- Tianjin Key Laboratory of Digestive Diseases, Tianjin Institute of Digestive Diseases, Department of Gastroenterology and Hepatology, Tianjin Medical University General Hospital, Tianjin, China
Tumor immune microenvironment (TIME), a tumor-derived immune component, is proven to be closely related to the development, metastasis, and recurrence of tumors. Gut microbiota and its fermented-metabolites short-chain fatty acids (SCFAs) play a critical role in maintaining the immune homeostasis of gastrointestinal tumors. Consisting mainly of acetate, propionate, and butyrate, SCFAs can interact with G protein-coupled receptors 43 of T helper 1 cell or restrain histone deacetylases (HDACs) of cytotoxic T lymphocytes to exert immunotherapy effects. Studies have shed light on SCFAs can mediate the differentiation and function of regulatory T cells, as well as cytokine production in TIME. Additionally, SCFAs can alter epigenetic modification of CD8+ T cells by inhibiting HDACs to participate in the immune response process. In gastrointestinal tumors, the abundance of SCFAs and their producing bacteria is significantly reduced. Direct supplementation of dietary fiber and probiotics, or fecal microbiota transplantation to change the structure of gut microbiota can both increase the level of SCFAs and inhibit tumor development. The mechanism by which SCFAs modulate the progression of gastrointestinal tumors has been elucidated in this review, aiming to provide prospects for the development of novel immunotherapeutic strategies.
1 Introduction
Accumulating evidence indicates that tumors have become the leading cause of death. GLOBOCAN counted the global cancer situation and found that the burden of cancer is increasing rapidly worldwide (1). It is universally acknowledged that gut microbiota is interrelated to the occurrence and development of tumors, especially gastrointestinal tumors. Gut microbiota can influence the gut microenvironment by modulating specific bioactive metabolites of bacteria (2). Short-chain fatty acids (SCFAs), as typical products of soluble fiber fermented by gut bacteria, exert critical functions in gut homeostasis. SCFAs can regulate energy metabolism, strengthen the intestinal barrier and exert anti-inflammatory properties. As key regulators of immune function, SCFAs can regulate T cells, B cells, macrophages, and other immune cells (3). Because of the combination of G protein-coupled receptors (GPCRs) or suppression of histone deacetylases (HDACs), SCFAs can affect the signal transduction pathway of immune response and modulate the release of immune-related inflammatory mediators, thus regulating the tumor immune microenvironment (TIME) (4).
TIME, consisting of tumor-infiltrating immune cells, tumor-associated other cells, tumor cells, and extracellular matrix, attracts more and more attention (5). Especially, immune components serve a critical regulative function. The treatment scheme based on the immune system, such as immune checkpoint inhibitors (ICIs), has been applied in the clinic (6, 7). In recent years, the effect of SCFAs on the TIME has been widely studied. Depending on HDAC inhibitor activity, SCFAs can directly affect T cell differentiation and function. In colorectal cancer (CRC) and pancreatic cancer experimental animal models, it has been demonstrated that butyrate could enhance the anti-tumor effect of CD8+ T cells (8, 9). Targeting tumor immunity, supplementation of microbiota-derived SCFAs has become a new way to diagnose, treat, and prevent tumors. Future work can focus on probiotics and fecal microbiota transplantation (FMT), improve the level of SCFAs, regulate gastrointestinal microecology, and activate effective anticancer effects.
2 SCFAs, immune cells and immune microenvironment
Recently, more and more attention is given to the influence of microorganisms and microbial metabolites on the host. SCFAs, consisting of less than 6 carbon numbers, are typical metabolites produced by symbiotic bacteria through fermentation of dietary fiber in the gastrointestinal tract and have been widely studied. The production of SCFAs is a complex process, which is carried out in the colon by a variety of bacteria (Table 1). Among all SCFAs, acetate, propionate, and butyrate are the most representative.
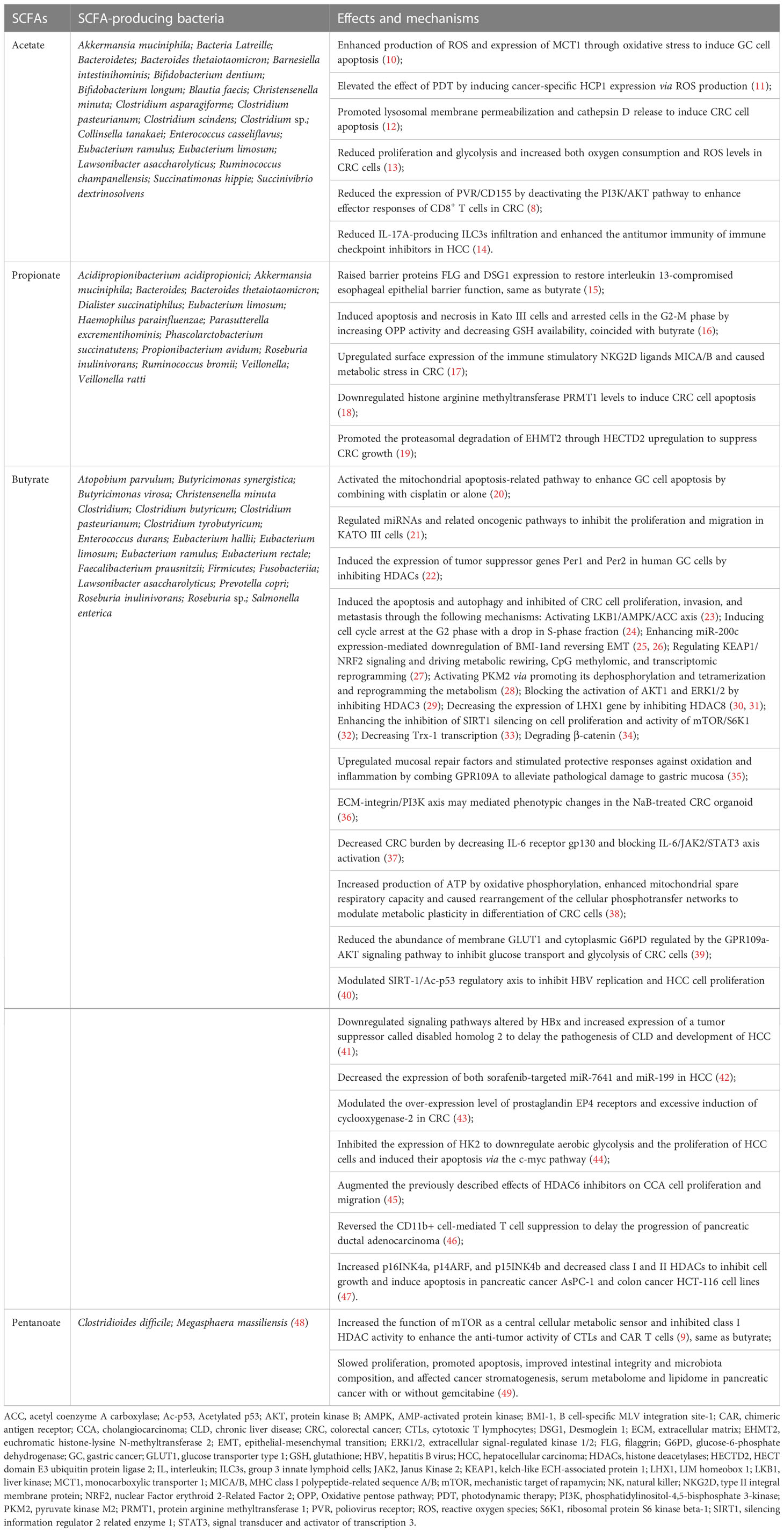
Table 1 Short chain fatty acids and associated-microbiota in tumor immune microenvironment. (Bacteria data sources: gutMGene).
When the SCFAs are produced, the first function is to serve as substrates to provide energy. Most SCFAs are absorbed by colonocytes via two transporters: the monocarboxylate transporter 1 (MCT-1) and the sodium-coupled monocarboxylate transporter 1 (SMCT-1) (50). SCFAs are transferred in an H+-dependent, electroneutral manner by MCT-1, whereas SCFA anions are transported via the electrogenic SMCT-1. In addition to powering the colonocytes, SCFAs are transported to various tissues and organs of the whole body through blood transportation and then regulate biological responses in two main mechanisms (51). On the one hand, SCFAs decrease the activity or expression of HDACs, contributing to increased histone acetylation. It is reported that abnormal activation of HDACs exists in several types of cancer (51). On the other hand, SCFAs combine with the GPCRs, mainly for GPR41 (renamed free fatty acid receptor (FFAR)3), GPR43 (renamed FFAR2), and GPR109A, to exert corresponding signal cascade effects (Figure 1). Some studies have shown that abnormal expression or activity of GPCRs is involved in various tumor progression. Previous studies have summarized the role of SCFAs in regulating energy metabolism, protecting gut integrity, and ameliorating the inflammatory response (52), but the effects of SCFAs on the immune system have not attracted enough attention.
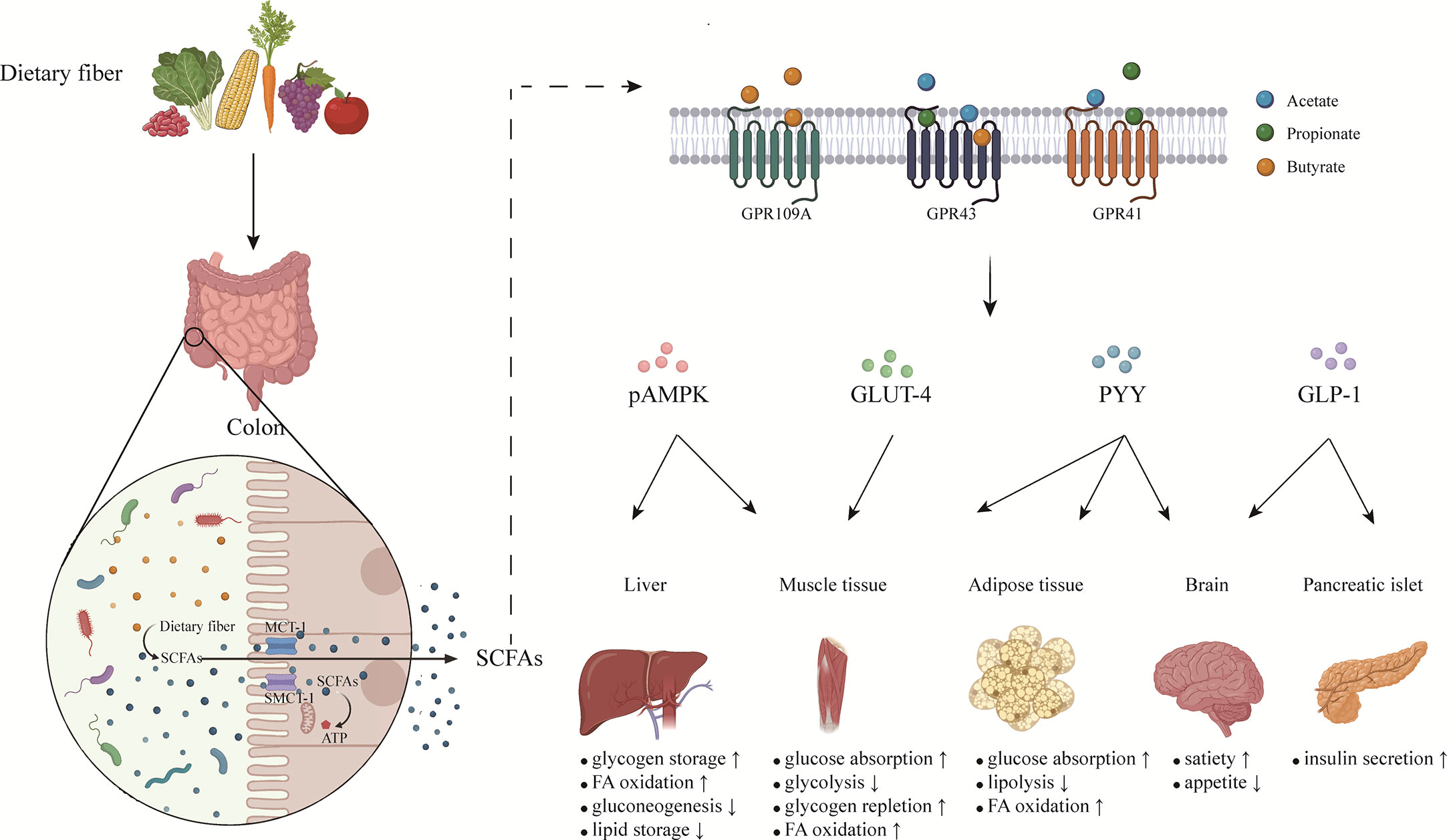
Figure 1 The effects of short chain fatty acids on metabolic homeostasis. SCFAs are produced by gut microbiota fermenting soluble dietary fiber in colon. Most SCFAs are absorbed by colonocytes via MCT-1 and SMCT-1, while small part of SCFAs pass directly through the intestinal barrier by passive diffusion. SCFAs transported to various organs of the whole body exert different functions mainly by binding with GPCRs on cells. By coordinating various organs and systems, SCFAs regulate energy balance and maintain metabolic homeostasis. SCFAs, short chain fatty acids; MCT-1, monocarboxylate transporter 1; SMCT-1, sodium-coupled monocarboxylate transporter 1; GLP-1, glucagon-like peptide-1; PYY, peptide tyrosine tyrosine; pAMPK, phosphorylated adenosine monophosphate activated protein kinase; GLUT-4, glucose transporter-4; FA, fatty acids.
