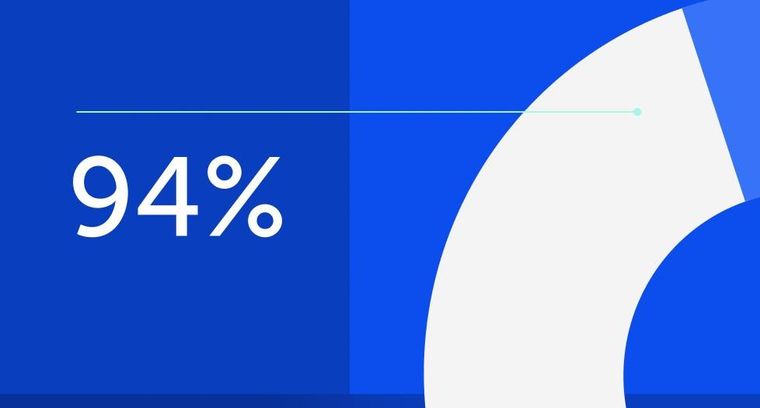
94% of researchers rate our articles as excellent or good
Learn more about the work of our research integrity team to safeguard the quality of each article we publish.
Find out more
BRIEF RESEARCH REPORT article
Front. Immunol., 11 April 2023
Sec. Multiple Sclerosis and Neuroimmunology
Volume 14 - 2023 | https://doi.org/10.3389/fimmu.2023.1158460
This article is part of the Research TopicNeuroimmune cell signaling in COVID-19View all 8 articles
Despite long-term sequelae of COVID-19 are emerging as a substantial public health concern, the mechanism underlying these processes still unclear. Evidence demonstrates that SARS-CoV-2 Spike protein can reach different brain regions, irrespective of viral brain replication resulting in activation of pattern recognition receptors (PRRs) and neuroinflammation. Considering that microglia dysfunction, which is regulated by a whole array of purinergic receptors, may be a central event in COVID-19 neuropathology, we investigated the impact of SARS-CoV-2 Spike protein on microglial purinergic signaling. Here, we demonstrate that cultured microglial cells (BV2 line) exposed to Spike protein induce ATP secretion and upregulation of P2Y6, P2Y12, NTPDase2 and NTPDase3 transcripts. Also, immunocytochemistry analysis shows that spike protein increases the expression of P2X7, P2Y1, P2Y6, and P2Y12 in BV2 cells. Additional, hippocampal tissue of Spike infused animals (6,5ug/site, i.c.v.) presents increased mRNA levels of P2X7, P2Y1, P2Y6, P2Y12, NTPDase1, and NTPDase2. Immunohistochemistry experiments confirmed high expression of the P2X7 receptor in microglial cells in CA3/DG hippocampal regions after spike infusion. These findings suggest that SARS-CoV-2 Spike protein modulates microglial purinergic signaling and opens new avenues for investigating the potential of purinergic receptors to mitigate COVID-19 consequences.
COVID-19, the disease caused by the severe acute respiratory syndrome coronavirus 2 (SARS-CoV-2), is associated with variable outcomes. Beyond the respiratory complications produced by the viral infection, a range of neurological complications may occur in the acute phase of COVID-19, including confusion, strokes, and neuromuscular disorders. Additionally, about 35% of infected patients with different ages and severity of the disease experience persistent attention and memory deficits, called as Long COVID (1, 2). Several studies have proposed that neurological dysfunctions seen in COVID-19 may be due to different mechanisms, including ischemic and inflammatory events. Moreover, SARS-CoV-2 may lead to pro-inflammatory and cytotoxic scenarios in the central nervous system regardless of local viral replication, induced only by its presence in the tissues (3). Nevertheless, whether the brain presence of SARS-CoV-2 viral particles and/or its products is a crucial event for the development of cognitive impairment in post-COVID patients remains unknown.
Microglia are the most prominent immune and cytokine-producing cells in the brain that respond to pathogen-associated molecular patterns (PAMPs) and damage-associated molecular patterns (DAMPs) to drive innate immune responses and neuroinflammation. Viral infections modulate microglial functions resulting in pathological synaptic remodeling, which culminates in altered cognition and behavior (4, 5). Matschke and colleagues (6) showed that post-mortem analysis of brain tissue from COVID-19 patients presented intense microgliosis and neuroinflammatory response. Microglial activation in SARS-CoV-2 infections has also been demonstrated in vivo and in vitro models (7–10). Recently, we and others demonstrated that SARS-CoV-2 Spike protein S1 subunit appears to act as a pathogen-associated molecular pattern through TLR4, activating microglia and inducing secretion of proinflammatory mediators (8, 10–13). Our group also demonstrated that mice brain infusion of Spike protein induces late cognitive dysfunction, recapitulating post-COVID syndrome, through microglia-dependent engulfment of synapses (13).
Purinergic system is one of the fundamental signaling systems that establish microglial behavior in a wide spectrum of conditions (14). Purinergic signaling is a preserved pathway across several species stimulated by nucleotides and nucleosides such as ATP and ADP and involves purinergic receptors and purinergic enzymes called ectonucleotidases. Purinergic receptors differ in their ligands and specificity. They are classified into three types: P1, mobilized by adenosine (composed of four subtypes: A1, A2A, A2B, and A3), and the subtypes that are mobilized by nucleotides: P2X(1–7) and P2Y(1,2,4,6,11–14) receptors (14–16).
P2X7, P2X4, P2Y4, P2Y6, P2Y12, A2A, and A3 are the primary purinergic receptors expressed by microglial cells. These receptors are associated with neurodegenerative events and may act as protective or degenerative mediators, depending on the disease and its progression (15, 17, 18). Many classes of purinergic receptors (P2Y12, P2Y6, P2Y4, P2X4, P2X7, A2A, and A3) can influence microglial behavior. Of note, P2X7 receptor is critical for microglial activation and secretion of proinflammatory cytokines, such as IL-1β, IL-18, and IL-6 (19, 20). P2Y12 and A3 receptors are primary participants after neuronal damage, modulating microglial activation and extension process (18). Furthermore, P2Y12 acts on microglia migration (18). Nevertheless, no studies have addressed the effects of SARS-CoV2 on microglial purinergic signaling. Therefore, to further investigate the impact of microgliosis in SARS-CoV-2 pathology, we investigated the effect of Spike protein on microglial purinergic signaling. Our findings suggest that SARS-CoV-2 Spike protein, independently of viral replication, is able to modify the expression of purinergic receptors involved in neuroinflammation, highlighting the relevance of these targets to managing long COVID symptoms.
