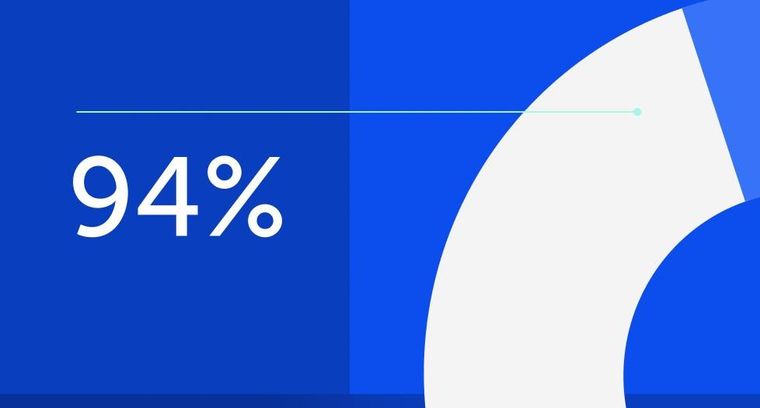
94% of researchers rate our articles as excellent or good
Learn more about the work of our research integrity team to safeguard the quality of each article we publish.
Find out more
REVIEW article
Front. Immunol., 04 April 2023
Sec. Inflammation
Volume 14 - 2023 | https://doi.org/10.3389/fimmu.2023.1158859
This article is part of the Research TopicAlterations in Innate Immune Metabolism During Lung RepairView all 7 articles
Granulocyte-macrophage colony-stimulating factor (GM-CSF) is a hematopoietic growth factor originally identified as a stimulus that induces the differentiation of bone marrow progenitor cells into granulocytes and macrophages. GM-CSF is now considered to be a multi-origin and pleiotropic cytokine. GM-CSF receptor signals activate JAK2 and induce nuclear signals through the JAK-STAT, MAPK, PI3K, and other pathways. In addition to promoting the metabolism of pulmonary surfactant and the maturation and differentiation of alveolar macrophages, GM-CSF plays a key role in interstitial lung disease, allergic lung disease, alcoholic lung disease, and pulmonary bacterial, fungal, and viral infections. This article reviews the latest knowledge on the relationship between GM-CSF and lung balance and lung disease, and indicates that there is much more to GM-CSF than its name suggests.
Granulocyte-macrophage colony-stimulating factor (GM-CSF, or CSF2), a member of the CSF family of hematopoietic growth factors, was originally identified as a stimulus that induces the differentiation of bone marrow precursor cells into granulocytes and macrophages (1). Studies now show that GM-CSF has a wide range of biological activities in innate and adaptive immunity, and it plays a key role in many autoimmune and inflammatory diseases (2, 3). Given that the most prominent phenotypic features of GM-CSF-deficient mice are surfactant alveolar accumulation and impaired alveolar macrophages (AMs) function (4), this review mainly focuses on the role of GM-CSF in lung balance and lung disease.
GM-CSF is a polypeptide growth factor with a molecular weight of 23 kDa, and its encoding gene is located at 5q22-31, with four exons and 2.5 kb in length. Human GM-CSF contains 127 amino acid residues and is present in serum and most tissues (5). GM-CSF-producing cells can be myeloid cells, such as monocytes/macrophages, eosinophils, neutrophils. They can also be non-myeloid cells, such as epithelial cells, endothelial cells, chondrocytes, fibroblasts, and even tumor cells (6–10). A variety of stimuli can induce GM-CSF production; in T cells, IL-1β, IL-12, IL-23, and prostaglandin E2 can stimulate the production of GM-CSF (11–14). The combination of IL-1 and TNF-α can induce GM-CSF production in a variety of cells, such as endothelial cells, smooth muscle cells, chondrocytes, and fibroblasts, T and B cells (15). In lymphocytes, GM-CSF production requires activation of the transcription factor NF-AT (16, 17), and the production of GM-CSF by adipocytes requires activation of NF-κB (18), indicating that a variety of transcription factors can mediate GM-CSF production. Certain cytokines such as IFN-γ, IL-4, and IL-10, and drugs such as cyclosporine A and glucocorticoids can inhibit GM-CSF production (19–23). The target cells of GM-CSF include a variety of myeloid cells, such as monocytes, macrophages, eosinophils, neutrophils, and dendritic cells (DCs) (3). GM-CSF has various effects on the biological activity of myeloid cells, such as cell activation and proliferation, increasing their chemotaxis and adhesion, promoting the production of pro-inflammatory factors, and improving cell phagocytosis and antigen presentation functions (24–29). Tissues of GM-CSF-deficient mice, especially the lungs, have increased susceptibility to pathogenic microorganisms (30–33). This indicates that GM-CSF plays an important role in maintaining immune function.
GM-CSF receptors consist of heterodimers, including α chains that specifically bind GM-CSF and β chains responsible for signal transduction; β chains are common chains of IL-3 and IL-5 receptors. Neither the α nor β chains of the GM-CSF receptor contain a tyrosine kinase catalytic domain, although the β chain is linked to the tyrosine kinase JAK2 (34, 35). After the GM-CSF receptor α chain binds to GM-CSF, it then binds to the β chain to form a multimer. The polymerization of the receptor leads to the activation of JAK2, and activated JAK2 phosphorylates the tyrosine residues on the β chain. Phosphorylated tyrosine recruits STAT-5 containing the SH2 domain, and JAK2 activates STAT-5, thereby activating the JAK-STAT pathway. In addition, JAK2 can cause the activation of PI3K, initiating the PI3K-Akt pathway. Phosphorylated tyrosine on the β chain of the GM-CSF receptor recruits the adaptor protein SHC to activate RAS and initiate the MAPK signaling pathway to induce nuclear signaling (Figure 1). The JAK2-STAT-5 pathway mainly controls cell differentiation and inflammatory signaling, whereas PI3K signaling promotes cell proliferation and survival, and the MAPK pathway is involved in cell growth, proliferation, and differentiation (6, 36, 37).
The GM-CSF receptor consists of a dimer of α and β common chains. Binding of GM-CSF to the α chain results in polymerization of the β subunit of the receptor; this causes the transactivation of JAK2, which phosphorylates multiple tyrosine sites on the β chain. Phosphorylated tyrosine recruits STAT5, which is then phosphorylated by JAK2. The two phosphorylated STAT5 molecules form a homologous dimer that translocates into the nucleus and initiates target gene transcription. In addition, activation of PI3K can be accomplished by JAK2-mediated phosphorylation of the regulatory subunit p85, thereby activating the PI3K-Akt pathway. Activation of the MAPK pathway is triggered by the recruitment of SHC by a phosphorylated tyrosine on the β subunit, which catalyzes RAS activation, leading to continuous activation of RAF, MEK, and ERK. JAK2-STAT5 signaling controls differentiation and inflammation, PI3K promotes cell proliferation and survival, and MAPK is involved in cell growth, proliferation and differentiation.
