- 1College of Public Health, Medical and Veterinary Sciences, James Cook University, Townsville, QLD, Australia
- 2Centre for Molecular Therapeutics, Australian Institute of Tropical Health and Medicine, James Cook University, Cairns, QLD, Australia
- 3Public Health Research Institute (PHRI), New Jersey Medical School, Rutgers University, Newark, NJ, United States
Tuberculosis (TB) remains one of the most lethal infectious diseases globally. The only TB vaccine approved by the World Health Organization, Bacille Calmette-Guérin (BCG), protects children against severe and disseminated TB but provides limited protection against pulmonary TB in adults. Although several vaccine candidates have been developed to prevent TB and are undergoing preclinical and clinical testing, BCG remains the gold standard. Currently, BCG is administered as an intradermal injection, particularly in TB endemic countries. However, mounting evidence from experimental animal and human studies indicates that delivering BCG directly into the lungs provides enhanced immune responses and greater protection against TB. Inhalation therapy using handheld delivery devices is used for some diseases and allows the delivery of drugs or vaccines directly into the human respiratory tract. Whether this mode of delivery could also be applicable for live attenuated bacterial vaccines such as BCG or other TB vaccine candidates remains unknown. Here we discuss how two existing inhalation devices, the mucosal atomization device (MAD) syringe, used for influenza vaccines, and the Respimat® Soft Mist™ inhaler, used for chronic obstructive pulmonary disease (COPD) therapy, could be repurposed for mucosal delivery of live attenuated TB vaccines. We also outline the challenges and outstanding research questions that will require further investigations to ensure usefulness of respiratory delivery devices that are cost-effective and accessible to lower- and middle-income TB endemic countries.
1 Introduction
Lower respiratory tract infections, excluding COVID-19, are the fourth major cause of death worldwide, with an annual incidence rate of 2.4 million (1). More than half of the mortality associated with respiratory infections is due to tuberculosis (TB), a bacterial disease predominantly caused by infections with Mycobacterium tuberculosis (Mtb). Although TB affects people globally, it is most prominent in low- and middle-income countries. In 2021, 10.6 million people suffered from active TB and 1.6 million people lost their lives worldwide (2).
Bacille Calmette-Guérin (BCG) is the only vaccine approved by the World Health Organization (WHO) to prevent disseminated forms of TB (meningitis and miliary) in children (3). BCG is a live attenuated strain of Mycobacterium bovis, a genetically related mycobacterial species to Mtb. The vaccine provides variable protection in adults, with the longest-lasting protection of 50-60 years reported in Alaskan natives and American Indians (4) and of 10-19 years in a Norwegian population (5). It has also been reported that BCG is less efficacious in TB endemic countries, including Asia and Africa, compared to non-endemic countries (6). The potential reasons for this variability in BCG efficacy have been discussed elsewhere (7).
BCG is marketed as a freeze-dried powder in an amber glass ampoule and transported to vaccination centers (8). Just before vaccination, the vial is reconstituted with a diluent (provided in a separate vial) at the vaccination center, and is administered to newborns (and sometimes older children) within six hours of reconstitution via the intradermal route by trained personnel (9). The BCG vaccination process can be daunting for both the receiving individual and the administering health worker.
There is mounting evidence from animal model studies (10–17) and historical observations in humans (18–24) that delivery of BCG via the mucosal route may be more efficacious and provides longer-lasting protection than the conventional intradermal route. While the correlates of protection after BCG vaccination are poorly defined/unknown, enhanced protection after mucosal vaccination has been linked to the generation of tissue-resident T cells (TRM). While intranasal, oral, intravenous and intratracheal route of BCG vaccination induces TRM in the lungs, parenteral intradermal or subcutaneous vaccination fails to do so (14, 16, 25–38).
Hence, many research groups have supported and proposed pulmonary delivery of TB vaccines, and progress has been made recently in this area of research (39). It is important to note that in addition to developing (a) suitable TB vaccine candidate(s) for mucosal delivery, investigations into suitable delivery devices for mass human application should be prioritized. As a disease of poverty in resource-limited and populous countries (40), mass vaccination using a reusable aerosol delivery device attached to a medical breathing circuit appears unviable and may carry a high risk of unintended TB transmission. Therefore, we believe that research efforts should be directed toward developing and evaluating a simple, cost-effective, user-friendly, and easily available single-use device with the ability to deliver the vaccine deep into human lungs. Both BCG (41) and MTBVAC (a promising TB vaccine in clinical trials) delivery via the intranasal route has successfully been tested in animal models, including those with co-morbidities (42). However, there is no published information on what kind of delivery device could deliver a live attenuated TB vaccine intranasally or intratracheally into human lungs. Below we discuss some of the challenges and opportunities associated with the mucosal delivery of live attenuated TB vaccines into human lungs.
2 Factors associated with vaccine efficacy by mucosal delivery
Generally, the efficacy and immunogenicity of a vaccine rely on a range of factors associated with the host, the environment, and the vaccine formulation, including the type, dose, administration route, needle size (if applicable), co-administered vaccines and timing (6). Vaccination with a live, attenuated BCG was more immunogenic than inactivated bacteria (43, 44), and the liquid formulation provoked a stronger immune response than the powder formulation (45). These results suggest that microbial viability in the vaccine formulation influences the host immune response, and that the ideal BCG replacement vaccine should constitute live bacteria.
Depending on the nature of the infecting Mtb strain, a minimum of three tubercle bacilli are enough to establish a productive infection (46). Inhalation of Mtb-containing aerosol droplets facilitates tubercle bacilli to reach the lung parenchyma, where they first encounter the alveolar lining fluid (ALF) or pulmonary surfactant. This fluid is comprised of lipids (90%) and proteins (10%). The lipid components decrease the surface tension and alter the multiplication and function of lymphocytes (47). On the other hand, the proteins interact with the surface glycolipids of the tubercle bacilli (48). Overall, the ALF actuates the pathogen capture and clearance by phagocytic cells such as alveolar macrophages. However, the absence of ALF due to preexisting conditions such as asthma or COPD increases host susceptibility to various infections, including TB (46, 49–51). It has been reported that Mtb blocks phago-lysosome fusion by perturbing the pH of the phagosome to 6.4. This suggests that Mtb prefers living in a slightly acidic environment, which may have important implications for the choice of diluent used to reconstitute the vaccine, to maximise the stability and viability of mucosally delivered BCG.
The Mtb/M. bovis cell wall consists of a thick waxy coat that protect the bacteria from the outside environment, contributing to the bacterial resistance to antibiotics (52). Based on pulmonary Mtb infection in model animals, it seems that this waxy coat contributes to bacterial survival in host lungs with varying physiological (pH, surface, partial pressure of oxygen and carbon dioxide, relative humidity, temperature and density) and microbial gradients (53). Thus, the waxy coat of live mycobacterial strains can impact the delivery of the vaccine directly into the lungs and affect subsequent host immune responses.
Despite lacking the region of difference 1 (RD1) (7), BCG contains several ‘decoy’ molecules, such as the glycoprotein LprG and Lipoarabinomannan (LAM), which evolved in support of virulence of mycobacteria. Both molecules delay the protective Th1 immune response via the induction of immunosuppressive cytokines and chemokines and could hence be counterproductive to prophylactic vaccination (54, 55).