BV-2 cells were cultured in RPMI-1640 medium (Sigma-Aldrich, MO, USA) supplemented with 10% fetal bovine serum (Sigma-Aldrich, MO, USA), antibiotics (100 IU penicillin/mL and 100 mg streptomycin/mL; Gibco®). Cells were tested for mycoplasma contamination.
In this study, we used 8–12-week-old male Swiss mice. Animals were housed in groups of five per cage with free access to food and water, a 12-h light/dark cycle, and controlled temperature and humidity. All procedures followed the Principles of Laboratory Animal Care (US National Institutes of Health) and were approved by the Institutional Animal Care and Use Committee of the Federal University of Rio de Janeiro (protocol number: 068/2).
The trimeric spike protein (1–1208aa) of SARS-CoV-2 was purified according to Cunha et al. (21) and obtained from the Cell Culture Engineering Laboratory of COPPE/UFRJ. Spike protein purity and antigenicity have already been confirmed in previous studies (21, 22). For in vitro experiments, BV-2 cells were plated in 6- or 24-well tissue culture plates (TPP AG, Switzerland) at 1 x 106 or 2 x 105 cells per well. Cells were left untreated or stimulated with 0.5 or 1 µg/mL SARS−CoV−2 spike protein. After spike protein stimulation, cell supernatants were tested for endotoxin contamination using the Pierce™ Chromogenic Endotoxin Quant Kit (Thermo Scientific, NJ, USA). Endotoxin levels were < 0.05 EU/mL.
To evaluate the effects of spike protein in vivo, adult male Swiss mice were anesthetized with 2.5% isoflurane (Cristália; São Paulo, Brazil) using a vaporizer system (Norwell, MA) and 6.5 µg spike protein or vehicle (saline) were slowly infused using a Hamilton syringe into the lateral ventricle (ICV). After 45 days of SARS−CoV−2 spike protein ICV infusion, animals were euthanized, and hippocampal tissues of each group were quickly separated and stored in liquid nitrogen before use.
BV-2 cells were plated in 6-well tissue culture plates (TPP AG, Switzerland) at 1 x 106 cells per well. Cells were left untreated or stimulated with 0.5 or 1 µg/mL SARS−CoV−2 spike protein for 2 h. The measurements of eATP from the culture supernatant were performed using the ATP determination kit (Life® Probes; #A22066) by real-time luminometry, according to the manufacturer’s instructions. The luminescence of samples plated onto black 96-well plates was read in a SpectraMax®M5/M5e Multimode Plate Reader (Molecular Devices), and results were expressed as picomoles of ATP.
The total RNA was isolated using the ReliaPrep™ RNA Miniprep Systems kit (Promega Corporation, WI, USA) according to the manufacturer’s instructions. RNA samples were quantified, and the purity was assessed using a Nanodrop Lite spectrophotometer (Thermo Scientific, NJ, USA). The synthesis of cDNA was performed using 1 µg of total RNA using the High-Capacity Reverse Transcription Kit with RNase Inhibitor (Thermo Fisher Scientific, NJ, USA) according to the manufacturer’s instructions in a Master Cycler Gradient thermocycler (Eppendorf, Hamburg, Germany).
The real-time quantitative PCR reactions (RT-qPCR) were performed using the GoTaq® qPCR Master Mix (Promega Corporation, WI, USA) in a QuantStudio 1 Real-Time PCR System (Thermo Scientific, NJ, USA). The reactions were performed in a final volume of 10 μl, using 2 μl of diluted cDNA (1:10) and 300 nM of each primer (5’-3’): P2rx4 forward AGACGGACCAGTGATGCCTAAC and reverse TGGAGTGGAGACCGAGTGAGA; P2rx7 forward AATCGGTGTGTTTCCTTTGG and reverse CCGGGTGACTTTGTTTGTCT; P2ry1 forward GACTGACTGGATCTTCGGGGA and reverse CCACCACAATGAGCCACACC; P2ry2 forward TGACGACTCAAGACGGACAG and reverse GTCCCCTACAGCTCCCCTAC; P2ry4 forward ACTGGCTTCTGCAAGTTCGT and reverse AGGCAGCCAGCTACTACCAA; P2ry6 forward TGCTGCTTGGGTAGTGTGTGG and reverse GTAAGGCTATGAAGGGCAGC; P2ry12 forward CACAGAGGGCTTTGGGAACTTA and reverse GATTCAGCAGAAGCAGGACCA; Entpd1 forward AGCTGCCCCTTATGGAAGAT and reverse TCAGTCCCACAGCAATCAAA; Entpd2 forward TTCCTGGGATGTCAGGTCT and reverse GTCTCTGGTGCTTGCCTTTC; Entpd3 forward ACCTGTCCCGTGCTTAAATG and reverse AGACAGAGTGAAGCCCCTGA; Nt5e forward CAGGAAATCCACCTTCCAAA and reverse AACCTTCAGGTAGCCCAGGT; Actb forward TATGCCAACACAGTGCTGTCTGG and reverse TACTCCTGCTTGCTGATCCACAT. The relative cDNA expression was calculated using the comparative cycle threshold method. The β-actin gene (Actb) was used as an endogenous control. The results were expressed as relative expression of the gene of interest/Actb.
Ecto-nucleoside triphosphate diphosphohydrolases (E-NTPDases) activities were estimated in a reaction medium consisting of a 20 mM Hepes buffer (pH 7.5) containing 1 mM CaCl2, 120 mM NaCl, 5 mM KCl, 60 mM glucose, 1 mM sodium azide and 0.1% mM albumin (all reagents from Sigma-Aldrich, MO, USA). BV2 cells (2 x 105) were used in a final volume of 200 µl of reaction medium, and enzymatic reactions were started by the addition of ATP for a final concentration of 2 mM, followed by incubation for 30 minutes at 37 °C. Reactions were stopped by the addition of 200 µl of 10% trichloroacetic acid (TCA) (Sigma-Aldrich, MO, USA). Incubation times, protein concentrations, reaction mixtures, and substrate concentrations were chosen based on a previous study (23, 24). The amount of inorganic phosphate (Pi) released was measured using the colorimetric method described by Chan et al. (25). Controls to correct for non-enzymatic Pi in the samples were performed. All reactions were performed in triplicates, and enzyme activities were expressed in nmol Pi released per minute per number of cells.
