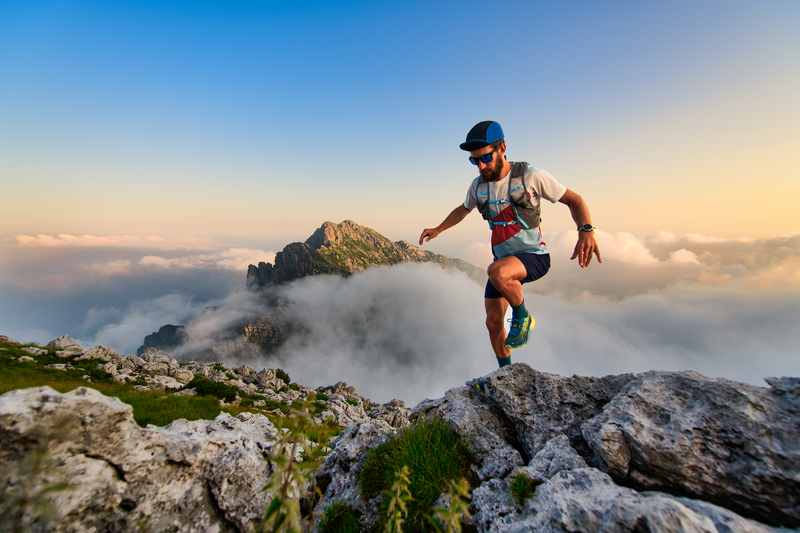
95% of researchers rate our articles as excellent or good
Learn more about the work of our research integrity team to safeguard the quality of each article we publish.
Find out more
OPINION article
Front. Immunol. , 27 June 2023
Sec. Cancer Immunity and Immunotherapy
Volume 14 - 2023 | https://doi.org/10.3389/fimmu.2023.1159337
This article is part of the Research Topic Expert Opinions and Perspectives in adoptive cell therapy for cancer: 2022 View all 7 articles
After the overwhelming clinical success of targeting hematological malignancies with CAR-T cells (1), the first signals of treatment are seen for solid tumors targeted by engineered immune cells (2). However, targeting solid tumors with this kind of immunotherapy still remains a challenge (3, 4). There are multiple mechanisms that make it difficult for adoptive cellular therapies to effectively target solid tumors.
First, most solid tumors lack homogeneous expression of a tumor-specific antigen making it difficult to find appropriate receptors to target them (5). The selection of targetable tumor antigens needs careful consideration to avoid targeting of healthy tissue, especially when considering engineered cellular therapies against solid cancers, where potent and safe antigens are rare (6). Additionally, the microenvironment of solid tumors holds unique features such as expression of immunosuppressive molecules and hypoxia that have a huge impact on T cell fitness (4, 7, 8). Finally, a combination of extracellular matrix deposition and anti-inflammatory signals, like attracting mesenchymal derived suppressor cells (MDSCs), prevent effective infiltration of T cells towards the tumor site (9).
In this article we will further discuss the roadblocks facing successful implementation of T cell therapies for the treatment of solid malignancies focusing on γδT cells and their receptors since they provide a new avenue to target novel tumor antigens. Characterization of these cells and their receptors holds the potential to generate novel strategies for targeting cancer and provide new engineering strategies to potentially overcome these hurdles.
The infiltration of γδT cell in tumors has been associated in many studies to have a favorable impact on patient survival (10–16), while some other studies made in murine models report that interleukin-17 (IL-17) producing γδT cells are tumor promoting (17, 18). While these data are very insightful, it has to be carefully handled when translating it to human clinical practices given that human and mouse γδT cell repertoires and functions are not fully compatible. Regardless of the ultimate effector function, activation of γδT cells is contingent upon the engagement of their surface receptors with antigens on the tumor cell. γδ T cells can be divided into two groups, Vδ2+ and Vδ2-, with Vδ1 forming the majority of Vδ2- T-cells. Vδ2- T cells are predominantly found in peripheral tissue and have also been shown to be enriched in carcinomas (11, 19–21). Multiple studies reported a correlating favorable clinical outcome either with the presence of Vδ2- T-cells (12, 13) or with γδT in general (13). This tissue-association might be advantageous for targeting and infiltrating solid tumors when using Vδ1TCR T cells as effector cells. Vδ2- TCRs can recognize a wide variety of ligands that are expressed on infected and malignant cells (22). A large number of studies have shown that numerous Vδ2- TCRs can recognize nonpolymorphic MHC I-like molecules MR1 and CD1 (23, 24). Most CD1 isoforms, CD1a, CD1b, and CD1c, are mainly found on cells of hematological origin and declassify them as potential ligands for solid tumors (25), but both MR1 as CD1d have been found to be expressed on solid tumors (25, 26). Other γδTCR ligands expressed on solid tumors and are recognized by specific Vδ2- TCR clones are endothelial protein C receptor (EPCR) (27), Annexin A2 (28), and EphA2 (29). Based on the wide breath of ligands recognized by Vδ2- TCRs (22), it is to be expected that many more ligands for this subset will be identified in the future. While many of these Vδ2- TCR ligands are also expressed on the surface healthy cells, such as EPCR on endothelial cells (30) and CD1d on APCs (31), no major safety concerns have been reported. For example, a study demonstrating that while an EPCR reactive Vδ2- TCR clone recognized cytomegalovirus (CMV)-infected or malignant endothelial cells it was not reactive against normal endothelial cells, due to increased expression of immune modulating molecules such as CD54 and CD58 (27). Additionally, to avoid toxicity towards healthy, antigen presenting cells (APCs), lipid-specific CD1d reactive Vδ2- TCRs can be used (32).
