- 1Guangdong Provincial Key Laboratory of Medical Molecular Diagnostics, The First Dongguan Affiliated Hospital, Guangdong Medical University, Dongguan, China
- 2Dongguan Key Laboratory of Environmental Medicine, School of Public Health, Guangdong Medical University, Dongguan, China
- 3Institute of Laboratory Medicine, School of Medical Technology, Guangdong Medical University, Dongguan, China
As an essential micronutrient, manganese plays an important role in the physiological process and immune process. In recent decades, cGAS-STING pathway, which can congenitally recognize exogenous and endogenous DNA for activation, has been widely reported to play critical roles in the innate immunity against some important diseases, such as infections and tumor. Manganese ion (Mn2+) has been recently proved to specifically bind with cGAS and activate cGAS-STING pathway as a potential cGAS agonist, however, is significantly restricted by the low stability of Mn2+ for further medical application. As one of the most stable forms of manganese, manganese dioxide (MnO2) nanomaterials have been reported to show multiple promising functions, such as drug delivery, anti-tumor and anti-infection activities. More importantly, MnO2 nanomaterials are also found to be a potential candidate as cGAS agonist by transforming into Mn2+, which indicates their potential for cGAS-STING regulations in different diseased conditions. In this review, we introduced the methods for the preparation of MnO2 nanomaterials as well as their biological activities. Moreover, we emphatically introduced the cGAS-STING pathway and discussed the detailed mechanisms of MnO2 nanomaterials for cGAS activation by converting into Mn2+. And we also discussed the application of MnO2 nanomaterials for disease treatment by regulating cGAS-STING pathway, which might benefit the future development of novel cGAS-STING targeted treatments based on MnO2 nanoplatforms.
1 Introduction
Host cells initiate innate immune responses by recognizing conserved pathogen structures through pathogen-associated molecular patterns (PAMPs) and host damage-associated molecular patterns (DAMPs) through diverse pattern recognition receptors (PRRs), both of which can identify abnormal DNA (1, 2). Cyclic GMP-AMP synthase (cGAS, cGAMP synthase) is a cytosolic DNA sensor belonging to the nucleotidyltransferase family. When binds to DNA, cGAS undergoes a conformational change to an active state and generates the second messenger circulating GMP-AMP (cGAMP) to activate a type-I interferon response by binding to STING, which coordinates immune defense mechanisms (3, 4). The cGAS-STING axis is activated not only by non-self DNA, but by extracellular,mitochondrial and nuclear DNA capable of entering the cytoplasm. Elevated levels of cytoplasmic DNA due to inflammation, autoimmunity, tumors, bacteria and viruses would lead to constitutive and systemic activation of cGAS-STING and promote the body’s immune defense. Increasing pieces of evidence suggest that cGAS-STING pathway is important in mediating cellular immune sensing, which therefore can be used as novel targets for the vaccine and drug development (5, 6).
In recent years, manganese has been widely concerned as an agonist of the cGAS-STING pathway. Manganese is the twelfth most abundant element on the planet, and it is an indispensable trace element in the normal human body. As the catalytic center and the structural core of various enzymes, manganese is involved in a large number of biological processes, including oxidative phosphorylation, glycosylation and signal transduction (7, 8). Due to these critical functions, manganese has been proved to play critical roles in some important diseases. For example, manganese ions (Mn2+) can regulate the complicated tumor microenvironment, including hypoxia regulation, glutathione depletion, glucose consumption and immunosuppressive tumor microenvironment regulation, which therefore introduce manganese for anti-tumor strategy development (9, 10). Combined with glucose oxidase, Mn2+ can also indirectly convert glucose into ROS to induce oxidative damage of tumor tissue, which might result in better anti-tumor activity of current chemotherapies (11). Moreover, manganese is also capable of regulating the immune system dependent on different mechanisms. For example, Mn2+ can effectively promote DC maturation and antigen presentation of DC and macrophages to augment CD8+ T cell and NK cell activation (12).
Moreover, manganese can activate the host immune system by regulating the cGAS-STING pathway (13, 14). It has been widely known that Mn2+ can enhance the sensitivity of cGAS to double-stranded DNA (dsDNA) and its enzymatic activity by binding with cGAS to enhance cGAMP-STING binding affinity (15, 16). Mn2+ directly activates cGAS without DNA and triggers a distinct catalytic synthesis of 2’3’-cGAMP,so Mn2+ can induce cells to produce type I IFNs without any infection (16, 17). Mn2+ can catalyze H2O2 into • OH, a kind of highly active reactive oxygen species (ROS) that is helpful to activate cGAS-STING signalings (18). Mn2+ also shows STING-independent immune activating potential by inducing phosphorylation of TBK1 and p65, which is further augmented and translated to IRF3 phosphorylation in the presence of STING agonists, resulting in amplification of the STING signalling cascade (19). Based on the above ability, manganese seems to bridge innate and adaptive immunity by promoting DC maturation and antigen presentation (17), which therefore shows their potential for anti-tumor and anti-infection treatments (8, 16, 20, 21).
The rapid development of nanotechnology in recent decades allow the more and more clinical application of functional nanomaterials (22, 23). Due to the complicated valence of manganese, there are different kinds of manganese nanomaterials, and MnO2 nanomaterials (MnO2 NPs) are one of the most stable and functional nanomaterials among them. MnO2 NPs have shown a wide range of applications in biomedicine, such as biological imaging therapy, drug delivery and immune regulation. MnO2 NPs can be used as an ideal biodegradable agent for specific drug delivery, while producing Mn2+ (24, 25). This can significantly promote the Fenton-like effect to amplify the generation of cytotoxic hydroxyl radicals (• OH) and achieve significant GSH depletion enhanced chemokinetic therapy (CDT) (25). Additionally, MnO2 is one of the best alternative contrast agents for T1-weighted magnetic resonance imaging (MRI) besides gadolinium-based contrast agents (24). Enzymatically synthesised MnO2 NPs (Bio-MnO2 NPs) also possess photothermal therapy (PTT) functions because of their extraordinary photothermal conversion efficiency (44%) (26). Additionally, MnO2 NPs, as a kind of TME-responsive drug carrier, can be rapidly decomposed in acidic and reduced environment to show on-demand drug release at the tumor sites, which highlighted the critical roles of MnO2 NPs in cancer therapy and theranostics.
Mn2+ produced by MnO2 NP in cells can regulate the cGAS-STING signal pathway and promote the pathway to play an important physiological function. This indicates the potential ability of MnO2 NP to target the cGAS-STING signal pathway for disease prevention and treatment.In this review, we summarized the ability and mechanism of MnO2 NPs to regulate cGAS-STING signaling events. Moreover, we also introduced the current progress of MnO2 NPs for potential disease treatment by regulating GAS-STING pathway, which might provide more possibilities to develop novel therapies in different disease conditions.
2 Biological characteristics of cGAS-STING pathway
2.1 Structure and function of cGAS
cGAS is an unstructured, highly basic protein of approximately 160 amino acid amino-terminal (N-terminal) domain and a globular approximately 360 amino acid domain of 520 amino acid protein (4, 27, 28). The catalytic domain of cGAs consists of two structural lobes in which the active site is located (4, 29, 30). Lobe 1 includes the evolutionarily conserved NTase core β-Sheet and a conserved acidic residue involved in the Mg2+-dependent catalytic transfer of nucleoside phosphates to hydroxyl acceptors is also found in DNA polymerases. Lobe II completes the active site and increases the interaction for nucleoside triphosphate donor binding (4).
Free cGAS (not bound to dsDNA) is not catalytically active because it has no catalytic site of the suitable structure. When cGAS is activated by dsDNA, binding of dsDNA (length > 45 b) between two cGAS monomers creates a structured catalytic site that results in cGAS activation. The structure of cGAS activation is a dimer with the two DNA strands sandwiched between two cGAS protomers (29, 30). This allosteric process of cGAS dimer formation converts cGAS from an inactive to an active state. There are two binding sites “ a “ and “ b “ for each cGAS protomer in the dimer, with one DNA strand binding to site “ a “of one cGAS protomer and site “b” of the other corresponding cGAs protomer. cGAS covers approximately 16-18 bp of DNA, and this binding is independent of the sequence information bound (4, 29, 30).
As a DNA receptor, cGAS can recognize different types of DNA, which may be related to different subcellular structural localization (4). However, the cellular localization of cGAS is still unclear. cGAS may exist in the nucleus, cytoplasm and cell membrane, because highly disordered N-terminal fragments of cGAS may play a role in the attachment of cGAS plasma membrane (31). As a DNA receptor, cGAS can recognize exogenous DNA, including infection related DNA from viruses and viruses or bacteria entering the cytoplasm (32, 33), as well as self sourced DNA, including mitochondrial cytoplasmic DNA (34), DNA in cytoplasmic micronucleus (35) and chromatin in nucleus. Structurally, it includes long dsDNA molecules, single strand DNA (ssDNA) with local secondary structure, short synthetic DNA with G-rich single strand protrusions, and RNA DNA hybrids (but RNA DNA hybrids constitute sub optimal agonists) (4).
2.2 Signaling processes and functions of cGAS-STING pathway
The classical biological function of cGAS is an indispensable bridge between the recognition of exogenous pathogen dsDNA and immune defense. In summary as shown in Figure 1, the combination of cGAS and dsDNA induces the dimerization of cGAS and leads to conformational changes, promoting the oligomerization and activation of cGAS (36). The cGAS dimer combines with two molecules of dsDNA to initiate liquid-liquid phase separation and form a trapezoidal structure, which enables cGAS to be allosterically activated and opens the catalytic pocket to catalyze the cyclization of ATP and GTP to form cGAMP (28, 36). cGAMP is the first cyclic dinucleotide found in multicellular animals and acts as an endogenous second messenger to trigger downstream cascade signals (28).