AMs are lung-specific tissue-resident macrophages that play an important role in maintaining alveolar homeostasis by engulfing inhaled bacteria and removing excess surfactant and cellular debris (38, 39). AMs mainly originate from fetal liver (FL) monocytes, but yolk sac (YS) monocytes and circulating monocytes can also be used as AMs precursors, indicating that there is a compensatory mechanism for the development of AMs (40). When FL monocytes differentiate into AMs in response to inflammation, the body can compensate by inducing YS monocytes or circulating monocytes to differentiate into AMs. Studies analyzing GM-CSF-deficient and GM-CSF receptor β chain-deficient mice in the 90s demonstrated a functional link between GM-CSF and AMs (4, 41). Lung histology of GM-CSF-deficient mice show amorphous eosinophilic accumulation and foamy AMs, similar to the pathological features of human pulmonary alveolar proteinosis (PAP). However, exogenous GM-CSF infusion can restore the AMs population in GM-CSF-deficient mice, suggesting that GM-CSFs play a key role in AMs development (42). GM-CSF is involved in the development of AMs from the embryonic stage, and the level of GM-CSF in the lungs is higher after the 17.5th day of the embryo. Increased levels of GM-CSF in the lungs are temporally consistent with the differentiation of AMs precursors into AMs (40). GM-CSF induces the expression and functional activity of PPAR-γ in fetal monocytes, and PPAR-γ regulates the developmental process of AMs as a master transcription factor. The amount of AMs in PPAR-γ deficient mice is relatively low and they show abnormalities that are similar to the abnormalities of AMs in GM-CSF-deficient mice and GM-CSF receptor β chain-deficient mice. This suggests that the GM-CSF signaling-induced PPAR-γ plays an important role in the development of AMs (43). Shibata et al. showed that the transcription factor PU.1 not only mediated the dependence of the final differentiation of AMs on GM-CSF, but also regulated innate immune functions such as pathogen killing of AMs and the catabolism of surfactants (44).
Normally, the sources of lung GM-CSF include both immune and non-immune cells, such as type 2 innate lymphocytes (ILC2), basophils, and epithelial cells (9, 45, 46). Among neonatal immune cells, ILC2 produces the highest level of GM-CSF, followed by γδT cells (45). The depletion of lymphocytes or basophils has no effect on the amount of AMs in neonatal and adult lungs, suggesting that GM-CSF derived from immune cells is dispensable for the development and maintenance of AMs. When GM-CSF in alveolar type 2 epithelial cells (AT2s) is depleted, AMs in neonatal and adult mice are almost completely depleted, indicating an integral role of AT2-derived GM-CSF in AMs development (47).
Pulmonary surfactant is composed of approximately 90% lipids and 10% proteins. Approximately 80–90% of the lipids are phospholipids, and surfactant-related proteins include SP-A, SP-B, SP-C, and SP-D. These proteins are involved in the intracellular transport of phospholipid components and contribute to the maintenance of surfactant properties in the alveoli (48, 49). Surfactant phospholipids and proteins are synthesized and secreted by AT2s (50, 51). Surfactants form single and multiple layers at the gas-liquid interface to reduce surface tension and prevent alveolar collapse. Surfactants become inactive small aggregate particles through mechanical or biological action, and are absorbed, reused, or decomposed by AT2s and AMs (52).
Studies on the relationship between GM-CSF and surfactant balance have demonstrated the significant accumulation of phospholipids and proteins in the lung of GM-CSF- deficient mice (53–55). Lung histology show a large number of inclusion bodies and enlarged AMs with amorphous eosinophilic substances. These characteristics are similar to the typical manifestations of human PAP (56, 57). GM-CSF is required for cholesterol clearance and that reduced cholesterol clearance is a primary macrophage defect in PAP pathogenesis (58). The expression of GM-CSF in the lungs completely corrects the alveolar protein deposition caused by endogenous GM-CSF gene-targeted ablation, and likewise, inhaled rather than systemic administration of GM-CSF corrects PAP in GM-CSF-deficient mice (57, 59–62). These findings suggest that the presence of GM-CSF locally in the lungs is necessary and sufficient to restore homeostasis of pulmonary surfactant.
Metabolic studies in GM-CSF-deficient mice have shown that the accumulation of surfactant in the alveoli of GM-CSF-deficient mice is due to the fact that the lack of GM-CSF signaling impairs the catabolism of the surfactant, whereas it does not directly alter its phospholipid and protein synthesis or secretion (5). In vitro studies of AMs isolated from GM-CSF-deficient mice identified significant defects in the catabolism of SP-A and surfactant phospholipids, as well as a marked reduction in the degradation of SP-A and DPPC by AMs (63). In addition, mice with increased GM-CSF expression in the lungs show an increased surfactant catabolic rate in AMs, whereas the uptake of surfactant components by GM-CSF-deficient mouse AMs is not hindered (5). These results suggest that in the absence of GM-CSF signaling, the main defect in surfactant homeostasis is caused by insufficient catabolism of surfactant proteins and lipids by AMs (5), which may be related to the need for GM-CSF signaling from AT2 for the development and differentiation of AMs. This also shows that there is a mutually beneficial symbiotic relationship between AT2 and AMs. AT2-derived GM-CSF guides the development of AMs by promoting PU.1 and PPAR-γ expression; in turn, mature AMs are essential for breaking down the surfactants produced by AT2 and maintaining the balance of the alveolar environment (Figure 2).
Surfactant proteins and phospholipids are synthesized in AT2s, transported through the endoplasmic reticulum to the Golgi apparatus for processing, and then transported to the lamellar body. The lamellar bodies are secreted into the alveolar fluid, where they form tubular myelin. Tubular myelin forms a surface-active phospholipid film at the air-liquid interface that spreads along the surface of the alveoli. Small aggregates of surfactants that have been inactivated by mechanical and biological processes are partially absorbed and reused by alveolar AT2s, and the other part is taken up and broken down by AMs. GM-CSF, which mainly originates from AT2s in the alveoli, promotes the differentiation and function of AMs by binding to the AMs surface GM-CSF receptor and initiates intracellular signals to stimulate the expression and activation of the transcription factors PU.1 and PPAR-r, thereby enhancing the catabolism of small aggregates.
Interstitial lung disease (ILD) includes a group of heterogeneous lung diseases characterized by pulmonary parenchymal inflammation and fibrosis. GM-CSF is involved in the progression of pulmonary fibrosis. GM-CSF production in bronchoalveolar lavage fluid is increased in patients with pulmonary fibrosis (64). GM-CSF stimulates macrophages to produce profibrotic cytokines and can directly induce airway smooth muscle cell fibrosis (3, 65). Autoimmune or inflammatory mechanisms play an important central role in the pathogenesis of connective tissue disease-associated with ILD (CTD-ILD) (66, 67).
SKG mice, which are a model of autoimmune arthritis, treated with yeast polysaccharides develop chronic progressive ILD. These mice exhibit massive lung infiltration of Th17 cells, GM-CSF-producing CD4+ T cells, and CD11b+Gr1+ neutrophils, accompanied by pulmonary fibrosis. Naive SKG mouse T cells differentiate into GM-CSF-producing cells; these enhance macrophage production of IL-6 and IL-1β, thereby promoting the differentiation of IL-17A and/or GM-CSF-producing T cells and the infiltration of neutrophils into the lungs. Neutralization of GM-CSF blocks the development of ILD, whereas neutralization of IL-17A does not, suggesting that GM-CSF, rather than IL-17A, is critical for the development of ILD in SKG mice (68). Kwon et al. also showed that GM-CSF played an important role in ILD development, but these authors believed that IL-17A+GM-CSF+ neutrophils were the main inflammatory cells infiltrated in the lungs of curdlan-treated SKG mice (69).
GM-CSF mediates allergen-induced Th2 sensitization and airway eosinophil inflammatory responses (70). The use of GM-CSF receptor α (Csf2ra)-deficient mice illustrates that GM-CSF signaling, although not necessary for the development of eosinophils in normal mice, promotes eosinophil accumulation in the lungs and aggravates airway inflammation in the allergic asthma model; this may be associated with GM-CSF-induced chemotaxis and could promote eosinophil survival (71). In a mouse model of asthma, allergen-stimulated airway epithelial cells release GM-CSF, which activates DCs and prolongs eosinophil survival (72). Intranasal administration of GM-CSF-neutralizing antibodies during allergen inhalation significantly reduces airway hyperreactivity and inhibits airway inflammation (73). In a chronic allergic airway inflammation model, although the GM-CSF signal could not regulate neutrophil migration, GM-CSF promoted antigen uptake by lung DCs, processed and transported to draining lymph nodes, thus enhancing the Th2/Th17 immune response, which in turn increased the recruitment of granulocytes to the lung and aggravated lung inflammation (74).