T cells are vital cells in TIME, especially decreased infiltration or dysfunction of T cells leads to poor clinical results in many cancer treatments (53). Since naive T cells expressed without GPR41 and GPR43 at functional levels, SCFAs could directly affect the differentiation of naive T cells relying on HDACs inhibitor activity. In this way, SCFAs promoted immature CD4+ T cells to differentiate into different regulatory and effector T cells, this was up to different polarization conditions which refer to cytokine phenotype and immunological milieu. SCFAs induced the activation of mTOR-S6K and STAT3 involved in the production of cytokines necessary for T cell differentiation. Whatever the polarization conditions, SCFAs promoted interleukin (IL)-10 expression, but could only facilitate the production of IL-17 or interferon-γ (IFN-γ) under specific environments (54). Interestingly, the secretion of IL-10 in differentiated T cells, such as T helper (Th)1 cell, depended on interaction with GPR43. In addition, SCFAs upregulated the expression of Blimp-1, which was associated with IL-10 production in Th1 cells and T cell function maintenance (Figure 2) (55). In high-fat diet-treated mice, the levels of IL-17 and IFN-γ were increased, while transforming growth factor-β (TGF-β) and IL-10 decreased. That meant higher differentiation of T cells toward Th17 and Th1 cells and lesser to T regulatory (Treg) cells. This situation could be reversed by SCFAs (56).
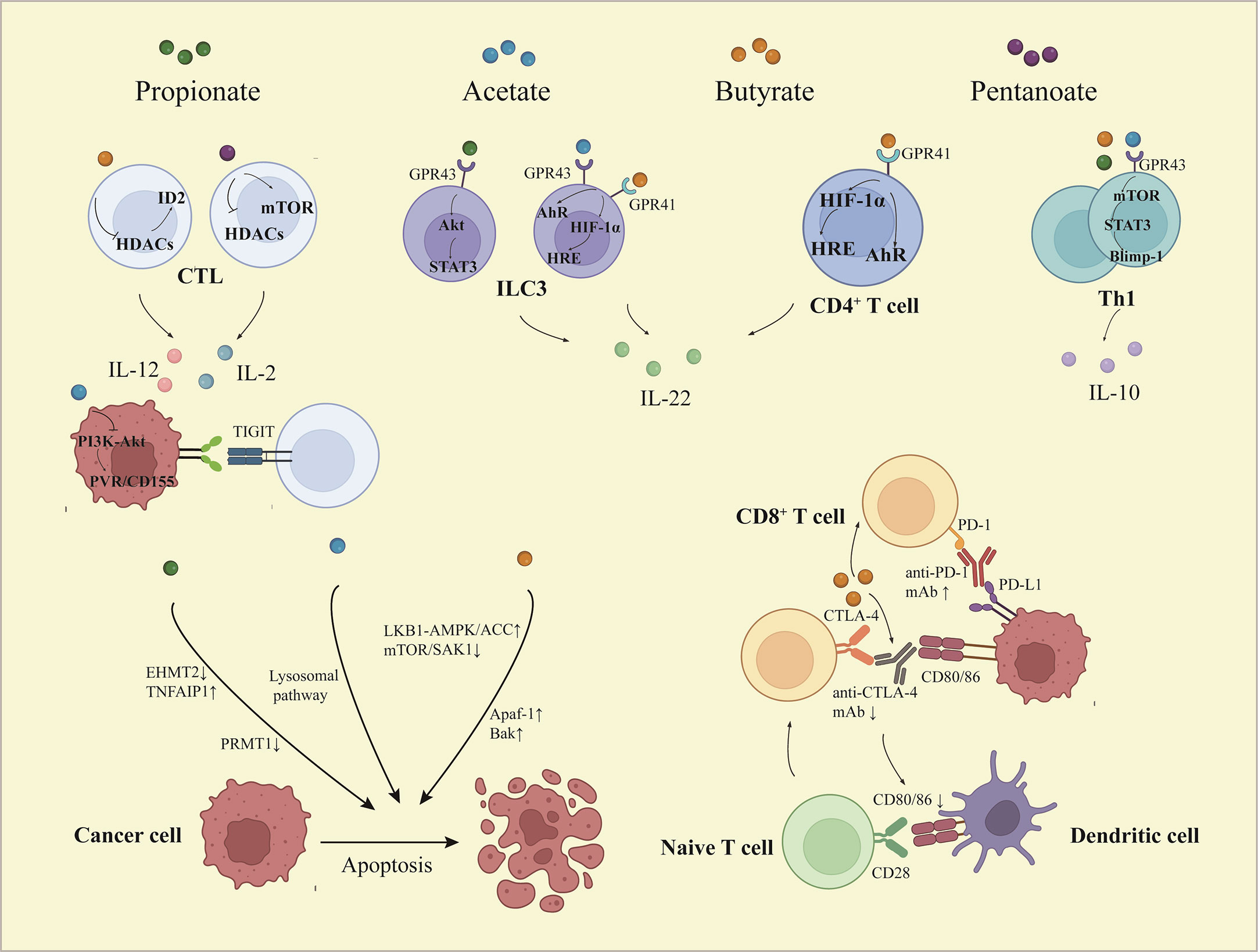
Figure 2 The effects of short chain fatty acids on tumor immune microenvironment. Depending on activation of STAT3 and mTOR, SCFAs promote Th1 cell expression of Blimp-1, which increases IL-10 expression. Butyrate and pentanoate promote the expression of IL-12 and IL-2 respectively in CD8+ T cells via HDACs inhibitory activity. Acetate decreases the expression of PVR/CD155 through inactivation of PI3K/AKT pathway, thus enhancing the response of CD8+ T cells. SCFAs induce IL-22 production in CD4+ T cells and ILCs, which is mediated by HIF-1α and AhR or involved by Stat3 and mTOR. Butyrate facilitates the anti-PD-1 mAb efficacy via regulating the T cell infiltration, while butyrate reduces efficacy of CTLA-4 blockade and limits DC maturation. Furthermore, SCFAs induce apoptosis of cancer cells through multiple ways. STAT3, signal transducer and activator of transcription 3; mTOR, mammalian target of rapamycin; Blimp-1, B lymphocyte-induced maturation protein 1; PI3K, phosphoinositide 3-kinase; AKT, the protein kinase B; PVR, poliovirus receptor; HRE, hypoxia response element; AhR, aryl hydrocarbon receptor; HIF-1α, hypoxia inducible factor-1α; IL, interleukin; Th, T helper; CTL, cytotoxic T lymphocyte; ILC, innate lymphocyte cell.
The discovery and investigation of IL-22 in CD4+ T cells and innate lymphoid cells could be modulated by SCFAs. Butyrate increased transcription factor HIF-1α and AhR expression through binding to GPR41, which was accompanied by STAT3 and mTOR activation. Meanwhile, butyrate-promoted HIF-1α combination with the HRE of the IL-22 promoter involved histone acetylation (Figure 2) (57). The process of CD4+ T cell differentiation is also subject to epigenetic regulation. Different CD4+ T cell subsets have specific transcription factors, such as T-bet for Th1 cells, RORγT for Th17 cells, and FOXP3 for Treg cells. Butyrate increased the expression of RORγT in differentiated Th17 cells by acetylating H4K16 but had no response to naive CD4+ T cells under the Th17 polarization condition (58).
Low butyrate concentration promoted the differentiation of CD4+ T cells to Foxp3+ Treg cells in a TGF-β1-dependent manner, while high butyrate concentration induced the T-bet expression and IFN-γ release no matter what conditions and subpopulations (59). This seemed to break our traditional understanding of the impact of SCFAs on mucosal immunity. More than that, exposure to high concentrations of SCFAs, especially butyrate, suppressed the proliferation and activation of CD4+ T cells and other CD4+ T cell subsets in the intestinal mucosa. This correlated strongly with histone acetylation and GPR43 activation (60).
Butyrate is involved in anti-tumor immunity by promoting CD8+ T cell effects. The promotional effect of cytotoxic T lymphocytes (CTLs) mediated anti-tumor responses treated with butyrate was dependent on a transcriptional regulator called ID2, whose level was much higher in CD8+ T cells in TIME. By inhibiting the activity of HDACs, butyrate induced ID2 expression and started the ID2-IL-12 signal pathway to improve the chemotherapy effect of oxaliplatin (61). Pentanoate enhanced the expression of IL-2, tumor necrosis factor-α (TNF-α), IFN-γ, and other effector molecules in CTLs via inhibition of HDAC activity (9). PVR/CD155 regulators are overexpressed in malignant tumors and bind to a T-cell immunoreceptor with Ig and ITIM domains to mediate immune escape. Acetate suppressed the level of PVR/CD155 by inhibiting PI3K/AKT pathway to enhance the anti-tumor ability of CD8+ T cells (Figure 2) (8). Activated γδ T cells are the main endogenous source of IL-17. Propionate repressed the secretion of IL-17, IL-22, and other cytokines in human γδ T cells by inhibiting HDACs, thereby preventing cancer progression (62).
3 SCFAs, gastrointestinal tumors, and tumor immunity
The abundance of SCFA and SCFA-producing bacteria in gastrointestinal tumors is significantly lower, and supplementation of SCFAs can inhibit the development of gastrointestinal tumors through a variety of mechanisms (Table 1).
3.1 Esophageal cancer
Research has shown that the destruction of mucosal barrier function is a high-risk factor for esophageal cancer. Propionate and butyrate treatment reversed the damaged esophageal epithelial barrier driven by IL-13. After treatment, the expression of barrier proteins FLG and DSG1 were significantly increased. HDAC antagonist occurred a similar effect, while GPCRs agonists did not, which means this function of SCFAs may be achieved by inhibiting HDAC activity (15). The alterations of gut microbiota composition in patients with esophageal squamous cell carcinoma could be observed, butyrate-producing bacteria decreased, while pro-inflammatory and carcinogenic bacteria increased (63). Additionally, clinical research showed that the concentrations of acetate and propionate in patients with complications after esophagectomy were significantly reduced, and preoperative supplementation of SCFAs may prevent infection and other complications (64).