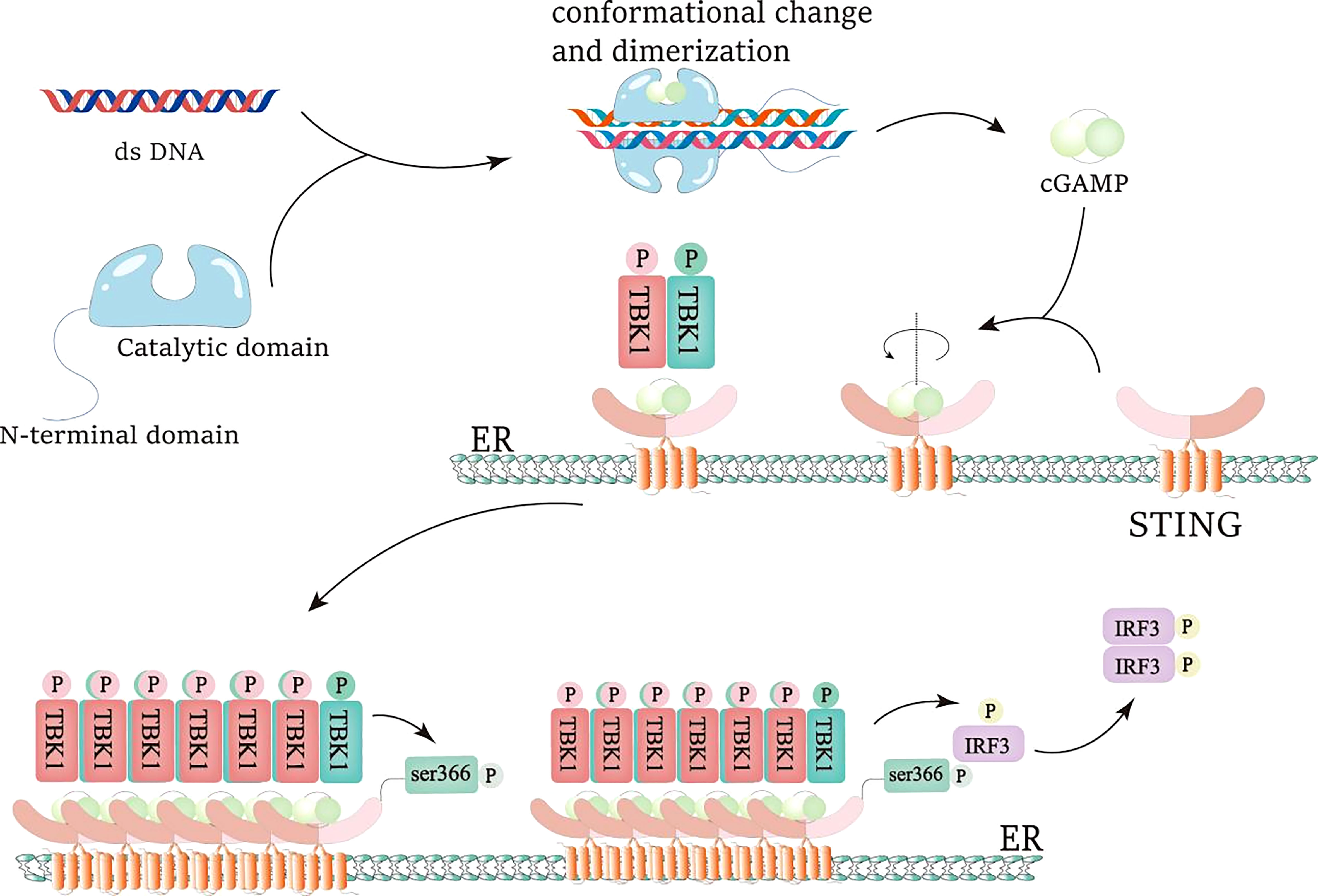
Figure 1 IRF3 activation of cGAS-STING pathway. dsDNA is recognized and combined by cGAS, resulting in the conformational change and dimerization of cGAS and the generation of cGAMP. When cGAMP is combined with the STING pocket structure, the STING dimer rotates 180°relative to the transmembrane helmets, which is beneficial to the oligomerization of the STING dimer and the promotion of the trans-phosphorylation of TBK1. Active TBK1 generates the docking site of IRF3 by phosphorylating the S366 site of STING. Then, active TBK1 phosphorylates IRF3, promotes IRF3 dimerization, and makes IRF3 obtain transcriptional activity.
The most important and typical downstream target of cGAS is STING signal pathway (4, 28, 37, 38). cGAMP combines with the small pocket of dimer STING to change the conformation of STING and promote the transportation of STING from endoplasmic reticulum to Golgi apparatus (4, 28). In this process, STING raised TANK binding kinase 1 (TBK1) to activate its autophosphorylation. Activated TBK1 can phosphorylate STING in turn, and enhance the interaction between STING and interferon regulatory factor 3 (IRF3), thereby further promoting TBK1’s phosphorylation of IRF3. Phosphorylated IRF3 dimerizes and enters the nucleus, thereby inducing transcription of type I interferon (IFN-1) and other cytokines (4, 28, 37). IFN-1 receptor is a heterodimer composed of IFNAR 1 and IFNAR 2 subunits (39). IFNs first bind a high-affinity receptor chain (IFNAR 2), and then recruit a low-affinity receptor chain (IFNAR 1) to produce a signal-active ternary complex. Receptor dimerization activates Tyk 2 and JAK 1 kinases that phosphorylate STAT 1 and STAT 2. The phosphorylated STAT 1 and STAT 2 heterodimers combine with IRF 9 to produce the transcription factor ISGF 3. ISGF 3 binds to ISREs and promotes the expression of a large number of ISGs. ISG can target different stages of virus replication and then resist virus infection. Unlike IFN-α, IFN-β can form a high-affinity complex with IFNAR 1 and conduct IFN-b signal transduction in an IFNAR 2 independent manner. In addition to their antiviral effects, IFN-I is also protective against several extracellular bacterial infections (40). For example, in Streptococcus, Escherichia coli, and Helicobacter infections, the increased expression of IFN-I is beneficial to anti-bacterial infection. At the same time, STING can also activate IκB kinase (IKK), and phosphorylate NF-κB Inhibitory Protein IκB αSer32 and Ser36, leading to their polyubiquitination and degradation, followed by NF-κB can enter the nucleus. The cGAS-STING-IKK signaling pathway, together with IRF3, induces classical NF-κB function, starts the transcription of immune stimulating gene, and passes the non classical NF-κB Pathway participate in other important activities (28, 37, 38, 41). cGAS recognizes abnormal exogenous and endogenous DNA, promotes activation of multiple immune stimulators, autophagy, remodeling of tumor environment, and production of inflammatory factors through cGAS-STING signal pathway, and plays an important role in inflammation, autoimmunity, anti-tumor, anti-bacterial, and viral infection (28, 32, 33, 38, 42–46).
cGAMP is a kind of cyclic dinucleotide (CDN) formed by the connection of GTP and ATP through phosphodiester bond. Because of the extensive polar and hydrophobic interactions between 2’3’- cGAMP and STING binding sites, it has a higher affinity with STING than other CDNs (3’3’, 2’2 ‘, 3’2’ - cGAMP or c-di GMP), and is a ligand with higher affinity for STING (4, 36). STING is a small protein (~40 kDa), which contains a short N-terminal cytoplasmic segment, four transmembrane (TM) helices, and is located in the endoplasmic reticulum (ER) membrane, the cytoplasmic ligand binding domain (LBD), and the C-terminal tail (CTT) responsible for binding TBK1 (47). STING is a transmembrane receptor protein stimulator (STING) of interferon genees residing on the endoplasmic reticulum (ER) membrane (45). Under steady state conditions, STING binding ER forms a dimer, producing a V-shaped pocket ligand binding domain (LBD) that can bind to a cyclic dinucleotide, enabling cGAMP to bind STING (4). The combination of cGAMP and STING leads to its transfer from the endoplasmic reticulum to the Golgi apparatus, which is activated by palmitoylation on Cys88 and Cys91 (28, 36). TBK1 was recruited onto the conservative PLPLRT/SD motif at the C-terminal tail of STING (48), and IFIT3 was used as the linker connecting TBK1 and STING (36). Ligand binding and STING oligomerization seem to promote the trans phosphorylation of TBK1. Then active TBK1 phosphorylates the C-terminal tail (CTT) of STING (this occurs on the adjacent dimer of STING, not on the dimer bound by TBK1). STING CTT phosphorylation creates a docking site for IRF3, which is then phosphorylated by TBK1. IRF3 was then dimerized to obtain transcriptional activity (4). In Golgi apparatus, STING-TBK1 interaction finally activates interferon regulatory factor 3 (IRF3) and NF-κB that the main effectors of these two innate immunities (4, 28, 36). The combination of cGAMP and STING leads to the activation of different downstream effector functions, including autophagy and cell death (45).
In addition to its main catalytic function, cGAS also has a variety of non catalytic functions. cGAS can promote the perception of extracellular cyclic dinucleotides, making extracellular cyclic dinucleotides enter cells through endocytosis. These extracellular cyclic dinucleotides enhance the formation of the active dimer form of cGAS, indicating that they may be able to transfer equilibrium to cGAS dimer, thereby promoting the activation of cGAS (4, 28). The function of cGAS in human body is extensive, and the regulation of cGAS activity can play an important role in the treatment of diseases. Therefore, the study of cGAS agonists is hopeful to increase the success rate of treatment of related diseases.
3 Medical application of MnO2 nanomaterials
3.1 Introduction of MnO2 nanomaterials
MnO2 nanomaterials have been reported to show excellent physical, chemical and biological properties, such as high specific surface area, high adsorption, strong oxidation, high catalytic activity, broad spectrum light absorption range, strong fluorescence quenching, good degradability and biosafety, etc (49–51). There are two widely known mechanisms for MnO2 nanomaterials to excute their functions in biological systems: the redox reaction between MnO2 and glutathione (GSH) (52), and the catalytic reactions of MnO2 nanomaterials to decompose H2O2 into O2 under acidic conditions (53). These characteristics make MnO2 nanomaterials a kind of outstanding multi-functional biomedical materials that can be used in many biomedical fields, such assensors (54), contrast agents (55), biological tumor therapy (56) and drug delivery (57).
3.2 Preparation method of MnO2 nanomaterials
Due to the attractive properties, the preparation methods of MnO2 nanomaterials have been widely developed recently (As shown in Table 1). The template method is a typical method to prepare MnO2 materials with templates of different forms, and then etch the templates to obtain MnO2 products. For example, Fei et al. reported a method to prepare hierarchical hollow manganese dioxide nanosheets with intricate and well-controlled 3D morphologies by combining the Kirkendall effect with a sacrificial crystalline template (58). Particularly, discrete spherical and cubic hollow MnO2 nanostructures with controlled morphologies can be prepared by changing the morphologies of MnCO3 precursors, which can be simply obtained by adding the (NH4)2SO4 solution in the reaction system. Moreover, the thicknesses of the shells of hierarchical hollow nanostructures can be adjusted easily by the relative quantities of KMnO4 reacted followed by selective removal of MnCO3 crystal template. This template approach will find wide acceptance and use in the field of template-directed nanostructure synthesis of MnO2 nanomaterials.
Hydrothermal method is a simple and relatively environment-friendly method based on self-assembly for MnO2 nanomaterials preparation. High quality single crystal MnO2 nanomaterials can be easily synthesized by simple hydrothermal treatment. For example, Guan et al.introduced a facile one-step hydrothermal method to synthesize a novel manganese dioxide-graphene nanosheets (GNSs) (59). GNSs were fully mixed with KMnO4 in an acidic environment, and KMnO4 was then reduced to MnO2 by a hydrothermal process to allow the nucleation and growth of MnO2 on GNSs for biosensing application. Tehseen et al. Also introduced a hydrothermal method to prepare manganese oxide nanofibrous (MnO2 NFs) by mixing manganese acetate tetrahydrate and sodium hydroxide for hydrothermal reaction (62). Manganese acetate tetrahydrate is converted into manganese hydroxide (II) and then manganese (III) hydroxide upon the addition of alkali. And the hydrothermal treatment occurs under alkaline reaction conditions at elevated temperature, manganese (III) hydroxide converts to manganese (IV) oxide (blackish brown) that builds the nuclei for the growth of nanomaterials. These hydrothermal methods can synthesize MnO2 nanomaterials with facile, one-pot, and cost-effective approach without using any template or structure directing compound.
Oxidation and reduction methods are also widely used for MnO2 nanomaterials preparation, which mainly include Mn2+ (manganese chloride) oxidation method and Mn7+ (potassium permanganate) reduction method. Manganese dioxide can be synthesized by oxidizing Mn2+ or reducing potassium permanganate with reducing agents and oxidants, to form manganese dioxide nanostructures. Oaki et al. reported the method for one-pot synthesis of manganese oxide nanosheets in an aqueous solution by mixing solutions containing Mn2+/EDTA and NaOH (60). Chelation to the divalent manganese ion and interaction with the resultant oxide involved to form birnessite nanosheets in aqueous solution at room temperature, which finally led to the formation of manganese oxide nanosheets. Yang et al. proposed a method for preparing MnO2 nanoparticles by reducing KMnO4 with excessive Na2S2O3 and the GOx and BSA-Chlorine e6 (BSA-Ce6) were employed onto the surface of MnO2 nanoparticles (NPs) (63). These oxidation reduction methods are widely used as a convenient, simple and effective method to prepare manganese dioxide materials without any specific requirements of hydrothermal treatment and special equipment.