BV-2 cells were plated in 24-well plates at a density of 2 x 105 cells per well and stimulated with 1 µg/mL SARS−CoV−2 spike protein for 24 h. After stimulation, cells were fixed with 4% paraformaldehyde and 4% sucrose for 15 min at room temperature, permeabilized with 0.5% Triton X-100 for 30 minutes (except for cells were stained with extracellular anti-P2X7 antibody), and then blocked with 10% horse serum and 1% bovine serum albumin (BSA) in phosphate-buffered saline (PBS) for 30 min at room temperature. Samples were then incubated overnight with the following primary antibodies diluted in 0.1% BSA in PBS: rabbit anti-P2X7 #APR-008 (1:100), rabbit anti-P2Y1 #APR-009 (1:100), rabbit anti-P2Y4 #APR-006 (1:300), rabbit anti-P2Y6 #APR-011 (1:500), rabbit anti-P2Y12 #APR-012 (1:50) (Alomone Labs, Jerusalem, Israel), goat anti-CD39 #AF4398 (1:200) (R&D Systems, Minneapolis, MN), rabbit ENTPD2/CD39L1 # BS-11515R (1:100) (Thermo Fisher Scientific, NJ, USA). Cells were then washed and incubated at room temperature for 1 h with the secondary antibody (diluted to 1:300, in 0.1% BSA in PBS): anti-rabbit IgG (H+L)-Alexa Fluor® 594 (Thermo Fisher Scientific, NJ, USA). Samples were stained with Hoescht nuclear dye (1:10,000) (Life Technologies, Eugene, OR) and then mounted and examined in a fluorescence microscope Zeiss AxioVert 200M. Three-dimensional images (Z-stack) were obtained on a Spinning Disk Confocal Microscope ZEISS Cell Observer SD (Peabody, MA, USA). Mean fluorescence intensity was measured using Zen Lite Blue software (Carl Zeiss). For this quantification, the background was initially subtracted, and the region of interest was selected in individual cells using the software’s freehand selection tool to calculate the mean fluorescence intensity in the presence of different treatments. The software calculated these results based on the intensity of the corresponding pixels. The experiments were performed in triplicate, and 70–90 cells per field were evaluated in ten fields per coverslip.
Slides containing the hippocampal formation were deparaffinized, and antigen retrieval was carried out by incubation in citrate buffer solution (pH 6.0) at 95 °C for 40 min. Then, samples were incubated with blocking buffer (PBS containing 0.025% Triton, 3% BSA, and 5% normal goat serum) for 2 h. Slides were then incubated overnight with primary antibody rabbit anti-Iba-1 (FUJIFILM Wako Pure Chemicals Corporation, Osaka, Japan; #019-19741). On the following day, samples were washed and incubated at room temperature for 1 h with the secondary antibody (diluted to 1:300, in 0.1% BSA in PBS): anti-rabbit IgG (H+L)-Alexa Fluor® 546 (Thermo Fisher Scientific, NJ, USA). For the second staining, samples were washed, blocked again, and incubated overnight with primary rabbit Anti-P2X7 (Alomone Labs, Jerusalem, Israel; #APR-008). On the third day, samples were washed and incubated at room temperature for 1 h with the secondary antibody (diluted to 1:300, in 0.1% BSA in PBS): anti-rabbit IgG (H+L)-Alexa Fluor® 488 (Thermo Fisher Scientific, NJ, USA). Negative controls with secondary antibodies were performed. Images were acquired using a fluorescence microscope Zeiss AxioVert 200M. The three-dimensional images (Z-stack) were generated on a Spinning Disk Confocal Microscope ZEISS Cell Observer SD (Peabody, MA, USA). Mean fluorescence intensity was measured using Zen Lite Blue software (Carl Zeiss). For this quantification, the background was initially subtracted, and the fluorescence of the entire field corresponding to the tissue was analyzed. The demarcation of the region was performed using the software’s freehand selection tool to calculate the average fluorescence intensity in the presence of different treatments. The software calculated these results based on the intensity of the corresponding pixels. Four fields per coverslip were evaluated with four animals per group.
Results were expressed as mean ± standard error of the mean. Statistical analysis was performed using the t-test or one-way analysis of variance followed by Tukey multiple range tests, using the Prism 8.0.1 software (GraphPad Software, La Jolla, CA, USA). Differences between groups were considered statistically significant when p < 0.05.
Extracellular ATP and its derivatives (i.e., ADP and adenosine) have been shown to modulate microglial cell activation and function through purinergic receptors. We observed that stimulation of BV-2 cells with SARS-CoV-2 Spike protein (1 µg/mL) induced ATP secretion (Figure 1A) and increased P2ry6, P2ry12, Entpd2, and Entpd3 transcript levels (p < 0.05; Figures 1G, H, J, K, respectively). No significant differences were observed for P2rx7, P2rx4, P2ry1, P2ry2, Entpd1, and Nt5e transcript levels (p > 0.05; Figures 1B–F, I, L, respectively) in cells treated with Spike protein at the time point evaluated. No significant changes were detected after 0.5 µg/mL Spike protein treatment (p > 0.05; Figure 1). Immunocytochemistry analysis showed that the presence of Spike protein (1 µg/mL) increased the protein expression of P2X7 (p < 0.05; Figures 2A–C), P2Y1 (p < 0.0001; Figures 2D–F), P2Y6 (p < 0.01; Figures 2J–L), and P2Y12 (p < 0.0001; Figures 2M–O) receptors in BV2 microglial cells. No differences were observed for P2Y4 (p>0,05; Figures 2G–I). These data suggest a crucial role of purinergic signaling through diphosphonucleosides (as ADP and UDP) in SARS-CoV-2 infection.
Figure 1 SARS−CoV−2 spike protein increases ATP secretion and purinergic signaling components transcript levels in microglial cells. BV-2 cells were left untreated (Ctrl) or stimulated with 0.5 or 1 µg/mL SARS−CoV−2 spike protein for 2h for ATP quantification in culture supernatants or 24 h for qPCRs. (A) ATP concentration in cell supernatants. The levels of transcripts for (B) P2X7, (C) P2X4, (D) P2Y1, (E) P2Y2, (F) P2Y4, (G) P2Y6, (H) P2Y12, (I) E-NTPDase1, (J) E-NTPDase2, (K) E-NTPDase3, and (L) CD73 were analyzed by RT-qPCR. Data are representative of three independent experiments (n=3) performed in triplicates and expressed as mean ± SEM. Statistically significant differences between Ctrl and treated groups are represented by asterisks (*, p < 0.05). One-way analysis of variance, Tukey’s test.