3 Likelihood of mucosal vaccination in humans
One of the biggest challenges in TB vaccine development is the lack of a human challenge model that could be used to evaluate the efficacy of new vaccine candidates. This is not surprising as it is not possible to safely infect humans with Mtb due to ethical reasons. However, the TB research group at Oxford University has recently started recruiting a small number of healthy human volunteers (aged 18 to 50) to participate in a BCG challenge trial. This study assesses whether humans can be safely infected with BCG via the aerosol route. In the trial, volunteers receive an escalating dose of BCG using an aerosol delivery device. Subsequently, the researchers intend to collect lung washings of the volunteers to determine the amount of recoverable BCG, to discover new biomarkers potentially involved in protection against TB, and to validate the BCG challenge model as a new way to test TB vaccine candidates (56). Although the primary aim of this study is not to test the efficacy of mucosal BCG delivery as a vaccination strategy against subsequent Mtb infection, the trial will likely provide critical information regarding the safety and tolerability of aerosol BCG vaccination. In addition, this trial will likely help determine an optimal BCG dose for mucosal vaccination to minimize any BCG dose-dependent lung pathology reported by Tree et al. in a murine model of TB (57).
In fact, the respiratory tract as a potential vaccine delivery route is under active investigation for other infections. For the last two decades, several research groups have proposed the pulmonary site as an ideal target to deliver vaccines and induce immunity to combat respiratory infections (58–61). To date, there are only six licensed intranasal vaccines for humans (Table 1), including vaccines against COVID-19 (68). This delay in the development of inhaled vaccines highlights the challenges underpinning the delivery of antigens into the respiratory tract. However, given the promising results from animal studies, an inhaled vaccine may be what is needed to protect against TB.
4 Potential TB vaccine delivery devices
A key component in mucosal delivery of a live TB vaccine to millions of people globally, including children, is the inhalation device. There are more than 230 inhalers available with varying mechanics that could conceptually be used to deliver TB vaccines (Table 2) (69, 72). For example, COPD and asthmatic patients are routinely prescribed specific inhalers for delivering disease-specific drugs directly into the lungs. This raises the possibility of using these pulmonary inhalers to deliver live mycobacteria vaccines. Currently, a broad range of liquid, mist, and powder-based inhalers are available and frequently prescribed (73). Based on the vaccine (liquid or powder) formulation, some of those available inhalers could be validated as a delivery device for inhaled live attenuated TB vaccines. However, given the low cost of current intradermal BCG vaccination, the cost of goods associated with making an inhalation delivery device will need to be considered to allow for global rollout. Furthermore, a needle-free delivery system, as an alternate to the current intradermal BCG vaccination should be simple and easy to use in infants and young children, particularly in resource-limited TB endemic countries.
In general, the inhalation capacity is compromised in those with preexisting health conditions such as asthma or COPD. In such situations, it is important to choose an inhaler whose actuation mechanism is preferably independent of the user’s inspiration rate to ensure the delivery of an accurate vaccine dose. This criterion narrows the list of suitable inhalers to only a few (Table 2). Below we elaborate on one potential device each for intratracheal and intranasal inhalation.
4.1 Intratracheal
The Respimat® Soft Mist™ inhaler (SMI; Boehringer Ingelheim, Ingelheim, Germany) (Figure 1A) is prescribed as an inhalation therapy to patients with COPD. It is a multidose, propellent-free liquid inhaler that produces an aerosol plume (75) of small droplet size with low momentum (76) on pressing a button. It delivers the bronchodilators (10-15 µL per actuation) at the velocity of 0.8m/sec that last longer (1.5 seconds) than other propellant-based inhalers. This allows synchronization of actuation and inspiration for better drug delivery (77).
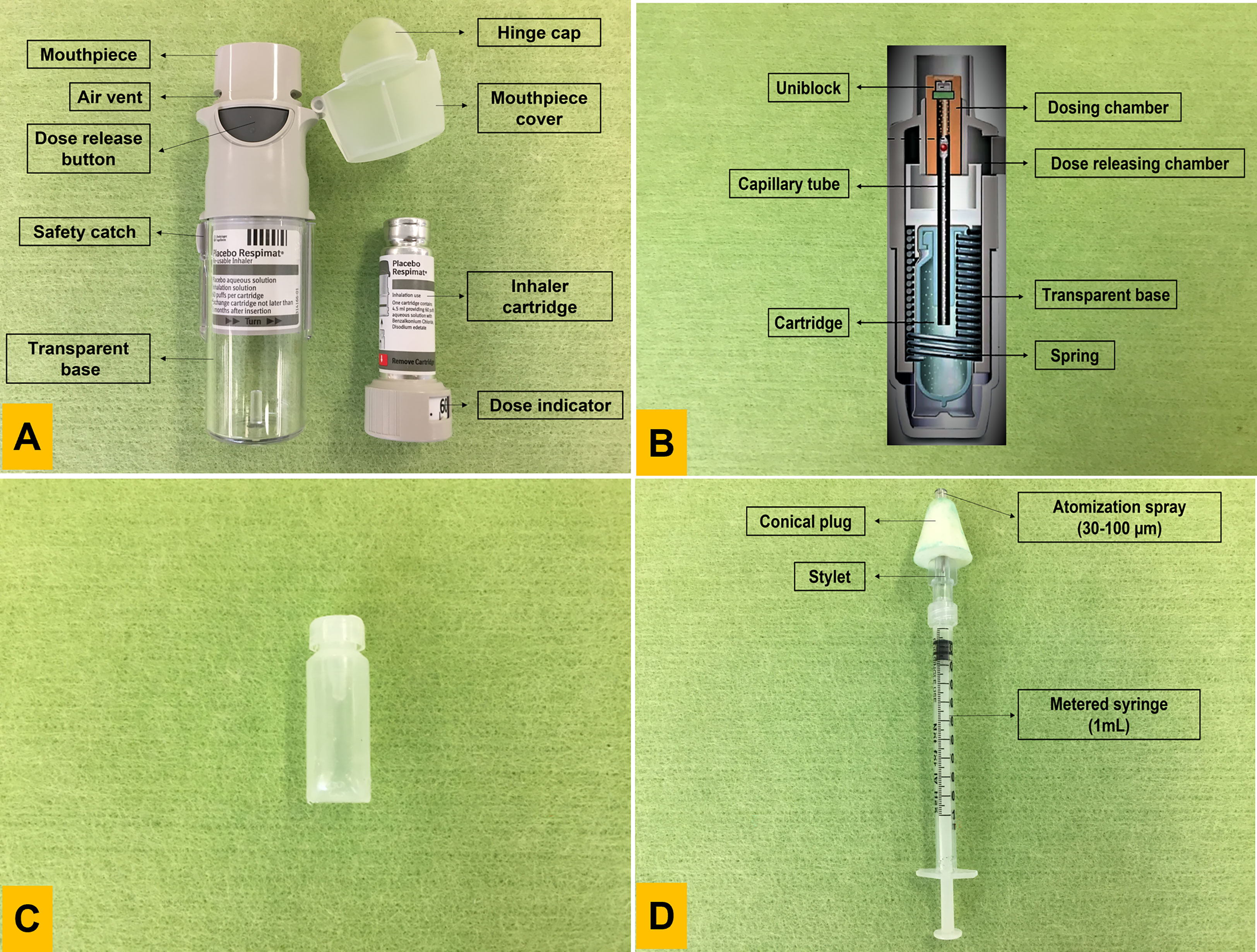
Figure 1 The Respimat inhaler and Mucosal Atomization Device syringe. (A) Usability diagram explaining its structural components and cartridge; (B) Cross section of the inhaler vertically to understand the working mechanics of the device; (C) Respimat inhaler cartridge that holds the drug. The plastic pouch is protected by an outer aluminium shell connected to a dose indicator; (D) The atomization device is connected to the syringe for mist production. Both components can be separated and reconnected as a single unit. Photo B was modified from (74).