Biomineralization is a technology that combines biological macromolecules with inorganic materials to prepare inorganic minerals by adjusting biological macromolecules for MnO2 nanomaterials preparation. Bioorganic substances (such as serum albumin) can convert ions into solid minerals under physical and chemical conditions (61), which is also partially based on the oxidation and reduction methods. During the preparation process of MnO2, biological organic substances guide the nucleation of Mn2+ to spontaneously form MnO2 nanostructures through growth or oxidation in alkaline solution (64). The biomineralization method provides the feasibility to synthesize multifunctional and biocompatible MnO2 nanomaterials as potential therapeutic agents (65). These biomineralization methods have demonstrated the advantages of cost-effectiveness, convenience and environmental friendliness, which thus can be widely applied for dioxide nanomaterial preparation.
3.3 Basic biological activity of MnO2 nanomaterials
The excellent physical, chemical and biological properties of MnO2 nanomaterials make it an excellent multifunctional biomaterial and can be used in many biomedical fields (66). MnO2 nanomaterials have been widely developed as drug carriers to enhance drug efficiency. The drugs used to treat tumors will reduce the effect of chemotherapy because of the drug resistance factors and hypoxia conditions in the tumor microenvironment, such as overexpression and activation of hypoxia inducible factors (eg, HIF-1a) and multidrug resistance (MDR) (eg, multiple p53 and P-glycoprotein [P-gp]). Manganese dioxide can improve the tumor microenvironment and make the drug loaded complex show better efficacy than single drug. Amini et al. proposed a strategy that preparing multifunctionality of polymer-lipid encapsulated MnO2 nanomaterials (PLMD NPs) as drug carrier to enhance the efficacy of doxorubicin (56). These MnO2 nanomaterials can enhance the penetration of doxorubicin to tumors for enhanced chemotherapy efficiency. In addition, manganese dioxide can reduce hydrogen peroxide in the microenvironment, reduce oxidative stress, and protect biological enzyme drugs. Manganese dioxide materials help biological enzyme drugs overcome the shortcomings of low stability, high cost and storage difficulties of natural enzymes, and improve their potential applications in the biomedical field. E. Marin et al. proposed a method to prepare an antioxidant microreactor by encapsulating manganese dioxide nanoparticles into layered polymer capsules (67). They used manganese dioxide nanoparticles to replace the encapsulation of traditional antioxidant enzymes, providing a more solid and stable inorganic substitute, which is a new strategy for preparing antioxidant polymer microreactors. Li et al.introduced chitosan-modified hollow MnO2 nanomaterials as a resveratrol loading system, which indicated that MnO2 nanomaterials could also serve as a potential central nervous system drug delivery system for the treatment of spinal cord injury (68). Wang et al. show that a leukemia cell membrane (LCM)-camouflaged hollow MnO2 nanocarrier (HM) with encapsulated doxorubicin (DOX) (denoted LHMD) could bind specifically to AML cells with a homologous targeting effect (69). LHMD rationally deliver chemotherapeutic agents and to trigger Mn2+ mediated STING pathway activation for potent immune and chemotherapy against AML cells.
MnO2 nanomaterials can release Mn2+ in the body, which therefore makes MnO2 nanomaterials possess the biological functions of Mn2+. Mn2+ can be used as an effective T1 weighted MRI contrast agent because of its five unpaired 3d electrons (70).Zhang et al. constructed an intelligent nanoplatform based on poly (N-vinylcaprolactam) (PVCL) nanogels (NGs) co-loaded with gold (Au) and manganese dioxide (MnO2) nanoparticles (NPs) for dual-mode computed tomography (CT)/magnetic resonance (MR) imaging (71). MnO2 nanomaterials can release Mn2+ more efficiently under tumor microenvironment conditions, which therefore provided an enhanced signal for MRI imaging and can be used for imaging-guided therapy. Xiao et al. also evaluated the MRI imaging performance of MnO2 nanomaterials under simulated tumor microenvironment conditions, and Found that the relaxation rate under tumor microenvironment conditions was 16 times higher than that under non tumor microenvironment conditions (70). These experiments show that MnO2 nanomaterials can be used as a potential T1 contrast agent for tumor specific MRI imaging or imaging-guided therapy method development.
Tumor microenvironment (TME) is usually characterized by low pH value, high glutathione (GSH) concentration, excessive production of hydrogen peroxide (H2O2) and severe hypoxia (72). These characteristics can provide a favorable internal environment for the generation and survival of tumor cells, and are closely related to tumor progression, metastasis and drug resistance. Manganese dioxide (MnO2) is widely used in nanocomposites for MTE regulation due to their pH responsiveness and excellent catalytic activity. MnO2 nanomaterials can catalyze excessive H2O2 in TME to generate oxygen to alleviate tumor hypoxia (73), and exhaust GSH excessively to reduce the antioxidant capacity of TME (74). In the presence of glucose oxidase (GOx), manganese dioxide can also catalyze the conversion of glucose into reactive oxygen species (ROS) through a cascade Fenton like reaction for hunger treatment of tumors (11). Based on these properties, MnO2 nanomaterials can significantly increase the generation of oxygen and ROS, which can relieve the hypoxia of TME and induce ROS associated damage of tumor cells, synergistically enhancing the efficiency of chemotherapy and photodynamic therapy (74).
Taking the above advantages, MnO2 nanomaterials can also act as a kind of novel immune immunomodulators by regulating different immune cells.
The levels of immunosuppressants are significantly increased under the condition of tumor hypoxia, such as adenosine, lactic acid and other substances that show inhibition for the activation and killing function of macrophages and cytotoxic immune cells (75–77). Murphy et al., designed a kind of PLGA-encapsulated MnO2 nanomaterials to enhance the cytotoxicity of NK cells against cancer cells by improving hypoxia and reducing immunosuppressants (78). Liang et al. also found that Gold Nanocages @ Manganese Dioxide (AuNC@MnO2) nanosystem could reverse the immunosuppressed TME by relieving tumor hypoxia through in situ oxygenation, and further enhance the recruitment of CD8+ T cells to tumor tissue for enhanced anti-tumor efficiency (79). These effects were found to be associated with the ability of AuNC@MnO2 to induce immunogenic cell death (ICD) to release damage related molecular patterns (DAMPs), and induce DC maturation and effector cell activation. In addition, MnO2 nanoparticles were also proved to act as a minimalist multimode vaccine adjuvant/delivery system to regulate antigen presenting cells for tumor immunotherapy (80). These results collectively suggested the attractive uses of MnO2 nanomaterials for potential immunotherapy.
4 The significance of MnO2 for cGAS-STING pathway
4.1 How Mn2+ involved in cGAS-STING pathway
cGAS-STING can regulate tumorigenesis, participate in autoimmunity and is also essential for the host defense against different kinds of pathogens (81). Thus, the active regulation of cGAS-STING signaling events is expected to develop novel therapeutics against tumor and infections. Mn2+ is the trace in cytoplasm, mitochondria and Golgi apparatus, and has a highly synergistic relationship with dsDNA ligands as a metal cofactor (82, 83). During virus infection, Mn2+ is released from membrane wrapped organelles and then accumulated into cytosol, which can further directly binds to cGAS in the cytosol, promoting the binding of cGAS and dsDNA to activate cGAS (84). After being activated, cGAS cyclizes ATP and GTP into cGAMP (the second messenger), and activates the innate immune response mediated by type I interferon (IFN-I) through interferon gene stimulator (STING). Thus, Mn2+ plays an important role in host defense by activating cGAS (82), which shows strong potential for the development of novel therapeutics and vaccines.
4.2 How MnO2 nanomaterials transform into Mn2+ in cells
MnO2 nanomaterials execute lots of their functions in the body in the form of Mn2+. As shown in Figure 2, when MnO2 nanomaterials enter into the acidic tumor microenvironment, they can be reduced to Mn2+ by the excessive H2O2 and GSH in the environment (85, 86). By using these two pathways, MnO2 nanomaterials can be transformed into Mn2+ to achieve multiple biological activities.
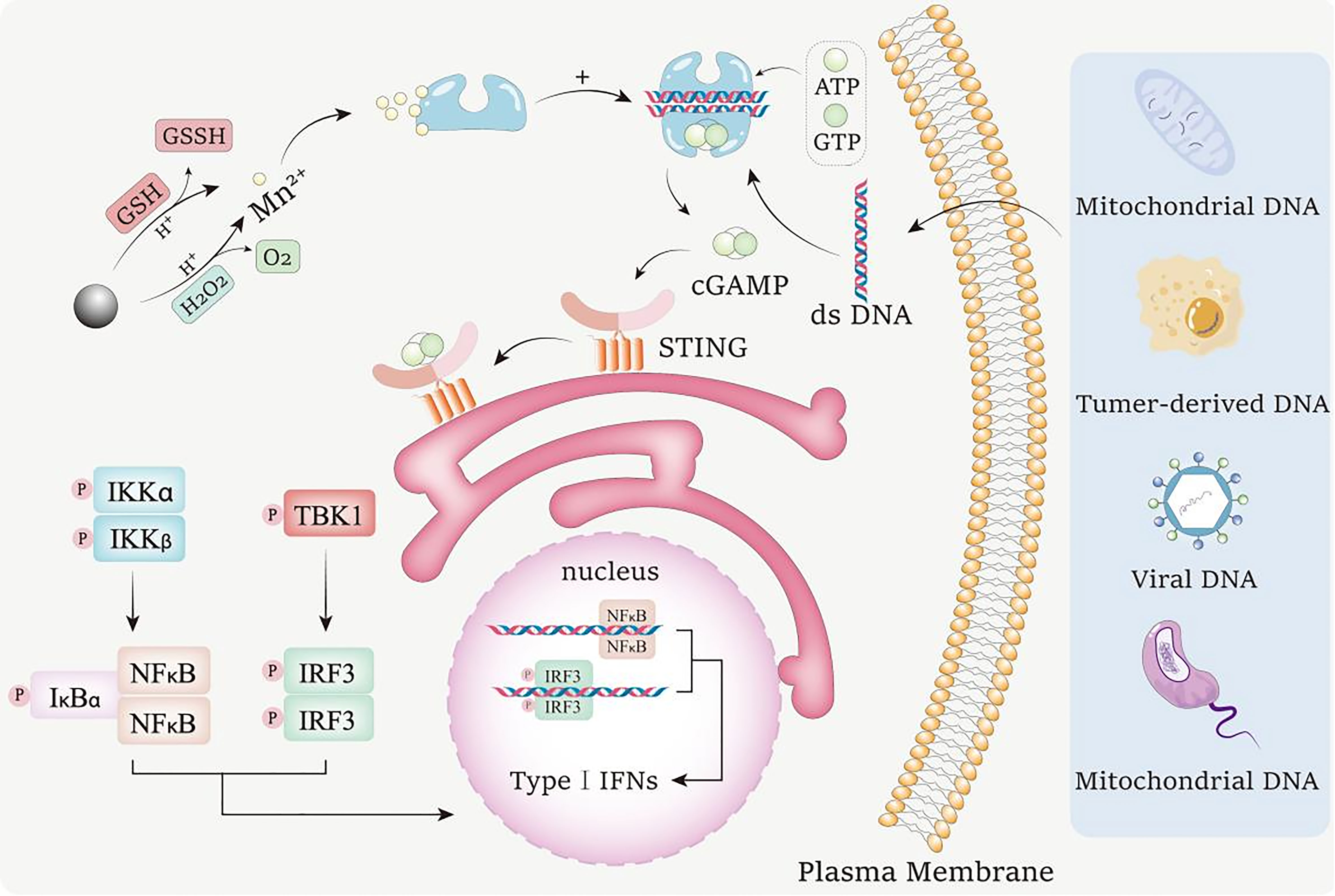
Figure 2 Mechanism for the conversion of MnO2 nanomaterials into Mn2+ to activate cGAS-STING pathway. MnO2 nanomaterials can undergo redox reactions with GSH and H2O2 in acidic tumor environments to consume excessive GSH and H2O2 in tumor environments. In response, Mn2+ is released, and the generated O2 can improve the tumor hypoxia state. Mn2+ enhances the ability of cGAS to recognize dsDNA from bacteria, tumor cells, viruses and mitochondria in the environment, and promotes the production of 2’,3’ - cyclic GMP AMP (2’,3’ - cGAMP). cGAMP binds to interferon gene stimulator (STING), resulting in tank binding kinase 1 (TBK1) dependent phosphorylation (P) of interferon regulator 3 (IRF3). The active IRF3 dimer transfers to the nucleus and activates the transcription of type I interferon gene. STING can also activate IκB kinase (IKK), phosphorylated NF-κB Inhibitory Protein IκB α, Lead to polyubiquitination and degradation, and then NF-κB can enter the nucleus and start the transcription of immune stimulating genes.