Figure 2 SARS−CoV−2 spike protein increases the expression of P2 receptors and ectonucleotidases in microglial cells. BV-2 cells were left untreated (Ctrl) or stimulated with 1 µg/mL SARS−CoV−2 spike protein for 24 h. Representative images and quantitative analysis for (A–C) P2X7, (D–F) P2Y1, (G–I) P2Y4, (J–L) P2Y6, (M–O) P2Y12, E-NTPDase1 (P–R), and E-NTPDase2 (S–U). Data are representative of three independent experiments (n=3) and expressed as mean ± SEM of 10 fields per condition. (V) ATP hydrolysis assay. Data are representative of three independent experiments (n=3) and expressed as mean ± SEM. Statistically significant differences between Ctrl and treated groups are represented by asterisks (*p < 0.05; **p < 0.01; ****p < 0.0001). Student’s t-test. Scale bars: 20 μm.
Ectonucleotidases are critical enzymes that control the availability of purinergic receptors ligands. We observed that BV-2 cells stimulated with Spike protein (1 µg/mL) increased E-NTPDase-1/CD39 (p < 0.0001; Figures 2P–R) and E-NTPDase2 (p < 0.0001; Figures 2S–U) membrane protein expression. In addition, ectonucleotidase assay showed an increased ATP hydrolysis in these cells stimulated with Spike protein (p < 0.05; Figure 2V).
Using hippocampal tissue of Spike-infused mice (6.5 µg/site, ICV), we found an increase in mRNA levels of P2rx7, P2rx4, P2ry6, and P2ry12 receptors (Figures 3A, B, F, G, respectively; p < 0.05). Likewise, we also found and up-regulation of ectonucleotidases Entpd1 and Entpd2 in the hippocampus of mice hippocampus after Spike brain infusion (Figures 3H, I; p < 0.05). No significant differences were observed for P2ry1, P2ry2, P2ry4, Entpd3, and Nt5e transcripts (p > 0.05; Figures 3C, D, E, J, K, respectively).
Figure 3 SARS−CoV−2 spike protein increases purinergic signaling components transcript levels in mice hippocampus. The levels of transcripts for (A) P2X7, (B) P2X4, (C) P2Y1, (D) P2Y2, (E) P2Y4, (F) P2Y6, (G) P2Y12, (H) E-NTPDase1, (I) E-NTPDase2, (J) E-NTPDase3, and (K) CD73 in hippocampus of mice post-spike ICV injection were analyzed by RT-qPCR. Data are expressed as mean ± SEM. Statistically significant differences between the control (Ctrl) and treated group are represented by asterisks (*, p < 0.05). Outliers were identified and excluded using Prism 8.0.1 software (GraphPad Software, La Jolla, CA, USA). Student’s t-test (Control group n = 3; Spike group n = 5–7).
The P2X7 receptor is the most relevant in neuroinflammatory diseases. Our immunofluorescence analysis confirmed an increase in microglial P2X7 expression in CA3 (Figures 4A, C; p > 0.01) and DG (Figures 4B, C; p < 0.001) hippocampal regions from spike-infused mice, suggesting that ATP-P2X7 signaling might be involved in neurological alterations induced by SARS-CoV-2 infection.
Figure 4 SARS−CoV−2 spike protein increases P2X7 receptor expression in hippocampal microglial cells. Representative images of P2X7 receptor and Iba-1 immunostaining in the CA3 (A) and dentate gyrus (DG) (B) hippocampal regions of Ctrl or spike-infused mice. Scale bar = 20 μm. (C) Quantification of P2X7 receptor fluorescence in Iba-1-positive cells from control (Ctrl) or spike-infused mice in CA3 and DG hippocampal regions. Data are expressed as mean ± SEM. Statistically significant differences between Ctrl and treated groups are represented by asterisks (**, p < 0.01; ***, p < 0.005). Student’s t-test (n = 4).
The emergence of SARS-CoV-2 triggered substantial efforts to understand its pathophysiology and long-term sequelae. Among COVID-19 manifestations, one of the most worrying is the development of multiple neurological complications (8, 26). Microglia are vital cells for inflammatory responses against central nervous system-invading pathogens. We and others previously found that SARS-CoV-2 spike protein induced microglia activation and cytokine secretion via TLR4 receptor activation (10, 13). In addition to TLRs, purinergic receptors modulate microglia activation and migration (18) and are critical for the host’s defense against infections (15, 27, 28). This study provides the first experimental evidence that SARS-CoV-2 spike protein induces ATP secretion and alters purinergic receptors and nucleotide metabolizing enzymes in vitro and in vivo in microglial cells.
Among the P2 receptors, P2X and P2Y11 receptors are chiefly activated by extracellular ATP; ATP and UTP activate P2Y2; while ADP activates P2Y1, P2Y12, and P2Y13. P2Y4, P2Y6, and P2Y14 are activated by uridine nucleotides UTP, UDP, and UDP-glucose, respectively (14, 16). Of note, the rat and mouse P2Y2 and P2Y4 are equipotently activated by ATP and UTP, and P2Y11 has not been cloned in rodents (29, 30). P2X7, P2X4, P2Y1, P2Y4, P2Y6, and P2Y12, are the central P2 receptors that modulate microglial function in health and disease. Even though P2X4 and P2X7 are similar in their structure, P2X4 is highly sensitive and can be sensitized by low ATP concentrations (16).
P2X4 and P2X7 receptors are ion channels upregulated in neuroinflammatory conditions (17, 31, 32). These receptors induce the production of free radicals and increase oxidative stress in inflammatory and neurodegenerative diseases (33, 34). TLR4 stimulation increases P2X7 expression in microglial cells and astrocytes (35–37). P2X7 receptor inhibition decreases LPS-induced cytokine production in cultured human microglial cells and brain tissue of septic mice (33, 36). Notably, the P2X7 receptor is the second signal for NLRP3 inflammasome activation and IL-1β secretion, a cytokine intimately related to synaptic loss and depressive-like behavior following inflammatory and chronic stress conditions (38–40).
Therefore, the increase in P2X7 receptor levels in BV2 microglial cells and the hippocampus of mice injected with SARS-CoV-2 spike protein suggests that P2X7 might contribute to neuroinflammatory events related to COVID-19. Indeed, the P2X7 receptor is involved in microglial-related neurological conditions, including Alzheimer’s disease, HIV-related dementia, and sepsis-associated encephalopathy (41–43). In Alzheimer’s disease, the characteristic accumulation of β-amyloid peptide may induce neuroinflammation through P2X7 receptor mobilization and subsequent activation of NLRP3/caspase-1 pathway (42). Furthermore, the aggregation of these peptides, associated with stimulation by LPS, activates microglia to an M1 proinflammatory profile characterized by TLR4/MyD88/nuclear factor-kappa B signaling pathway activation and cytokine release. Pharmacological or genetic ablation of the P2X7 receptor shifts microglial cells toward an anti-inflammatory and neuroprotective M2 profile, with a reduction in IL-1β production and better cognitive outcomes (42). Interestingly, the HIV glycoprotein gp120 also increases P2X7 receptor protein and mRNA levels in BV2 microglial cells, contributing to nuclear factor-kappa B activation and secretion of inflammatory molecules, leading to microglial loss and neurological alterations such as memory loss and cognitive impairment (41, 44).