Unlike above discussed Vδ2- T cells, Vδ2+ T cells, also referred as Vγ9Vδ2 T cells are mainly present in blood and their role of cancer immune surveillance have been studied the most among all γδT cells (33). The process of identifying the ligand complex for the invariant Vγ9Vδ2 TCRs has been a long and winding path, that started with the identification of phosphoantigens (34) that are bound by the intracellular domain of butrophylin 3A1 (BTN3A1) (35). This process leads to a re-localization of BTN3A1 to the cell surface (36, 37), where it can form a complex with BTN2A1 (38–40). Only when this phosphoantigen driven complex of BTN3A1 and BTN2A1 is formed on the plasma membrane, Vγ9Vδ2 TCRs can be activated. This multistep ligand complex formation serves a safety threshold that prevents Vγ9Vδ2 TCR mediated toxicity towards healthy tissue but enables the eradication of tumors in many preclinical models (41–43).
While γδT cells have their natural potential to target cancer, as described above, the most clinical trials to date, that have assessed the efficacy and safety of γδT cells as adoptive cellular therapy did show moderate clinical efficacy (44–47) where only incidentally e.g. prolonged survival of patients has been reported (46). However, the potential of natural, tumor infiltrating γδT cells has recently been demonstrated in colorectal cancer (10) and kidney cancer (16), supporting the idea to further investigate the details of receptors present on γδT cells for the treatment of cancer. While providing an emerging universe of tumor specific receptors, one has to carefully assess possible toxicity against healthy tissues in advanced 3-dimensional preclinical models (41, 42, 48) that resemble the homeostatic environment of the human body.
T cell dysfunction has been one of the major causes of failure of CAR-T cell treatments as it results in poor T cell expansion and short-term persistence resulting in reduced anti-tumor efficacy (8, 49). Despite efforts to improve CAR designs, CAR-T cell exhaustion remains one of the main limitations of this kind of therapy (50–52). Thus, although CAR-T field has significantly growth in the last years, some studies advocate for the use of natural TCR signaling to reduce exhaustion of T cells (53, 54). The main reason for this is that CAR’s artificial design accelerates exhaustion of T cells when compared to TCR based therapies, mostly due to the described tonic signaling in the absence of antigen (54–56). In this line, several designs have been explored to make CAR more TCR-like, such as HLA-independent TCR (HIT) or synthetic TCR and antigen receptor (STAR) (57, 58). The CAR scFv sequence in these receptors is fused to the constant domains of an αβTCR, thereby preserving TCR signaling while using the CAR’s ability to recognize tumors in an HLA independent way. An elegant alternative to these designs is engineering αβ T cells to express tumor-reactive Vγ9Vδ2 TCRs (called TEGs) (41, 59). In this way, the use of γδTCRs in T cell therapy appear to be advantageous when compared with CARs or αβTCRs, as they supply T cells with natural TCR signaling while preserving the ability of recognize tumors in an HLA-independent way (44).
Optimal co-stimulation has been described as key to overcome exhaustion and improve T cell fitness and persistence in the context of cancer (60–62). Therefore, as costimulatory signals are highly involved in T cell metabolic reprogramming (63, 64) and T cell exhaustion is closely related with metabolic dysfunction, manipulation of co-stimulation in T cell therapies will result in improved metabolic T cell fitness, which is key to achieve robust anti-tumor responses (63). One example is the addition of co-stimulatory domains to the first generation of CARs, which has shown to improve persistence of these cells (65, 66). This led to the development of second and third generation of CARs with improved proliferation ability. Therefore, combining natural TCR signaling properties, by using γδTCRs to target tumors, with improved co-stimulation might be the answer to CAR-T limitations.
One way to improve the co-stimulation of T cells can be achieved by expressing chimeric costimulatory receptors (CCRs) in combination with a CAR or a TCR (67–70). These receptors preserve the structure of conventional second-generation CARs but lack the CD3ζ domain, therefore providing only costimulatory signals to the T cell. Uncoupling of signal 1 (CD3 signal) and signal 2 (co-stimulation) by this dual targeting has been shown to be beneficial (71–73) as T cells will only activate once synergistic signals are delivered upon encounter of both antigens. While these receptors improve T cell proliferation, they also reduce exhaustion (71) thereby improving T cell persistence in the tumor niche and leading to an improved therapeutic effect (71, 74).
A type of CCRs are the so-called switch chimeric co-receptors (75–78), which use the extracellular domain of a described inhibitory receptor (such as PD-1 or TIGIT) and link it to the intracellular domain of activating costimulatory receptors (such as CD28 or 4-1BB) or eventually DAP10, when expressed in γδT cells (70). Thus, these receptors turn inhibitory signals, that would normally induce exhaustion of T cells, into activating signals. This strategy improves not only T cell fitness, by improving co-stimulation, but also makes engineered T cells resistant to tumor microenvironment immunosuppressive factors.
Finally, it is important to further investigate the mechanisms that impact T cell fitness as not all the T cells subsets respond equal to the same stimulus. For example, TGF-β has been shown to improve cytotoxic activity of Vδ2+ T cells (79) while it is been described to suppress αβ T cells function (80). Furthermore, IL-15 has been shown to improve tumor killing capacity of γδT cells isolated from AML patients (81). Therefore, comprehensive studies and rational engineering it is key to develop effective therapies. In conclusion, to achieve durable anti-tumor responses the next generation of T cell-based immunotherapies should include fine-tuning of co-stimulation, to preserve T cell fitness, ensure persistence, and skew the T cells to the most potent phenotype.
The lack of efficacy observed for different T cell treatments targeting various antigens in solid tumors suggest the presence of general barriers that inhibit the efficacy of these immunotherapies. The cellular and extra-cellular composition of the tumor microenvironment can influence the tumor biology and response to immune therapy (82). The dense extracellular matrix (ECM) of solid tumors is a physical barrier for T cells to penetrate leading to low numbers of infiltrating, endogenous T cells in solid tumors (4). Meanwhile, immunosuppressive cells such as myeloid-derived suppressive cells and regulatory T cells in the tumor microenvironment (TME) inhibit antitumor activity of T cells that do infiltrate in the TME (83). Different engineering strategies are being developed to overcome these general barriers of T-cell therapies in solid malignancies.