3.2 Gastric cancer
Lower concentrations of propionate and butyrate were detected in the plasma of patients with gastrointestinal metaplasia or gastric cancer (65). It would appear that SCFAs could be used to evaluate the progression of gastric cancer. Besides, butyrate inhibited the proliferation and migration of the KATO III in a dose-dependent manner, which was associated with its effect on regulating microRNA (miRNA) regulatory networks (66). Taking Clostridium butyricum (C. butyricum) after gastrectomy could increase the concentrations of SCFAs, enhance immunity, reduce inflammation, and prevent postoperative complications (67). Vivo experiment demonstrated that acetate induced the apoptosis of gastric cancer cells, and subsequently, oxidative stress played an essential role has been proved in vitro. A large amount of acetate intake increased ROS production and MCT1 expression in gastric cancer cells (10). The over-expression of ROS upregulated HCP1, both of which resulted in the increase of porphyrin intake by gastric cancer cells. As a photosensitizer of photodynamic therapy, excessive absorption of porphyrin caused by acetate enhanced the efficacy (11). Epigenetic modifications may be one of the mechanisms that regulate the development of gastric cancer. Serving as an HDAC inhibitor, butyrate modified tumor suppressor genes Per1 and Per2 and induced their expression in the KATO III and NCI-N87 (22). The traditional remedy used in combination with SCFAs appeared to have better reactions and less toxicity. In the nude mouse xenograft tumor model, butyrate-cisplatin treatment suppressed the growth, migration, and invasion of gastric cancer cells and accelerated apoptosis relying on the mitochondrial apoptotic pathway (20). In addition, butyrate alone can induce apoptosis of gastric cancer cells by mitochondrial pathway, which has been confirmed in human cell lines BGC-823 and SGC-7901 (68).
3.3 Colorectal cancer
Previous studies revealed the concentrations of acetate, propionate, and butyrate in the population at high risk of CRC were significantly reduced, and the incidence of CRC was higher in individuals with lower SCFA levels than in healthy individuals (69). All kinds of SCFAs exhibited anti-cancer behaviors. Compared with treating alone, the compounds had a superposition effect (70).
3.3.1 Acetate
Acetate can enhance apoptosis and reduce proliferation in cancer cells and has been confirmed in different CRC cell lines (71), so it has become a key factor in the treatment of CRC, but the concrete mechanism remains unclear. In CRC patients, SCFAs were reduced and acetate metabolism was converted to acetyl-CoA (72). A past study showed that the acetate-mediated apoptosis was dependent on the lysosomal pathway triggered by partial lysosomal membrane permeabilization. However, the subsequent release of cathepsin D in the lysosome-dependent selective death pathway lowered the sensitivity of acetate. From these, cathepsin D inhibitors may be considered a good option combined with acetate (12). Appropriate regulation of mitochondrial function is essential for proliferation impeded by exogenous acetate in normoxic conditions. Specifically, acetate suppressed glycolysis and triggered ROS generation. However, increased proliferation of cancer cells with acetate occurred in the absence of oxygen, which relied on the up-regulation of ACSS2 and activation of HIF-2 (13). At the same time, acetate promoted the growth of HCT-116- and HT-29-derived tumors by activating the ACSS2/HIF-2 signaling pathway in the presence of glucose deficiency (73). At physiological concentrations, acetate promoted the growth of COLO 205 cells through activating AcK and enhancing oxidative phosphorylation and accelerated the proliferation of HCT-116 cells by elevating glycolysis. Nevertheless, butyrate or propionate drove apoptosis of two cell lines via the mitochondrial pathway at physiological doses (74). Thus, the anticancer effect of acetate can be altered in response to changes in environment and concentration. Moreover, acetate, as a regulator for immune checkpoint ligand PVR/CD155 driven by PI3K/AKT signaling, can enhance functional responses of CD8+ T cells in TIME and promote the production of IFN-γ, which is expected to become a related drug to promote tumor immunity. Based on the above discussion, the efficacy of ICIs may benefit from acetate (8).
3.3.2 Propionate
The content of propionate in colorectal cancer tissue decreased, and the addition of propionate to the SW480 cell line significantly inhibited growth (75). SCFAs regulated immune stimulatory and inhibitory ligands and were involved in the immune cell-mediated killing. The upregulation of NKG2D ligands MICA/B induced by propionate depends on neither inhibition of HDACs nor a combination of GPR41/GPR43 receptors, but mitochondrial activity, while butyrate is decided by its HDACs inhibitor activity. This effect of propionate was closely related to the PEPCK-M enzyme and mTORC2/PDK1/AKT pathway which mediate tumor suppressor protein p21 expression (17). In addition to immune immunoregulation, epigenetic modulation is a promising target for SCFAs to play anti-cancer roles. Propionate resulted in down-regulated expression of PRMT1 by preventing p70 S6 kinase phosphorylation, leading to selective death of CRC cells (18). Furthermore, propionate induced the HECTD2 upregulation resulting in the degradation of EHMT2, thus promoting the expression of downstream TNFAIP1 and ultimately apoptosis (19). Epigenetic modification is non-negligible in propionate-mediated anticancer treatments. However, the latest mendelian randomization analysis found that there was no strong evidence to prove the correlation between the concentration of propionate in feces and the risk of colorectal cancer (76). It may be necessary to comprehensively detect SCFAs and their producing bacteria.
3.3.3 Butyrate
It is universally acknowledged that butyrate inhibited the proliferation of CRC cells but nourished normal colon cell growth. CRC cells preferred glucose as substrate rather than butyrate, which was described as the butyrate paradox (77). That is, glycolysis replaced oxidative phosphorylation. Compared with other SCFAs, butyrate has a stronger inhibitory effect on CRC cell lines (78). On the one hand, butyrate can inhibit pro-inflammatory mediators TNF-α, IL-1β, IL-6, and IL-8, and up-regulate anti-inflammatory factor IL-10; on the other hand, butyrate can promote anti-tumor immunity by promoting CD8+T cells to play a role. In addition, butyrate maintains the integrity of the intestinal barrier by promoting epithelial cell proliferation, increasing the mucus layer, and improving tight junctions (79). In HCT116 cells, butyrate-mediated apoptosis was inseparable from p300-Wnt signaling (80). Among them, colon cancer cells were more sensitive to butyrate under the oncogenic Wnt signaling gene expression mode than the receptor-mediated Wnt signaling gene expression mode (81). The structure and stability of gut microbiota were significantly altered in CRC and intimately associated with its progression (82). Butyrate administration improved microecological disorders reflected in the decreased pathogens and the ratio of Firmicutes to Bacteroidetes and increased abundance of probiotics (83–87). In contrast to acetate and propionate, butyrate has a stronger effect on the regulatory networks which are essential to the cell cycle in CRC. Butyrate regulated the expression of cancer-related miRNA, of which miR-139 and miR-542 were well-known representatives. Specifically, they were conducted as collaborative objects of butyrate to modulate EIF4G2 and BIRC5 genes in the cell cycle (88). Butyrate regulated the c-Myc/p21 pathway to induce cell cycle arrest in the G2 phase, with the inclusion of 27 apoptosis-related genes (24). Moreover, butyrate triggered a cell cycle block at the G1 phase requiring a complicated lncRNA-miRNA-mRNA regulatory network (89). Butyrate supplementation reversed the overexpression of CSE1L and appeared to show synergy with p53, eventually arresting cancer cells at the G1 and G2/M phases (90). Meanwhile, cell cycle arrest in the G2/M phase occurred in response to butyrate-induced p21 and γ-H2AX increase, along with cyclin B1 decrease. Not only that but butyrate had an inhibitory effect on cancer cell migration by upregulating miR-200c and suppressing its direct target BMI-1 (25). BMI-1 was an essential regulator that induced epithelial-mesenchymal transition (EMT) dependent on AKT/GSK-3β/snail pathway to drive cancer metastasis, butyrate prevented the effect of BMI-1 (26). Treatment of butyrate decreased Trx-1 expression in CRC cells instead of normal colonocytes. Studies have proven that Trx-1 interaction with S100P promoted EMT through S100A4 upregulation which was mediated by AKT (33, 91, 92). With the administration of butyrate, organoids demonstrated that the extracellular matrix-integrin/PI3K-Akt axis was involved in CRC cell morphology variation and apoptosis (36).
Butyrate prevented migration and invasion of CRC cells, it was essentially due to the inhibition of HDAC3, which blocked the activation of AKT1 and ERK1/2 (29). Additionally, butyrate interacted with LHX1 to prevent HDAC8 which was up-regulated in tumor tissues. Opposite effects of butyrate on LHX1 mRNA expression occurred in HT-29 and HCT-116 cells despite inhibiting the proliferation of both cell lines (30, 31). Butyrate silenced SIRT-1 belonging to the HDAC family to deactivate mTOR/S6K1 signaling, thus attenuating growth and promoting apoptosis of HCT-116 cells (32). The binding site of IL-6 called gp130 was decreased and occupied by TRAFs which were upregulated by butyrate, leading to inhibition of the JAK2/STAT3 pathway beneficial for CRC (37). Distinguished from other SCFAs, butyrate reversed the excessive expression of prostaglandin EP4 receptors and the production of cyclooxygenase-2 to reduce phenotypic alteration from normal cells to cancer (43). Moreover, butyrate induced CRC cells autophagy through activation of the LKB1-AMPK/ACC signaling pathway and degradation of β-catenin (23, 34).
Attended to altering the epigenetics and metabolic spectrum of CRC cells, butyrate exerted its anti-cancer properties. By regulating the DNA methylation of KEAP1, butyrate blocked NRF2-ARE signaling to enhance its anticancer potential. The change in mitochondrial metabolism and related metabolites participated in the modulation of epigenetics by butyrate (27). Butyrate activated tricarboxylic acid cycle relevant enzymes IDH1 and PDH, thereby the level of downstream product α-KG increased. Considering as a signaling molecule, α-KG affected the demethylation of MSH2 and MLH1 related to apoptosis (93). Meanwhile, α-KG attenuated methylation of DNA and histone H3K4me3, resulting in the Wnt signaling pathway being suppressed in CRC (94). Research showed that enhanced glycolysis and reduced utilization of butyrate in CRC resulted from the decrease of pyruvate kinase M1 (95).
Warburg effect referred to the metabolic adaptation that increased glycolysis in cancer cells and could be obstructed by butyrate-involved metabolic modulation. The membrane content of GLUT1 and cytoplasmic level of G6PD were decreased in response to butyrate and contributed to decreased glucose absorption and utilization, and this process was dictated by the GPR109a-AKT pathway (39). Butyrate-induced inhibition of aerobic glycolysis via promoting tetramerization and dephosphorylation of PKM2, thus reversing metabolic dominance in cancer cells (28). For CRC cells, the metabolic change in response to butyrate was reflected in promoting oxidative metabolism rather than glycolysis (38). In addition, iron death is the way iron-dependent cells die programmatically, and butyrate induces iron death in CRC cells through the CD44/SLC7A11 signaling pathway (96).