The release of Mn2+ from MnO2 nanomaterials is a process of reduction. Firstly, MnO2 nanomaterials can act as an oxidant in the reaction with H2O2 (87). MnO2 oxidizes H2O2 to O2 and generates MnOOH, which is an intermediate in the process of Mn2+ generation. Then, MnOOH is also used as an oxidant to oxidize H2O2 into H2O and O2 under an acidic environment, while MnOOH itself is reduced into Mn2+. When H2O2 is insufficient in the reaction system, MnOOH can undergo a disproportionation reaction under acidic conditions to generate tetravalent MnO2 and Mn2+. The reaction formulas during the reactions between MnO2 and H2O2 are as shown as following:
Moreover, MnO2 nanomaterials can also interact with GSH to release Mn2+. It has been widely reported that there is excessive GSH in the tumor microenvironment, which provides sufficient reductant for the reduction of MnO2 (88). Under acidic conditions, GSH is oxidized to glutathione disulfide (GSSG) through mercaptan disulfide exchange, while MnO2 is reduced to Mn2+ (89). The reaction formula during the reaction between MnO2 and GSH are as shown as following:
The tumor microenvironment provides acidic conditions for the above series of reactions. These Mn2+ released from MnO2 can participate in the subsequent immune responses for tumor inhibition, and the hypoxia conditions in the tumor microenvironment are also improved while the GSH level is downregulated, which makes the tumor more sensitive to ROS-mediated inhibition.
4.3 Mechanisms of Mn2+ activation of cGAS-STING pathway
Mn2+ can enhance the nucleotide transferase (NTase) activity of cGAS to substrate, especially the homologous substrate ATP/GTP. The non-homologous substrates ATP/ATP and GTP/GTP can also be cyclized when cGAS binds to short dsDNA, but the products can’t activate STING. When cGAS binds to long dsDNA, the homologous substrate ATP/GTP is cyclized into cGAMP to activate STING and induce subsequent signal transmission. This method, which depends on the length of cytoplasmic dsDNA, is the key to measuring stress levels by cGAS. Long dsDNA (≥ 50 base pairs (bps)) always indicates disease status, such as the presence of viral genome or mislocation of mitochondrial dsDNA, while shorter segments always indicate slight DNA damage or degraded viral genome (82). Mn2+ can combine with cGAS to improve the sensitivity of cGAS to dsDNA, so that cGAS can recognize and combine with dsDNA in low dsDNA concentration environment, and enhance the binding rate of cGAS and dsDNA (90, 91). This ability reduces the requirement of dsDNA concentration for the activation of cGAS, and improves the activation efficiency of cGAS. Manganese also has positive effects on the length dependence of dsDNA to reduce the length dependence of cGAS activation on dsDNA by directly enhancing the catalytic activity. This reduces the effect of dsDNA length on the cGAS activation intensity (82)]. In disease environments that require high levels of cGAS activation, such as tumor environments, this function of Mn2+ has great potential in the immunotherapy of diseases.
More interestingly, Mn2+ can activate cGAS alone in the absence of dsDNA, and the maximum activation activity in the absence of dsDNA in the system is equivalent to that induced by dsDNA. Thus, Mn2+ can directly combine with cGAS to activate cGAS signalings in a DNA independent manner with different mechanisms (83). When dsDNA binds to cGAS, the dimerization and oligomerization of cGAS are critical to the activation of cGAS. However, the cGAS activated by Mn2+ is independent of the typical dimerization triggered by dsDNA. Intact DNA-cGAS interactions, mediated by both the N-terminal R/K-rich region and the DNA-binding sites at the C-terminal male abnormal 21 (Mab21) domain, are critical for cGAS activation in cells. However, Mn2+ can also activate cGAS in mutants lacking both of these terminals. Structural analysis shows that the conformational changes of cGAS are similar to those of dsDNA induced cGAS activation, but shows unique η1 Helix to expand the catalytic pocket, allowing substrate entry and cGAMP synthesis (83). This means that Mn2+- cGAS has experienced a conformational change similar to that of dsDNA -cGAS, but shows differences in the unique η1 helix formed in Mn2+ activated cGAS. This structure leads to a significant widening of the entrance of the catalytic pocket, making the active site close to the substrate. However, the tightness of cGAS binding to Mn2+ is 85 times lower than that in the presence of dsDNA (82).
The way that Mn2+ activates cGAS to synthesize cGAMP is also different from that of dsDNA. In the presence of Mn2+, cGAS synthesizes cGAMP by a unique mechanism. In the process of cGAMP synthesis, there will be the formation of intermediate products, linear dinucleotide pppGpG. In the cGAS-Mn2+- pppGpG ternary complex, compared with the dsDNA activated cGAS, 5’pppG (2’5’) pG binds to cGAS in the opposite direction. Two guanine residues are linked by a 2’5’phosphate bond with the first and the second guanine adapting anti and syn conformation. In the structure of cGAS-Mn2+- pppGpG, the two cations (Mn2+) are only coordinated by the triphosphate group of the bound dinucleotide, independent of the catalytic triad residues (Asp211, Glu213 and Glu307) required by mcGAS-dsDNA pppGpG. Unlike the previously reported structures of DNA-activated cGAS, in cGAS-Mn2+ 5’pG(2’5’)pA and cGAS-Mn2+- c[G(2’5’)pA(3’5’)p], the adenine residues of both dinucleotides stacked with Tyr421, whereas the guanines located at the opposite side, which implies that the linear intermediate would not have to flip over within the catalytic pocket before being cyclized to accelerate the overall catalytic activity of cGAS. And by binding with cGAS to synthesize cGAMP, Mn2+ can enhance the surface STING function of the endoplasmic reticulum, then further enhance IRF3 phosphorylation, leading to the amplification of STING signal cascade and the production of type I IFNs (92).
4.4 MnO2 nanoparticles based therapeutic strategies by manipulating cGAS-STING pathway
Tumor remains a substantial challenge to human health with millions of deaths every year worldwide. Stimulator of interferon genes (STING) signal activation is a significant component to enhance innate immunity, which has been used to realize broad-spectrum immunotherapy. Thus, cGAS-STING pathway has been regarded as one of the most promising targets for tumor therapy, but how to develop an effective strategy to control cGAS-STING pathway for tumor therapy is still a big challenge.
Different forms of manganese dioxide nanocomposites have shown excellent performance in the three parts of cancer treatment, including chemotherapy, phototherapy, and collaborative treatment (93). In the field of cancer treatment, manganese dioxide nanomaterials show superior advantages and unprecedented performance. Mn2+ containing nanomaterials is expected to directly activate cGAS-STING signaling. For example, Wang et al. described a bovine serum albumin (BSA)/ferritin-based nanoagonist incorporating Mn2+, which could activate cGAS-STING signaling in dendritic cells (DCs) to elicit robust adaptive anti-tumor immunity. Mn2+ could enhance the sensitivity of cGAS to dsDNA and augment STING signaling, which could further enhance the tumor-specific T cell-mediated immune response against poorly immunogenic solid tumors in vivo, offering a robust approach for immunotherapy in the clinics. However, Mn2+ is not stable and very easily to be oxidized, which requires more stable Mn containing nanoparticles that can steadily provide Mn2+ for effective activation of cGAS-STING pathway.
Taking the advantages of high stability and biocompability, MnO2 nanoparticles have been demonstrated to show strong anti-tumor activities by releasing manganese ions in the tumor environment to enhance the activation of cGAS-STING pathway. This promising cGAS-STING regulating function is widely used for the development of various therapeutic anti-tumor strategies based on MnO2 nanomaterials. For example, Song et al. built a MnO2 contained M@P@HA nanoparticles as novel cGAS-STING amplifiers to enhance the innate immunotherapy against tumor (94). These M@P@HA nanoparticles, with photodynamic therapy (PDT) reagent protoporphyrin (PpIX) loading, could generate ROS-mediated disruption of cellular redox homeostasis and cytoplasm leakage of damaged mitochondrial double-stranded (ds) DNA under laser irradiation. Moreover, the M@P@HA nanoparticles can be decomposed for MnO2 exposure, which could interact with the intracellular GSH to release Mn2+. These released Mn2+ could significantly activate cGAS and increase the activity of the related proteins of cGAS-STING signaling to further amplify the anti-tumor immunity of tumor-associated macrophages (TAM). They also found that, by amplifying the cGAS-STING signaling of tumor tissue and activating innate immunity, this nanosystem can also further activate CD8+ T cell infiltration even in a tumor with low immunogenicity for synergistic tumor therapy.
MnO2 nanomaterials have been widely used to play a role as biocompatible nano carriers, which can be combined with their cGAS-STING activation for more effective tumor treatment. Lu et al. reported an HMnMPH nanoplatform consisting of hollow manganese dioxide (HMn) loaded with STING agonist (MSA-2), CRISPR-Cas9/sg-PD-L1 plasmid and hyaluronic acid (HA) (95). In tumor microenvironment, HMnPMH can be degraded to release Mn2+ and STING agonists, which can promote the release of type I interferon and proinflammatory factors by continuous activation of cGAS-STING pathway. CRISPR-Cas9 plasmid released by the nanosystem can also relieve PD-L1 immune checkpoint and reactivate immunosuppressive T cells, which can trigger long-term immunotherapy by combining with the cGAS-STING pathway mediated innate immunity activation. Du et al. also prepared a pH/glutamate responsive drug loading hollow manganese dioxide (H-MnO2) - based chlorine6 (Ce6) - modified DNAzyme thermal nanosystem to combine gene therapy and immunotherapy (96). The biodegradation of H-MnO2 releases DNase as PD-L1 mRNA targeting reagents to activate gene therapy with the assistance of DNase cofactors. The released Mn2+ is also involved in tumor immunotherapy through immune activation of the cGAS-STING pathway. And ROS produced by photosensitizers Ce6 and GA could further lead to immunogenic cell Death for more effective tumor inhibition. Yang et al. created an intelligent biodegradable hollow manganese dioxide (H-MnO2) nano-platform (97). H-MnO2 with hollow structure can be loaded with anti-tumor drugs, and can be used for specific imaging and on-demand drug release in tumor microenvironment (TME), and can also be used to regulate hypoxia TME to enhance cancer treatment.
Similarly, Du et al. reported a nanoplatform naming CA-1@HMnO2/HA-P-mAb for potential cancer chemoimmunotherapy (98). MAb, an antibody against Neuropilin-1 (Nrp-1) on regulatory T cells (Tregs), endows the nanosystem Tregs targeting effects to avoid the transmission of inhibitory signals to T cells. MnO2 inside the nanosystem can deplete the overexpressed GSH in tumor cells to produce Mn2+, which can activate the cGAS-STING pathway in 4T1 cells and up regulate the expression of IFN to promote dendritic cell maturation and tumor specific antigen presentation. Similar to curcumin (Cur), CA-1 has the characteristics of inducing tumor death through chemotherapy to enhance tumor immunogenicity, and effectively recruit CD4+T helper cells (Th) and CD8+ cytotoxic T lymphocytes (CTL) for the enhancement of anti-tumor immune responses.
