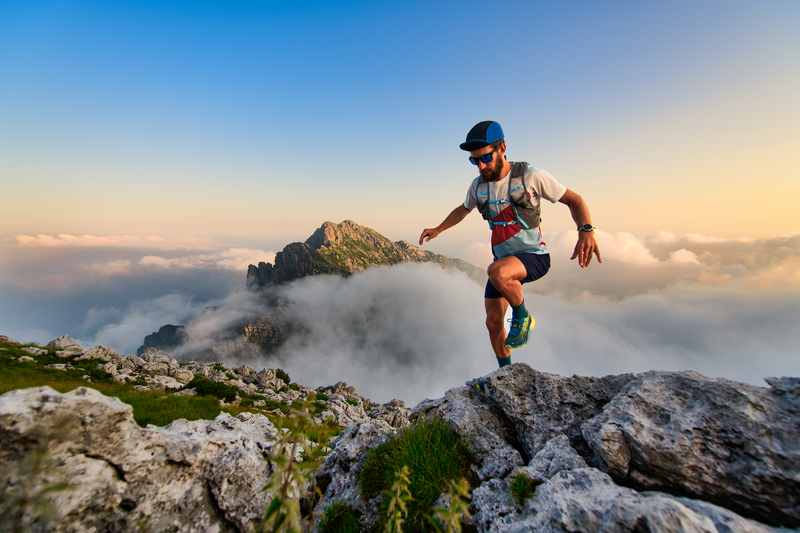
95% of researchers rate our articles as excellent or good
Learn more about the work of our research integrity team to safeguard the quality of each article we publish.
Find out more
REVIEW article
Front. Immunol. , 20 March 2023
Sec. Vaccines and Molecular Therapeutics
Volume 14 - 2023 | https://doi.org/10.3389/fimmu.2023.1153042
This article is part of the Research Topic Plant-derived Vaccine Adjuvants View all 8 articles
Respiratory infections are a major public health concern caused by pathogens that colonize and invade the respiratory mucosal surface. Nasal vaccines have the advantage of providing protection at the primary site of pathogen infection, as they induce higher levels of mucosal secretory IgA antibodies and antigen-specific T and B cell responses. Adjuvants are crucial components of vaccine formulation that enhance the immunogenicity of the antigen to confer long-term and effective protection. Saponins, natural glycosides derived from plants, shown potential as vaccine adjuvants, as they can activate the mammalian immune system. Several licensed human vaccines containing saponins-based adjuvants administrated through intramuscular injection have demonstrated good efficacy and safety. Increasing evidence suggests that saponins can also be used as adjuvants for nasal vaccines, owing to their safety profile and potential to augment immune response. In this review, we will discuss the structure-activity-relationship of saponins, their important role in nasal vaccines, and future prospects for improving their efficacy and application in nasal vaccine for respiratory infection.
The human respiratory tract is a common entry point for various infectious microorganisms, including viruses such as coronavirus, respiratory syncytial virus (RSV), and influenza virus, as well as bacteria such as Streptococcus pneumoniae, Staphylococcus aureus, Acinetobacter baumannii, and Mycobacterium tuberculosis, which cause a significant global health concern, especially for older, or immunocompromised people (1–3). Vaccination is a cost-effective and effective way to prevent these infections. Although systemic vaccines against severe acute respiratory syndrome coronavirus 2 (SARS-CoV-2) or influenza virus can induce robust systemic immunity, they are not sufficient to prevent virus transmission and only reduce the development of severe disease (4, 5). Thus, a vaccine regimen that promotes mucosal immune responses in respiratory tract is crucial in preventing pathogens invasion (5–7).
Nasal vaccines are considered as a promising strategy by inducing antigen-specific protective immune responses in both mucosal surfaces and throughout the body. The respiratory route has unique physiological and immunological characteristics, and the nasopharyngeal-associated lymphoid tissue (NALT) is a key induction site for mucosal immunity after nasal vaccination (8). Compared to common injectable vaccines, nasal vaccination is more likely to elicit a robust mucosal response, characterized by antigen-specific T cell response in combination with secretory immunoglobulin A (sIgA) (9). Secreted IgA helps bind and eliminate pathogens before they can cause an infection (7). In addition to inducing mucosal immune response, nasal vaccine can also elicit systemic immune responses (7). Therefore, nasal vaccines are an attractive strategy for combating pathogens that use the respiratory tract as an entry point into the body. Furthermore, nasal vaccination has several benefits, such as a larger mucosal surface area for antigen uptake and convenience of being needle-free and easy to self-administer.
So far, only three nasal vaccines have been licensed for human use. The first is FluMist™, an attenuated influenza virus vaccine, approved by U.S. Food and Drug Administration (FDA) (10). The other two are SARS-CoV-2 vaccines, one is iNCOVACC™, a chimpanzee adenovirus-vectored SARS-CoV-2 vaccine licensed for restricted use in emergencies in India (11, 12); Another is CA4-dNS1-nCoV-RBD, a nasal spray influenza virus vector vaccine approved in China for emergency use (13). Despite the potential benefits of these vaccines, there are safety concerns since they use live viruses. Recombinant protein-based subunit vaccines are a safer alternative, but their immunogenicity is often weak, making it challenging to stimulate mucosal immunity. Meanwhile, the unique physiological and immunologic properties of the respiratory mucosa, such as the mucus layer and cilia movement, can pose obstacles in the development of nasal vaccines, and pH and enzyme conditions can degrade antigens (14). To overcome these challenges, adjuvants play a crucial role in enhancing antigen immunogenicity and vaccine efficacy in the respiratory mucosa (15).
Adjuvants are important components of vaccine formulations together with antigens and function to enhance the immunogenicity of the co-inoculated antigens to confer long-term and effective protection against pathogens (16). They can be broadly categorized into immunostimulatory molecules and delivery systems that transport the vaccines to the immune system (16). While various mucosal adjuvants such as cholera toxin, Escherichia coli heat-labile toxin, polyethyleneimine, alum, chitosan, and others have been tested in experimental subunit vaccines for respiratory infections, none of them have been approved for use in nasal vaccines (17).
Saponins, which are extracted from plants, have generated great interest as vaccine adjuvants due to their multiple biological and immunomodulatory properties (18). Quil A and its purified fraction QS-21 are the most widely used saponins as adjuvants, owing to their exceptional ability to enhance antibody responses and activate T helper type 1 cells (Th1) and cytotoxic T lymphocyte (CTL) immune responses by activating dendritic cells (DCs) (19, 20). Additionally, QS-21-based adjuvants, including adjuvant systems (AS01, AS02, et al.) and immunostimulating complexes (ISCOMs) have been developed with improved adjuvant effects and lower toxicity (19). AS01 has been successfully used as an adjuvant in the licensed vaccines, including the herpes zoster vaccine (Shingrix®) and the malaria RTS,S/AS01vaccine (Mosquirix™), showing their good efficacy and safety (20, 21). Currently, licensed adjuvants containing saponins are administrated intramuscularly. Saponins have also been used as adjuvants in nasal vaccines in several experiments and they are believed to have great potential to elicit strong mucosal and systemic immune responses (22). In this review, we aim to explain the structure-activity relationship of saponins, the crucial role of saponin-derived adjuvants in nasal vaccines, and provide insights into the future research on saponins-based adjuvants.
Saponins are a type of naturally occurring glycosides that are found in many plants. They consist of a steroid or triterpene trunk linked to one or more carbohydrate chains (23, 24). The structure of saponins varies greatly, which contributes to the diverse biological activities that they possess, such as immunomodulatory, anti-tumor, anti-inflammatory, antiviral, antifungal, cholesterol-lowering, and others (25). The adjuvant activity of saponins is mainly attributed to their ability to activate the mammalian immune system (25). Quil A, a saponin mixture extracted from the bark of the South American tree Quillaja saponaria Molina, has been widely used as an adjuvant due to its good adjuvant activity (26, 27). The purified fraction of Quil A, known as QS-21, was later separated using reversed-phase high performance liquid chromatography (RP-HPLC) and showed better adjuvant activity with lower toxicity (28). QS-21 has since become a popular adjuvant in vaccine studies and has been included in some approved vaccines (29).
QS21 is a triterpene glycoside that is soluble in water and consists of two isomers, QS-21 Apiose (QS-21 Api) and QS-21 Xylose (QS-21-Xyl) in a 2:1 ratio (Figure 1) (24). It features a central quillaic acid triterpene core, surrounded by complex oligosaccharide chains that are attached to C-3 and C-28 positions of the triterpene aglycone (Figure 1). The trisaccharide moiety at the C3 position is made up of d-glucuronic acid, d-galactose, and d-xylose. A linear tetrasaccharide, consisting of d-fucose, l-rhamnose, d-xylose, and either d-apiose or d-xylose (for QS-21Api or QS-21Xyl, respectively), is attached at the C-28 carboxylate of the triterpene via an ester bond. Finally, the triterpene saponins are completed by a structurally complex l-arabinose-terminated fatty acyl chain linked to the 4-position of the fucose residue, making them amphiphilic in nature (Figure 1) (30).
Figure 1 Structure of QS-21. QS-21 contains a central quillaic acid triterpene core, which is surrounded by complex oligosaccharide chains that are attached to C-3 and C-28 positions of the triterpene aglycone. The triterpene is essential for antigen cross-presentation and activation of innate immunity. The C4-aldehyde substituent on the triterpene is involved in the formation of Schiff base with amino groups on T cell surface receptors and providing a co-stimulatory signal to activate T cell. The acyl chain on QS-21 is associated with CTL responses. The branched trisaccharide is dispensable for activity. Linear tetracosaccharides can be structurally-modified to study the in vivo biological distribution of QS-21. Its carbohydrate domain involves in the uptake of antigen by APCs and the stimulation of specific cytokines that activate cellular and/or humoral responses.
