- Departamento de Microbiología Molecular, Instituto de Biotecnología, Universidad Nacional Autónoma de México, Cuernavaca, Morelos, Mexico
Bacillus thuringiensis (Bt) produces different insecticidal proteins effective for pest control. Among them, Cry insecticidal proteins have been used in transgenic plants for the control of insect pests. However, evolution of resistance by insects endangers this technology. Previous work showed that the lepidopteran insect Plutella xylostella PxHsp90 chaperone enhanced the toxicity of Bt Cry1A protoxins by protecting them from degradation by the larval gut proteases and by enhancing binding of the protoxin to its receptors present in larval midgut cells. In this work, we show that PxHsp70 chaperone also protects Cry1Ab protoxin from gut proteases degradation, enhancing Cry1Ab toxicity. We also show that both PxHsp70 and PxHsp90 chaperones act cooperatively, increasing toxicity and the binding of Cry1Ab439D mutant, affected in binding to midgut receptors, to cadherin receptor. Also, insect chaperones recovered toxicity of Cry1Ac protein to a Cry1Ac-highly resistant P. xylostella population, NO-QAGE, that has a disruptive mutation in an ABCC2 transporter linked to Cry1Ac resistance. These data show that Bt hijacked an important cellular function for enhancing its infection capability, making use of insect cellular chaperones for enhancing Cry toxicity and for lowering the evolution of insect resistance to these toxins.
1 Introduction
Bacillus thuringiensis (Bt) produce diverse insecticidal proteins that have been used in the control of insect pests in agriculture (1, 2). The most successful application of Bt insecticidal proteins has been their expression in transgenic crops, showing efficient pest control with an important reduction on the use of chemical insecticides (3). Among the different insecticidal proteins produced by Bt, the Cry proteins are the most commercially used. These proteins bind to different membrane gut proteins known as Cry-receptors such as aminopeptidase N, alkaline phosphatase, cadherin or ABC transporters (4). However, the successful application of Cry toxins in insect pest control, has been challenged by the evolution of resistance to Cry toxin action in different insect pests, where high levels of resistance have been shown to be linked to mutations affecting the expression of Cry receptors (5, 6). Besides the resistance problem, there are some important crop pests that show low susceptibility to the Cry toxins that are currently used in transgenic plants (7), thus it is important to improve Cry toxin action against these particular insect pests.
Cry proteins are synthesized as protoxins and show specific toxicity to the insect larval stages. The Cry1 protoxins are composed of seven structural domains that are activated by the larval gut proteases leading to the formation of the three-domain toxin core. Domain I is involved in toxin oligomerization and pore formation, while domains II and III are involved in the insect specificity by their specific binding to different membrane receptors inducing toxin oligomerization, insertion into the cell membrane and pore formation, causing larval gut cells-burst by osmotic shock (4, 8). Recent data have shown that Cry protoxins also bind to some Cry-receptors before being activated by gut proteases, inducing a robust pore formation activity in the midgut cells (9, 10). It was reported that the oligomers formed from protoxin or from activated toxin differ in their sensitivity to heat, membrane insertion capabilities and their pore characteristics (9). Therefore, it has been proposed that Cry toxins have a dual mode of action, one for the protoxin and another for the activated toxin (10, 11). Several insects that have evolved resistance to the activated Cry1Ac toxin, were still susceptible to the protoxin, supporting a dual mode of action (11).
Proteomic analysis indicated that, besides binding of Cry proteins to larval membrane gut proteins, Cry toxins can also bind to some intracellular proteins such as actin, V-ATP synthase beta subunit and the Hsp70 chaperone among others (12, 13). Since Cry proteins exert their toxicity in the plasma membrane by forming lytic pores, the potential role of all these intracellular proteins in the mechanism of action of Cry proteins has not been studied in detail. Recently, it was shown that Plutella xylostella Hsp90 chaperone enhanced the toxicity of Cry1Ab and Cry1Ac toxins against different lepidopteran pests (14). The enhancing effect of Hsp90 on Cry1A toxicity was shown to be due to the protection of the protoxin degradation by gut proteases and also by improving protoxin binding to the cadherin receptor (14). Interestingly, P. xylostella Hsp70 also enhanced Cry1Ac toxicity, in contrast to a bacterium GroEL chaperone that showed marginal effect (14). It was shown that insect Hsp70 or Hsp90 chaperones resist degradation by the larval gut proteases, in contrast to GroEL that is highly sensitive to degradation, explaining the efficacy of Hsp70 or Hsp90 in enhancing Cry toxicity when fed to the larvae (14).