A spring (Figure 1B) provides mechanical energy that forces the solution through a fine nozzle (uniblock), thereby atomizing the solution release as two fine liquid jets. When those jets collide at a pre-set angle, it causes the production of a particle cloud or soft mist, which allows inhalation deep into the lung (78). A study by Taube et al. analysed data from two independent studies, comprised of over 90,000 patients on the performance of Respimat® Soft Mist™ inhaler. They reported that 85% and 84% of patients, respectively, were content with using and handling the inhaler. Interestingly, over 95% of people continued using the inhaler after the study ended (79).
Could the Respimat® Soft Mist™ inhaler be used as a mucosal TB vaccine delivery device? Conceptually, replacing the solution contained in the Respimat® Soft Mist™ inhaler cartridge with the live TB vaccine may be possible. The inhaler cartridge (Figure 1C) is composed of an aluminium cylinder that accommodates a contractable double-walled plastic pouch containing liquid solution (80). However, open questions such as the following remain: 1) Can the cartridge deliver viable mycobacterial bacilli deep into the lungs? 2) Can the device deliver a consistent dose? 3) What is the viability of live mycobacteria within the cartridge environment? 4) Is the nozzle size suitable for delivery of the waxy cell wall of mycobacteria? 5) Is the inhaler suitable for use in infants and young children? 6) What are the implications for storage, transport, and shelf life of the inhaler?
4.2 Intranasal
The mucosal atomization device (MAD) syringe (Figure 1D) is composed of a metered syringe (usually 1mL) connected to an atomization device that generates aerosols similar to the Respimat® Soft Mist™ inhaler. The MAD consists of an atomization spray (the mist generator), an inlet to allow a 180° posture of a nasal plug, and a soft conical plug that acts as a nose seal to abstain expulsion of the vaccine. The mist produced by the device generates particles of 30-100 µm size. The device is patient actuated. The syringe plunger pushes the liquid through the MAD, and the atomization device converts the pushed liquid into a mist.
The device mechanism is simple and has successfully been used to deliver influenza vaccines such as Flumist™ and Nasovac. The simplistic aerosol-producing mechanism of the syringe makes the MAD economical and affordable. Furthermore, the convenience, ease of use and availability in low- and middle-income countries imply that the MAD could be another potential delivery device for live TB vaccines. Given that the MAD has already been approved and used to deliver an influenza vaccine via the intranasal route (Table 1), regulatory approval processes and manufacture should be translatable to TB vaccines.
Recently, Wei et al. have reported that 0.3mL was the optimum volume for intranasal delivery of drugs via the MAD without tracheal aspiration in rabbits. However, with a delivery volume of 0.45 or 0.6mL, the MAD was able to deliver particles to the rabbit trachea (81). Although these findings demonstrate a proof-of-principle that the MAD syringe can potentially deliver drugs/vaccines to the lower respiratory tract, further investigations are required to evaluate the optimal delivery volume of TB vaccines, particularly in humans.
BCG is generally administered right after birth or within the first few months of life (7). Compared to adults, newborns are characterized by distinctive breathing patterns (high breathing frequency up to 40 breaths per minute) (82). This variation is due to the difference in the nasal anatomy, chest wall geometry, respiratory muscles, presence of fluids and pressure (83). Wilkins and colleagues evaluated the MAD syringe for its intranasal vaccine delivery using five nasal replicas of infants aged 3-24 months. The study reported an overall delivery efficacy of 86.57 ± 14.23% for all models when administering 0.1mL of a model vaccine in each nostril (84). While these findings suggest that the MAD syringe may be suitable as a TB vaccine delivery device for neonates, the Respimat® Soft Mist™ inhaler on the other hand may require further evaluations as a suitable delivery device for newborns. Nevertheless, based on the mechanics described above, it is likely that both delivery devices could be used to deliver not only BCG but also TB vaccine candidates that are based on viral vectors, protein subunits or nucleic acids.
5 Discussion
To further validate the suitability of the Respimat® Soft Mist™ inhaler and the MAD for the delivery of live mycobacteria, the following points should be considered: firstly, the devices should be evaluated for consistency in the delivery dose. Once accurate delivery has been confirmed, testing could be extended to 3D models of the human respiratory system. Using a human respiratory CT scan, specialized software such as Mimics (Materialise, Belgium) could print a 3D human respiratory system using a medical-grade printer. This would allow inhaler efficiency testing in a human-like anatomical system. Lastly, the proposed devices should be tested using a next-gen impactor, a specialized pharmaceutical instrument used to determine the drug/vaccine’s aerodynamic particle size and depth of deposition of particles in the lungs.
The rod-shaped M. bovis is 2-4 µm long and 0.2-0.5 µm in diameter (85). This size is compatible with the minimum requirement of a particle (0.5-5 µm aerodynamic size) to get delivered deep into the lungs by sedimentation (Figure 2) (87). Adding a surfactant to the liquid vaccine formulation would further decrease the surface tension and increase the vaccine dispersal inside the lungs (88). Nevertheless, maintaining the vaccine formulation within the device without a reduction in bacterial viability represents another challenge. However, based on the literature from BCG vaccine studies, it is logical to assume that cold storage of cartridges containing the vaccine formulations should be feasible without significantly compromising the bacterial viability. The widespread global use of mRNA-based COVID-19 vaccines, which require storage at very low temperatures, has shown that logistics and storage conditions are no longer a bottleneck for maintaining and delivering vaccines that require deep freezing.