The underlying structure basis for the adjuvant activity of saponins has been extensively studied. Factors such as branched sugar chains, aldehyde groups, and an acyl residue on the aglycone can contribute to the adjuvant activity. QS-21 may bind to cell surface lectins through its carbohydrate domain, leading to the uptake of antigen by antigen-presenting cells (APCs) and the stimulation of specific cytokines that activate cellular and/or humoral responses (19, 31). The aldehyde group on the triterpene has been identified as crucial for adjuvanticity of saponins, as QS-21 derivatives modified at an aldehyde did not exhibit adjuvant activity for antibody stimulation or induction of CTL responses (32). The imine-forming carbonyl group can also form Schiff bases with amino groups on T cell surface receptors, leading to co-stimulation for T cell activation and inducing Th1 immunity and a CTL specific response (32, 33). The acyl chains on QS-21 have been associated with cytotoxic T-cell proliferation activity, since the removal of the acyl chain has been shown to be inactive for stimulation of antibody and CTL responses (24, 34). However, other saponins, such as soyasaponins and lablabosides, have been shown to have strong adjuvant activity despite lacking acyl residues (35). These studies suggest that acyl chain might contribute to the adjuvant activity, but not play an essential role. The amphiphilic structure of saponins, with a hydrophobic aglycone backbone and hydrophilic sugar side chains, has been related to the adjuvant activity (30, 36, 37). The triterpene’s affinity for cholesterol is essential for antigen cross-presentation and QS-21 destabilizes lysosomal membranes through cholesterol-dependent cytocytosis, leading to activation of innate immunity (19, 31, 38). Additionally, the amphiphilic nature of saponins makes them easy to formulation into other adjuvant complexes, such as liposomes or nanoparticle.
In addition to Quil A and QS-21, other plant sources of saponins with immune-stimulatory properties and low toxicities have been investigated, such as Quillaja brasiliensis, Panax notoginseng (39), Panax ginseng (40), Platycodon grandiflorum (41), Pulsatilla chinensis (42), Soybeans (43), Polygala tenuifolia (44), and Paris polyphylla (45). However, the limited availability of these sources, low isolation yields, and high purification costs have led to the exploration of other methods to obtain new saponins. Modification and synthesis of saponins are being developed to create more effective adjuvants with lower toxicity. The goal is to develop synthetic saponins with improved adjuvant-antigen activity and lower toxicity (46). For example, Shirahata et al. prepared a series of new simplified oleanolic acid saponins with a glycosyl ester moiety at C28 and found that cinnamoyl esterification of the glucose residue at C-28 was critical for providing mucosal adjuvant activity after intranasal immunization (46). Synthetic or semisynthetic saponins, such as GPI-0100, a semi-synthetic derivative of Quil A, and VSA-1, a newly developed semisynthetic analog of QS-21, have showed shows promising immunostimulatory activity in enhancing the immune responses (47–49).
Saponins are well-known for their ability to induce systemic immune responses when co-injected with antigen via intramuscular immunization (36). Additionally, saponins are effective as mucosal adjuvants when delivered intranasally (Table 1). Intranasal administration of a DNA vaccine encoding the envelope of human immunodeficiency virus type 1 (HIV-1) along with QS-21 as an adjuvant has been shown to increase both systemic and mucosal immune responses against HIV-1, including production of intestinal sIgA and cytolytic activity of mesenteric lymph node cells (50). Saponins derived from Polygala tenuifolia or Chenopodium quinoa have demonstrated enhanced antigen-specific immunoglobulin G (IgG) and local IgA responses to co-administered antigens in lungs when used as adjuvants following intranasal administration (44, 51). In another study, QS-21 loaded on liposome induced higher levels of sIgA compared to liposome without QS-21 when used as an adjuvant for a tetanus toxoid antigen after nasal administration (52). The use of oleanolic acid 28-cinnamoylglucoside as an adjuvant in a nasal-administered influenza split vaccine showed a slight but statistically significantly enhancement in anti-influenza virus sIgA in the nasal washes (46). Moreover, intranasal immunization of saponins-adjuvant vaccines showed protective effects against influenza virus and Toxoplasma gondii cysts challenge (44, 49, 53, 54). These studies highlight the potential of saponins as adjuvants for nasal vaccines.
ISCOMs was first described in the 1980s by Morein et al. as a novel type of immunostimulating complex. It has a spherical cage-like structure composed of saponin, cholesterol, phospholipids, and antigens (55, 56). On the other hand, ISCOMsATRIX (IMX), also called empty ISCOMs, has similar structure and composition to ISCOMs but without the incorporated antigens (56, 57). Both ISCOMs and IMX have been found to be highly immunostimulating due to the combination of an in-built adjuvant (Quil A) with a particulate delivery system. Moreover, they are less toxic and do not have hemolytic activity due to the embedding of saponins into cholesterol (56). The assembly of ISCOMs relies on hydrophobic interactions, making them suitable for incorporation of hydrophobic antigens derived from envelope viruses or cell membranes. In contrast, IMX has a negatively charged surface, which enables it easily to interact with a broad range of positively charged antigens to make IMX vaccines (57). However, this approach limits the binding of neutral or negatively charged hydrophilic antigens to IMX (57). Thus, further research is needed to expand the range of antigens available for IMX vaccines and simplify the production process (58). ISCOMs and IMX vaccines have been developed for a variety of diseases, including viruses, bacteria, parasites, and tumors (56). Both have shown good safety and well-tolerated in animal and human studies, with the ability to induce strong antigen-specific cellular or humoral immune responses (56, 59). There are several ISCOMs vaccines registered for veterinary use, but no ISCOMs or IMX vaccines have been approved for human use yet (60).
Studies have demonstrated that intranasal administration of ISCOMs or IMX vaccines with a variety of antigens can elicit potent mucosal cellular and humoral immune responses, including local IgG and sIgA antibodies in the respiratory tract, as well as systemic and distal mucosal responses, such as in the genital and intestinal tracts (61–70). Pulmonary delivery of ISCOMs or IMX vaccines has also been shown to induce both systemic and mucosal antibody responses against various antigens, including those of influenza virus and Mycobacterium tuberculosis (71–73). Protective efficacy of intranasal immunization with ISCOMs or IMX vaccines has been demonstrated in several studies against various pathogens such as influenza virus, Helicobacter pylori, Angiostrongylus costaricensis, and Eimeria tenella (74–80). These findings highlight the great potential of ISCOMs or IMX as adjuvants for nasal vaccine development (Table 2) (22).
Since the 1990s, saponins-based adjuvants have moved forward to combine saponins with other adjuvants to provide a synergistic adjuvant effect. GlaxoSmithKline (GSK) biologicals developed several Adjuvant Systems (AS) that incorporate QS-21 with other immunostimulants in different formulations (81). For instance, AS01 is a liposome-based system containing QS-21 and 3-O-desacyl–monophosphoryl lipid A (MPL), while AS02 contains QS-21 and MPL in an oil-in-water emulsion (81). AS05 is a liposome-based system with QS-21, MPL, and alum, while AS15 contains QS-21, MPL, and CpG7909 (82, 83). The U.S. Army has also developed an adjuvant called Army Liposome Formulation containing QS21 (ALFQ) which with different liposome properties to AS01 (84). MPL, a detoxifying lipid from Salmonella Minnesota LPS, is a Toll-like receptor 4 (TLR4) agonist and promotes the production of pro-inflammatory cytokines by activating APCs (85, 86). The combination of QS-21 and MPL in AS enhances innate immunity, stimulates antigen-specific T cell responses, and converts mouse antibodies to IgG2c subtype (87, 88). The use of saponins in combination with cholesterol in adjuvant complexes can also reduce hemolytic toxicity. These adjuvant systems have shown good effects in various vaccines against pathogens such as malaria, tuberculosis, SARS-CoV-2, HIV, and Campylobacter jejuni (20, 88–93). In particular, AS01 has been licensed for use in the malaria RTS,S/AS01 vaccine and herpes zoster vaccine (Shingrix®) (94). However, there are currently no reports of the use of ASs or ALFQ in nasal vaccines.
AS01, AS05, AS15, and ALFQ are liposome-based adjuvants. Liposomes can protect antigens from degradation and increase antigens absorption across the nasal epithelium, which can prolong the time antigens remain in the respiratory tract (95). They are biocompatible, biodegradable, and safe for nasal vaccine development (95). Intranasal vaccination of influenza vaccine with liposome-based adjuvants has been shown to protect mice from both homologous and heterologous influenza virus challenge (96). Therefore, as liposome-based adjuvants, ASs and ALFQ could be promising options for nasal vaccines development, which needs further investigation.
Matrix M is the third generation of ISCOMs technology that contains two matrix particles, Matrix-A and Matrix-C, each made from a different, well-characterized saponin fraction from purified Quillaja saponin fractions A and C, along with cholesterol and phospholipids (97, 98). Matrix-C is a highly adjuvant active saponin, while Matrix-A is a weaker but very well-tolerated saponin. The optimal ratio of mixture of Matrix-A and Matrix-C can be explored with co-administering the antigen to achieve the best balance of adjuvant activity and safety (98, 99). Matrix M has been shown to effectively activate and recruit immune cells such as DCs, B cells, and T cells to draining lymph nodes, resulting in strong cellular and humoral immune responses (100, 101). It has been used in clinical trials for vaccines against influenza, malaria, and SARS-CoV-2 (98, 99, 102–104). Interestingly, Matrix-M has mucosal adjuvant properties that enhance mucosal immune responses. Intranasal immunization of Matrix-M (in a ratio of 91:9 of Matrix-A and Matrix–C) adjuvanted a virosomal influenza H5N1 vaccine elicited a significant cross-reactive serum antibody response and protected against a highly pathogenic viral challenge in a mouse model (105). Another study found that intranasal immunization of mice with a Matrix-M-adjuvanted DNA vaccine encoding the P6 outer membrane protein of nontypeable Haemophilus influenza resulted in the induction of P6-specific nasal IgA and serum IgG, as well as enhanced bacterial clearance (Table 3) (106). These results highlight that Matrix-M adjuvant is a promising mucosal adjuvant for nasal vaccines formulations.