Hsp70 is recognized as an Hsp90 co-chaperone. Hsp70 assist unfolded intermediates by recognizing stretches of hydrophobic residues, and re folding these intermediates to their native state, while Hsp90 assist later stages in the folding or activation of these proteins (15, 16). Here we show that besides the independent enhancement of Cry1Ab toxicity performed by both chaperones, the PxHsp70 and PxHsp90 chaperones act together in assisting Cry1A protoxins for receptor binding. In addition, both PxHsp70 and PxHsp90 act cooperatively assisting Cry1Ac toxicity and countering the insect resistance to Cry proteins that is linked to mutations affecting ABCC2 receptor. These results suggest that the use of insect chaperones along with Cry toxins could be useful for the control of insect pests that show low susceptibility to these toxins and also to counteract the Cry toxin resistance that has evolved in different insect pests.
2 Materials and methods
2.1 Expression of Cry1Ab, Cry1AbR99E, Cry1AbE129K, Cry1AbG439D and Cry1Ac
To produce Cry1A protoxins, the acrystalliferous Bt407 cry- strain transformed with the corresponding plasmids (pHT315-Cry1Ab, pHT315-Cry1AbR99E, pHT315-Cry1AbE129K, pHT315-Cry1AbG439D) or the HD73 strain were grown in sporulation medium plates until complete sporulation (17). For the growth of Cry1Ab or Cry1Ab mutants this medium was supplemented with erythromycin 10 μg/ml. The spore/crystals were harvested and washed three times with distilled water and finally suspended in solubilization buffer (50 mM Na2CO3 pH 10.5, 0.02% β-mercaptoethanol) and incubated for 1 h at 37°C. The soluble protoxins were separated from non-soluble spores and debris by centrifugation, the protein concentration of the soluble material was determined by Bradford method using BSA as standard protein and the quality of soluble protoxin samples was analyzed in 12% acrylamide SDS-PAGE electrophoresis.
2.2 Production of PxHsp70, PxHsp90 and cadherin fragment CR12
For the production of PxHsp70 or PxHsp90 chaperones, the genes of these proteins were cloned in pET28b expression vector and their production was done in E. coli BL21cells as reported (14). Briefly, 2.5 ml of overnight cultures of BL21-PxHsp90 and BL21-PxHsp70 strains in 2x-TY medium supplemented with 50 μg/ml kanamycin were used to inoculate 250 ml of the same medium and incubated at 37°C with 200 rpm until an OD600 of 0.6 was reached. After this, the expression of PxHsp90 or PxHsp70 was induced by adding 0.5 mM isopropyl β-D-1-thiogalactopyranoside (IPTG) to the cultures and incubating overnight at 30°C with 150 rpm shaking. Cells were harvested by centrifugation, frozen and suspended in PBS containing 8 M Urea. Then the samples were lyzed by sonication four times for 50 sec at 4°C and centrifuged 40 min at 48,000 rpm. The supernatant was loaded into Ni-NTA agarose column, washed with 10 volumes of 35 mM imidazole in PBS and eluted with 250 mM imidazole in PBS supplemented with 1 mM ATP and 1 mM MgCl2. The chaperone samples were finally dialyzed by using Amicon Ultra 30K filters into PBS buffer supplemented with 1 mM ATP, 1 mM MgCl2. These chaperone samples were used immediately or stored at 4°C for using them the next day.
Cloning of the cadherin fragment CR12 into pET22 vector was previously reported (18). The pET22-CR12 plasmid was transformed into E. coli ER2566 strain (Invitrogen, Carlsbad CA) and cells were grown at 37°C in TY medium supplemented with 100 mg/ml ampicillin and 0.1% glucose until they reached an OD of 0.7 at 600 nm. The expression of CR12 fragment was induced with 1 mM IPTG and grown for additional 4 h at 25°C. Cells were harvested by centrifugation, frozen and suspended in PBS containing 8 M Urea. The samples were lysed by sonication four times, for 50 sec at 4°C and centrifuged 40 min at 48,000 rpm. The CR12 peptide protein was purified through a nickel affinity column and eluted with 250 mM imidazole in PBS as described above for purification of the chaperone proteins.