Our analysis revealed an increased expression of P2Y1, P2Y6, and P2Y12 receptors (in addition to the P2X7 receptor) in BV2 cells and mice hippocampus. The P2Y12 receptor is deeply involved in microglia motility (18, 45, 46). P2Y12 expression and levels of inducible nitric oxide synthase (iNOS) increase in mouse brain following nitroglycerin treatment in the chronic migraine murine model. Furthermore, inhibition of the P2Y12 receptor reduced iNOS expression in mouse medulla and decreased iNOS, IL-1β, and TNF-α concentrations in BV2 cells pretreated with LPS (47).
The P2Y6 receptor plays a crucial role in microglia after brain injury, promoting the clearance of damaged cells and dangerous debris by stimulating phagocytosis. This phenomenon occurs primarily in brain lesions caused by an ischemic stroke, accelerating the recovery of the damaged area (48, 49). Nonetheless, this phagocytic process can be unspecific and culminates in destroying viable cells. Indeed, knockout of the P2Y6 microglial receptor inhibits phagocytosis of these intact cells, preventing neuronal loss and memory difficulties in two models of neurodegeneration linked to Alzheimer’s disease, related to β-amyloid peptides or tau proteins (50). Inhibition of P2Y6/UDP signaling using exogenous apyrases, reactive Blue 2 (a general P2 inhibitor), or a P2Y6 receptor-specific inhibitor prevents neuronal loss after LPS stimulation (51). LPS treatment coupled to a purinergic ligand (2-methylthioladenosine-5′-diphosphate; 2MeSADP) increased the expression of ionized calcium-binding adapter molecule 1 (Iba-1), a marker of microglia activation, and increased P2Y6 receptor, CD11b and DAP12 expression, which are proteins involved in microglial phagocytosis (52). Indeed, LPS stimulation induces UDP secretion in BV2 cells, increasing the production of inflammatory cytokines. The knockdown of the P2Y6 receptor improves neuronal cell viability and diminishes apoptosis in cells (SH-SY5Y, N2a, and PC12 cells) exposed to a conditioned medium from LPS-stimulated BV-2 cells. These findings suggest that these P2Y receptors might be involved in neuroinflammatory and neurological consequences of SARS-CoV-2 infection.
Ectonucleotidases are critical enzymes that regulate the composition and magnitude of purinergic signaling by controlling extracellular nucleotide levels. These enzymes differ in their functionality and substrate preferences. E-NTPDase1/CD39 has catalytic properties for the hydrolysis of ATP and ADP at the same ratio (1:1). In contrast, E-NTPDase2 and E-NTPDase3 prefer to hydrolyze ATP over ADP in a proportion higher than 30-fold and 3-fold, respectively (53, 54). In the current work, we found that the relative expression of the enzymes that prefer to hydrolyze triphosphate nucleotides (i.e., E-NTPDase2 and E-NTPDase3) were upregulated after spike protein stimulation in vitro, probably favoring ADP/UDP generation and (therefore) a diphosphate nucleotide signaling pathway by activating P2Y1, P2Y6, and P2Y12 receptors. In vivo, E-NTPDase1 and 2 were also upregulated, agreeing with our in vitro data.
Although these P2Y receptors are related to neuroinflammation, the rise of ADP production could be correlated with a mechanism to prevent the disease progression because infected neurons secrete nucleotides that activate purinergic receptors in microglia to digest and eliminate compromised cells, as seen in herpes simplex virus-1 encephalitis (55); these authors observed increased E-NTPDase 1 activity which degrades ATP into ADP and activates the P2Y12 receptor in microglia, extending and migrating to damaged sites and eliminating virus-infected neurons. Future studies with knockout animals and specific inhibitors for these P2 receptors and ectonucleotidases are required to understand their role in SARS-CoV-2 infection. Further studies are also required to evaluate purinergic signaling in other cell types using different Spike concentrations or the active virus because the amount of circulating Spike during the course of infection, as well as the extent of its persistence in the blood, varies considerably among COVID patients (56, 57), making it difficult to replicate it in cell cultures. Nevertheless, the concentrations of Spike protein used in our in vitro and in vivo studies were in the same order of magnitude used in previous studies (8, 10, 58, 59).
In summary, this study provides the first evidence that SARS-CoV-2 infection alters purinergic signaling. SARS-CoV-2 spike protein upregulates purinergic receptors involved in neuroinflammatory and neurodegenerative diseases (i.e., P2X7, P2X4, P2Y6, and P2Y12). In addition, spike protein increased the expression of E-NTPDase1 and 2, which favors the formation of diphosphate nucleotides and, consequently, the activation of P2Y6 and P2Y12 receptors. Our findings suggest that these receptors have substantial potential to serve as therapeutic targets to treat or limit neurological changes and cognitive impairments following SARS-CoV-2 infection. Such findings open new avenues for future studies evaluating the specific roles of purinergic receptors in SARS-CoV-2 infection.
The raw data supporting the conclusions of this article will be made available by the authors, without undue reservation.
The animal study was reviewed and approved by Institutional Animal Care and Use Committee of the Federal University of Rio de Janeiro (protocol number: 068/2).
LS made substantial contributions to conception and design, data collection, analysis, and interpretation. LS and RL-A drafted the manuscript. VA and SS participated in material preparation, data collection, and analysis. EP-P, RR, MC, GF, LA, EL, and FF-D participated in material preparation and data collection. EK, JS, FF-D, GP, CF, and RC-S contributed to the conception, design and interpretation of data, and manuscript preparation. All authors contributed to the article and approved the submitted version.
Conselho Nacional de Desenvolvimento Científico e Tecnológico do Brasil – CNPq (305857/2020-7 and 306839/2019-9). Coordenação de Aperfeiçoamento de Pessoal de Nível Superior (CAPES), and Fundação de Amparo à Pesquisa do Estado do Rio de Janeiro - FAPERJ (E-26/202.701/2019, E-26/010.002260/2019, E-26/010.101036/2018, E-26/202.774/2018; E-26/210.240/2020; E-26/211.138/2021, 26/210.823/2021, E-26/211.325/2021, E-26/210.779/2021, E-26/201.086/2022, E-26/200.195/2023).