Modulation of the chemokine signaling of the tumor-reactive T cells can lead to improved T cell infiltration by increasing chemotaxis towards the tumor site. For example, expression of the colony stimulating factor receptor (CSF-R) in CAR-T cells improved migration towards solid tumor models producing CSF (84). Arming T cells with other chemokine receptors have shown similar results where CCR4, CCR2b and CXCR3 overexpression in the T cell products led to increased infiltration in the TME and thereby increased tumor targeting (85–87).
Upon infiltration of immune cells in the TME, multiple mechanisms can render the T cells inactive via expression of immunosuppressive molecules. Well-known checkpoint molecules such as PD-1 and TIM3 are not only affecting αβ T cells but also act on γδ T cells as has been recently shown (10) in colorectal cancer. However, γδ T cells are also often regulated by unique sets of inhibitory natural killer (NK) receptors: for example, tumor and stromal cells can express ligands for immune checkpoints in T cells like HLA-E binding NKG2A on γδ tumor infiltrating lymphocytes (TILs) (88). To overcome this, numerous cytokines have been tested to make armed CAR-T cells also known as T cell redirected for antigen-unrestricted cytokine-initiated killing (TRUCKs) (89). CAR-T cells targeting different solid tumor models were shown to improve their anti-tumor activity, increase their resistance to regulatory T cell signaling and improve local proliferation upon arming the T cells with IL-12 expression (90–92). Expression of other cytokines such as IL-7, IL-15 and IL-18 have shown to provide similar results by increasing therapy efficacy via increasing local inflammation in the TME (92–94). Chemokine and cytokine arming of γδTCR based T cell therapies could increase efficacy since other T cell engineering approaches for CAR-T cells.
Additionally, CAR-T cells can be engineered to express ECM-modifying enzymes to facilitate better penetration to the tumor site. Heparinase expressing GD2 CAR-T cells improved their infiltrating capacity in solid tumor models compared to CAR-T cells lacking heparin expression (95, 96). Arming CAR-T cell with prolyl endopeptidase is another approach for targeting the ECM in the TME (97). Expression of prolyl endopeptidase in CAR-T cells improved their anti-tumor activity, however some toxicity towards healthy tissue was observed with both ECM targeting approaches. Introducing these types of modifications could be very promising for improving the therapeutic effect of γδTCR T cells in solid tumors.
Current developments in the field of engineered adoptive cellular therapies, especially CAR-T cell therapies show promising results in the treatment of haematological malignancies; more specifically B cell-derived tumors. However, adapting these T cells therapies to solid tumor treatments options requires overcoming certain impediments posed by solid malignancies and their TME (Figure 1). Fortunately, these T cells-based therapies allow for ex vivo modifications of the treatment to address these tumor-specific challenges posed in the TME of solid tumors where lesson learned from tumor specific γδT may provide a possible solution.
Figure 1 Schematic representation of T-cell engineering approaches. Biological mechanisms that prevent effective adoption of gd T-cell therapies for the treatment of solid malignancies and suggested engineering strategies to overcome these hurdles are shown.
Selection of the tumor-reactive receptor and the tumor specific/associated antigen remains the first important step in optimizing T cell therapies in solid tumors. To this end, γδTCRs are an interesting option due to their unique recognition patterns. Secondly, the addition of a co-stimulatory signal, especially in combination with a naturally low affinity γδTCR can help improve T cell fitness via either one of the three suggested signalling approaches. Expressing a chimeric costimulatory receptor to mimic signal 2 will help the T cells to retain their anti-tumor activity upon prolonged exposure in the TME. Furthermore, the induction of inflammation via secretion of cytokines such as at the tumor site can help the tumor infiltrating γδTCR T cells to overcome the immunosuppressive signals present in the TME. Finally, expression of chemokine(receptors) or ECM modifying molecules can help increase T cell infiltration in the solid tumor microenvironment.
In conclusion, promising approaches for improving the efficacy and scope of T cell therapies are being developed to overcome the current roadblocks in the treatment of solid malignancies. Using γδTCRs as tumor-reactive receptors, and combining these with appropriate co-stimulation via expression of additional chimeric costimulatory receptor to improve fitness and providing additional mechanisms to improve γδTCR T-cell infiltration like boosting chemotaxis, will be key assets to enhance efficacy of T cell therapies for solid malignancies. While further modifying the T cells does contain risks, these solutions will help to optimize efficacy of engineered T cell therapies and introduce this technology for a more widespread use in anticancer therapy.
All authors listed have made a substantial, direct, and intellectual contribution to the work and approved it for publication.
This work was supported by Grants KWF 11979, KWF 11393, KWF 12586, KWF 13043, KWF 13493 to authors JK, ZS and DB from the Dutch Cancer Society KWF.
JK, ZS and DB are inventors on various patents regarding T cell immune therapies, JK is shareholder at Gadeta BV.
The remaining authors declare that the research was conducted in the absence of any commercial or financial relationships that could be construed as a potential conflict of interest.
All claims expressed in this article are solely those of the authors and do not necessarily represent those of their affiliated organizations, or those of the publisher, the editors and the reviewers. Any product that may be evaluated in this article, or claim that may be made by its manufacturer, is not guaranteed or endorsed by the publisher.