2.3 Analysis of Cry1Ab protoxin stability to proteolysis in the presence of PxHsp70
For the analysis of the effect of PxHsp70 on the stability of Cry1Ab protoxin to protease treatment, 150 μg of PxHsp70 or BSA were incubated with 2.5 μg Cry1Ab protoxin in PBS buffer supplemented with 1 mM ATP and 1mM MgCl2 in a final volume of 50 μl. Trypsin, (60 ng in 2 μl) was added and incubated at 37°C with agitation at 850 rpm. Ten μl samples were taken after different incubation times (3, 5, 10, 20 or 60 min) mixed with 3 μl of 4X-Laemmli buffer and boiled 3 min. Time 0 corresponds to the time that takes to add trypsin to the mixture, remove a 10 μl sample and boil it for 3 min Laemmli buffer to stop the reaction. All other time samples were removed at the indicated times and treated similarly to time 0 sample. These samples were loaded in SDS/PAGE 10% acrylamide gel and transferred into PVDF membrane (Millipore) for western blot detection.
In the case of the treatment with midgut juice from P. xylostella, the midgut juice was prepared from midgut tissues dissected from 4th instar larvae as previously described (14). Briefly, twenty dissected midguts were paced in an Eppendorf tube and centrifuged at 2200 xg for one min. The supernatant was collected and protein concentration was determined by Bradford assay using BSA as standard. A total of 150 μg PxHsp70 or BSA were incubated with 2.5 μg Cry1Ab protoxin in PBS buffer supplemented with 1 mM ATP and 1 mM MgCl2 in a final volume of 50 μl. Fifty ng of midgut juice protein in 2 μl, were added and incubated at 37°C with 850 rpm agitation. Ten μl samples were taken after different incubation times (30, 60, 90, or 120 min) mixed with 3 μl of 4X-Laemmli buffer and boiled for 3 min. Time 0 corresponds to the time that takes to add the midgut juice protein to the mixture, remove a 10 μl sample and stop the reaction by boiling 3 min in the Laemmli buffer. Samples were loaded in SDS/PAGE 10% acrylamide gel and transferred into PVDF membrane (Millipore) for western blot detection. The PVDF membranes were blocked with 3% non-fat dried skimmed milk in PBS. Then, membranes were incubated 1 h at room temperature with anti-Cry1Ab antibody (1:20,000 dilution) in PBS. After washing three times for 15 min with washing buffer (0.05% tween in PBS) the membrane was incubated with a HRP conjugated anti-IgG rabbit antibody (Sigma) (1:30,000 dilution) for 1 h at room temperature and washed three times with washing buffer. SuperSignal™ chemiluminescence (Pierce) was used for signal detection according to manufacturer´s instructions.
2.4 Binding of PxHsp70 to Cry1Ab protoxins
Binding of Hsp70 to Cry1Ab, Cry1AbR99E, Cry1AbE129K or Cry1AbG439D was analyzed by ELISA binding assays. ELISA 96 well plates were coated with 0.5 μg of the different Cry proteins suspended in 50 μl of PBS, by overnight incubation at 4°C. Unbound protoxins were washed away with PBS buffer and the plate was blocked with 2% Slim milk in PBS for 2 h at 37°C. The blocking buffer was removed and 50 μl of Hsp70 solution (250 or 500 nM) were added to the wells and incubated 1 h at 37°C. The ELISA plates were washed three times with PBS buffer and then incubated with 50 μl anti-polyHistidine-Peroxidase conjugated antibody (1:5,000 dilution) (Sigma-Aldrich) for 1 h a 30°C. Finally, 50 μl of 1 mg/ml o-phenylenediamine in substrate buffer (100 mM K3PO4, pH 5) supplemented with 2 μl of hydrogen peroxide was added to each well and the reaction was stopped by adding 25 μl of 6 N HCl. The plates were read on microtiter plate reader at OD 490 nm. Data represent means of triplicates and each experiment was repeated twice. The p value was calculated by using a student’s t -test for two groups (https://www.graphpad.com/quickcalcs/ttest1/), p values < 0.05 were considered as statistically significant differences.