The authors thank Prof. Leda R. Castilho and her team at the Cell Culture Engineering Laboratory of COPPE/UFRJ for providing the trimeric spike protein in the prefusion conformation. The authors also thank Sthefanie Rodrigues Batista da Silva for technical support.
The authors declare that the research was conducted in the absence of any commercial or financial relationships that could be construed as a potential conflict of interest.
All claims expressed in this article are solely those of the authors and do not necessarily represent those of their affiliated organizations, or those of the publisher, the editors and the reviewers. Any product that may be evaluated in this article, or claim that may be made by its manufacturer, is not guaranteed or endorsed by the publisher.
1. Becker C, Beck K, Zumbrunn S, Memma V, Herzog N, Bissmann B, et al. Long COVID 1 year after hospitalisation for COVID-19: a prospective bicentric cohort study. Swiss Med Wkly (2021) 151:w30091. doi: 10.4414/smw.2021.w30091
2. Spudich S, Nath A. Nervous system consequences of COVID-19. Science (2022) 375:267–9. doi: 10.1126/science.abm2052
3. Pedrosa C, da SG, Goto-Silva L, Temerozo JR, Souza LRQ, Vitória G, et al. Non-permissive SARS-CoV-2 infection in human neurospheres. Stem Cell Res (2021) 54:1–12. doi: 10.1016/j.scr.2021.102436
4. Shi L, Fatemi SH, Sidwell RW, Patterson PH. Maternal influenza infection causes marked behavioral and pharmacological changes in the offspring. J Neurosci (2003) 23 (1), 297–302. doi: 10.1523/JNEUROSCI.23-01-00297.2003
5. Figueiredo CP, Barros-Aragão FGQ, Neris RLS, Frost PS, Soares C, Souza INO, Zeidler JD, et al. Zika virus replicates in adult human brain tissue and impairs synapses and memory in mice. Nat Commun (2019) 10 (1), 3890. doi: 10.1038/s41467-019-11866-7
6. Matschke J, Lütgehetmann M, Hagel C, Sperhake JP, Schröder AS, Edler C, et al. Neuropathology of patients with COVID-19 in Germany: a post-mortem case series. Lancet Neurol (2020) 19:919–29. doi: 10.1016/S1474-4422(20)30308-2
7. Mishra R, Banerjea AC. SARS-CoV-2 spike targets USP33-IRF9 axis via exosomal miR-148a to activate human microglia. Front Immunol (2021) 12:656700. doi: 10.3389/fimmu.2021.656700
8. Frank MG, Nguyen KH, Ball JB, Hopkins S, Kelley T, Baratta MV, et al. SARS-CoV-2 spike S1 subunit induces neuroinflammatory, microglial and behavioral sickness responses: evidence of PAMP-like properties. Brain. Behav Immun (2022) 100:267–77. doi: 10.1016/j.bbi.2021.12.007
9. Jeong GU, Lyu J, Kim K-D, Chung YC, Yoon GY, Lee S, et al. SARS-CoV-2 infection of microglia elicits proinflammatory activation and apoptotic cell death. Microbiol Spectr (2022) 10:1–17. doi: 10.1128/spectrum.01091-22
10. Olajide OA, Iwuanyanwu VU, Adegbola OD, Al-Hindawi AA. SARS-CoV-2 spike glycoprotein S1 induces neuroinflammation in BV-2 microglia. Mol Neurobiol (2022) 59:445–58. doi: 10.1007/s12035-021-02593-6
11. Shirato K, Kizaki T. SARS-CoV-2 spike protein S1 subunit induces pro-inflammatory responses via toll-like receptor 4 signaling in murine and human macrophages. Heliyon (2021) 7:e06187. doi: 10.1016/j.heliyon.2021.e06187
12. Zhao Y, Kuang M, Li J, Zhu L, Jia Z, Guo X, et al. SARS-CoV-2 spike protein interacts with and activates TLR41. Cell Res (2021) 31:818–20. doi: 10.1038/s41422-021-00495-9
13. Fontes-Dantas FL, Fernandes GG, Gutman EG, De Lima EV, Antonio LS, Hammerle MB, et al. SARS-CoV-2 spike protein induces TLR4-mediated long-term cognitive dysfunction recapitulating post-COVID-19 syndrome in mice. Cell Rep (2023) 42:112189. doi: 10.1016/j.celrep.2023.112189
14. Illes P, Müller CE, Jacobson KA, Grutter T, Nicke A, Fountain SJ, et al. Update of P2X receptor properties and their pharmacology: IUPHAR review 30. Br J Pharmacol (2020), 178:489–514. doi: 10.1111/bph.15299
15. Alves VS, Leite-Aguiar R, Silva JP, Coutinho-Silva R, Savio LEB. Purinergic signaling in infectious diseases of the central nervous system. Brain. Behav Immun (2020) 1–11:480–490. doi: 10.1016/j.bbi.2020.07.026
16. Jacobson KA, Delicado EG, Gachet C, Kennedy C, von Kügelgen I, Li B, et al. Update of P2Y receptor pharmacology: IUPHAR review 27. Br J Pharmacol (2020) 177:2413–33. doi: 10.1111/bph.15005
17. Burnstock G. An introduction to the roles of purinergic signalling in neurodegeneration, neuroprotection and neuroregeneration. Neuropharmacology (2015) 104:4–17. doi: 10.1016/j.neuropharm.2015.05.031
18. Illes P, Rubini P, Ulrich H, Zhao Y, Tang Y. Regulation of microglial functions by purinergic mechanisms in the healthy and diseased CNS. Cells (2020) 9:1–24. doi: 10.3390/cells9051108
19. Ferrari D, Chiozzi P, Falzoni S, Hanau S, Di Virgilio F. Purinergic modulation of interleukin-1β release from microglial cells stimulated with bacterial endotoxin. J Exp Med (1997) 185:579–82. doi: 10.1084/jem.185.3.579
20. Shieh CH, Heinrich A, Serchov T, van Calker D, Biber K. P2X7-dependent, but differentially regulated release of IL-6, CCL2, and TNF-α in cultured mouse microglia. Glia (2014) 62:592–607. doi: 10.1002/glia.22628
21. Cunha LER, Stolet AA, Strauch MA, Pereira VAR, Dumard CH, Gomes AMO, et al. Polyclonal F(ab’)2 fragments of equine antibodies raised against the spike protein neutralize SARS-CoV-2 variants with high potency. iScience (2021) 24:1-24. doi: 10.1016/j.isci.2021.103315