AMs in alcoholics are defective in cell adhesion, cytokine production, and phagocytosis (75, 76). Alcohol may affect lung immune function by affecting GM-CSF signaling (77, 78). Although the GM-CSF concentration in the blood and lungs of alcoholics is not markedly different from that in non-alcoholics, chronic alcohol exposure significantly decreases the levels of the GM-CSF receptor for AMs (77), and this decrease is mediated by a decrease in the activity of the transcription factor PU.1 (44, 78). GM-CSF increases PU.1 activity in alcohol-exposed AMs and restores agonist-induced cytokine production and phagocytosis in AMs (78).
The mechanism underlying the decreased activity of PU.1 in alcoholic lungs may be associated with a decrease in antioxidant defense mechanisms (79). Chronic alcohol exposure decreases the lung levels of Nrf2, a major transcription factor that regulates the expression of many antioxidant genes in cells. Nrf2 regulates the expression of PU.1 in the lungs, suggesting that the decrease in antioxidant response function may be related to the decrease in GM-CSF activity in alcoholic lungs and lung immunodeficiency (76).
Chronic alcohol exposure can also lead to reduced levels of alveolar epithelial GM-CSF receptors, suggesting that alcohol can extensively disrupt GM-CSF signaling in the lungs (78), and that the absence of GM-CSF signaling disrupts the epithelial barrier function in the distal lung epithelium. This could explain why alcoholics are more likely to develop acute respiratory distress syndrome (ARDS) based on increased alveolar wall permeability (76, 80, 81). Therefore, the lack of GM-CSF signaling plays a key role in pulmonary dysfunction in patients with alcoholism, and GM-CSF replacement therapy has been used to improve lung complications in patients with alcoholism (82).
In bacterial, fungal, and viral infections of the lungs, first-line defense cells such as AMs and airway epithelial cells (AECs) initiate an immune response to the microbial challenge (83). Because prolonged or excessive immune responses can damage the respiratory tract, it is important to modulate the inflammatory response to maintain an appropriate airway immune response (84, 85).
Streptococcus pneumoniae is the most common pathogen causing pneumonia and fatal pneumonia (86). Recombinant GM-CSF delivered through the airway improved the defense response against Group B streptococcus in GM-CSF-deficient mice, and wild-type mice cleared Group B streptococcus faster when receiving aerosol GM-CSF (32). Pulmonary delivery of GM-CSF 2 to 4 weeks prior to infection significantly reduced mortality in S. pneumoniae infected mice, and this increased survival was accompanied by an increase in the expression of inducible nitric oxide synthase and antibacterial activity in lung sentinel cells, as well as a significant decrease in caspase-3-dependent apoptosis and secondary necrosis. This suggests that prophylactic delivery of GM-CSF into the lungs triggers a lasting immunostimulatory response that improves alveolar immunity against pneumococcus (87). In mice intranasally infected with influenza A virus (IAV), followed by treatment with atomized recombinant GM-CSF and re-infection with S. pneumoniae, inhalation of GM-CSF had significant survival benefits against the secondary attack of S. pneumoniae and significantly reduced the incidence of S. pneumoniae bacteremia (88).
Patients with acquired GM-CSF deficiency are susceptible to Cryptococcus and other opportunistic fungi (89–91). In GM-CSF-deficient mice, control of cryptococcal lung infection is impaired. Deficiency of GM-CSF decreases the following processes: 1) total lung leukocyte recruitment; 2) Th2 and Th17 responses; 3) the number of CD11b (+) DCs and macrophages; and 4) the activation and localization of DCs and macrophages in alveoli. These results indicate that GM-CSF promotes the local activation, differentiation, accumulation, and alveolar localization of DCs and macrophages in the lungs of cryptococcus lung-infected mice, suggesting that GM-CSF is a core factor in the protective immune response against mycosis (91).
Influenza virus (IV) infection is a common cause of acute respiratory failure in the pediatric intensive care unit (92). The primary target cells for human IV invasion are AECs (93). GM-CSF secreted by AECs plays an important role in preventing influenza-induced pneumonia (94). The ability of GM-CSF-deficient mouse AMs to clear pathogenic microorganisms is damaged, which reduces resistance to influenza virus infection. However, alveolar GM-CSF increases the proliferation and resistance of mouse AMs, thus protecting mice from the deadly IV infection (85). Pulmonary CD103+DC is a key factor mediating GM-CSF-dependent lung protection after IV infection (95, 96). After IV infection, lung CD103+DC is activated and expanded, and GM-CSF mediates its migration and antigen presentation in draining mediastinal lymph nodes. This is associated with better viral clearance and Ag-specific T-cell recruitment. Therefore, GM-CSF-dependent crosstalk between IV-infected AECs and CD103+DCs is important for effective IV clearance (96).
Coronavirus disease 2019 (COVID-2), which is caused by severe acute respiratory syndrome coronavirus type 2 (SARS-CoV-2), has evolved into a global pandemic and treatments are urgently needed (97–100). GM-CSF, an important hematopoietic growth factor and pro-inflammatory cytokine, has attracted attention as a therapeutic target in COVID-19 (99). The circulating levels of GM-CSF are increased in patients with COVID-19 compared with healthy controls (101, 102). Patients with COVID-19 have an increased percentage of white blood cells expressing GM-CSF (103). As an important immunomodulatory cytokine, GM-CSF helps clear respiratory microorganisms by activating AMs, suggesting that it could help clear SARS-CoV-2 early in the course of COVID-19 (104, 105). However, GM-CSF is harmful as part of the cytokine storm in the late stage of severe lung injury caused by COVID-19. Therefore, blocking GM-CSF or the GM-CSF receptor may be an effective treatment strategy to block the progression of acute respiratory failure in patients with COVID-19 by reducing the cytokine storm and the infiltration of inflammatory myeloid cells (102).
GM-CSF is a cytokine of multiple cellular origins and pleiotropy, and in the lungs, it is mainly derived from AT2s (47). TNF-α and IL-1β can also induce the production of GM-CSF by endothelial cells and fibroblasts in the lung. The GM-CSF produced by these cells in response to stimulation helps guide leukocyte infiltration into the tissues (106–109). Quiescent alveolar epithelial cells produce very little GM-CSF, whereas AT2s are the main source of GM-CSF in the alveoli in response to stimulation by factors such as infection or inflammation (47). However, the molecular mechanism of GM-CSF production remains unclear. GM-CSF can affect the plasticity of AT2s in an autocrine manner and influence the differentiation of AT2s to alveolar type 1 epithelial cells (AT1s) by regulating cell cycle genes (110–112). GM-CSF also decreases the susceptibility of AT2s to oxidative stress damage, protecting AT2s from the effects of hyperoxia (112).
GM-CSF induces the transcription factors PU.1 and PPAR-γ to drive AM differentiation and maintenance (43, 44). Moreover, GM-CSF can drive the innate immune function of AMs such as pathogen clearance (44). GM-CSF-deficient mice are prone to respiratory infections, and restoring GM-CSF expression reverses this susceptibility, suggesting that GM-CSF-driven AMs play an important role in the innate immunity of the lungs.
Pulmonary surfactant is synthesized and secreted by AT2s. GM-CSF deficiency has little effect on the production or secretion of surfactant lipids or proteins from AT2s, although it has a significant inhibitory effect on the ability of AMs to clear surfactant substances (proteins and lipids) (104, 113–115). The decrease in surfactant clearance of AMs is largely due to disruption of signaling events mediated by the transcription factor PU.1 or PPAR-γ (116, 117). Therefore, GM-CSF signaling drives the differentiation and development of AMs through the PU.1 and PPAR-γ transcription factors and mediates the catabolism of surfactant proteins and lipids. It should be noted that AT2s can also be involved in the catabolism of surfactants. Therefore, future studies will confirm whether GM-CSF affects the catabolism of surfactant in AT2s.