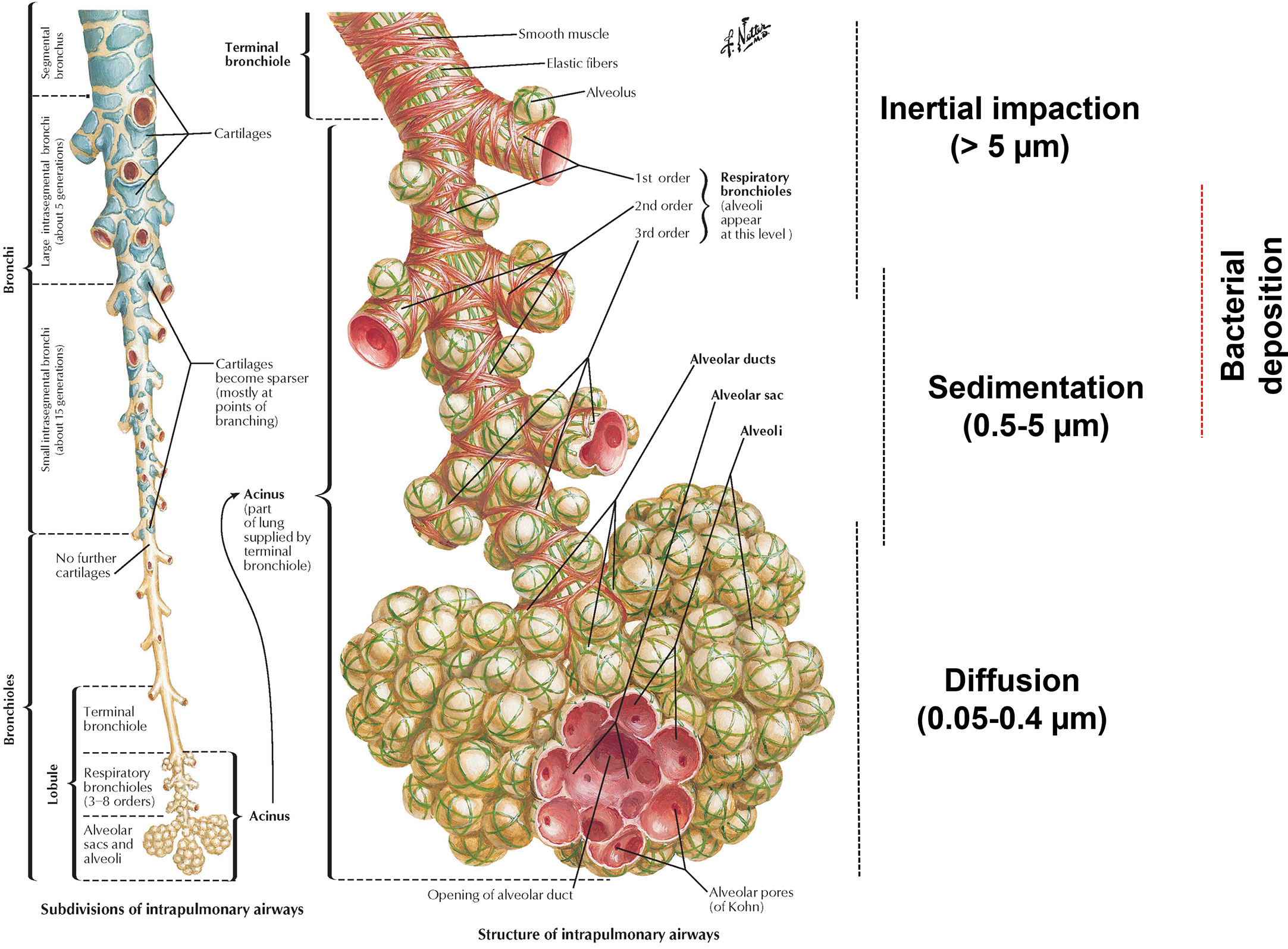
Figure 2 The relationship between particle size and lung deposition. The left section demonstrates the structure of intrapulmonary airways. The right section details the bacterial deposition based on size (0.5-10 µm) in the lower respiratory tract (86). The picture usage rights were purchased from netterimages.com (Image ID: 49221).
Currently, BCG is given predominantly to children through intradermal injection, and as such mucosal delivery devices for BCG vaccination should be applicable to the national vaccination program for children of all age groups, including infants in TB-endemic countries. In addition, BCG re-vaccination in adolescents and adults is receiving renewed interest for improving the protection against TB. In addition to the unexpected positive results of the BCG re-vaccination control group in an H4IC31 trial (89), Rakshit et al. demonstrated that BCG re-vaccination (TUBERVAC, BCG Russia) greatly stimulated Ag85A and BCG-specific CD4+ T and CD8+ T cells in previously BCG vaccinated (at birth) health care workers. Additionally, BCG re-vaccination also increased mycobacteria-specific Th 17 responses (90), and a systematic review conducted by Bannister et al. indicated that BCG is safe to use as a booster dose (91). Collectively, these studies demonstrate the benefits of a booster BCG dose in adolescents and adults. Thus, an easy-to-use mucosal delivery device would likely simplify BCG re-vaccination programs.
The licensed BCG vaccine strains are available as a lyophilized powder (1.5 mg) that is reconstituted with a diluent (0.9% sodium chloride and diluted Sauton medium) just before administration (92). However, the proportion of viable bacteria in the prepared vaccine suspension strongly influences the magnitude of the host immune response (8, 45). It is also known that the thermal stability of a powder formulation is greater than that of a liquid (93). Hence, it is feasible to assume that the cartridges for mucosal delivery devices could also be filled with lyophilized bacteria, with the ability to reconstitute at the vaccination center just before inhalation.
The user instructions of approved mucosal delivery units strongly recommend holding the breath for at least 10 seconds immediately after usage (94, 95). This is followed by rinsing the mouth with water in some cases. Therefore, while delivering a live attenuated recombinant TB vaccine with these types of inhalers or an intranasal syringe may require additional procedures to ensure accidental exposure and/or transmission of the vaccine to other people or the environment. All these issues require further investigation, and data from such studies need to be considered when designing a vaccine delivery device for TB control.
Currently, the Respimat® Soft Mist™ inhaler costs approximately US$45, excluding the cartridge. It is a multi-dose inhaler that holds 4.5 mL of fluid in each cartridge. The same inhaler can be used for up to six cartridges before being discarded. However, a more simplistic version could be developed to deliver a single-dose TB vaccine. For example, by excluding the mouthpiece cap and dose indicator (Figure 1A), a more transparent base could be produced to verify proper cartridge insertion. Such a generic, single-use and easily understandable mechanism might assist in reducing the overall manufacturing cost of the delivery device. Local production may also impact manufacturing costs and may increase affordability in low- and middle-income countries.
Since the Respimat® Soft Mist™ inhaler delivers the content of the cartridge independently of the patient’s inhalation rate (96), it could also be used to deliver a TB vaccine effectively in patients with co-morbid respiratory diseases. The optical density of the bronchodilator solution given for COPD using the Respimat® Soft Mist™ inhaler will likely differ from the TB vaccine candidate formulation. This may require further adjustments to the device (angle to produce fine liquid jets, increasing nozzle diameter, compatibility of the plastic cartridge pouch with the vaccine etc.). The MAD syringe, on the other hand, costs around US$6 and is based on relatively simple mechanics. If proven efficient in delivering a live attenuated TB vaccine via the pulmonary route, this device may have broad application globally, including in resource-limited countries.
