- 1Key Laboratory of Major Chronic Diseases of Nervous System of Liaoning Province, Health Sciences Institute of China Medical University, Shenyang, China
- 2Department of Rehabilitation, Shengjing Hospital of China Medical University, Shenyang, China
- 3Rubedo Life Sciences, Sunnyvale, CA, United States
The brain has long been considered an immune-privileged organ due to the presence of the blood-brain barrier (BBB). However, recent discoveries have revealed the underestimated role of T cells in the brain through the meningeal lymphatic system. Age is the primary risk factor for Alzheimer’s disease (AD), resulting in marked age-dependent changes in T cells. Manipulating peripheral T cell immune response has been shown to impact AD, but the relationship between T cell aging and AD remains poorly understood. Given the limited success of targeting amyloid beta (Aβ) and the growing evidence of T cells’ involvement in non-lymphoid organ aging, a deeper understanding of the relationship between T cells and AD in the context of aging is crucial for advancing therapeutic progress. In this review, we comprehensively examine existing studies on T cells and AD and offer an integrated perspective on their interconnections in the context of aging. This understanding can inform the development of new interventions to prevent or treat AD.
Introduction
T cell aging begins with thymic involution, one of the most well-studied hallmarks of immune aging, and occurs during childhood and puberty (1). As a result, the thymus significantly reduces its production of new naïve T cells, and the maintenance of peripheral naïve T cells heavily relies on homeostatic proliferation. Although the homeostatic proliferation of T cells can replenish the T cell compartment, the number of naïve T cells still declines significantly with age, especially for CD8 T cells (2, 3). The inability to maintain naivety leads to failure of self-renewal of naïve T cells via hemostatic proliferation in old individuals, which occurs more often within CD8 T cells than within CD4 T cells. In line with this difference, terminally differentiated T effector memory CD45RA+ (TEMRA) cells, which display senescent characteristics, accumulate more among CD8 T cells than among CD4 T cells in old individuals (4). Interestingly, virtual memory cells in humans that develop features of cellular senescence accumulate with age and are found among TEMRA cells (5, 6), suggesting generation of TEMRA cells partially comes from cytokines stimulation. Although fewer TEMRA cells exist among CD4 T cells, aged CD4 T cells also display powerful pro-inflammatory or anti-inflammatory features, which closely link to disorders in old individuals (7, 8). Besides, the long-term memory and T follicular helper (Tfh) cell development potential of aged naïve T cells is impaired due to upregulated CD39, miR-21 expression, and impaired proteostasis (9–12), suggesting that age-related changes already exist at the naïve stage. Indeed, single-cell RNA sequencing of naïve CD4 T cells revealed a distinct profile of aged naïve T cells (13). Furthermore, the functionality of aged memory T cells is still inferior due to decreased CD73+ memory cells (14). Thus, age-related alterations in naïve cells, memory cells, homeostatic proliferation, and activation shape significantly affect the function of T cells in old individuals (Figure 1).
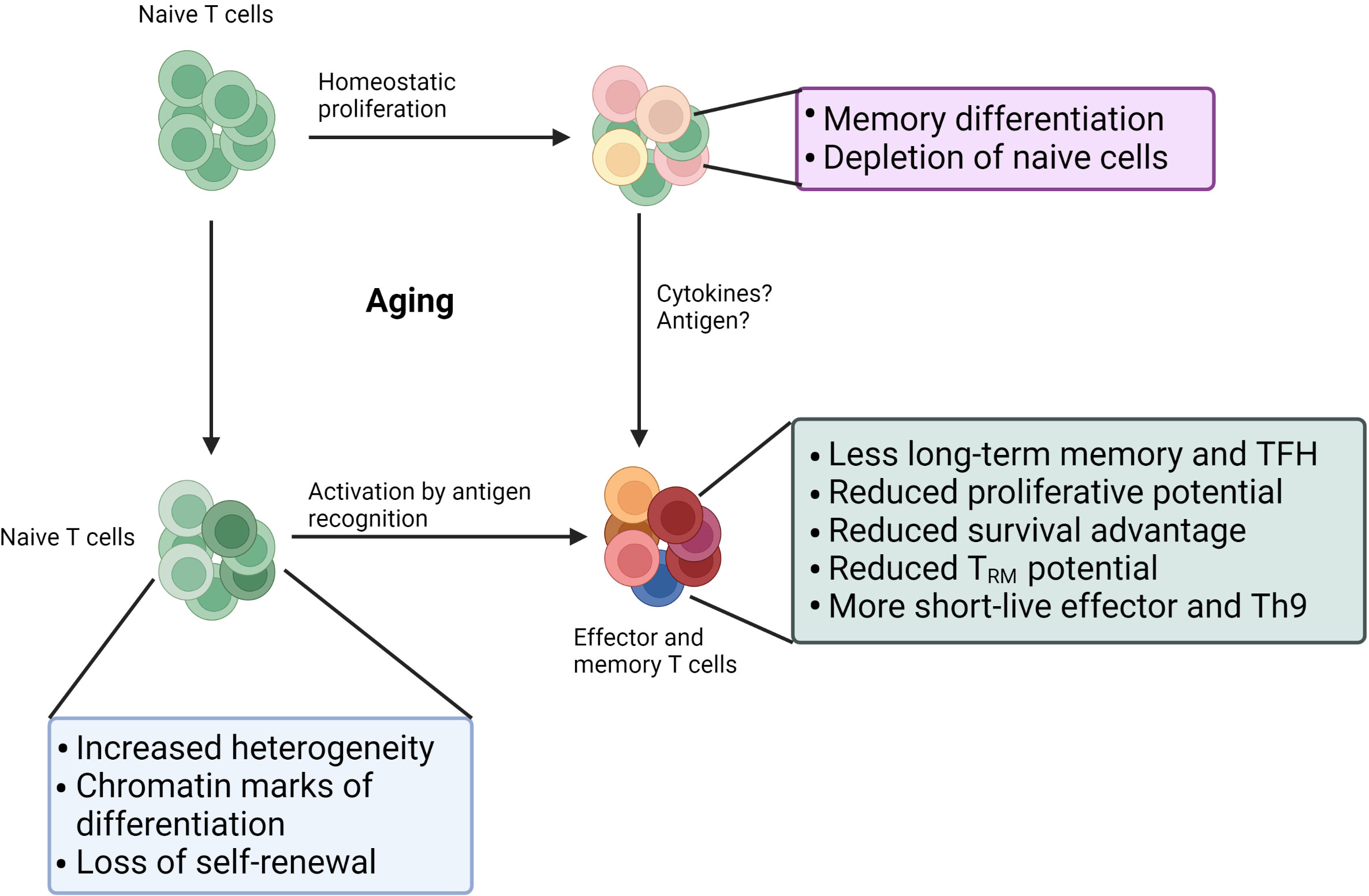
Figure 1 Age-related changes in the T cell. Self-renewal of naïve T cell cells via homeostatic proliferation often fails in old individuals: 1) memory differentiation driven by homeostatic cytokines; 2) death due to lack of survival signal. Age also leads to significant changes in naïve T cell compartment: more differentiated states, increased heterogeneity, and reduced quiescence. Those changes in naïve T cells combined with changes in activation stages result in dysfunctions in aged effector or memory T cells. Furthermore, age impairs the function of existing memory.