1. Roschewski M, Longo DL, Wilson WH. CAR T-cell therapy for Large b-cell lymphoma - who, when, and how? N Engl J Med (2022) 386(7):692–6. doi: 10.1056/NEJMe2118899
2. Majzner RG, Ramakrishna S, Yeom KW, Patel S, Chinnasamy H, Schultz LM, et al. GD2-CAR T cell therapy for H3K27M-mutated diffuse midline gliomas. Nature (2022) 603(7903):934–41. doi: 10.1038/s41586-022-04489-4
3. Marofi F, Motavalli R, Safonov VA, Thangavelu L, Yumashev AV, Alexander M, et al. CAR T cells in solid tumors: challenges and opportunities. Stem Cell Res Ther (2021) 12(1):81. doi: 10.1186/s13287-020-02128-1
4. Hou AJ, Chen LC, Chen YY. Navigating CAR-T cells through the solid-tumour microenvironment. Nat Rev Drug Discov (2021) 20(7):531–50. doi: 10.1038/s41573-021-00189-2
5. Wagner J, Wickman E, DeRenzo C, Gottschalk S. CAR T cell therapy for solid tumors: bright future or dark reality? Mol Ther (2020) 28(11):2320–39. doi: 10.1016/j.ymthe.2020.09.015
6. Bailey SR, Berger TR, Graham C, Larson RC, Maus MV. Four challenges to CAR T cells breaking the glass ceiling. Eur J Immunol (2022) p:e2250039. doi: 10.1002/eji.202250039
7. Gao TA, Chen YY. Engineering next-generation CAR-T cells: overcoming tumor hypoxia and metabolism. Annu Rev Chem Biomol Eng (2022) 13:193–216. doi: 10.1146/annurev-chembioeng-092120-092914
8. Gumber D, Wang LD. Improving CAR-T immunotherapy: overcoming the challenges of T cell exhaustion. EBioMedicine (2022) 77:103941. doi: 10.1016/j.ebiom.2022.103941
9. Lanitis E, Dangaj D, Irving M, Coukos G. Mechanisms regulating T-cell infiltration and activity in solid tumors. Ann Oncol (2017) 28(suppl_12):xii18–32. doi: 10.1093/annonc/mdx238
10. de Vries NL, van de Haar J, Veninga V, Chalabi M, Ijsselsteijn ME, Ploeg der van M, et al. Gammadelta T cells are effectors of immunotherapy in cancers with HLA class I defects. Nature (2023) 613(7945):743–50. doi: 10.1038/s41586-022-05593-1
11. Gherardin NA, et al. Gammadelta T cells in merkel cell carcinomas have a proinflammatory profile prognostic of patient survival. Cancer Immunol Res (2021) 9(6):612–23. doi: 10.1158/2326-6066.CIR-20-0817
12. Donia M, Ellebaek E, Straten Pt Andersen MH, Svane IM. Analysis of Vdelta1 T cells in clinical grade melanoma-infiltrating lymphocytes. Oncoimmunology (2012) 1(8):1297–304. doi: 10.4161/onci.21659
13. Gentles AJ, Newman AM, Liu CL, Bratman SV, Feng W, Kim D, et al. The prognostic landscape of genes and infiltrating immune cells across human cancers. Nat Med (2015) 21(8):938–45. doi: 10.1038/nm.3909
14. Zakeri N, Hall A, Swadling L, Pallett LJ, Schmidt NM, Diniz MO, et al. Characterisation and induction of tissue-resident gamma delta T-cells to target hepatocellular carcinoma. Nat Commun (2022) 13(1):1372. doi: 10.1038/s41467-022-29012-1
15. Rosso DA, Rosato M, Iturrizaga J, González N, Shiromizu CM, Keitelman IA, et al. Glioblastoma cells potentiate the induction of the Th1-like profile in phosphoantigen-stimulated gammadelta T lymphocytes. J Neurooncol (2021) 153(3):403–15. doi: 10.1007/s11060-021-03787-7
16. Rancan C, Arias-Badia M, Dogra P, Chen B, Aran D, Yang H, et al. Exhausted intratumoral Vdelta2(-) gammadelta T cells in human kidney cancer retain effector function. Nat Immunol (2023) 24:612–24. doi: 10.1038/s41590-023-01448-7
17. Coffelt SB, Kersten K, Doornebal CW, Weiden J, Vrijland K, Hau C-S, et al. IL-17-producing gammadelta T cells and neutrophils conspire to promote breast cancer metastasis. Nature (2015) 522(7556):345–8. doi: 10.1038/nature14282
18. Lo Presti E, Toia F, Oieni S, Buccheri S, Turdo A, Mangiapane LR, et al. Squamous cell tumors recruit gammadelta T cells producing either IL17 or IFNgamma depending on the tumor stage. Cancer Immunol Res (2017) 5(5):397–407. doi: 10.1158/2326-6066.CIR-16-0348
19. Hidalgo JV, Bronsert P, Orlowska-Volk M, Díaz LB, Stickeler E, Werner M, et al. Histological analysis of gammadelta T lymphocytes infiltrating human triple-negative breast carcinomas. Front Immunol (2014) 5:632. doi: 10.3389/fimmu.2014.00632
20. Dadi S, Chhangawala S, Whitlock BM, Franklin RA, Luo CT, Oh SA, et al. Cancer immunosurveillance by tissue-resident innate lymphoid cells and innate-like T cells. Cell (2016) 164(3):365–77. doi: 10.1016/j.cell.2016.01.002
21. Janssen A, Hidalgo JV, Beringer DX, Dooremalen van S, Fernando F, Diest van E, et al. Gammadelta T-cell receptors derived from breast cancer-infiltrating T lymphocytes mediate antitumor reactivity. Cancer Immunol Res (2020) 8(4):530–43. doi: 10.1158/2326-6066.CIR-19-0513
22. Willcox BE, Willcox CR. Gammadelta TCR ligands: the quest to solve a 500-million-year-old mystery. Nat Immunol (2019) 20(2):121–8. doi: 10.1038/s41590-018-0304-y
23. Van Rhijn I, Le Nours J. CD1 and MR1 recognition by human gammadelta T cells. Mol Immunol (2021) 133:95–100. doi: 10.1016/j.molimm.2020.12.008
