for SHP-2 and LacZ. A minimum of 20 sand fly guts from each group were examined. Sand flies were anesthetized on ice and transferred to a saline solution (0.9% NaCl) for dissection of the guts. Parasite loads were estimated under 40x magnification objective lens and classified as low (below 100 parasites), medium (between 100 and 1,000 parasites), or heavy infection (above 1,000 parasites) (63). Simultaneously, localization of parasites in various portions of the sand fly midgut was recorded (63, 64).
To assess the parasite developmental stages, sand fly gut smears were prepared on glass slides and Giemsa-stained. The glass slides were inspected using an Olympus BX51 microscope (Olympus, Japan) under a 100x magnification objective lens. Images of 400 randomly selected Leishmania promastigotes were captured from two independent dsRNA injected sand fly groups. The width and length of cells and their flagellum were measured using the microscope scale plugin in ImageJ 1.52a software (65). Different categories of parasites were defined as elongated nectomonads (body length ≥ 14 μm), procyclic promastigotes (body length < 14 μm and flagellar length ≤ body length), metacyclic promastigotes (body length < 14 μm and flagellar length ≥ 2x body length), and leptomonads (remaining parasites) (64, 66).
Statistical analysis
Ordinary two-way ANOVA with Sidak’s correction for multiple comparisons test was used to calculate significant differences in gene expression levels obtained by qPCR. This same method was applied to assess significant differences in infection estimation, localization, and parasite stages from data obtained by light microscopy observations. The statistical analysis was carried in GraphPad Prism software (version 6.07) (GraphPad Software Inc., USA).
Results
Lutzomyia longipalpis immunity gene sequences
Cactus gene sequences were previously identified (28). The additional L. longipalpis coding sequences of SHP-2 (GenBank KP030756) were deposited in the NCBI GenBank database. We analyzed sequences of the two Toll-related repressors selected in the present work. The deduced amino acid sequence of cactus contains a domain of six ankyrin repeats present in the inhibitor of NF-κB (I-κB) family (67, 68). The phylogenetic analysis showed that the L. longipalpis sequence formed a group with a P. papatasi sequence and was included in a larger group of I-κB sequences from dipterans such as Drosophila, Bactrocera, and Rhagoletis flies. The vertebrate I-κB formed an outgroup composed of I-κBα, I-κBβ, and I-κBε sequences (Figure S1).
The deduced amino acid sequence of SHP-2 (GenBank AKU77025) contains the catalytic domain of tyrosine-protein phosphatase non-receptor type 11 and type 6 (PTPn11 and PTPn6) (CDD cd14544), which contains the sarcoma homology 2 (SH2) (IPR000980) and tyrosine-specific protein phosphatase PTPase (IPR000242) domains. The PTPn11 and PTPn6 are also known as SHP-2 and SHP-1, respectively. The phylogenetic analysis showed that the L. longipalpis SHP-2 amino acid sequence formed a separate branch from the mosquito SHP-2/PTPn11 sequences. It is included in the larger group of dipterans together with Drosophila, Musca, and Stomoxys corkscrew sequences. The vertebrate PTPn6 sequences formed an outgroup (Figure S2).
Expression of Toll pathway regulators during sand fly Leishmania infection
We chose an experimental setting representing a potential challenge to the sand fly’s immunity and investigated the gene expression of the two Toll-related repressors, cactus and SHP-2. Considering that in the mammalian model Leishmania infection of macrophages modulates their immune response (41, 42), we tested and proved the hypothesis that the sand fly repressors cactus and SHP-2 were also modulated under the same infectious conditions. Our results showed that the transcription of cactus and SHP-2 increased on the second day post-infection (Figures 1A, B).
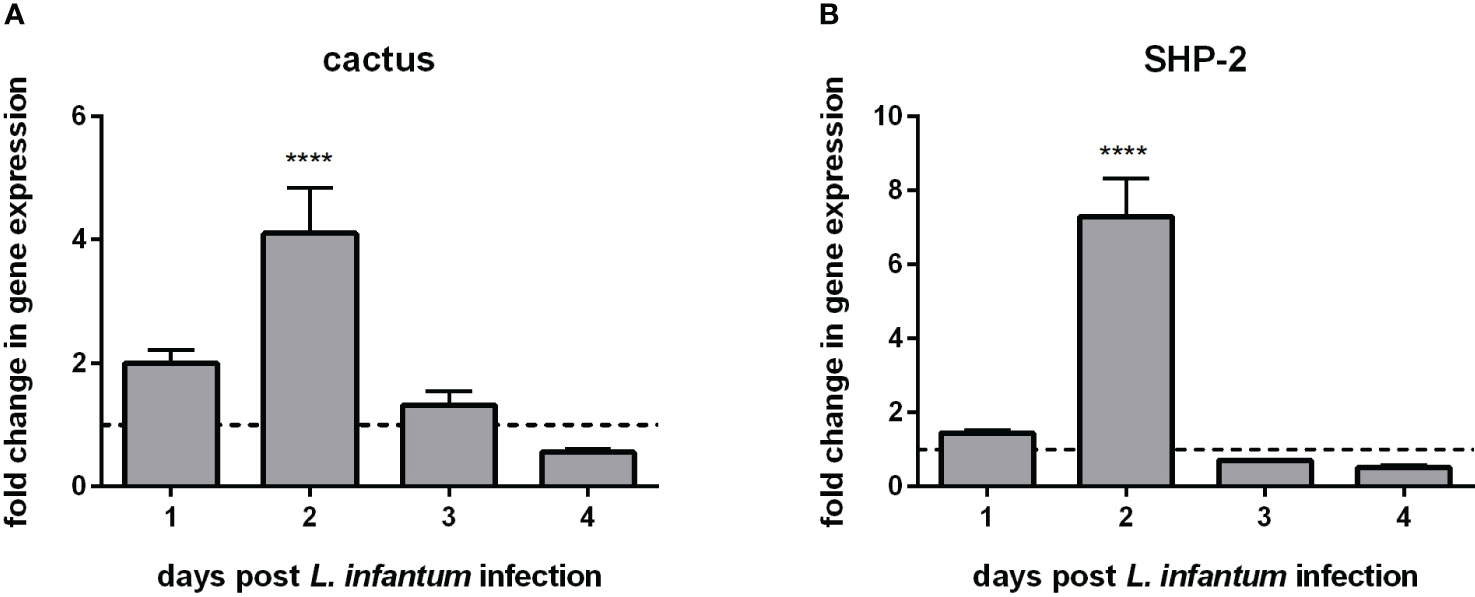
Figure 1 Gene expression of Toll-related repressors in L. longipalpis infected with L. infantum: (A) expression of cactus and (B) expression of SHP-2. Relative gene expression was calculated compared to the endogenous reference gene RP49 and expressed as fold change (y-axis) compared to the non-infected control group (horizontal dotted line). Samples of experimental and control groups were collected in pools of 10 sand flies on days 1, 2, 3, and 4 post-Leishmania infection (x-axis). Vertical bars represent the mean with standard error (SEM) of 3 biological replicates. The differences were significant, as calculated using two-way ANOVA (**** p < 0.0001).
Expression of SHP-2 in L. longipalpis infected with GP63-over and under-expressing Leishmania
The gene expression analysis of L. infantum-infected sand flies indicated that such infection increased sand fly immunity repressors. In macrophages, the Leishmania GP63 metalloprotease is one of the molecules responsible for suppressing the mammalian host immune response (41, 42). We showed a similar action of this protease in sand fly females by infecting them with strains of L. amazonensis showing up- and down-regulated expression of GP63. SHP-2 expression was elevated in the sand flies infected with overexpressing GP63 parasites in relation to infections with Leishmania with down-regulated GP63 (Figure 2).