Alzheimer’s disease (AD) is an age-related neurodegenerative brain disorder and is the most prevalent neurodegenerative disease in the aged population (15). Its cardinal characteristics are the extracellular deposit of amyloid fibrillary amyloid beta (Aβ) and neurofibrillary tangles mediated by aggregated hyperphosphorylated tau protein (16, 17). Several therapeutic drugs have been developed, and many clinical trials have been conducted based on the amyloid cascade hypothesis. However, most AD-related clinic trials have failed, necessitating that promote researchers revisit the mechanisms underlying AD (18).
An increasing body of evidence demonstrates that dysfunctional neuroinflammation and systemic inflammation are found in AD and associated with clinical indications (19). Indeed, Yousefzadeh et al. found that an aged immune system can drive the senescence of solid organs, including the brain (20). Accumulation of senescent cells in the brain has been reported to worsen pathologic impairments in AD mice models (21, 22). Hence, the aged immune system is supposed to exacerbate the pathologic process of AD. It raises a question: as a critical part of the immune system, what is the role of aged T cells in the senescence of non-immune organs? A recent study revealed that Tfam deficiency in T cells induced inflammaging, which led to multimorbidity and premature senescence, including neurologic impairments (23). Although the authors did not evaluate the AD-specific alterations, neurodegenerative changes were observed (23). Tfam-deficient T cells exhibit some identical features as aged T cells; arguably, T cell aging plays an essential role in AD. A recent report supports it: the frequency of TEMRA CD8 T cell—its accumulation is one of the hallmarks of T cell aging–in peripheral and cerebrospinal fluid (CSF) increases in AD patients. It is negatively correlated with cognition (24). This review summarizes the findings of such age-related changes and the potential role of T cell aging in AD.
Thymic involution and AD
One of the primary characteristics of T cell aging is thymic involution, which results in reduced generation of naïve T cells (25). In humans, the thymus is a primary source of T cells before puberty. However, its contribution decreases to less than 20% by early adulthood and further declines with age due to thymic involution. Thymic involution in mice is more gradual than in humans, and thymic output still plays a role in maintaining naïve T cells even in aged mice (26–28). Thymic involution directs the senescence of cells through three aspects: 1) impaired negative selection, 2) imbalanced Treg generation, and 3) reduced thymopoiesis (29).
Impaired negative selection in the aged thymus leads to an increased generation of self-reactive T cells (29). Aβ-specific T cells increase in AD patients and old individuals (30), which can infiltrate the brain parenchyma and promote AD in mice (31). Whether the increased Aβ-specific T cells in AD patients and old individuals is a consequence of thymic involution remains elusive. Thymic involution also dramatically changes Treg generation. Due to reduced TCR signaling strength in the atrophied thymus, the generation of tTreg (thymic Treg) cells increased (32), which has been shown to play an important role in AD. Depending on their location, Treg cells’ role in AD is protective or pathogenic (33). Treg cells residing in the choroid plexus (CP) inhibit CP leukocyte trafficking via IL-10, which is detrimental to AD (34, 35). However, Treg cells in the brain inhibit neuroinflammation and could ameliorate the pathology of AD (36). Based on these facts, increased Aβ-specific T cells and generation of Treg cells in aged thymus probably promote AD. The following two sections will discuss reduced thymopoiesis-mediated decreased naïve T cells and increased senescent T cells.
Age-related thymic involution is mainly driven by increased sex steroids, mediated by steroid signaling in thymic epithelial cells (TEC) (37). Surgical or chemical sex steroid ablation improves thymic rejuvenation. In addition to age, specific infection and physiological changes, such as schistosomiasis and pregnancy, induce acute thymic involution (38, 39), which affect the process of infection and pregnancy through T cell response. Whether changes in AD influence thymic involution remains an interesting question. As we know, AD is also associated with age-related sex hormone fluctuations, but the effect of sex steroids on AD is controversial. Numerous epidemiologic studies suggest a protective role for estrogen in Alzheimer’s disease (40, 41). However, a recent study indicates that long-term hormone replacement therapy (HRT) is associated with an increased risk of AD in postmenopausal women (42, 43). Long-term treatment with hormones, especially at an earlier age, accelerates thymic involution, which is probably detrimental to AD. Consistently, Leuprorelin, a luteinizing hormone-releasing hormone (LHRH) agonist, inhibits sex steroids generation, rejuvenates T cell immunity via increased thymic activity (44), and also has been found to ameliorate neuropathologic processes in APP/PS1 mice and delay loss of cognition in a subgroup of AD patients (45, 46). Another piece of evidence comes from the study of congenital heart disease (CHD); the thymus is removed during cardiac surgery in newborns with CHD, leading to T cell aging (47). Adults with CHD have an increased risk of developing AD (48), which is probably associated with thymectomy. Further investigations are warranted to explore the potential relationship between AD and thymic activity.
Naïve/memory T cells imbalance and AD
As described in the previous section, due to reduced thymic output, naïve/memory T cell imbalance is the most significant hallmark of T cell aging. Although homeostatic proliferation can considerably compensate naïve T cells in young individuals, self-renewal via homeostatic proliferation often fails in old individuals (49). Homeostatic proliferation depends on signaling from IL7 and MHC/self-antigen (50). Decreased IL-7 receptor expression on aged T cells and less accessibility to secondary lymph organs contribute to the loss of naïve T cells with age (51, 52). Besides, due to declined TRIB2, aged naïve T cells are prone to differentiation towards memory response to homeostatic cytokines that further contribute to the loss of naïve T cells (53).
Recent studies have shown that naïve T cells are more heterogeneous than previously thought (54)—aging shapes dramatically naïve T cells’ epigenetic landscape (55–57). Age-related changes in naïve T cell chromatin accessibility are similar to those in activated and differentiated T cells, and this phenomenon is more pronounced in naïve CD8 T cells (58–60). At the single-cell level, aging increases heterogeneity in gene expression during early activation, indicating diversity in differentiation states (13, 61). Age-associated transcriptional factors and microRNA network changes account for those differentiation states (9, 13, 60, 62–64). A phenotypically unique subset of “young naïve T cells” –CD45RAhighCD27highCD38+CD25neg–is lost with age (65). More differentiated states in aged naïve T cells imply less effector plasticity. Increased transforming growth factor-β receptor 3 and CD39 in aged naïve CD4 T cells favor Th9 and inhibit Tfh differentiation, respectively (11, 66). Moreover, due to increased miR-21, naïve CD4 T cells from old individuals are prone to differentiate into inflammatory effector cells rather than Tfh and memory precursor cells (9). In addition to the frequency of naïve T cells, aging changes dramatically the states of naïve T cells.