3.4 Hepatocellular carcinoma
As a significant hazardous factor, Hepatitis B Virus (HBV) fosters the progression of hepatocellular carcinoma (HCC). Butyrate substantially suppressed the proliferation of Hep G2.2.15 cells and replication of resident HBV by inhibiting SIRT-1 and thereby promoting p53 acetylation (40). HBx, an oncogenic protein encoded by HBV, may lead to the accelerated occurrence and development of HCC in multiple ways. Along with the downregulation of HBx-related pathways, SCFAs resulted in the incremental expression of tumor suppressor DAB2, which suppressed RAS activity and thus delayed the progress of HCC (41). Previous studies indicated that butyrate inhibited the AKT/mTOR pathway by increasing ROS generation, thus contributing to apoptosis and autophagy of Huh 7 cells (97). The HCC mice intervened with fecal Lactobacillus reuteri transplantation appeared to postpone cancer progression. The related mechanism was that acetate produced by Lactobacillus reuteri metabolism inhibited the production of IL-17A, the effector molecule of group 3 innate lymphoid cells, through HDAC inhibition and induction of Sox acetylation. Separately, in combination with SCFAs, PD-1 inhibitors showed an enhanced antitumor effect in HCC mice (14). Lachnospiracea had the effect of reducing liver fibrosis, which was partly due to SCFAs mediated. It has been proved that oral SCFAs can inhibit fibrosis in mdr2−/− mice treated with vancomycin (98). Moreover, propionate may enhance the chemotherapeutic efficacy of conventional chemotherapeutic agents in HCC. Studies showed that propionate induced TNF-α expression by activating GPR41 and increased cisplatin-induced activation of caspase-3, thereby mediating HepG2 apoptosis (99). The 16s RNA expression of butyrate-producing bacteria in HCC patients decreased, and butyrate supplementation could promote apoptosis and inhibit proliferation in HepG2 cells. More than that, butyrate may enhance the therapeutic potential of sorafenib which has shown therapeutic efficacy by targeting miR-7641 and miR-199, whose expression could be reduced with butyrate treatment (42). By reducing HK2 dependent on c-myc signaling, butyrate resisted glycolysis to enhance the efficacy of sorafenib (44). For HCC patients treated with lenvatinib, the metabolism of butyrate in patients without diarrhea and other adverse reactions was relatively rich and active (100). The latest research indicated that acetate supplementation could induce the level of NAT2 in HepG2 cells, similar to glucose and insulin which led to changes in metabolism-related genes (101). Not only that, butyrate showed the same anti-cancer effect in cholangiocarcinoma cells. Butyrate and HDAC6 inhibitors have synergistic effects on preventing proliferation, migration, and EMT (45).
Alcoholic fatty liver disease (AFLD) and nonalcoholic fatty liver disease (NAFLD) can progress to cirrhosis, eventually turning into liver cancer. Butyrate inhibited gasdermin D-mediated pyroptosis to improve intestinal barrier disruption and endotoxemia, thereby attenuating hepatic steatosis and inflammation in AFLD (102). Moreover, butyrate induced alteration of the hepatic lipid profile and alleviated hepatic steatosis to treat NAFLD relying on the regulation of the LKB1-AMPK-Insig signaling pathway (103). Taken together, SCFAs restricted the evolution of HCC in the premalignant stages.
3.5 Pancreatic cancer
It is found that the levels of propionate and butyrate were reduced and the composition of fecal microbiota was altered in patients with pancreatic ductal adenocarcinoma compared to controls (104, 105). Targeting tumor-specific immune cells, SCFAs showed strong anti-cancer effects either in isolation or in combination with other tumor remedies. It was shown that associated with HDAC inhibition, butyrate and pentanoate upregulated the production of IL-2, CD25, and mTOR, which were involved in the regulation of T cell activation. With triggering augmented effector molecules, butyrate and pentanoate also increased the tumor-killing capacity of CTLs. In addition, SCFA also shows excellent prospects in adoptive immune therapy. ROR1-CAR T cells pretreated with butyrate or pentanoate showed a better therapeutic effect in the pancreatic cancer mouse model (9). Another study showed that butyrate delayed the development of carcinoma by reversing the immunosuppressive function of CD11b cells and enhancing the immune function of CD8+T cells in pancreatic ductal adenocarcinoma patients (46). The influence of SCFAs was not only limited to TIME but also extended to tumor-associated genes. After the treatment of butyrate, the upregulation of p16INK4a, p14ARF, and p15INK4b mediated by inhibiting HDACs activities can be observed in AsPC-1 (47). In BxPC-3 and PANC-1 cell lines, butyrate inhibited proliferation and induced apoptosis, either alone or in combination with gemcitabine. Especially, combined medication alleviated gastrointestinal mucosa, liver, and kidney damage caused by gemcitabine. Because of the inhibition of HDACs, butyrate also modulated TIME-related components (49). SCFAs can regulate multiple signal pathways to interfere with the progress of pancreatic cancer, and may also be used as early cancer predictive markers (106).
4 Targeting microbiota-SCFAs axis for treatments of gastrointestinal tumors
Probiotics can fight against gastrointestinal tumors by increasing the abundance of gut microbiota, regulating the activities of some enzymes that contribute to the production of carcinogenic compounds and improving the intestinal barrier (107). 23 randomized controlled trials indicated that diverse probiotics supplementation ameliorated symptoms and improved life quality, as well as reduce the adverse reactions of traditional treatment of patients with CRC (108). Certainly, increasing the production of SCFAs is a pivotal way. Screening potential probiotic Streptococcus salivarius from human colostrum can inhibit the proliferation of CRC cells by more than 55%. Streptococcus salivarius adhered directly and induced apoptosis in cancer cells, promoted the production of SCFAs, and regulated the activated B and T lymphocytes (109). C. butyricum restrained the progression of gastrointestinal tumors relying on butyrate. C. butyricum modulated Wnt/β-catenin signaling in Apcmin/+ mice to reduce high-fat diet-induced CRC, suppressed colitis-associated colon cancer via inhibiting the NF-κB pathway, and enhanced the ICIs curative effect on lung cancer (85, 110, 111). Propionibacterium freudenreichii induced the intrinsic apoptosis of CRC cells by producing propionate and acetate which act on mitochondria (112). Pediococcus acidilactici UAMS, as a high butyrate-producing bacterium, was isolated from bhaati jaanr and inhibited the proliferation of HT29 and SW480 (113). Roseburia intestinalis, Faecalibacterium prausnitzii, Lactiplantibacillus plantarum, and Eubacterium callanderi have also been proven to enhance the anti-cancer immune response in CRC accompanied by butyrate production (114–117). Lactic acid-producing bacteria played a major role in controlling intestinal carcinogenesis because of SCFAs synthesis (118). Treatment with VSL#3 probiotics increased the levels of propionate and butyrate, resulting in recruiting Th17 cells via the CCL20/CCR6 axis to attenuate lung metastasis of melanoma (119). Lactobacillus rhamnosus GG ATCC 53103, Limosilactobacillus reuteri DSM 17938, Lactobacillus johnsonii LC1 and other probiotics not only inhibited CRC cells proliferation but also improved chemotherapy responsiveness (120). Therefore, supplementation of probiotics can increase SCFAs to a certain extent, and then play an essential role in cancer prevention and management.
As the precursor of SCFAs, dietary fiber is one of the representative prebiotics. Dietary fiber is a kind of nutrient that naturally exists in plants and cannot be digested and absorbed by the human intestinal tract. It can reduce the incidence rate and death risk of cardiovascular disease, cholelithiasis, diabetes, cancer, and other diseases. Research has corroborated that taking more dietary fiber decreased the risk of multiple diseases including CRC. There is a statistically significant and strong correlation between dietary fiber consumption and the chance of developing colorectal adenoma and CRC (121–123). As a representative dietary fiber, pectin significantly increased the diversity of gut microbiota, especially butyrate-producing bacteria, and promoted T cell infiltration in TIME, thus enhancing the anti-programmed death-1 (anti-PD-1) monoclonal antibody (mAb) effect (124). Omega-3 polyunsaturated fatty acids (PUFAs) that cannot be synthesized by the human body and must be ingested from food, have been proven to improve hyperlipidemia, coronary heart disease, and atherosclerosis. The increase of butyrate-producing bacteria abundance could be observed when daily taking more PUFAs. Meanwhile, probiotics Lactobacillus increased while Fusobacterium nucleatum decreased. Studies have shown that some PUFAs can assist chemotherapeutic drugs 5-FU and oxaliplatin for colorectal cancer and reduce side effects (125, 126).
In addition to supplementing probiotics, prebiotics, and synbiotics, FMT may also be an option to increase SCFAs and resist gastrointestinal tumors. In carcinogen-inducing conventional mice or germ-free mice, FMT from patients with CRC reduced gut microbiota richness and promoted gastrointestinal tumor formation (127). Treatment of dextran sulfate sodium or azoxymethane can induce CRC in laboratory mice, and FMT from wild mice to experimental mice could improve this process (128). Although there was no clinical evidence that FMT can directly treat CRC, a study suggested that FMT can help improve the efficacy of chemotherapy. Transplanting the feces of healthy donor mice into FOLFOX-treated mice restored the composition of fecal intestinal microbiota destroyed after FOLFOX treatment and reduced the severity of diarrhea and intestinal mucosal inflammation (129). Furthermore, FMT confirmed the auxiliary effect of pectin on anti-PD-1 mAb (124). FMT enhanced the anti-PD-1 therapy efficacy by increasing the diversity of microbiota and modulating immune function (130). FMT is the most direct way to shape the microbiome, the direct increase of SCFAs-producing bacteria through FMT provides a broad prospect for the treatment of gastrointestinal cancer.
The concentrations of SCFAs and the abundance of SCFAs-producing bacteria in patients who responded to anti-PD-1 immunotherapy were significantly higher than those in non-responders (131). Most recently, a cohort study has confirmed that SCFAs assumed potential biomarkers for identifying solid tumor patients who may benefit from PD-1 inhibitors (Nivolumab or Pembrolizumab) treatment (132). Although ICIs have been demonstrated in clinical practice with great success, it is accompanied by a wide range of adverse reactions, among which cardiotoxicity is the deadliest. Supplementation of butyrate and recolonization of Prevotella loescheii relieved the cardiotoxicity and gut microbiota dysbiosis induced by BMS-1 (133). Nevertheless, the extent to which SCFAs affect ICIs has remained controversial. A high concentration of blood butyrate and propionate reduced the anti-cytotoxic T lymphocyte-associated antigen-4 (CTLA-4) efficacy whether in mice models or in patients. By restraining dendritic cell maturation and T cell accumulation in TIME, SCFAs limited the therapeutic effect of anti-CTLA-4 mAb (Figure 2) (134).
In addition to immunotherapy, SCFAs also modulate tumor responsiveness to radiochemotherapy and immunotherapy. Clinical research found that after preoperative neoadjuvant radiochemotherapy, responder CRC patients show more enriched levels of butyrate-producing bacteria and SCFAs in the feces than those who did not (135). Butyrate can be used as the synergist of oxaliplatin to synergistically enhance the anti-cancer effect (136). Butyrate directly facilitated the chemotherapy efficacy of oxaliplatin by regulating CD8+ T cells. In addition, the level of butyrate was high in the serum of responder patients compared to non-responder patients in CRC (61). Compared with radiation administration alone, the radiation-butyrate combination significantly enhanced the anti-cancer effect. Butyrate could induce cell cycle arrest by promoting FOXO3A-mediated transcription, alongside protecting normal cells from radiation damage. Since HDACs inhibitors have been proven to enhance the sensitivity of radiotherapy, it may be speculated that butyrate enhanced the efficacy of radiotherapy because of the inhibition of HDACs. Butyrate also enhanced the therapeutic effect of 5-FU on CRC by suppressing Warburg Effect, this process was due to the activation of GPCRs (137). Butyrate could also enhance the efficacy of 5-FU through the GPR109a-AKT signal pathway (39). Meanwhile, SCFAs impaired the pro-inflammatory effect of 5-FU and increased the expression of TJ protein in the mucosa (138). However, abnormal activity of butyrate-producing bacteria and excessive butyrate in patients induced resistance to chemotherapeutics. It is demonstrated that CRC cell lines that were resistant to butyrate showed obvious chemoresistance (139). More clinical trials are being explored (Table 2).