24. Castro CD, Boughter CT, Broughton AE, Ramesh A, Adams EJ. Diversity in recognition and function of human gammadelta T cells. Immunol Rev (2020) 298(1):134–52. doi: 10.1111/imr.12930
25. Consonni M, Dellabona P, Casorati G. Potential advantages of CD1-restricted T cell immunotherapy in cancer. Mol Immunol (2018) 103:200–8. doi: 10.1016/j.molimm.2018.09.025
26. Crowther MD, Dolton G, Legut M, Caillaud ME, Lloyd A, Attaf M, et al. Genome-wide CRISPR-Cas9 screening reveals ubiquitous T cell cancer targeting via the monomorphic MHC class I-related protein MR1. Nat Immunol (2020) 21(2):178–85. doi: 10.1038/s41590-019-0578-8
27. Willcox CR, Pitard V, Netzer S, Couzi L, Salim M, Silberzahn T, et al. Cytomegalovirus and tumor stress surveillance by binding of a human gammadelta T cell antigen receptor to endothelial protein c receptor. Nat Immunol (2012) 13(9):872–9. doi: 10.1038/ni.2394
28. Marlin R, Pappalardo A, Kaminski H, Willcox CR, Pitard V, Netzer S, et al. Sensing of cell stress by human gammadelta TCR-dependent recognition of annexin A2. Proc Natl Acad Sci USA (2017) 114(12):3163–8. doi: 10.1073/pnas.1621052114
29. Harly C, Joyce SP, Domblides C, Bachelet T, Pitard V, Mannat C, et al. Human gammadelta T cell sensing of AMPK-dependent metabolic tumor reprogramming through TCR recognition of EphA2. Sci Immunol (2021) 6(61). doi: 10.1126/sciimmunol.aba9010
30. Laszik Z, Mitro A, Taylor FB Jr, Ferrell G, Esmon CT. Human protein c receptor is present primarily on endothelium of large blood vessels: implications for the control of the protein c pathway. Circulation (1997) 96(10):3633–40. doi: 10.1161/01.CIR.96.10.3633
31. Brigl M, Brenner MB. CD1: antigen presentation and T cell function. Annu Rev Immunol (2004) 22:817–90. doi: 10.1146/annurev.immunol.22.012703.104608
32. Luoma AM, Castro CD, Mayassi T, Bembinster LA, Bai L, Picard D, et al. Crystal structure of Vdelta1 T cell receptor in complex with CD1d-sulfatide shows MHC-like recognition of a self-lipid by human gammadelta T cells. Immunity (2013) 39(6):1032–42. doi: 10.1016/j.immuni.2013.11.001
33. Sebestyen Z, Prinz I, Déchanet-Merville J, Silva-Santos B, Kuball J. Translating gammadelta (gammadelta) T cells and their receptors into cancer cell therapies. Nat Rev Drug Discov (2020) 19(3):169–84. doi: 10.1038/s41573-019-0038-z
34. Tanaka Y, Morita CT, Tanaka Y, Nieves E, Brenner MB, Bloom BR, et al. Natural and synthetic non-peptide antigens recognized by human gamma delta T cells. Nature (1995) 375(6527):155–8. doi: 10.1038/375155a0
35. Harly C, Guillaume Y, Nedellec S, Peigné C-M, Mönkkönen H, Mönkkönen J, et al. Key implication of CD277/butyrophilin-3 (BTN3A) in cellular stress sensing by a major human gammadelta T-cell subset. Blood (2012) 120(11):2269–79. doi: 10.1182/blood-2012-05-430470
36. Vantourout P, Laing A, Hayday AC. Heteromeric interactions regulate butyrophilin (BTN) and BTN-like molecules governing gammadelta T cell biology. Proc Natl Acad Sci USA (2018) 115(5):1039–44. doi: 10.1073/pnas.1701237115
37. Vyborova A, Beringer +DX, Fasci D, Karaiskaki F, Diest van E, Kramer L, et al. gamma9delta2T cell diversity and the receptor interface with tumor cells. J Clin Invest (2020) 130(9):4637–51. doi: 10.1172/JCI132489
38. Rigau M, Ostrouska S, Fulford TS, Johnson DN, Woods K, Ruan Z, et al. Butyrophilin 2A1 is essential for phosphoantigen reactivity by gammadelta T cells. Science (2020) 367(6478). doi: 10.1126/science.aay5516
39. Karunakaran MM, Willcox CR, Salim M, Paletta D, Fichtner AS, Noll A, et al. Butyrophilin-2A1 directly binds germline-encoded regions of the Vgamma9Vdelta2 TCR and is essential for phosphoantigen sensing. Immunity (2020) 52(3):487–498 e6. doi: 10.1016/j.immuni.2020.02.014
40. Hsiao CHC, Nguyen K, Jin Y, Vinogradova O, Wiemer AJ. Ligand-induced interactions between butyrophilin 2A1 and 3A1 internal domains in the HMBPP receptor complex. Cell Chem Biol (2022) 29(6):985–995 e5. doi: 10.1016/j.chembiol.2022.01.004
41. Johanna I, Straetemans T, Heijhuurs S, Aarts-Riemens T, Norell H, Bongiovanni L, et al. Evaluating in vivo efficacy - toxicity profile of TEG001 in humanized mice xenografts against primary human AML disease and healthy hematopoietic cells. J Immunother Cancer (2019) 7(1):69. doi: 10.1186/s40425-019-0558-4
42. van Diest E, López Hernández P, Meringa AD, Vyborova A, Karaiskaki F, Heijhuurs S, et al. Gamma delta TCR anti-CD3 bispecific molecules (GABs) as novel immunotherapeutic compounds. J Immunother Cancer (2021) 9(11). doi: 10.1136/jitc-2021-003850
43. De Gassart A, Le K-S, Brune P, Agaugué S, Sims J, Goubard A, et al. Development of ICT01, a first-in-class, anti-BTN3A antibody for activating Vgamma9Vdelta2 T cell-mediated antitumor immune response. Sci Transl Med (2021) 13(616):eabj0835. doi: 10.1126/scitranslmed.abj0835