Peripheral T cells in AD patients and mouse models have been studied widely (67), and a decreased ratio of naïve to memory T cells is a consistent phenotype. Tau and Aβ have been reported to trigger T cell-specific response (30, 68), suggesting that peripheral tau and Aβ protein mimic “infection” and drive naïve T cell differentiation toward memory cells. However, the explanation is likely more complex. Substantial clonally expanded CD8 T cells in AD patients specifically recognize antigens from Epstein-Barr Virus (EBV), not AD-related proteins (24). Hence, other factors contribute to the loss or differentiation of naïve T cells in AD. Studies from atherosclerosis, another age-related disease, show that accumulation of cholesterol in T cells induces T cell aging phenotype, including less frequent naïve T cells (69). Interestingly, CD8 virtual memory T cells accumulate with age and upregulate lipid rafts (6, 70). Dysfunction of lipid metabolism in AD is associated with lipid raft changes and activation of innate immune cells (71), suggesting that lipid metabolism dysfunction in AD possibly accelerates differentiation of naïve T cells via elevated cholesterol or lipid rafts.
Poor naivety maintenance of T cells in AD may contribute to impaired cognition, and age-related downregulation of the naïve marker CCR7 on T cells is associated with worsened cognition (72). Furthermore, CCR7-deficiency exacerbates brain Aβ deposition and cognitive decline in 5xFAD mice, and anti-CD25 treatment improves cognition (72). Although the authors attributed it to the depletion of Treg cells and did not evaluate the states of naïve T cells with anti-CD25 injection, recently Zhang et al. showed that Helios downregulated CD25 and prevented an effector cell response, anti-CD25 treatment shifted the chromatin accessibility states toward those of younger individuals and away from an effector memory pattern (13). Hence, in addition to the depletion of Treg, CD25 inhibition probably also influences pathologies of AD via maintaining the naïve pattern of T cells. More specific inventions are required to investigate the impact of naïve cells on AD. TRIB2 is a vital regulator of naivety, and its stabilizing drugs have been applied to clinical treatment (53). It is thus of great interest to evaluate the effect of TRIB2-mediated maintenance of naïve T cells to AD.
Senescent T cells and AD
Aging leads to the accumulation of senescent cells, including senescent T cells, which share many standard features of senescence with other senescent cells, including short telomeres and reduced telomerase activity, DNA damage, mitochondrial dysfunction, and β-galactosidase activity (73). Functionally, senescent T cells are defective in their TCR-induced proliferation and secrete abundant inflammatory factors—as part of the SASP (senescence-associated secretory phenotype). They lack the costimulatory receptors CD27 and CD28 and upregulate natural killer cell (NK)-related markers (74). Human TEMRA cells, which display this characteristic of cellular senescence and are associated with increased p38 signaling (4), are a primary subset of senescent T cells. Senescent T cell accumulation is likely a result of lifelong antigen stimulation, especially chronic virus infection (75). However, virtual memory T cells, driven by cytokines, also accumulate with age and exhibit similar features of senescence (6). CD4 T cells also acquire NK receptors and become cytotoxic with age, possibly associated with downregulated ThPOK (7, 76, 77). ThPOK expression declines in aged CD4 T cells (53). Thus, ThPOK may play a crucial role in accumulating senescent T cells.
CD8 TEMRA cells are found to increase in peripheral blood, and cerebrospinal fluid of AD patients and are correlated with impaired cognition (24). CD8 T cells from human brains (including AD patients) lack CD27 and CD28 expression, which are characteristics of TEMRA cells (78). Surprisingly, TCR-sequencing reveals that a high proportion of clonally expanded CD8 T cells among these TEMRA cells recognize EBV antigen (24), suggesting that these TEMRA cells probably play a role in AD via a bystander function. Why these TEMRA cells increase in AD patients is unclear. It is speculated that these TEMRA cells generate in the periphery via antigen or cytokine stimulation and migrate into the brain. Further investigation is needed to understand the mechanisms underlying peripheral alterations of AD associated with TEMRA cell accumulation.
CD8 TEMRA cells upregulate NK markers, which mediate the killing of senescent cells independent of TCR recognition (79). Increased frequency of CD8 TEMRA cells is supposed to enhance the clearance of senescent cells in the brain. However, intracerebral senescent cells accumulate in AD mice, which negatively affects the process of AD (21, 22), suggesting that the cytotoxicity of CD8 TEMRA cells in AD is impaired. Similarly, the ability of aged CD8 T cells to clear senescent cells is diminished due to elevated NKG2A and PD-1 and reduced binding of perforin (79–82). Furthermore, the low cytotoxicity of CD8 T cells is likely brain-specific. Compared to circulating CD8 T cells, CD8 T cells from human brains express less GZMB and perforin (78). Hence, reduced immune surveillance of senescent cells in the brain by CD8 T cells may contribute to AD development.
On the other hand, GZMK and TNFα secreted from senescent CD8 T cells promote the senescence of other cells, including brain cells, which probably exacerbate the accumulation of senescent cells in the brain (8, 23). Hence, the role of CD8 T cells in AD is complex (83, 84). Clearance of senescent cells in the brain requires functionally cytotoxic CD8 T cells. However, aged CD8 T cells are unable to kill senescent cells and induce more cellular senescence in the brain via SASP signals. In addition to the contribution of cellular senescence in the brain, the accumulation of aged CD8 T cells in the brain directly promotes axon and myelin degeneration via GzmB and IFNγ-mediated microglia activation (85, 86). These emphasize the importance of correcting the dysfunction of aged CD8 T cells, not simply via depletion or supplementation.
Senescent CD4 T cells acquire extreme pro-inflammatory and anti-inflammatory phenotypes with reduced effector plasticity. Peripheral CD4 T cells from AD patients exhibit a bias differentiation as seen in old individuals with increased activity of Th17, Th9, and Th1 (87–89). These T cell subsets can infiltrate the brain and be involved in the pathology of AD (31, 90, 91). IL-17 has been reported to trigger the onset of cognitive and synaptic deficits and the breakdown of the brain blood barrier, and blockade of IL-17 decreased cognitive impairments (92–94). The role of Th1 in AD is controversial (95). Intraventricular injection of Th1 cells favored microglia activation and clearance of Aβ (96, 97). However, when transferred peripherally, Th1 cells increased Aβ plaque burden (31). Although senescent T cells contribute to inflammaging and are detrimental in age-related disorders by producing pro-inflammatory cytokines, activating T cell responses by blocking PD-1 or transient depletion of peripheral Treg cells ameliorates disease pathology in mouse models of AD (34, 98). PD-1+ T cells and Treg cells accumulate in the periphery with aging in both mice and humans (99, 100), and PD-1 blockade and depletion of Treg cells appear to correct some age-related dysfunctions. These suggest that restoring the function of senescent T cells in the periphery could ameliorate AD pathology.
