- 1Department of Laboratory Medicine, Peking University Third Hospital, Beijing, China
- 2Core Unit of National Clinical Research Center for Laboratory Medicine, Peking University Third Hospital, Beijing, China
- 3Institute of Medical Technology, Peking University Health Science Center, Beijing, China
APS (antiphospholipid syndrome) is a systematic autoimmune disease presenting with the high levels of aPLs (antiphospholipid antibodies). These autoantibodies are involved in various clinical manifestations, mainly including arterial or venous thrombosis formation, proinflammatory response, and recurrent pregnant loss. Pyroptosis is a form of lytic programmed cell death, and it aggravates autoimmune diseases progression via activating NOD-like receptors, especially the NLRP3 inflammasome and its downstream inflammatory factors IL (interleukin)-1β and IL-18. However, the underlying mechanisms of pyroptosis-induced APS progression remain to be elucidated. ECs (endothelial cells), monocytes, platelets, trophoblasts, and neutrophils are prominent participants in APS development. Of significance, pyroptosis of APS-related cells leads to the excessive release of proinflammatory and prothrombotic factors, which are the primary contributors to APOs (adverse pregnancy outcomes), thrombosis formation, and autoimmune dysfunction in APS. Furthermore, pyroptosis-associated medicines have made encouraging advancements in attenuating inflammation and thrombosis. Given the potential of pyroptosis in regulating APS development, this review would systematically expound the molecular mechanisms of pyroptosis, and elaborate the role of pyroptosis-mediated cellular effects in APS progression. Lastly, the prospective therapeutic approaches for APS would be proposed based on the regulation of pyroptosis.
1 Introduction
APS (antiphospholipid syndrome) is considered as a systemic autoimmune disease accompanied with inflammatory response, venous or arterial vascular thrombosis, recurrent fetal loss, and early pregnancy loss or pregnancy morbidity (1). Most of APS patients undergo multiple organs-systems injury due to the presence of at least one of serum aPLs (antiphospholipid antibodies), including aCL (anti-cardiolipin antibodies), anti-β2GPI (anti-beta2-glycoprotein I) or LAC (lupus anticoagulant) (1). At present, APS is mainly composed of PAPS (primary APS) and CAPS (catastrophic APS) (2). CAPS, as the variant of APS, is a rare, life-threatening disease with acute multisystem thrombosis and SIRS (systemic inflammatory response syndrome) (2). Besides, APS may occur in other systemic autoimmune diseases, particularly SLE (systemic lupus erythematosus) (3).
Pyroptosis is regarded as an essential innate immune defense against pathogens infection (4, 5). It has received growing attention due to its contributions to various diseases, mainly involving in gasdermins-mediated programmed death (6). Gasdermin superfamily is composed of gasdermin A/B/C/D/E (GSDMA/B/C/D/E) and DFNB59 (Pejvakin, PJVK) in human (7). Among these conserved proteins, GSDMD and GSDME are most extensively studied in pyroptosis (8, 9). Except DFNB59, other gasdermin members consist of the N-terminal PFD (pore-forming domain) and the C-terminal RD (repressor domain) (7). The interaction of PFD with RD maintains the oligomeric construct of gasdermins (10). Upon activated by endogenous and exogenous stimulus, the N-terminal PFD of gasdermins is separated from the C-terminal RD by caspases or granzymes, then the N-terminal PFD oligomerizes to form pores in the cell membrane, driving cell swelling and rupture, inflammatory molecules production, and pyroptotic cell death (11).
ECs (endothelial cells), neutrophils, monocytes, trophoblasts, and platelets are key players of APS progression. A great deal of evidence has showed that pyroptosis of these cells causes autoimmune disorders and accelerates diseases progression by triggering inflammasome activation and thrombosis formation, such as RA (rheumatoid arthritis) and SLE (12, 13). Nonetheless, the role of pyroptosis in promoting APS progression remains to be profoundly explored, which would reveal novel mechanisms for improving the treatment strategies of APS. Herein, this review would introduce the present molecular mechanisms of pyroptosis, and elucidate the contributions of pyroptosis of ECs, neutrophils, monocytes, trophoblasts, and platelets to APS development. In the last, the role of pyroptosis-related inhibitors in optimizing APS therapy would be exhibited.
2 Molecular mechanisms of pyroptosis
As we all known, pyroptosis is mainly composed of the caspase‐1-dependent canonical pathway and the caspase‐4/5/11-mediated non‐canonical pathway (14) (Figure 1). Canonical pyroptotic cell death is regulated by inflammasomes, like NLRP3 (NLR family, pyrin domain containing 3), NLRP1, NLRC4 (NLR family CARD domain containing 4), AIM2 (absent in melanoma 2), and pyrin, consequently augmenting GSDMD and caspase-1 cleavage, as well as IL-1β and IL-18 release (15). The assembly of inflammasomes is initiated by PRRs (pattern recognition receptors), which are capable of recognizing PAMPs (pathogen-associated molecular patterns) and DAMPs (danger-associated molecular patterns) to boost downstream proinflammatory cytokines release (16). Caspase-4/5/11-mediated noncanonical pyroptosis lacks the upstream sensors, but it can be directly activated by the binding of intracellular LPS (lipopolysaccharide) with the N-terminal CARD (caspase activation and recruitment domain), subsequently generating GSDMD pores and releasing matured IL-1β and IL-18 (17).
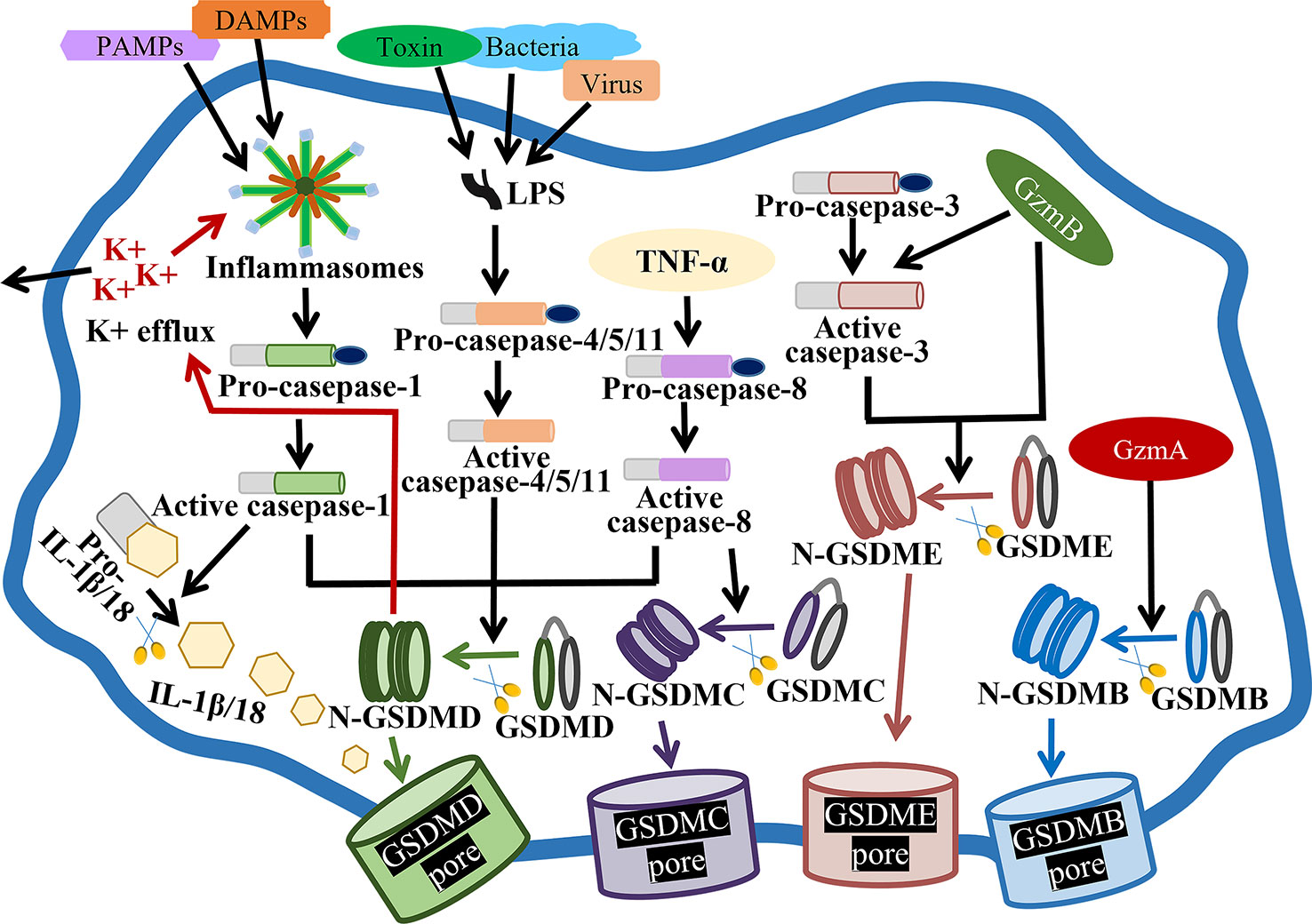
Figure 1 Molecular mechanisms of pyroptosis. In the canonical pathway, PAMPs and DAMPs receive intracellular signaling to stimulate inflammasomes and active caspase-1. Cleaved-caspase-1 cleaves GSDMD, pro-IL-1β and pro-IL-18. N-GSDMD perforates the cell membrane by forming nonselective pores, further causing cell lysis and death. In addition, IL-1β and IL-18 are secreted from the pores formed by N-GSDMD. In the noncanonical pathway, virus, bacteria or toxin-secreted LPS activates caspase-4, -5 and -11, triggering pyroptosis by cleaving GSDMD. In addition, the cleavage of GSDMD results in efflux of K+, ultimately mediating the assembly of NLRP3 inflammasome, resulting in the cleavage of pro-IL-1β and pro-IL-18. In the caspase-3-mediated pathway, active caspase-3 cleaves GSDME to form N-GSDME, inducing pyroptosis. In the caspase-8-mediated pathway, TNF-α treatment induces the activation of caspase-8, which cleaves GSDMD and GSDMC, resulting in pyroptosis. In the granzyme-mediated pathway, released GzmB rapidly activates caspase-3 and GSDME, causing extensive pyroptosis, and GzmB also directly activates GSDME. In addition, GzmA hydrolyzes GSDMB and directly activates GSDME.