The exact mechanism of action of saponins-based adjuvants after nasal administration is not yet well-understood. However, based on the unique immune structure of the nose and the known mechanism of saponins-based adjuvants (20), the current understanding of how they work as effective adjuvants for nasal vaccine can be reviewed (Figure 2).
The mucosal immune system is composed of inductive and effector sites. NALT and local and regional cervical draining lymph nodes are induction sites that can trigger both systemic and local mucosal immune responses following nasal vaccination (8, 107). NALT is an organized structure that contains all the immune cells and is covered by a lymphoid epithelium with microfold (M) cells, which are specialized for antigen uptake (8, 107). M cells can transport antigens and adjuvants to APCs, such as DCs, which accumulate immediately below the epithelium and M cells. The APCs phagocytose, process and present antigens to the surrounding naive T cells (108, 109) (Figure 2A). Vaccines administered nasally can partially pass into the lungs, where alveolar macrophages take them into the interstitium and to draining lymph nodes to activate DCs (110, 111) (Figure 2B). Saponins-based adjuvants in liposome or ISCOMs can deliver antigens to epithelial cells and APCs. The negatively charged IMX nanoparticles have mucoadhesion properties, and the intranasal administration of IMX vaccine has been shown to be retained in the nasal cavity for a longer period of time, allowing antigens to access immune inductive sites in the nasal mucosa and inducing to high mucosal and systemic immune responses (57). Saponins can activate the production of cytokines by resident innate cells, including stromal cells, which then recruit neutrophils, monocyte, and DCs into the respiratory tract to take up more antigens (20).
Figure 2 The mechanisms of action of saponins-based adjuvant in nasal vaccines. (A) Saponins-based adjuvants promote antigen delivery and uptake by nasal epithelial cells or M cells to the underlying lymphoid follicles. Then dendritic cells (DCs) internalize antigens and adjuvants to be activated and present antigens to stimulate T cells. With the help of activated T cells, the activation of B cells further leads to the formation of the germinal center (GC) in the nasal lymphoid follicles. Afterwards, activated B cells differentiate into plasma cells that secrete IgA, which forms a dimer and is transported back to the effect site of the nasal mucosa, where it provides antigen-specific targeting of respiratory pathogens. Meanwhile, saponins-activated DCs carry antigens to migrate to regional lymph nodes, where they interact with T and B cells to generate antigen-specific T cell and B cells. (B) Nasal vaccines, passed through nose to the lung, may also be phagocytized by alveolar macrophages or DCs, and then taken into the interstitium and to hilar lymph nodes. (C) In draining lymph node, DCs present antigens to corresponding B- or T-cell to elicit adaptive immune responses. (D) Activated B cells and T cells enter the blood circulation to distant systemic or mucosal sites to induce systemic immune responses.
Proper induction of innate immune response in the respiratory mucosal and draining lymph nodes is crucial for the quality and magnitude of the adaptive responses and vaccine efficacy. Saponins, such as AS01 and QS-21, have been shown to activate and stimulate APCs to release inflammatory cytokines at draining lymph nodes when administrated intramuscularly (88). Saponins can activate innate immune response by binding to the lectin receptors in the innate immune cells, such as DC-SIGN on DCs, and eliciting cytokines and chemokines production in APCs (38, 88, 112) (Figure 2A), which leads to a rapid and substantial influx of neutrophils, monocytes, DCs and T-cell populations in the lymph node. AS01 can also enhance the expression of the costimulatory molecules such as CD86 and CD40 on the cell surface of APCs (88). The activated innate immune responses are largely resolved by day 7 (88). These immunostimulant responses could also occur in the respiratory mucosa, NALT, and cervical lymph node, when saponins are delivered intranasally (Figure 2A). As intranasal delivery of IMX-adjuvanted human T-cell lymphotropic virus type 1 vaccine was shown to modulate cytokines expression, with increased IFN-γ and IL-10 expression and decreased TGF-β1 levels (68).
The induction of antigen-specific resident memory cells is important for long-term protection against pathogens, which is where mucosal vaccines play a critical role. In NALT or draining lymph node, activated DCs (by saponins and antigens) are capable of activating T cells that differentiate into effector cells and later into memory cells. Activated CD4+ T cells, especially follicular helper T cells (Tfh) induce the development of IgA-secreting B cells in NALT or draining lymph nodes (Figure 2A, C). AS01 may activate Tfh responses, which are correlated with antigen specific IgG and memory B cells (67, 113–115). The resulting B lymphocytes migrate locally to the lymphatic follicles and proliferate in the germinal center, leading to mucosal and systemic immune responses characterized by the secretion of IgA and IgG, respectively (15). Antigen-specific dimeric immunoglobulin A (dIgA) is transported by epithelial cells through polymeric immunoglobulin receptors (pIgRs) and released as sIgA into the nasal lumen (116, 117) (Figure 2A). The antigen and adjuvant-loaded DCs the mature and migrate to the follicular B-cell areas and interfollicular T-cell zone in local draining lymph nodes of nasal tissue or lungs, where they present the antigen to neighboring naive T cells, triggering adaptive immune responses (Figure 2C) (106, 118). T cells and B lymphoblasts activated by APCs migrate throughout the body via the circulatory system, such as Intranasal immunization of Matrix-M adjuvanted influenza vaccine can activate antigen-specific CD4+ and CD8+ T cells responses in spleen (105). In addition, immune cells can diffuse through the common mucosal immune system that connects the induction site to the effector sites (Figure 2D) (119). Thus, adaptive immune responses are not confined to the site of induction, but also occur at distant mucosal region (63, 119) (Figure 2D). Such as, ISCOMs vaccines after nasal administration can induce local antibody secreting cells, CTL, and memory B and T cell responses in lungs (62, 63, 120, 121). The underlying mechanism of saponins to induce mucosal and systemic immune response after intranasal administration requires further study.
Antigen-specific T cells and IgA+ B cells that are induced in NALT and lymph nodes migrate to the mucosa of the respiratory tract through the thoracic duct and circulation. At these effector sites, the IgA+ B cells differentiate to IgA+ plasma cells to secret sIgA, which is very important in preventing infections by inhibiting the adhesion, invasion, and spread of pathogens to epithelial cells (15, 16, 116) (Figure 2A). Intranasal immunizations with ISCOMs vaccines containing different antigens induced not only local IgG and sIgA antibody production in the lungs, but also in distal mucosal system (61, 63–65). The antigen-specific T cells that homing to mucosal region can further differentiate into tissue-resident memory cells that express cell surface markers such as the CD69 and CD103. These memory cells persist in the mucosal tissue for extended periods without entering the circulation and provide a front-line defense against pathogenic invasion by rapidly reactivation in response to antigenic pathogens. Numerous studies have shown that intranasal administration of saponins can induce sIgA production in the respiratory mucosa (Tables 1-3) (Figure 2A) (44, 50, 53, 61, 64, 68). Further research is needed to understand the regulation of tissue-resident memory T cell and B cell responses triggered by nasal administration of saponins.
Ensuring the safety of the effective adjuvants is the first priority in vaccine development, particularly for those based on saponins used in nasal vaccine, where their safety profile in humans is not yet fully established. Saponins are amphiphilic compounds containing both hydrophobic and hydrophilic regions and their hydrophobic regions can interact with the cell membranes of red blood cells and disrupt the membrane integrity, leading to hemolysis. To mitigate the toxicity, saponins can be formulated with other components, such as cholesterol used in AS01 and ISCOMs, which can interact with the hydrophobic region of saponins to prevent their interaction with cell membranes (122). The adjuvant effects of saponins are dependent on their immunostimulatory effect, which can stimulate transient inflammation at the injection site. The effects usually are mild and transient, returning to normal within several days after administration. However, high dose of adjuvants may cause strong inflammation or cell death. Although saponins and saponin-based adjuvants are generally considered safe for parenteral immunization (93, 102, 106 (90, 99, 102, 103, 106, 123), they have been associated with some adverse effects, including local pain, redness, swelling, and fever, especially at the injection site (124, 125). So, intranasal administration of saponin-based adjuvants may induce inflammation and cellular damage in respiratory tract and lungs at high doses, which is a safety concern that requires careful evaluation in the development of nasal vaccines (50). Another important safety concern for nasal adjuvants is their potential side-effects on the central nervous system, since they may be transported from the olfactory epithelium to central nervous system (126, 127). Thus, evaluating the local and systemic toxicities of nasal vaccines containing saponin-derived adjuvants is crucial, which should be carefully evaluated for a good balance of efficacy and side effects in pre-clinical and clinical studies.
Successful development of saponin-based adjuvants for nasal vaccines requires consideration of the unique physiological, chemical, and immunological properties of the nasal cavity. The physiobiological barrier system, including the mucus and mucociliary movement, may hinder antigens and adjuvants absorption (14). Nasal mucus containing proteases and aminopeptidases may degrade the vaccine components (14). However, saponins-based adjuvant such as ASs or ISCOMs, which use liposome as a delivery system, may overcome these barriers and transport antigens and adjuvants to epithelial cells and APCs, which require further evaluation. Additionally, administering vaccines through the nasal cavity may result in the passage of vaccines into the lungs or oral cavity, making it difficult to determine the exact amount of antigens or adjuvants that reach the immune system. To address this issue, metered-dose nasal spray devices can be used to control the amount of solution delivered per spray, allowing for the evaluation of efficacy and side effects to determine the optimal spray dose. In conclusion, overcoming these obstacles is critical when developing saponins-based adjuvant for nasal vaccines.