Concluding remarks
Numerous poor outcomes from clinical trials on AD have stimulated the revisiting of AD etiology. Treatments targeting Aβ have shown discouraged outcomes in AD patients, suggesting that clearing Aβ alone is insufficient to delay the AD process. The role of Aβ in AD needs to be revisited in the context of new emerging hypotheses, such as senescence cascade and neuroinflammation, which are closely linked to T cells. Aging is the most significant risk factor for AD, dramatically shaping T cells’ function. Recent evidence shows that the immune system, especially T cells, plays a central role in whole-body senescence, highlighting the relevance of T cells in AD.
Current studies about T cell aging and AD are limited and indirect. We summarize the potential relationship between T cell aging and AD (Figure 2) and critical questions to warrant further investigation: 1) What is the role of intracerebral CD8 TEMRA cells in AD? Why cannot they kill accumulated senescent cells? 2) Whether induction of T cell aging, for example, thymectomy, exacerbates the pathology of AD, and what are the mechanisms? 3) How do changes in AD influence T cell aging? Answering these questions will deepen our understanding of the etiology of AD and the mechanism of T cell aging and open new possibilities by developing drugs to treat AD via targeting aged T cells.
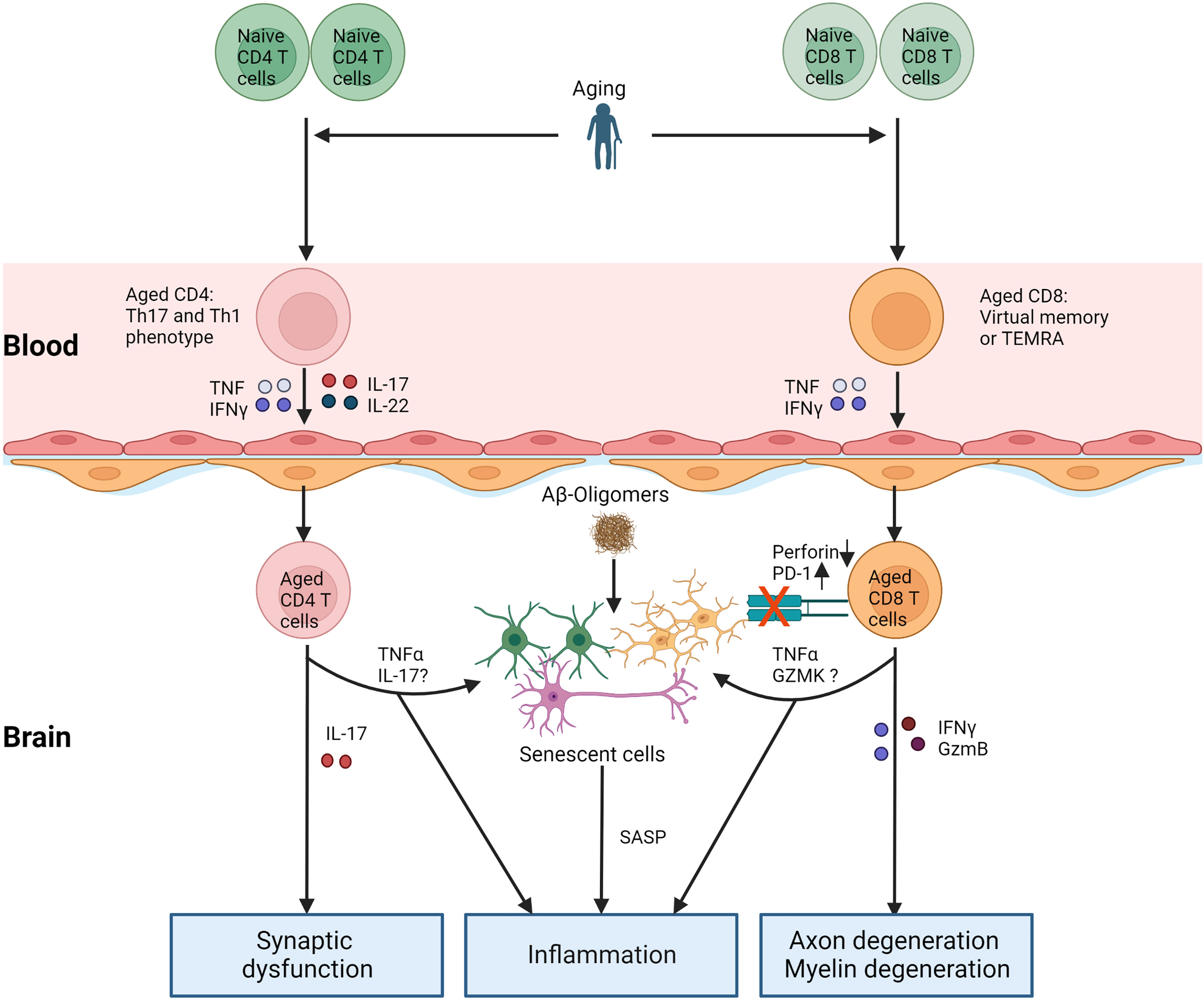
Figure 2 T cell aging and AD. With age, the number of naïve T cells decreases, and relative memory T cells (Th1 and Th17 for CD4 T cells, TEMRA, or virtual memory for CD8 T cells) accumulate. Cytokines secreted by aged T cells increase the permeability of BBB, which increases other immune cells or their entry into the brain. On the one hand, aged T cells in the brain directly contribute to neuroinflammation and damage to the nervous system by releasing cytokines. Aged T cells in the brain worsen the pathology of AD via the accumulation of senescent cells. TNFα, IL-17, and GZMK from aged T cells probably induce senescence in brain cells. Furthermore, increased PD-1 or decreased perforin in aged CD8 T cells in the brain inhibits the clearance of senescent cells. Aβ: amyloid β, TEMRA: T effector memory CD45RA+. SASP: senescence-associated secretory phenotype.
Author contributions
LG and XL prepared the manuscript. TG reviewed the manuscript. ZYW and WC edited and finalized the manuscript. All authors contributed to the article and approved the submitted version.
Funding
This work was supported by the National Natural Sciences Foundation of China (32270938 to WC) and the Natural Science Foundation of Liaoning Province (2019JH2/10300003 to ZYW).
Conflict of interest
Author TG was employed by company Rubedo Life Sciences.
The remaining authors declare that the research was conducted in the absence of any commercial or financial relationships that could be construed as a potential conflict of interest.
Publisher’s note
All claims expressed in this article are solely those of the authors and do not necessarily represent those of their affiliated organizations, or those of the publisher, the editors and the reviewers. Any product that may be evaluated in this article, or claim that may be made by its manufacturer, is not guaranteed or endorsed by the publisher.