MnO2 nanomaterials can also regulate the anoxic tumor microenvironment, which can be combined with the activation of cGAS-STING pathway for more effective anti-tumor treatment. Liu et al. reported the biomineralized manganese oxide nanoparticles (bio-MnO2 nanoparticles) prepared by mild enzymatic reaction (99). Bio-MnO2 nanoparticles can react with endogenous H2O2 to produce O2 and catalyze ROS production in tumors, thereby improving the radiosensitivity of NSCLC cells. At the same time, the released Mn2+ activates the activity of cGAS-STING pathway, promotes the death of immunogenic cells, and increases the infiltration of cytotoxic T cells. These results indicate that MnO2 nanoparticles also play an important role in RT induced anti-tumor immune response in non-small cell lung cancer (NSCLC). Zhao et al. prepared a MnO2 coated gold nanorods (GNRs) with silicon dioxide (SiO2) covering, and further camouflaged with myeloid suppressor cells (MDSCs) membrane to form GNRs@SiO2 @ MnO2@MDSCs (GSMM) (18). MnO2 can release Mn2+ in acidic micro tumor environment, which catalyzes H2O2 into O2 for anoxic tumor microenvironment remission and ·OH for CKT (chemical kinetics therapy). Moreover, Mn2+ can activate cGAS-STING to induce the secretion of type I interferon, proinflammatory cytokines and chemokines. At the same time, the NIR-II window photothermal imaging and photoacoustic imaging of GSMM are realized through the local surface plasmon resonance of MnO2 coating and GNRs in the near-infrared II window. PTT and CDT mediated tumor cell immunogenic cell death can further enhance anti-tumor immunity.
Cancer vaccines are viewed as one of the most promising immunotherapy strategies to eradicate malignant tumors by eliciting tumor-specific immune responses. However, the development of cancer vaccines is significantly restricted by the low immunogenicity of antigens and invalidation of adjuvants. To develop a novel cancer vaccine, Tang et al. designed a TME responsive MnO2 melittin nanoparticles (M-M NPs) to consume GSH via Fenton-like reaction (100). The released Mn2+ could further activate cGAS-STING signaling and promote the maturation of antigen-presenting cells to elicit systemic anti-tumor immune responses, such as the augmentation of tumor-specific T cells and pro-inflammatory cytokines/chemokines production. More importantly, M-M NPs could also promote the MHC-I cross-dressing by DCs to prime tumor-specific CD8+ T cells. These results proposed a strategy to enhance the cancer vaccine efficiency which showed great therapeutic effect on tumor immunotherapy.
MnO2 nanomaterials can also act as immune adjuvants and synergists for tumor treatment. Li et al. reported MnO2@MPDA-PEG nanoparticles, through the incorporation of MnO2 into polyethylene glycolate mesoporous polydopamine nanoparticles (MPDA-PEG nanoparticles) as a kind of mild photothermal effect assisted nanosystem (101). In colorectal tumors, nano drugs can release Mn2+ in the tumor microenvironment, which can be used as immune adjuvants to promote DC maturation and T cell activation. At the same time, it can improve the tumor microenvironment and make the tumor change from “cold” to “hot”, thus promoting the immunotherapy of aPD-L1. Mn2+, a magnetic resonance imaging (MRI) contrast agent for tumor imaging, can also induce BMDC maturation under mild photothermal treatment. This MnO2@MPDA-PEG nanosystem thus could be a promising dual-imaging theragnostic nanodrug to potentiate the systemic antitumor immunity. Song et al. used model antigen ovalbumin (OVA) to construct tumor vaccine OVA/MnO2 to test the mechanism and efficacy of MnO2 based tumor vaccine adjuvant/delivery system (80). As an advanced adjuvant pool, MnO2 nanoparticles can continuously release Mn2+ and enhance the immune response through the cGAS-STING pathway in DCs. These MnO2 nanoparticles can also be used as an ideal delivery system to deliver antigens to the cytoplasm of DCs to induce cellular immune response, indicating their promising roles as minimalist multi-mode vaccine adjuvant/delivery system. Liang et al. constructed TDNeMnO2 complex by combing tetrahedral nanostructured DNA (TDNs) with MnO2 nanosheet to activate macrophages (102). TDNs and Mn2+ have synergistic effects in triggering cGAS-STING activation, production of Ifnb/Isgs, M1 polarization and antigen presentation, providing a new anti-tumor immunotherapy strategy based on STING mediated TAM reprogramming. Zheng et al. constructed a Mn-enriched photonic nanodrug (MnPB MnOx), which is made by integrating MnOx onto the surface of Mn-doped PB nanoparticles. All components of MnPB MnOx have biocompatibility and biodegradability, and sufficient Mn ensure efficient activation of cGAS-STING, thus activating innate immunity. MnPB enhances tumor hyperthermia under near-infrared radiation, and cooperates with MnOx which can catalyze the production of reactive oxygen species, further promoting the stimulation of immune response. In the system of bilateral in-situ 4T1 tumor-bearing mice, the innate and adaptive immunity has been successfully activated, and the local treatment can produce the function of inhibiting the systemic response of distant tumors has been confirmed (103).
5 Conclusion and perspectives
MnO2 nanomaterials have indicated strong potential to activate the cGAS-STING pathway for enhanced innate immunological responses. Here we discussed the excellent biological properties and preparation methods of MnO2 nanoparticles, as well as the importance of cGAS-STING signaling pathway in diseases and their specific mechanisms. Furthermore, we emphatically discussed how MnO2 nanomaterials activate cGAS by converting into Mn2+, as well as the application of MnO2 nanomaterials for disease treatment of diseases by mediating cGAS-STING pathway.
cGAS-STING signal pathway, a natural immune pathway that has attracted increasing attentions in recent decade, plays an important role in cellular immune responses. The ability of cGAS to recognize and combine with DNA can activate cGAS to catalyze the formation of cyclic dinucleotides (cGAMP) for the activation of the downstream cascade cGAS-STING signaling pathway, which can promote the transcription of type I interferon (IFN) (104). Type I IFNs can further promote DC maturation, antigen presentation, T cell activation, infiltration and other aspects, thus promoting adaptive immunity.
Mn2+ has been proved to show cGAS-STING activation activities as a novel cGAS agonist (81). Although Mn2+ based nanomaterials can be directly used to activate cGAS-STING signaling, their biomedical uses are dramatically restricted by their low stability and biocompatibility. MnO2 nanomaterials are a kind of manganes-based multifunctional biomedical materials with excellent physical, chemical and biological properties. Interestingly, as shown in Figure 2, MnO2 nanomaterials can be converted into Mn2+ by the reactions with intracellular H2O2 or GSH (105), which thus provide the possibilities for cGAS-STING signaling regulations using MnO2 nanomaterials.
The reserve of manganese is huge, the preparation of manganese based materials is simple, the cost is low, and its transportation and storage are very convenient. As an activator of the cGAS-STING pathway, Mn2+ can enhance the sensitivity of cGAS to detect DNA and promote the synthesis of cGAMP with a ten-thousand-fold efficiency, and enhance the binding ability of STING and cGAMP hundreds of times. Moreover, Mn2+ can directly activate cGAS without relying on dsDNA. Manganese-based materials can be used as drug carriers and improve the tumor environment, and enhance the efficacy of chemotherapy, phototherapy, and cooperative therapy on tumors.
Based on the ability to activate cGAS and promote cGAS-STING signaling pathway, MnO2 nanomaterials can be used for the development of chemotherapeutic synergists, immune adjuvants and vaccines. To enhance drug efficiency, MnO2 nanomaterials can act as drug carrier for targeted drug delivery, which could also result in the reduced side effects of drugs (106). Moreover, as shown in Figure 3, the cGAS-STING pathway activating functions of MnO2 nanomaterials could allow the use of MnO2 nanomaterials as synergists to further enhance the efficacy of drug treatment. Additionally, MnO2 nanomaterials can also serve as immune adjuvants to enhance the innate and adaptive immunity, which could further improve the development of more effective vaccines. And for its cargo loading roles, MnO2 nanomaterials can also be loaded with the active cargo of vaccine for targeted delivery of the vaccines, which therefore can enhance the efficiency of vaccines more effectively by combining with their adjuvant roles.
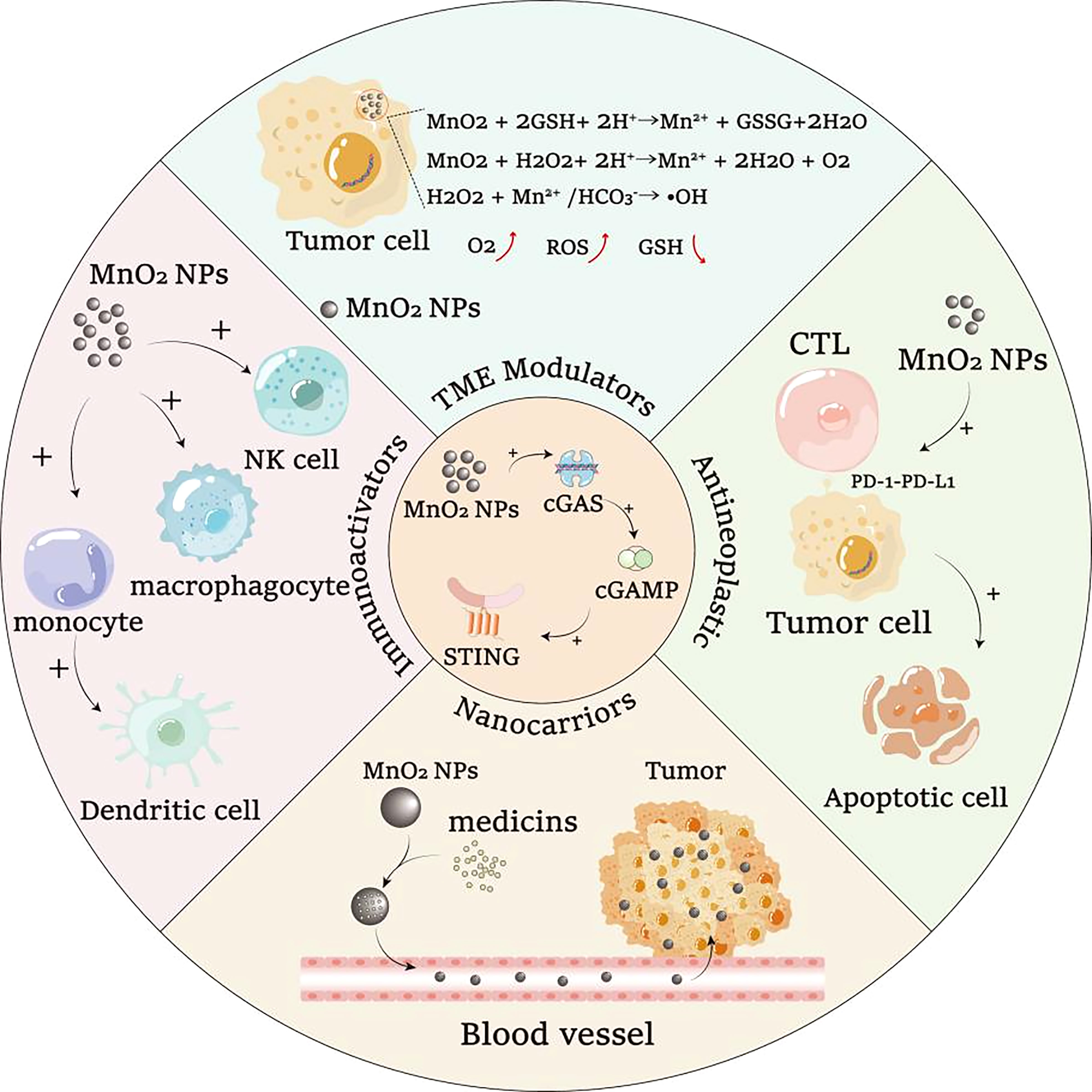
Figure 3 The ability of MnO2 nanomaterials to activate cGAS-STING signaling pathway can cooperate with other biological functions of MnO2 nanomaterials for potential synergistic treatment of diseases.