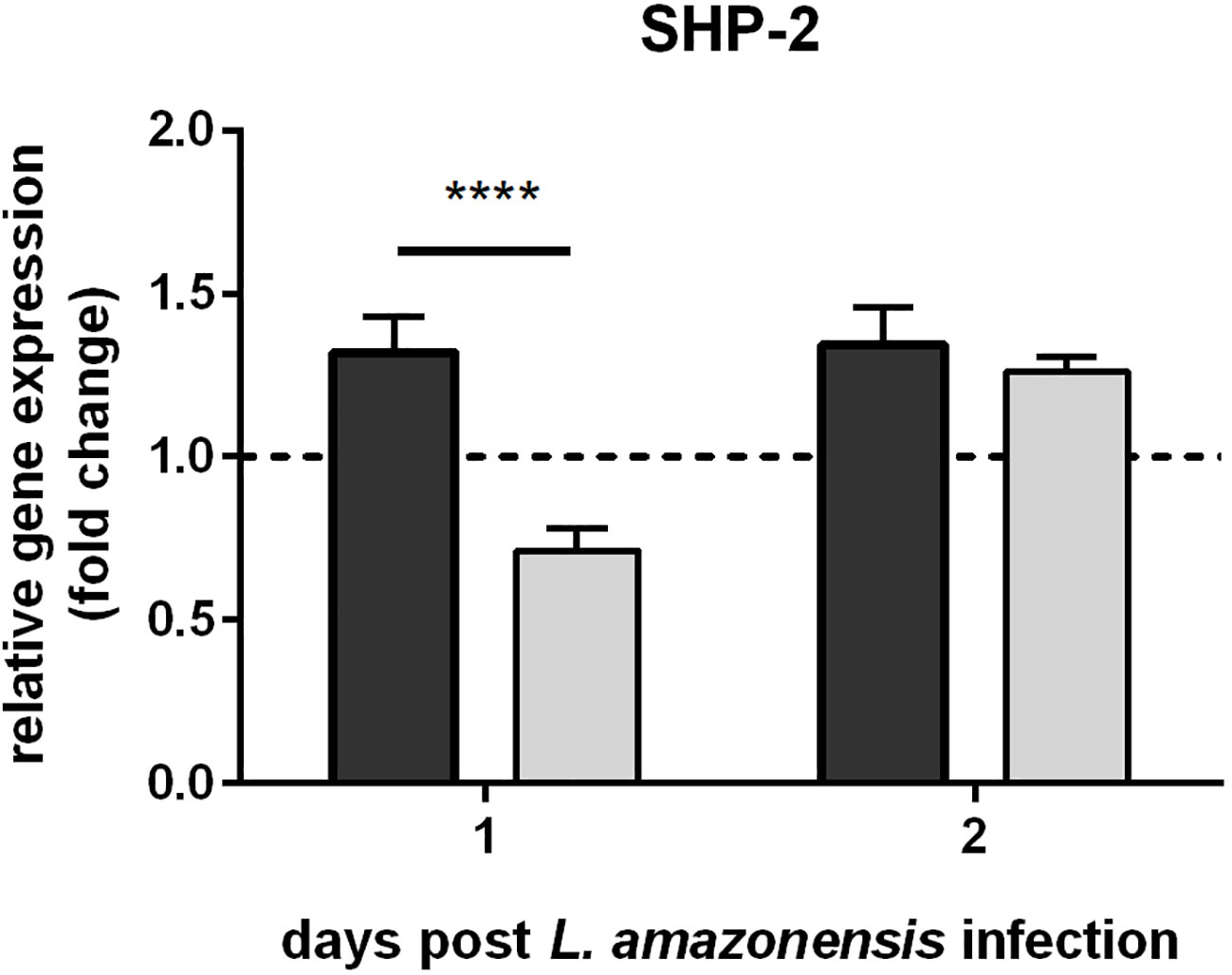
Figure 2 SHP-2 gene expression in L. longipalpis infected with L. amazonensis up- and down-regulated expression of GP63: SHP-2 relative gene expression was calculated with reference to the constitutively expressed ribosomal gene RP49 and expressed as fold change (y-axis) in comparison to the control group infected with wild type L. amazonensis strain (horizontal dotted line). Dark and light grey bars represent SHP-2 expression in sand flies infected with GP63-overexpressing and -underexpressing L. amazonensis, respectively. RNAs were isolated from a pooled ssample of 10 sand flies for each group on days 1 and 2 post-Leishmania infection (x-axis). The mean with standard error (SEM) shown was calculated from 3 biological replicates in each group. Statistical significance was calculated using two-way ANOVA (**** p < 0.0001).
Uptake of GP63 from Leishmania by sand fly cells in vitro
The uptake of GP63 was demonstrated by co-culture of L. longipalpis LL5 cells with three different Leishmania spp., i.e. L. amazonensis, L. braziliensis, and L. infantum. By immunofluorescence microscopy using an anti-GP63 antibody, the association of GP63 with LL5 cells was detected only when they were co-cultured for one hour with any of the three Leishmania species (La, Lb, and Li) used, but not with medium alone as control (Ctr) (Figure 3).
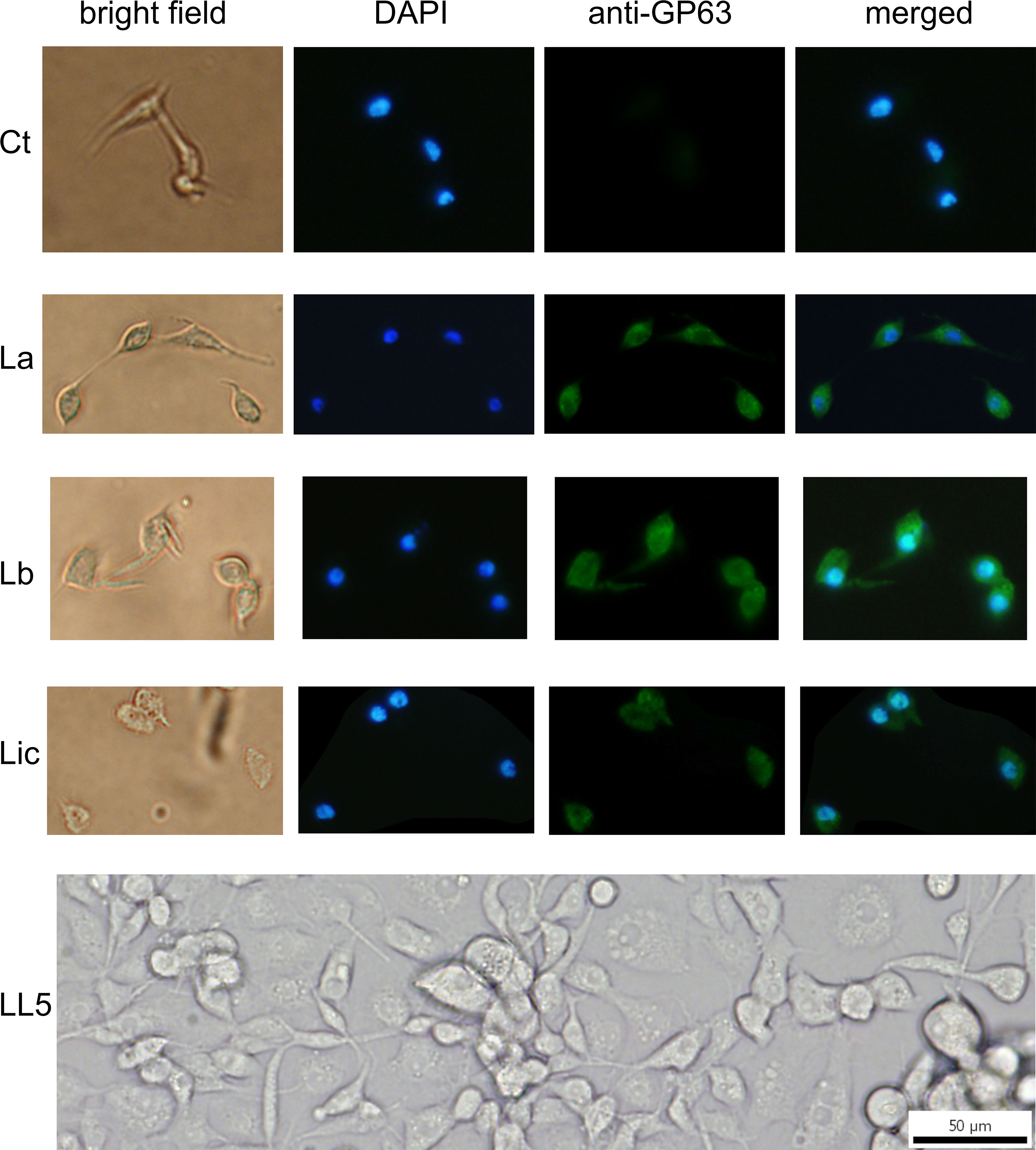
Figure 3 GP63 detection in LL5 cells after co-culture with Leishmania parasites: L. longipalpis LL5 embryonic cells were co-cultured for one hour at 30 °C with each of the three different Leishmania species separately. These cells were submitted to fluorescent microscopy after incubation with DAPI and anti-GP63 FITC-antibody. Ctr – LL5 control culture without Leishmania; La – L. amazonensis; Lb – L. braziliensis; Li – L. infantum. Bright field, DAPI (blue), anti-GP63 antibody (green), and merged images were aligned vertically. LL5 – control cell culture.
Association of GP63 with LL5 cells after incubation with Leishmaniaconditioned medium
Association of Leishmania GP63 with LL5 cells was also found under exactly the same experimental conditions as described for Figure 3, except that Leishmania conditioned or spent media were used instead of Leishmania cells (Figure 4). This observation is consistent with the previous finding that Leishmania promastigotes shed GP63 into their culture medium (56).