Besides, apoptosis-related caspases, such as caspase-3 and -8, are thought to stimulate gasdermins-mediated pyroptosis (Figure 1). For example, caspase-3-mediated GSDME cleavage causes pyroptosis or secondary pyroptosis via generating a N-GSDME fragment and forming perforated membranes (18, 19). In mouse macrophages, caspase-8 functions as a regulator of pyroptosis, also contributing to GSDMD pores formation and pyroptotic cell death (20). As well, caspase-8 specifically lyses GSDMC to produce a N-GSDMC fragment, and generates pores on the cell membrane to induce pyroptosis (21).
Granzymes have been reported to kill target cells by inducing pyroptosis, such as GzmB (granzyme B) and GzmA (Figure 1). GzmB has been identified to activate caspase-3, then caspase-3 cleaves GSDME and induces GSDME-mediated extensive pyroptosis (22). Moreover, GzmB can directly cleave GSDME and cause pyroptosis (23). Additionally, lymphocytes-derived GzmA has been proved to cleave GSDMB at Lys229/Lys244 site, hydrolyze gasdermins at non-aspartic acid sites, form pores, stimulate pyroptosis and kill GSDMB-positive cells (24).
3 The role of pyroptosis in monocytes
Monocytes are key participants in natural immunity and host defense, engaging in aPLs-mediated inflammation, thrombosis, and autoimmune response (25). Monocytes dysfunction plays a prominent role in APS progression through producing inflammatory cytokines and TF (tissue factor) (26). Previously, pyroptosis has been reported as caspase-1-mediated monocytes death and is mainly detected in monocytes and macrophages. Hence, clarifying the underlying mechanisms of pyroptotic cell death of monocytes and macrophages is essential for alleviating APS development (Table 1).
During the process of canonical pyroptosis, activated monocytes release caspase-1, GSDMD, IL-1β and IL-18 (64). Moreover, the plasma membrane damage of monocytes and macrophages causes K+ efflux and NLRP3 activation, subsequently triggering pyroptosis and accelerating APS progression (65). Mechanically, NLRP3 inflammasome in monocytes is activated by cAbl kinase-mediated ASC phosphorylation at Y146 site and LPC (lysophosphatidylcholine) (27, 28), further promoting IL-18 and IL-1β release. Particularly, Abl kinase inhibitors provide a novel therapeutical intervention for APS by reducing ASC phosphorylation, further blocking monocytes pyroptosis and inflammatory cytokines release (27). In addition, NSA (necrosulfonamide) can directly bind with GSDMD at Cys191 site and prohibit p30-GSDMD pores formation in both human monocytes and macrophages, further blocking NLRP3- and pyrin-mediated pyroptotic cell death and IL-1β release. Most importantly, NSA does not affect other innate immune pathways or other cell death pathways, suggesting a promising therapeutical strategy for ameliorating inflammatory reactions in APS (66).
NLRC4, another canonical inflammasome, is reported to cooperate with NLRP3 to stimulate inflammation in human macrophages, indicating an unexpected overlap between distinct inflammasome scaffolds (29). Besides, monocytes infection activates AIM2 inflammasome, caspase-1 and GSDMD cleavage, and the expression level of GSDMD on the cell membrane and cytoplasm of monocytes is linked with the severity of infection (30). Concerning NLRP1 inflammasome, it is prominently located in the nucleus of monocytic THP-1 cells and is closely correlated with the genetical risk of several autoimmune diseases (31). Also, the assembly of NLRP1 inflammasome is facilitated by TLRs (toll-like receptors) activation, subsequently inducing caspase-1 activation and bioactive IL-1β secretion (31). Of significance, treatment of monocytes with SB (silibinin) downregulates NF-κB pathway and inactivates NLRP1/NLRP3 inflammasomes in pregnant women with PE (preeclampsia), suggesting a prospective therapeutical strategy for APS-related APOs via abrogating inflammation (67). In these pyroptotic monocytes and macrophages, canonical inflammasomes would trigger thrombosis and accelerate APS progression via promoting TF release. And the deficiency of caspase-1 or GSDMD is identified to block flow restriction–induced venous thrombosis (68), but also renders monocytes and macrophages insensitivity to pyroptotic cell death (69). These findings reveal that targeting canonical pyroptosis of monocytes might improve APS therapy.
Endotoxic LPS is an essential stimulator of noncanonical pyroptosis pathway. TLR4/MD-2 (myeloid differentiation-2) complex and caspase-4/11 are extracellular and intracellular LPS receptors, respectively. Upon stimulated by LPS, TLR4/MD-2 complex is highly expressed on the surface of macrophages, monocytes, and ECs, then directly interacting with the lipid A motif of LPS. Similarly, caspase-4/11 boosts monocytes pyroptosis by binding with diverse LPS variants (33). Additionally, the administration of LPS/Ng (LPS plus nigericin) strengthens TRAF3 (tumor necrosis factor receptor-associated factor 3)-modulated ULK1 (unc-51 like autophagy activating kinase 1) ubiquitin and degradation in THP-1 cells. Further, the downregulated ULK1 significantly induces AIF nuclear relocation and stimulates caspase-1 activation, consequently promoting IL-1β maturation and pyroptotic cell death (34). Presently, GSDMB has been confirmed to participate in autoimmune diseases. The highly expressed GSDMB not only promotes N-GSDMD cleavage and LDH (lactate dehydrogenase) release in monocytes, but also triggers caspase-4/GSDMD-dependent non-canonical pyroptosis by directly binding to the CARD domain of caspase-4. Therefore, GSDMB-mediated pyroptosis reveals a potential approach for relieving systemic inflammation in APS (35).
In particular, increasing clinical observations have detected the high levels of GSDMD or GSDME in several autoimmune diseases, and it is positively related with the disease activity. For instance, monocytes from RA patients are more susceptible to pyroptosis due to the higher GSDME level (36). Furthermore, TNF (tumor necrosis factor) treatment heightens pyroptosis of monocytes and macrophages by activating caspase-3/GSDME pathway. Caspase-3 inhibitor or silencing of GSDME dramatically inhibits TNF‐induced pyroptosis and alleviates arthritis, which might become a promising therapeutic method for APS-related inflammation (36). Likewise, another clinical evidence has shown that serum from RA patients promotes GSDMD-dependent pyroptosis of monocytes. Moreover, the coordination of PTX3 (pentaxin 3) with C1q remarkably enhances GSDMD-dependent pyroptosis by activating NLRP3 inflammasome, GSDMD and caspase-1 cleavage, and IL-6 release. Conversely, IL-6 strengthens PTX3 plus C1q-induced pyroptosis of monocytes, providing new insights for blocking pyroptosis-mediated persistent proinflammatory response in APS (32). In SLE patients, overexpressed GSDMD exerts a crucial function in the pathogenesis of monocytes and macrophages, and DSF (disulfiram) prominently represses GSDMD-mediated pyroptosis of THP-1 cells and relieves the disease severity (13).
Pyroptosis is a complicated formation of cell death, it is often accompanied by other biological processes, like apoptosis and necroptosis. Small molecule inhibitors of the serine peptidases DPP8 and DPP9 enhance pro-caspase-1 activation and GSDMD-regulated pyroptosis of monocytes and macrophages (37, 38). Meanwhile, caspase-1 induces apoptotic cell death in the absence of GSDMD via activating caspases-3 and -7 in monocytes. Further, caspases-3 and -7 cleave GSDMD at position D87 and hinder GSDMD-modulated pyroptosis, which shows a bidirectional crosstalk between pyroptosis and apoptosis pathways in monocytes and macrophages (37). On the other side, RIPK3 (receptor-interacting serine/threonine protein kinase 3)-mediated necroptosis and GSDMD-mediated pyroptosis collaborate to amplify inflammatory response in macrophages and ECs. Ablation of Ripk3 or Gsdmd efficiently prevents IL-1β maturation and release, revealing two potential targets for combined therapeutic interventions of APS (70).
4 The role of pyroptosis in neutrophils
Neutrophils are important leukocytes for the innate immune response, and they promote pyroptosis-mediated inflammation and thrombosis in APS patients (71). At the same time, pyroptosis of neutrophils stimulates neutrophils-mediated NETs (neutrophil extracellular traps) release and NETosis (concomitant cell death) (72). Further, NETs and NETosis have the capacity to affect autoimmunity, drive inflammatory cytokines production, as well as propelling thrombus formation by activating platelets and coagulation (73–75). Herein, pyroptosis-mediated signaling in neutrophils might execute a crucial function in APS pathology due to the pivotal roles of NETs and NETosis in thrombosis and inflammation (73–75) (Table 1).