In addition to maintaining surfactant balance and promoting AM differentiation and metabolism, GM-CSF signaling plays an important role in lung infection or inflammation. GM-CSF protects against pneumococcal pneumonia in the lungs by upregulating iNOS expression through PU.1 and STAT5 signals, which contribute to the antibacterial activity of AMs against pneumococcal pneumonia (87). In GM-CSF-deficient mice, control of fungal lung infections is impaired (118). GM-CSF is involved in host defense against fungal lung infections by promoting the differentiation, accumulation, activation and alveolar localization of lung DCs and macrophages (91). Lung CD103+DC is a key factor mediating GM-CSF-dependent lung protection after IV infection. IV induces the production of GM-CSF in alveolar epithelial cells, which leads to the activation and migration of CD103+DCs to drainage mediastinal lymph node (MLN). This increases the accumulation of IFN-γ+CD4+ and IFN-γ+CD8+T cells to the alveoli, accelerates IV clearance, and mediates recovery from epithelial damage (96). In the early stages of SARS-CoV-2 infection, the role of GM-CSF may be protected as it helps limit virus-related damage. Therefore, the inhaled formulation of human recombinant GM-CSF, sargramostim, is being tested in patients with acute hypoxic respiratory failure associated with COVID-19. In the later stages of SARS-CoV-2 infection, the severity of the disease appears to be driven by improper release of cytokines such as GM-CSF. These inflammatory mediators are involved in inflammatory lung injury, making patients prone to respiratory failure and eventually leading to ARDS. Therefore, inhibition of GM-CSF signaling may be a reasonable treatment in the late stages of COVID-19 (102).
GM-CSF was first known as a hematopoietic growth factor that is used to boost bone marrow production; however, as described in this review, its function goes far beyond what was identified in earlier studies. In addition to promoting the metabolism of pulmonary surfactant and the maturation and differentiation of AMs, GM-CSF plays a key role in lung bacterial, fungal, and viral infections, interstitial lung disease, allergic lung disease, alcoholic lung, and other disease states. Therefore, GM-CSF would be an attractive target for future research on lung balance and lung disease.
YC and FL: writing original draft, searching literature. MH: searching literature, reviewing and editing. ML and CS: supervision, writing, reviewing, and editing. All authors discussed the article and approved the submitted version.
This work was supported by Natural Science Research Project of Anhui Educational Committee (No. KJ2020ZD49), 512 Talent Cultivation Program of Bengbu Medical College (No. by51201103).
The authors declare that the research was conducted in the absence of any commercial or financial relationships that could be construed as a potential conflict of interest.
All claims expressed in this article are solely those of the authors and do not necessarily represent those of their affiliated organizations, or those of the publisher, the editors and the reviewers. Any product that may be evaluated in this article, or claim that may be made by its manufacturer, is not guaranteed or endorsed by the publisher.
1. Burgess AW, Metcalf D. The nature and action of granulocyte-macrophage colony stimulating factors. Blood (1980) 56(6):947–58. doi: 10.1182/blood.V56.6.947.947
2. Codarri L, Gyulveszi G, Tosevski V, Hesske L, Fontana A, Magnenat L, et al. RORγt drives production of the cytokine GM-CSF in helper T cells, which is essential for the effector phase of autoimmune neuroinflammation. Nat Immunol (2011) 12(6):560–7. doi: 10.1038/ni.2027
3. Shiomi A, Usui T. Pivotal roles of GM-CSF in autoimmunity and inflammation. . Mediators Inflammation (2015) 2015:568543. doi: 10.1155/2015/568543
4. Stanley E, Lieschke GJ, Grail D, Metcalf D, Hodgson G, Gall JA, et al. Granulocyte/macrophage colony-stimulating factor-deficient mice show no major perturbation of hematopoiesis but develop a characteristic pulmonary pathology. Proc Natl Acad Sci U.S.A. (1994) 91(12):5592–6. doi: 10.1073/pnas.91.12.5592
5. Trapnell BC, Whitsett JA. Gm-CSF regulates pulmonary surfactant homeostasis and alveolar macrophage-mediated innate host defense. Annu Rev Physiol (2002) 64:775–802. doi: 10.1146/annurev.physiol.64.090601.113847
6. Becher B, Tugues S, Greter M. GM-CSF: From growth factor to central mediator of tissue inflammation. Immunity (2016) 45(5):963–73. doi: 10.1016/j.immuni.2016.10.026
7. Pearson C, Thornton EE, Mckenzie B, Schaupp AL, Huskens N, Griseri T, et al. ILC3 GM-CSF production and mobilisation orchestrate acute intestinal inflammation. Elife (2016) 5:e10066. doi: 10.7554/eLife.10066
8. Willart MA, Deswarte K, Pouliot P, Braun H, Beyaert R, Lambrecht BN, et al. Interleukin-1α controls allergic sensitization to inhaled house dust mite via the epithelial release of GM-CSF and IL-33. J Exp Med (2012) 209(8):1505–17. doi: 10.1084/jem.20112691
9. Sheih A, Parks WC, Ziegler SF. GM-CSF produced by the airway epithelium is required for sensitization to cockroach allergen. Mucosal Immunol (2017) 10(3):705–15. doi: 10.1038/mi.2016.90
10. Khanna S, Graef S, Mussai F, Thomas A, Wali N, Yenidunya BG, et al. Tumor-derived GM-CSF promotes granulocyte immunosuppression in mesothelioma patients. Clin Cancer Res (2018) 24(12):2859–72. doi: 10.1158/1078-0432.CCR-17-3757
11. El-Behi M, Ciric B, Dai H, Yan Y, Cullimore M, Safavi F, et al. The encephalitogenicity of T(H)17 cells is dependent on IL-1- and IL-23-induced production of the cytokine GM-CSF. Nat Immunol (2011) 12(6):568–75. doi: 10.1038/ni.2031
12. Lukens JR, Barr MJ, Chaplin DD, Chi H, Kanneganti TD. Inflammasome-derived IL-1β regulates the production of GM-CSF by CD4(+) T cells and γδ T cells. J Immunol (2012) 188(7):3107–15. doi: 10.4049/jimmunol.1103308
13. Duhen T, Campbell DJ. IL-1β promotes the differentiation of polyfunctional human CCR6+CXCR3+ Th1/17 cells that are specific for pathogenic and commensal microbes. J Immunol (2014) 193(1):120–9. doi: 10.4049/jimmunol.1302734
14. Quill H, Gaur A, Phipps RP. Prostaglandin E2-dependent induction of granulocyte-macrophage colony-stimulating factor secretion by cloned murine helper T cells. J Immunol (1989) 142(3):813–8. doi: 10.4049/jimmunol.142.3.813
15. Hamilton JA. GM-CSF in inflammation and autoimmunity. Trends Immunol (2002) 23(8):403–8. doi: 10.1016/s1471-4906(02)02260-3
16. Johnson BV, Bert AG, Ryan GR, Condina A, Cockerill PN. Granulocyte-macrophage colony-stimulating factor enhancer activation requires cooperation between NFAT and AP-1 elements and is associated with extensive nucleosome reorganization. Mol Cell Biol (2004) 24(18):7914–30. doi: 10.1128/MCB.24.18.7914-7930.2004
17. Shang C, Attema J, Cakouros D, Cockerill PN, Shannon MF. Nuclear factor of activated T cells contributes to the function of the CD28 response region of the granulocyte macrophage-colony stimulating factor promoter. Int Immunol (1999) 11(12):1945–56. doi: 10.1093/intimm/11.12.1945
18. Ying R, Li SW, Chen JY, Zhang HF, Yang Y, Gu ZJ, et al. Endoplasmic reticulum stress in perivascular adipose tissue promotes destabilization of atherosclerotic plaque by regulating GM-CSF paracrine. J Transl Med (2018) 16(1):105. doi: 10.1186/s12967-018-1481-z
19. Ozawa H, Aiba S, Nakagawa, Tagami H. Interferon-gamma and interleukin-10 inhibit antigen presentation by langerhans cells for T helper type 1 cells by suppressing their CD80 (B7-1) expression. Eur J Immunol (1996) 26(3):648–52. doi: 10.1002/eji.1830260321
20. Jansen JH, Wientjens GJ, Fibbe WE, Willemze R, Kluin-Nelemans HC. Inhibition of human macrophage colony formation by interleukin 4. J Exp Med (1989) 170(2):577–82. doi: 10.1084/jem.170.2.577
21. Sagawa K, Mochizuki M, Sugita S, Nagai K, Sudo T, Itoh K. Suppression by IL-10 and IL-4 of cytokine production induced by two-way autologous mixed lymphocyte reaction. Cytokine (1996) 8(6):501–6. doi: 10.1006/cyto.1996.0068
22. Hatfield SM, Roehm NW. Cyclosporine and FK506 inhibition of murine mast cell cytokine production. . J Pharmacol Exp Ther (1992) 260(2):680–8.