Different adjuvants have unique immunomodulatory effects and the protective immune responses for different pathogens can vary. To achieve enhanced and broad protective immune responses for co-administered antigens, a combination of saponins with different mucosal adjuvants or delivery systems can be used, potentially resulting in additive enhancing effects on mucosal immune responses
AS01 is a successful example of combining two adjuvants, MPL and QS-21, in one formulation. Other attempt to combine different adjuvants includes intranasal administration of the ginseng stem-leaf saponins (GSLS) in combination with selenium (GSLS-Se). This combination enhanced the adjuvant effect on live vaccines for Newcastle disease virus and infectious bronchitis virus in chickens, promoting significantly higher antigen-specific antibody responses, increased lymphocyte proliferation and production of IFN-γ and IL-4 compared to GSLS alone. The increased antibody was able to neutralize corresponding viruses (128). Antigen-specific sIgA and the numbers of IgG+, IgA+, IgM+ plasma cells were significantly higher in GSLS-Se group than the control in the Harderian gland (129). Another example is the combination of cyclic guanosine monophosphate-adenosine monophosphate (cGAMP) and saponins, which improved protective response to influenza. Saponins can increase the permeability of cell membrane, allowing more cGAMP to enter the cells and increasing the utilization rate of saponins, leading to stronger immune effects (130, 131). Furthermore, nasal delivery systems such as microspheres or nanospheres made of chitosan, PLGA (poly[D,L-lactic-co-glycolic acid]), alginate, or cross-linked dextran have been employed to encapsulate saponins for nasal administration (132–135). These studies provide compelling evidence for the potential of developing mucosal adjuvants through combinations of saponins and other adjuvants.
ISCOMs and IMX complexes are also a promising option for the development of combined adjuvant carriers with other adjuvants to increase adjuvant activity. Combination of a mucosal adjuvant cholera toxin B (CTB) with ISCOMs has shown increased adjuvant effects. Intranasal immunization of synthetic peptide polymerized with the CTB in ISCOMs showed increased protection against polymerized synthetic peptide in ISCOMs (78). In addition, the cholera toxin subunit A1 (CTA1) fused to a dimer of the Ig-binding D-region of Staphylococcus aureus protein A (CTA1-DD) has been incorporated into ISCOMs to create CTA1-DD/ISCOMs adjuvant. This adjuvant significantly augments the immunogenicity of the antigen, as demonstrated by increased levels of specific serum antibodies, balanced Th1 and Th2 priming, and strong activation of DCs (136–138). Moreover, intranasal vaccination with CTA1-DD/ISCOMs adjuvanted antigens Ag85B-ESAT-6 from M. tuberculosis significantly reduced the M. tuberculosis burden in the lungs compared to control animals (139). However, combination of adjuvants does not always show enhanced effects. For instance, the combination of chitosan with IMX nanoparticles in a vaccine using the PR8 antigen induced a weaker immune response compared to IMX nanoparticles alone after intranasal administration (70).
Combining different routes of adjuvant delivery through prime-boost strategies has the potential to enhance both mucosal and systemic immune responses. For example, a systemic prime-intranasal boost strategy with an influenza vaccine adjuvanted with the liposomal dual TLR4/7 adjuvant has been shown to enhance both systemic and local/mucosal immunity. This regimen results in the secretion of antigen-specific sIgA and development of tissue-resident memory T cells in the respiratory tracts, as well as cross-reactive sIgA to multiple influenza virus strains (140). Similarly, this prime-boost strategy has been successful in preventing SARS-CoV-2 transmission and disease development through vaccination (141). Therefore, combining different routes of adjuvant delivery can provide comprehensive and effective immune responses at both the systemic and mucosal levels. Such strategies can also be applied to the study of saponin-based adjuvants in nasal vaccines. However, the optimal combination of administration routes may vary depending on the specific vaccine and pathogen being targeted, and thus requires thoroughly experimentation.
Achieving effective vaccine-induced immune responses requires a deeper understanding of the mode of action of saponin-based adjuvants, which can expedite the development of novel vaccine strategies. Collaborative research efforts between various disciplines, including chemistry, biochemistry, molecular biology, immunology, material science, and artificial intelligence, are crucial to achieve this goal. By using of multiomics technology such as transcriptomics, proteomics, and metabolomics at bulk and single-cell levels, researchers can uncover the function and mechanism of saponins-based adjuvants. Machine learning algorithms have also been applied to identify immune signatures associated with adjuvant formulations like AS01B, AS02A, AS03, CpG, and MF59 (142–144). Combining machine learning with in-depth profiling of vaccine-induced immune signatures including cytokine, cellular, and antibody responses can lead to identify adjuvant-specific immune response characteristics that can predict the efficacy and safety of the adjuvants in human (142–145). Moreover, immune response patterns in mice may not be predictive of responses in human (146). Therefore, organoids derived from lymphoid tissues, such as tonsils, will proved a powerful platform for studying key immune mechanisms related to human and enable rapid preclinical research on saponins-based adjuvants (146).
Saponin-based adjuvants have been demonstrated to have minimal side effects and are relatively non-toxic. Their administration via the nasal route has been shown to enhance the immune response, making them an appealing option for the development of nasal vaccines. This review aims to highlight the potential of saponins-based adjuvants for respiratory mucosal vaccines, offer further adjuvants candidates for the purpose of rational delivery system design, and ultimately drive the progress in the field of nasal vaccine development. We hope that this review will provide valuable insights and stimulate further research in this field.
YS conceived the ideas and finalized the review. KC drafted the manuscript. NW, XZ, MW, YL participated for collecting the materials. All authors contributed to the article and approved the submitted version.
This work was supported by National Natural Science Foundation of China (NSFC) under Grant No. 32270944; 1·3·5 project for disciplines of excellence, West China Hospital, Sichuan University under Grant No. ZYXY21004.
The authors declare that the research was conducted in the absence of any commercial or financial relationships that could be construed as a potential conflict of interest.
All claims expressed in this article are solely those of the authors and do not necessarily represent those of their affiliated organizations, or those of the publisher, the editors and the reviewers. Any product that may be evaluated in this article, or claim that may be made by its manufacturer, is not guaranteed or endorsed by the publisher.
1. Jose RJ. Respiratory infections: A global burden. Ann Res Hosp (2018) 2:12. doi: 10.21037/arh.2018.09.0
2. Kang L, Jing W, Liu J, Liu M. Trends of global and regional aetiologies, risk factors and mortality of lower respiratory infections from 1990 to 2019: An analysis for the global burden of disease study 2019. Respirology (2023) 28(2):166–75. doi: 10.1111/resp.14389
3. Jin X, Ren J, Li R, Gao Y, Zhang H, Li J, et al. Global burden of upper respiratory infections in 204 countries and territories, from 1990 to 2019. EClinicalMedicine (2021) 37:100986. doi: 10.1016/j.eclinm.2021.100986
4. Houston S. SARS-CoV-2 mucosal vaccine. Nat Immunol (2023) 24(1):1. doi: 10.1038/s41590-022-01405-w
5. Hasegawa H, van Reit E, Kida H. Mucosal immunization and adjuvants. Curr topics Microbiol Immunol (2015) 386:371–80. doi: 10.1007/82_2014_402
6. Lavelle EC, Ward RW. Mucosal vaccines — fortifying the frontiers. Nat Rev Immunol (2022) 22(4):236–50. doi: 10.1038/s41577-021-00583-2
7. Nakahashi-Ouchida R, Fujihashi K, Kurashima Y, Yuki Y, Kiyono H. Nasal vaccines: Solutions for respiratory infectious diseases. Trends Mol Med (2023) 29(2):124–40. doi: 10.1016/j.molmed.2022.10.009
8. Pabst R. Mucosal vaccination by the intranasal route. nose-associated lymphoid tissue (NALT)-structure, function and species differences. Vaccine (2015) 33(36):4406–13. doi: 10.1016/j.vaccine.2015.07.022
9. Bitter C, Suter-Zimmermann K, Surber C. Nasal drug delivery in humans. Curr Probl Dermatol (2011) 40:20–35. doi: 10.1159/000321044
10. Carter NJ, Curran MP. Live attenuated influenza vaccine (Flumist(R); fluenz): A review of its use in the prevention of seasonal influenza in children and adults. Drugs (2011) 71(12):1591–622. doi: 10.2165/11206860-000000000-00000
11. Waltz E. China And India approve nasal COVID vaccines - are they a game changer? Nature (2022) 609(7927):450. doi: 10.1038/d41586-022-02851-0
12. Sunagar R, Prasad SD, Ella R, Vadrevu KM. Preclinical evaluation of safety and immunogenicity of a primary series intranasal COVID-19 vaccine candidate (BBV154) and humoral immunogenicity evaluation of a heterologous prime-boost strategy with COVAXIN (BBV152). Front Immunol (2022) 13:1063679. doi: 10.3389/fimmu.2022.1063679
13. Zhu F, Zhuang C, Chu K, Zhang L, Zhao H, Huang S, et al. Safety and immunogenicity of a live-attenuated influenza virus vector-based intranasal SARS-CoV-2 vaccine in adults: Randomised, double-blind, placebo-controlled, phase 1 and 2 trials. Lancet Respir Med (2022) 10(8):749–60. doi: 10.1016/S2213-2600(22)00131-X
14. Nian X, Zhang J, Huang S, Duan K, Li X, Yang X. Development of nasal vaccines and the associated challenges. Pharmaceutics (2022) 14(10):1983. doi: 10.3390/pharmaceutics14101983
15. Correa VA, Portilho AI, De Gaspari E. Vaccines, adjuvants and key factors for mucosal immune response. Immunology (2022) 167(2):124–38. doi: 10.1111/imm.13526
16. Jia Y, Krishnan L, Omri A. Nasal and pulmonary vaccine delivery using particulate carriers. Expert Opin Drug Del (2015) 12(6):993–1008. doi: 10.1517/17425247.2015.1044435
17. Anggraeni R, Ana ID, Wihadmadyatami H. Development of mucosal vaccine delivery: An overview on the mucosal vaccines and their adjuvants. Clin Exp Vaccine Res (2022) 11(3):235–48. doi: 10.7774/cevr.2022.11.3.235
18. Sparg SG, Light ME, van Staden J. Biological activities and distribution of plant saponins. J Ethnopharmacol (2004) 94(2):219–43. doi: 10.1016/j.jep.2004.05.016
19. Marciani DJ. Elucidating the mechanisms of action of saponin-derived adjuvants. Trends Pharmacol Sci (2018) 39(6):573–85. doi: 10.1016/j.tips.2018.03.005
20. Lacaille-Dubois MA. Updated insights into the mechanism of action and clinical profile of the immunoadjuvant QS-21: A review. Phytomedicine (2019) 60:152905. doi: 10.1016/j.phymed.2019.152905
21. Nadeem AY, Shehzad A, Islam SU, Al-Suhaimi EA, Lee YS. Mosquirix rts, S/AS01 vaccine development, immunogenicity, and efficacy. Vaccines (2022) 10(5):713. doi: 10.3390/vaccines10050713
22. Hu KF, Lovgren-Bengtsson K, Morein B. Immunostimulating complexes (ISCOMS) for nasal vaccination. Adv Drug del Rev (2001) 51(1-3):149–59. doi: 10.1016/s0169-409x(01)00165-x
23. Kensil CR, Wu JY, Soltysik S. Structural and immunological characterization of the vaccine adjuvant QS-21. Pharm Biotechnol (1995) 6:525–41. doi: 10.1007/978-1-4615-1823-5_22
24. Kensil CR, Soltysik S, Wheeler DA, Wu JY. Structure/Function studies on QS-21, a unique immunological adjuvant from quillaja saponaria. Adv Exp Med Biol (1996). 404:165–72. doi: 10.1007/978-1-4899-1367-8_15
25. Francis G, Kerem Z, Makkar HP, Becker K. The biological action of saponins in animal systems: A review. Br J Nutr (2002) 88(6):587–605. doi: 10.1079/bjn2002725
26. Dalsgaard K. Saponin adjuvants. 3. isolation of a substance from quillaja saponaria Molina with adjuvant activity in food-and-Mouth disease vaccines. Archiv fur die gesamte Virusforschung (1974) 44(3):243–54. doi: 10.1007/BF01240612
27. Dalsgaard. A study of the isolation and characterization of the saponin quil a. evaluation of its adjuvant activity, with a special reference to the application in the vaccination of cattle against foot-and-Mouth disease. Acta veterinaria Scand Supplementum (1978) 69):7–40.