In general, pyroptosis is initiated by intracellular pathogens infection, but the function of inflammasomes is different even between the two related immune cell lineages. For instance, canonical inflammasomes activation triggers caspase‐1-mediated macrophages pyroptosis, while they selectively activate caspase‐1/IL‐1β signaling without concomitant pyroptotic cell death in neutrophils (76, 77). Thereby, neutrophils might be resistant to pyroptosis and exhibit weaker GSDMD cleavage. In NLRP3 inflammasome-activated neutrophils, N-GSDMD is trafficked to azurophilic granules and neutrophils elastase is released into the cytosol, resulting in the secondary cleavage of GSDMD and mature IL-1β secretion, instead of accumulating N-GSDMD pores in the plasma membrane (78). Evidence has reported the resistant mechanisms of neutrophils pyroptosis (79). On the one side, the preserved mitochondrial membrane under active NLRP3 inflammasome leads to the pyroptosis resistance of neutrophils via mitochondria-dependent NLRP3 desensitization at DAMP-rich inflammatory region (77). The absence of neutrophils pyroptosis prolongs neutrophils’ lifespan, IL‐1β and IL‐18 production, and enables the clearance of microbial insult or cellular debris (76). On the other side, neutrophils might suppress SARM1 expression to subvert caspase‐1‐dependent pyroptosis, and the overexpressed SARM1 may stimulate caspase‐1‐dependent pyroptosis of neutrophils (80). Thereby, it would be relatively interesting to explore the contributions of neutrophils to inflammatory reactions in APS progression.
Despite the absence of GSDMD pores and the resistance of pyroptosis in neutrophils, several observations have detected pyroptotic cell death in neutrophils. For example, neutrophils can undergo pyroptotic cell death via assembling NLRC4 inflammasome and producing IL-1β (39). Another report suggests that infection induces neutrophils pyroptosis via ATP-mediated P2X7R signaling, resulting in cytoplasmic low K+ activation of NLRP3 inflammasome together with mature IL-1β and IL-18 release (40, 41). Furthermore, there is an interplay between apoptosis and pyroptosis. A study has found that RIPK1 can activate GSDME during bacterial infection. Then GSDME is cleaved by caspase-8, which does not only stimulate neutrophils lysis through activating extrinsic and intrinsic apoptosis, but also drive neutrophils pyroptosis (42).
Most significantly, pyroptosis may coordinate with NETosis to deteriorate the progression of autoimmune diseases (43, 81). It has been proved that NETosis can induce monocytes and macrophages pyroptosis to aggravate the secretion of inflammatory and prothrombotic factors, which might lead to plaque erosion and extensive thrombosis in APS patients (82). GSDMD, a pyroptosis executor, has been reported to facilitate NETs excretion from neutrophils. In noncanonical signaling, GSDMD is activated by intracellular LPS and cleaved by caspase-11, which plays a vital role in NETosis and TF release (43). For one thing, caspase-11 and GSDMD accelerate neutrophils plasma membrane rupture at the final stage of NETs release. For another thing, caspase-11 and GSDMD promote nuclear demodulation and DNA expansion at the early stage of NETosis (43). Fortunately, a pyrazolo-oxazepine scaffold–based molecule has been investigated to bind with GSDMD, further inhibiting NETosis (43). Accordingly, GSDMD inhibitors can be regarded as attractive therapeutical targets for forfending NETosis in APS.
5 The role of pyroptosis in ECs
Generally, pyroptosis of vascular ECs enhances vascular permeability and causes endothelial damage. This process would recruit more immune cells to adhere to the vascular wall, producing proinflammatory factors and promoting the formation of thrombotic plaques (83, 84). Current observations have demonstrated the key role of ECs pyroptosis in APS pathophysiology (Table 1).
ROS (reactive oxygen species) acts as the hub role in activating NLRP3 inflammasome and caspase-1-dependent canonical pyroptosis of ECs, and some environmental factors markedly stimulate ROS production. For example, treatment of human ECs with nicotine results in ROS/NLRP3/ASC activation, caspase-1/GSDMD-dependent pyroptosis and vascular endothelial injury (85). Acrolein, a common environmental pollutant, is linked with cardiovascular diseases, and exposure of HUVECs (human umbilical vein endothelial cells) to acrolein stimulates NLRP3 inflammasome assembly and pyroptosis via promoting ROS secretion in HUVECs (44). Cd (cadmium) is a crucial environmental pollutant associated with cardiovascular diseases, Cd-treated HUVECs increase ROS secretion and activate NLRP3 inflammasome, further strengthening pyroptosis of HUVECs (45). DBDPE (decabromodiphenyl ethane) is a novel environmental pollutant, it has been investigated to induce vascular endothelial injury and cardiovascular damage. DBDPE not only promotes ROS generation, but also causes NLRP3-mediated vascular ECs pyroptosis, as evidenced by the elevated NLRP3, ASC, and caspase-1 (46). As well, RCN2 (reticulocalbin-2) and SDHB (succinate dehydrogenase complex subunit B) are important regulators of ROS generation, mitochondrial injury, and ECs pyroptosis. And the inhibition of RCN2 or SDHB significantly attenuates pyroptosis, downregulates ROS and pyroptosis-related proteins (50, 51).
HMGB1 (high mobility group box 1) is a highly conserved and damage-related nuclear protein, the released HMGB1 can trigger proinflammatory response and canonical pyroptosis of ECs via activating RAGE (TLR4/advanced glycation end product)/cathepsin B/NLRP3 signaling (47). HDAC11 (histone deacetylase 11) is a class IV histone deacetylase, it promotes NLRP3/caspase-1/GSDMD-mediated vascular ECs pyroptosis under TNF-α treatment, and the HDAC11 inhibitor might prevent pyroptosis (48, 49). These canonical pyroptosis processes are characterized by the elevated caspase-1 cleavage, GSDMD expression, IL-1β and IL-18 production, LDH activity, and PI (propidium iodide) positive cells. And caspase-1 inhibitors can effectively repress pyroptosis, showing useful approaches for alleviating ECs damage-induced thrombosis in APS (85).
On the other side, the critical role of caspase-4/11 in modulating noncanonical ECs pyroptosis has been identified. In TNF-α-treated arterial ECs, activated caspase-4 and -11 simultaneously stimulate GSDMD cleavage and GSDME activity by binding with caspase-3. Both of the two pathways aggravate ECs pyroptosis and endothelial dysfunction, suggesting that caspase-4 and -11 are potential therapeutic targets of APS (52). Also, LPS-stimulated noncanonical ECs pyroptosis is dependent on the cleavage of caspase-11 and GSDMD, the formation of N-GSDMD pores on the mitochondria, and the release of mtDNA (mitochondrial DNA) (53). The interaction of LPS with HMGB1 would amplify caspase-11-dependent ECs pyroptosis (86). Besides, the upregulated HDAC11 triggers the activity of caspase-3 and GSDME via interacting with ERG (ETS-related gene), consequently decreasing ERG acetylation and inducing pyroptosis of TNF-α-treated HUVECs (49).
Accumulating studies have found that the inhibition of ECs pyroptosis can effectively mitigate ECs-mediated inflammation and thrombosis. Melatonin (N-acetyl-5-methoxytryptamine), a neuroendocrine hormone, is characterized with superior antioxidant properties. It has been utilized for forfending vascular ECs pyroptosis by improving mitochondrial function and reducing UQCRC1 (ubiquinol-cytochrome c reductase core protein 1) methylation (87). Interestingly, melatonin can regulate long noncoding RNA MEG3/miR-223/NLRP3 axis to prevent ECs pyroptosis and attenuate the expression of canonical pyroptosis-related molecules (88).
In addition, medicines extracted from natural materials have shown a great anti-pyroptotic value. Neferine is an alkaloid ingredient from the lotus seed embryo, it can reduce ROS generation and block LPS/ATP-induced ECs pyroptosis via inactivating ROS/NLRP3/caspase-1 signaling (89). DHM (dihydromyricetin), a natural flavonoid, exerts anti-oxidative and anti-inflammatory bioactivities. DHM markedly abrogates intracellular ROS and mtROS (mitochondrial ROS) generation, further blocking NLRP3-dependent pyroptosis in vascular ECs (90). SAL (salidroside) inhibits the activation of caspase-1, GSDMD, and IL-1β in HUVECs, indicating that SAL impedes aortic ECs pyroptosis (91). Colchicine, a classical nonspecific anti-inflammatory traditional drug, can alleviate cholesterol crystal-induced ECs pyroptosis through activating AMPK/SIRT1 (Sirtuin1) pathway (92).
6 The role of pyroptosis in trophoblasts
Trophoblasts, the key cells at the maternal-fetal interface, play an essential role in placenta implantation and reproduction. Pyroptosis of trophoblasts, a unique cell death pathway, occurs in the placenta to aggravate APS-related APOs, as characterized by the elevated proinflammatory cytokines, oxidative stress, and the highly-expressed pyroptosis-related proteins (54) (Table 1).