Up to now, most of the studies of MnO2 nanomaterials are focused on their anti-tumor application, with few of studies for anti-infectious treatments. In fact, as one of the most important innate immunological regulation pathways, cGAS-STING signaling plays critical roles in virus and bacteria infection by regulating both innate and adaptive immunity. Mn2+ has also been proved to show anti-viral activities by regulating cGAS-STING signalings (84). And due to the potent cGAS-STING activation ability, we believe that MnO2 nanomaterials could be served as novel cGAS agonist for anti-infectious therapeutic and vaccine development.
Moreover, although MnO2 nanomaterials have showed attractive potentials for the development of novel treatments and vaccines by regulating cGAS-STING pathway, there are still some critical issues, such as their cytotoxicity, immunogenicity and pharmacokinetic and metabolism, are still needed to be further investigated. Up to now, the systemic safety of MnO2 nanomaterials in vivo within a long duration, which is critical for the biological and medical application of MnO2 nanomaterials, remained to be further evaluated. Although the mechanisms of MnO2 nanomaterials to activate cGAS-STING pathway through the forms of Mn2+ has been depicted by different studies, the wide-range immunogenicity of MnO2 nanomaterials have not been well demonstrated. At present, the research of MnO2 nanomaterials is in the early stage, and stability is the key factor that should be considered in biological experiments. Whether the toxicity and degradation of MnO2 materials will be affected by their different forms and sizes, as well as their combination with different drugs and materials. To ensure the safety of further potential clinical applications, a detailed biological and biological safety assessment of MnO2 and its nanocomposites is required. Although there are still some unsolved problems, innovative applications based on MnO2 and its nanocomposites still have great potential. Moreover, the in vivo pharmacokinetic and metabolism of MnO2 nanomaterials, which are very important for the biosafety uses of MnO2 nanomaterials, are also needed to be further investigated in different animal models. The systemic studies of these issues would benefit our understandings of the biosafety for MnO2 nanomaterials and facilitate the development of novel MnO2 nanomaterials for clinical uses.
At present, STING agonists of CDNs also exist. When CDNs STING agonists are injected intravenously, they will be consumed at non-tumor sites and play a role in making STING abnormally excited in normal tissues, which may lead to inflammation or autoimmune diseases (107). Therefore, intratumoral injection is required, but this limits its immune function. MnO2 and its composite nano-materials can target tumors in the form of responding to the tumor microenvironment and loading and modifying targeted biological agents, and play a role in tumors. These characteristics enable MnO2 and its composite nano-materials to be injected intravenously and give full play to the immune function. At present, various reports on the activation of STING by MnO2 and its composite nanomaterials have shown good results. The multi-form and loading function of MnO2 and its composite nano-materials is a huge breakthrough in innovation, and there is a huge potential to replace traditional agonists and apply them in clinical practice.
In conclusion, MnO2 nanomaterials have demonstrated strong potentials as novel cGAS agonists to activate cGAS-STING pathway. Although lots of issues need to be further investigated and clarified, we believe that the promising activity of MnO2 nanomaterials for cGAS-STING regulation would benefit the development of novel treatments and vaccines against some important diseases, such as tumor and infection.
Author contributions
TZ, CH, WZ and YR drafted this manuscript and share first authorship. YM, DC, YHH, SF, WL, YFH, KL, and HL helped to revise the manuscript. J-FX, JP and XG helped to revise the manuscript and were responsible for leading this work. All authors contributed to the article and approved the submitted version.
Funding
This work was supported by National Natural Science Foundation of China (82272348, 82270013 and 81870016), Guangdong Basic and Applied Basic Research Foundation (2021A1515110843, 2022A1515010525 and 2022A1515011223), High Talent Project of Guangdong Province (2021QN02Y720), Characteristic Innovation Project of Universities in Guangdong Province (2021KTSCX038), Key Project of Universities in Guangdong Province (2022ZDZX2021), Innovation Team Project of Universities in Guangdong Province (2022KCXTD010), Funds for PHD researchers of Guangdong Medical University (GDMUB2021004 and 4SG22209G), Discipline Construction Project of Guangdong Medical University (4SG21229GDGFY01 and 4SG21279P), Open Research Fund of Songshan Lake Materials Laboratory (2021SLABFN10) and Open Research Fund for Key Laboratory of Tropical Disease Control Sun Yat-Sen University, Ministry of Education (2022kfkt01).
Acknowledgments
We tender our apologies to those authors whose deserving research was not cited in this manuscript.
Conflict of interest
The authors declare that the research was conducted in the absence of any commercial or financial relationships that could be construed as a potential conflict of interest.
Publisher’s note
All claims expressed in this article are solely those of the authors and do not necessarily represent those of their affiliated organizations, or those of the publisher, the editors and the reviewers. Any product that may be evaluated in this article, or claim that may be made by its manufacturer, is not guaranteed or endorsed by the publisher.
References
1. Zheng J, Mo J, Zhu T, Zhuo W, Yi Y, Hu S, et al. Comprehensive elaboration of the cGAS-STING signaling axis in cancer development and immunotherapy. Mol Cancer (2020) 19:133. doi: 10.1186/s12943-020-01250-1
2. Carty M, Guy C, Bowie AG. Detection of viral infections by innate immunity. Biochem Pharmacol (2021) 183:114316. doi: 10.1016/j.bcp.2020.114316
3. Wan D, Jiang W, Hao J. Research advances in how the cGAS-STING pathway controls the cellular inflammatory response. Front Immunol (2020) 11:615. doi: 10.3389/fimmu.2020.00615
4. Hopfner K-P, Hornung V. Molecular mechanisms and cellular functions of cGAS-STING signalling. Nat Rev Mol Cell Biol (2020) 21:501–21. doi: 10.1038/s41580-020-0244-x
5. Ma Z, Damania B. The cGAS-STING defense pathway and its counteraction by viruses. Cell Host Microbe (2016) 19:150–8. doi: 10.1016/j.chom.2016.01.010
6. Decout A, Katz JD, Venkatraman S, Ablasser A. The cGAS–STING pathway as a therapeutic target in inflammatory diseases. Nat Rev Immunol (2021) 21:548–69. doi: 10.1038/s41577-021-00524-z
7. Wu Q, Mu Q, Xia Z, Min J, Wang F. Manganese homeostasis at the host-pathogen interface and in the host immune system. Semin Cell Dev Biol (2021) 115:45–53. doi: 10.1016/j.semcdb.2020.12.006
8. Erikson KM, Aschner M. Manganese: Its role in disease and health. Met Ions Life Sci (2019) 19. doi: 10.1515/9783110527872-016
9. Xu W, Qing X, Liu S, Chen Z, Zhang Y. Manganese oxide nanomaterials for bacterial infection detection and therapy. J Mater Chem B (2022) 10:1343–58. doi: 10.1039/d1tb02646a
10. Yang G, Ji J, Liu Z. Multifunctional MnO2 nanoparticles for tumor microenvironment modulation and cancer therapy. Wiley Interdiscip Rev Nanomed Nanobiotechnol (2021) 13:e1720. doi: 10.1002/wnan.1720
11. Zhu C, Ma Q, Gong L, Di S, Gong J, Wang Y, et al. Manganese-based multifunctional nanoplatform for dual-modal imaging and synergistic therapy of breast cancer. Acta Biomater (2022) 141:429–39. doi: 10.1016/j.actbio.2022.01.019
12. Cui C, Wang S, Lu W, Wang Y, Li J, Qu K, et al. The adjuvanticity of manganese for microbial vaccines via activating the IRF5 signaling pathway. Biochem Pharmacol (2021) 192:114720. doi: 10.1016/j.bcp.2021.114720
13. Sun Z, Wang Z, Wang T, Wang J, Zhang H, Li Z, et al. Biodegradable MnO-based nanoparticles with engineering surface for tumor therapy: Simultaneous fenton-like ion delivery and immune activation. ACS Nano (2022) 16:11862–75. doi: 10.1021/acsnano.2c00969
14. Li Z, Chu Z, Yang J, Qian H, Xu J, Chen B, et al. Immunogenic cell death augmented by manganese zinc sulfide nanoparticles for metastatic melanoma immunotherapy. ACS Nano (2022) 16:15471–83. doi: 10.1021/acsnano.2c08013
15. Rozenberg JM, Kamynina M, Sorokin M, Zolotovskaia M, Koroleva E, Kremenchutckaya K, et al. The role of the metabolism of zinc and manganese ions in human cancerogenesis. Biomedicines (2022) 10:1072. doi: 10.3390/biomedicines10051072
16. Lv M, Chen M, Zhang R, Zhang W, Wang C, Zhang Y, et al. Manganese is critical for antitumor immune responses via cGAS-STING and improves the efficacy of clinical immunotherapy. Cell Res (2020) 30:966–79. doi: 10.1038/s41422-020-00395-4
17. Zhang R, Wang C, Guan Y, Wei X, Sha M, Yi M, et al. Manganese salts function as potent adjuvants. Cell Mol Immunol (2021) 18:1222–34. doi: 10.1038/s41423-021-00669-w
18. Zhao Y, Pan Y, Zou K, Lan Z, Cheng G, Mai Q, et al. Biomimetic manganese-based theranostic nanoplatform for cancer multimodal imaging and twofold immunotherapy. Bioact Mater (2023) 19:237–50. doi: 10.1016/j.bioactmat.2022.04.011
19. Sun X, Zhang Y, Li J, Park KS, Han K, Zhou X, et al. Amplifying STING activation by cyclic dinucleotide-manganese particles for local and systemic cancer metalloimmunotherapy. Nat Nanotechnol (2021) 16:1260–70. doi: 10.1038/s41565-021-00962-9
20. Hou L, Tian C, Yan Y, Zhang L, Zhang H, Zhang Z. Manganese-based nanoactivator optimizes cancer immunotherapy via enhancing innate immunity. ACS Nano (2020) 14:3927–40. doi: 10.1021/acsnano.9b06111
21. Yu X, Zhao Z, Jiang Z. Recent progress on the activation of the cGAS-STING pathway and its regulation by biomolecular condensation. J Mol Cell Biol (2022) 14:6. doi: 10.1093/jmcb/mjac042