28. Kensil CR, Patel U, Lennick M, Marciani D. Separation and characterization of saponins with adjuvant activity from quillaja saponaria Molina cortex. J Immunol (1991) 146(2):431–7. doi: 10.4049/jimmunol.146.2.431
29. Garcon N, Van Mechelen M. Recent clinical experience with vaccines using MPL- and QS-21-Containing adjuvant systems. Expert Rev Vaccines (2011) 10(4):471–86. doi: 10.1586/erv.11.29
30. Kim YJ, Wang P, Navarro-Villalobos M, Rohde BD, Derryberry J, Gin DY. Synthetic studies of complex immunostimulants from quillaja saponaria: Synthesis of the potent clinical immunoadjuvant QS-21Aapi. J Am Chem Soc (2006) 128(36):11906–15. doi: 10.1021/ja062364i
31. Detienne S, Welsby I, Collignon C, Wouters S, Coccia M, Delhaye S, et al. Central role of CD169+ lymph node resident macrophages in the adjuvanticity of the QS-21 component of AS01. Sci Rep (2016) 6:39475. doi: 10.1038/srep39475
32. Soltysik S, Wu JY, Recchia J, Wheeler DA, Newman MJ, Coughlin RT, et al. Structure/Function studies of QS-21 adjuvant: Assessment of triterpene aldehyde and glucuronic acid roles in adjuvant function. Vaccine (1995) 13(15):1403–10. doi: 10.1016/0264-410x(95)00077-e
33. Newman MJ, Wu JY, Gardner BH, Munroe KJ, Leombruno D, Recchia J, et al. Saponin adjuvant induction of ovalbumin-specific CD8+ cytotoxic T lymphocyte responses. J Immunol (Baltimore Md 1950) (1992) 148(8):2357–62. doi: 10.4049/jimmunol.148.8.2357
34. Marciani DJ, Press JB, Reynolds RC, Pathak AK, Pathak V, Gundy LE, et al. Development of semisynthetic triterpenoid saponin derivatives with immune stimulating activity. Vaccine (2000) 18(27):3141–51. doi: 10.1016/s0264-410x(00)00118-3
35. Oda K, Matsuda H, Murakami T, Katayama S, Ohgitani T, Yoshikawa M. Adjuvant and haemolytic activities of 47 saponins derived from medicinal and food plants. Biol Chem (2000) 381(1):67–74. doi: 10.1515/BC.2000.009
36. Sun HX, Xie Y, Ye YP. Advances in saponin-based adjuvants. Vaccine (2009) 27(12):1787–96. doi: 10.1016/j.vaccine.2009.01.091
37. Sarntheingraf C, La MC. Association of saponins in water and water–gelatine mixtures. Thermochim Acta (2004) 418(1):79–84. doi: 10.1016/j.tca.2003.11.044
38. Marty-Roix R, Vladimer GI, Pouliot K, Weng D, Buglione-Corbett R, West K, et al. Identification of QS-21 as an inflammasome-activating molecular component of saponin adjuvants. J Biol Chem (2016) 291(3):1123–36. doi: 10.1074/jbc.M115.683011
39. Sun H, Yang Z, Ye Y. Structure and biological activity of protopanaxatriol-type saponins from the roots of panax notoginseng. Int Immunopharmacol (2006) 6(1):14–25. doi: 10.1016/j.intimp.2005.07.003
40. Zhai L, Li Y, Wang W, Wang Y, Hu S. Effect of oral administration of ginseng stem-and-Leaf saponins (GSLS) on the immune responses to Newcastle disease vaccine in chickens. Vaccine (2011) 29(31):5007–14. doi: 10.1016/j.vaccine.2011.04.097
41. Xie Y, Pan H, Sun H, Li D. A promising balanced Th1 and Th2 directing immunological adjuvant, saponins from the root of platycodon grandiflorum. Vaccine (2008) 26(31):3937–45. doi: 10.1016/j.vaccine.2008.01.061
42. Sun Y, Liu J, Yu H, Gong C. Isolation and evaluation of immunological adjuvant activities of saponins from the roots of pulsatilla chinensis with less adverse reactions. Int Immunopharmacol (2010) 10(5):584–90. doi: 10.1016/j.intimp.2010.02.006
43. Sun T, Yan X, Guo W, Zhao D. Evaluation of cytotoxicity and immune modulatory activities of soyasaponin Ab: An in vitro and in vivo study. Phytomedicine (2014) 21(13):1759–66. doi: 10.1016/j.phymed.2014.09.002
44. Nagai T, Suzuki Y, Kiyohara H, Susa E, Kato T, Nagamine T, et al. Onjisaponins, from the root of Polygala tenuifolia Willdenow, as effective adjuvants for nasal influenza and diphtheria-pertussis-tetanus vaccines. Vaccine (2001) 19(32):4824–34. doi: 10.1016/s0264-410x(01)00215-8
45. Zhang XF, Cui Y, Huang JJ, Zhang YZ, Nie Z, Wang LF, et al. Immuno-stimulating properties of diosgenyl saponins isolated from Paris polyphylla. Bioorg med Chem Lett (2007) 17(9):2408–13. doi: 10.1016/j.bmcl.2007.02.039
46. Shirahata T, Nagai T, Hirata N, Yokoyama M, Katsumi T, Konishi N, et al. Syntheses and mucosal adjuvant activity of simplified oleanolic acid saponins possessing cinnamoyl ester. Bioorg med Chem (2017) 25(6):1747–55. doi: 10.1016/j.bmc.2016.09.052
47. Bhatnagar N, Kim KH, Subbiah J, Park BR, Wang P, Gill HS, et al. Adjuvant effects of a new saponin analog VSA-1 on enhancing homologous and heterosubtypic protection by influenza virus vaccination. Vaccines (2022) 10(9):1383. doi: 10.3390/vaccines10091383
48. Kim H, Yu J, Bai D, Nahm MH, Wang P. Potentiating pneumococcal glycoconjugate vaccine PCV13 with saponin adjuvant VSA-1. Front Immunol (2022) 13:1079047. doi: 10.3389/fimmu.2022.1079047
49. Liu H, Patil HP, de Vries-Idema J, Wilschut J, Huckriede A. Enhancement of the immunogenicity and protective efficacy of a mucosal influenza subunit vaccine by the saponin adjuvant GPI-0100. PloS One (2012) 7(12):e52135. doi: 10.1371/journal.pone.0052135
50. Sasaki S, Sumino K, Hamajima K, Fukushima J, Ishii N, Kawamoto S, et al. Induction of systemic and mucosal immune responses to human immunodeficiency virus type 1 by a DNA vaccine formulated with QS-21 saponin adjuvant Via intramuscular and intranasal routes. J Virol (1998) 72(6):4931–9. doi: 10.1128/JVI.72.6.4931-4939.1998
51. Estrada A, Li B, Laarveld B. Adjuvant action of chenopodium quinoa saponins on the induction of antibody responses to intragastric and intranasal administered antigens in mice. Comp immunol Microbiol Infect Dis (1998) 21(3):225–36. doi: 10.1016/s0147-9571(97)00030-1
52. Moghadam Ariaee F, Tafaghodi M. Mucosal adjuvant potential of quillaja saponins and cross-linked dextran microspheres, Co-administered with liposomes encapsulated with tetanus toxoid. Iranian J Pharm Res IJPR (2012) 11(3):723–32.