Exposure of trophoblasts to hypoxia elicits excessive UPR (unfolded protein response) activity and ER (endoplasmic reticulum) stress, but also impairs autophagy (54). Hyperactivated UPR upregulates TXNIP (thioredoxin-interacting protein) expression, boosting NLRP3 inflammasome, and subsequently promoting the production of active caspase-1, GSDMD, IL-18 and IL-1β (54). What’s more, attenuated autophagy triggers trophoblasts pyroptosis to expand inflammation and deteriorate APS-related APOs (54). In addition, several biomolecules implicate in modulating trophoblasts pyroptosis. For instance, HOXA9 boosts AMPK/TXNIP/NLRP3 inflammasome by directly increasing chemerin transcription in H/R (hypoxia/reoxygenation)-stressed trophoblasts, further hastening inflammation and pyroptosis of trophoblasts, and aggravating PE. The pathogenic role of chemerin might trigger APS-correlated APOs, providing an effective target for protecting against APOs (55).
Non-coding RNAs (ncRNAs) also exert prominent functions in inducing trophoblasts pyroptosis. A study has detected the increased lncRNA NEAT1 (nuclear paraspeckle assembly transcript 1) expression in LPS-treated trophoblasts, and it might participate in the development of APS-related APOs by inducing pyroptosis. Overexpressed lncRNA NEAT1 heightens TLR4 transcription by competitively binding to miR-302b-3p, and further enhancing LPS-induced trophoblasts pyroptosis together with the increased caspase-1 and N-GSDMD activity (57). MiR-124-3p is also related with trophoblasts pyroptosis, and its expression is increased in the placental tissues of PE patients. The upregulated miR-124-3p triggers pyroptosis by decreasing PLGF (placental growth factor) expression and enhancing ROS production accompanied by the upregulated NLRP3, caspase-1 and GSDMD (56). On the contrary, LINC00240 is lowly-expressed in the placenta tissues of PE patients. The downregulated LINC00240 aggravates oxidative stress-induced pyroptosis through increasing miR-155 and reducing Nrf2 expression, and LINC00240 overexpression mitigates the pyroptotic death of trophoblasts (58). Additionally, the lowly-expressed miR-520c-3p is observed in the placental tissues of PE patients and H/R-treated trophoblasts, and melatonin may suppress trophoblasts pyroptosis via modulating miR-520c-3p/SETD7 axis (93). Taken together, these ncRNAs are expected to be possible therapeutic targets for APS-correlated APOs treatment
Recently, trophoblasts pyroptosis has been reported to be modulated by glycolytic enzymes, showing the tightly relation of cellular pyroptosis with metabolic phenotypes. PFKFB3 (6-Phosphofructo-2-kinase/fructose-2,6-bisphosphatase 3) is a widely accepted glycolytic enzyme. Pharmacological MET (metformin) dramatically impedes TLR4/NF-κB/PFKFB3 signaling, which not only rectifies glycometabolic reprogramming and oxidative stress, but also represses NLRP3-induced trophoblasts pyroptosis. Thus, MET-alleviated pyroptosis is partly attributed to PFKFB3-dependent glycometabolism reprogramming and redox disorders, proposing MET as a potential therapeutic approach for APOs (59).
7 The role of pyroptosis in platelets
Platelets are small anucleate multifunctional blood cells, implicating in APS-correlated APOs via modulating coagulation, thrombosis, inflammation, and innate immunity. Most importantly, inflammasomes activation in platelets is a key participant in upregulating pore-forming proteins and inducing pyroptotic cell death by expressing TLRs (94) (Table 1).
Observations have found that platelets pyroptosis is mainly modulated by TLR4/ROS activation. For instance, sepsis-derived S100A8/A9 induces platelets pyroptosis in a GSDMD-dependent manner via boosting TLR4/ROS/NLRP3/caspase-1 pathway, leading to the release of oxidized mtDNA and the formation of NETs. NETs in turn release S100A8/A9 and accelerate platelets pyroptosis, forming a positive feedback loop to amplify proinflammatory cytokines production in APS (60). HMGB1 also boosts NLRP3 inflammasome and its adaptor molecule ASC via activating TLR4/ROS signaling, resulting in platelets pyroptosis and thrombocytopenia (61, 62). Thus, antioxidant drugs might effectively hinder platelets pyroptosis in APS by reducing ROS secretion. For instance, pretreating platelets with antioxidant NAC (N-acetylcysteine) significantly downregulates the level of NLRP3, cleaved caspase-1, IL-1β, and decreases the proportion of pyroptotic platelets (61, 62). Besides, ND (nanodiamond) has been identified as a carrier for diagnostic and therapeutic platforms. Nevertheless, an investigation has indicated that ND stimulates platelets aggregation and pyroptosis to trigger thrombocytopenia, which is dependent on P-selectin-induced mitochondrial superoxide production and NLRP3 inflammasome activation (63). Thereby, administration of ND with lower doses might reduce platelets-related adverse effects (63).
Of particular, platelets are capable of boosting pyroptosis of macrophages and neutrophils via enhancing NLRP3 transcription, ASC oligomerization, caspase-1 activity, and IL-1β secretion. These platelets-mediated effects are independent of cell-to-cell contact, consequently stimulating the expression of calcium-sensing receptors on macrophages (95). Hence, platelets provide an additional regulation for NLRP3 inflammasome and IL-1β-driven pyroptosis (95). On the other side, the increased caspase-11 and NLRP3 in platelets pyroptosis effectively activate the TF secretion from platelets, macrophages, and neutrophils, which facilitates thrombosis in APS development (96).
8 The potential of pyroptosis-associated medicines in APS therapy
Present studies strongly indicate the crucial role of pyroptosis in the pathogenesis and progression of autoimmune diseases, especially canonical pyroptosis. Thus, pyroptosis-based treatment strategies might improve the therapeutic efficacy of APS, such as GSDMD, NLRP3 inflammasome, and caspase-1-related medicines (Figure 2 and Table 2).
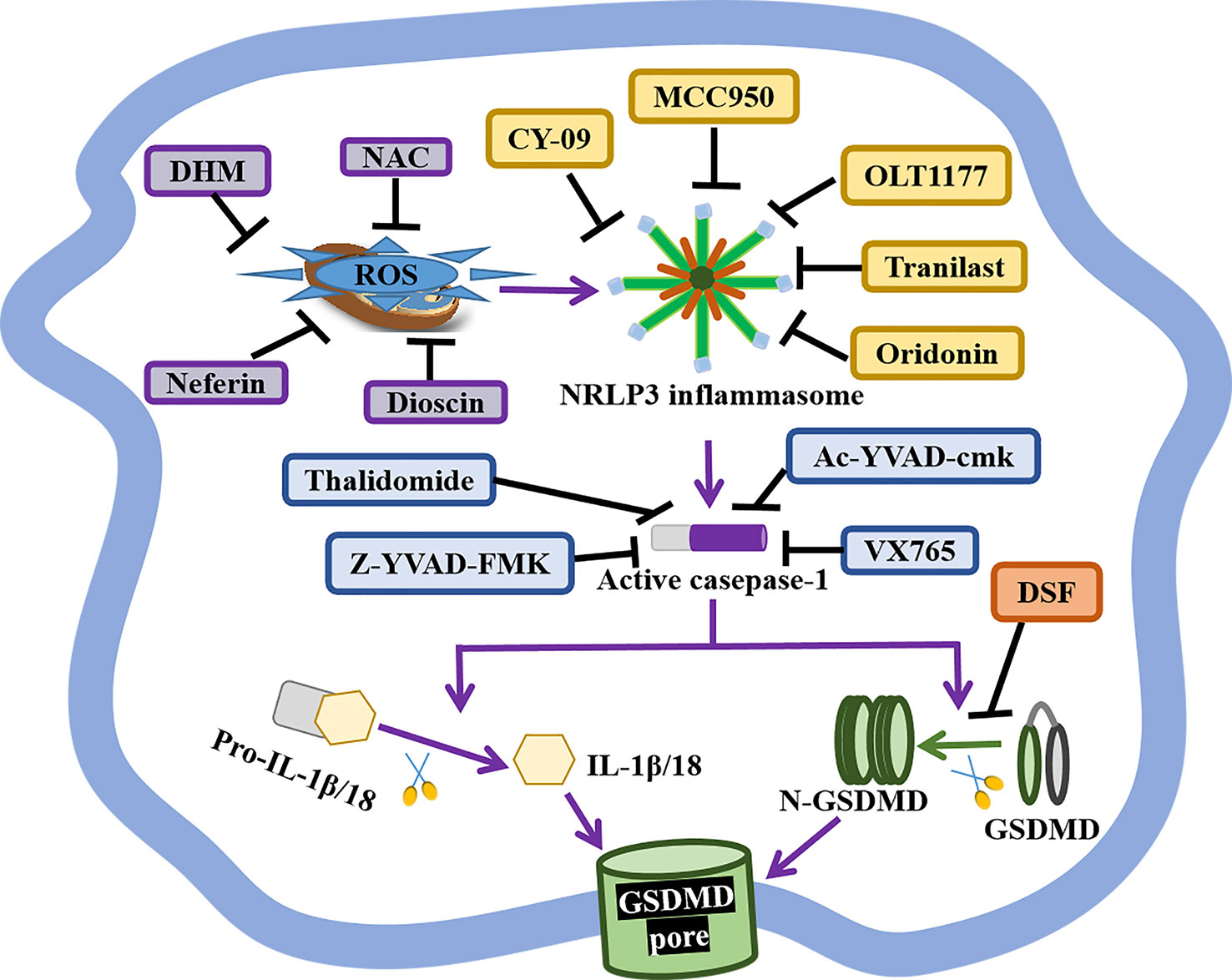
Figure 2 The status of GSDMD, NLRP3 inflammasome, and caspase-1-related inhibitors. GSDMD, NIRP3 inflammasome, and caspase-1 are indispensable in the canonical pyroptosis pathway, and corresponding inhibitors have shown great therapeutical potential for APS. DSF is an inhibitor of GSDMD pores formation. The selective NLRP3 inhibitor MCC950, OLT1177, Tranilast, Oridonin, CY-09 specifically inhibit NLRP3 inflammasome activity and pyroptotic cell death. As well, the anti-oxidant Neferine, DHM, NAC and Dioscin block NLRP3-mediated pyroptosis via reducing the production of ROS. Caspase-1 inhibitor Ac-YVAD-cmk, VX765, Thalidomide, Z-YVAD-FMK markedly repress pyroptosis by forfending caspase-1 activation.