References
1. Thapa P, Farber DL. The role of the thymus in the immune response. Thorac Surg Clinics (2019) 29(2):123–31. doi: 10.1016/j.thorsurg.2018.12.001
2. Thome JJC, Grinshpun B, Kumar BV, Kubota M, Ohmura Y, Lerner H, et al. Long-term maintenance of human naive T cells through in situ homeostasis in lymphoid tissue sites. Sci Immunol (2016) 1(6):eaah6506. doi: 10.1126/sciimmunol.aah6506
3. Czesnikiewicz-Guzik M, Lee W-W, Cui D, Hiruma Y, Lamar DL, Yang Z-Z, et al. T Cell subset-specific susceptibility to aging. Clin Immunol (2008) 127(1):107–18. doi: 10.1016/j.clim.2007.12.002
4. Callender LA, Carroll EC, Beal RWJ, Chambers ES, Nourshargh S, Akbar AN, et al. Human CD8+ EMRA T cells display a senescence-associated secretory phenotype regulated by p38 MAPK. Aging Cell (2018) 17(1):e12675. doi: 10.1111/acel.12675
5. White JT, Cross EW, Burchill MA, Danhorn T, McCarter MD, Rosen HR, et al. Virtual memory T cells develop and mediate bystander protective immunity in an IL-15-dependent manner. Nat Commun (2016) 7(1):11291. doi: 10.1038/ncomms11291
6. Quinn KM, Fox A, Harland KL, Russ BE, Li J, Nguyen THO, et al. Age-related decline in primary CD8+ T cell responses is associated with the development of senescence in virtual memory CD8+ T cells. Cell Rep (2018) 23(12):3512–24. doi: 10.1016/j.celrep.2018.05.057
7. Elyahu Y, Hekselman I, Eizenberg-Magar I, Berner O, Strominger I, Schiller M, et al. Aging promotes reorganization of the CD4 T cell landscape toward extreme regulatory and effector phenotypes. Sci Adv (2019) 5(8):eaaw8330. doi: 10.1126/sciadv.aaw8330
8. Mogilenko DA, Shpynov O, Andhey PS, Arthur L, Swain A, Esaulova E, et al. Comprehensive profiling of an aging immune system reveals clonal GZMK+CD8+ T cells as conserved hallmark of inflammaging. Immunity (2021) 54(1):99–115.e12. doi: 10.1016/j.immuni.2020.11.005
9. Kim C, Hu B, Jadhav RR, Jin J, Zhang H, Cavanagh MM. Activation of miR-21-Regulated pathways in immune aging selects against signatures characteristic of memory T cells. Cell Rep (2018) 25(8):2148–62.e5. doi: 10.1016/j.celrep.2018.10.074
10. Fang F, Yu M, Cavanagh MM, Hutter Saunders J, Qi Q, Ye Z, et al. Expression of CD39 on activated T cells impairs their survival in older individuals. Cell Rep (2016) 14(5):1218–31. doi: 10.1016/j.celrep.2016.01.002
11. Cao W, Fang F, Gould T, Li X, Kim C, Gustafson C, et al. Ecto-NTPDase CD39 is a negative checkpoint that inhibits follicular helper cell generation. J Clin Invest (2020) 130(7):3422–36. doi: 10.1172/JCI132417
12. Jin J, Li X, Hu B, Kim C, Cao W, Zhang H, et al. FOXO1 deficiency impairs proteostasis in aged T cells. Sci Adv (2020) 6(17):eaba1808. doi: 10.1126/sciadv.aba1808
13. Zhang H, Jadhav RR, Cao W, Goronzy IN, Zhao TV, Jin J, et al. Aging-associated HELIOS deficiency in naive CD4+ T cells alters chromatin remodeling and promotes effector cell responses. Nat Immunol (2022) 24:96–109. doi: 10.1038/s41590-022-01369-x
14. Fang F, Cao W, Zhu W, Lam N, Li L, Gaddam S, et al. The cell-surface 5’-nucleotidase CD73 defines a functional T memory cell subset that declines with age. Cell Rep (2021) 37(6). doi: 10.1016/j.celrep.2021.109981
15. Selkoe DJ, Hardy J. The amyloid hypothesis of alzheimer’s disease at 25 years. EMBO Mol Med (2016) 8(6):595–608. doi: 10.15252/emmm.201606210
16. Hyman BT, Phelps CH, Beach TG, Bigio EH, Cairns NJ, Carrillo MC, et al. National institute on aging–alzheimer’s association guidelines for the neuropathologic assessment of alzheimer’s disease. Alzheimer’s Dementia (2012) 8(1):1–13. doi: 10.1016/j.jalz.2011.10.007
17. DeTure MA, Dickson DW. The neuropathological diagnosis of alzheimer’s disease. Mol Neurodegener (2019) 14(1):32. doi: 10.1186/s13024-019-0333-5
18. Walton CC, Begelman D, Nguyen W, Andersen JK. Senescence as an amyloid cascade: The amyloid senescence hypothesis. Front Cell Neurosci (2020) 14:129–. doi: 10.3389/fncel.2020.00129
19. Bettcher BM, Tansey MG, Dorothée G, Heneka MT. Peripheral and central immune system crosstalk in Alzheimer disease — a research prospectus. Nat Rev Neurology (2021) 17(11):689–701. doi: 10.1038/s41582-021-00549-x
20. Yousefzadeh MJ, Flores RR, Zhu Y, Schmiechen ZC, Brooks RW, Trussoni CE, et al. An aged immune system drives senescence and ageing of solid organs. Nature (2021) 594(7861):100–5. doi: 10.1038/s41586-021-03547-7
21. Bussian TJ, Aziz A, Meyer CF, Swenson BL, van Deursen JM, Baker DJ. Clearance of senescent glial cells prevents tau-dependent pathology and cognitive decline. Nature (2018) 562(7728):578–82. doi: 10.1038/s41586-018-0543-y
22. Zhang P, Kishimoto Y, Grammatikakis I, Gottimukkala K, Cutler RG, Zhang S, et al. Senolytic therapy alleviates aβ-associated oligodendrocyte progenitor cell senescence and cognitive deficits in an alzheimer’s disease model. Nat Neurosci (2019) 22(5):719–28. doi: 10.1038/s41593-019-0372-9
23. Desdín-Micó G, Soto-Heredero G, Aranda JF, Oller J, Carrasco E, Gabandé-Rodríguez E, et al. T Cells with dysfunctional mitochondria induce multimorbidity and premature senescence. Science (2020) 368(6497):1371–6. doi: 10.1126/science.aax0860
24. Gate D, Saligrama N, Leventhal O, Yang AC, Unger MS, Middeldorp J, et al. Clonally expanded CD8 T cells patrol the cerebrospinal fluid in alzheimer’s disease. Nature (2020) 577(7790):399–404. doi: 10.1038/s41586-019-1895-7
25. Elyahu Y, Monsonego A. Thymus involution sets the clock of the aging T-cell landscape: Implications for declined immunity and tissue repair. Ageing Res Rev (2021) 65:101231. doi: 10.1016/j.arr.2020.101231