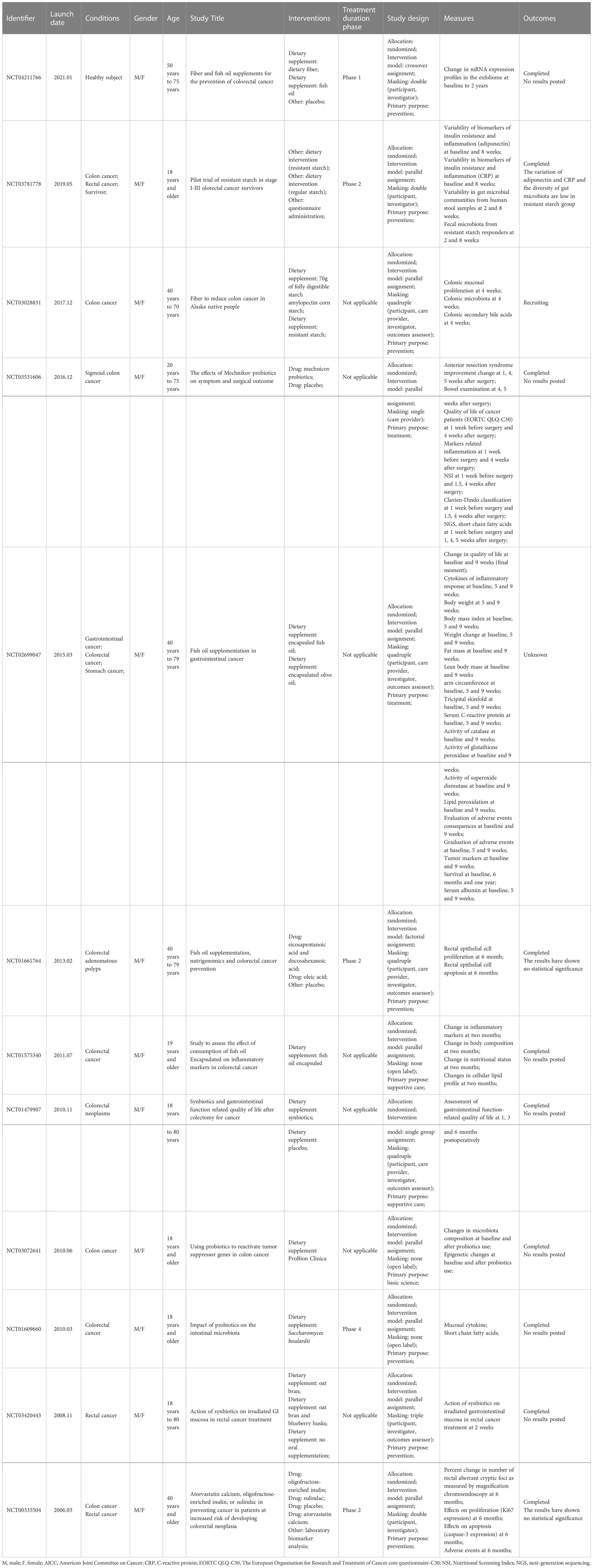
Table 2 Approved clinical trials explore the effects of short-chain fatty acids on the treatment and prognosis of patients with colorectal cancer (Data sources: ClinicalTrials).
5 Conclusions and perspectives
Immune cells and molecules have become highlights of tumor treatment. ICIs, established in TIME, targeted kill tumor cells by regulating autoimmune cells and immune molecules while reducing damage to normal tissues, and have become the representative of tumor immunotherapy. Gut microbiota and its metabolites affect the sensitivity and responsiveness of the host to anti-cancer therapy. SCFAs serve as decisive products of gut microbiota and have been proven to inhibit the proliferation, invasion, and migration and induce apoptosis of gastrointestinal tumor cells. The latest findings support the potential of SCFAs in immunotherapy. SCFAs can modify the differentiation and function of immune cells as well as the production and release of cytokines, and control tumor growth and metastasis by multiple signal pathways. In addition, SCFAs can also help to improve the therapeutic effect of radiotherapy and chemotherapy and reduce adverse reactions. However, clinical trials are relatively insufficient at present, and the polymicrobial combination leads to large individual differences in therapeutic effects. The supplement of specific foods and probiotics can assist complex anti-tumor treatment, enhance the curative effect and improve the prognosis. FMT is the most direct transformation method of microbial composition, which may become a new part of complex tumor treatment in the future.
Author contributions
YD collected literature, drafted the manuscript, and prepared figures. KXZ modified figures and edited the manuscript. JGW, XW, and YYD collected data, and designed and made tables. HQH and JYW provided critical feedback and helped shape the manuscript. TYL, BMW, and HLC reviewed the manuscript and provided funding acquisition. All authors contributed to the article and approved the submitted version.
Funding
This research is supported by the grants (82070545, 82100574 and 81970477) from the National Natural Science Foundation of China, and the Key Project of Science and Technology Pillar Program of Tianjin (20YFZCSY00020).
Acknowledgments
The authors salute all the researchers and patients involved in the clinical trial.
Conflict of interest
The authors declare that the research was conducted in the absence of any commercial or financial relationships that could be construed as a potential conflict of interest.
Publisher’s note
All claims expressed in this article are solely those of the authors and do not necessarily represent those of their affiliated organizations, or those of the publisher, the editors and the reviewers. Any product that may be evaluated in this article, or claim that may be made by its manufacturer, is not guaranteed or endorsed by the publisher.
Glossary
References
1. Sung H, Ferlay J, Siegel RL, Laversanne M, Soerjomataram I, Jemal A, et al. Global cancer statistics 2020: GLOBOCAN estimates of incidence and mortality worldwide for 36 cancers in 185 countries. CA Cancer J Clin (2021) 71(3):209–49. doi: 10.3322/caac.21660
2. Hanus M, Parada-Venegas D, Landskron G, Wielandt AM, Hurtado C, Alvarez K, et al. Immune system, microbiota, and microbial metabolites: The unresolved triad in colorectal cancer microenvironment. Front Immunol (2021) 12:612826. doi: 10.3389/fimmu.2021.612826
3. Parada Venegas D, de la Fuente MK, Landskron G, González MJ, Quera R, Dijkstra G, et al. Short chain fatty acids (SCFAs)-mediated gut epithelial and immune regulation and its relevance for inflammatory bowel diseases. Front Immunol (2019) 10:277. doi: 10.3389/fimmu.2019.00277
4. Aoe S, Yamanaka C, Fuwa M, Tamiya T, Nakayama Y, Miyoshi T, et al. Effects of BARLEYmax and high-β-glucan barley line on short-chain fatty acids production and microbiota from the cecum to the distal colon in rats. PLoS One (2019) 14(6):e0218118. doi: 10.1371/journal.pone.0218118
5. Pansy K, Uhl B, Krstic J, Szmyra M, Fechter K, Santiso A, et al. Immune regulatory processes of the tumor microenvironment under malignant conditions. Int J Mol Sci (2021) 22(24):13311. doi: 10.3390/ijms222413311
6. Reck M, Rodríguez-Abreu D, Robinson AG, Hui R, Csőszi T, Fülöp A, et al. Five-year outcomes with pembrolizumab versus chemotherapy for metastatic non-Small-Cell lung cancer with PD-L1 tumor proportion score ≥ 50. J Clin Oncol (2021) 39(21):2339–49. doi: 10.1200/jco.21.00174
7. Emens LA, Molinero L, Loi S, Rugo HS, Schneeweiss A, Diéras V, et al. Atezolizumab and nab-paclitaxel in advanced triple-negative breast cancer: Biomarker evaluation of the IMpassion130 study. J Natl Cancer Inst (2021) 113(8):1005–16. doi: 10.1093/jnci/djab004
8. Tran NL, Lee IK, Choi J, Kim SH, Oh SJ. Acetate decreases PVR/CD155 expression via PI3K/AKT pathway in cancer cells. BMB Rep (2021) 54(8):431–6. doi: 10.5483/BMBRep.2021.54.8.060
9. Luu M, Riester Z, Baldrich A, Reichardt N, Yuille S, Busetti A, et al. Microbial short-chain fatty acids modulate CD8(+) T cell responses and improve adoptive immunotherapy for cancer. Nat Commun (2021) 12(1):4077. doi: 10.1038/s41467-021-24331-1
10. Terasaki M, Ito H, Kurokawa H, Tamura M, Okabe S, Matsui H, et al. Acetic acid is an oxidative stressor in gastric cancer cells. J Clin Biochem Nutr (2018) 63(1):36–41. doi: 10.3164/jcbn.17-49
11. Kurokawa H, Ito H, Matano D, Terasaki M, Matsui H. Acetic acid enhances the effect of photodynamic therapy in gastric cancer cells via the production of reactive oxygen species. J Clin Biochem Nutr (2022) 71(3):206–11. doi: 10.3164/jcbn.22-34
12. Marques C, Oliveira CS, Alves S, Chaves SR, Coutinho OP, Corte-Real M, et al. Acetate-induced apoptosis in colorectal carcinoma cells involves lysosomal membrane permeabilization and cathepsin d release. Cell Death Dis (2013) 4:e507. doi: 10.1038/cddis.2013.29
13. Sahuri-Arisoylu M, Mould RR, Shinjyo N, Bligh SWA, Nunn AVW, Guy GW, et al. Acetate induces growth arrest in colon cancer cells through modulation of mitochondrial function. Front Nutr (2021) 8:588466. doi: 10.3389/fnut.2021.588466
14. Hu C, Xu B, Wang X, Wan WH, Lu J, Kong D, et al. Gut microbiota-derived short-chain fatty acids regulate group 3 innate lymphoid cells in HCC. Hepatology (2022) 77(1):48–64. doi: 10.1002/hep.32449
15. Kleuskens MTA, Haasnoot ML, Herpers BM, Ampting M, Bredenoord AJ, Garssen J, et al. Butyrate and propionate restore interleukin 13-compromised esophageal epithelial barrier function. Allergy (2022) 77(5):1510–21. doi: 10.1111/all.15069
16. Matthews GM, Howarth GS, Butler RN. Short-chain fatty acid modulation of apoptosis in the kato III human gastric carcinoma cell line. Cancer Biol Ther (2007) 6(7):1051–7. doi: 10.4161/cbt.6.7.4318
17. Hogh RI, Moller SH, Jepsen SD, Mellergaard M, Lund A, Pejtersen M, et al. Metabolism of short-chain fatty acid propionate induces surface expression of NKG2D ligands on cancer cells. FASEB J (2020) 34(11):15531–46. doi: 10.1096/fj.202000162R
18. Ryu TY, Kim K, Son MY, Min JK, Kim J, Han TS, et al. Downregulation of PRMT1, a histone arginine methyltransferase, by sodium propionate induces cell apoptosis in colon cancer. Oncol Rep (2019) 41(3):1691–9. doi: 10.3892/or.2018.6938
19. Ryu TY, Kim K, Han TS, Lee MO, Lee J, Choi J, et al. Human gut-microbiome-derived propionate coordinates proteasomal degradation via HECTD2 upregulation to target EHMT2 in colorectal cancer. ISME J (2022) 16(5):1205–21. doi: 10.1038/s41396-021-01119-1
20. Li Y, He P, Liu Y, Qi M, Dong W. Combining sodium butyrate with cisplatin increases the apoptosis of gastric cancer In vivo and In vitro via the mitochondrial apoptosis pathway. Front Pharmacol (2021) 12:708093. doi: 10.3389/fphar.2021.708093
21. Abbati G, Incerti F, Boarini C, Pileri F, Bocchi D, Ventura P, et al. Safety and efficacy of sucrosomial iron in inflammatory bowel disease patients with iron deficiency anemia. Intern Emerg Med (2019) 14(3):423–31. doi: 10.1007/s11739-018-1993-9
22. Hernandez-Rosas F, Hernandez-Oliveras A, Flores-Peredo L, Rodriguez G, Zarain-Herzberg A, Caba M, et al. Histone deacetylase inhibitors induce the expression of tumor suppressor genes Per1 and Per2 in human gastric cancer cells. Oncol Lett (2018) 16(2):1981–90. doi: 10.3892/ol.2018.8851
23. Luo S, Li Z, Mao L, Chen S, Sun S. Sodium butyrate induces autophagy in colorectal cancer cells through LKB1/AMPK signaling. J Physiol Biochem (2019) 75(1):53–63. doi: 10.1007/s13105-018-0651-z
24. Zeng H, Hamlin SK, Safratowich BD, Cheng WH, Johnson LK. Superior inhibitory efficacy of butyrate over propionate and acetate against human colon cancer cell proliferation via cell cycle arrest and apoptosis: linking dietary fiber to cancer prevention. Nutr Res (2020) 83:63–72. doi: 10.1016/j.nutres.2020.08.009
25. Xu Z, Tao J, Chen P, Chen L, Sharma S, Wang G, et al. Sodium butyrate inhibits colorectal cancer cell migration by downregulating bmi-1 through enhanced miR-200c expression. Mol Nutr Food Res (2018) 62(6):e1700844. doi: 10.1002/mnfr.201700844
26. Xu Z, Zhou Z, Zhang J, Xuan F, Fan M, Zhou D, et al. Targeting BMI-1-mediated epithelial-mesenchymal transition to inhibit colorectal cancer liver metastasis. Acta Pharm Sin B (2021) 11(5):1274–85. doi: 10.1016/j.apsb.2020.11.018
27. Wang L, Shannar AAF, Wu R, Chou P, Sarwar MS, Kuo HC, et al. Butyrate drives metabolic rewiring and epigenetic reprogramming in human colon cancer cells. Mol Nutr Food Res (2022) 66(12):e2200028. doi: 10.1002/mnfr.202200028
28. Li Q, Cao L, Tian Y, Zhang P, Ding C, Lu W, et al. Butyrate suppresses the proliferation of colorectal cancer cells via targeting pyruvate kinase M2 and metabolic reprogramming. Mol Cell Proteomics (2018) 17(8):1531–45. doi: 10.1074/mcp.RA118.000752
29. Li Q, Ding C, Meng T, Lu W, Liu W, Hao H, et al. Butyrate suppresses motility of colorectal cancer cells via deactivating Akt/ERK signaling in histone deacetylase dependent manner. J Pharmacol Sci (2017) 135(4):148–55. doi: 10.1016/j.jphs.2017.11.004
30. Forouzesh F, Ghiaghi M, Rahimi H. Effect of sodium butyrate on HDAC8 mRNA expression in colorectal cancer cell lines and molecular docking study of LHX1 - sodium butyrate interaction. Excli J (2020) 19:1038–51. doi: 10.17179/excli2020-2010
31. Ghiaghi M, Forouzesh F, Rahimi H. Effect of sodium butyrate on LHX1 mRNA expression as a transcription factor of HDAC8 in human colorectal cancer cell lines. Avicenna J Med Biotechnol (2019) 11(4):317–24.