GSDMD is the crucial effectors of pyroptosis, and the inhibition of GSDMD might prevent inflammation and thrombosis in APS (Figure 2 and Table 2). A study has reported that GSDMD succination blocks its interaction with caspases, attenuating its oligomerization, and limiting its capacity to induce inflammation and pyroptotic cell death (108). DSF, as FDA-approved drug for treating alcohol addiction, is an inhibitor of pores formation by specially triggering the covalent modification of Cys191/Cys192 in GSDMD. DSF abrogates pyroptosis, NETs release, and IL-1β secretion, showing a potential application in APS treatment (97, 98).
NLRP3 inflammasome takes part in diverse hallmarks of APS progression, so NLRP3-based APS treatment is valuable (Figure 2 and Table 2). Dioscin is a new NLRP3 inflammasome inhibitor, it significantly prohibits the production of ROS, the expression of NLRP3, caspase-1, and IL-1β in dioscin-treated macrophages (99). Likewise, the selective NLRP3 inhibitor MCC950 ameliorates inflammation and pyroptosis in macrophages via hindering ASC/caspase-1/N-GSDMD axis (100). OLT1177, as an active β-sulfonyl nitrile, it specifically hinders NLRP3 inflammasome activation in vitro, but also mitigates caspase-1 activity and IL-1β production in monocytes from CAPS patients and attenuates the severity of LPS-induced systemic inflammation in vivo (101). Tranilast, the analog of a tryptophan metabolite, is recognized as an anti-allergic agent. It directly binds to the NACHT domain of NLRP3 to block the NLRP3–NLRP3 interaction and the subsequent ASC oligomerization, showing the great therapeutic and preventive effects on NLRP3-related diseases (102). Oridonin is the major bioactive constituent of Rabdosia Rubescens, it binds to cysteine 279 of NACHT via a covalent bond to prevent the NEK7–NLRP3 interaction and the NLRP3 inflammasome activation, but has no effect on AIM2 or NLRC4 activation, LPS-induced NLRP3, IL-1β and, TNF-α production (103). Besides, the ATPase activity of NLRP3 may be a potential drug candidate for the treatment of NLRP3-related diseases, like CY-90. CY-09 can directly bind to the ATP-binding motif of NLRP3 NACHT domain and forfend NLRP3 ATPase activity and NLRP3 oligomerization (104). Moreover, CY-09 is the first well-recognized compound to specifically inhibit NLRP3 inflammasome both in vitro and in vivo, and its inhibitory mechanism has been clearly elucidated (104).
Caspase-1, as an upstream effector, is also crucial for pyroptosis, and selective caspase-1 inhibitors are worth developing (Figure 2 and Table 2). Caspase-1 inhibitor Ac-YVAD-cmk prohibits humoral immunity response in EAMG (experimental autoimmune myasthenia gravis) rats via suppressing IL-6/STAT3/Bcl-6 pathways, providing insights for the development of APS therapy methods (105). Caspase-1 inhibitor VX765 restrains pyroptosis by restraining caspase-1/GSDMD pathway to alleviate colitis in mice, indicating a dose-dependent therapeutic effect on APS (106). Caspase-1 inhibitor Z-YVAD-FMK markedly represses pyroptosis of platelets by forfending ROS/NLRP3/caspase-1 activation (62). Thalidomide is an effective anti-inflammatory drug that significantly impedes caspase-1 activity, it might become a promising agent to better APS therapy (107).
9 Conclusion
Evidence of pryroptosis has been gradually detected in APS-correlated cells, which has an impact on the activity of inflammasomes and the generation of prothrombotic and proinflammatory cytokines. Clarifying the related mechanisms might be beneficial for deeply exploring the pathogenesis of APS and developing new therapeutic biomarkers for APS. The selective inhibitors of GSDMD, inflammasomes, and caspase-1 are identified to ameliorate inflammation and thrombosis, and some of them exhibit excellent therapeutic efficacy in several autoimmune diseases. Thereby, these pyroptosis-correlated inhibitors might bring about valuable options for APS therapy. Of particular, the combination of pyroptosis-correlated drugs with common anticoagulant or anti-inflammatory medicines might be more effective for APS treatment, and further studies should focus on verify their clinic safety in APS patients.
Author contributions
YT designed and wrote the review, drew the figures and tables. QL, ZL and SY wrote and revised this manuscript. LC revised this manuscript and reviewed the figures and tables. All authors contributed to the article and approved the submitted version.
Funding
This work was supported by the National Natural Science Foundation of China (62071011), Key Clinical Specialty Funding Project of Beijing and Hospital-Enterprise Joint Funding Project.
Acknowledgments
We thank the support of the funding for this manuscript.
Conflict of interest
The authors declare that the research was conducted in the absence of any commercial or financial relationships that could be construed as a potential conflict of interest.
Publisher’s note
All claims expressed in this article are solely those of the authors and do not necessarily represent those of their affiliated organizations, or those of the publisher, the editors and the reviewers. Any product that may be evaluated in this article, or claim that may be made by its manufacturer, is not guaranteed or endorsed by the publisher.
Glossary
References
1. Schreiber K, Hunt BJ. Managing antiphospholipid syndrome in pregnancy. Thromb Res (2019) 181(Suppl 1):S41–S6. doi: 10.1016/S0049-3848(19)30366-4
2. Cervera R, Rodriguez-Pinto I, Espinosa G. The diagnosis and clinical management of the catastrophic antiphospholipid syndrome: A comprehensive review. J Autoimmun (2018) 92:1–11. doi: 10.1016/j.jaut.2018.05.007
3. Meroni PL, Tsokos GC. Editorial: Systemic lupus erythematosus and antiphospholipid syndrome. Front Immunol (2019) 10:199. doi: 10.3389/fimmu.2019.00199
4. Shi J, Gao W, Shao F. Pyroptosis: Gasdermin-mediated programmed necrotic cell death. Trends Biochem Sci (2017) 42:245–54. doi: 10.1016/j.tibs.2016.10.004
5. Yu P, Zhang X, Liu N, Tang L, Peng C, Chen X. Pyroptosis: Mechanisms and diseases. Signal Transduct Target Ther (2021) 6:128. doi: 10.1038/s41392-021-00507-5
6. Magnani L, Colantuoni M, Mortellaro A. Gasdermins: New therapeutic targets in host defense, inflammatory diseases, and cancer. Front Immunol (2022) 13:898298. doi: 10.3389/fimmu.2022.898298
7. Sarrio D, Martinez-Val J, Molina-Crespo A, Sanchez L, Moreno-Bueno G. The multifaceted roles of gasdermins in cancer biology and oncologic therapies. Biochim Biophys Acta Rev Cancer (2021) 1876:188635. doi: 10.1016/j.bbcan.2021.188635
8. Dong S, Shi Y, Dong X, Xiao X, Qi J, Ren L, et al. Gasdermin e is required for induction of pyroptosis and severe disease during enterovirus 71 infection. J Biol Chem (2022) 298:101850. doi: 10.1016/j.jbc.2022.101850
9. Aki T, Funakoshi T, Unuma K, Uemura K. Inverse regulation of GSDMD and GSDME gene expression during LPS-induced pyroptosis in RAW264.7 macrophage cells. Apoptosis (2022) 27:14–21. doi: 10.1007/s10495-022-01708-1
10. Liu Z, Wang C, Yang J, Zhou B, Yang R, Ramachandran R, et al. Crystal structures of the full-length murine and human gasdermin d reveal mechanisms of autoinhibition, lipid binding, and oligomerization. Immunity (2019) 51:43–9 e4. doi: 10.1016/j.immuni.2019.04.017
11. Kovacs SB, Miao EA. Gasdermins: Effectors of pyroptosis. Trends Cell Biol (2017) 27:673–84. doi: 10.1016/j.tcb.2017.05.005
12. Zhao J, Jiang P, Guo S, Schrodi SJ, He D. Apoptosis, autophagy, NETosis, necroptosis, and pyroptosis mediated programmed cell death as targets for innovative therapy in rheumatoid arthritis. Front Immunol (2021) 12:809806. doi: 10.3389/fimmu.2021.809806