In conclusion, emerging research findings support the idea that mucosal delivery of TB vaccines might confer superior protection than intradermal inoculations. Here, we propose that mucosal delivery of live attenuated TB vaccines, including BCG, is feasible and could be done by repurposing existing delivery devices for other respiratory diseases. Further research is required to dissect the challenges outlined above and to validate the usability of various inhaler types. Once proven technically feasible and safe, introducing an inhalation platform for the TB vaccine will undoubtedly contribute to increased vaccination rates globally and, consequently, to a reduction of TB burden.
Data availability statement
The original contributions presented in the study are included in the article/supplementary material. Further inquiries can be directed to the corresponding author.
Author contributions
MP wrote the manuscript and proposed the delivery devices. AK, SM-H, and SS provided editorial and intellectual input. All authors contributed to the article and approved the submitted version.
Funding
SS and AK are supported by a grant from NIAID Grant number: 1R01AI161822-01. AK is also supported by an NHMRC Investigator Grant (APP2008715).
Acknowledgments
Figure 2 was purchased from Netter™ Images (Elsevier). Figure 1B was modified from Iwanaga, et al. Clin Drug Investig 39, 1021-1030.
Conflict of interest
The authors declare that the research was conducted in the absence of any commercial or financial relationships that could be construed as a potential conflict of interest.
Publisher’s note
All claims expressed in this article are solely those of the authors and do not necessarily represent those of their affiliated organizations, or those of the publisher, the editors and the reviewers. Any product that may be evaluated in this article, or claim that may be made by its manufacturer, is not guaranteed or endorsed by the publisher.
References
1. Lavelle EC, Ward RW. Mucosal vaccines - fortifying the frontiers. Nat Rev Immunol (2022) 22(4):236–50. doi: 10.1038/s41577-021-00583-2
2. WHO. Global tuberculosis report. (2022). Available at: https://www.who.int/teams/global-tuberculosis-programme/tb-reports.
3. Kupz A. Live attenuated vaccines for tuberculosis. Biochemist (2021) 43(4):28–32. doi: 10.1042/bio_2021_149
4. Aronson NE, Santosham M, Comstock GW, Howard RS, Moulton LH, Rhoades ER, et al. Long-term efficacy of BCG vaccine in American indians and Alaska natives: A 60-year follow-up study. Jama (2004) 291(17):2086–91. doi: 10.1001/jama.291.17.2086
5. Nguipdop-Djomo P, Heldal E, Rodrigues LC, Abubakar I, Mangtani P. Duration of BCG protection against tuberculosis and change in effectiveness with time since vaccination in Norway: A retrospective population-based cohort study. Lancet Infect Dis (2016) 16(2):219–26. doi: 10.1016/s1473-3099(15)00400-4
6. Lynn DJ, Benson SC, Lynn MA, Pulendran B. Modulation of immune responses to vaccination by the microbiota: Implications and potential mechanisms. Nat Rev Immunol (2022) 22(1):33–46. doi: 10.1038/s41577-021-00554-7
7. Ahmed A, Rakshit S, Adiga V, Dias M, Dwarkanath P, D’Souza G, et al. A century of BCG: Impact on tuberculosis control and beyond. Immunol Rev (2021) 301(1):98–121. doi: 10.1111/imr.12968
8. Angelidou A, Conti MG, Diray-Arce J, Benn CS, Shann F, Netea MG, et al. Licensed Bacille Calmette-Guérin (BCG) formulations differ markedly in bacterial viability, RNA content and innate immune activation. Vaccine (2020) 38(9):2229–40. doi: 10.1016/j.vaccine.2019.11.060
9. World Health Organisation. WHO guidance note: Vaccine diluents, revision 2015 (2015). Available at: https://www.who.int/publications/i/item/WHO-IVB-15.08.
10. Barclay WR, Busey WM, Dalgard DW, Good RC, Janicki BW, Kasik JE, et al. Protection of monkeys against airborne tuberculosis by aerosol vaccination with bacillus calmette-guerin. Am Rev Respir Dis (1973) 107(3):351–8. doi: 10.1164/arrd.1973.107.3.351
11. Garcia-Contreras L, Wong YL, Muttil P, Padilla D, Sadoff J, Derousse J, et al. Immunization by a bacterial aerosol. Proc Natl Acad Sci U.S.A. (2008) 105(12):4656–60. doi: 10.1073/pnas.0800043105
12. Derrick SC, Kolibab K, Yang A, Morris SL. Intranasal administration of mycobacterium bovis BCG induces superior protection against aerosol infection with mycobacterium tuberculosis in mice. Clin Vaccine Immunol (2014) 21(10):1443–51. doi: 10.1128/cvi.00394-14
13. Perdomo C, Zedler U, Kühl AA, Lozza L, Saikali P, Sander LE, et al. Mucosal BCG vaccination induces protective lung-resident memory T cell populations against tuberculosis. mBio (2016) 7(6):e01686-16. doi: 10.1128/mBio.01686-16
14. Bull NC, Stylianou E, Kaveh DA, Pinpathomrat N, Pasricha J, Harrington-Kandt R, et al. Enhanced protection conferred by mucosal BCG vaccination associates with presence of antigen-specific lung tissue-resident PD-1(+) KLRG1(-) CD4(+) T cells. Mucosal Immunol (2019) 12(2):555–64. doi: 10.1038/s41385-018-0109-1
15. Aguilo N, Uranga S, Mata E, Tarancon R, Gomez AB, Marinova D, et al. Respiratory immunization with a whole cell inactivated vaccine induces functional mucosal immunoglobulins against tuberculosis in mice and non-human primates. Front Microbiol (2020) 11:1339. doi: 10.3389/fmicb.2020.01339
16. Sathkumara HD, Muruganandah V, Cooper MM, Field MA, Alim MA, Brosch R, et al. Mucosal delivery of ESX-1-expressing BCG strains provides superior immunity against tuberculosis in murine type 2 diabetes. Proc Natl Acad Sci U.S.A. (2020) 117(34):20848–59. doi: 10.1073/pnas.2003235117
17. Heijmenberg I, Husain A, Sathkumara HD, Muruganandah V, Seifert J, Miranda-Hernandez S, et al. ESX-5-targeted export of ESAT-6 in BCG combines enhanced immunogenicity & efficacy against murine tuberculosis with low virulence and reduced persistence. Vaccine (2021) 39(50):7265–76. doi: 10.1016/j.vaccine.2021.08.030
18. Gandevia B. Historical review of the use of parasympatholytic agents in the treatment of respiratory disorders. Postgrad Med J (1975) 51(7 suppl):13–20.
19. Feldman RP, Goodrich JT. The Edwin smith surgical papyrus. Childs Nerv Syst (1999) 15(6-7):281–4. doi: 10.1007/s003810050395
20. Nikander K, Turpeinen M, Wollmer P. The conventional ultrasonic nebulizer proved inefficient in nebulizing a suspension. J Aerosol Med (1999) 12(2):47–53. doi: 10.1089/jam.1999.12.47
21. Norn S, Kruse PR, Kruse E. [History of opium poppy and morphine]. Dan Medicinhist Arbog (2005) 33:171–84.
22. Sanders M. Inhalation therapy: An historical review. Prim Care Respir J (2007) 16(2):71–81. doi: 10.3132/pcrj.2007.00017
23. Heydari M, Hashempur MH, Zargaran A. Medicinal aspects of opium as described in avicenna’s canon of medicine. Acta Med Hist Adriat (2013) 11(1):101–12.