2.5 Binding analysis of Cry1Ab protoxins to cadherin fragment CR12 in the presence of PxHsp70 and PxHsp90
ELISA plates were coated with 0.5 µg of M. sexta cadherin fragment (CR12) in 100 µl of PBS per well over night at 4°C. Plates were washed three times with PBS and then blocked with 200 µl/well of PBS-M (PBS, 2% skim milk) for 2 h at 37°C. After blocking, the plates were washed three times with PBS and incubate 1 h at 37°C with different concentrations of Cry1Ab protoxins (25, 50 or 100 nM) in the presence or absence of 30-fold higher PxHsp70 concentration, previously incubated with the protoxin during 10 min at 30°C in a total 100 µl volume of PBST (PBS + 0.1% Tween 20). Wells were then washed three times with PBS. The bound proteins were finally detected by using anti-Cry1Ab polyclonal antibody (1: 20,000 dilution) for 1 h at 37°C in 100 µl PBST buffer. Blots were washed three times with PBST and three times with PBS, and then incubated with 100 µl of PBST containing anti-rabbit HRP conjugated antibody (1: 20,000 dilution) (Santa Cruz Biotechnology) for 1 h at 37°C. Finally, the reaction was developed with HRP substrates o-phenylenediamine and hydrogen peroxide as described above. Each experiment was performed in duplicate with three repetitions. The statistical calculations (mean and standard deviation) and graphics were performed using the Microsoft Excel Program. The p value was calculated by using a student’s t -test for two groups (https://www.graphpad.com/quickcalcs/ttest1/), p values < 0.05 were considered as statistically significant differences.
2.6 Analysis of Cry1Ab or Cry1Ac toxicity in the presence of PxHsp70 and PxHsp90
Bioassays were performed using 24 wells plates containing one larvae per well. Third instar larvae of P. xylostella were used. Larval diet was surface contaminated with different concentrations of Cry1Ab or Cry1Ab mutants soluble protoxins as stated in each experiment plus different concentrations of chaperone. Controls with the same protoxins concentration without chaperone addition were included. For the bioassays against P. xylostella NO-QAGE strain, the larval diet was surface contaminated with 25 ng/cm2 of Cry1Ac soluble protoxin plus different concentrations of chaperone, and the controls were incubated with Cry1Ac protoxin alone without chaperone. The soluble protoxin was previously incubated with the mixture of chaperones for 30 min at 37°C in 1mM MgCl2, 1mM ATP, in PBS buffer before contamination of the larval diet. Mortality was assessed after 7 days. Each experiment was performed in triplicate (72 larvae per treatment). As negative controls, the diet was surface contaminated with the highest chaperone concentration or water. Data are shown in mean ± SD. The p value was calculated by using a student’s t -test for two groups (https://www.graphpad.com/quickcalcs/ttest1/), p values < 0.05 were considered as statistically significant differences.
3 Results
3.1 PxHsp70 protects Cry1Ab protoxin from midgut proteases
Previously we showed that PxHsp70 enhanced Cry1Ac toxicity against Plutella xylostella in a dose dependent way (14). In addition, Cry1Ab protoxin was shown to be protected by PxHsp90 from degradation by P. xylostella midgut proteases which correlated with the enhancing effect of PxHsp90 on Cry1Ab toxicity (14). To determine if PxHsp70 also has a similar protection effect of Cry1Ab protoxin against midgut proteases, we analyzed the stability of Cry1Ab protoxin after trypsin treatment, which is routinely used for in vitro activation of Cry toxins, or after treatment with P. xylostella midgut juice in the presence or absence of PxHsp70. In the control without PxHsp70, BSA was added at the same concentration as PxHsp70 in order to discard a non-specific protein protecting effect against protease treatment. Figure 1A shows that in the presence of PxHsp70, Cry1Ab protoxin was protected from trypsin degradation since the 130 kDa protoxin band was still observed after 10 min of trypsin treatment in contrast to the control samples without PxHsp70, where the protoxin band was not observed at all incubation times, indicating a fast degradation under these conditions. A similar result was obtained when Cry1Ab protoxin was incubated with P. xylostella midgut juice since the 130 kDa band was still observed after 90 min incubation with midgut juice in the presence of PxHsp70 in contrast with the control samples where Cry1Ab 130 kDa protoxin band was not observed, suggesting immediate degradation by the midgut juice proteases (Figure 1B).