53. Zulpo DL, Headley SA, Biazzono L, da Cunha IA, IgArashi M, de Barros LD, et al. Oocyst shedding in cats vaccinated by the nasal and rectal routes with crude rhoptry proteins of Toxoplasma gondii. Exp Parasitol (2012) 131(2):223–30. doi: 10.1016/j.exppara.2012.04.006
54. da Cunha IA, Zulpo DL, Bogado AL, de Barros LD, Taroda A, IgArashi M, et al. Humoral and cellular immune responses in pigs immunized intranasally with crude rhoptry proteins of Toxoplasma gondii plus Quil-A. Vet Parasitol (2012) 186(3-4):216–21. doi: 10.1016/j.vetpar.2011.11.034
55. Morein B, Sundquist B, Hoglund S, Dalsgaard K, Osterhaus A. ISCOM, a novel structure for antigenic presentation of membrane proteins from enveloped viruses. Nature (1984) 308(5958):457–60. doi: 10.1038/308457a0
56. Sun HX, Xie Y, Ye YP. ISCOMS and ISCOMATRIX . Vaccine (2009) 27(33):4388–401. doi: 10.1016/j.vaccine.2009.05.032
57. Pearse MJ, Drane D. ISCOMATRIX adjuvant for antigen delivery. Adv Drug del Rev (2005) 57(3):465–74. doi: 10.1016/j.addr.2004.09.006
58. Malliaros J, Quinn C, Arnold FH, Pearse MJ, Drane DP, Stewart TJ, et al. Association of antigens to ISCOMATRIX ™ adjuvant using metal chelation leads to improved ctl responses. Vaccine (2004) 22(29):3968–75. doi: 10.1016/j.vaccine.2004.03.054
59. Pearse MJ, Drane D. ISCOMATRIX adjuvant: A potent inducer of humoral and cellular immune responses. Vaccine (2002) 22(19):2391–5. doi: 10.1016/j.vaccine.2003.12.031
60. Morein B, Hu KF, Abusugra I. Current status and potential application of ISCOMS in veterinary medicine. Adv Drug del Rev (2004) 56(10):1367–82. doi: 10.1016/j.addr.2004.02.004
61. Lovgren K. The serum antibody response distributed in subclasses and isotypes after intranasal and subcutaneous immunization with influenza virus immunostimulating complexes. Scand J Immunol (1988) 27(2):241–5. doi: 10.1111/j.1365-3083.1988.tb02343.x
62. Jones PD, Tha Hla R, Morein B, Lovgren K, Ada GL. Cellular immune responses in the murine lung to local immunization with influenza A virus glycoproteins in micelles and immunostimulatory complexes (ISCOMS). Scand J Immunol (1988) 27(6):645–52. doi: 10.1111/j.1365-3083.1988.tb02397.x
63. Ugozzoli M, O'Hagan DT, Ott GS. Intranasal immunization of mice with herpes simplex virus type 2 recombinant gD2: The effect of adjuvants on mucosal and serum antibody responses. Immunology (1998) 93(4):563–71. doi: 10.1046/j.1365-2567.1998.00441.x
64. Hu KF, Elvander M, Merza M, Akerblom L, Brandenburg A, Morein B. The immunostimulating complex (ISCOM) is an efficient mucosal delivery system for respiratory syncytial virus (RSV) envelope antigens inducing high local and systemic antibody responses. Clin Exp Immunol (1998) 113(2):235–43. doi: 10.1046/j.1365-2249.1998.00650.x
65. Hu KF, Ekstrom J, Merza M, Lovgren-Bengtsson K, Morein B. Induction of antibody responses in the common mucosal immune system by respiratory syncytical virus immunostimulating complexes. Med Microbiol Immunol (1999) 187(4):191–8. doi: 10.1007/s004300050092
66. Pandey RS, Dixit VK. Evaluation of ISCOM vaccines for mucosal immunization against hepatitis B. J Drug Targeting (2010) 18(4):282–91. doi: 10.3109/10611860903450015
67. Cibulski SP, Mourglia-Ettlin G, Teixeira TF, Quirici L, Roehe PM, Ferreira F, et al. Novel ISCOMS from quillaja brasiliensis saponins induce mucosal and systemic antibody production, T-cell responses and improved antigen uptake. Vaccine (2016) 34(9):1162–71. doi: 10.1016/j.vaccine.2016.01.029
68. Kabiri M, Sankian M, Hosseinpour M, Tafaghodi M. The novel immunogenic chimeric peptide vaccine to elicit potent cellular and mucosal immune responses against HTLV-1. Int J pharmaceutics (2018) 549(1-2):404–14. doi: 10.1016/j.ijpharm.2018.07.069
69. Coulter A, Harris R, Davis R, Drane D, Cox J, Ryan D, et al. Intranasal vaccination with ISCOMATRIX adjuvanted influenza vaccine. Vaccine (2003) 21(9-10):946–9. doi: 10.1016/s0264-410x(02)00545-5
70. Mosafer J, Badiee A, Mohammadamini Z, Komeilinezhad A, Tafaghodi M. Immunization against PR8 influenza virus with chitosan-coated ISCOMATRIX nanoparticles. Artif cells nanomed Biotechnol (2018) 46(sup2):587–93. doi: 10.1080/21691401.2018.1464460
71. Wee JL, Scheerlinck JP, Snibson KJ, Edwards S, Pearse M, Quinn C, et al. Pulmonary delivery of ISCOMATRIX influenza vaccine induces both systemic and mucosal immunity with antigen dose sparing. Mucosal Immunol (2008) 1(6):489–96. doi: 10.1038/mi.2008.59
72. Vujanic A, Wee JL, Snibson KJ, Edwards S, Pearse M, Quinn C, et al. Combined mucosal and systemic immunity following pulmonary delivery of ISCOMATRIX adjuvanted recombinant antigens. Vaccine (2010) 28(14):2593–7. doi: 10.1016/j.vaccine.2010.01.018
73. Pabreja S, Garg T, Rath G, Goyal AK. Mucosal vaccination against tuberculosis using Ag85a-loaded immunostimulating complexes. Artif cells nanomed Biotechnol (2016) 44(2):532–9. doi: 10.3109/21691401.2014.966195
74. Lovgren K, Kaberg H, Morein B. An experimental influenza subunit vaccine (ISCOM): Induction of protective immunity to challenge infection in mice after intranasal or subcutaneous administration. Clin Exp Immunol (1990) 82(3):435–9. doi: 10.1111/j.1365-2249.1990.tb05467.x
75. Rivera-Patron M, Moreno M, Baz M, Roehe PM, Cibulski SP, Silveira F. ISCOM-like nanoparticles formulated with quillaja brasiliensis saponins are promising adjuvants for seasonal influenza vaccines. Vaccines (2021) 9(11):1350. doi: 10.3390/vaccines9111350
76. Garcia JL, Guimaraes Jda S Jr., Headley SA, Bogado AL, Bugni FM, Ramalho DC, et al. Eimeria tenella: Utilization of a nasal vaccine with sporozoite antigens incorporated into ISCOM as protection for broiler breeders against a homologous challenge. Exp Parasitol (2008) 120(2):185–90. doi: 10.1016/j.exppara.2008.07.007
77. Berezin VE, Bogoyavlenskyi AP, Khudiakova SS, Alexuk PG, Omirtaeva ES, Zaitceva IA, et al. Immunostimulatory complexes containing eimeria tenella antigens and low toxicity plant saponins induce antibody response and provide protection from challenge in broiler chickens. Vet Parasitol (2010) 167(1):28–35. doi: 10.1016/j.vetpar.2009.09.045
78. Solano-Parada J, Gonzalez-Gonzalez G, Torro LM, dos Santos MF, Espino AM, Burgos M, et al. Effectiveness of intranasal vaccination against Angiostrongylus costaricensis using a Serine/Threonine phosphatase 2 a synthetic peptide and recombinant antigens. Vaccine (2010) 28(32):5185–96. doi: 10.1016/j.vaccine.2010.05.072
79. Sanders MT, Deliyannis G, Pearse MJ, McNamara MK, Brown LE. Single dose intranasal immunization with ISCOMATRIX vaccines to elicit antibody-mediated clearance of influenza virus requires delivery to the lower respiratory tract. Vaccine (2009) 27(18):2475–82. doi: 10.1016/j.vaccine.2009.02.054
80. Skene CD, Doidge C, Sutton P. Evaluation of ISCOMATRIX™ and ISCOM™ vaccines for immunisation against helicobacter pylori. Vaccine (2008) 26(31):3880–4. doi: 10.1016/j.vaccine.2008.05.004
81. Garcon N, Chomez P, Van Mechelen M. Glaxosmithkline adjuvant systems in vaccines: Concepts, achievements and perspectives. Expert Rev Vaccines (2007) 6(5):723–39. doi: 10.1586/14760584.6.5.723
82. Stewart VA, McGrath SM, Walsh DS, Davis S, Hess AS, Ware LA, et al. Pre-clinical evaluation of new adjuvant formulations to improve the immunogenicity of the malaria vaccine RTS,S/AS02A. Vaccine (2006) 24(42-43):6483–92. doi: 10.1016/j.vaccine.2006.06.033
83. Garcon N, Silvano J, Kuper CF, Baudson N, Gerard C, Forster R, et al. Non-clinical safety evaluation of repeated intramuscular administration of the As15 immunostimulant combined with various antigens in rabbits and cynomolgus monkeys. J Appl Toxicol JAT (2016) 36(2):238–56. doi: 10.1002/jat.3167
84. Alving CR, Peachman KK, Matyas GR, Rao M, Beck Z. Army liposome formulation (ALF) family of vaccine adjuvants. Expert Rev Vaccines (2020) 19(3):279–92. doi: 10.1080/14760584.2020.1745636
85. Baldridge J, Myers K, Johnson D, Persing D, Hershberg R. Monophosphoryl lipid a and synthetic lipid a mimetics as TLR4-based adjuvants and immunomodulators. Vaccine Adjuvants. Infectious Disease. Humana Press (2006), 235–55. doi: 10.1007/978-1-59259-970-7_12
86. Casella CR, Mitchell TC. Putting endotoxin to work for us: Monophosphoryl lipid a as a safe and effective vaccine adjuvant. Cell Mol Life Sci (2008) 65(20):3231–40. doi: 10.1007/s00018-008-8228-6