22. Alvim RGF, Lima TM, Rodrigues DAS, Marsili FF, Bozza VBT, Higa LM, et al. From a recombinant key antigen to an accurate, affordable serological test: Lessons learnt from COVID-19 for future pandemics. Biochem Eng J (2022), 186:108537. doi: 10.1016/j.bej.2022.108537
23. Vuaden FC, Savio LEB, Bastos CMA, Bogo MR, Bonan CD. Adenosine A2A receptor agonist (CGS-21680) prevents endotoxin-induced effects on nucleotidase activities in mouse lymphocytes. Eur J Pharmacol (2011) 651:212–7. doi: 10.1016/j.ejphar.2010.11.003
24. Savio LEB, De Andrade Mello P, Santos SACS, De Sousa JC, Oliveira SDS, Minshall RD, et al. P2X7 receptor activation increases expression of caveolin-1 and formation of macrophage lipid rafts, thereby boosting CD39 activity. J Cell Sci (2020) 133:1–10. doi: 10.1242/JCS.237560
25. Chan KM, Delfert D, Junger KD. A direct colorimetric assay for Ca2+-stimulated ATPase activity. Anal Biochem (1986) 157:375–80. doi: 10.1016/0003-2697(86)90640-8
26. Camargo-Martínez W, Lozada-Martínez I, Escobar-Collazos A, Navarro-Coronado A, Moscote-Salazar L, Pacheco-Hernández A, et al. Post-COVID 19 neurological syndrome: implications for sequelae’s treatment. J Clin Neurosci (2021) 88:219–25. doi: 10.1016/j.jocn.2021.04.001
27. Savio LEB, Coutinho-Silva R. Immunomodulatory effects of P2X7 receptor in intracellular parasite infections. Curr Opin Pharmacol (2019) 47:53–8. doi: 10.1016/j.coph.2019.02.005
28. Coutinho-Silva R, Savio LEB. Purinergic signalling in host innate immune defence against intracellular pathogens. Biochem Pharmacol (2021), 187:114405. doi: 10.1016/j.bcp.2021.114405
29. Webb TE, Henderson DJ, Roberts JA, Barnard EA. Molecular cloning and characterization of the rat P2Y4 receptor. J Neurochem (1998) 71:1348–57. doi: 10.1046/j.1471-4159.1998.71041348.x
30. Suarez-Huerta N, Pouillon V, Boeynaems J, Robaye B. Molecular cloning and characterization of the mouse P2Y4 nucleotide receptor. Eur J Pharmacol (2001) 416:197–202. doi: 10.1016/s0014-2999(01)00875-5
31. Tewari M, Monika, Varghese RK, Menon M, Seth P. Astrocytes mediate HIV-1 tat-induced neuronal damage via ligand-gated ion channel P2X7R. J Neurochem (2015) 132:464–76. doi: 10.1111/jnc.12953
32. Zhou F, Liu X, Gao L, Zhou X, Cao Q, Niu L, et al. HIV-1 tat enhances purinergic P2Y4 receptor signaling to mediate inflammatory cytokine production and neuronal damage via PI3K/Akt and ERK MAPK pathways. J Neuroinflamm (2019) 16:1–13. doi: 10.1186/s12974-019-1466-8
33. Savio LEB, Andrade MGJ, de Andrade Mello P, Santana PT, Moreira-Souza ACA, Kolling J, et al. P2X7 receptor signaling contributes to sepsis-associated brain dysfunction. Mol Neurobiol (2017) 54:6459–70. doi: 10.1007/s12035-016-0168-9
34. Savio LEB, Leite-Aguiar R, Alves VS, Coutinho-Silva R, Wyse ATS. Purinergic signaling in the modulation of redox biology. Redox Biol (2021) 47:1–12. doi: 10.1016/j.redox.2021.102137
35. Ferrari D, Pizzirani C, Adinolfi E, Lemoli RM, Curti A, Idzko M, et al. The P2X7 receptor: a key player in IL-1 processing and release. J Immunol (2006) 176:3877–83. doi: 10.4049/jimmunol.176.7.3877
36. Choi HB, Ryu JK, Kim SU, McLarnon JG. Modulation of the purinergic P2X7 receptor attenuates lipopolysaccharide-mediated microglial activation and neuronal damage in inflamed brain. J Neurosci (2007) 27:4957–68. doi: 10.1523/JNEUROSCI.5417-06.2007
37. Facci L, Barbierato M, Zusso M, Skaper SD, Giusti P. Serum amyloid a primes microglia for ATP-dependent interleukin-1β release. J Neuroinflamm (2018) 15:1–11. doi: 10.1186/s12974-018-1205-6
38. Mishra A, Kim HJ, Shin AH, Thayer SA. Synapse loss induced by interleukin-1β requires pre- and post-synaptic mechanisms. J Neuroimmune Pharmacol (2012) 7:571–8. doi: 10.1007/s11481-012-9342-7.Synapse
39. Maraula G, Lana D, Coppi E, Gentile F, Mello T, Melani A, et al. The selective antagonism of P2X7 and P2Y1 receptors prevents synaptic failure and affects cell proliferation induced by oxygen and glucose deprivation in rat dentate gyrus. PloS One (2014) 9:1–23. doi: 10.1371/journal.pone.0115273
40. Yue N, Huang H, Zhu X, Han Q, Wang Y, Li B, et al. Activation of P2X7 receptor and NLRP3 inflammasome assembly in hippocampal glial cells mediates chronic stress-induced depressive-like behaviors. J Neuroinflamm (2017) 14:1–15. doi: 10.1186/s12974-017-0865-y
41. Liu Y, Chen GQ, Liu BY, Chen Q, Qian YM, Qin SS, et al. P2X7 receptor in the hippocampus is involved in gp120-induced cognitive dysfunction. Genet Mol Res (2017) 16:1–13. doi: 10.4238/gmr16019356
42. Thawkar BS, Kaur G. Inhibitors of NF-κB and P2X7/NLRP3/Caspase 1 pathway in microglia: Novel therapeutic opportunities in neuroinflammation induced early-stage alzheimer’s disease. J Neuroimmunol (2019) 326:62–74. doi: 10.1016/j.jneuroim.2018.11.010
43. Leite-Aguiar R, Alves VS, Savio LEB, Coutinho-Silva R. Targeting purinergic signaling in the dynamics of disease progression in sepsis. Front Pharmacol (2021) 11:626484. doi: 10.3389/fphar.2020.626484
44. Chen Q, Wu H, Qin S, Liu C, Chen Y, Yang Y, et al. The P2X7 receptor involved in gp120-induced cell injury in BV2 microglia. Inflammation (2016) 39:1814–26. doi: 10.1007/s10753-016-0417-0