13. Zhuang L, Luo X, Wu S, Lin Z, Zhang Y, Zhai Z, et al. Disulfiram alleviates pristane-induced lupus via inhibiting GSDMD-mediated pyroptosis. Cell Death Discovery (2022) 8:379. doi: 10.1038/s41420-022-01167-2
14. Lacey CA, Mitchell WJ, Dadelahi AS, Skyberg JA. Caspase-1 and caspase-11 mediate pyroptosis, inflammation, and control of brucella joint infection. Infect Immun (2018) 86. doi: 10.1128/IAI.00361-18
15. Lu F, Lan Z, Xin Z, He C, Guo Z, Xia X, et al. Emerging insights into molecular mechanisms underlying pyroptosis and functions of inflammasomes in diseases. J Cell Physiol (2020) 235:3207–21. doi: 10.1002/jcp.29268
16. Burdette BE, Esparza AN, Zhu H, Wang S. Gasdermin d in pyroptosis. Acta Pharm Sin B (2021) 11:2768–82. doi: 10.1016/j.apsb.2021.02.006
17. Matikainen S, Nyman TA, Cypryk W. Function and regulation of noncanonical caspase-4/5/11 inflammasome. J Immunol (2020) 204:3063–9. doi: 10.4049/jimmunol.2000373
18. Wang Y, Gao W, Shi X, Ding J, Liu W, He H, et al. Chemotherapy drugs induce pyroptosis through caspase-3 cleavage of a gasdermin. Nature (2017) 547:99–103. doi: 10.1038/nature22393
19. Rogers C, Fernandes-Alnemri T, Mayes L, Alnemri D, Cingolani G, Alnemri ES. Cleavage of DFNA5 by caspase-3 during apoptosis mediates progression to secondary necrotic/pyroptotic cell death. Nat Commun (2017) 8:14128. doi: 10.1038/ncomms14128
20. Orning P, Weng D, Starheim K, Ratner D, Best Z, Lee B, et al. Pathogen blockade of TAK1 triggers caspase-8-dependent cleavage of gasdermin d and cell death. Science (2018) 362:1064–9. doi: 10.1126/science.aau2818
21. Hou J, Zhao R, Xia W, Chang CW, You Y, Hsu JM, et al. PD-L1-mediated gasdermin c expression switches apoptosis to pyroptosis in cancer cells and facilitates tumour necrosis. Nat Cell Biol (2020) 22:1264–75. doi: 10.1038/s41556-020-0575-z
22. Liu Y, Fang Y, Chen X, Wang Z, Liang X, Zhang T, et al. Gasdermin e-mediated target cell pyroptosis by CAR T cells triggers cytokine release syndrome. Sci Immunol (2020) 5. doi: 10.1126/sciimmunol.aax7969
23. Zhang Z, Zhang Y, Xia S, Kong Q, Li S, Liu X, et al. Gasdermin e suppresses tumour growth by activating anti-tumour immunity. Nature (2020) 579:415–20. doi: 10.1038/s41586-020-2071-9
24. Zhou Z, He H, Wang K, Shi X, Wang Y, Su Y, et al. Granzyme a from cytotoxic lymphocytes cleaves GSDMB to trigger pyroptosis in target cells. Science (2020) 368:965-+. doi: 10.1126/science.aaz7548
25. Jakubzick CV, Randolph GJ, Henson PM. Monocyte differentiation and antigen-presenting functions. Nat Rev Immunol (2017) 17:349–62. doi: 10.1038/nri.2017.28
26. Xourgia E, Tektonidou MG. An update on antiphospholipid syndrome. Curr Rheumatol Rep (2022) 23:84. doi: 10.1007/s11926-021-01051-5
27. Gavrilin MA, Prather ER, Vompe AD, McAndrew CC, Wewers MD. cAbl kinase regulates inflammasome activation and pyroptosis via ASC phosphorylation. J Immunol (2021) 206:1329–36. doi: 10.4049/jimmunol.2000969
28. Correa R, Silva LFF, Ribeiro DJS, Almeida RDN, Santos IO, Correa LH, et al. Lysophosphatidylcholine induces NLRP3 inflammasome-mediated foam cell formation and pyroptosis in human monocytes and endothelial cells. Front Immunol (2019) 10:2927. doi: 10.3389/fimmu.2019.02927
29. Qu Y, Misaghi S, Newton K, Maltzman A, Izrael-Tomasevic A, Arnott D, et al. NLRP3 recruitment by NLRC4 during salmonella infection. J Exp Med (2016) 213:877–85. doi: 10.1084/jem.20132234
30. Junqueira C, Crespo A, Ranjbar S, Ingber J, Parry B, Ravid S, et al. SARS-CoV-2 infects blood monocytes to activate NLRP3 and AIM2 inflammasomes, pyroptosis and cytokine release. medRxiv (2021). doi: 10.1101/2021.03.06.21252796
31. Levandowski CB, Mailloux CM, Ferrara TM, Gowan K, Ben S, Jin Y, et al. NLRP1 haplotypes associated with vitiligo and autoimmunity increase interleukin-1beta processing via the NLRP1 inflammasome. Proc Natl Acad Sci U.S.A. (2013) 110:2952–6. doi: 10.1073/pnas.1222808110
32. Wu XY, Li KT, Yang HX, Yang B, Lu X, Zhao LD, et al. Complement C1q synergizes with PTX3 in promoting NLRP3 inflammasome over-activation and pyroptosis in rheumatoid arthritis. J Autoimmun (2020) 106:102336. doi: 10.1016/j.jaut.2019.102336
33. Zamyatina A, Heine H. Lipopolysaccharide recognition in the crossroads of TLR4 and caspase-4/11 mediated inflammatory pathways. Front Immunol (2020) 11:585146. doi: 10.3389/fimmu.2020.585146
34. Shen Y, Liu WW, Zhang X, Shi JG, Jiang S, Zheng L, et al. TRAF3 promotes ROS production and pyroptosis by targeting ULK1 ubiquitination in macrophages. FASEB J (2020) 34:7144–59. doi: 10.1096/fj.201903073R
35. Chen Q, Shi P, Wang Y, Zou D, Wu X, Wang D, et al. GSDMB promotes non-canonical pyroptosis by enhancing caspase-4 activity. J Mol Cell Biol (2019) 11:496–508. doi: 10.1093/jmcb/mjy056
36. Zhai Z, Yang F, Xu W, Han J, Luo G, Li Y, et al. Attenuation of rheumatoid arthritis through the inhibition of tumor necrosis factor-induced caspase 3/Gasdermin e-mediated pyroptosis. Arthritis Rheumatol (2022) 74:427–40. doi: 10.1002/art.41963
37. Taabazuing CY, Okondo MC, Bachovchin DA. Pyroptosis and apoptosis pathways engage in bidirectional crosstalk in monocytes and macrophages. Cell Chem Biol (2017) 24:507–14 e4. doi: 10.1016/j.chembiol.2017.03.009
38. Johnson DC, Taabazuing CY, Okondo MC, Chui AJ, Rao SD, Brown FC, et al. DPP8/DPP9 inhibitor-induced pyroptosis for treatment of acute myeloid leukemia. Nat Med (2018) 24:1151–6. doi: 10.1038/s41591-018-0082-y
39. Ryu JC, Kim MJ, Kwon Y, Oh JH, Yoon SS, Shin SJ, et al. Neutrophil pyroptosis mediates pathology of p. aeruginosa lung infection in the absence of the NADPH oxidase NOX2. Mucosal Immunol (2017) 10:757–74. doi: 10.1038/mi.2016.73
40. Yang C, Lei L, Collins JWM, Briones M, Ma L, Sturdevant GL, et al. Chlamydia evasion of neutrophil host defense results in NLRP3 dependent myeloid-mediated sterile inflammation through the purinergic P2X7 receptor. Nat Commun (2021) 12:5454. doi: 10.1038/s41467-021-25749-3
41. Raneia ESPA, de Lima DS, Mesquita Luiz JP, Camara NOS, Alves-Filho JCF, Pontillo A, et al. Inflammatory effect of bothropstoxin-I from bothrops jararacussu venom mediated by NLRP3 inflammasome involves ATP and P2X7 receptor. Clin Sci (Lond) (2021) 135:687–701. doi: 10.1042/CS20201419
42. Chen KW, Demarco B, Ramos S, Heilig R, Goris M, Grayczyk JP, et al. RIPK1 activates distinct gasdermins in macrophages and neutrophils upon pathogen blockade of innate immune signaling. Proc Natl Acad Sci U.S.A. (2021) 118. doi: 10.1073/pnas.2101189118
43. Chen KW, Monteleone M, Boucher D, Sollberger G, Ramnath D, Condon ND, et al. Noncanonical inflammasome signaling elicits gasdermin d-dependent neutrophil extracellular traps. Sci Immunol (2018) 3. doi: 10.1126/sciimmunol.aar6676
44. Jiang C, Jiang L, Li Q, Liu X, Zhang T, Dong L, et al. Acrolein induces NLRP3 inflammasome-mediated pyroptosis and suppresses migration via ROS-dependent autophagy in vascular endothelial cells. Toxicology (2018) 410:26–40. doi: 10.1016/j.tox.2018.09.002
45. Chen H, Lu Y, Cao Z, Ma Q, Pi H, Fang Y, et al. Cadmium induces NLRP3 inflammasome-dependent pyroptosis in vascular endothelial cells. Toxicol Lett (2016) 246:7–16. doi: 10.1016/j.toxlet.2016.01.014
46. Zheng D, Shi Z, Yang M, Liang B, Zhou X, Jing L, et al. NLRP3 inflammasome-mediated endothelial cells pyroptosis is involved in decabromodiphenyl ethane-induced vascular endothelial injury. Chemosphere (2021) 267:128867. doi: 10.1016/j.chemosphere.2020.128867