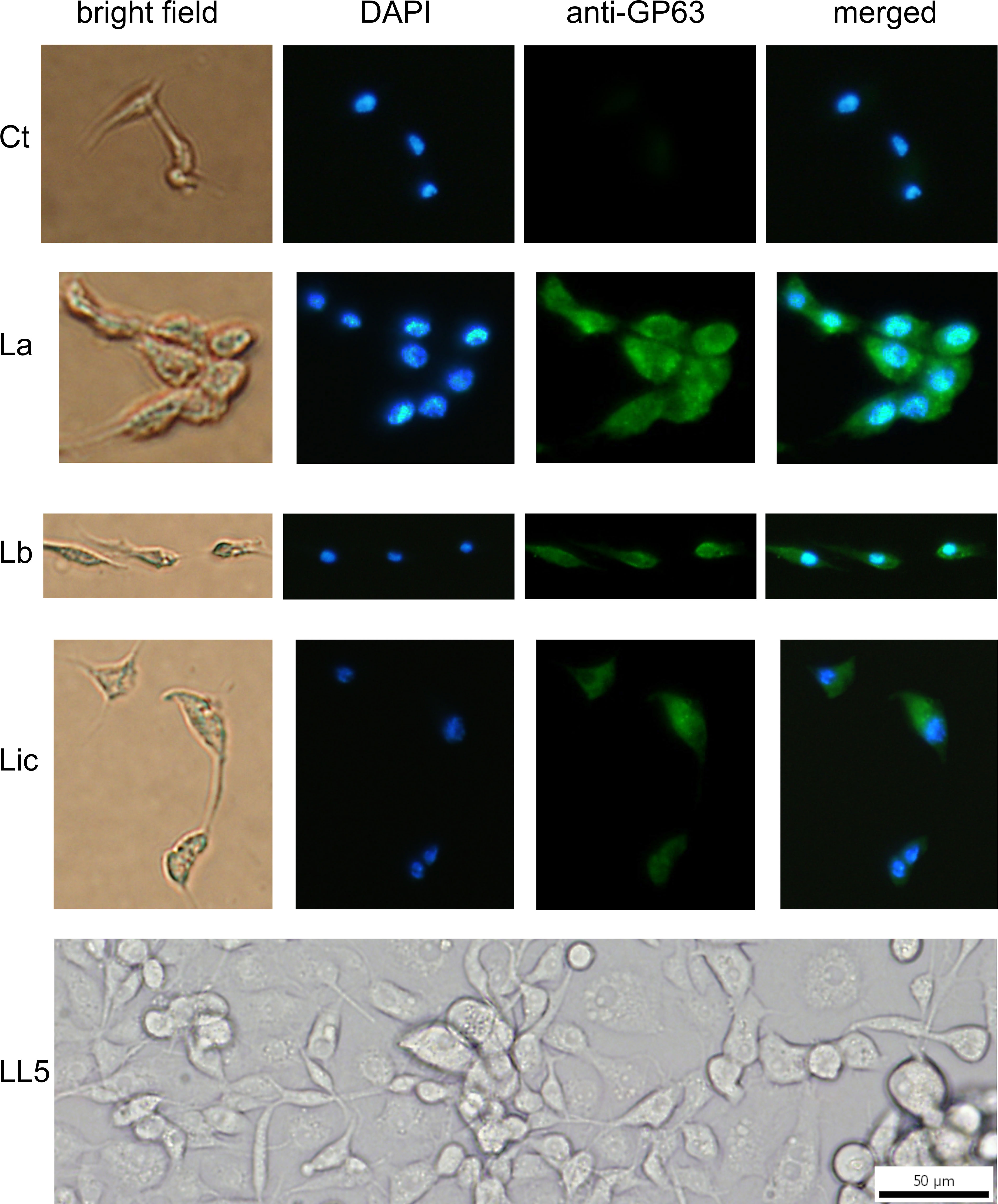
Figure 4 GP63 detection in LL5 cells after incubation with Leishmania conditioned medium: L. longipalpis LL5 embryonic cells were incubated for one hour at 30 °C with conditioned medium from each of the three different Leishmania species. Fluorescent microscopy with DAPI and anti-GP63 antibody was as described. Ctr – LL5 control without Leishmania conditioned medium; La – L. amazonensis; Lb – L. braziliensis; Li – L. infantum. Bright field, DAPI (blue), anti-GP63 antibody (green), and merged images were aligned vertically. LL5 – control cell culture.
Localization of GP63 in L. longipalpis gut after feeding on blood containing L. infantum exosomes, parasites or conditioned medium
Our findings of Leishmania GP63’s association with LL5 cells in vitro led us to examine this in vivo in the midgut cells of female sand flies fed with three different blood meal mixtures: a) blood containing L. infantum exosomes; b) blood containing L. infantum parasites; c) blood containing L. infantum conditioned medium. The artificial feeding with blood containing exosomes or Leishmania showed that GP63 was detected in midguts dissected 2 h after feeding and largely cleared from the gut lumen by 24 h post-feeding. Although less intense, GP63 was also detected in the guts of sand flies that ingested the conditioned medium. Additionally, GP63 can be detected as traversing the midgut epithelium, colocalizing with the midgut muscular fibers and small vesicular structures. A similar GP63 localization was also observed in midguts exposed only to parasites (Figure 5).
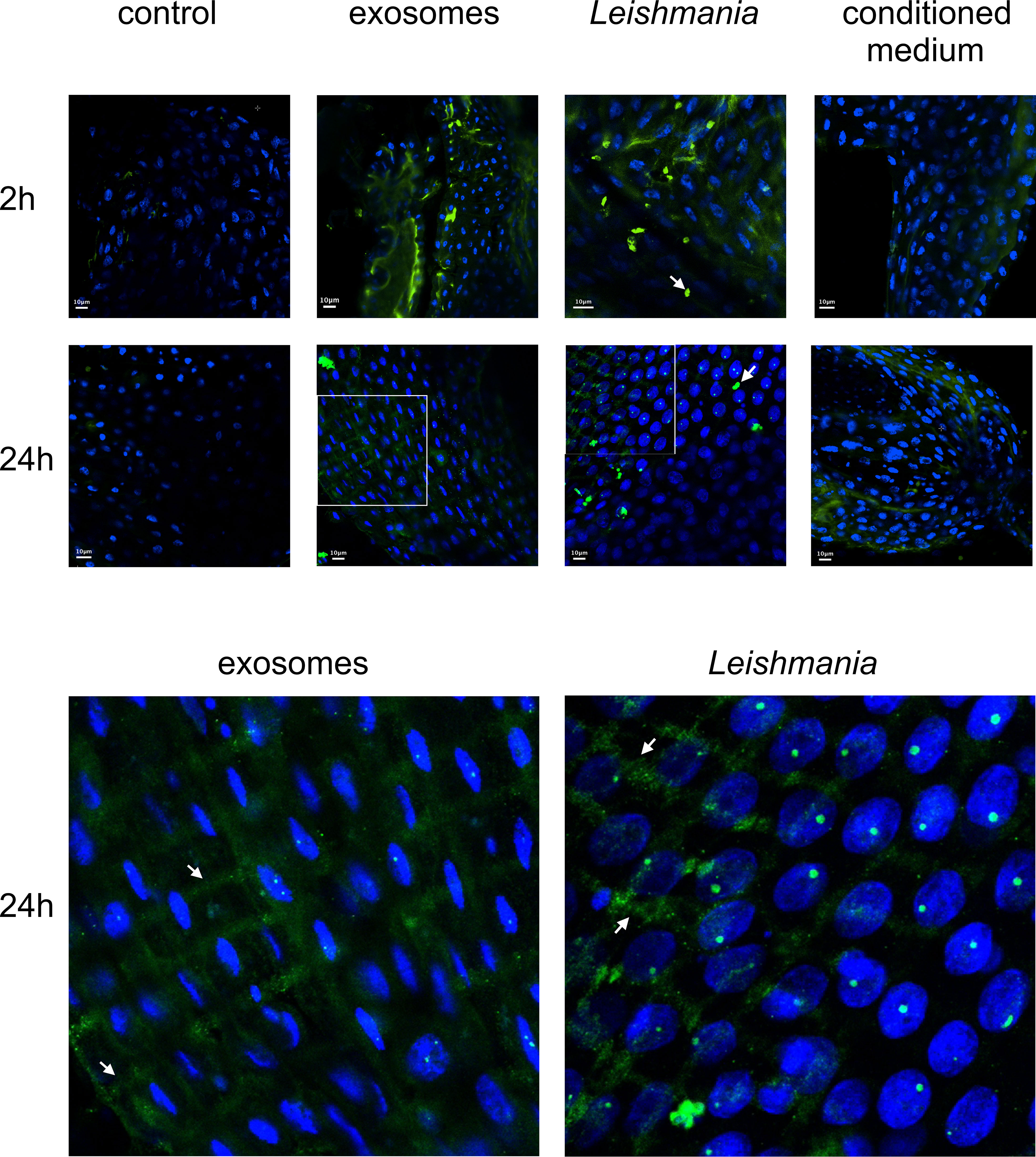
Figure 5 Confocal microscope images of midguts from sand flies fed on blood containing L. infantum exosomes, parasites, or conditioned medium: Midguts were dissected at 2 h or 24 h after artificial blood-feeding (top section) with blood only (control), blood seeded with L. infantum exosomes (exosomes), L. infantum parasites (Leishmania), and L. infantum conditioned medium (conditioned medium). Parasites are indicated by white arrows (top section). Criss-cross midgut muscle and small sand fly vesicular structures are indicated by white arrows in the 4.25 times zoomed images from 24 h guts (exosomes and Leishmania) (bottom section). Green = FITC-anti-GP63 antibody, Blue = DAPI.
Silencing of SHP-2 in sand flies reduces their Leishmania infection
The foregoing data presented led us to suppress the expression of SHP-2 by RNAi-mediated gene silencing in sand flies for testing their susceptibility to Leishmania infection. Injection of SHP-2 dsRNA successfully reduced the SHP-2 gene expression most significantly on day 1 followed by its gradual recovery in the next 2 days (Figure 6A). L. infantum infection of the SHP-2 dsRNA injected group was increasingly suppressed for 3 days and did not return to the level of the control group until day 8 (Figure 6B).