32. Cao M, Zhang Z, Han S, Lu X. Butyrate inhibits the proliferation and induces the apoptosis of colorectal cancer HCT116 cells via the deactivation of mTOR/S6K1 signaling mediated partly by SIRT1 downregulation. Mol Med Rep (2019) 19(5):3941–7. doi: 10.3892/mmr.2019.10002
33. Lin F, Zhang P, Zuo Z, Wang F, Bi R, Shang W, et al. Thioredoxin-1 promotes colorectal cancer invasion and metastasis through crosstalk with S100P. Cancer Lett (2017) 401:1–10. doi: 10.1016/j.canlet.2017.04.036
34. Garavaglia B, Vallino L, Ferraresi A, Esposito A, Salwa A, Vidoni C, et al. Butyrate inhibits colorectal cancer cell proliferation through autophagy degradation of β-catenin regardless of APC and β-catenin mutational status. Biomedicines (2022) 10(5):1131. doi: 10.3390/biomedicines10051131
35. Zhou Y, Ji X, Chen J, Fu Y, Huang J, Guo R, et al. Short-chain fatty acid butyrate: A novel shield against chronic gastric ulcer. Exp Ther Med (2021) 21(4):329. doi: 10.3892/etm.2021.9760
36. Li F, Wu Y, Yan Y, Wu S, Zhu J, Zhang G, et al. Transcriptomic landscape of sodium butyrate-induced growth inhibition of human colorectal cancer organoids. Mol Omics (2022) 18(8):754–64. doi: 10.1039/d2mo00127f
37. Yuan Y, Li B, Kuang Y, Ni S, Zhuge A, Yang J, et al. The fiber metabolite butyrate reduces gp130 by targeting TRAF5 in colorectal cancer cells. Cancer Cell Int (2020) 20:212. doi: 10.1186/s12935-020-01305-9
38. Klepinina L, Klepinin A, Truu L, Chekulayev V, Vija H, Kuus K, et al. Colon cancer cell differentiation by sodium butyrate modulates metabolic plasticity of caco-2 cells via alteration of phosphotransfer network. PLoS One (2021) 16(1):e0245348. doi: 10.1371/journal.pone.0245348
39. Geng HW, Yin FY, Zhang ZF, Gong X, Yang Y. Butyrate suppresses glucose metabolism of colorectal cancer cells via GPR109a-AKT signaling pathway and enhances chemotherapy. Front Mol Biosci (2021) 8:634874. doi: 10.3389/fmolb.2021.634874
40. Pant K, Mishra AK, Pradhan SM, Nayak B, Das P, Shalimar D, et al. Butyrate inhibits HBV replication and HBV-induced hepatoma cell proliferation via modulating SIRT-1/Ac-p53 regulatory axis. Mol Carcinog (2019) 58(4):524–32. doi: 10.1002/mc.22946
41. McBrearty N, Arzumanyan A, Bichenkov E, Merali S, Merali C, Feitelson M. Short chain fatty acids delay the development of hepatocellular carcinoma in HBx transgenic mice. Neoplasia (2021) 23(5):529–38. doi: 10.1016/j.neo.2021.04.004
42. Kumar M, Kaur R, Kanthaje S, Dhiman RK, Chakraborti A. Bacterial metabolite butyrate in modulating sorafenib-targeted microRNAs to curtail its resistance in hepatocellular carcinoma. J Cancer Res Clin Oncol (2022) 30. doi: 10.1007/s00432-022-04544-7
43. Kurata N, Tokashiki N, Fukushima K, Misao T, Hasuoka N, Kitagawa K, et al. Short chain fatty acid butyrate uptake reduces expressions of prostanoid EP4 receptors and their mediation of cyclooxygenase-2 induction in HCA-7 human colon cancer cells. Eur J Pharmacol (2019) 853:308–15. doi: 10.1016/j.ejphar.2019.04.014
44. Yu Q, Dai W, Ji J, Wu L, Feng J, Li J, et al. Sodium butyrate inhibits aerobic glycolysis of hepatocellular carcinoma cells via the c-myc/hexokinase 2 pathway. J Cell Mol Med (2022) 26(10):3031–45. doi: 10.1111/jcmm.17322
45. Pant K, Richard S, Gradilone SA. Short-chain fatty acid butyrate induces cilia formation and potentiates the effects of HDAC6 inhibitors in cholangiocarcinoma cells. Front Cell Dev Biol (2021) 9:809382. doi: 10.3389/fcell.2021.809382
46. Pan P, Zhu Z, Oshima K, Aldakkak M, Tsai S, Huang YW, et al. Black raspberries suppress pancreatic cancer through modulation of NKp46(+), CD8(+), and CD11b(+) immune cells. Food Front (2020) 1(1):70–82. doi: 10.1002/fft2.1
47. Sanaei M, Kavoosi F. Effect of sodium butyrate on p16INK4a, p14ARF, p15INK4b, class I HDACs (HDACs 1, 2, 3) class II HDACs (HDACs 4, 5, 6), cell growth inhibition and apoptosis induction in pancreatic cancer AsPC-1 and colon cancer HCT-116 cell lines. Asian Pac J Cancer Prev (2022) 23(3):795–802. doi: 10.31557/APJCP.2022.23.3.795
48. Yuille S, Reichardt N, Panda S, Dunbar H, Mulder IE. Human gut bacteria as potent class I histone deacetylase inhibitors in vitro through production of butyric acid and valeric acid. PLoS One (2018) 13(7):e0201073. doi: 10.1371/journal.pone.0201073
49. Panebianco C, Villani A, Pisati F, Orsenigo F, Ulaszewska M, Latiano TP, et al. Butyrate, a postbiotic of intestinal bacteria, affects pancreatic cancer and gemcitabine response in in vitro and in vivo models. BioMed Pharmacother (2022) 151:113163. doi: 10.1016/j.biopha.2022.113163
50. Gomes SD, Oliveira CS, Azevedo-Silva J, Casanova MR, Barreto J, Pereira H, et al. The role of diet related short-chain fatty acids in colorectal cancer metabolism and survival: Prevention and therapeutic implications. Curr Med Chem (2020) 27(24):4087–108. doi: 10.2174/0929867325666180530102050
51. Dang F, Wei W. Targeting the acetylation signaling pathway in cancer therapy. Semin Cancer Biol (2021) 85:209–18. doi: 10.1016/j.semcancer.2021.03.001
52. Hou H, Chen D, Zhang K, Zhang W, Liu T, Wang S, et al. Gut microbiota-derived short-chain fatty acids and colorectal cancer: Ready for clinical translation? Cancer Lett (2022) 526:225–35. doi: 10.1016/j.canlet.2021.11.027
53. Philip M, Schietinger A. CD8(+) T cell differentiation and dysfunction in cancer. Nat Rev Immunol (2022) 22(4):209–23. doi: 10.1038/s41577-021-00574-3
54. Park J, Kim M, Kang SG, Jannasch AH, Cooper B, Patterson J, et al. Short-chain fatty acids induce both effector and regulatory T cells by suppression of histone deacetylases and regulation of the mTOR-S6K pathway. Mucosal Immunol (2015) 8(1):80–93. doi: 10.1038/mi.2014.44
55. Sun M, Wu W, Chen L, Yang W, Huang X, Ma C, et al. et al: Microbiota-derived short-chain fatty acids promote Th1 cell IL-10 production to maintain intestinal homeostasis. Nat Commun (2018) 9(1):3555. doi: 10.1038/s41467-018-05901-2
56. Mandaliya DK, Patel S, Seshadri S. The combinatorial effect of acetate and propionate on high-fat diet induced diabetic inflammation or metaflammation and T cell polarization. Inflammation (2021) 44(1):68–79. doi: 10.1007/s10753-020-01309-7
57. Yang W, Yu T, Huang X, Bilotta AJ, Xu L, Lu Y, et al. Intestinal microbiota-derived short-chain fatty acids regulation of immune cell IL-22 production and gut immunity. Nat Commun (2020) 11(1):4457. doi: 10.1038/s41467-020-18262-6
58. Sałkowska A, Karaś K, Walczak-Drzewiecka A, Dastych J, Ratajewski M. Differentiation stage-specific effect of histone deacetylase inhibitors on the expression of RORγT in human lymphocytes. J Leukoc Biol (2017) 102(6):1487–95. doi: 10.1189/jlb.6A0617-217R
59. Kespohl M, Vachharajani N, Luu M, Harb H, Pautz S, Wolff S, et al. et al: The microbial metabolite butyrate induces expression of Th1-associated factors in CD4(+) T cells. Front Immunol (2017) 8:1036. doi: 10.3389/fimmu.2017.01036
60. Kibbie JJ, Dillon SM, Thompson TA, Purba CM, McCarter MD, Wilson CC. Butyrate directly decreases human gut lamina propria CD4 T cell function through histone deacetylase (HDAC) inhibition and GPR43 signaling. Immunobiology (2021) 226(5):152126. doi: 10.1016/j.imbio.2021.152126
61. He Y, Fu L, Li Y, Wang W, Gong M, Zhang J, et al. Gut microbial metabolites facilitate anticancer therapy efficacy by modulating cytotoxic CD8(+) T cell immunity. Cell Metab (2021) 33(5):988–1000.e1007. doi: 10.1016/j.cmet.2021.03.002
62. Dupraz L, Magniez A, Rolhion N, Richard ML, Da Costa G, Touch S, et al. Gut microbiota-derived short-chain fatty acids regulate IL-17 production by mouse and human intestinal γδ T cells. Cell Rep (2021) 36(1):109332. doi: 10.1016/j.celrep.2021.109332
63. Cheung MK, Yue GGL, Lauw S, Li CSY, Yung MY, Ng SC, et al. Alterations in gut microbiota of esophageal squamous cell carcinoma patients. J Gastroenterol Hepatol (2022) 37(10):1919–27. doi: 10.1111/jgh.15941
64. Motoori M, Tanaka K, Sugimura K, Miyata H, Saito T, Miyazaki Y, et al. Impact of preoperative fecal short chain fatty acids on postoperative infectious complications in esophageal cancer patients. BMC Gastroenterol (2020) 20(1):74. doi: 10.1186/s12876-020-01217-y
65. Kim YL, Lee W, Chung SH, Yu BM, Lee YC, Hong J. Metabolic alterations of short-chain fatty acids and TCA cycle intermediates in human plasma from patients with gastric cancer. Life Sci (2022) 309:121010. doi: 10.1016/j.lfs.2022.121010
66. Sun G, Duan H, Meng J, Zhang D. Profiling and characterization of microRNAs responding to sodium butyrate treatment in gastric cancer cells. Comb Chem High Throughput Screen (2022) 25(11):1875–88. doi: 10.2174/1386207325666211027154207
67. Cao W, Zheng C, Xu X, Jin R, Huang F, Shi M, et al. Clostridium butyricum potentially improves inflammation and immunity through alteration of the microbiota and metabolism of gastric cancer patients after gastrectomy. Front Immunol (2022) 13:1076245. doi: 10.3389/fimmu.2022.1076245
68. Zhang K, Ji X, Song Z, Wu F, Qu Y, Jin X, et al. Butyrate inhibits gastric cancer cells by inducing mitochondria-mediated apoptosis. Comb Chem High Throughput Screen (2022) 26(3):630–8. doi: 10.2174/1386207325666220720114642