23. Adcock IM, Caramori G. Cross-talk between pro-inflammatory transcription factors and glucocorticoids. . Immunol Cell Biol (2001) 79(4):376–84. doi: 10.1046/j.1440-1711.2001.01025.x
24. Curran CS, Evans MD, Bertics PJ. GM-CSF production by glioblastoma cells has a functional role in eosinophil survival, activation, and growth factor production for enhanced tumor cell proliferation. J Immunol (2011) 187(3):1254–63. doi: 10.4049/jimmunol.1001965
25. Sakagami T, Uchida K, Suzuki T, Carey BC, Wood RE, Wert SE, et al. Human GM-CSF autoantibodies and reproduction of pulmonary alveolar proteinosis. N Engl J Med (2009) 361(27):2679–81. doi: 10.1056/NEJMc0904077
26. Gomez-Cambronero J, Horn J, Paul CC, Baumann MA. Granulocyte-macrophage colony-stimulating factor is a chemoattractant cytokine for human neutrophils: involvement of the ribosomal p70 S6 kinase signaling pathway. J Immunol (2003) 171(12):6846–55. doi: 10.4049/jimmunol.171.12.6846
27. Fleetwood AJ, Lawrence T, Hamilton JA, Cook AD. Granulocyte-macrophage colony-stimulating factor (CSF) and macrophage CSF-dependent macrophage phenotypes display differences in cytokine profiles and transcription factor activities: implications for CSF blockade in inflammation. J Immunol (2007) 178(8):5245–52. doi: 10.4049/jimmunol.178.8.5245
28. Berclaz PY, Shibata Y, Whitsett JA, Trapnell BC. GM-CSF, via PU.1, regulates alveolar macrophage fcgamma r-mediated phagocytosis and the IL-18/IFN-gamma -mediated molecular connection between innate and adaptive immunity in the lung. Blood (2002) 100(12):4193–200. doi: 10.1182/blood-2002-04-1102
29. Morrissey PJ, Bressler L, Park LS, Alpert A, Gillis S. Granulocyte-macrophage colony-stimulating factor augments the primary antibody response by enhancing the function of antigen-presenting cells. J Immunol (1987) 139(4):1113–9. doi: 10.4049/jimmunol.139.4.1113
30. Chroneos ZC, Midde K, Sever-Chroneos Z, Jagannath C. Pulmonary surfactant and tuberculosis. Tuberculosis (Edinb) (2009) 89(Suppl 1):S10–4. doi: 10.1016/S1472-9792(09)70005-8
31. Gonzalez-Juarrero M, Hattle JM, Izzo A, Junqueira-Kipnis AP, Shim TS, Trapnell BC, et al. Disruption of granulocyte macrophage-colony stimulating factor production in the lungs severely affects the ability of mice to control mycobacterium tuberculosis infection. J Leukoc Biol (2005) 77(6):914–22. doi: 10.1189/jlb.1204723
32. Levine AM, Reed JA, Kurak KE, Cianciolo E, Whitsett JA. GM-CSF-deficient mice are susceptible to pulmonary group b streptococcal infection. J Clin Invest (1999) 103(4):563–9. doi: 10.1172/JCI5212
33. Riopel J, Tam M, Mohan K, Marino MW, Stevenson MM. Granulocyte-macrophage colony-stimulating factor-deficient mice have impaired resistance to blood-stage malaria. Infect Immun (2001) 69(1):129–36. doi: 10.1128/IAI.69.1.129-136.2001
34. Achuthan AA, Lee KMC, Hamilton JA. Targeting GM-CSF in inflammatory and autoimmune disorders. Semin Immunol (2021) 54:101523. doi: 10.1016/j.smim.2021.101523
35. Kumar A, Taghi Khani A, Sanchez Ortiz A, Swaminathan S. GM-CSF: A double-edged sword in cancer immunotherapy. Front Immunol (2022) 13:901277. doi: 10.3389/fimmu.2022.901277
36. Achuthan A, Aslam ASM, Nguyen Q, Lam PY, Fleetwood AJ, Frye AT, et al. Glucocorticoids promote apoptosis of proinflammatory monocytes by inhibiting ERK activity. Cell Death Dis (2018) 9(3):267. doi: 10.1038/s41419-018-0332-4
37. Rodriguez RM, Suarez-Alvarez B, Lavín JL, Ascensión AM, Gonzalez M, Lozano JJ, et al. Signal integration and transcriptional regulation of the inflammatory response mediated by the GM-/M-CSF signaling axis in human monocytes. Cell Rep (2019) 29(4):860–872 e5. doi: 10.1016/j.celrep.2019.09.035
38. Hussell T, Bell TJ. Alveolar macrophages: plasticity in a tissue-specific context. Nat Rev Immunol (2014) 14(2):81–93. doi: 10.1038/nri3600
39. Kopf M, Schneider C, Nobs SP. The development and function of lung-resident macrophages and dendritic cells. . Nat Immunol (2015) 16(1):36–44. doi: 10.1038/ni.3052
40. Woo YD, Jeong D, Chung DH. Development and functions of alveolar macrophages. Mol Cells (2021) 44(5):292–300. doi: 10.14348/molcells.2021.0058
41. Nishinakamura R, Wiler R, Dirksen U, Morikawa Y, Arai K, Miyajima A, et al. The pulmonary alveolar proteinosis in granulocyte macrophage colony-stimulating factor/interleukins 3/5 beta c receptor-deficient mice is reversed by bone marrow transplantation. J Exp Med (1996) 183(6):2657–62. doi: 10.1084/jem.183.6.2657
42. Guilliams M, De Kleer I, Henri S, Post S, Vanhoutte L, De Prijck S, et al. Alveolar macrophages develop from fetal monocytes that differentiate into long-lived cells in the first week of life via GM-CSF. J Exp Med (2013) 210(10):1977–92. doi: 10.1084/jem.20131199
43. Schneider C, Nobs SP, Kurrer M, Rehrauer H, Thiele C, Kopf M. Induction of the nuclear receptor PPAR-γ by the cytokine GM-CSF is critical for the differentiation of fetal monocytes into alveolar macrophages. Nat Immunol (2014) 15(11):1026–37. doi: 10.1038/ni.3005
44. Shibata Y, Berclaz PY, Chroneos ZC, Yoshida M, Whitsett JA, Trapnell BC. GM-CSF regulates alveolar macrophage differentiation and innate immunity in the lung through PU.1. Immunity (2001) 15(4):557–67. doi: 10.1016/s1074-7613(01)00218-7
45. Clements D, Idoyaga J. Alveolar macrophages and epithelial cells: The art of living together. J Exp Med (2021) 218(10). doi: 10.1084/jem.20211583
46. Cohen M, Giladi A, Gorki AD, Solodkin DG, Zada M, Hladik A, et al. Lung single-cell signaling interaction map reveals basophil role in macrophage imprinting. Cell (2018) 175(4):1031–1044 e18. doi: 10.1016/j.cell.2018.09.009
47. Gschwend J, Sherman SPM, Ridder F, Feng X, Liang HE, Locksley RM, et al. Alveolar macrophages rely on GM-CSF from alveolar epithelial type 2 cells before and after birth. J Exp Med (2021) 218(10). doi: 10.1084/jem.20210745
48. Tlatelpa-Romero B, Cázares-Ordoñez V, Oyarzábal LF, Vázquez-De-Lara LG. The role of pulmonary surfactant phospholipids in fibrotic lung diseases. Int J Mol Sci (2022) 24(1). doi: 10.3390/ijms24010326