22. Song W, Anselmo AC, Huang L. Nanotechnology intervention of the microbiome for cancer therapy. Nat Nanotechnol (2019) 14:1093–103. doi: 10.1038/s41565-019-0589-5
23. Ouyang J, Xie A, Zhou J, Liu R, Wang L, Liu H, et al. Minimally invasive nanomedicine: nanotechnology in photo-/ultrasound-/radiation-/magnetism-mediated therapy and imaging. Chem Soc Rev (2022) 51:4996–5041. doi: 10.1039/D1CS01148K
24. He T, Jiang C, He J, Zhang Y, He G, Wu J, et al. Manganese-Dioxide-Coating-Instructed plasmonic modulation of gold nanorods for activatable duplex-Imaging-Guided NIR-II photothermal-chemodynamic therapy. Adv Mater (2021) 33:e2008540. doi: 10.1002/adma.202008540
25. Ming L, Song L, Xu J, Wang R, Shi J, Chen M, et al. Smart manganese dioxide-based lanthanide nanoprobes for triple-negative breast cancer precise gene synergistic chemodynamic therapy. ACS Appl Mater Interfaces (2021) 13:35444–55. doi: 10.1021/acsami.1c08927
26. Liu J, Feng L, Wu Y. Enzymatically synthesised MnO2 nanoparticles for efficient near-infrared photothermal therapy and dual-responsive magnetic resonance imaging. Nanoscale (2021) 13:11093–103. doi: 10.1039/d1nr02400k
27. Oduro PK, Zheng X, Wei J, Yang Y, Wang Y, Zhang H, et al. The cGAS-STING signaling in cardiovascular and metabolic diseases: Future novel target option for pharmacotherapy. Acta Pharm Sin B (2022) 12:50–75. doi: 10.1016/j.apsb.2021.05.011
28. Du J-M, Qian M-J, Yuan T, Chen R-H, He Q-J, Yang B, et al. cGAS and cancer therapy: a double-edged sword. Acta Pharmacol Sin (2022) 43:2202–11. doi: 10.1038/s41401-021-00839-6
29. Joshi B, Joshi JC, Mehta D. Regulation of cGAS activity and downstream signaling. Cells (2022) 11:2812. doi: 10.3390/cells11182812
30. Hu M-M, Shu H-B. Innate immune response to cytoplasmic DNA: Mechanisms and diseases. Annu Rev Immunol (2020) 38:79–98. doi: 10.1146/annurev-immunol-070119-115052
31. Yu L, Liu P. Cytosolic DNA sensing by cGAS: regulation, function, and human diseases. Signal Transduct Target Ther (2021) 6:170. doi: 10.1038/s41392-021-00554-y
32. Wein T, Sorek R. Bacterial origins of human cell-autonomous innate immune mechanisms. Nat Rev Immunol (2022) 22:629–38. doi: 10.1038/s41577-022-00705-4
33. Eaglesham JB, Kranzusch PJ. Conserved strategies for pathogen evasion of cGAS-STING immunity. Curr Opin Immunol (2020) 66:27–34. doi: 10.1016/j.coi.2020.04.002
34. Smith JA. STING, the endoplasmic reticulum, and mitochondria: Is three a crowd or a conversation? Front Immunol (2020) 11:611347. doi: 10.3389/fimmu.2020.611347
35. Guo X, Hintzsche H, Xu W, Ni J, Xue J, Wang X. Interplay of cGAS with micronuclei: Regulation and diseases. Mutat Res Rev Mutat Res (2022) 790:108440. doi: 10.1016/j.mrrev.2022.108440
36. Hoong BYD, Gan YH, Liu H, Chen ES. cGAS-STING pathway in oncogenesis and cancer therapeutics. Oncotarget (2020) 11:2930–55. doi: 10.18632/oncotarget.27673
37. Mekers VE, Kho VM, Ansems M, Adema GJ. cGAS/cGAMP/STING signal propagation in the tumor microenvironment: Key role for myeloid cells in antitumor immunity. Radiother Oncol (2022) 174:158–67. doi: 10.1016/j.radonc.2022.07.014
38. Benmerzoug S, Ryffel B, Togbe D, Quesniaux VFJ. Self-DNA sensing in lung inflammatory diseases. Trends Immunol (2019) 40:719–34. doi: 10.1016/j.it.2019.06.001
39. Lazear HM, Schoggins JW, Diamond MS. Shared and distinct functions of type I and type III interferons. Immunity (2019) 50:907–23. doi: 10.1016/j.immuni.2019.03.025
40. Carrero JA. Confounding roles for type I interferons during bacterial and viral pathogenesis. Int Immunol (2013) 25:663–9. doi: 10.1093/intimm/dxt050
41. Zhang Q, Chen C, Xia B, Xu P. Chemical regulation of the cGAS-STING pathway. Curr Opin Chem Biol (2022) 69:102170. doi: 10.1016/j.cbpa.2022.102170
42. Luo W-W, Shu H-B. Delicate regulation of the cGAS-MITA-mediated innate immune response. Cell Mol Immunol (2018) 15:666–75. doi: 10.1038/cmi.2016.51
43. Hu Y, Chen B, Yang F, Su Y, Yang D, Yao Y, et al. Emerging role of the cGAS-STING signaling pathway in autoimmune diseases: Biologic function, mechanisms and clinical prospection. Autoimmun Rev (2022) 21:103155. doi: 10.1016/j.autrev.2022.103155
44. Zheng W, Xia N, Zhang J, Chen N, Meurens F, Liu Z, et al. How the innate immune DNA sensing cGAS-STING pathway is involved in autophagy. Int J Mol Sci (2021) 22:13232. doi: 10.3390/ijms222413232
45. Wischnewski M, Ablasser A. Interplay of cGAS with chromatin. Trends Biochem Sci (2021) 46:822–31. doi: 10.1016/j.tibs.2021.05.011
46. Liao W, Du C, Wang J. The cGAS-STING pathway in hematopoiesis and its physiopathological significance. Front Immunol (2020) 11:573915. doi: 10.3389/fimmu.2020.573915
47. Zhang X, Bai X-C, Chen ZJ. Structures and mechanisms in the cGAS-STING innate immunity pathway. Immunity (2020) 53:43–53. doi: 10.1016/j.immuni.2020.05.013
48. Ge Z, Ding S. Regulation of cGAS/STING signaling and corresponding immune escape strategies of viruses. Front Cell Infect Microbiol (2022) 12:954581. doi: 10.3389/fcimb.2022.954581
49. Lin J, Song T, Liu Z, Yang D, Xiang R, Hua W, et al. Effects of biodegradable biomedical porous MnO2 nanoparticles on blood components and functions. Colloids Surf B Biointerfaces (2022) 217:112667. doi: 10.1016/j.colsurfb.2022.112667
50. Deng Z, Li X, Liu H, He Y, Zhang J, Yuan J, et al. A nanoprobe for ratiometric imaging of glutathione in living cells based on the use of a nanocomposite prepared from dual-emission carbon dots and manganese dioxide nanosheets. Mikrochim Acta (2020) 187:537. doi: 10.1007/s00604-020-04495-1
51. Wu M, Hou P, Dong L, Cai L, Chen Z, Zhao M, et al. Manganese dioxide nanosheets: from preparation to biomedical applications. Int J Nanomedicine (2019) 14:4781–800. doi: 10.2147/IJN.S207666
52. Kuai X, Zhu Y, Yuan Z, Wang S, Lin L, Ye X, et al. Perfluorooctyl bromide nanoemulsions holding MnO2 nanoparticles with dual-modality imaging and glutathione depletion enhanced HIFU-eliciting tumor immunogenic cell death. Acta Pharm Sin B (2022) 12:967–81. doi: 10.1016/j.apsb.2021.07.025
53. Wang H, Wang W, Liu L, Wang M, Li G, Li H, et al. Biodegradable hollow polydopamine@manganese dioxide as an oxygen self-supplied nanoplatform for boosting chemo-photodynamic cancer therapy. ACS Appl Mater Interfaces (2021) 13:57009–22. doi: 10.1021/acsami.1c18601
54. Zheng J, Zhao H, Ning G, Sun W, Wang L, Liang H, et al. A novel affinity peptide-antibody sandwich electrochemical biosensor for PSA based on the signal amplification of MnO2-functionalized covalent organic framework. Talanta (2021) 233:122520. doi: 10.1016/j.talanta.2021.122520
55. Wu J, Williams GR, Niu S, Yang Y, Li Y, Zhang X, et al. Biomineralized bimetallic oxide nanotheranostics for multimodal imaging-guided combination therapy. Theranostics (2020) 10:841–55. doi: 10.7150/thno.40715
56. Amini MA, Abbasi AZ, Cai P, Lip H, Gordijo CR, Li J, et al. Combining tumor microenvironment modulating nanoparticles with doxorubicin to enhance chemotherapeutic efficacy and boost antitumor immunity. J Natl Cancer Inst (2019) 111:399–408. doi: 10.1093/jnci/djy131
57. Qiu H, Gong H, Bao Y, Jiang H, Tong W. Reactive oxygen species-scavenging hollow MnO2 nanozymes as carriers to deliver budesonide for synergistic inflammatory bowel disease therapy. Biomater Sci (2022) 10:457–66. doi: 10.1039/d1bm01525g
58. Fei JB, Cui Y, Yan XH, Qi W, Yang Y, Wang KW, et al. Controlled preparation of MnO2 hierarchical hollow nanostructures and their application in water treatment. Adv Mater (2008) 20:452–6. doi: 10.1002/adma.200701231
59. Guan J-F, Huang Z-N, Zou J, Jiang X-Y, Peng D-M, Yu J-G. A sensitive non-enzymatic electrochemical sensor based on acicular manganese dioxide modified graphene nanosheets composite for hydrogen peroxide detection. Ecotoxicol Environ Saf (2020) 190:110123. doi: 10.1016/j.ecoenv.2019.110123
60. Oaki Y, Imai H. One-pot synthesis of manganese oxide nanosheets in aqueous solution: chelation-mediated parallel control of reaction and morphology. Angew Chem Int Ed Engl (2007) 46:4951–5. doi: 10.1002/anie.200700244
61. Fang J, Wang Q, Yang G, Xiao X, Li L, Yu T. Albumin-MnO2 gated hollow mesoporous silica nanosystem for modulating tumor hypoxia and synergetic therapy of cervical carcinoma. Colloids Surf B Biointerfaces (2019) 179:250–9. doi: 10.1016/j.colsurfb.2019.03.070
62. Tehseen B, Rehman A, Rahmat M, Bhatti HN, Wu A, Butt FK, et al. Solution growth of 3D MnO2 mesh comprising 1D nanofibres as a novel sensor for selective and sensitive detection of biomolecules. Biosens Bioelectron (2018) 117:852–9. doi: 10.1016/j.bios.2018.06.061
63. Chen S, Zhu J, Wu X, Han Q, Wang X. Graphene oxide–MnO2 nanocomposites for supercapacitors. ACS Nano (2010) 4:2822–30. doi: 10.1021/nn901311t
64. Zhang Z, Ji Y. Nanostructured manganese dioxide for anticancer applications: preparation, diagnosis, and therapy. Nanoscale (2020) 12:17982–8003. doi: 10.1039/d0nr04067c
65. Xiao B, Zhou X, Xu H, Wu B, Hu D, Hu H, et al. Integration of polymerization and biomineralization as a strategy to facilely synthesize nanotheranostic agents. ACS Nano (2018) 12:12682–91. doi: 10.1021/acsnano.8b07584
66. Chen J, Meng H, Tian Y, Yang R, Du D, Li Z, et al. Recent advances in functionalized MnO2 nanosheets for biosensing and biomedicine applications. Nanoscale Horiz (2019) 4:321–38. doi: 10.1039/c8nh00274f
67. Marin E, Tapeinos C, Lauciello S, Ciofani G, Sarasua JR, Larrañaga A. Encapsulation of manganese dioxide nanoparticles into layer-by-layer polymer capsules for the fabrication of antioxidant microreactors. Mater Sci Eng C Mater Biol Appl (2020) 117:111349. doi: 10.1016/j.msec.2020.111349
68. Li Y, Zou Z, An J, Wu Q, Tong L, Mei X, et al. Chitosan-modified hollow manganese dioxide nanoparticles loaded with resveratrol for the treatment of spinal cord injury. Drug Delivery (2022) 29:2498–512. doi: 10.1080/10717544.2022.2104957