26. den Braber I, Mugwagwa T, Vrisekoop N, Westera L, Mögling R, Bregje de Boer A, et al. Maintenance of peripheral naive T cells is sustained by thymus output in mice but not humans. Immunity (2012) 36(2):288–97. doi: 10.1016/j.immuni.2012.02.006
27. Hale JS, Boursalian TE, Turk GL, Fink PJ. Thymic output in aged mice. Proc Natl Acad Sci (2006) 103(22):8447–52. doi: 10.1073/pnas.0601040103
28. Hsu H-C, Li L, Zhang H-G, Mountz JD. Genetic regulation of thymic involution. Mech Ageing Dev (2005) 126(1):87–97. doi: 10.1016/j.mad.2004.09.016
29. Thomas R, Wang W, Su D-M. Contributions of age-related thymic involution to immunosenescence and inflammaging. Immun Ageing (2020) 17(1):2. doi: 10.1186/s12979-020-0173-8
30. Monsonego A, Zota V, Karni A, Krieger JI, Bar-Or A, Bitan G, et al. Increased T cell reactivity to amyloid beta protein in older humans and patients with Alzheimer disease. J Clin Invest (2003) 112(3):415–22. doi: 10.1172/JCI200318104
31. Browne TC, McQuillan K, McManus RM, O’Reilly J-A, Mills KHG, Lynch MA. IFN-γ production by amyloid β–specific Th1 cells promotes microglial activation and increases plaque burden in a mouse model of alzheimer’s disease. J Immunol (2013) 190(5):2241–51. doi: 10.4049/jimmunol.1200947
32. Oh J, Wang W, Thomas R, Su D-M. Capacity of tTreg generation is not impaired in the atrophied thymus. PloS Biol (2017) 15(11):e2003352. doi: 10.1371/journal.pbio.2003352
33. Coder B, Wang W, Wang L, Wu Z, Zhuge Q, Su D-M. Friend or foe: The dichotomous impact of T cells on neuro-de/re-generation during aging. Oncotarget (2016) 8(4):7116–37. doi: 10.18632/oncotarget.12572
34. Baruch K, Rosenzweig N, Kertser A, Deczkowska A, Sharif AM, Spinrad A, et al. Breaking immune tolerance by targeting Foxp3+ regulatory T cells mitigates alzheimer’s disease pathology. Nat Commun (2015) 6(1):7967. doi: 10.1038/ncomms8967
35. Kunis G, Baruch K, Rosenzweig N, Kertser A, Miller O, Berkutzki T, et al. IFN-γ-dependent activation of the brain’s choroid plexus for CNS immune surveillance and repair. Brain (2013) 136(11):3427–40. doi: 10.1093/brain/awt259
36. Xie L, Choudhury GR, Winters A, Yang S-H, Jin K. Cerebral regulatory T cells restrain microglia/macrophage-mediated inflammatory responses via IL-10. Eur J Immunol (2015) 45(1):180–91. doi: 10.1002/eji.201444823
37. Taves MD, Ashwell JD. Effects of sex steroids on thymic epithelium and thymocyte development. Front Immunol (2022) 13. doi: 10.3389/fimmu.2022.975858
38. Paolino M, Koglgruber R, Cronin SJF, Uribesalgo I, Rauscher E, Harreiter J, et al. RANK links thymic regulatory T cells to fetal loss and gestational diabetes in pregnancy. Nature (2021) 589(7842):442–7. doi: 10.1038/s41586-020-03071-0
39. Xu L, Wei C, Chen Y, Wu Y, Shou X, Chen W, et al. IL-33 induces thymic involution-associated naive T cell aging and impairs host control of severe infection. Nat Commun (2022) 13(1):6881. doi: 10.1038/s41467-022-34660-4
40. Imtiaz B, Tuppurainen M, Rikkonen T, Kivipelto M, Soininen H, Kröger H, et al. Postmenopausal hormone therapy and Alzheimer disease: A prospective cohort study. Neurology (2017) 88(11):1062–8. doi: 10.1212/WNL.0000000000003696
41. Yaffe K, Sawaya G, Lieberburg I, Grady D. Estrogen therapy in postmenopausal WomenEffects on cognitive function and dementia. JAMA (1998) 279(9):688–95. doi: 10.1001/jama.279.9.688
42. Maki PM, Girard LM, Manson JE. Menopausal hormone therapy and cognition. BMJ (2019) 364:l877. doi: 10.1136/bmj.l877
43. Savolainen-Peltonen H, Rahkola-Soisalo P, Hoti F, Vattulainen P, Gissler M, Ylikorkala O, et al. Use of postmenopausal hormone therapy and risk of alzheimer’s disease in Finland: Nationwide case-control study. BMJ (2019) 364:l665. doi: 10.1136/bmj.l665
44. Velardi E, Tsai JJ, van den Brink MRM. T Cell regeneration after immunological injury. Nat Rev Immunol (2021) 21(5):277–91. doi: 10.1038/s41577-020-00457-z
45. Casadesus G, Webber KM, Atwood CS, Pappolla MA, Perry G, Bowen RL, et al. Luteinizing hormone modulates cognition and amyloid-β deposition in Alzheimer APP transgenic mice. Biochim Biophys Acta (BBA) - Mol Basis Disease (2006) 1762(4):447–52. doi: 10.1016/j.bbadis.2006.01.008
46. Butler T, Goldberg JD, Galvin JE, Maloney T, Ravdin L, Glodzik L, et al. Rationale, study design and implementation of the LUCINDA trial: Leuprolide plus cholinesterase inhibition to reduce neurologic decline in alzheimer’s. Contemp Clin Trials (2021) 107:106488. doi: 10.1016/j.cct.2021.106488
47. Sauce D, Larsen M, Fastenackels S, Duperrier A, Keller M, Grubeck-Loebenstein B, et al. Evidence of premature immune aging in patients thymectomized during early childhood. J Clin Invest (2009) 119(10):3070–8. doi: 10.1172/JCI39269
48. Bagge CN, Henderson VW, Laursen HB, Adelborg K, Olsen M, Madsen NL. Risk of dementia in adults with congenital heart disease. Circulation (2018) 137(18):1912–20. doi: 10.1161/CIRCULATIONAHA.117.029686
49. Goronzy JJ, Weyand CM. Mechanisms underlying T cell ageing. Nat Rev Immunol (2019) 19(9):573–83. doi: 10.1038/s41577-019-0180-1
50. Surh CD, Sprent J. Homeostasis of naive and memory T cells. Immunity (2008) 29(6):848–62. doi: 10.1016/j.immuni.2008.11.002
51. Thompson HL, Smithey MJ, Uhrlaub JL, Jeftić I, Jergović M, White SE, et al. Lymph nodes as barriers to T-cell rejuvenation in aging mice and nonhuman primates. Aging Cell (2019) 18(1):e12865. doi: 10.1111/acel.12865
52. Ucar D, Márquez EJ, Chung CH, Marches R, Rossi RJ, Uyar A, et al. The chromatin accessibility signature of human immune aging stems from CD8(+) T cells. J Exp Med (2017) 214(10):3123–44. doi: 10.1084/jem.20170416
53. Cao W, Sturmlechner I, Zhang H, Jin J, Hu B, Jadhav RR, et al. TRIB2 safeguards naive T cell homeostasis during aging. Cell Rep (2023) 42(3). doi: 10.1016/j.celrep.2023.112195