45. Gyoneva S, Orr AG, Traynelis SF. Differential regulation of microglial motility by ATP/ADP and adenosine. Park. Relat Disord (2009) 15:S195–9. doi: 10.1016/S1353-8020(09)70813-2
46. De Simone R, Niturad CE, De Nuccio C, Ajmone-Cat MA, Visentin S, Minghetti L. TGF-ß and LPS modulate ADP-induced migration of microglial cells through P2Y1 and P2Y12 receptor expression. J Neurochem (2010) 115:450–9. doi: 10.1111/j.1471-4159.2010.06937.x
47. Jing F, Zhang Y, Long T, He W, Qin G, Zhang D, et al. P2Y12 receptor mediates microglial activation via RhoA/ROCK pathway in the trigeminal nucleus caudalis in a mouse model of chronic migraine. J Neuroinflamm (2019) 16:1–20. doi: 10.1186/s12974-019-1603-4
48. Koizumi S, Shigemoto-Mogami Y, Nasu-Tada K, Shinozaki Y, Ohsawa K, Tsuda M, et al. UDP Acting at P2Y6 receptors is a mediator of microglial phagocytosis. Nature (2007) 446:1091–5. doi: 10.1038/nature05704
49. Wen RX, Shen H, Huang SX, Wang LP, Li ZW, Peng P, et al. P2Y6 receptor inhibition aggravates ischemic brain injury by reducing microglial phagocytosis. CNS Neurosci Ther (2020) 26:416–29. doi: 10.1111/cns.13296
50. Puigdellívol M, Milde S, Vilalta A, Cockram TOJ, Allendorf DH, Lee JY, et al. The microglial P2Y6 receptor mediates neuronal loss and memory deficits in neurodegeneration. Cell Rep (2021) 37:1–11. doi: 10.1016/j.celrep.2021.110148
51. Neher JJ, Neniskyte U, Hornik T, Brown GC. Inhibition of UDP/P2Y6 purinergic signaling prevents phagocytosis of viable neurons by activated microglia in vitro and in vivo. Glia (2014) 62:1463–75. doi: 10.1002/glia.22693
52. Sunkaria A, Bhardwaj S, Halder A, Yadav A, Sandhir R. Migration and phagocytic ability of activated microglia during post-natal development is mediated by calcium-dependent purinergic signalling. Mol Neurobiol (2016) 53:944–54. doi: 10.1007/s12035-014-9064-3
53. Robson SC, Sévigny J, Zimmermann H. The e-NTPDase family of ectonucleotidases: Structure function relationships and pathophysiological significance. Purinergic Signal (2006) 2:409–30. doi: 10.1007/s11302-006-9003-5
54. Morandini A, Savio L, Coutinho-Silva R. The role of P2X7 receptor in infectious inflammatory diseases and the influence of ectonucleotidases. Biomed J (2014) 37:169–77. doi: 10.4103/2319-4170.127803
55. Fekete R, Cserép C, Lénárt N, Tóth K, Orsolits B, Martinecz B, et al. Microglia control the spread of neurotropic virus infection via P2Y12 signalling and recruit monocytes through P2Y12-independent mechanisms. Acta Neuropathol (2018) 136:461–82. doi: 10.1007/s00401-018-1885-0
56. Ogata AF, Maley AM, Wu C, Gilboa T, Norman M, Lazarovits R, et al. Ultra-sensitive serial profiling of SARS-CoV-2 antigens and antibodies in plasma to understand disease progression in COVID-19 patients with severe disease. Clin Chem (2020) 66:1562–72. doi: 10.1093/clinchem/hvaa213
57. Swank Z, Senussi Y, Manickas-Hill Z, Yu XG, Li JZ, Alter G, et al. Persistent circulating severe acute respiratory syndrome coronavirus 2 spike is associated with post-acute coronavirus disease 2019 sequelae. Clin Infect Dis (2023) 76:e487–90. doi: 10.1093/cid/ciac722
58. Dosch SF, Mahajan SD, Collins AR. SARS coronavirus spike protein-induced innate immune response occurs via activation of the NF-kappaB pathway in human monocyte macrophages in vitro. Virus Res (2009) 142:19–27. doi: 10.1016/j.virusres.2009.01.005
Keywords: SARS-CoV-2 spike protein, COVID-19, ectonucleotidase, P2 receptors, CD39, neuroinflammation, microglia
Citation: Alves VS, Santos SACS, Leite-Aguiar R, Paiva-Pereira E, Reis RRd, Calazans ML, Fernandes GG, Antônio LS, de Lima EV, Kurtenbach E, Silva JL, Fontes-Dantas FL, Passos GF, Figueiredo CP, Coutinho-Silva R and Savio LEB (2023) SARS-CoV-2 Spike protein alters microglial purinergic signaling. Front. Immunol. 14:1158460. doi: 10.3389/fimmu.2023.1158460
Received: 03 February 2023; Accepted: 23 March 2023;
Published: 11 April 2023.
Edited by:
Abbas Azadmehr, Babol University of Medical Sciences, IranReviewed by:
Juliana Da Cruz Corrêa-Velloso, The State University of New Jersey, United StatesCopyright © 2023 Alves, Santos, Leite-Aguiar, Paiva-Pereira, Reis, Calazans, Fernandes, Antônio, de Lima, Kurtenbach, Silva, Fontes-Dantas, Passos, Figueiredo, Coutinho-Silva and Savio. This is an open-access article distributed under the terms of the Creative Commons Attribution License (CC BY). The use, distribution or reproduction in other forums is permitted, provided the original author(s) and the copyright owner(s) are credited and that the original publication in this journal is cited, in accordance with accepted academic practice. No use, distribution or reproduction is permitted which does not comply with these terms.
*Correspondence: Luiz Eduardo Baggio Savio, c2F2aW9AYmlvZi51ZnJqLmJy
†These authors share first authorship
Disclaimer: All claims expressed in this article are solely those of the authors and do not necessarily represent those of their affiliated organizations, or those of the publisher, the editors and the reviewers. Any product that may be evaluated in this article or claim that may be made by its manufacturer is not guaranteed or endorsed by the publisher.
Research integrity at Frontiers
Learn more about the work of our research integrity team to safeguard the quality of each article we publish.