49. Krygier A, Szmajda-Krygier D Ś, Wiechowski R, Pietrzak J, Wosiak A, Wodziński D, et al. Molecular pathogenesis of fibrosis, thrombosis and surfactant dysfunction in the lungs of severe COVID-19 patients. Biomolecules (2022) 12(12). doi: 10.3390/biom12121845
50. Calkovska A, Kolomaznik M, Calkovsky V. Alveolar type II cells and pulmonary surfactant in COVID-19 era. Physiol Res (2021) 70(S2):S195–208. doi: 10.33549/physiolres.934763
51. Andreeva AV, Kutuzov MA, Voyno-Yasenetskaya TA. Regulation of surfactant secretion in alveolar type II cells. Am J Physiol Lung Cell Mol Physiol (2007) 293(2):L259–71. doi: 10.1152/ajplung.00112.2007
52. Ikegami M. Surfactant catabolism. Respirology (2006) 11(Suppl):S24–7. doi: 10.1111/j.1440-1843.2006.00803.x
53. Dranoff G, Crawford AD, Sadelain M, Ream B, Rashid A, Bronson RT, et al. Involvement of granulocyte-macrophage colony-stimulating factor in pulmonary homeostasis. Science (1994) 264(5159):713–6. doi: 10.1126/science.8171324
54. Nishinakamura R, Nakayama N, Hirabayashi Y, Inoue T, Aud D, Mcneil T, et al. Mice deficient for the IL-3/GM-CSF/IL-5 beta c receptor exhibit lung pathology and impaired immune response, while beta IL3 receptor-deficient mice are normal. Immunity (1995) 2(3):211–22. doi: 10.1016/1074-7613(95)90046-2
55. Ikegami M, Ueda T, Hull W, Whitsett JA, Mulligan RC, Dranoff G, et al. Surfactant metabolism in transgenic mice after granulocyte macrophage-colony stimulating factor ablation. Am J Physiol (1996) 270(4 Pt 1):L650–8. doi: 10.1152/ajplung.1996.270.4.L650
56. Jouneau S, Menard C, Lederlin M. Pulmonary alveolar proteinosis. . Respirol (2020) 25(8):816–26. doi: 10.1111/resp.13831
57. Reed JA, Ikegami M, Cianciolo ER, Lu W, Cho PS, Hull W, et al. Aerosolized GM-CSF ameliorates pulmonary alveolar proteinosis in GM-CSF-deficient mice. Am J Physiol (1999) 276(4):L556–63. doi: 10.1152/ajplung.1999.276.4.L556
58. Sallese A, Suzuki T, Mccarthy C, Bridges J, Filuta A, Arumugam P, et al. Targeting cholesterol homeostasis in lung diseases. Sci Rep (2017) 7(1):10211. doi: 10.1038/s41598-017-10879-w
59. Huffman JA, Hull WM, Dranoff G, Mulligan RC, Whitsett JA. Pulmonary epithelial cell expression of GM-CSF corrects the alveolar proteinosis in GM-CSF-deficient mice. J Clin Invest (1996) 97(3):649–55. doi: 10.1172/JCI118461
60. Vlahos R, Bozinovski S, Chan SP, Ivanov S, Lindén A, Hamilton JA, et al. Neutralizing granulocyte/macrophage colony-stimulating factor inhibits cigarette smoke-induced lung inflammation. Am J Respir Crit Care Med (2010) 182(1):34–40. doi: 10.1164/rccm.200912-1794OC
61. Basilico P, Cremona TP, Oevermann A, Piersigilli A, Benarafa C. Increased myeloid cell production and lung bacterial clearance in mice exposed to cigarette smoke. Am J Respir Cell Mol Biol (2016) 54(3):424–35. doi: 10.1165/rcmb.2015-0017OC
62. Ataya A, Knight V, Carey BC, Lee E, Tarling EJ, Wang T. The role of GM-CSF autoantibodies in infection and autoimmune pulmonary alveolar proteinosis: A concise review. Front Immunol (2021) 12:752856. doi: 10.3389/fimmu.2021.752856
63. Yoshida M, Ikegami M, Reed JA, Chroneos ZC, Whitsett JA. GM-CSF regulates protein and lipid catabolism by alveolar macrophages. Am J Physiol Lung Cell Mol Physiol (2001) 280(3):L379–86. doi: 10.1152/ajplung.2001.280.3.L379
64. Taniguchi H, Katoh S, Kadota J, Matsubara Y, Fukushima K, Mukae H, et al. Interleukin 5 and granulocyte-macrophage colony-stimulating factor levels in bronchoalveolar lavage fluid in interstitial lung disease. Eur Respir J (2000) 16(5):959–64. doi: 10.1183/09031936.00.16595900
65. Xing Z, Braciak T, Ohkawara Y, Sallenave JM, Foley R, Sime PJ, et al. Gene transfer for cytokine functional studies in the lung: the multifunctional role of GM-CSF in pulmonary inflammation. J Leukoc Biol (1996) 59(4):481–8. doi: 10.1002/jlb.59.4.481
66. De Lauretis A, Veeraraghavan S, Renzoni E. Review series: Aspects of interstitial lung disease: connective tissue disease-associated interstitial lung disease: how does it differ from IPF? how should the clinical approach differ? Chron Respir Dis (2011) 8(1):53–82. doi: 10.1177/1479972310393758
67. Vij R, Strek ME. Diagnosis and treatment of connective tissue disease-associated interstitial lung disease. Chest (2013) 143(3):814–24. doi: 10.1378/chest.12-0741
68. Shiomi A, Usui T, Ishikawa Y, Shimizu M, Murakami K, Mimori T. GM-CSF but not IL-17 is critical for the development of severe interstitial lung disease in SKG mice. J Immunol (2014) 193(2):849–59. doi: 10.4049/jimmunol.1303255
69. Kwon OC, Lee EJ, Chang EJ, Youn J, Ghang B, Hong S, et al. IL-17A+GM-CSF+ neutrophils are the major infiltrating cells in interstitial lung disease in an autoimmune arthritis model. Front Immunol (2018) 9:1544. doi: 10.3389/fimmu.2018.01544
70. Cates EC, Fattouh R, Wattie J, Inman MD, Goncharova S, Coyle AJ, et al. Intranasal exposure of mice to house dust mite elicits allergic airway inflammation via a GM-CSF-mediated mechanism. J Immunol (2004) 173(10):6384–92. doi: 10.4049/jimmunol.173.10.6384
71. Nobs SP, Kayhan M, Kopf M. GM-CSF intrinsically controls eosinophil accumulation in the setting of allergic airway inflammation. J Allergy Clin Immunol (2019) 143(4):1513–1524 e2. doi: 10.1016/j.jaci.2018.08.044
72. Lee KMC, Achuthan AA, Hamilton JA. GM-CSF: A promising target in inflammation and autoimmunity. Immunotargets Ther (2020) 9:225–40. doi: 10.2147/ITT.S262566
73. Yamashita N, Tashimo H, Ishida H, Kaneko F, Nakano J, Kato H, et al. Attenuation of airway hyperresponsiveness in a murine asthma model by neutralization of granulocyte-macrophage colony-stimulating factor (GM-CSF). Cell Immunol (2002) 219(2):92–7. doi: 10.1016/s0008-8749(02)00565-8
74. Nobs SP, Pohlmeier L, Li F, Kayhan M, Becher B, Kopf M. GM-CSF instigates a dendritic cell-t-cell inflammatory circuit that drives chronic asthma development. J Allergy Clin Immunol (2021) 147(6):2118–2133 e3. doi: 10.1016/j.jaci.2020.12.638