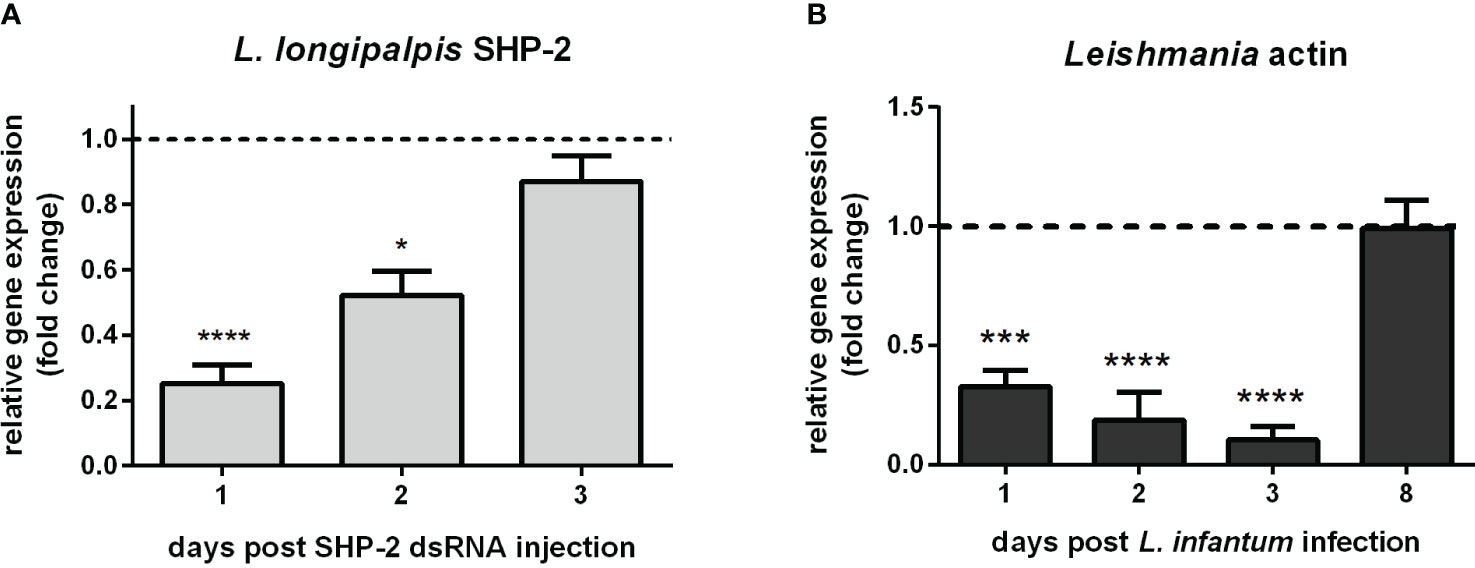
Figure 6 L. longipalpis SHP-2 and Leishmania actin gene expression in SHP-2-silenced and L. infantum-infected females: (A) SHP-2 gene expression in SHP-2 dsRNA injected sand flies. Relative gene expression was calculated compared to the endogenous reference gene RP49 and expressed as fold change (y-axis) compared to the control group injected with LacZ dsRNA (horizontal dotted line); (B) parasite detection through the relative expression of Leishmania actin gene in SHP-2 silenced and L. infantum-infected sand flies. Relative gene expression was calculated with reference to the endogenous reference gene RP49 and expressed as fold change (y-axis) by comparison to the control group injected with LacZ dsRNA and infected with Leishmania (horizontal dotted line). Samples of experimental and control groups were collected in pools of 10 whole sand flies on day 1, 2, 3, and 8 post-parasite infection (x-axis). Vertical bars represent the mean with standard error (SEM) of 3 biological replicates. Significant differences were calculated using two-way ANOVA (* p< 0.05; *** p< 0.001; **** p < 0.0001).
Leishmania development in sand flies after transient RNAi-silencing of their SHP-2
The parasite detection by qPCR indicated that the SHP-2 gene silencing decreased Leishmania numbers in the early phase of infection. The assessed amount of parasite was not significantly changed in the SHP-2 silenced group later in the parasitic infection. Nevertheless, the initial parasite reduction could cause changes in the gut colonization and parasite differentiation progress. We estimated the infection load, assessed the parasite localization in L. longipalpis gut, and evaluated the Leishmania morphology 8 days post-infection. At this time point, as infection advances and debris of blood digestion are excreted, parasites should be detectable in several parts of the sand fly gut, and the infective forms of the parasite become abundant. No significant differences were detected in infection levels in SHP-2-dsRNA injected sand flies compared to the LacZ-dsRNA injected control group (Figure 7A). There was a quite variable, thus non-significant detection of parasites in the stomodeal valve in SHP-2-dsRNA injected groups (Figure 7B). We also evaluated the parasite morphology in Giemsa-stained gut smears, and no significant differences were observed in parasite developmental forms between the dsRNA-injected groups (Figure 7C).
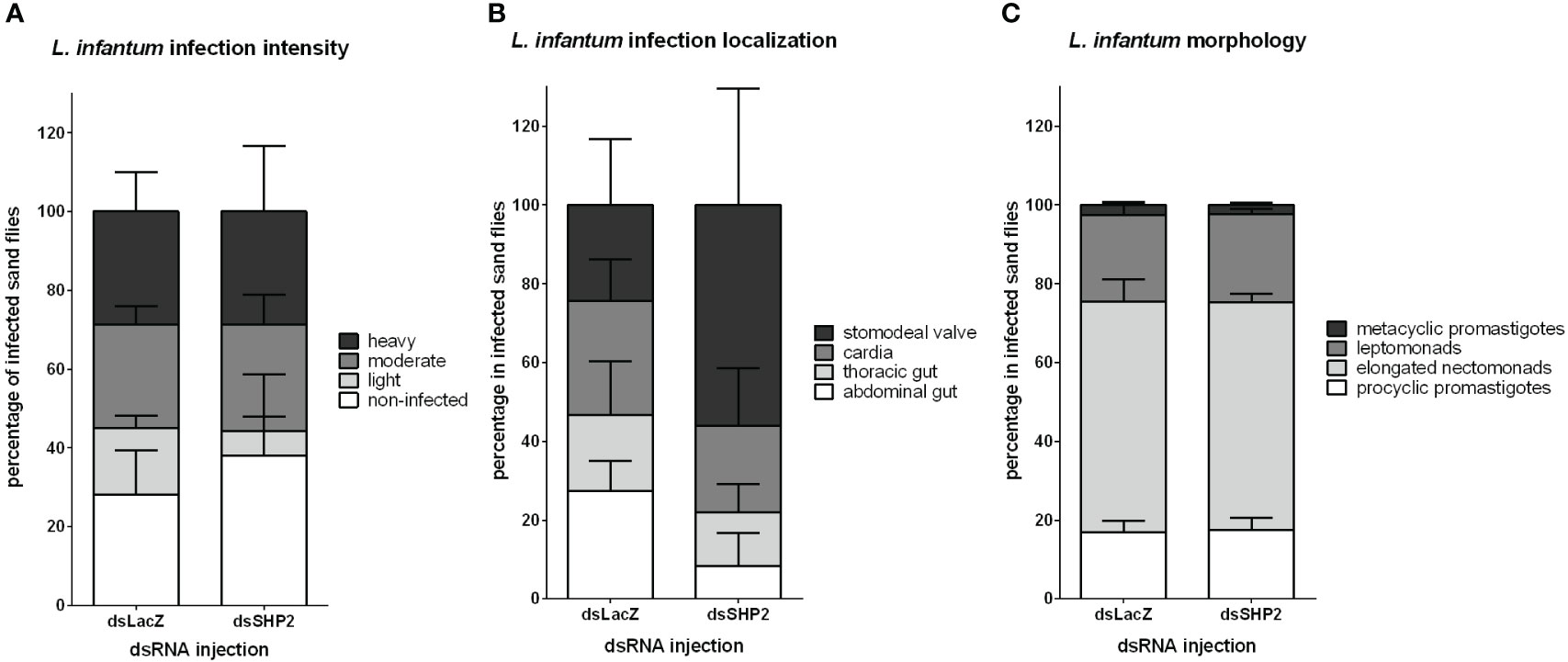
Figure 7 L. infantum development in SHP-2-silenced L. longipalpis: L. infantum infection intensity, location and developmental stages (8 days post-infection) in dsRNA-injected sand flies. (A) The y-axis represents the percentage of all individually inspected insects (minimum of 20 sand flies in each dsRNA injected group). Bars from white to shades of grey indicate infection intensity or parasite loads: non-infected (white), low or light infection (light grey), moderate or medium infection (mid grey), and heavy infection (dark grey). (B) The y-axis represents the percentage of infected insects used in infection progress evaluation in the gut. Bars from white to dark grey indicate Leishmania location in the sand fly gut: parasites reached the stomodeal valve (dark grey), cardia (mid grey), thoracic gut (light grey) or stayed in the abdominal gut (white). (C) Bars from white to dark grey indicate parasite developmental stages: metacyclic promastigote (dark grey); leptomonad (mid grey); elongated nectomonad (light grey); procyclic promastigote (white). The x-axis represents dsRNA-injected groups. No significant differences were found between the experimental and control groups (two-way ANOVA).
Discussion
The complex balance of the sand fly immune response toward pathogens is a key process for vectorial transmission outcome. We chose to investigate key regulatory genes involved in inhibiting immune pathways upon insect vector infection by Leishmania, in search of mechanisms reminiscent of what is seen in the parasite-mammalian host interactions.
L. longipalpis gene sequence homologous to cactus, a Toll pathway repressor (69), was previously identified (28, 70, 71). Cactus is the invertebrate homolog of the vertebrate I-κB family that includes I-κBα and I-κBβ. Our phylogenetic analysis showed that the L. longipalpis cactus peptide sequence was closely related to Drosophila sequences, in agreement with the I-κB evolutionary analysis by (72). The analyses of signature domains and similarities shared with other dipteran sequences increased supporting information for the previously identified sequences.
Another Toll-related regulator is SHP-2 (73), a protein tyrosine phosphatase, which is involved in PTP-mediated phosphorylation, an important cellular regulatory mechanism. Specifically, SHPs are key modulators of important immunity pathways, including Toll in mammals (42, 74). The L. longipalpis SHP-2 translated sequence contains the PTPn11 and PTPn6 signature domains of the SHP protein subfamily in vertebrates and invertebrates (75). Their signature domains comprise two SH2 domains interacting with phosphotyrosine docking sites and a PTP catalytic domain. The L. longipalpis SHP-2 sequence shares similarities with mosquito PTPn11 and fruit fly corkscrew sequences that are homologous to the mammalian SHP (76–78).