44. Sebestyen Z, Prinz I, Déchanet-Merville J, Silva-Santos B, Kuball J. Translating gammadelta (γδ) T cells and their receptors into cancer cell therapies. Nat Rev Drug Discov (2020) 19(3):169–84. doi: 10.1038/s41573-019-0038-z
45. Mensurado S, Blanco-Dominguez R, Silva-Santos B. The emerging roles of gammadelta T cells in cancer immunotherapy. Nat Rev Clin Oncol (2023) 20:178–91. doi: 10.1038/s41571-022-00722-1
46. Xu Y, et al. Allogeneic Vgamma9Vdelta2 T-cell immunotherapy exhibits promising clinical safety and prolongs the survival of patients with late-stage lung or liver cancer. Cell Mol Immunol (2021) 18(2):427–39. doi: 10.1038/s41423-020-0515-7
47. Fournie JJ, Sicard H, Poupot M, Bezombes C, Blanc A, Romagné F, et al. What lessons can be learned from gammadelta T cell-based cancer immunotherapy trials? Cell Mol Immunol (2013) 10(1):35–41. doi: 10.1038/cmi.2012.39
48. Braham MVJ, Minnema MC, Aarts T, Sebestyen Z, Straetemans T, Vyborova A, et al. Cellular immunotherapy on primary multiple myeloma expanded in a 3D bone marrow niche model. Oncoimmunology (2018) 7(6):e1434465. doi: 10.1080/2162402X.2018.1434465
49. Fraietta JA, Lacey SF, Orlando EJ, Pruteanu-Malinici I, Gohil M, Lundh S, et al. Determinants of response and resistance to CD19 chimeric antigen receptor (CAR) T cell therapy of chronic lymphocytic leukemia. Nat Med (2018) 24(5):563–71. doi: 10.1038/s41591-018-0010-1
50. Kouro T, Himuro H, Sasada T. Exhaustion of CAR T cells: potential causes and solutions. J Transl Med (2022) 20(1):239. doi: 10.1186/s12967-022-03442-3
51. Wherry EJ, Kurachi M. Molecular and cellular insights into T cell exhaustion. Nat Rev Immunol (2015) 15(8):486–99. doi: 10.1038/nri3862
52. Poorebrahim M, Melief J, Coaña Pico Y, Wickström SL, Cid-Arregui A, Kiessling R, et al. Counteracting CAR T cell dysfunction. Oncogene (2021) 40(2):421–35. doi: 10.1038/s41388-020-01501-x
53. Salter AI, Rajan A, Kennedy JJ, Ivey RG, Shelby SA, Leung I, et al. Comparative analysis of TCR and CAR signaling informs CAR designs with superior antigen sensitivity and in vivo function. Sci Signal (2021) 14(697). doi: 10.1126/scisignal.abe2606
54. Wachsmann TLA, Wouters AK, Remst DFG, Hagedoorn RS, Meeuwsen MH, Diest van E, et al. Comparing CAR and TCR engineered T cell performance as a function of tumor cell exposure. Oncoimmunology (2022) 11(1):2033528. doi: 10.1080/2162402X.2022.2033528
55. Calderon H, Mamonkin M, Guedan S. Analysis of CAR-mediated tonic signaling. Methods Mol Biol (2020) 2086:223–36. doi: 10.1007/978-1-0716-0146-4_17
56. Gomes-Silva D, Mukherjee M, Srinivasan M, Krenciute G, Dakhova O, Zheng Y, et al. Tonic 4-1BB costimulation in chimeric antigen receptors impedes T cell survival and is vector-dependent. Cell Rep (2017) 21(1):17–26. doi: 10.1016/j.celrep.2017.09.015
57. Mansilla-Soto J, Eyquem J, Haubner S, Hamieh M, Feucht J, Paillon N, et al. HLA-independent T cell receptors for targeting tumors with low antigen density. Nat Med (2022) 28(2):345–52. doi: 10.1038/s41591-021-01621-1
58. Liu Y, Liu G, Wang J, Zheng Z-Y, Jia L. Chimeric STAR receptors using TCR machinery mediate robust responses against solid tumors. Sci Transl Med (2021) 13(586). doi: 10.1126/scitranslmed.abb5191
59. Marcu-Malina V, Heijhuurs S, Buuren van M, Hartkamp L, Strand S, Sebestyen Z, et al. Redirecting alphabeta T cells against cancer cells by transfer of a broadly tumor-reactive gammadeltaT-cell receptor. Blood (2011) 118(1):50–9. doi: 10.1182/blood-2010-12-325993
60. Long AH, Haso WM, Shern JF, Wanhainen KM, Murgai M, Ingaramo M, et al. 4-1BB costimulation ameliorates T cell exhaustion induced by tonic signaling of chimeric antigen receptors. Nat Med (2015) 21(6):581–90. doi: 10.1038/nm.3838
61. Guedan S, Posey AD Jr, Shaw C, Wing A, Da T, Patel PR, et al. Enhancing CAR T cell persistence through ICOS and 4-1BB costimulation. JCI Insight (2018) 3(1). doi: 10.1172/jci.insight.96976
62. Kowolik CM, Topp MS, Gonzalez S, Pfeiffer T, Olivares S, Gonzalez N, et al. CD28 costimulation provided through a CD19-specific chimeric antigen receptor enhances in vivo persistence and antitumor efficacy of adoptively transferred T cells. Cancer Res (2006) 66(22):10995–1004. doi: 10.1158/0008-5472.CAN-06-0160
63. Pellegrino M, Bufalo Del F, Angelis De B, Quintarelli C, Caruana I, Billy E, et al. Manipulating the metabolism to improve the efficacy of CAR T-cell immunotherapy. Cells (2020) 10(1). doi: 10.3390/cells10010014
64. Kawalekar OU, RS, Fraietta JA, Guo L, McGettigan SE, Posey AD Jr, et al. Distinct signaling of coreceptors regulates specific metabolism pathways and impacts memory development in CAR T cells. Immunity (2016) 44(2):380–90. doi: 10.1016/j.immuni.2016.01.021