24. Alizadeh A, Moshiri M, Alizadeh J, Balali-Mood M. Black henbane and its toxicity - a descriptive review. Avicenna J Phytomed (2014) 4(5):297–311.
25. Xing Z, McFarland CT, Sallenave JM, Izzo A, Wang J, McMurray DN. Intranasal mucosal boosting with an adenovirus-vectored vaccine markedly enhances the protection of BCG-primed guinea pigs against pulmonary tuberculosis. PloS One (2009) 4(6):e5856. doi: 10.1371/journal.pone.0005856
26. White AD, Sibley L, Dennis MJ, Gooch K, Betts G, Edwards N, et al. Evaluation of the safety and immunogenicity of a candidate tuberculosis vaccine, MVA85A, delivered by aerosol to the lungs of macaques. Clin Vaccine Immunol (2013) 20(5):663–72. doi: 10.1128/cvi.00690-12
27. Blazevic A, Eickhoff CS, Stanley J, Buller MR, Schriewer J, Kettelson EM, et al. Investigations of TB vaccine-induced mucosal protection in mice. Microbes Infect (2014) 16(1):73–9. doi: 10.1016/j.micinf.2013.09.006
28. Jeyanathan M, Shao ZQ, Yu XF, Harkness R, Jiang R, Li JQ, et al. AdHu5Ag85A respiratory mucosal boost immunization enhances protection against pulmonary tuberculosis in BCG-primed non-human primates. PloS One (2015) 10(8):20. doi: 10.1371/journal.pone.0135009
29. Lai R, Afkhami S, Haddadi S, Jeyanathan M, Xing Z. Mucosal immunity and novel tuberculosis vaccine strategies: Route of immunisation-determined T-cell homing to restricted lung mucosal compartments. Eur Respir Rev (2015) 24(136):356–60. doi: 10.1183/16000617.00002515
30. Ahmed M, Smith DM, Hamouda T, Rangel-Moreno J, Fattom A, Khader SA. A novel nanoemulsion vaccine induces mucosal interleukin-17 responses and confers protection upon mycobacterium tuberculosis challenge in mice. Vaccine (2017) 35(37):4983–9. doi: 10.1016/j.vaccine.2017.07.073
31. Gupta T, LaGatta M, Helms S, Pavlicek RL, Owino SO, Sakamoto K, et al. Evaluation of a temperature-restricted, mucosal tuberculosis vaccine in guinea pigs. Tuberculosis (Edinb) (2018) 113:179–88. doi: 10.1016/j.tube.2018.10.006
32. Thakur A, Rodríguez-Rodríguez C, Saatchi K, Rose F, Esposito T, Nosrati Z, et al. Dual-isotope SPECT/CT imaging of the tuberculosis subunit vaccine H56/CAF01: Induction of strong systemic and mucosal IgA and T-cell responses in mice upon subcutaneous prime and intrapulmonary boost immunization. Front Immunol (2018) 9:2825. doi: 10.3389/fimmu.2018.02825
33. Eickhoff CS, Blazevic A, Killoran EA, Morris MS, Hoft DF. Induction of mycobacterial protective immunity by sublingual BCG vaccination. Vaccine (2019) 37(36):5364–70. doi: 10.1016/j.vaccine.2019.07.034
34. Thakur A, Pinto FE, Hansen HS, Andersen P, Christensen D, Janfelt C, et al. Intrapulmonary (i.pulmon.) pull immunization with the tuberculosis subunit vaccine candidate H56/CAF01 after intramuscular (i.m.) priming elicits a distinct innate myeloid response and activation of antigen-presenting cells than i.m. or i.pulmon. prime immunization alone. Front Immunol (2020) 11:803. doi: 10.3389/fimmu.2020.00803
35. Dijkman K, Aguilo N, Boot C, Hofman SO, Sombroek CC, Vervenne RAW, et al. Pulmonary MTBVAC vaccination induces immune signatures previously correlated with prevention of tuberculosis infection. Cell Rep Med (2021) 2(1):100187. doi: 10.1016/j.xcrm.2020.100187
36. Ning H, Zhang W, Kang J, Ding T, Liang X, Lu Y, et al. Subunit vaccine ESAT-6:c-di-AMP delivered by intranasal route elicits immune responses and protects against mycobacterium tuberculosis infection. Front Cell Infect Microbiol (2021) 11:647220. doi: 10.3389/fcimb.2021.647220
37. Riste M, Marshall JL, Satti I, Harris SA, Wilkie M, Lopez Ramon R, et al. Phase I trial evaluating the safety and immunogenicity of candidate TB vaccine MVA85A, delivered by aerosol to healthy m.tb-infected adults. Vaccines (Basel) (2021) 9(4):396. doi: 10.3390/vaccines9040396
38. Liang ZM, Li H, Qu MJ, Liu YD, Wang YZ, Wang HR, et al. Intranasal bovine beta-clefensin-5 enhances antituberculosis immunity in a mouse model by a novel protein-based respiratory mucosal vaccine. Virulence (2022) 13(1):949–62. doi: 10.1080/21505594.2022.2080342
39. Kaur SS. Pulmonary drug delivery system: Newer patents. Pharm Pat Anal (2017) 6(5):225–44. doi: 10.4155/ppa-2017-0019
40. McNerney R, Maeurer M, Abubakar I, Marais B, McHugh TD, Ford N, et al. Tuberculosis diagnostics and biomarkers: Needs, challenges, recent advances, and opportunities. J Infect Dis (2012) 205 Suppl 2:S147–158. doi: 10.1093/infdis/jir860
41. Leversen NA, Sviland L, Wiker HG, Mustafa T. Long-term persistence of BCG pasteur in lungs of C57BL/6 mice following intranasal infection. Scand J Immunol (2012) 75(5):489–99. doi: 10.1111/j.1365-3083.2012.02683.x
42. Tarancón R, Mata E, Uranga S, Gómez AB, Marinova D, Otal I, et al. Therapeutic efficacy of pulmonary live tuberculosis vaccines against established asthma by subverting local immune environment. EBioMedicine (2021) 64:103186. doi: 10.1016/j.ebiom.2020.103186