87. Coccia M, Collignon C, Hervé C, Chalon A, Welsby I, Detienne S, et al. Cellular and molecular synergy in AS01-adjuvanted vaccines results in an early ifnγ response promoting vaccine immunogenicity. NPJ Vaccines (2017) 2:25. doi: 10.1038/s41541-017-0027-3
88. Didierlaurent AM, Laupeze B, Di Pasquale A, Hergli N, Collignon C, Garcon N. Adjuvant system AS01: Helping to overcome the challenges of modern vaccines. Expert Rev Vaccines (2017) 16(1):55–63. doi: 10.1080/14760584.2016.1213632
89. Spertini F, Audran R, Lurati F, Ofori-Anyinam O, Zysset F, Vandepapelière P, et al. The candidate tuberculosis vaccine Mtb72f/As02 in ppd positive adults: A randomized controlled phase I/Ii study. Tuberculosis (Edinburgh Scotland) (2013) 93(2):179–88. doi: 10.1016/j.tube.2012.10.011
90. Hutter JN, Robben PM, Lee C, Hamer M, Moon JE, Merino K, et al. First-in-Human assessment of safety and immunogenicity of low and high doses of plasmodium falciparum malaria protein 013 (FMP013) administered intramuscularly with ALFQ adjuvant in healthy malaria-naive adults. Vaccine (2022) 40(40):5781–90. doi: 10.1016/j.vaccine.2022.08.048
91. Wuertz KM, Barkei EK, Chen WH, Martinez EJ, Lakhal-Naouar I, Jagodzinski LL, et al. A SARS-CoV-2 spike ferritin nanoparticle vaccine protects hamsters against alpha and beta virus variant challenge. NPJ Vaccines (2021) 6(1):129. doi: 10.1038/s41541-021-00392-7
92. King HAD, Joyce MG, Lakhal-Naouar I, Ahmed A, Cincotta CM, Subra C, et al. Efficacy and breadth of adjuvanted SARS-CoV-2 receptor-binding domain nanoparticle vaccine in macaques. Proc Natl Acad Sci United States America (2021) 118(38):e2106433118. doi: 10.1073/pnas.2106433118
93. Ramakrishnan A, Schumack NM, Gariepy CL, Eggleston H, Nunez G, Espinoza N, et al. Enhanced immunogenicity and protective efficacy of a campylobacter jejuni conjugate vaccine coadministered with liposomes containing monophosphoryl lipid a and QS-21. mSphere (2019) 4(3):e00101-19. doi: 10.1128/mSphere.00101-19
94. Laupeze B, Herve C, Di Pasquale A, Tavares Da Silva F. Adjuvant systems for vaccines: 13 Years of post-licensure experience in diverse populations have progressed the way adjuvanted vaccine safety is investIgAted and understood. Vaccine (2019) 37(38):5670–80. doi: 10.1016/j.vaccine.2019.07.098
95. Duong VA, Nguyen TT, Maeng HJ. Recent advances in intranasal liposomes for drug, gene, and vaccine delivery. Pharmaceutics (2023) 15(1):207. doi: 10.3390/pharmaceutics15010207
96. Sia ZR, Chiem K, Huang WC, Seffouh A, Teimouri Dereshgi A, Hogan T, et al. Respiratory vaccination with hemagglutinin nanoliposomes protects mice from homologous and heterologous strains of influenza virus. J Virol (2022) 96(19):e0100622. doi: 10.1128/jvi.01006-22
97. Reimer JM, Karlsson KH, Lövgren-Bengtsson K, Magnusson SE, Fuentes A, Stertman L. Matrix-M™ adjuvant induces local recruitment, activation and maturation of central immune cells in absence of antigen. PloS One (2012) 7(7):e41451. doi: 10.1371/journal.pone.0041451
98. Lovgren Bengtsson K, Morein B, Osterhaus AD. ISCOM technology-based Matrix-M adjuvant: Success in future vaccines relies on formulation. Expert Rev Vaccines (2011) 10(4):401–3. doi: 10.1586/erv.11.25
99. Cox RJ, Pedersen G, Madhun AS, Svindland S, Saevik M, Breakwell L, et al. Evaluation of a virosomal H5N1 vaccine formulated with Matrix-M adjuvant in a phase I clinical trial. Vaccine (2011) 29(45):8049–59. doi: 10.1016/j.vaccine.2011.08.042
100. Mulamba C, Williams C, Kreppel K, Ouedraogo JB, Olotu AI. Evaluation of the Pfs25-IMX313/Matrix-M malaria transmission-blocking candidate vaccine in endemic settings. Malaria J (2022) 21(1):159. doi: 10.1186/s12936-022-04173-y
101. Bengtsson KL, Song H, Stertman L, Liu Y, Flyer DC, Massare MJ, et al. Matrix-M adjuvant enhances antibody, cellular and protective immune responses of a Zaire Ebola/Makona virus glycoprotein (Gp) nanoparticle vaccine in mice. Vaccine (2016) 34(16):1927–35. doi: 10.1016/j.vaccine.2016.02.033
102. Venkatraman N, Anagnostou N, Bliss C, Bowyer G, Wright D, Lovgren-Bengtsson K, et al. Safety and immunogenicity of heterologous prime-boost immunization with viral-vectored malaria vaccines adjuvanted with Matrix-M. Vaccine (2017) 35(45):6208–17. doi: 10.1016/j.vaccine.2017.09.028
103. Datoo MS, Natama MH, Some A, Traore O, Rouamba T, Bellamy D, et al. Efficacy of a low-dose candidate malaria vaccine, R21 in adjuvant Matrix-M, with seasonal administration to children in Burkina Faso: A randomised controlled trial. Lancet (2021) 397(10287):1809–18. doi: 10.1016/S0140-6736(21)00943-0
104. Heath PT, Galiza EP, Baxter DN, Boffito M, Browne D, Burns F, et al. Safety and efficacy of NVX-CoV2373 Covid-19 vaccine. New Engl J Med (2021) 385(13):1172–83. doi: 10.1056/NEJMoa2107659
105. Pedersen G, Major D, Roseby S, Wood J, Madhun AS, Cox RJ. Matrix-M adjuvanted virosomal H5N1 vaccine confers protection against lethal viral challenge in a murine model. Influenza other Respir viruses (2011) 5(6):426–37. doi: 10.1111/j.1750-2659.2011.00256.x
106. Kodama S, Hirano T, Noda K, Umemoto S, Suzuki M. Nasal immunization with plasmid DNA encoding P6 protein and immunostimulatory complexes elicits nontypeable haemophilus influenzae-specific long-term mucosal immune responses in the nasopharynx. Vaccine (2011) 29(10):1881–90. doi: 10.1016/j.vaccine.2010.12.129
107. Hellfritzsch M, Scherliess R. Mucosal vaccination Via the respiratory tract. Pharmaceutics (2019) 11(8):375. doi: 10.3390/pharmaceutics11080375
108. Fujimura Y. Evidence of M cells as portals of entry for antigens in the nasopharyngeal lymphoid tissue of humans. Virchows Archiv (2000) 436(6):560–6. doi: 10.1007/s004289900177
109. Sung SS, Fu SM, Rose CE Jr., Gaskin F, Ju ST, Beaty SR. A major lung CD103 (alphaE)-Beta7 integrin-positive epithelial dendritic cell population expressing langerin and tight junction proteins. J Immunol (2006) 176(4):2161–72. doi: 10.4049/jimmunol.176.4.2161
110. Blank F, Stumbles PA, Seydoux E, Holt PG, Fink A, Rothen-Rutishauser B, et al. Size-dependent uptake of particles by pulmonary antigen-presenting cell populations and trafficking to regional lymph nodes. Am J Respir Cell Mol Biol (2013) 49(1):67–77. doi: 10.1165/rcmb.2012-0387OC
111. Wang J, Li P, Yu Y, Fu Y, Jiang H, Lu M, et al. Pulmonary Surfactant-Biomimetic nanoparticles potentiate heterosubtypic influenza immunity. Science (2020) 367(6480):eaau0810. doi: 10.1126/science.aau0810
112. Marciani DJ. Is fucose the answer to the immunomodulatory paradox of quillaja saponins? Int Immunopharmacol (2015) 29(2):908–13. doi: 10.1016/j.intimp.2015.10.028
113. Nielsen CM, Ogbe A, Pedroza-Pacheco I, Doeleman SE, Chen Y, Silk SE, et al. Protein/AS01(B) vaccination elicits stronger, more Th2-skewed antigen-specific human T follicular helper cell responses than heterologous viral vectors. Cell Rep Med (2021) 2(3):100207. doi: 10.1016/j.xcrm.2021.100207
114. Dendouga N, Fochesato M, Lockman L, Mossman S, Giannini SL. Cell-mediated immune responses to a varicella-zoster virus glycoprotein e vaccine using both a TLR agonist and QS21 in mice. Vaccine (2012) 30(20):3126–35. doi: 10.1016/j.vaccine.2012.01.088
115. Leroux-Roels G, Van Belle P, Vandepapeliere P, Horsmans Y, Janssens M, Carletti I, et al. Vaccine adjuvant systems containing monophosphoryl lipid A and QS-21 induce strong humoral and cellular immune responses against hepatitis B surface antigen which persist for at least 4 years after vaccination. Vaccine (2015) 33(8):1084–91. doi: 10.1016/j.vaccine.2014.10.078
116. Kaetzel CS. The polymeric immunoglobulin receptor: Bridging innate and adaptive immune responses at mucosal surfaces. Immunol Rev (2005) 206(1):83–99. doi: 10.1111/j.0105-2896.2005.00278.x
117. Johansen FE, Kaetzel CS. Regulation of the polymeric immunoglobulin receptor and IgA transport: New advances in environmental factors that stimulate pIgR expression and its role in mucosal immunity. Mucosal Immunol (2011) 4(6):598–602. doi: 10.1038/mi.2011.37
118. Iborra S, Martínez-López M, Khouili Sofía C, Enamorado M, Cueto Francisco J, Conde-Garrosa R, et al. Optimal generation of tissue-resident but not circulating memory T cells during viral infection requires crosspriming by DNGR-1+ dendritic cells. Immunity (2016) 45(4):847–60. doi: 10.1016/j.immuni.2016.08.019
119. Wu HY, Russell MW. Nasal Lymphoid Tissue, Intranasal Immunization, and Compartmentalization of the Common Mucosal Immune System. Immunologic research (1997) 16(2):187–201. doi: 10.1007/BF02786362