Like many other insects, the sand fly immune response can be activated to fight infections or balance the natural microbiota (79). The role of cactus in regulating the downstream expression of AMPs in L. longipalpis was first investigated in the LL5 cells. The RNAi-mediated gene silencing in non-challenged cells resulted in the upregulation of cecropin and defensin 2 (28). This finding reflected an association of the repressor suppression with the upregulation of effector molecules related to the Toll pathway.
The sand fly immune responses interact with and respond differently to commensal and harmful microbes. For instance, sand fly females can produce an anti-bacterial response in the hemolymph after experimental injections with Gram-positive or -negative bacteria (80, 81). In addition, the gene expression of AMPs in L. longipalpis increased when females were experimentally fed on sucrose meal seeded with bacteria (35) or when bacteria loads in the gut increased after feeding on the regular sucrose meal (36). In Leishmania-infected sand flies, defensin 1 gene expression was reduced after L. mexicana infection (35), while attacin, cecropin, and defensin 2 expression was increased after L. infantum infection (36). In these last two studies, the differential expression was assessed in whole-body samples, indicating several tissues’ immune responses. On the other hand, a transcriptome analysis of the L. longipalpis midguts showed that immunity genes are not among the most differentially expressed upon L. infantum infection, especially in a late phase of infection (33). Therefore, the systemic response may have a major role in the sand fly immunity, while the gut response is stable regardless of the parasitic infection.
Another possibility is that the sand fly tolerates Leishmania infection to a certain point due to the oral infection route of infection, which avoids inner cell responses and other immune repertoire like cellular immunity from hemocytes in the hemolymph. Interestingly, in Anopheles mosquitoes, there is a threshold of the number of Plasmodium berghei parasites that can infect the vector without eliciting a complement-like response in the hemocoel (82).
We investigated here the possibility of the parasitic infection modulating the Toll pathway repressors in L. longipalpis. We selected cactus and SHP-2 that are involved in regulating the Toll pathway. These two repressors were upregulated two days post L. infantum infection, indicating that this pathway is repressed in the early phase of the parasite infection cycle, possibly reflecting suppression of the Toll pathway. The Leishmania procyclic promastigotes might cause this effect on the initial days of infection. It is unlikely that such upregulation happened due to close contact of parasites with the sand fly gut epithelium. Before the end of blood digestion, the sand fly peritrophic matrix is not completely degraded, and parasites are enclosed in the food bolus (83). Therefore, a parasite-secreted molecule is envisioned to mediate the upregulation of cactus and SHP-2, thus reducing the vector’s immune response. Another interesting example of parasite-induced changes to the host occurs with P. falciparum parasites expressing the Pfs47 gene that codes for a 6-cysteine protein family protein and prevents the activation of several caspases. Consequently, it inhibits JNK-mediated apoptosis (84, 85).
Among several molecules secreted by the parasite, GP63 is a well-studied molecule involved in the modulation of the vertebrate host’s immune response. Indeed, the Leishmania GP63 was implicated in activating the murine macrophage SHP-1 leading to a down-modulation of the Toll pathway. The SHP family has a single homolog in invertebrates (86); therefore, it is possible that the sand fly SHP-2 has a role similar to the mammalian SHP-1. Our results from Leishmania-sand fly data here presented showed that the parasite GP63 induces insect gene expression. Interestingly, Hassani and Olivier (87) showed that GP63 carried by exosomes increased the production of a series of proteins from uninfected macrophages, including SHP-1 (87). Therefore, our finding that this parasite metalloprotease caused a gene upregulation in the sand fly is quite significant.
Moreover, our results indicate that there is a common strategy shared by L. infantum and L. amazonensis, which appears beneficial to the parasite for colonization in the insect gut. In the current study, L. amazonensis over-expressing GP63 resulted in an increased number of infected L. longipalpis females in the early phase of infection. In contrast, the strain under-expressing GP63 resulted in fewer infected sand flies (88). Previous quantification of GP63 in these strains, measured by flow cytometry, revealed a more than 16-fold difference between the over-expressing and under-expressing strains (54). These results indicate that the GP63 expression and the SHP-2 upregulation are connected, favoring the parasite infection of the vector similar to that shown in the macrophages (41, 42).
The LL5 embryonic cells co-cultured with different Leishmania species and Leishmania conditioned medium showed that parasite produced GP63 for internalization by these sand fly cells. Therefore, our results showed a similar event between the metalloprotease and the insect cells in vivo. The Leishmania conditioned medium contains a complex mixture of secreted molecules, of which several are known virulent factors, including GP63 (89–92). We also detected the association of parasite metalloprotease with the LL5 cells, independent of a direct contact between live parasites and sand fly cells.
It is possible that during the Leishmania developmental cycle within the sand fly, GP63 is made available to modulate the sand fly epithelial gut cells response, triggering the suppression of insect immunity. We experimentally fed L. longipalpis females with different mixtures of blood containing parasites, their conditioned medium, or exosomes to test this hypothesis. In this experimental setting, we included Leishmania exosomes that contain a complex molecular cargo but are also known to carry abundant GP63 (56, 93, 94). Interestingly, our results showed that the GP63 could be detected on the gut’s epithelial layer and muscular fibers during the early phase of the infection. The detection of Leishmania GP63 in sand fly vesicular-like structures suggested that GP63 have been internalized in endocytic vesicles; thus, reflecting a potential sand fly gut molecular transport through transcytosis (95). Other metalloproteases can target mammalian epithelial cells. For example, the bacterial metalloproteases gelatinase from Enterococcus faecalis (96) and SslE (secreted and surface associated lipoprotein) also known as YghJ from Escherichia coli (97) can compromise the integrity of gut epithelial cells in mammals. Therefore, other metalloproteases like GP63 may indeed interact with the host epithelial cells and be internalized by the sand fly gut.
Several studies investigated the cross-talk between intestinal parasites and their hosts, with extracellular vesicles having an important role in this interplay (98, 99). More specifically, the host intestinal cells can internalize exosomes from intestinal worms (100). Nevertheless, the effect of protozoan-secreted molecules or extracellular vesicles on their vectors is scarcely explored.
To test the role of L. longipapis SHP-2 during the parasite infection, we used RNAi-mediated gene silencing to suppress its expression. Our results showed that the parasite development was compromised during the early phase of infection, concomitantly with the days when gene silencing was most efficient. Therefore, the suppression of the sand fly immunity repressor potentially caused an increased immune response that caused the reduction of parasites. Interestingly, on an common experimental infection, while parasite multiplication occurs in the early phase of blood digestion, there is an associated increase of gene expression of AMPs attacin, cecropin, and defensin 2 (LlDef2) in L. longipalpis (36). In P. papatasi, the gene expression of a gut-specific defensin (PpDef1) increased at the end of blood digestion when another parasite multiplicative phase occur (37). Analogously, the suppression of LlDef2 and PpDef1 expression by RNAi-mediated gene silencing favored the parasites (36, 101). These studies corroborate the role of the sand fly immune response in balancing the parasite infection. In addition, to test if the reduction of parasite survival in the earlier infection phase would have a consequent effect on the progress of the infection, we analyzed infection parameters on 8 days post-infection. At this time, it is possible to detect parasites colonizing different parts of the sand fly gut and several differentiation forms, thus reflecting the ability of the parasite to advance in its cycle in the vector. At this stage of infection, the number of infected sand flies, the intensity of parasite loads, and parasite developmental forms did not suffer the impact of the early SHP-2 silencing and parasite reduction. In the context of the parasite GP63 and sand fly SHP-2, our current results and results from Hajmova et al. (88) show that they have an important role in the initial phase of infection. In addition, we observed a non-significant but intriguing increase in parasites localized at the stomodeal valve. This finding reflects that the early suppression of the SHP-2, with a consequent less favorable condition for the parasites, may have caused the variable migration to the stomodeal valve.
In conclusion, our results suggest that, as well as in macrophages, Leishmania can activate the invertebrate host equivalent to SHP-1, thus inhibiting the Toll pathway via sand fly immunosuppression. The present work is the first report of a putative Leishmania protein capable of modulating the immune response of the insect vector.
Data availability statement
The datasets presented in this study can be found in online repositories. The names of the repository/repositories and accession number(s) can be found in the article/Supplementary Material.
Author contributions
Conceptualization, YT-C, and ANP. Methodology, ELT, TL, and ANP. Validation, ELT, BT-N, and ANP. Investigation and formal analysis, ELT, BT-N, DMF, TD-B, TL, and ANP. Resources, KPC, YT-C and PV. Data curation, ELT, BT-N, TD-B, and ANP. Writing—original draft preparation, ELT. Review and editing, ELT, KPC, PV, YT-C, and ANP. Visualization, ELT and ANP. Funding acquisition, project administration, and supervision, ELT, PV, YT-C, and ANP. All authors contributed to the article and approved the submitted version.
Funding
This research was funded by IOC-Fiocruz (PAEF Program), INCT-EM (Institutos Nacionais em Ciencia e Tecnologia - Entomologia Molecular), research grant number CNPq/Proc. 465678/2014-9 and Projeto Inova Fiocruz - Geração de Conhecimento. We are grateful for support from the Coordination for the Improvement of Higher Education Personnel (CAPES) - Brazil. BT-N received a fellowship from CAPES. ELT, TL, and PV were supported by ERD Funds, project CePaViP (16_019/0000759). ELT and PV were also supported by the Czech Science Foundation (GACR 21-15700S).
Acknowledgments
The authors are grateful to the Genomic Platform—DNA Sequencing - RPT01A (Rede de Plataformas Tecnológicas FIOCRUZ) for DNA sequencing.