65. Tokarew N, Ogonek J, Endres S, Bergwelt-Baildon von M, Kobold S. Teaching an old dog new tricks: next-generation CAR T cells. Br J Cancer (2019) 120(1):26–37. doi: 10.1038/s41416-018-0325-1
66. Imai C, Sjöstrand M, Naik J, Mansilla-Soto J, Kefala D, Kladis G, et al. Chimeric receptors with 4-1BB signaling capacity provoke potent cytotoxicity against acute lymphoblastic leukemia. Leukemia (2004) 18(4):676–84. doi: 10.1038/sj.leu.2403302
67. Katsarou A, Guo HF, Latouche JB, Tan C, Cheung NK, Sadelain M, et al. Combining a CAR and a chimeric costimulatory receptor enhances T cell sensitivity to low antigen density and promotes persistence. Sci Transl Med (2021) 13(623):eabh1962. doi: 10.1126/scitranslmed.abh1962
68. Krause A, et al. Antigen-dependent CD28 signaling selectively enhances survival and proliferation in genetically modified activated human primary T lymphocytes. J Exp Med (1998) 188(4):619–26. doi: 10.1084/jem.188.4.619
69. Sadelain M, Brentjens R, Rivière I. The basic principles of chimeric antigen receptor design. Cancer Discov (2013) 3(4):388–98. doi: 10.1158/2159-8290.CD-12-0548
70. Fisher J, Sharma R, Don Wisidagamage D, Barisa M, Hurtado MO, Abramowski P, et al. Engineering gammadeltaT cells limits tonic signaling associated with chimeric antigen receptors. Sci Signal (2019) 12(598). doi: 10.1126/scisignal.aax1872
71. Wilkie S, Schalkwyk van MCI, Hobbs S, Davies DM, Stegen der van SJC, Pereira ACP, et al. Dual targeting of ErbB2 and MUC1 in breast cancer using chimeric antigen receptors engineered to provide complementary signaling. J Clin Immunol (2012) 32(5):1059–70. doi: 10.1007/s10875-012-9689-9
72. Liao Q, Mao Y, He H, Ding X, Zhang X, Xu J, et al. PD-L1 chimeric costimulatory receptor improves the efficacy of CAR-T cells for PD-L1-positive solid tumors and reduces toxicity in vivo. biomark Res (2020) 8(1):57. doi: 10.1186/s40364-020-00237-w
73. Lanitis E, Poussin M, Klattenhoff AW, Song D, Sandaltzopoulos R, June CH, et al. Chimeric antigen receptor T cells with dissociated signaling domains exhibit focused antitumor activity with reduced potential for toxicity in vivo. Cancer Immunol Res (2013) 1(1):43–53. doi: 10.1158/2326-6066.CIR-13-0008
74. Halim L, Das KK, Larcombe-Young D, Ajina A, Candelli A, Benjamin R, et al. Engineering of an avidity-optimized CD19-specific parallel chimeric antigen receptor that delivers dual CD28 and 4-1BB Co-stimulation. Front Immunol (2022) 13:836549. doi: 10.3389/fimmu.2022.836549
75. Vienot A, Pallandre J-R, Renaude E, Viot J, Bouard A, Spehner L, et al. Chemokine switch regulated by TGF-β1 in cancer-associated fibroblast subsets determines the efficacy of chemo-immunotherapy. Oncoimmunology (2022) 11(1):2144669. doi: 10.1080/2162402X.2022.2144669
76. Supimon K, Sangsuwannukul T, Sujjitjoon J, Chieochansin T, Junking M, P-T, et al. Cytotoxic activity of anti-mucin 1 chimeric antigen receptor T cells expressing PD-1-CD28 switch receptor against cholangiocarcinoma cells. Cytotherapy (2022) 25:148–61. doi: 10.1016/j.jcyt.2022.10.006
77. Chen C, Gu Y-M, Zhang F, Zhang Z-C, Zhang Y-T, He Y-D, et al. Construction of PD1/CD28 chimeric-switch receptor enhances anti-tumor ability of c-met CAR-T in gastric cancer. Oncoimmunology (2021) 10(1):1901434. doi: 10.1080/2162402X.2021.1901434
78. Hoogi S, Eisenberg V, Mayer S, Shamul A, Barliya T, Cohen CJ, et al. A TIGIT-based chimeric co-stimulatory switch receptor improves T-cell anti-tumor function. J Immunother Cancer (2019) 7(1):243. doi: 10.1186/s40425-019-0721-y
79. Peters C, Meyer A, Kouakanou L, Feder J, Schricker T, Lettau M, et al. TGF-β enhances the cytotoxic activity of Vδ2 T cells. Oncoimmunology (2019) 8(1):e1522471. doi: 10.1080/2162402X.2018.1522471
80. Dahmani A, Delisle JS. TGF-β in T cell biology: implications for cancer immunotherapy. Cancers (Basel) (2018) 10(6). doi: 10.3390/cancers10060194
81. Van Acker HH, Anguille S, Willemen Y, Bergh den Van JM, Berneman ZN, Lion E, et al. Interleukin-15 enhances the proliferation, stimulatory phenotype, and antitumor effector functions of human gamma delta T cells. J Hematol Oncol (2016) 9(1):101. doi: 10.1186/s13045-016-0329-3
82. Junttila MR, de Sauvage FJ. Influence of tumour micro-environment heterogeneity on therapeutic response. Nature (2013) 501(7467):346–54. doi: 10.1038/nature12626
83. Verma NK, Wong BHS, Poh ZS, Udayakumar A, Verma R, Goh RKJ, et al. Obstacles for T-lymphocytes in the tumour microenvironment: therapeutic challenges, advances and opportunities beyond immune checkpoint. EBioMedicine (2022) 83:104216. doi: 10.1016/j.ebiom.2022.104216