120. Newman MJ, Wu JY, Gardner BH, Anderson CA, Kensil CR, Recchia J, et al. Induction of cross-reactive cytotoxic T-lymphocyte responses specific for HIV-1 gp120 using saponin adjuvant (QS-21) supplemented subunit vaccine formulations. Vaccine (1997) 15(9):1001–7. doi: 10.1016/s0264-410x(96)00293-9
121. Kashala O, Amador R, Valero MV, Moreno A, Barbosa A, Nickel B, et al. Safety, tolerability and immunogenicity of new formulations of the Plasmodium falciparum malaria peptide vaccine SPf66 combined with the immunological adjuvant QS-21. Vaccine (2002) 20(17-18):2263–77. doi: 10.1016/s0264-410x(02)00115-9
122. Cibulski S, Teixeira TF, Varela APM, de Lima MF, Casanova G, Nascimento YM, et al. IMXQB-80: A quillaja brasiliensis saponin-based nanoadjuvant enhances zika virus specific immune responses in mice. Vaccine (2021) 39(3):571–9. doi: 10.1016/j.vaccine.2020.12.004
123. Cawlfield A, Genito CJ, Beck Z, Bergmann-Leitner ES, Bitzer AA, Soto K, et al. Safety, toxicity and immunogenicity of a malaria vaccine based on the circumsporozoite protein (FMP013) with the adjuvant army liposome formulation containing QS21 (ALFQ). Vaccine (2019) 37(29):3793–803. doi: 10.1016/j.vaccine.2019.05.059
124. Baay M, Bollaerts K, Verstraeten T. A systematic review and meta-analysis on the safety of newly adjuvanted vaccines among older adults. Vaccine (2018) 36(29):4207–14. doi: 10.1016/j.vaccine.2018.06.004
125. BIgAeva E, Doorn E, Liu H, Hak E. Meta-analysis on randomized controlled trials of vaccines with QS-21 or ISCOMATRIX adjuvant: Safety and tolerability. PloS One (2016) 11(5):e0154757. doi: 10.1371/journal.pone.0154757
126. Mutsch M, Zhou W, Rhodes P, Bopp M, Chen RT, Linder T, et al. Use of the inactivated intranasal influenza vaccine and the risk of Bell's palsy in Switzerland. New Engl J Med (2004) 350(9):896–903. doi: 10.1056/NEJMoa030595
127. Lewis DJ, Huo Z, Barnett S, Kromann I, Giemza R, Galiza E, et al. Transient facial nerve paralysis (Bell's palsy) following intranasal delivery of a genetically detoxified mutant of Escherichia coli heat labile toxin. PloS One (2009) 4(9):e6999. doi: 10.1371/journal.pone.0006999
128. Ma X, Bi S, Wang Y, Chi X, Hu S. Combined adjuvant effect of ginseng stem-leaf saponins and selenium on immune responses to a live bivalent vaccine of Newcastle disease virus and infectious bronchitis virus in chickens. Poultry Sci (2019) 98(9):3548–56. doi: 10.3382/ps/pez207
129. Ma X, Chi X, Yuan L, Wang Y, Li Z, Xu W, et al. Immunomodulatory effect of ginseng stem-leaf saponins and selenium on harderian gland in immunization of chickens to Newcastle disease vaccine. Vet Immunol immunopathol (2020) 225:110061. doi: 10.1016/j.vetimm.2020.110061
130. Vassilieva EV, Li S, Korniychuk H, Taylor DM, Wang S, Prausnitz MR, et al. Cgamp/Saponin adjuvant combination improves protective response to influenza vaccination by microneedle patch in an aged mouse model. Front Immunol (2021) 11:583251. doi: 10.3389/fimmu.2020.583251
131. Vassilieva EV, Taylor DW, Compans RW. Combination of sting pathway agonist with saponin is an effective adjuvant in immunosenescent mice. Front Immunol (2019) 10:3006. doi: 10.3389/fimmu.2019.03006
132. Dehghan S, Kheiri MT, Abnous K, Eskandari M, Tafaghodi M. Preparation, characterization and immunological evaluation of alginate nanoparticles loaded with whole inactivated influenza virus: Dry powder formulation for nasal immunization in rabbits. Microb pathogen (2018) 115:74–85. doi: 10.1016/j.micpath.2017.12.011
133. Tafaghodi M, Eskandari M. The mucosal adjuvant potential of cross-linked dextran microspheres as dry powder. Iranian J basic Med Sci (2012) 15(3):873–9.
134. Dehghan S, Tafaghodi M, Bolourieh T, Mazaheri V, Torabi A, Abnous K, et al. Rabbit nasal immunization against influenza by dry-powder form of chitosan nanospheres encapsulated with influenza whole virus and adjuvants. Int J pharmaceutics (2014) 475(1-2):1–8. doi: 10.1016/j.ijpharm.2014.08.032
135. Dehghan S, Tavassoti Kheiri M, Tabatabaiean M, Darzi S, Tafaghodi M. Dry-powder form of chitosan nanospheres containing influenza virus and adjuvants for nasal immunization. Arch pharmacal Res (2013) 36(8):981–92. doi: 10.1007/s12272-013-0043-4
136. Helgeby A, Robson NC, Donachie AM, Beackock-Sharp H, Lövgren K, Schön K, et al. The combined CTA1-DD/ISCOM adjuvant vector promotes priming of mucosal and systemic immunity to incorporated antigens by specific targeting of B cells. J Immunol (2006) 176(6):3697–706. doi: 10.4049/jimmunol.176.6.3697
137. Lycke N. From toxin to adjuvant: The rational design of a vaccine adjuvant vector, CTA1-DD/ISCOM. Cell Microbiol (2004) 6(1):23–32. doi: 10.1046/j.1462-5822.2003.00338.x
138. Eliasson DG, Helgeby A, Schon K, Nygren C, El-Bakkouri K, Fiers W, et al. A novel non-toxic combined Cta1-dd and ISCOMS adjuvant vector for effective mucosal immunization against influenza virus. Vaccine (2011) 29(23):3951–61. doi: 10.1016/j.vaccine.2011.03.090
139. Andersen CS, Dietrich J, Agger EM, Lycke NY, Lovgren K, Andersen P. The combined CTA1-DD/ISCOMS vector is an effective intranasal adjuvant for boosting prior mycobacterium bovis BCG immunity to mycobacterium tuberculosis. Infect Immun (2007) 75(1):408–16. doi: 10.1128/IAI.01290-06
140. Sato-Kaneko F, Yao S, Lao FS, Sako Y, Jin J, Shukla NM, et al. A dual adjuvant system for intranasal boosting of local and systemic immunity for influenza vaccination. Vaccines (2022) 10(10):1694. doi: 10.3390/vaccines10101694
141. Christensen D, Polacek C, Sheward DJ, Hanke L, Moliner-Morro A, McInerney G, et al. Protection against SARS-CoV-2 transmission by a parenteral prime-intranasal boost vaccine strategy. EBioMedicine (2022) 84:104248. doi: 10.1016/j.ebiom.2022.104248
142. Chaudhury S, Duncan EH, Atre T, Storme CK, Beck K, Kaba SA, et al. Identification of immune signatures of novel adjuvant formulations using machine learning. Sci Rep (2018) 8(1):17508. doi: 10.1038/s41598-018-35452-x
143. Chaudhury S, Duncan EH, Atre T, Dutta S, Spring MD, Leitner WW, et al. Combining immunoprofiling with machine learning to assess the effects of adjuvant formulation on human vaccine-induced immunity. Hum Vaccines immunotherapeutics (2020) 16(2):400–11. doi: 10.1080/21645515.2019.1654807
144. Giarola-Silva S, Coelho-Dos-Reis JGA, Mourao MM, Campi-Azevedo AC, Nakagaki Silva EE, Luiza-Silva M, et al. Distinct patterns of cellular immune response elicited by influenza non-adjuvanted and AS03-adjuvanted monovalent H1n1(Pdm09) vaccine. Antiviral Res (2017) 144:70–82. doi: 10.1016/j.antiviral.2017.05.009
145. Hioki K, Hayashi T, Natsume-Kitatani Y, Kobiyama K, Temizoz B, Negishi H, et al. Machine learning-assisted screening of herbal medicine extracts as vaccine adjuvants. Front Immunol (2022) 13:847616. doi: 10.3389/fimmu.2022.847616
Keywords: saponins, adjuvant, mucosal adjuvant, ISCOMs, adjuvant systems
Citation: Chen K, Wang N, Zhang X, Wang M, Liu Y and Shi Y (2023) Potentials of saponins-based adjuvants for nasal vaccines. Front. Immunol. 14:1153042. doi: 10.3389/fimmu.2023.1153042
Received: 28 January 2023; Accepted: 07 March 2023;
Published: 20 March 2023.
Edited by:
Haibo Li, Army Medical University, ChinaReviewed by:
Viviane Maimoni Goncalves, Butantan Institute, BrazilCopyright © 2023 Chen, Wang, Zhang, Wang, Liu and Shi. This is an open-access article distributed under the terms of the Creative Commons Attribution License (CC BY). The use, distribution or reproduction in other forums is permitted, provided the original author(s) and the copyright owner(s) are credited and that the original publication in this journal is cited, in accordance with accepted academic practice. No use, distribution or reproduction is permitted which does not comply with these terms.
*Correspondence: Yun Shi, c2hpeXVuQHdjaHNjdS5jbg==
Disclaimer: All claims expressed in this article are solely those of the authors and do not necessarily represent those of their affiliated organizations, or those of the publisher, the editors and the reviewers. Any product that may be evaluated in this article or claim that may be made by its manufacturer is not guaranteed or endorsed by the publisher.
Research integrity at Frontiers
Learn more about the work of our research integrity team to safeguard the quality of each article we publish.