69. Alvandi E, Wong WKM, Joglekar MV, Spring KJ, Hardikar AA. Short-chain fatty acid concentrations in the incidence and risk-stratification of colorectal cancer: a systematic review and meta-analysis. BMC Med (2022) 20(1):323. doi: 10.1186/s12916-022-02529-4
70. Gomes S, Baltazar F, Silva E, Preto A. Microbiota-derived short-chain fatty acids: New road in colorectal cancer therapy. Pharmaceutics (2022) 14(11):2359. doi: 10.3390/pharmaceutics14112359
71. Brody LP, Sahuri-Arisoylu M, Parkinson JR, Parkes HG, So PW, Hajji N, et al. Cationic lipid-based nanoparticles mediate functional delivery of acetate to tumor cells in vivo leading to significant anticancer effects. Int J Nanomedicine (2017) 12:6677–85. doi: 10.2147/IJN.S135968
72. Kong C, Liang L, Liu G, Du L, Yang Y, Liu J, et al. Integrated metagenomic and metabolomic analysis reveals distinct gut-microbiome-derived phenotypes in early-onset colorectal cancer. Gut (2022) 11:gutjnl-2022-327156. doi: 10.1136/gutjnl-2022-327156
73. Garcia JA, Chen R, Xu M, Comerford SA, Hammer RE, Melton SD, et al. Acss2/HIF-2 signaling facilitates colon cancer growth and metastasis. PLoS One (2023) 18(3):e0282223. doi: 10.1371/journal.pone.0282223
74. Rodriguez-Enriquez S, Robledo-Cadena DX, Gallardo-Perez JC, Pacheco-Velazquez SC, Vazquez C, Saavedra E, et al. Acetate promotes a differential energy metabolic response in human HCT 116 and COLO 205 colon cancer cells impacting cancer cell growth and invasiveness. Front Oncol (2021) 11:697408. doi: 10.3389/fonc.2021.697408
75. Yu T, Ji L, Lou L, Ye S, Fang X, Li C, et al. Fusobacterium nucleatum affects cell apoptosis by regulating intestinal flora and metabolites to promote the development of colorectal cancer. Front Microbiol (2022) 13:841157. doi: 10.3389/fmicb.2022.841157
76. Lu Y, Zhao YC, Chang-Claude J, Gruber SB, Gsur A, Offit K, et al. Genetic predictors for fecal propionate and butyrate-producing microbiome pathway are not associated with colorectal cancer risk: A mendelian randomization analysis. Cancer Epidemiol Biomarkers Prev (2022) 32(2):281–6. doi: 10.1158/1055-9965.Epi-22-0861
77. Salvi PS, Cowles RA. Butyrate and the intestinal epithelium: Modulation of proliferation and inflammation in homeostasis and disease. Cells (2021) 10(7):1775. doi: 10.3390/cells10071775
78. Jaye K, Li CG, Chang D, Bhuyan DJ. The role of key gut microbial metabolites in the development and treatment of cancer. Gut Microbes (2022) 14(1):2038865. doi: 10.1080/19490976.2022.2038865
79. Kaźmierczak-Siedlecka K, Marano L, Merola E, Roviello F. Połom K: Sodium butyrate in both prevention and supportive treatment of colorectal cancer. Front Cell Infect Microbiol (2022) 12:1023806. doi: 10.3389/fcimb.2022.1023806
80. Bordonaro M. Further analysis of p300 in mediating effects of butyrate in colorectal cancer cells. J Cancer (2020) 11(20):5861–6. doi: 10.7150/jca.47160
81. Bordonaro M. Oncogenic and receptor-mediated wnt signaling influence the sensitivity of colonic cells to butyrate. J Cancer (2023) 14(3):446–53. doi: 10.7150/jca.82393
82. Avuthu N, Guda C. Meta-analysis of altered gut microbiota reveals microbial and metabolic biomarkers for colorectal cancer. Microbiol Spectr (2022) 10(4):e0001322. doi: 10.1128/spectrum.00013-22
83. Fang W, Xue H, Chen X, Chen K, Ling W. Supplementation with sodium butyrate modulates the composition of the gut microbiota and ameliorates high-fat diet-induced obesity in mice. J Nutr (2019) 149(5):747–54. doi: 10.1093/jn/nxy324
84. Ma X, Zhou Z, Zhang X, Fan M, Hong Y, Feng Y, et al. Sodium butyrate modulates gut microbiota and immune response in colorectal cancer liver metastatic mice. Cell Biol Toxicol (2020) 36(5):509–15. doi: 10.1007/s10565-020-09518-4
85. Chen D, Jin D, Huang S, Wu J, Xu M, Liu T, et al. Clostridium butyricum, a butyrate-producing probiotic, inhibits intestinal tumor development through modulating wnt signaling and gut microbiota. Cancer Lett (2020) 469:456–67. doi: 10.1016/j.canlet.2019.11.019
86. Kang J, Sun M, Chang Y, Chen H, Zhang J, Liang X, et al. Butyrate ameliorates colorectal cancer through regulating intestinal microecological disorders. Anticancer Drugs (2022) 34(2):227–37. doi: 10.1097/cad.0000000000001413
87. Kang J, Sun M, Chang Y, Chen H, Zhang J, Liang X, et al. Butyrate ameliorates colorectal cancer through regulating intestinal microecological disorders. Anticancer Drugs (2023) 34(2):227–37. doi: 10.1097/cad.0000000000001413
88. Ali SR, Orang A, Marri S, McKinnon RA, Meech R, Michael MZ. Integrative transcriptomic network analysis of butyrate treated colorectal cancer cells. Cancers (Basel) (2021) 13(4):636. doi: 10.3390/cancers13040636
89. Xi Y, Jing Z, Wei W, Chun Z, Quan Q, Qing Z, et al. Inhibitory effect of sodium butyrate on colorectal cancer cells and construction of the related molecular network. BMC Cancer (2021) 21(1):127. doi: 10.1186/s12885-021-07845-1
90. Chang CC, Kao WY, Liu CY, Su HH, Kan YA, Lin PY, et al. Butyrate supplementation regulates expression of chromosome segregation 1-like protein to reverse the genetic distortion caused by p53 mutations in colorectal cancer. Int J Oncol (2022) 60(6):64. doi: 10.3892/ijo.2022.5354
91. Zuo Z, Zhang P, Lin F, Shang W, Bi R, Lu F, et al. Interplay between trx-1 and S100P promotes colorectal cancer cell epithelial-mesenchymal transition by up-regulating S100A4 through AKT activation. J Cell Mol Med (2018) 22(4):2430–41. doi: 10.1111/jcmm.13541
92. Wang W, Fang D, Zhang H, Xue J, Wangchuk D, Du J, et al. Sodium butyrate selectively kills cancer cells and inhibits migration in colorectal cancer by targeting thioredoxin-1. Onco Targets Ther (2020) 13:4691–704. doi: 10.2147/OTT.S235575
93. Sun X, Zhu MJ. Butyrate inhibits indices of colorectal carcinogenesis via enhancing α-Ketoglutarate-Dependent DNA demethylation of mismatch repair genes. Mol Nutr Food Res (2018) 62(10):e1700932. doi: 10.1002/mnfr.201700932
94. Tran TQ, Hanse EA, Habowski AN, Li H, Ishak Gabra MB, Yang Y, et al. α-ketoglutarate attenuates wnt signaling and drives differentiation in colorectal cancer. Nat Cancer (2020) 1(3):345–58. doi: 10.1038/s43018-020-0035-5
95. Park B, Kim JY, Riffey OF, Dowker-Key P, Bruckbauer A, McLoughlin J, et al. Pyruvate kinase M1 regulates butyrate metabolism in cancerous colonocytes. Sci Rep (2022) 12(1):8771. doi: 10.1038/s41598-022-12827-9
96. Bian Z, Sun X, Liu L, Qin Y, Zhang Q, Liu H, et al. Sodium butyrate induces CRC cell ferroptosis via the CD44/SLC7A11 pathway and exhibits a synergistic therapeutic effect with erastin. Cancers (Basel) (2023) 15(2):423. doi: 10.3390/cancers15020423
97. Pant K, Saraya A, Venugopal SK. Oxidative stress plays a key role in butyrate-mediated autophagy via Akt/mTOR pathway in hepatoma cells. Chem Biol Interact (2017) 273:99–106. doi: 10.1016/j.cbi.2017.06.001
98. Awoniyi M, Wang J, Ngo B, Meadows V, Tam J, Viswanathan A, et al. Protective and aggressive bacterial subsets and metabolites modify hepatobiliary inflammation and fibrosis in a murine model of PSC. Gut (2022) 72(4):671–85. doi: 10.1136/gutjnl-2021-326500
99. Kobayashi M, Mikami D, Uwada J, Yazawa T, Kamiyama K, Kimura H, et al. A short-chain fatty acid, propionate, enhances the cytotoxic effect of cisplatin by modulating GPR41 signaling pathways in HepG2 cells. Oncotarget (2018) 9(59):31342–54. doi: 10.18632/oncotarget.25809
100. Inukai Y, Yamamoto K, Honda T, Ito T, Imai N, Ishizu Y, et al. Differences in the intestinal microbiome associated with diarrhea during lenvatinib treatment for hepatocellular carcinoma. Dig Dis (2022) 41(1):138–47. doi: 10.1159/000524298
101. Hong KU, Salazar-González RA, Walls KM, Hein DW. Transcriptional regulation of human arylamine n-acetyltransferase 2 gene by glucose and insulin in liver cancer cell lines. Toxicol Sci (2022) 190(2):158–72. doi: 10.1093/toxsci/kfac103
102. Zhang T, Li J, Liu CP, Guo M, Gao CL, Zhou LP, et al. Butyrate ameliorates alcoholic fatty liver disease via reducing endotoxemia and inhibiting liver gasdermin d-mediated pyroptosis. Ann Transl Med (2021) 9(10):873. doi: 10.21037/atm-21-2158
103. Zhao ZH, Wang ZX, Zhou D, Han Y, Ma F, Hu Z, et al. Sodium butyrate supplementation inhibits hepatic steatosis by stimulating liver kinase B1 and insulin-induced gene. Cell Mol Gastroenterol Hepatol (2021) 12(3):857–71. doi: 10.1016/j.jcmgh.2021.05.006
104. Zhou W, Zhang D, Li Z, Jiang H, Li J, Ren R, et al. The fecal microbiota of patients with pancreatic ductal adenocarcinoma and autoimmune pancreatitis characterized by metagenomic sequencing. J Transl Med (2021) 19(1):215. doi: 10.1186/s12967-021-02882-7
105. Hashimoto S, Tochio T, Funasaka K, Funahashi K, Hartanto T, Togashi Y, et al. Changes in intestinal bacteria and imbalances of metabolites induced in the intestines of pancreatic ductal adenocarcinoma patients in a Japanese population: a preliminary result. Scand J Gastroenterol (2022) 58(2):193–8. doi: 10.1080/00365521.2022.2114812
106. Temel HY, Kaymak Ö, Kaplan S, Bahcivanci B, Gkoutos GV, Acharjee A. Role of microbiota and microbiota-derived short-chain fatty acids in PDAC. Cancer Med (2022) 12(5):5661–75. doi: 10.1002/cam4.5323