54. van den Broek T, Borghans JAM, van Wijk F. The full spectrum of human naive T cells. Nat Rev Immunol (2018) 18(6):363–73. doi: 10.1038/s41577-018-0001-y
55. Goronzy JJ, Hu B, Kim C, Jadhav RR, Weyand CM. Epigenetics of T cell aging. J Leukocyte Biol (2018) 104(4):691–9. doi: 10.1002/JLB.1RI0418-160R
56. Keenan CR, Allan RS. Epigenomic drivers of immune dysfunction in aging. Aging Cell (2019) 18(1):e12878. doi: 10.1111/acel.12878
57. Cheung P, Vallania F, Warsinske HC, Donato M, Schaffert S, Chang SE, et al. Single-cell chromatin modification profiling reveals increased epigenetic variations with aging. Cell (2018) 173(6):1385–97.e14. doi: 10.1016/j.cell.2018.03.079
58. Moskowitz DM, Zhang DW, Hu B, Saux SL, Yanes RE, Ye Z, et al. Epigenomics of human CD8 T cell differentiation and aging. Sci Immunol (2017) 2(8):eaag0192. doi: 10.1126/sciimmunol.aag0192
59. van der Geest KSM, Abdulahad WH, Teteloshvili N, Tete SM, Peters JH, Horst G, et al. Low-affinity TCR engagement drives IL-2-dependent post-thymic maintenance of naive CD4+ T cells in aged humans. Aging Cell (2015) 14(5):744–53. doi: 10.1111/acel.12353
60. Hu B, Jadhav RR, Gustafson CE, Le Saux S, Ye Z, Li X, et al. Distinct age-related epigenetic signatures in CD4 and CD8 T cells. Front Immunol (2020) 11:575168. doi: 10.3389/fimmu.2020.585168
61. Martinez-Jimenez CP, Eling N, Chen H-C, Vallejos CA, Kolodziejczyk AA, Connor F, et al. Aging increases cell-to-cell transcriptional variability upon immune stimulation. Science (2017) 355(6332):1433–6. doi: 10.1126/science.aah4115
62. Gustafson CE, Cavanagh MM, Jin J, Weyand CM, Goronzy JJ. Functional pathways regulated by microRNA networks in CD8 T-cell aging. Aging Cell (2019) 18(1):e12879. doi: 10.1111/acel.12879
63. Ye Z, Li G, Kim C, Hu B, Jadhav RR, Weyand CM, et al. Regulation of miR-181a expression in T cell aging. Nat Commun (2018) 9(1):3060. doi: 10.1038/s41467-018-05552-3
64. Li G, Yu M, Lee W-W, Tsang M, Krishnan E, Weyand CM, et al. Decline in miR-181a expression with age impairs T cell receptor sensitivity by increasing DUSP6 activity. Nat Med (2012) 18(10):1518–24. doi: 10.1038/nm.2963
65. Lambert S, Cao W, Zhang H, Colville A, Liu J-Y, Weyand CM, et al. The influence of three-dimensional structure on naïve T cell homeostasis and aging. Front Aging (2022) 3. doi: 10.3389/fragi.2022.1045648
66. Hu B, Li G, Ye Z, Gustafson CE, Tian L, Weyand CM, et al. Transcription factor networks in aged naïve CD4 T cells bias lineage differentiation. Aging Cell (2019) 18(4):e12957. doi: 10.1111/acel.12957
67. Dai L, Shen Y. Insights into T-cell dysfunction in alzheimer’s disease. Aging Cell (2021) 20(12):e13511. doi: 10.1111/acel.13511
68. Lindestam Arlehamn CS, Pham J, Alcalay RN, Frazier A, Shorr E, Carpenter C, et al. Widespread tau-specific CD4 T cell reactivity in the general population. J Immunol (2019) 203(1):84–92. doi: 10.4049/jimmunol.1801506
69. Bazioti V, La Rose AM, Maassen S, Bianchi F, de Boer R, Halmos B, et al. T Cell cholesterol efflux suppresses apoptosis and senescence and increases atherosclerosis in middle aged mice. Nat Commun (2022) 13(1):3799. doi: 10.1038/s41467-022-31135-4
70. Larbi A, Dupuis G, Khalil A, Douziech N, Fortin C, Fülöp T. Differential role of lipid rafts in the functions of CD4+ and CD8+ human T lymphocytes with aging. Cell Signalling (2006) 18(7):1017–30. doi: 10.1016/j.cellsig.2005.08.016
71. Shi Y, Holtzman DM. Interplay between innate immunity and Alzheimer disease: APOE and TREM2 in the spotlight. Nat Rev Immunol (2018) 18(12):759–72. doi: 10.1038/s41577-018-0051-1
72. Mesquita SD, Herz J, Wall M, Dykstra T, Lima KAD, Norris GT, et al. Aging-associated deficit in CCR7 is linked to worsened glymphatic function, cognition, neuroinflammation, and β-amyloid pathology. Sci Adv (2021) 7(21):eabe4601. doi: 10.1126/sciadv.abe4601
73. Akbar AN, Henson SM, Lanna A. Senescence of T lymphocytes: Implications for enhancing human immunity. Trends Immunol (2016) 37(12):866–76. doi: 10.1016/j.it.2016.09.002
74. Pereira BI, De Maeyer RPH, Covre LP, Nehar-Belaid D, Lanna A, Ward S, et al. Sestrins induce natural killer function in senescent-like CD8+ T cells. Nat Immunol (2020) 21(6):684–94. doi: 10.1038/s41590-020-0643-3
75. Akbar AN, Henson SM. Are senescence and exhaustion intertwined or unrelated processes that compromise immunity? Nat Rev Immunol (2011) 11(4):289–95. doi: 10.1038/nri2959
76. Hashimoto K, Kouno T, Ikawa T, Hayatsu N, Miyajima Y, Yabukami H, et al. Single-cell transcriptomics reveals expansion of cytotoxic CD4 T cells in supercentenarians. Proc Natl Acad Sci (2019) 116(48):24242–51. doi: 10.1073/pnas.1907883116
77. Serroukh Y, Gu-Trantien C, Hooshiar Kashani B, Defrance M, Vu Manh T-P, Azouz A, et al. The transcription factors Runx3 and ThPOK cross-regulate acquisition of cytotoxic function by human Th1 lymphocytes. eLife (2018) 7:e30496. doi: 10.7554/eLife.30496.036
78. Smolders J, Heutinck KM, Fransen NL, Remmerswaal EBM, Hombrink P, ten Berge IJM, et al. Tissue-resident memory T cells populate the human brain. Nat Commun (2018) 9(1):4593. doi: 10.1038/s41467-018-07053-9
79. Pereira BI, Devine OP, Vukmanovic-Stejic M, Chambers ES, Subramanian P, Patel N, et al. Senescent cells evade immune clearance via HLA-e-mediated NK and CD8+ T cell inhibition. Nat Commun (2019) 10(1):2387. doi: 10.1038/s41467-019-10335-5
80. Ovadya Y, Landsberger T, Leins H, Vadai E, Gal H, Biran A, et al. Impaired immune surveillance accelerates the accumulation of senescent cells and aging. Nat Commun (2018) 9(1):5435. doi: 10.1038/s41467-018-07825-3