Conflict of interest
The authors declare that the research was conducted in the absence of any commercial or financial relationships that could be construed as a potential conflict of interest.
Publisher’s note
All claims expressed in this article are solely those of the authors and do not necessarily represent those of their affiliated organizations, or those of the publisher, the editors and the reviewers. Any product that may be evaluated in this article, or claim that may be made by its manufacturer, is not guaranteed or endorsed by the publisher.
Supplementary material
The Supplementary Material for this article can be found online at: https://www.frontiersin.org/articles/10.3389/fimmu.2023.1162596/full#supplementary-material
References
1. Sheehan G, Garvey A, Croke M, Kavanagh K. Innate humoral immune defences in mammals and insects: The same, with differences? Virulence (2018) 9(1):1625–39. doi: 10.1080/21505594.2018.1526531
2. Lemaitre B, Hoffmann J. The host defense of Drosophila melanogaster. Annu Rev Immunol (2007) 25:697–743. doi: 10.1146/annurev.immunol.25.022106.141615
3. Kingsolver MB, Hardy RW. Making connections in insect innate immunity. Proc Natl Acad Sci U S A (2012) 109(46):18639–40. doi: 10.1073/pnas.1216736109
4. Bang IS. JAK/STAT signaling in insect innate immunity. Entomological Res (2019) 49:339–53. doi: 10.1111/1748-5967.12384
5. Feldhaar H, Gross R. Immune reactions of insects on bacterial pathogens and mutualists. Microbes Infect (2008) 10(9):1082–8. doi: 10.1016/j.micinf.2008.07.010
6. Ali Mohammadie Kojour M, Han YS, Jo YH. An overview of insect innate immunity. Entomological Res (2020) 50(6):282–91. doi: 10.1111/1748-5967.12437
7. De Gregorio E, Spellman PT, Tzou P, Rubin GM, Lemaitre B. The Toll and Imd pathways are the major regulators of the immune response in Drosophila. EMBO J (2002) 21(11):2568–79. doi: 10.1093/emboj/21.11.2568
8. Agaisse H, Petersen UM, Boutros M, Mathey-Prevot B, Perrimon N. Signaling role of hemocytes in Drosophila JAK/STAT-dependent response to septic injury. Dev Cell (2003) 5(3):441–50. doi: 10.1016/S1534-5807(03)00244-2
9. Lee KZ, Ferrandon D. Negative regulation of immune responses on the fly. EMBO J (2011) 30(6):988–90. doi: 10.1038/emboj.2011.47
10. Ganesan S, Aggarwal K, Paquette N, Silverman N. NF-κB/rel proteins and the humoral immune responses of drosophila melanogaster. Curr Topics Microbiol Immunol (2011) 349:25. doi: 10.1007/82_2010_107
11. Morrison DK, Murakami MS, Cleghon V. Protein kinases and phosphatases in the drosophila genome. J Cell Biol (2000) 150(2):57. doi: 10.1083/jcb.150.2.F57
12. Neel BG. Structure and function of SH2-domain containing tyrosine phosphatases. Semin Cell Dev Biol (1993) 4(6):419–32. doi: 10.1006/scel.1993.1050
13. Klingmoller U, Lorenz U, Cantley LC, Neel BG, Lodish HF. Specific recruitment of SH-PTP1 to the erythropoietin receptor causes inactivation of JAK2 and termination of proliferative signals. Cell (1995) 80:729–38. doi: 10.1016/0092-8674(95)90351-8
14. Muller P, Kuttenkeuler D, Gesellchen V, Zeidler MP, Boutros M. Identification of JAK/STAT signalling components by genome-wide RNA interference. Nature (2005) 436(7052):871–5. doi: 10.1038/nature03869
15. Davis MM, Engstrom Y. Immune response in the barrier epithelia: lessons from the fruit fly Drosophila melanogaster. J Innate Immun (2012) 4(3):273–83. doi: 10.1159/000332947
16. Matetovici I, De Vooght L, Van Den Abbeele J. Innate immunity in the tsetse fly (Glossina), vector of African trypanosomes. Dev Comp Immunol (2019) 98:181–8. doi: 10.1016/j.dci.2019.05.003
17. Carmona-Peña SP, Contreras-Garduño J, Castro DP, Manjarrez J, Vázquez-Chagoyán JC. The innate immune response of triatomines against Trypanosoma cruzi and Trypanosoma rangeli with an unresolved question: Do triatomines have immune memory? Acta Tropica (2021) 224:106108. doi: 10.1016/j.actatropica.2021.106108
18. Gabrieli P, Caccia S, Varotto-Boccazzi I, Epis S. Mosquito trilogy: microbiota, immunity and pathogens, and their implications for the control of disease transmission. Front Microbiol (2021) 12:630438. doi: 10.3389/fmicb.2021.630438
19. Xi Z, Ramirez JL, Dimopoulos G. The aedes aEgypti toll pathway controls dengue virus infection. PloS Pathog (2008) 4(7):e1000098. doi: 10.1371/journal.ppat.1000098
20. Sanders HR, Foy BD, Evans AM, Gill SS. Sindbis virus induces transport processes and alters expression of innate immunity pathway genes in the midgut of the disease vector, Aedes aEgypti. Insect Biochem Mol Biol (2005) 35(11):1293–307. doi: 10.1016/j.ibmb.2005.07.006
21. Vizioli J, Bulet P, Hoffmann JA, Kafatos FC, Müller HM, Dimopoulos G. Gambicin: A novel immune responsive antimicrobial peptide from the malaria vector Anopheles Gambiae. Proc Natl Acad Sci United States America (2001) 98(22):12630–5. doi: 10.1073/pnas.221466798
22. Souza-Neto JA, Sim S, Dimopoulos G. An evolutionary conserved function of the JAK-STAT pathway in anti-dengue defense. Proc Natl Acad Sci U S A (2009) 106(42):17841–6. doi: 10.1073/pnas.0905006106
23. Angleró-Rodríguez YI, MacLeod HJ, Kang S, Carlson JS, Jupatanakul N, Dimopoulos G. Aedes aegypti molecular responses to Zika Virus: Modulation of infection by the toll and Jak/Stat immune pathways and virus host factors. Front Microbiol (2017) 8:2050(OCT). doi: 10.3389/fmicb.2017.02050
24. Gupta L, Molina-Cruz A, Kumar S, Barillas-Mury C. The STAT Pathway Mediates Late-Phase Immunity against Plasmodium in the Mosquito Anopheles Gambiae. Cell Host Microbe (2009) 5(5):498–507. doi: 10.1016/j.chom.2009.04.003
25. Bahia AC, Kubota MS, Tempone AJ, Traub-Cseko YM. The JAK-STAT pathway controls Plasmodium vivax load in early stages of Anopheles aquasalis infection. PLoS Negl Trop Dis (2011) 5(11):e1317. doi: 10.1371/journal.pntd.0001317
26. Boulanger N, Brun R, Ehret-Sabatier L, Kunz C, Bulet P. Immunopeptides in the defense reactions of Glossina morsitans to bacterial and Trypanosoma brucei brucei infections. Insect Biochem Mol Biol (2002) 32(4):369–75. doi: 10.1016/S0965-1748(02)00029-2
27. Vieira CS, Waniek PJ, Castro DP, Mattos DP, Moreira OC, Azambuja P. Impact of Trypanosoma cruzi on antimicrobial peptide gene expression and activity in the fat body and midgut of Rhodnius prolixus. Parasites Vectors (2016) 9:119. doi: 10.1186/s13071-016-1398-4
28. Tinoco-Nunes B, Telleria EL, Da Silva-Neves M, Traub-Csekö YM. The sandfly Lutzomyia longipalpis LL5 embryonic cell line has active Toll and Imd pathways and shows immune responses to bacteria, yeast and Leishmania. Parasites Vectors (2016) 9:222. doi: 10.1186/s13071-016-1507-4
29. da Silva Goncalves D, Iturbe-Ormaetxe I, Martins-da-Silva A, Moreira LA. Wolbachia introduction into Lutzomyia longipalpis (Diptera: Psychodidae) cell lines and its effects on immune-related gene expression and interaction with Leishmania infantum. Parasit Vectors (2019) 12(1):33. doi: 10.1186/s13071-018-3227-4
30. Telleria EL, Azevedo-Brito DA, Kykalová B, Traub-Csekö YM. Leishmania infantum Infection Modulates the Jak-STAT Pathway in Lutzomyia longipalpis LL5 Embryonic Cells and Adult Females, and Affects Parasite Growth in the Sand Fly. Front Trop Dis (2021) 2:747820. doi: 10.3389/fitd.2021.747820
31. Telleria EL, Sant’Anna MRV, Ortigão-Farias JR, Dillon RJ. Caspar-like gene depletion reduces leishmania infection in sand fly host Lutzomyia longipalpis. J Biol Chem (2012) 287(16):12985–93. doi: 10.1074/jbc.M111.331561