107. Lee SY, Lee DY, Kang JH, Kim JH, Jeong JW, Kim HW, et al. Relationship between gut microbiota and colorectal cancer: Probiotics as a potential strategy for prevention. Food Res Int (2022) 156:111327. doi: 10.1016/j.foodres.2022.111327
108. Dikeocha IJ, Al-Kabsi AM, Eid EEM, Hussin S, Alshawsh MA. Probiotics supplementation in patients with colorectal cancer: a systematic review of randomized controlled trials. Nutr Rev (2021) 80(1):22–49. doi: 10.1093/nutrit/nuab006
109. Srikham K, Thirabunyanon M. Bioprophylactic potential of novel human colostrum probiotics via apoptotic induction of colon cancer cells and cell immune activation. BioMed Pharmacother (2022) 149:112871. doi: 10.1016/j.biopha.2022.112871
110. Liu M, Xie W, Wan X, Deng T. Clostridium butyricum modulates gut microbiota and reduces colitis associated colon cancer in mice. Int Immunopharmacol (2020) 88:106862. doi: 10.1016/j.intimp.2020.106862
111. Tomita Y, Ikeda T, Sakata S, Saruwatari K, Sato R, Iyama S, et al. Association of probiotic clostridium butyricum therapy with survival and response to immune checkpoint blockade in patients with lung cancer. Cancer Immunol Res (2020) 8(10):1236–42. doi: 10.1158/2326-6066.Cir-20-0051
112. Cousin FJ, Jouan-Lanhouet S, Théret N, Brenner C, Jouan E, Le Moigne-Muller G, et al. The probiotic propionibacterium freudenreichii as a new adjuvant for TRAIL-based therapy in colorectal cancer. Oncotarget (2016) 7(6):7161–78. doi: 10.18632/oncotarget.6881
113. Jaiswal S, Pradhan SN, Jain D, Dhassiah Peter MP, Antony U. Probiotic and functional characterization of pediococcus acidilactici isolated from bhaati jaanr, traditional fermented rice porridge. Appl Biochem Biotechnol (2022) 194(12):5734–47. doi: 10.1007/s12010-022-04041-0
114. Zhang C, Ma K, Nie K, Deng M, Luo W, Wu X, et al. Assessment of the safety and probiotic properties of roseburia intestinalis: A potential "Next generation probiotic". Front Microbiol (2022) 13:973046. doi: 10.3389/fmicb.2022.973046
115. Ryu SW, Kim JS, Oh BS, Choi WJ, Yu SY, Bak JE, et al. Gut microbiota eubacterium callanderi exerts anti-colorectal cancer activity. Microbiol Spectr (2022) 10(6):e0253122. doi: 10.1128/spectrum.02531-22
116. Dikeocha IJ, Al-Kabsi AM, Chiu HT, Alshawsh MA. Faecalibacterium prausnitzii ameliorates colorectal tumorigenesis and suppresses proliferation of HCT116 colorectal cancer cells. Biomedicines (2022) 10(5):1128. doi: 10.3390/biomedicines10051128
117. Botta C, Spyridopoulou K, Bertolino M, Rantsiou K, Chlichlia K, Cocolin L. Lactiplantibacillus plantarum inhibits colon cancer cell proliferation as function of its butyrogenic capability. BioMed Pharmacother (2022) 149:112755. doi: 10.1016/j.biopha.2022.112755
118. Garbacz K. Anticancer activity of lactic acid bacteria. Semin Cancer Biol (2022) 86(Pt3):356–66. doi: 10.1016/j.semcancer.2021.12.013
119. Chen L, Zhou X, Wang Y, Wang D, Ke Y, Zeng X. Propionate and butyrate produced by gut microbiota after probiotic supplementation attenuate lung metastasis of melanoma cells in mice. Mol Nutr Food Res (2021) 65(15):e2100096. doi: 10.1002/mnfr.202100096
120. Doublier S, Cirrincione S, Scardaci R, Botta C, Lamberti C, Giuseppe FD, et al. Putative probiotics decrease cell viability and enhance chemotherapy effectiveness in human cancer cells: role of butyrate and secreted proteins. Microbiol Res (2022) 260:127012. doi: 10.1016/j.micres.2022.127012
121. Reynolds A, Mann J, Cummings J, Winter N, Mete E, Te Morenga L. Carbohydrate quality and human health: a series of systematic reviews and meta-analyses. Lancet (2019) 393(10170):434–45. doi: 10.1016/s0140-6736(18)31809-9
122. Arayici ME, Mert-Ozupek N, Yalcin F, Basbinar Y, Ellidokuz H. Soluble and insoluble dietary fiber consumption and colorectal cancer risk: A systematic review and meta-analysis. Nutr Cancer (2022) 74(7):2412–25. doi: 10.1080/01635581.2021.2008990
123. Kim H, Youn J, Yang SY, Song JH, Kim YS, Lee JE. Association between dietary fiber intake and colorectal adenoma. Nutr Cancer (2022) 74(10):3446–56. doi: 10.1080/01635581.2022.2083189
124. Zhang SL, Mao YQ, Zhang ZY, Li ZM, Kong CY, Chen HL, et al. Pectin supplement significantly enhanced the anti-PD-1 efficacy in tumor-bearing mice humanized with gut microbiota from patients with colorectal cancer. Theranostics (2021) 11(9):4155–70. doi: 10.7150/thno.54476
125. Gheorghe AS, Negru ȘM, Preda M, Mihăilă RI, Komporaly IA, Dumitrescu EA, et al. Biochemical and metabolical pathways associated with microbiota-derived butyrate in colorectal cancer and omega-3 fatty acids implications: A narrative review. Nutrients (2022) 14(6):1152. doi: 10.3390/nu14061152
126. Volpato M, Hull MA. Omega-3 polyunsaturated fatty acids as adjuvant therapy of colorectal cancer. Cancer Metastasis Rev (2018) 37(2-3):545–55. doi: 10.1007/s10555-018-9744-y
127. Wong SH, Zhao L, Zhang X, Nakatsu G, Han J, Xu W, et al. Gavage of fecal samples from patients with colorectal cancer promotes intestinal carcinogenesis in germ-free and conventional mice. Gastroenterology (2017) 153(6):1621–33.e1626. doi: 10.1053/j.gastro.2017.08.022
128. Rosshart SP, Vassallo BG, Angeletti D, Hutchinson DS, Morgan AP, Takeda K, et al. Wild mouse gut microbiota promotes host fitness and improves disease resistance. Cell (2017) 171(5):1015–28.e1013. doi: 10.1016/j.cell.2017.09.016
129. Chang CW, Lee HC, Li LH, Chiang Chiau JS, Wang TE, Chuang WH, et al. Fecal microbiota transplantation prevents intestinal injury, upregulation of toll-like receptors, and 5-Fluorouracil/Oxaliplatin-Induced toxicity in colorectal cancer. Int J Mol Sci (2020) 21(2):386. doi: 10.3390/ijms21020386
130. Huang J, Zheng X, Kang W, Hao H, Mao Y, Zhang H, et al. Metagenomic and metabolomic analyses reveal synergistic effects of fecal microbiota transplantation and anti-PD-1 therapy on treating colorectal cancer. Front Immunol (2022) 13:874922. doi: 10.3389/fimmu.2022.874922
131. Danne C, Sokol H. Butyrate, a new microbiota-dependent player in CD8+ T cells immunity and cancer therapy? Cell Rep Med (2021) 2(7):100328. doi: 10.1016/j.xcrm.2021.100328
132. Nomura M, Nagatomo R, Doi K, Shimizu J, Baba K, Saito T, et al. Association of short-chain fatty acids in the gut microbiome with clinical response to treatment with nivolumab or pembrolizumab in patients with solid cancer tumors. JAMA Netw Open (2020) 3(4):e202895. doi: 10.1001/jamanetworkopen.2020.2895
133. Chen Y, Liu Y, Wang Y, Chen X, Wang C, Chen X, et al. Prevotellaceae produces butyrate to alleviate PD-1/PD-L1 inhibitor-related cardiotoxicity via PPARα-CYP4X1 axis in colonic macrophages. J Exp Clin Cancer Res (2022) 41(1):1. doi: 10.1186/s13046-021-02201-4
134. Coutzac C, Jouniaux JM, Paci A, Schmidt J, Mallardo D, Seck A, et al. Systemic short chain fatty acids limit antitumor effect of CTLA-4 blockade in hosts with cancer. Nat Commun (2020) 11(1):2168. doi: 10.1038/s41467-020-16079-x
135. Sánchez-Alcoholado L, Laborda-Illanes A, Otero A, Ordóñez R, González-González A, Plaza-Andrades I, et al. Relationships of gut microbiota composition, short-chain fatty acids and polyamines with the pathological response to neoadjuvant radiochemotherapy in colorectal cancer patients. Int J Mol Sci (2021) 22(17):9549. doi: 10.3390/ijms22179549
136. Shuwen H, Yangyanqiu W, Jian C, Boyang H, Gong C, Jing Z. Synergistic effect of sodium butyrate and oxaliplatin on colorectal cancer. Transl Oncol (2023) 27:101598. doi: 10.1016/j.tranon.2022.101598
137. Park M, Kwon J, Shin HJ, Moon SM, Kim SB, Shin US, et al. Butyrate enhances the efficacy of radiotherapy via FOXO3A in colorectal cancer patient−derived organoids. Int J Oncol (2020) 57(6):1307–18. doi: 10.3892/ijo.2020.5132
138. Yue X, Wen S, Long-Kun D, Man Y, Chang S, Min Z, et al. Three important short-chain fatty acids (SCFAs) attenuate the inflammatory response induced by 5-FU and maintain the integrity of intestinal mucosal tight junction. BMC Immunol (2022) 23(1):19. doi: 10.1186/s12865-022-00495-3
Keywords: short-chain fatty acids, gastrointestinal tumors, tumor immunity, probiotics, fecal microbiota transplantation, immunotherapy
Citation: Dong Y, Zhang K, Wei J, Ding Y, Wang X, Hou H, Wu J, Liu T, Wang B and Cao H (2023) Gut microbiota-derived short-chain fatty acids regulate gastrointestinal tumor immunity: a novel therapeutic strategy? Front. Immunol. 14:1158200. doi: 10.3389/fimmu.2023.1158200
Received: 03 February 2023; Accepted: 17 March 2023;
Published: 14 April 2023.
Edited by:
Peng Chen, Lanzhou University, ChinaReviewed by:
Karolina Kaźmierczak-Siedlecka, Medical University of Gdansk, PolandGlauben Tamara Landskron, Universidad Finis Terrae, Chile
Copyright © 2023 Dong, Zhang, Wei, Ding, Wang, Hou, Wu, Liu, Wang and Cao. This is an open-access article distributed under the terms of the Creative Commons Attribution License (CC BY). The use, distribution or reproduction in other forums is permitted, provided the original author(s) and the copyright owner(s) are credited and that the original publication in this journal is cited, in accordance with accepted academic practice. No use, distribution or reproduction is permitted which does not comply with these terms.
*Correspondence: Hailong Cao, Y2FvaGFpbG9uZ0B0bXUuZWR1LmNu
†These authors have contributed equally to this work