75. Mehta AJ, Yeligar SM, Elon L, Brown LA, Guidot DM. Alcoholism causes alveolar macrophage zinc deficiency and immune dysfunction. Am J Respir Crit Care Med (2013) 188(6):716–23. doi: 10.1164/rccm.201301-0061OC
76. Slovinsky WS, Romero F, Sales D, Shaghaghi H, Summer R. The involvement of GM-CSF deficiencies in parallel pathways of pulmonary alveolar proteinosis and the alcoholic lung. Alcohol (2019) 80:73–9. doi: 10.1016/j.alcohol.2018.07.006
77. Joshi PC, Applewhite L, Ritzenthaler JD, Roman J, Fernandez AL, Eaton DC, et al. Chronic ethanol ingestion in rats decreases granulocyte-macrophage colony-stimulating factor receptor expression and downstream signaling in the alveolar macrophage. J Immunol (2005) 175(10):6837–45. doi: 10.4049/jimmunol.175.10.6837
78. Joshi PC, Applewhite L, Mitchell PO, Fernainy K, Roman J, Eaton DC, et al. GM-CSF receptor expression and signaling is decreased in lungs of ethanol-fed rats. Am J Physiol Lung Cell Mol Physiol (2006) 291(6):L1150–8. doi: 10.1152/ajplung.00150.2006
79. Mehta AJ, Joshi PC, Fan X, Brown LA, Ritzenthaler JD, Roman J, et al. Zinc supplementation restores PU.1 and Nrf2 nuclear binding in alveolar macrophages and improves redox balance and bacterial clearance in the lungs of alcohol-fed rats. Alcohol Clin Exp Res (2011) 35(8):1519–28. doi: 10.1111/j.1530-0277.2011.01488.x
80. Moss M, Steinberg KP, Guidot DM, Duhon GF, Treece P, Wolken R, et al. The effect of chronic alcohol abuse on the incidence of ARDS and the severity of the multiple organ dysfunction syndrome in adults with septic shock: an interim and multivariate analysis. Chest (1999) 116(1 Suppl):97S–8S. doi: 10.1378/chest.116.suppl_1.97S
81. Joshi PC, Mehta A, Jabber WS, Fan X, Guidot DM. Zinc deficiency mediates alcohol-induced alveolar epithelial and macrophage dysfunction in rats. Am J Respir Cell Mol Biol (2009) 41(2):207–16. doi: 10.1165/rcmb.2008-0209OC
82. Pelaez A, Bechara RI, Joshi PC, Brown LA, Guidot DM. Granulocyte/macrophage colony-stimulating factor treatment improves alveolar epithelial barrier function in alcoholic rat lung. Am J Physiol Lung Cell Mol Physiol (2004) 286(1):L106–11. doi: 10.1152/ajplung.00148.2003
83. Iwasaki A, Foxman EF, Molony RD. Early local immune defences in the respiratory tract. Nat Rev Immunol (2017) 17(1):7–20. doi: 10.1038/nri.2016.117
84. Newton AH, Cardani A, Braciale TJ. The host immune response in respiratory virus infection: balancing virus clearance and immunopathology. Semin Immunopathol (2016) 38(4):471–82. doi: 10.1007/s00281-016-0558-0
85. Mccormick TS, Hejal RB, Leal LO, Ghannoum MA. GM-CSF: Orchestrating the pulmonary response to infection. Front Pharmacol (2021) 12:735443. doi: 10.3389/fphar.2021.735443
86. Ortqvist A, Hedlund J, Kalin M. Streptococcus pneumoniae: epidemiology, risk factors, and clinical features. Semin Respir Crit Care Med (2005) 26(6):563–74. doi: 10.1055/s-2005-925523
87. Steinwede K, Tempelhof O, Bolte K, Maus R, Bohling J, Ueberberg B, et al. Local delivery of GM-CSF protects mice from lethal pneumococcal pneumonia. J Immunol (2011) 187(10):5346–56. doi: 10.4049/jimmunol.1101413
88. Umstead TM, Hewage EK, Mathewson M, Beaudoin S, Chroneos ZC, Wang M, et al. Lower respiratory tract delivery, airway clearance, and preclinical efficacy of inhaled GM-CSF in a postinfluenza pneumococcal pneumonia model. Am J Physiol Lung Cell Mol Physiol (2020) 318(4):L571–9. doi: 10.1152/ajplung.00296.2019
89. Rosen LB, Freeman AF, Yang LM, Jutivorakool K, Olivier KN, Angkasekwinai N, et al. Anti-GM-CSF autoantibodies in patients with cryptococcal meningitis. J Immunol (2013) 190(8):3959–66. doi: 10.4049/jimmunol.1202526
90. Saijo T, Chen J, Chen SC, Rosen LB, Yi J, Sorrell TC, et al. Anti-granulocyte-macrophage colony-stimulating factor autoantibodies are a risk factor for central nervous system infection by cryptococcus gattii in otherwise immunocompetent patients. mBio (2014) 5(2):e00912-14. doi: 10.1128/mBio.00912-14
91. Chen GH, Teitz-Tennenbaum S, Neal LM, Murdock BJ, Malachowski AN, Dils AJ, et al. Local GM-CSF-Dependent differentiation and activation of pulmonary dendritic cells and macrophages protect against progressive cryptococcal lung infection in mice. J Immunol (2016) 196(4):1810–21. doi: 10.4049/jimmunol.1501512
92. Nair H, Nokes DJ, Gessner BD, Dherani M, Madhi SA, Singleton RJ, et al. Global burden of acute lower respiratory infections due to respiratory syncytial virus in young children: a systematic review and meta-analysis. Lancet (2010) 375(9725):1545–55. doi: 10.1016/S0140-6736(10)60206-1
93. Sanders CJ, Doherty PC, Thomas PG. Respiratory epithelial cells in innate immunity to influenza virus infection. Cell Tissue Res (2011) 343(1):13–21. doi: 10.1007/s00441-010-1043-z
94. Rösler B, Herold S. Lung epithelial GM-CSF improves host defense function and epithelial repair in influenza virus pneumonia-a new therapeutic strategy? Mol Cell Pediatr (2016) 3(1):29. doi: 10.1186/s40348-016-0055-5
95. Greter M, Helft J, Chow A, Hashimoto D, Mortha A, Agudo-Cantero J, et al. GM-CSF controls nonlymphoid tissue dendritic cell homeostasis but is dispensable for the differentiation of inflammatory dendritic cells. Immunity (2012) 36(6):1031–46. doi: 10.1016/j.immuni.2012.03.027
96. Unkel B, Hoegner K, Clausen BE, Lewe-Schlosser P, Bodner J, Gattenloehner S, et al. Alveolar epithelial cells orchestrate DC function in murine viral pneumonia. J Clin Invest (2012) 122(10):3652–64. doi: 10.1172/JCI62139
97. Anderson G, Casasanta D, Cocchieri A, D'agostino F, Zega M, Damiani G, et al. Diagnostic features of SARS-COVID-2-positive patients: A rapid review and meta-analysis. J Clin Nurs (2021) 30(13-14):1826–37. doi: 10.1111/jocn.15688
98. Hu B, Guo H, Zhou P, Shi ZL. Characteristics of SARS-CoV-2 and COVID-19. Nat Rev Microbiol (2021) 19(3):141–54. doi: 10.1038/s41579-020-00459-7
99. Lang FM, Lee KM, Teijaro JR, Becher B, Hamilton JA. GM-CSF-based treatments in COVID-19: reconciling opposing therapeutic approaches. Nat Rev Immunol (2020) 20(8):507–14. doi: 10.1038/s41577-020-0357-7