81. Hazeldine J, Hampson P, Lord JM. Reduced release and binding of perforin at the immunological synapse underlies the age-related decline in natural killer cell cytotoxicity. Aging Cell (2012) 11(5):751–9. doi: 10.1111/j.1474-9726.2012.00839.x
82. Wang T-W, Johmura Y, Suzuki N, Omori S, Migita T, Yamaguchi K, et al. Blocking PD-L1–PD-1 improves senescence surveillance and ageing phenotypes. Nature (2022) 611(7935):358–64. doi: 10.1038/s41586-022-05388-4
83. Unger MS, Li E, Scharnagl L, Poupardin R, Altendorfer B, Mrowetz H, et al. CD8+ T-cells infiltrate alzheimer’s disease brains and regulate neuronal- and synapse-related gene expression in APP-PS1 transgenic mice. Brain Behavior Immunity (2020) 89:67–86. doi: 10.1016/j.bbi.2020.05.070
84. Stojić-Vukanić Z, Nicole O, Nacka-Aleksić M, Leštarević S, Leposavić G. CD8+ T cell-mediated mechanisms contribute to the progression of neurocognitive impairment in both multiple sclerosis and alzheimer’s disease? Front Immunol (2020) 11. doi: 10.3389/fimmu.2020.566225
85. Kaya T, Mattugini N, Liu L, Ji H, Cantuti-Castelvetri L, Wu J, et al. CD8+ T cells induce interferon-responsive oligodendrocytes and microglia in white matter aging. Nat Neurosci (2022) 25(11):1446–57. doi: 10.1038/s41593-022-01183-6
86. Groh J, Knöpper K, Arampatzi P, Yuan X, Lößlein L, Saliba A-E, et al. Accumulation of cytotoxic T cells in the aged CNS leads to axon degeneration and contributes to cognitive and motor decline. Nat Aging (2021) 1(4):357–67. doi: 10.1038/s43587-021-00049-z
87. Saresella M, Calabrese E, Marventano I, Piancone F, Gatti A, Alberoni M, et al. Increased activity of Th-17 and Th-9 lymphocytes and a skewing of the post-thymic differentiation pathway are seen in alzheimer’s disease. Brain Behavior Immunity (2011) 25(3):539–47. doi: 10.1016/j.bbi.2010.12.004
88. Oberstein TJ, Taha L, Spitzer P, Hellstern J, Herrmann M, Kornhuber J, et al. Imbalance of circulating Th17 and regulatory T cells in alzheimer’s disease: A case control study. Front Immunol (2018) 9. doi: 10.3389/fimmu.2018.01213
89. Wang X, Sun G, Feng T, Zhang J, Huang X, Wang T, et al. Sodium oligomannate therapeutically remodels gut microbiota and suppresses gut bacterial amino acids-shaped neuroinflammation to inhibit alzheimer’s disease progression. Cell Res (2019) 29(10):787–803. doi: 10.1038/s41422-019-0216-x
90. Zhang J, Ke K-F, Liu Z, Qiu Y-H, Peng Y-P. Th17 cell-mediated neuroinflammation is involved in neurodegeneration of Aβ1-42-Induced alzheimer’s disease model rats. PloS One (2013) 8(10):e75786. doi: 10.1371/journal.pone.0075786
91. McManus RM, Higgins SC, Mills KHG, Lynch MA. Respiratory infection promotes T cell infiltration and amyloid-β deposition in APP/PS1 mice. Neurobiol Aging (2014) 35(1):109–21. doi: 10.1016/j.neurobiolaging.2013.07.025
92. Brigas HC, Ribeiro M, Coelho JE, Gomes R, Gomez-Murcia V, Carvalho K, et al. IL-17 triggers the onset of cognitive and synaptic deficits in early stages of alzheimer’s disease. Cell Rep (2021) 36(9). doi: 10.1016/j.celrep.2021.109574
93. Cristiano C, Volpicelli F, Lippiello P, Buono B, Raucci F, Piccolo M, et al. Neutralization of IL-17 rescues amyloid-β-induced neuroinflammation and memory impairment. Br J Pharmacol (2019) 176(18):3544–57. doi: 10.1111/bph.14586
94. Cipollini V, Anrather J, Orzi F, Iadecola C. Th17 and cognitive impairment: Possible mechanisms of action. Front Neuroanat (2019) 13. doi: 10.3389/fnana.2019.00095
95. Machhi J, Yeapuri P, Lu Y, Foster E, Chikhale R, Herskovitz J, et al. CD4+ effector T cells accelerate alzheimer’s disease in mice. J Neuroinflammation. (2021) 18(1):272. doi: 10.1186/s12974-021-02308-7
96. Fisher Y, Strominger I, Biton S, Nemirovsky A, Baron R, Monsonego A. Th1 polarization of T cells injected into the cerebrospinal fluid induces brain immunosurveillance. J Immunol (2014) 192(1):92–102. doi: 10.4049/jimmunol.1301707
97. Mittal K, Eremenko E, Berner O, Elyahu Y, Strominger I, Apelblat D, et al. CD4 T cells induce a subset of MHCII-expressing microglia that attenuates Alzheimer pathology. iScience (2019) 16:298–311. doi: 10.1016/j.isci.2019.05.039
98. Baruch K, Deczkowska A, Rosenzweig N, Tsitsou-Kampeli A, Sharif AM, Matcovitch-Natan O, et al. PD-1 immune checkpoint blockade reduces pathology and improves memory in mouse models of alzheimer’s disease. Nat Med (2016) 22(2):135–7. doi: 10.1038/nm.4022
99. Raynor J, Lages CS, Shehata H, Hildeman DA, Chougnet CA. Homeostasis and function of regulatory T cells in aging. Curr Opin Immunol (2012) 24(4):482–7. doi: 10.1016/j.coi.2012.04.005
Keywords: T cell aging, Alzheimer’s disease (AD), thymic involution, senescence, neuroinflammation
Citation: Guo L, Li X, Gould T, Wang Z-Y and Cao W (2023) T cell aging and Alzheimer’s disease. Front. Immunol. 14:1154699. doi: 10.3389/fimmu.2023.1154699
Received: 31 January 2023; Accepted: 15 March 2023;
Published: 04 April 2023.
Edited by:
Mingzhu Zheng, Southeast University, ChinaReviewed by:
Ryu Watanabe, Osaka Metropolitan University, JapanQinghui Mu, Stanford University, United States
Copyright © 2023 Guo, Li, Gould, Wang and Cao. This is an open-access article distributed under the terms of the Creative Commons Attribution License (CC BY). The use, distribution or reproduction in other forums is permitted, provided the original author(s) and the copyright owner(s) are credited and that the original publication in this journal is cited, in accordance with accepted academic practice. No use, distribution or reproduction is permitted which does not comply with these terms.
*Correspondence: Wenqiang Cao, d3FjYW9AY211LmVkdS5jbg==; Zhan-You Wang, d2FuZ3p5QGNtdS5lZHUuY24=
†These authors have contributed equally to this work