47. Jia C, Zhang J, Chen H, Zhuge Y, Chen H, Qian F, et al. Endothelial cell pyroptosis plays an important role in Kawasaki disease via HMGB1/RAGE/cathespin b signaling pathway and NLRP3 inflammasome activation. Cell Death Dis (2019) 10:778. doi: 10.1038/s41419-019-2021-3
48. Yao F, Jin Z, Lv X, Zheng Z, Gao H, Deng Y, et al. Hydroxytyrosol acetate inhibits vascular endothelial cell pyroptosis via the HDAC11 signaling pathway in atherosclerosis. Front Pharmacol (2021) 12:656272. doi: 10.3389/fphar.2021.656272
49. Yao F, Jin Z, Zheng Z, Lv X, Ren L, Yang J, et al. HDAC11 promotes both NLRP3/caspase-1/GSDMD and caspase-3/GSDME pathways causing pyroptosis via ERG in vascular endothelial cells. Cell Death Discovery (2022) 8:112. doi: 10.1038/s41420-022-00906-9
50. Zhao J, Liu Z, Chang Z. Lipopolysaccharide induces vascular endothelial cell pyroptosis via the SP1/RCN2/ROS signaling pathway. Eur J Cell Biol (2021) 100:151164. doi: 10.1016/j.ejcb.2021.151164
51. Wu P, Chen J, Chen J, Tao J, Wu S, Xu G, et al. Trimethylamine n-oxide promotes apoE(-/-) mice atherosclerosis by inducing vascular endothelial cell pyroptosis via the SDHB/ROS pathway. J Cell Physiol (2020) 235:6582–91. doi: 10.1002/jcp.29518
52. Wu Y, Pan B, Zhang Z, Li X, Leng Y, Ji Y, et al. Caspase-4/11-Mediated pulmonary artery endothelial cell pyroptosis contributes to pulmonary arterial hypertension. Hypertension (2022) 79:536–48. doi: 10.1161/HYPERTENSIONAHA.121.17868
53. Barnett KC, Ting JP. Mitochondrial GSDMD pores DAMPen pyroptosis. Immunity (2020) 52:424–6. doi: 10.1016/j.immuni.2020.02.012
54. Cheng SB, Nakashima A, Huber WJ, Davis S, Banerjee S, Huang Z, et al. Pyroptosis is a critical inflammatory pathway in the placenta from early onset preeclampsia and in human trophoblasts exposed to hypoxia and endoplasmic reticulum stressors. Cell Death Dis (2019) 10:927. doi: 10.1038/s41419-019-2162-4
55. Quan XZ, Ye JH, Yang XZ, Xie Y. HOXA9-induced chemerin signals through CMKLR1/AMPK/TXNIP/NLRP3 pathway to induce pyroptosis of trophoblasts and aggravate preeclampsia. Exp Cell Res (2021) 408:112802. doi: 10.1016/j.yexcr.2021.112802
56. Tao J, Xia LZ, Liang L, Chen Y, Wei D, Meng J, et al. MiR-124-3p promotes trophoblast cell HTR-8/SVneo pyroptosis by targeting placental growth factor. Placenta (2020) 101:176–84. doi: 10.1016/j.placenta.2020.08.011
57. Fu D, Ju Y, Zhu C, Pan Y, Zhang S. LncRNA NEAT1 promotes TLR4 expression to regulate lipopolysaccharide-induced trophoblastic cell pyroptosis as a molecular sponge of miR-302b-3p. Mol Biotechnol (2022) 64:670–80. doi: 10.1007/s12033-021-00436-2
58. Wu HY, Liu K, Zhang JL. LINC00240/miR-155 axis regulates function of trophoblasts and M2 macrophage polarization via modulating oxidative stress-induced pyroptosis in preeclampsia. Mol Med (2022) 28:119. doi: 10.1186/s10020-022-00531-3
59. Zhang Y, Liu W, Zhong Y, Li Q, Wu M, Yang L, et al. Metformin corrects glucose metabolism reprogramming and NLRP3 inflammasome-induced pyroptosis via inhibiting the TLR4/NF-kappaB/PFKFB3 signaling in trophoblasts: Implication for a potential therapy of preeclampsia. Oxid Med Cell Longev (2021) 2021:1806344. doi: 10.1155/2021/1806344
60. A feedback loop between platelets and NETs amplifies inflammation in severe sepsis. Nat Cardiovasc Res (2022) 1:698–9. doi: 10.1038/s44161-022-00110-z
61. Yin H, Wu M, Lu Y, Wu X, Yu B, Chen R, et al. HMGB1-activatied NLRP3 inflammasome induces thrombocytopenia in heatstroke rat. PeerJ (2022) 10:e13799. doi: 10.7717/peerj.13799
62. Wang S, Liu Y, Li G, Feng Q, Hou M, Peng J. Reduced intracellular antioxidant capacity in platelets contributes to primary immune thrombocytopenia via ROS-NLRP3-caspase-1 pathway. Thromb Res (2021) 199:1–9. doi: 10.1016/j.thromres.2020.12.008
63. Hung SC, Ke LC, Lien TS, Huang HS, Sun DS, Cheng CL, et al. Nanodiamond-induced thrombocytopenia in mice involve p-Selectin-Dependent Nlrp3 inflammasome-mediated platelet aggregation, pyroptosis and apoptosis. Front Immunol (2022) 13:806686. doi: 10.3389/fimmu.2022.806686
64. Shi J, Zhao Y, Wang K, Shi X, Wang Y, Huang H, et al. Cleavage of GSDMD by inflammatory caspases determines pyroptotic cell death. Nature (2015) 526:660–5. doi: 10.1038/nature15514
65. Beckwith KS, Beckwith MS, Ullmann S, Saetra RS, Kim H, Marstad A, et al. Plasma membrane damage causes NLRP3 activation and pyroptosis during mycobacterium tuberculosis infection. Nat Commun (2020) 11:2270. doi: 10.1038/s41467-020-16143-6
66. Rathkey JK, Zhao J, Liu Z, Chen Y, Yang J, Kondolf HC, et al. Chemical disruption of the pyroptotic pore-forming protein gasdermin d inhibits inflammatory cell death and sepsis. Sci Immunol (2018) 3. doi: 10.1126/sciimmunol.aat2738
67. Matias ML, Gomes VJ, Romao-Veiga M, Ribeiro VR, Nunes PR, Romagnoli GG, et al. Silibinin downregulates the NF-kappaB pathway and NLRP1/NLRP3 inflammasomes in monocytes from pregnant women with preeclampsia. Molecules (2019) 24. doi: 10.3390/molecules24081548
68. Zhang Y, Cui J, Zhang G, Wu C, Abdel-Latif A, Smyth SS, et al. Inflammasome activation promotes venous thrombosis through pyroptosis. Blood Adv (2021) 5:2619–23. doi: 10.1182/bloodadvances.2020003041
69. Okondo MC, Johnson DC, Sridharan R, Go EB, Chui AJ, Wang MS, et al. DPP8 and DPP9 inhibition induces pro-caspase-1-dependent monocyte and macrophage pyroptosis. Nat Chem Biol (2017) 13:46–53. doi: 10.1038/nchembio.2229
70. Chen H, Li Y, Wu J, Li G, Tao X, Lai K, et al. RIPK3 collaborates with GSDMD to drive tissue injury in lethal polymicrobial sepsis. Cell Death Differ (2020) 27:2568–85. doi: 10.1038/s41418-020-0524-1
71. Iba T, Levy JH. Inflammation and thrombosis: Roles of neutrophils, platelets and endothelial cells and their interactions in thrombus formation during sepsis. J Thromb Haemost (2018) 16:231–41. doi: 10.1111/jth.13911
72. Singhal A, Kumar S. Neutrophil and remnant clearance in immunity and inflammation. Immunology (2022) 165:22–43. doi: 10.1111/imm.13423
73. Laridan E, Martinod K, De Meyer SF. Neutrophil extracellular traps in arterial and venous thrombosis. Semin Thromb Hemost (2019) 45:86–93. doi: 10.1055/s-0038-1677040
74. Perdomo J, Leung HHL, Ahmadi Z, Yan F, Chong JJH, Passam FH, et al. Neutrophil activation and NETosis are the major drivers of thrombosis in heparin-induced thrombocytopenia. Nat Commun (2019) 10:1322. doi: 10.1038/s41467-019-09160-7
75. Delgado-Rizo V, Martinez-Guzman MA, Iniguez-Gutierrez L, Garcia-Orozco A, Alvarado-Navarro A, Fafutis-Morris M. Neutrophil extracellular traps and its implications in inflammation: An overview. Front Immunol (2017) 8:81. doi: 10.3389/fimmu.2017.00081
76. Karmakar M, Katsnelson MA, Dubyak GR, Pearlman E. Neutrophil P2X7 receptors mediate NLRP3 inflammasome-dependent IL-1beta secretion in response to ATP. Nat Commun (2016) 7:10555. doi: 10.1038/ncomms10555
77. Son S, Yoon SH, Chae BJ, Hwang I, Shim DW, Choe YH, et al. Neutrophils facilitate prolonged inflammasome response in the DAMP-rich inflammatory milieu. Front Immunol (2021) 12:746032. doi: 10.3389/fimmu.2021.746032
78. Karmakar M, Minns M, Greenberg EN, Diaz-Aponte J, Pestonjamasp K, Johnson JL, et al. N-GSDMD trafficking to neutrophil organelles facilitates IL-1beta release independently of plasma membrane pores and pyroptosis. Nat Commun (2020) 11:2212. doi: 10.1038/s41467-020-16043-9
79. Liu L, Sun B. Neutrophil pyroptosis: New perspectives on sepsis. Cell Mol Life Sci (2019) 76:2031–42. doi: 10.1007/s00018-019-03060-1