32. Louradour I, Ghosh K, Inbar E, Sacks DL. CRISPR/Cas9 mutagenesis in Phlebotomus papatasi: The immune deficiency pathway impacts vector competence for Leishmania major. MBio (2019) 10(4):e01941–19. doi: 10.1128/mBio.01941-19
33. Coutinho-Abreu IV, Serafim TD, Meneses C, Kamhawi S, Oliveira F, Valenzuela JG. Leishmania infection induces a limited differential gene expression in the sand fly midgut. BMC Genomics (2020) 21:608. doi: 10.1186/s12864-020-07025-8
34. Sloan MA, Sadlova J, Lestinova T, Ligoxygakis P. The Phlebotomus papatasi systemic transcriptional response to trypanosomatid-contaminated blood does not differ from the non-infected blood meal. Parasites Vectors (2021) 14(15):1–14. doi: 10.1186/s13071-020-04498-0
35. Telleria EL, Sant’Anna MRV, Alkurbi MO, Pitaluga AN, Dillon RJ, Traub-Csekö YM. Bacterial feeding, Leishmania infection and distinct infection routes induce differential defensin expression in Lutzomyia longipalpis. Parasites Vectors (2013) 6:12. doi: 10.1186/1756-3305-6-12
36. Telleria EL, Tinoco-Nunes B, Leštinová T, Traub-Csekö YM. Lutzomyia longipalpis Antimicrobial Peptides: Differential Expression during Development and Potential Involvement in Vector Interaction with Microbiota and Leishmania. Microorganisms (2021) 9(6):1271. doi: 10.3390/microorganisms9061271
37. Kykalová B, Tichá L, Volf P, Telleria EL. Phlebotomus papatasi Antimicrobial Peptides in Larvae and Females and a Gut-Specific Defensin Upregulated by Leishmania major Infection. Microorganisms (2021) 9(11):2307. doi: 10.3390/microorganisms9112307
38. Di-Blasi T, Telleria EL, Marques C, Traub-Csekö YM. Lutzomyia longipalpis tgf-β has a role in leishmania infantum chagasi survival in the vector. Front Cell Infection Microbiol (2019) 9:71. doi: 10.3389/fcimb.2019.00071
39. de Castro Neto AL, da Silveira JF, Mortara RA. Comparative analysis of virulence mechanisms of trypanosomatids pathogenic to humans. Front Cell Infection Microbiol (2021) 11:669079. doi: 10.3389/fcimb.2021.669079
40. Kotb Elmahallawy E, Alkhaldi AAM, Wikel S, Sa AAMA. Insights into leishmania molecules and their potential contribution to the virulence of the parasite. Veterinary Sci (2021) 8(2):33. doi: 10.3390/vetsci8020033
41. Gomez MA, Contreras I, Hallé M, Tremblay ML, McMaster RW, Olivier M. Leishmania GP63 alters host signaling through cleavage-activated protein tyrosine phosphatases. Sci Signaling (2009) 2(90):ra58. doi: 10.1126/SCISIGNAL.2000213
42. Abu-Dayyeh I, Shio MT, Sato S, Akira S, Cousineau B, Olivier M. Leishmania-induced IRAK-1 inactivation is mediated by SHP-1 interacting with an evolutionarily conserved KTIM motif. PLoS Negl Trop Dis (2008) 2(12):e305. doi: 10.1371/journal.pntd.0000305
43. Boguski MS, Lowe TMJ, Tolstoshev CM. dbEST — database for “expressed sequence tags”. Nat Genet (1993) 4:332–3. doi: 10.1038/ng0893-332
44. Giraldo-Calderón GI, Emrich SJ, MacCallum RM, Wieck R. VectorBase: An updated Bioinformatics Resource for invertebrate vectors and other organisms related with human diseases. Nucleic Acids Res (2015) 43(D1):D707–13. doi: 10.1093/nar/gku1117
45. Acland A, Agarwala R, Barrett T, Zbicz K. Database resources of the national center for biotechnology information. Nucleic Acids Res (2014) 42(D1):D7–D17. doi: 10.1093/nar/gkt1146
46. Li L, Stoeckert CJ, Roos DS. OrthoMCL: identification of ortholog groups for eukaryotic genomes. Genome Res (2003) 13(9):2178–89. doi: 10.1101/gr.1224503
47. Blum M, Chang HY, Chuguransky S, Finn RD. The InterPro protein families and domains database: 20 years on. Nucleic Acids Res (2021) 49(D1):D344–54. doi: 10.1093/nar/gkaa977
48. Lu S, Wang J, Chitsaz F, Marchler-Bauer A. CDD/SPARCLE: the conserved domain database in 2020. Nucleic Acids Res (2020) 48(D1):D265–8. doi: 10.1093/nar/gkz991
49. Edgar RC. MUSCLE: Multiple sequence alignment with high accuracy and high throughput. Nucleic Acids Res (2004) 32(5):1792–7. doi: 10.1093/nar/gkh340
50. Kumar S, Stecher G, Li M, Knyaz C, Tamura K. MEGA X: Molecular evolutionary genetics analysis across computing platforms. Mol Biol Evol (2018) 35:1547–9. doi: 10.1093/molbev/msy096
51. Jones DT, Taylor WR, Thornton JM. The rapid generation of mutation data matrices from protein sequences. Bioinformatics (1992) 8(3):275–82. doi: 10.1093/bioinformatics/8.3.275
52. Le SQ, Gascuel O. An improved general amino acid replacement matrix. Mol Biol Evol (2008) 25(7):1307–20. doi: 10.1093/molbev/msn067
53. Tesh RB, Modi GB. Development of a continuous cell line from the sand fly Lutzomyia longipalpis (Diptera: Psychodidae), and its susceptibility to infection with arboviruses. J Med Entomol (1983) 20(2):199–202. doi: 10.1093/jmedent/20.2.199
54. Chen D-Q, Kolli BK, Yadava N, Chang K-P. Episomal Expression of Specific Sense and Antisense mRNAs in Leishmania amazonensis: Modulation of gp63 Level in Promastigotes and Their Infection of Macrophages In Vitro. Infection Immun (2000) 68(1):80–6. doi: 10.1128/IAI.68.1.80-86.2000
55. Hendricks L, Wright N. Diagnosis of cutaneous leishmaniasis by in vitro cultivation of saline aspirates in Schneider’s drosophila medium. Am J Trop Med Hygiene (1979) 28(6):962–4. doi: 10.4269/ajtmh.1979.28.962
56. Forrest DM, Batista M, Marchini FK, Tempone AJ, Traub-Csekö YM. Proteomic analysis of exosomes derived from procyclic and metacyclic-like cultured Leishmania infantum chagasi. J Proteomics (2020) 227(15):103902. doi: 10.1016/j.jprot.2020.103902
57. Keerthikumar S, Chisanga D, Ariyaratne D, Mathivanan S. ExoCarta: A web-Based compendium of exosomal cargo. J Mol Biol (2016) 428(4):688–92. doi: 10.1016/j.jmb.2015.09.019
58. Lawyer P, Killick-Kendrick M, Rowland T, Rowton E, Volf P. Laboratory colonization and mass rearing of phlebotomine sand flies (Diptera, Psychodidae). Parasite (2017) 24:42. doi: 10.1051/parasite/2017041
59. Molina-Cruz A, DeJong RJ, Charles B, Gupta L, Kumar S, Jaramillo-Gutierrez G, et al. Reactive Oxygen Species Modulate Anopheles gambiae Immunity against Bacteria and Plasmodium. Journal of Biological Chemistry (2008) 283(6):3217–3223. doi: 10.1074/jbc.M705873200
60. Di-Blasi T, Lobo AR, Nascimento LM, Córdova-Rojas JL, Pestana K, Marín-Villa M, et al. The Flagellar Protein FLAG1/SMP1 is a Candidate for Leishmania–Sand Fly Interaction. Vector-Borne and Zoonotic Diseases (2015) 15(3):202–9. doi: 10.1089/vbz.2014.1736
61. Meireles-Filho AC, Amoretty PR, Souza NA, Kyriacou CP, Peixoto AA. Rhythmic expression of the cycle gene in a hematophagous insect vector. BMC Molecular Biol (2006) 7:38. doi: 10.1186/1471-2199-7-38
62. Sant’Anna MRV, Alexander B, Bates PA, Dillon RJ. Gene silencing in phlebotomine sand flies: Xanthine dehydrogenase knock down by dsRNA microinjections. Insect Biochem Mol Biol (2008) 38(6):652–60. doi: 10.1016/j.ibmb.2008.03.012
63. Myskova J, Votypka J, Volf P. Leishmania in sand flies: comparison of quantitative polymerase chain reaction with other techniques to determine the intensity of infection. J Med Entomology (2008) 45(1):133–8. doi: 10.1093/jmedent/45.1.133
64. Sádlová J, Price HP, Smith BA, Votỳpka J, Volf P, Smith DF. The stage-regulated HASPB and SHERP proteins are essential for differentiation of the protozoan parasite Leishmania major in its sand fly vector, Phlebotomus papatasi. Cell Microbiol (2010) 12(12):1765–79. doi: 10.1111/j.1462-5822.2010.01507.x