84. Lo AS, Taylor JR, Farzaneh F, Kemeny DM, Dibb NJ, Maher J, et al. Harnessing the tumour-derived cytokine, CSF-1, to co-stimulate T-cell growth and activation. Mol Immunol (2008) 45(5):1276–87. doi: 10.1016/j.molimm.2007.09.010
85. Di Stasi A, Angelis De B, Rooney CM, Zhang L, Mahendravada A, Foster AE, et al. T Lymphocytes coexpressing CCR4 and a chimeric antigen receptor targeting CD30 have improved homing and antitumor activity in a Hodgkin tumor model. Blood (2009) 113(25):6392–402. doi: 10.1182/blood-2009-03-209650
86. Nagarsheth N, Wicha MS, Zou W. Chemokines in the cancer microenvironment and their relevance in cancer immunotherapy. Nat Rev Immunol (2017) 17(9):559–72. doi: 10.1038/nri.2017.49
87. Craddock JA, Lu A, Bear A, Pule M, Brenner MK, Rooney CM, et al. Enhanced tumor trafficking of GD2 chimeric antigen receptor T cells by expression of the chemokine receptor CCR2b. J Immunother (2010) 33(8):780–8. doi: 10.1097/CJI.0b013e3181ee6675
88. Cazzetta V, Bruni E, Terzoli S, Carenza C, Franzese S, Piazza R, et al. NKG2A expression identifies a subset of human Vdelta2 T cells exerting the highest antitumor effector functions. Cell Rep (2021) 37(3):109871. doi: 10.1016/j.celrep.2021.109871
89. Chmielewski M, Hombach AA, Abken H. Of CARs and TRUCKs: chimeric antigen receptor (CAR) T cells engineered with an inducible cytokine to modulate the tumor stroma. Immunol Rev (2014) 257(1):83–90. doi: 10.1111/imr.12125
90. Pegram HJ, Lee J C, Hayman EG, Imperato GH, Tedder TF, Sadelain M, et al. Tumor-targeted T cells modified to secrete IL-12 eradicate systemic tumors without need for prior conditioning. Blood (2012) 119(18):4133–41. doi: 10.1182/blood-2011-12-400044
91. Koneru M, Purdon TJ, Spriggs D, Koneru S, Brentjens RJ. IL-12 secreting tumor-targeted chimeric antigen receptor T cells eradicate ovarian tumors in vivo. Oncoimmunology (2015) 4(3):e994446. doi: 10.4161/2162402X.2014.994446
92. Yeku OO, Purdon TJ, Koneru M, Spriggs D, Brentjens RJ. Armored CAR T cells enhance antitumor efficacy and overcome the tumor microenvironment. Sci Rep (2017) 7(1):10541. doi: 10.1038/s41598-017-10940-8
93. Hoyos V, Savoldo B, Hoyos V, Weber G, Liu H, Kim ES, et al. Engineering CD19-specific T lymphocytes with interleukin-15 and a suicide gene to enhance their anti-lymphoma/leukemia effects and safety. Leukemia (2010) 24(6):1160–70. doi: 10.1038/leu.2010.75
94. Chmielewski M, Abken H. CAR T cells releasing IL-18 convert to T-bet(high) FoxO1(low) effectors that exhibit augmented activity against advanced solid tumors. Cell Rep (2017) 21(11):3205–19. doi: 10.1016/j.celrep.2017.11.063
95. Caruana I, Savoldo B, Hoyos V, Weber G, Liu H, Kim ES, et al. Heparanase promotes tumor infiltration and antitumor activity of CAR-redirected T lymphocytes. Nat Med (2015) 21(5):524–9. doi: 10.1038/nm.3833
96. Mardomi A, Abediankenari S. Matrix metalloproteinase 8: could it benefit the CAR-T cell therapy of solid tumors?- a- commentary on therapeutic potential. Cancer Microenviron (2018) 11(1):93–6. doi: 10.1007/s12307-018-0208-2
Keywords: T-cell therapy, γδTCR, cancer, immune therapy, antigen, fitness, TME (tumor microenvironment), migration
Citation: Meringa AD, Hernández-López P, Cleven A, de Witte M, Straetemans T, Kuball J, Beringer DX and Sebestyen Z (2023) Strategies to improve γδTCRs engineered T-cell therapies for the treatment of solid malignancies. Front. Immunol. 14:1159337. doi: 10.3389/fimmu.2023.1159337
Received: 05 February 2023; Accepted: 09 June 2023;
Published: 27 June 2023.
Edited by:
Maria Themeli, Amsterdam University Medical Center, NetherlandsReviewed by:
Jonathan Fisher, University College London, United KingdomCopyright © 2023 Meringa, Hernández-López, Cleven, de Witte, Straetemans, Kuball, Beringer and Sebestyen. This is an open-access article distributed under the terms of the Creative Commons Attribution License (CC BY). The use, distribution or reproduction in other forums is permitted, provided the original author(s) and the copyright owner(s) are credited and that the original publication in this journal is cited, in accordance with accepted academic practice. No use, distribution or reproduction is permitted which does not comply with these terms.
*Correspondence: Z. Sebestyen, ei5zZWJlc3R5ZW5AdW1jdXRyZWNodC5ubA==
†These authors have contributed equally to this work
Disclaimer: All claims expressed in this article are solely those of the authors and do not necessarily represent those of their affiliated organizations, or those of the publisher, the editors and the reviewers. Any product that may be evaluated in this article or claim that may be made by its manufacturer is not guaranteed or endorsed by the publisher.
Research integrity at Frontiers
Learn more about the work of our research integrity team to safeguard the quality of each article we publish.