43. Chen JM, Alexander DC, Behr MA, Liu J. Mycobacterium bovis BCG vaccines exhibit defects in alanine and serine catabolism. Infect Immun (2003) 71(2):708–16. doi: 10.1128/IAI.71.2.708-716.2003
44. Dockrell HM, Butkeviciute E. Can what have we learnt about BCG vaccination in the last 20 years help us to design a better tuberculosis vaccine? Vaccine (2022) 40(11):1525–33. doi: 10.1016/j.vaccine.2021.01.068
45. Price DN, Kunda NK, Ellis R, Muttil P. Design and optimization of a temperature-stable dry powder BCG vaccine. Pharm Res (2019) 37(1):11. doi: 10.1007/s11095-019-2739-8
46. Chandra P, Grigsby SJ, Philips JA. Immune evasion and provocation by mycobacterium tuberculosis. Nat Rev Microbiol (2022) 20(12):750–66. doi: 10.1038/s41579-022-00763-4
47. Han S, Mallampalli RK. The role of surfactant in lung disease and host defense against pulmonary infections. Ann Am Thorac Soc (2015) 12(5):765–74. doi: 10.1513/AnnalsATS.201411-507FR
48. Arcos J, Sasindran SJ, Fujiwara N, Turner J, Schlesinger LS, Torrelles JB. Human lung hydrolases delineate mycobacterium tuberculosis-macrophage interactions and the capacity to control infection. J Immunol (2011) 187(1):372–81. doi: 10.4049/jimmunol.1100823
49. Hohlfeld J, Fabel H, Hamm H. The role of pulmonary surfactant in obstructive airways disease. Eur Respir J (1997) 10(2):482–91. doi: 10.1183/09031936.97.10020482
50. Ferguson JS, Voelker DR, McCormack FX, Schlesinger LS. Surfactant protein d binds to mycobacterium tuberculosis bacilli and lipoarabinomannan via carbohydrate-lectin interactions resulting in reduced phagocytosis of the bacteria by macrophages. J Immunol (1999) 163(1):312–21. doi: 10.4049/jimmunol.163.1.312
51. Agudelo CW, Kumley BK, Area-Gomez E, Xu Y, Dabo AJ, Geraghty P, et al. Decreased surfactant lipids correlate with lung function in chronic obstructive pulmonary disease (COPD). PloS One (2020) 15(2):e0228279. doi: 10.1371/journal.pone.0228279
52. Abdallah AM, Gey van Pittius NC, Champion PA, Cox J, Luirink J, Vandenbroucke-Grauls CM, et al. Type VII secretion–mycobacteria show the way. Nat Rev Microbiol (2007) 5(11):883–91. doi: 10.1038/nrmicro1773
53. Man WH, de Steenhuijsen Piters WAA, Bogaert D. The microbiota of the respiratory tract: gatekeeper to respiratory health. Nat Rev Microbiol (2017) 15(5):259–70. doi: 10.1038/nrmicro.2017.14
54. Olsen AW, Hansen PR, Holm A, Andersen P. Efficient protection against mycobacterium tuberculosis by vaccination with a single subdominant epitope from the ESAT-6 antigen. Eur J Immunol (2000) 30(6):1724–32. doi: 10.1002/1521-4141(200006)30:6<1724::Aid-immu1724>3.0.Co;2-a
55. Ivanyi J. Tuberculosis vaccination needs to avoid ‘decoy’ immune reactions. Tuberculosis (Edinb) (2021) 126:102021. doi: 10.1016/j.tube.2020.102021
56. University of Oxford. Aerosol-based TB vaccine begins human trials. (2022). Available at: https://www.ox.ac.uk/news/2022-07-06-aerosol-based-tb-vaccine-begins-human-trial.
57. Tree JA, Williams A, Clark S, Hall G, Marsh PD, Ivanyi J. Intranasal Bacille Calmette-Guérin (BCG) vaccine dosage needs balancing between protection and lung pathology. Clin Exp Immunol (2004) 138(3):405–9. doi: 10.1111/j.1365-2249.2004.02648.x
58. Bivas-Benita M, Ottenhoff THM, Junginger HE, Borchard G. Pulmonary DNA vaccination: Concepts, possibilities and perspectives. J Controlled Release (2005) 107(1):1–29. doi: 10.1016/j.jconrel.2005.05.028
59. Kraan H, Vrieling H, Czerkinsky C, Jiskoot W, Kersten G, Amorij JP. Buccal and sublingual vaccine delivery. J Controlled Release (2014) 190:580–92. doi: 10.1016/j.jconrel.2014.05.060
60. Marasini N, Kaminskas LM. Subunit-based mucosal vaccine delivery systems for pulmonary delivery - are they feasible? Drug Dev Ind Pharm (2019) 45(6):882–94. doi: 10.1080/03639045.2019.1583758
61. Trincado V, Gala RP, Morales JO. Buccal and sublingual vaccines: A review on oral mucosal immunization and delivery systems. Vaccines (2021) 9(10):20. doi: 10.3390/vaccines9101177
62. Mossad SB. Demystifying FluMist, a new intranasal, live influenza vaccine. Cleve Clin J Med (2003) 70(9):801–6. doi: 10.3949/ccjm.70.9.801
63. Dhere R, Yeolekar L, Kulkarni P, Menon R, Vaidya V, Ganguly M, et al. A pandemic influenza vaccine in India: From strain to sale within 12 months. Vaccine (2011) 29 Suppl 1:A16–21. doi: 10.1016/j.vaccine.2011.04.119
64. Bharat Biotech. BBV154 - a novel adenovirus vectored, intranasal vaccine for COVID-19. (2022). Available at: https://www.bharatbiotech.com/intranasal-vaccine.html.
65. CanSino Biologics. CanSino biologics celebrates the rollout of the inhaled vaccine, convidecia air™, in shanghai (2022). Available at: https://www.cansinotech.com/html/1/179/180/1113.html.