80. Chen KW, Lawlor KE, von Pein JB, Boucher D, Gerlic M, Croker BA, et al. Cutting edge: Blockade of inhibitor of apoptosis proteins sensitizes neutrophils to TNF- but not lipopolysaccharide-mediated cell death and IL-1beta secretion. J Immunol (2018) 200:3341–6. doi: 10.4049/jimmunol.1701620
81. Sollberger G, Choidas A, Burn GL, Habenberger P, Di Lucrezia R, Kordes S, et al. Gasdermin d plays a vital role in the generation of neutrophil extracellular traps. Sci Immunol (2018) 3. doi: 10.1126/sciimmunol.aar6689
82. Li H, Li Y, Song C, Hu Y, Dai M, Liu B, et al. Neutrophil extracellular traps augmented alveolar macrophage pyroptosis via AIM2 inflammasome activation in LPS-induced ALI/ARDS. J Inflammation Res (2021) 14:4839–58. doi: 10.2147/JIR.S321513
83. Ruiz M, Frej C, Holmer A, Guo LJ, Tran S, Dahlback B. High-density lipoprotein-associated apolipoprotein m limits endothelial inflammation by delivering sphingosine-1-Phosphate to the sphingosine-1-Phosphate receptor 1. Arterioscler Thromb Vasc Biol (2017) 37:118–29. doi: 10.1161/ATVBAHA.116.308435
84. Liu Y, Tie L. Apolipoprotein m and sphingosine-1-phosphate complex alleviates TNF-alpha-induced endothelial cell injury and inflammation through PI3K/AKT signaling pathway. BMC Cardiovasc Disord (2019) 19:279. doi: 10.1186/s12872-019-1263-4
85. Wu X, Zhang H, Qi W, Zhang Y, Li J, Li Z, et al. Nicotine promotes atherosclerosis via ROS-NLRP3-mediated endothelial cell pyroptosis. Cell Death Dis (2018) 9:171. doi: 10.1038/s41419-017-0257-3
86. Deng M, Tang Y, Li W, Wang X, Zhang R, Zhang X, et al. The endotoxin delivery protein HMGB1 mediates caspase-11-Dependent lethality in sepsis. Immunity (2018) 49:740–53 e7. doi: 10.1016/j.immuni.2018.08.016
87. Zeng J, Tao J, Xia L, Zeng Z, Chen J, Wang Z, et al. Melatonin inhibits vascular endothelial cell pyroptosis by improving mitochondrial function via up-regulation and demethylation of UQCRC1. Biochem Cell Biol (2021) 99:339–47. doi: 10.1139/bcb-2020-0279
88. Zhang Y, Liu X, Bai X, Lin Y, Li Z, Fu J, et al. Melatonin prevents endothelial cell pyroptosis via regulation of long noncoding RNA MEG3/miR-223/NLRP3 axis. J Pineal Res (2018) 64. doi: 10.1111/jpi.12449
89. Tang YS, Zhao YH, Zhong Y, Li XZ, Pu JX, Luo YC, et al. Neferine inhibits LPS-ATP-induced endothelial cell pyroptosis via regulation of ROS/NLRP3/Caspase-1 signaling pathway. Inflammation Res (2019) 68:727–38. doi: 10.1007/s00011-019-01256-6
90. Hu Q, Zhang T, Yi L, Zhou X, Mi M. Dihydromyricetin inhibits NLRP3 inflammasome-dependent pyroptosis by activating the Nrf2 signaling pathway in vascular endothelial cells. Biofactors (2018) 44:123–36. doi: 10.1002/biof.1395
91. Xing SS, Yang J, Li WJ, Li J, Chen L, Yang YT, et al. Salidroside decreases atherosclerosis plaque formation via inhibiting endothelial cell pyroptosis. Inflammation (2020) 43:433–40. doi: 10.1007/s10753-019-01106-x
92. Yang M, Lv H, Liu Q, Zhang L, Zhang R, Huang X, et al. Colchicine alleviates cholesterol crystal-induced endothelial cell pyroptosis through activating AMPK/SIRT1 pathway. Oxid Med Cell Longev (2020) 2020:9173530. doi: 10.1155/2020/9173530
93. Liu Z, Chen B, Chang J, Feng L, Zhao X. Melatonin regulates trophoblast pyroptosis, invasion and migration in preeclampsia by inhibiting HtrA1 transcription through the microRNA-520c-3p/SETD7 axis. Am J Reprod Immunol (2022) 87:e13523. doi: 10.1111/aji.13523
94. Hally K, Fauteux-Daniel S, Hamzeh-Cognasse H, Larsen P, Cognasse F. Revisiting platelets and toll-like receptors (TLRs): At the interface of vascular immunity and thrombosis. Int J Mol Sci (2020) 21. doi: 10.3390/ijms21176150
95. Rolfes V, Ribeiro LS, Hawwari I, Bottcher L, Rosero N, Maasewerd S, et al. Platelets fuel the inflammasome activation of innate immune cells. Cell Rep (2020) 31:107615. doi: 10.1016/j.celrep.2020.107615
96. Ryan TAJ, Preston RJS, O'Neill LAJ. Immunothrombosis and the molecular control of tissue factor by pyroptosis: Prospects for new anticoagulants. Biochem J (2022) 479:731–50. doi: 10.1042/BCJ20210522
97. Hu JJ, Liu X, Xia S, Zhang Z, Zhang Y, Zhao J, et al. FDA-Approved disulfiram inhibits pyroptosis by blocking gasdermin d pore formation. Nat Immunol (2020) 21:736–45. doi: 10.1038/s41590-020-0669-6
98. Silva CMS, Wanderley CWS, Veras FP, Sonego F, Nascimento DC, Goncalves AV, et al. Gasdermin d inhibition prevents multiple organ dysfunction during sepsis by blocking NET formation. Blood (2021) 138:2702–13. doi: 10.1182/blood.2021011525
99. Yin W, Liu S, Dong M, Liu Q, Shi C, Bai H, et al. A new NLRP3 inflammasome inhibitor, dioscin, promotes osteogenesis. Small (2020) 16:e1905977. doi: 10.1002/smll.201905977
100. Zeng W, Wu D, Sun Y, Suo Y, Yu Q, Zeng M, et al. The selective NLRP3 inhibitor MCC950 hinders atherosclerosis development by attenuating inflammation and pyroptosis in macrophages. Sci Rep (2021) 11:19305. doi: 10.1038/s41598-021-98437-3
101. Marchetti C, Swartzwelter B, Gamboni F, Neff CP, Richter K, Azam T, et al. OLT1177, a beta-sulfonyl nitrile compound, safe in humans, inhibits the NLRP3 inflammasome and reverses the metabolic cost of inflammation. Proc Natl Acad Sci U.S.A. (2018) 115:E1530–E9. doi: 10.1073/pnas.1716095115
102. Huang Y, Jiang H, Chen Y, Wang X, Yang Y, Tao J, et al. Tranilast directly targets NLRP3 to treat inflammasome-driven diseases. EMBO Mol Med (2018) 10. doi: 10.15252/emmm.201708689
103. He H, Jiang H, Chen Y, Ye J, Wang A, Wang C, et al. Oridonin is a covalent NLRP3 inhibitor with strong anti-inflammasome activity. Nat Commun (2018) 9:2550. doi: 10.1038/s41467-018-04947-6
104. Jiang H, He H, Chen Y, Huang W, Cheng J, Ye J, et al. Identification of a selective and direct NLRP3 inhibitor to treat inflammatory disorders. J Exp Med (2017) 214:3219–38. doi: 10.1084/jem.20171419
105. Wang CC, Zhang M, Li H, Li XL, Yue LT, Zhang P, et al. Caspase-1 inhibitor regulates humoral responses in experimental autoimmune myasthenia gravis via IL-6- dependent inhibiton of STAT3. Neurosci Lett (2017) 656:169–76. doi: 10.1016/j.neulet.2017.05.040
106. Wang L, Dong X, Feng S, Pan H, Jang X, Chen L, et al. VX765 alleviates dextran sulfate sodium-induced colitis in mice by suppressing caspase-1-mediated pyroptosis. Int Immunopharmacol (2022) 102:108405. doi: 10.1016/j.intimp.2021.108405
107. Zeng Q, Fu J, Korrer M, Gorbounov M, Murray PJ, Pardoll D, et al. Caspase-1 from human myeloid-derived suppressor cells can promote T cell-independent tumor proliferation. Cancer Immunol Res (2018) 6:566–77. doi: 10.1158/2326-6066.CIR-17-0543
Keywords: APS, pyroptosis, mechanisms, cellular effects, therapy
Citation: Tan Y, Liu Q, Li Z, Yang S and Cui L (2023) Pyroptosis-triggered pathogenesis: New insights on antiphospholipid syndrome. Front. Immunol. 14:1155222. doi: 10.3389/fimmu.2023.1155222
Received: 31 January 2023; Accepted: 13 March 2023;
Published: 31 March 2023.
Edited by:
Durga Prasanna Misra, Sanjay Gandhi Post Graduate Institute of Medical Sciences (SGPGI), IndiaReviewed by:
Jun Chen, Hubei University of Medicine, ChinaCristiano Alessandri, Sapienza University of Rome, Italy
Copyright © 2023 Tan, Liu, Li, Yang and Cui. This is an open-access article distributed under the terms of the Creative Commons Attribution License (CC BY). The use, distribution or reproduction in other forums is permitted, provided the original author(s) and the copyright owner(s) are credited and that the original publication in this journal is cited, in accordance with accepted academic practice. No use, distribution or reproduction is permitted which does not comply with these terms.
*Correspondence: Liyan Cui, Y2xpeWFuQDE2My5jb20=