65. Rasband W. ImageJ website(2004). Available at: https://imagej.nih.gov/ij/.
66. Walters LL, Chaplin GL, Modi GB, Tesh RB. Ultrastructural biology of Leishmania (Viannia) panamensis (=Leishmania Braziliensis panamensis) in Lutzomyia gomezi (Diptera: Psychodidae): a natural host-parasite association. Am J Trop Med Hyg (1989) 40(1):19–39. doi: 10.4269/ajtmh.1989.40.19
67. Jaffray E, Wood KM, Hay RT. Domain organization of I kappa B alpha and sites of interaction with NF-kappa B p65. Mol Cell Biol (1995) 15(4):2166–72. doi: 10.1128/MCB.15.4.2166
68. Perkins ND. Integrating cell-signalling pathways with NF-κB and IKK function. Nat Rev Mol Cell Biol (2007) 8:49–62. doi: 10.1038/nrm2083
69. Valanne S, Wang J-H, Rämet M. The drosophila toll signaling pathway. J Immunol (2011) 186(2):649–56. doi: 10.4049/jimmunol.1002302
70. Dillon RJ, Ivens AC, Churcher C, Bates PA. Analysis of ESTs from Lutzomyia longipalpis sand flies and their contribution toward understanding the insect–parasite relationship. Genomics (2006) 88(6):831–40. doi: 10.1016/j.ygeno.2006.06.011
71. Pitaluga AN, Mason PW, Traub-Cseko YM. Non-specific antiviral response detected in RNA-treated cultured cells of the sandfly, Lutzomyia longipalpis. Dev Comp Immunol (2008) 32(3):191–7. doi: 10.1016/j.dci.2007.06.008
72. Basith S, Manavalan B, Gosu V, Choi S. Evolutionary, Structural and Functional Interplay of the IκB Family Members. PLOS ONE (2013) 8(1):e54178. doi: 10.1371/journal.pone.0054178
73. Lannoy V, Côté-Biron A, Asselin C, Rivard N. Phosphatases in toll-like receptors signaling: the unfairly-forgotten. Cell Communication Signaling (2021) 19(10):1–15. doi: 10.1186/s12964-020-00693-9
74. Yuk JM, Shin DM, Lee HM, Jo EK. The orphan nuclear receptor SHP acts as a negative regulator in inflammatory signaling triggered by Toll-like receptors. Nat Immunol (2011) 12(8):742–51. doi: 10.1038/ni.2064
75. Neel BG, Gu H, Pao L. The ‘Shp’ing news: SH2 domain-containing tyrosine phosphatases in cell signaling. Trends Biochem Sci (2003) 28(6):284–93. doi: 10.1016/S0968-0004(03)00091-4
76. Freeman RM, Plutzky J, Neel BG. Identification of a human src homology 2-containing protein-tyrosine-phosphatase: a putative homolog of Drosophila corkscrew. Proc Natl Acad Sci United States America (1992) 89(23):11239–43. doi: 10.1073/pnas.89.23.11239
77. Perkins LA, Larsen I, Perrimon N. corkscrew encodes a putative protein tyrosine phosphatase that functions to transduce the terminal signal from the receptor tyrosine kinase torso. Cell (1992) 70(2):225–36. doi: 10.1016/0092-8674(92)90098-W
78. Moretti DM, Ahuja LG, Nunes RD, Silva-Neto MAC. Molecular analysis of Aedes aegypti classical protein tyrosine phosphatases uncovers an ortholog of mammalian PTP-1B implicated in the control of egg production in mosquitoes. PLoS One (2014) 9(8):e104878. doi: 10.1371/journal.pone.0104878
79. Telleria EL, Martins-Da-Silva A, Tempone AJ, Traub-Cseko YM. Leishmania, microbiota and sand fly immunity. Parasitology (2018) 145(10):1336–53. doi: 10.1017/S0031182018001014
80. Nimmo DD, Ham PJ, Ward RD, Maingon R. The sandfly Lutzomyia longipalpis shows specific humoral responses to bacterial challenge. Med Veterinary Entomology (1997) 11(4):324–8. doi: 10.1111/j.1365-2915.1997.tb00417.x
81. Boulanger N, Lowenberger C, Volf P, Bulet P. Characterization of a defensin from the sand fly Phlebotomus duboscqi induced by challenge with bacteria or the protozoan parasite Leishmania major. Infection Immun (2004) 72(12):7140–6. doi: 10.1128/IAI.72.12.7140-7146.2004
82. Habtewold T, Groom Z, Christophides GK. Immune resistance and tolerance strategies in malaria vector and non-vector mosquitoes. Parasites Vectors (2017) 10:186. doi: 10.1186/s13071-017-2109-5
83. Dostálová A, Volf P. Leishmania development in sand flies: parasite-vector interactions overview. Parasites Vectors (2012) 5:276. doi: 10.1186/1756-3305-5-276
84. Molina-Cruz A, Garver LS, Alabaster A, Barillas-Mury C. The human malaria parasite Pfs47 gene mediates evasion of the mosquito immune system. Sci (New York N.Y.) (2013) 340(6135):984–7. doi: 10.1126/science.1235264
85. Ramphul UN, Garver LS, Molina-Cruz A, Canepa GE, Barillas-Mury C. Plasmodium falciparum evades mosquito immunity by disrupting JNK-mediated apoptosis of invaded midgut cells. Proc Natl Acad Sci U S A (2015) 112(5):1273–80. doi: 10.1073/pnas.1423586112
86. Liongue C, O’Sullivan LA, Trengove MC, Ward AC. Evolution of JAK-STAT pathway components: mechanisms and role in immune system development. PLoS One (2012) 7(3):e32777. doi: 10.1371/journal.pone.0032777
87. Hassani K, Olivier M. Immunomodulatory impact of leishmania-induced macrophage exosomes: a comparative proteomic and functional analysis. PLoS Negl Trop Dis (2013) 7(5):e2185. doi: 10.1371/journal.pntd.0002185
88. Hajmova M, Chang KP, Kolli B, Volf P. Down-regulation of gp63 in Leishmania amazonensis reduces its early development in Lutzomyia longipalpis. Microbes Infect (2004) 6(7):646–9. doi: 10.1016/j.micinf.2004.03.003
89. Silverman JM, Chan SK, Robinson DP, Reiner NE. Proteomic analysis of the secretome of Leishmania donovani. Genome Biol (2008) 9(2):R35. doi: 10.1186/gb-2008-9-2-r35
90. Corrales RM, Sereno D, Mathieu-Daudé F. Deciphering the Leishmania exoproteome: what we know and what we can learn. FEMS Immunol Med Microbiol (2010) 58(1):27–38. doi: 10.1111/j.1574-695X.2009.00608.x
91. Arango Duque G, Jardim A, Gagnon É, Fukuda M, Descoteaux A. The host cell secretory pathway mediates the export of Leishmania virulence factors out of the parasitophorous vacuole. PLoS Pathog (2019) 15(7):e1007982. doi: 10.1371/journal.ppat.1007982
92. Pissarra J, Pagniez J, Petitdidier E, Holzmuller P. Proteomic analysis of the promastigote secretome of seven leishmania species. J Proteome Res (2022) 21(1):30–48. doi: 10.1021/acs.jproteome.1c00244
93. Atayde VD, Hassani K, da Silva Lira Filho A, Olivier M. Leishmania exosomes and other virulence factors: Impact on innate immune response and macrophage functions. Cell Immunol (2016) 309:7–18. doi: 10.1016/j.cellimm.2016.07.013
94. Dong G, Filho AL, Olivier M. Modulation of host-pathogen communication by extracellular vesicles (EVs) of the protozoan parasite Leishmania. Front Cell Infection Microbiol (2019) 9:100. doi: 10.3389/fcimb.2019.00100
95. Huang JH, Jing X, Douglas AE. The multi-tasking gut epithelium of insects. Insect Biochem Mol Biol (2015) 67:15–20. doi: 10.1016/j.ibmb.2015.05.004
96. Steck N, Hoffmann M, Sava IG, Haller D. Enterococcus faecalis metalloprotease compromises epithelial barrier and contributes to intestinal inflammation. Gastroenterology (2011) 141(3):959–71. doi: 10.1053/j.gastro.2011.05.035
97. Luo Q, Kumar P, Vickers TJ, Fleckenstein JM. Enterotoxigenic escherichia coli secretes a highly conserved mucin-degrading metalloprotease to effectively engage intestinal epithelial cells. Infection Immun (2014) 82(2):509–21. doi: 10.1128/IAI.01106-13
98. Deolindo P, Evans-Osses I, Ramirez MI. Microvesicles and exosomes as vehicles between protozoan and host cell communication. Biochem Soc Trans (2013) 41(1):252–7. doi: 10.1042/BST20120217
99. Coakley G, Maizels RM, Buck AH. Exosomes and other extracellular vesicles: the new communicators in parasite infections. Trends Parasitol (2015) 31(10):477–89. doi: 10.1016/j.pt.2015.06.009
100. Marcilla A, Trelis M, Cortés A, Bernal D. Extracellular vesicles from parasitic helminths contain specific excretory/Secretory proteins and are internalized in intestinal host cells. PLoS One (2012) 7(9):e45974. doi: 10.1371/journal.pone.0045974
Keywords: sand fly, immunity, signaling pathway, protein-tyrosine phosphatase, SHP-2, vector-parasite interaction
Citation: Telleria EL, Tinoco-Nunes B, Forrest DM, Di-Blasi T, Leštinová T, Chang KP, Volf P, Pitaluga AN and Traub-Csekö YM (2023) Evidence of a conserved mammalian immunosuppression mechanism in Lutzomyia longipalpis upon infection with Leishmania. Front. Immunol. 14:1162596. doi: 10.3389/fimmu.2023.1162596
Received: 09 February 2023; Accepted: 13 October 2023;
Published: 02 November 2023.
Edited by:
Humberto Lanz-Mendoza, National Institute of Public Health, MexicoReviewed by:
Ingeborg Becker, National Autonomous University of Mexico, MexicoCarlos Logullo, Federal University of Rio de Janeiro, Brazil
Copyright © 2023 Telleria, Tinoco-Nunes, Forrest, Di-Blasi, Leštinová, Chang, Volf, Pitaluga and Traub-Csekö. This is an open-access article distributed under the terms of the Creative Commons Attribution License (CC BY). The use, distribution or reproduction in other forums is permitted, provided the original author(s) and the copyright owner(s) are credited and that the original publication in this journal is cited, in accordance with accepted academic practice. No use, distribution or reproduction is permitted which does not comply with these terms.
*Correspondence: Yara Maria Traub-Csekö, eXRyYXViQGlvYy5maW9jcnV6LmJy; André Nóbrega Pitaluga, cGl0YWx1Z2FAaW9jLmZpb2NydXouYnI=
†These authors have contributed equally to this work