100. Wang Z, Li S, Huang B. Alveolar macrophages: Achilles' heel of SARS-CoV-2 infection. Signal Transduct Target Ther (2022) 7(1):242. doi: 10.1038/s41392-022-01106-8
101. Huang C, Wang Y, Li X, Ren L, Zhao J, Hu Y, et al. Clinical features of patients infected with 2019 novel coronavirus in wuhan, China. Lancet (2020) 395(10223):497–506. doi: 10.1016/S0140-6736(20)30183-5
102. Bonaventura A, Vecchié A, Wang TS, Lee E, Cremer PC, Carey B, et al. Targeting GM-CSF in COVID-19 pneumonia: Rationale and strategies. Front Immunol (2020) 11:1625. doi: 10.3389/fimmu.2020.01625
103. Zhou Y, Fu B, Zheng X, Wang D, Zhao C, Qi Y, et al. Pathogenic T-cells and inflammatory monocytes incite inflammatory storms in severe COVID-19 patients. Natl Sci Rev (2020) 7(6):998–1002. doi: 10.1093/nsr/nwaa041
104. Paine R 3rd, Morris SB, Jin H, Wilcoxen SE, Phare SM, Moore BB, et al. Impaired functional activity of alveolar macrophages from GM-CSF-deficient mice. Am J Physiol Lung Cell Mol Physiol (2001) 281(5):L1210–8. doi: 10.1152/ajplung.2001.281.5.L1210
105. Lv J, Wang Z, Qu Y, Zhu H, Zhu Q, Tong W, et al. Distinct uptake, amplification, and release of SARS-CoV-2 by M1 and M2 alveolar macrophages. Cell Discovery (2021) 7(1):24. doi: 10.1038/s41421-021-00258-1
106. Burg J, Krump-Konvalinkova V, Bittinger F. Kirkpatrick CJ. GM-CSF expression by human lung microvascular endothelial cells: in vitro and in vivo findings. Am J Physiol Lung Cell Mol Physiol (2002) 283(2):L460–7. doi: 10.1152/ajplung.00249.2001
107. Fitzgerald SM, Chi DS, Hall HK, Reynolds SA, Aramide O, Lee SA, et al. GM-CSF induction in human lung fibroblasts by IL-1beta, TNF-alpha, and macrophage contact. J Interferon Cytokine Res (2003) 23(2):57–65. doi: 10.1089/107999003321455453
108. Koga Y, Hisada T, Ishizuka T, Utsugi M, Ono A, Yatomi M, et al. CREB regulates TNF-alpha-induced GM-CSF secretion via p38 MAPK in human lung fibroblasts. Allergol Int (2016) 65(4):406–13. doi: 10.1016/j.alit.2016.03.006
109. Hamilton JA. GM-CSF-Dependent inflammatory pathways. Front Immunol (2019) 10:2055. doi: 10.3389/fimmu.2019.02055
110. Cakarova L, Marsh LM, Wilhelm J, Mayer K, Grimminger F, Seeger W, et al. Macrophage tumor necrosis factor-alpha induces epithelial expression of granulocyte-macrophage colony-stimulating factor: impact on alveolar epithelial repair. Am J Respir Crit Care Med (2009) 180(6):521–32. doi: 10.1164/rccm.200812-1837OC
111. Mir-Kasimov M, Sturrock A, Mcmanus M, Paine R 3rd. Effect of alveolar epithelial cell plasticity on the regulation of GM-CSF expression. Am J Physiol Lung Cell Mol Physiol (2012) 302(6):L504–11. doi: 10.1152/ajplung.00303.2010
112. Sturrock A, Seedahmed E, Mir-Kasimov M, Boltax J, Mcmanus ML, Paine R 3rd. GM-CSF provides autocrine protection for murine alveolar epithelial cells from oxidant-induced mitochondrial injury. Am J Physiol Lung Cell Mol Physiol (2012) 302(3):L343–51. doi: 10.1152/ajplung.00276.2011
113. Trapnell BC, Carey BC, Uchida K, Suzuki T. Pulmonary alveolar proteinosis, a primary immunodeficiency of impaired GM-CSF stimulation of macrophages. Curr Opin Immunol (2009) 21(5):514–21. doi: 10.1016/j.coi.2009.09.004
114. Dalrymple H, Barna BP, Malur A, Malur AG, Kavuru MS, Thomassen MJ. Alveolar macrophages of GM-CSF knockout mice exhibit mixed M1 and M2 phenotypes. BMC Immunol (2013) 14:41. doi: 10.1186/1471-2172-14-41
115. Enzler T, Gillessen S, Dougan M, Allison JP, Neuberg D, Oble DA, et al. Functional deficiencies of granulocyte-macrophage colony stimulating factor and interleukin-3 contribute to insulitis and destruction of beta cells. Blood (2007) 110(3):954–61. doi: 10.1182/blood-2006-08-043786
116. Berclaz PY, Carey B, Fillipi MD, Wernke-Dollries K, Geraci N, Cush S, et al. GM-CSF regulates a PU.1-dependent transcriptional program determining the pulmonary response to LPS. Am J Respir Cell Mol Biol (2007) 36(1):114–21. doi: 10.1165/rcmb.2006-0174OC
117. Malur A, Baker AD, Mccoy AJ, Wells G, Barna BP, Kavuru MS, et al. Restoration of PPARγ reverses lipid accumulation in alveolar macrophages of GM-CSF knockout mice. Am J Physiol Lung Cell Mol Physiol (2011) 300(1):L73–80. doi: 10.1152/ajplung.00128.2010
118. Chen GH, Olszewski MA, Mcdonald RA, Wells JC, Paine R 3rd, Huffnagle GB, et al. Role of granulocyte macrophage colony-stimulating factor in host defense against pulmonary cryptococcus neoformans infection during murine allergic bronchopulmonary mycosis. Am J Pathol (2007) 170(3):1028–40. doi: 10.2353/ajpath.2007.060595
Keywords: GM-CSF, alveolar macrophages, surfactant, lung homeostasis, lung disease
Citation: Chen Y, Li F, Hua M, Liang M and Song C (2023) Role of GM-CSF in lung balance and disease. Front. Immunol. 14:1158859. doi: 10.3389/fimmu.2023.1158859
Received: 04 February 2023; Accepted: 24 March 2023;
Published: 04 April 2023.
Edited by:
Kuo-Feng Hua, National Ilan University, TaiwanReviewed by:
Brenna Carey, Cincinnati Children’s Research Foundation, United StatesCopyright © 2023 Chen, Li, Hua, Liang and Song. This is an open-access article distributed under the terms of the Creative Commons Attribution License (CC BY). The use, distribution or reproduction in other forums is permitted, provided the original author(s) and the copyright owner(s) are credited and that the original publication in this journal is cited, in accordance with accepted academic practice. No use, distribution or reproduction is permitted which does not comply with these terms.
*Correspondence: Chuanwang Song, YmJtY3Njd0Bmb3htYWlsLmNvbQ==; Meng Liang, bG1oa0BtYWlsLnVzdGMuZWR1LmNu
Disclaimer: All claims expressed in this article are solely those of the authors and do not necessarily represent those of their affiliated organizations, or those of the publisher, the editors and the reviewers. Any product that may be evaluated in this article or claim that may be made by its manufacturer is not guaranteed or endorsed by the publisher.
Research integrity at Frontiers
Learn more about the work of our research integrity team to safeguard the quality of each article we publish.