66. Banihashemi SR, Es-Haghi A, Fallah Mehrabadi MH, Nofeli M, Mokarram AR, Ranjbar A, et al. Safety and efficacy of combined Intramuscular/Intranasal RAZI-COV PARS vaccine candidate against SARS-CoV-2: A preclinical study in several animal models. Front Immunol (2022) 13:836745. doi: 10.3389/fimmu.2022.836745
67. Balakrishnan VS. The arrival of Sputnik V. Lancet Infect Dis (2020) 20(10):1128. doi: 10.1016/s1473-3099(20)30709-x
68. Waltz E. China And India approve nasal COVID vaccines - are they a game changer? Nature (2022) 609(7927):450. doi: 10.1038/d41586-022-02851-0
70. Sanchis J, Corrigan C, Levy ML, Viejo JL. Inhaler devices - from theory to practice. Respir Med (2013) 107(4):495–502. doi: 10.1016/j.rmed.2012.12.007
71. Molimard M, Kottakis I, Jauernig J, Lederhilger S, Nikolaev I. Performance characteristics of breezhaler(®) and aerolizer(®) in the real-world setting. Clin Drug Investig (2021) 41(5):415–24. doi: 10.1007/s40261-021-01021-w
72. Cataldo D, Hanon S, Peché RV, Schuermans DJ, Degryse JM, De Wulf IA, et al. How to choose the right inhaler using a patient-centric approach? Adv Ther (2022) 39(3):1149–63. doi: 10.1007/s12325-021-02034-9
73. Ortsäter G, Borgström F, Baldwin M, Miltenburger C. Incorporating the environmental impact into a budget impact analysis: The example of adopting RESPIMAT(®) re-usable inhaler. Appl Health Econ Health Policy (2020) 18(3):433–42. doi: 10.1007/s40258-019-00540-0
74. Iwanaga T, Tohda Y, Nakamura S, Suga Y. The Respimat((R)) soft mist inhaler: Implications of drug delivery characteristics for patients. Clin Drug Investig (2019) 39(11):1021–30. doi: 10.1007/s40261-019-00835-z
75. Anderson P. Use of respimat soft mist inhaler in COPD patients. Int J Chron Obstruct Pulmon Dis (2006) 1(3):251–9. doi: 10.2147/copd.2006.1.3.251
76. Worth Longest P, Hindle M. Evaluation of the respimat soft mist inhaler using a concurrent CFD and in vitro approach. J Aerosol Med Pulm Drug Delivery (2009) 22(2):99–112. doi: 10.1089/jamp.2008.0708
77. Mehri R, Alatrash A, Ogrodnik N, Matida EA, Fiorenza F. In vitro measurements of spiriva respimat dose delivery in mechanically ventilated tracheostomy patients. J Aerosol Med Pulm Drug Delivery (2021) 34(4):242–50. doi: 10.1089/jamp.2019.1570
78. Sorino C, Negri S, Spanevello A, Visca D, Scichilone N. Inhalation therapy devices for the treatment of obstructive lung diseases: the history of inhalers towards the ideal inhaler. Eur J Intern Med (2020) 75:15–8. doi: 10.1016/j.ejim.2020.02.023
79. Taube C, Bayer V, Zehendner CM, Valipour A. Assessment of patient experiences with Respimat((R)) in everyday clinical practice. Pulm Ther (2020) 6(2):371–80. doi: 10.1007/s41030-020-00127-4
80. Dalby RN, Eicher J, Zierenberg B. Development of Respimat((R)) soft mist inhaler and its clinical utility in respiratory disorders. Med Devices (Auckl) (2011) 4:145–55. doi: 10.2147/MDER.S7409
81. Wei Y, Hori A, Chen IY, Tamogi H, Hirokawa T, Kato K, et al. Maximum volume of nasal administration using a mucosal atomization device without aspiration in Japanese white rabbits. J Vet Med Sci (2022) 84(6):792–8. doi: 10.1292/jvms.21-0648
82. Rusconi F, Castagneto M, Gagliardi L, Leo G, Pellegatta A, Porta N, et al. Reference values for respiratory rate in the first 3 years of life. Pediatrics (1994) 94(3):350–5. doi: 10.1542/peds.94.3.350
83. LoMauro A, Aliverti A. Physiology masterclass: Extremes of age: newborn and infancy. Breathe (Sheff) (2016) 12(1):65–8. doi: 10.1183/20734735.013315
84. Wilkins JV Jr., Golshahi L, Rahman N, Li L. Evaluation of intranasal vaccine delivery using anatomical replicas of infant nasal airways. Pharm Res (2021) 38(1):141–53. doi: 10.1007/s11095-020-02976-9
85. Zhang A, Groves MJ. Size characterization of mycobacterium bovis BCG (Bacillus calmette guérin) vaccine, tice substrain. Pharm Res (1988) 5(9):607–10. doi: 10.1023/a:1015954316139
86. Labiris NR, Dolovich MB. Pulmonary drug delivery. part I: physiological factors affecting therapeutic effectiveness of aerosolized medications. Br J Clin Pharmacol (2003) 56(6):588–99. doi: 10.1046/j.1365-2125.2003.01892.x
87. Stylianou E, Paul MJ, Reljic R, McShane H. Mucosal delivery of tuberculosis vaccines: a review of current approaches and challenges. Expert Rev Vaccines (2019) 18(12):1271–84. doi: 10.1080/14760584.2019.1692657
88. Khanal A, Sharma R, Corcoran TE, Garoff S, Przybycien TM, Tilton RD. Surfactant driven post-deposition spreading of aerosols on complex aqueous subphases. 1: High deposition flux representative of aerosol delivery to Large airways. J Aerosol Med Pulm Drug Delivery (2015) 28(5):382–93. doi: 10.1089/jamp.2014.1168
89. Nemes E, Geldenhuys H, Rozot V, Rutkowski KT, Ratangee F, Bilek N, et al. Prevention of m. tuberculosis infection with H4:IC31 vaccine or BCG revaccination. N Engl J Med (2018) 379(2):138–49. doi: 10.1056/NEJMoa1714021
90. Rakshit S, Ahmed A, Adiga V, Sundararaj BK, Sahoo PN, Kenneth J, et al. BCG Revaccination boosts adaptive polyfunctional Th1/Th17 and innate effectors in IGRA+ and IGRA- Indian adults. JCI Insight (2019) 4(24):e130540. doi: 10.1172/jci.insight.130540
91. Bannister S, Sudbury E, Villanueva P, Perrett K, Curtis N. The safety of BCG revaccination: A systematic review. Vaccine (2021) 39(20):2736–45. doi: 10.1016/j.vaccine.2020.08.016
92. Therapeutics Goods Administration. BCG Vaccine AJV - mycobacterium bovis BCG (Bacillus calmette-guerin), Danish strain 1331 (AJ vaccines) with diluted sauton AJV (New Zealand). (2021). Available at: https://www.tga.gov.au/resources/section-19a-approvals/bcg-vaccine-ajv-mycobacterium-bovis-bcg-bacillus-calmette-guerin-danish-strain-1331-aj-vaccines-diluted-sauton-ajv-new-zealand.
93. Kanojia G, Have RT, Soema PC, Frijlink H, Amorij JP, Kersten G. Developments in the formulation and delivery of spray dried vaccines. Hum Vaccin Immunother (2017) 13(10):2364–78. doi: 10.1080/21645515.2017.1356952
94. Levy ML, Dekhuijzen PN, Barnes PJ, Broeders M, Corrigan CJ, Chawes BL, et al. Inhaler technique: facts and fantasies. a view from the aerosol drug management improvement team (ADMIT). NPJ Prim Care Respir Med (2016) 26:16017. doi: 10.1038/npjpcrm.2016.17
95. Sanchis J, Gich I, Pedersen S. Systematic review of errors in inhaler use: Has patient technique improved over time? Chest (2016) 150(2):394–406. doi: 10.1016/j.chest.2016.03.041
Keywords: tuberculosis, vaccine delivery, Respimat® Soft Mist™, mucosal atomization device (MAD) syringe, mucosal vaccine, mucosal
Citation: Puri M, Miranda-Hernandez S, Subbian S and Kupz A (2023) Repurposing mucosal delivery devices for live attenuated tuberculosis vaccines. Front. Immunol. 14:1159084. doi: 10.3389/fimmu.2023.1159084
Received: 05 February 2023; Accepted: 20 March 2023;
Published: 30 March 2023.
Edited by:
Juraj Ivanyi, King’s College London, United KingdomReviewed by:
Armando Acosta, Universiti Sains Malaysia Health Campus, MalaysiaCopyright © 2023 Puri, Miranda-Hernandez, Subbian and Kupz. This is an open-access article distributed under the terms of the Creative Commons Attribution License (CC BY). The use, distribution or reproduction in other forums is permitted, provided the original author(s) and the copyright owner(s) are credited and that the original publication in this journal is cited, in accordance with accepted academic practice. No use, distribution or reproduction is permitted which does not comply with these terms.
*Correspondence: Andreas Kupz, YW5kcmVhcy5rdXB6QGpjdS5lZHUuYXU=