69. Wang X, Huang R, Wu W, Xiong J, Wen Q, Zeng Y, et al. Amplifying STING activation by bioinspired nanomedicine for targeted chemo- and immunotherapy of acute myeloid leukemia. Acta Biomater (2023) 157:381–94. doi: 10.1016/j.actbio.2022.11.007
70. Xiao T, He M, Xu F, Fan Y, Jia B, Shen M, et al. Macrophage membrane-camouflaged responsive polymer nanogels enable magnetic resonance imaging-guided Chemotherapy/Chemodynamic therapy of orthotopic glioma. ACS Nano (2021) 15:20377–90. doi: 10.1021/acsnano.1c08689
71. Zhang C, Tu W, Chen X, Xu B, Li X, Hu C, et al. Intelligent design of polymer nanogels for full-process sensitized radiotherapy and dual-mode computed tomography/magnetic resonance imaging of tumors. Theranostics (2022) 12:3420–37. doi: 10.7150/thno.70346
72. Terrén I, Orrantia A, Vitallé J, Zenarruzabeitia O, Borrego F. NK cell metabolism and tumor microenvironment. Front Immunol (2019) 10:2278. doi: 10.3389/fimmu.2019.02278
73. He T, Xu H, Zhang Y, Yi S, Cui R, Xing S, et al. Glucose oxidase-instructed traceable self-Oxygenation/Hyperthermia dually enhanced cancer starvation therapy. Theranostics (2020) 10:1544–54. doi: 10.7150/thno.40439
74. Deng X, Song Q, Zhang Y, Liu W, Hu H, Zhang Y. Tumour microenvironment-responsive nanoplatform based on biodegradable liposome-coated hollow MnO2 for synergistically enhanced chemotherapy and photodynamic therapy. J Drug Target (2022) 30:334–47. doi: 10.1080/1061186X.2021.1999961
75. Mastelic-Gavillet B, Navarro Rodrigo B, Décombaz L, Wang H, Ercolano G, Ahmed R, et al. Adenosine mediates functional and metabolic suppression of peripheral and tumor-infiltrating CD8+ T cells. J Immunother Cancer (2019) 7:257. doi: 10.1186/s40425-019-0719-5
76. Colegio OR, Chu N-Q, Szabo AL, Chu T, Rhebergen AM, Jairam V, et al. Functional polarization of tumour-associated macrophages by tumour-derived lactic acid. Nature (2014) 513:559–63. doi: 10.1038/nature13490
77. Ippolito L, Morandi A, Giannoni E, Chiarugi P. Lactate: A metabolic driver in the tumour landscape. Trends Biochem Sci (2019) 44:153–66. doi: 10.1016/j.tibs.2018.10.011
78. Murphy DA, Cheng H, Yang T, Yan X, Adjei IM. Reversing hypoxia with PLGA-encapsulated manganese dioxide nanoparticles improves natural killer cell response to tumor spheroids. Mol Pharm (2021) 18:2935–46. doi: 10.1021/acs.molpharmaceut.1c00085
79. Liang R, Liu L, He H, Chen Z, Han Z, Luo Z, et al. Oxygen-boosted immunogenic photodynamic therapy with gold nanocages@manganese dioxide to inhibit tumor growth and metastases. Biomaterials (2018) 177:149–60. doi: 10.1016/j.biomaterials.2018.05.051
80. Song T, Liao Y, Zuo Q, Liu N, Liu Z. MnO2 nanoparticles as a minimalist multimode vaccine adjuvant/delivery system to regulate antigen presenting cells for tumor immunotherapy. J Mater Chem B (2022) 10:3474–90. doi: 10.1039/d1tb02650j
81. Zhao X, Wang Y, Jiang W, Wang Q, Li J, Wen Z, et al. Herpesvirus-mimicking DNAzyme-loaded nanoparticles as a mitochondrial DNA stress inducer to activate innate immunity for tumor therapy. Adv Mater (2022) 34:e2204585. doi: 10.1002/adma.202204585
82. Hooy RM, Massaccesi G, Rousseau KE, Chattergoon MA, Sohn J. Allosteric coupling between Mn2+ and dsDNA controls the catalytic efficiency and fidelity of cGAS. Nucleic Acids Res (2020) 48:4435–47. doi: 10.1093/nar/gkaa084
83. Zhao Z, Ma Z, Wang B, Guan Y, Su X-D, Jiang Z. Mn2+ directly activates cGAS and structural analysis suggests Mn2+ induces a noncanonical catalytic synthesis of 2’3’-cGAMP. Cell Rep (2020) 32:108053. doi: 10.1016/j.celrep.2020.108053
84. Wang C, Guan Y, Lv M, Zhang R, Guo Z, Wei X, et al. Manganese increases the sensitivity of the cGAS-STING pathway for double-stranded DNA and is required for the host defense against DNA viruses. Immunity (2018) 48:675–687.e7. doi: 10.1016/j.immuni.2018.03.017
85. Li L, Xiao B, Mu J, Zhang Y, Zhang C, Cao H, et al. A MnO2 nanoparticle-dotted hydrogel promotes spinal cord repair via regulating reactive oxygen species microenvironment and synergizing with mesenchymal stem cells. ACS Nano (2019) 13:14283–93. doi: 10.1021/acsnano.9b07598
86. Lin L-S, Song J, Song L, Ke K, Liu Y, Zhou Z, et al. Simultaneous fenton-like ion delivery and glutathione depletion by MnO2 -based nanoagent to enhance chemodynamic therapy. Angew Chem Int Ed Engl (2018) 57:4902–6. doi: 10.1002/anie.201712027
87. Luo X-L, Xu J-J, Zhao W, Chen H-Y. A novel glucose ENFET based on the special reactivity of MnO2 nanoparticles. Biosens Bioelectron (2004) 19:1295–300. doi: 10.1016/j.bios.2003.11.019
88. Deng R, Xie X, Vendrell M, Chang Y-T, Liu X. Intracellular glutathione detection using MnO(2)-nanosheet-modified upconversion nanoparticles. J Am Chem Soc (2011) 133:20168–71. doi: 10.1021/ja2100774
89. Zhang K, Qi C, Cai K. Manganese-based tumor immunotherapy. Adv Mater (2022):e2205409. doi: 10.1002/adma.202205409
90. Wang C, Sun Z, Zhao C, Zhang Z, Wang H, Liu Y, et al. Maintaining manganese in tumor to activate cGAS-STING pathway evokes a robust abscopal anti-tumor effect. J Control Release (2021) 331:480–90. doi: 10.1016/j.jconrel.2021.01.036
91. Wang C, Zhang R, Wei X, Lv M, Jiang Z. Metalloimmunology: The metal ion-controlled immunity. Adv Immunol (2020) 145:187–241. doi: 10.1016/bs.ai.2019.11.007
92. Wang X, Liu Y, Xue C, Hu Y, Zhao Y, Cai K, et al. A protein-based cGAS-STING nanoagonist enhances T cell-mediated anti-tumor immune responses. Nat Commun (2022) 13:5685. doi: 10.1038/s41467-022-33301-0
93. Wen J, Yang K, Sun S. MnO2-based nanosystems for cancer therapy. Chem Commun (Camb) (2020) 56:7065–79. doi: 10.1039/d0cc02782k
94. Song W, Song S-J, Kuang J, Yang H, Yu T, Yang F, et al. Activating innate immunity by a STING signal amplifier for local and systemic immunotherapy. ACS Nano (2022) 16:15977–93. doi: 10.1021/acsnano.2c03509
95. Lu Q, Chen R, Du S, Chen C, Pan Y, Luan X, et al. Activation of the cGAS-STING pathway combined with CRISPR-Cas9 gene editing triggering long-term immunotherapy. Biomaterials (2022) 291:121871. doi: 10.1016/j.biomaterials.2022.121871
96. Du S, Chen C, Qu S, Song H, Yang J, Li Y, et al. DNAzyme-assisted nano-herb delivery system for multiple tumor immune activation. Small (2022) 18:e2203942. doi: 10.1002/smll.202203942
97. Yang G, Xu L, Chao Y, Xu J, Sun X, Wu Y, et al. Hollow MnO2 as a tumor-microenvironment-responsive biodegradable nano-platform for combination therapy favoring antitumor immune responses. Nat Commun (2017) 8:902. doi: 10.1038/s41467-017-01050-0
98. Du B, Bai Y, Jiao Q, Zhao M, Pang M, Ma H, et al. Simultaneous innate immunity activation and immunosuppression improvement by biodegradable nanoplatform for boosting antitumor chemo-immunotherapy. Chem Eng J (2022) 441:136093. doi: 10.1016/j.cej.2022.136093
99. Liu X, Kifle MT, Xie H, Xu L, Luo M, Li Y, et al. Biomineralized manganese oxide nanoparticles synergistically relieve tumor hypoxia and activate immune response with radiotherapy in non-small cell lung cancer. Nanomaterials (Basel) (2022) 12:3138. doi: 10.3390/nano12183138
100. Tang S, Zhou L, He H, Cui L, Ren Z, Tai Y, et al. MnO2-melittin nanoparticles serve as an effective anti-tumor immunotherapy by enhancing systemic immune response. Biomaterials (2022) 288:121706. doi: 10.1016/j.biomaterials.2022.121706
101. Li C, Li T, Niu K, Xiao Z, Huang J, Pan X, et al. Mild phototherapy mediated by manganese dioxide-loaded mesoporous polydopamine enhances immunotherapy against colorectal cancer. Biomater Sci (2022) 10:3647–56. doi: 10.1039/d2bm00505k
102. Liang S, Li J, Zou Z, Mao M, Ming S, Lin F, et al. Tetrahedral DNA nanostructures synergize with MnO2 to enhance antitumor immunity via promoting STING activation and M1 polarization. Acta Pharm Sin B (2022) 12:2494–505. doi: 10.1016/j.apsb.2021.12.010
103. Zheng Y, Chen J, Song X-R, Chang M-Q, Feng W, Huang H, et al. Manganese-enriched photonic/catalytic nanomedicine augments synergistic anti-TNBC photothermal/nanocatalytic/immuno-therapy via activating cGAS-STING pathway. Biomaterials (2023) 293:121988. doi: 10.1016/j.biomaterials.2022.121988
104. Kwon J, Bakhoum SF. The cytosolic DNA-sensing cGAS-STING pathway in cancer. Cancer Discovery (2020) 10:26–39. doi: 10.1158/2159-8290.CD-19-0761
105. Zhu D, Zhu X-H, Ren S-Z, Lu Y-D, Zhu H-L. Manganese dioxide (MnO2) based nanomaterials for cancer therapies and theranostics. J Drug Target (2021) 29:911–24. doi: 10.1080/1061186X.2020.1815209
106. Wang Z, Wu C, Liu J, Hu S, Yu J, Yin Q, et al. Aptamer-mediated hollow MnO2 for targeting the delivery of sorafenib. Drug Delivery (2023) 30:28–39. doi: 10.1080/10717544.2022.2149897
Keywords: MnO2 nanomaterials, manganese ions, cGAS agonist, cGAS-STING pathway, novel therapeutics
Citation: Zhang T, Hu C, Zhang W, Ruan Y, Ma Y, Chen D, Huang Y, Fan S, Lin W, Huang Y, Liao K, Lu H, Xu J-F, Pi J and Guo X (2023) Advances of MnO2 nanomaterials as novel agonists for the development of cGAS-STING-mediated therapeutics. Front. Immunol. 14:1156239. doi: 10.3389/fimmu.2023.1156239
Received: 01 February 2023; Accepted: 24 March 2023;
Published: 19 April 2023.
Edited by:
Chaofeng Han, Second Military Medical University, ChinaCopyright © 2023 Zhang, Hu, Zhang, Ruan, Ma, Chen, Huang, Fan, Lin, Huang, Liao, Lu, Xu, Pi and Guo. This is an open-access article distributed under the terms of the Creative Commons Attribution License (CC BY). The use, distribution or reproduction in other forums is permitted, provided the original author(s) and the copyright owner(s) are credited and that the original publication in this journal is cited, in accordance with accepted academic practice. No use, distribution or reproduction is permitted which does not comply with these terms.
*Correspondence: Jun-Fa Xu, eHVqdW5mYUBnZG11LmVkdS5jbg==; Jiang Pi, amlhbmdwaUBnZG11LmVkdS5jbg==; Xinrong Guo, Z3VveGluckBnZG11LmVkdS5jbg==
†These authors have contributed equally to this work