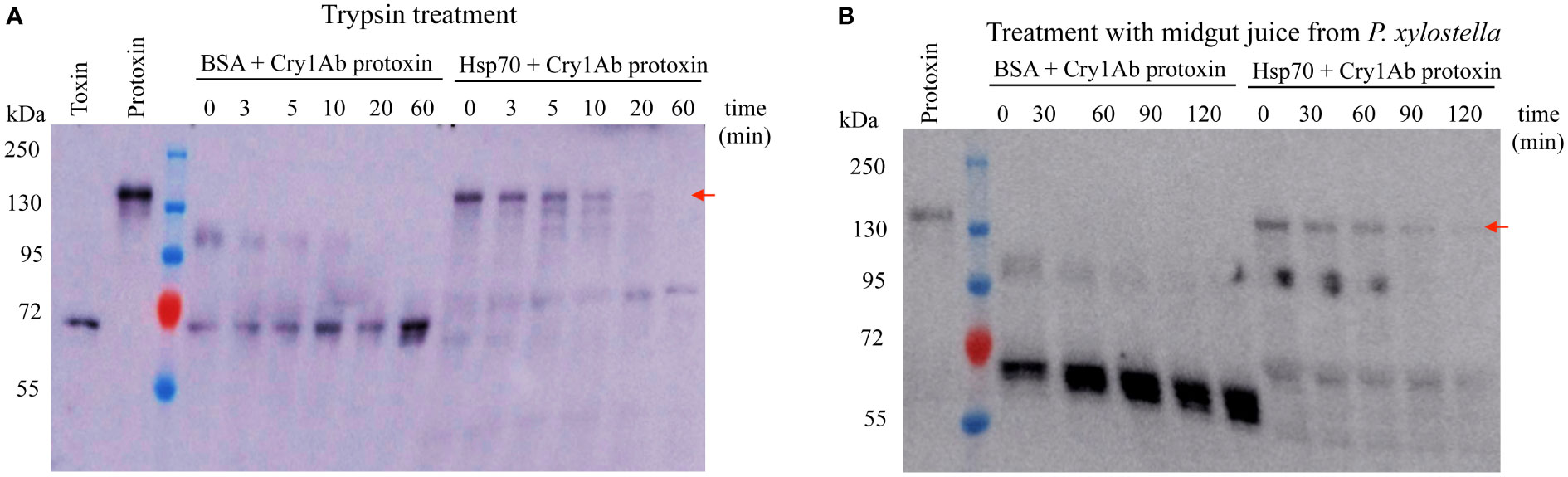
Figure 1 PxHsp70 protects Cry1Ab protoxin from protease degradation. (A) Trypsin treatment (1:40 w:w) of one μg Cry1Ab protoxin for different time points in the presence of either BSA or PxHsp70 and revealed by western blot using anti-Cry1Ab antibody. (B) Treatment of Cry1Ab protoxin with midgut juice from P. xylostella as described in methods. One μg Cry1Ab protoxin was treated with the midgut proteases for different time points in the presence of either BSA or PxHsp70 and revealed by western blot using anti-Cry1Ab antibody. Representative results of three replicas are shown. The arrows indicate the position of the 130 kDa Cry1Ab protoxin.
3.2 PxHsp70 and PxHsp90 cooperatively assist Cry1Ab in receptor binding
An interesting feature of Hsp90 chaperone is its capacity to suppress mutations of its client proteins (19, 20). Previously, we showed that PxHsp90 preferentially recovered the toxicity of Cry1AbG439D mutant affected in receptor binding (21) in contrast to the toxicity of Cry1AbR99E or Cry1AbE129K mutants affected on oligomerization or oligomer membrane insertion respectively (22, 23), indicating that Hsp90 assist Cry1Ab binding to midgut receptors (14). To determine what step of the mode of action of Cry1Ab protein is assisted by PxHsp70, the toxicity of the three non-toxic Cry1Ab mutants described above was analyzed in the presence of PxHsp70 against P. xylostella larvae. First, Figure 2A shows that PxHsp70 (50 ng/cm2, 200 ng/cm2 or 500 ng/cm2) enhanced the toxicity of Cry1Ab (2.5 ng/cm2) from 3% mortality to 100% mortality (p> 0.0001) as was previously reported (14). Toxicity assays of 25 ng/cm2 from each Cry1Ab mutant performed in the presence of 200 ng/cm2 or 500 ng/cm2 of PxHsp70 showed that Cry1AbG439D recover substantial toxicity (from 10% up to 54% mortality in the presence of 500 ng/cm2 of PxHsp70; p<0.0024) (Figure 2B) in contrast of the Cry1AbR99E (from 5% mortality to 30% mortality in the presence of 500 ng/cm2 of PxHsp70; p<0.0016) or Cry1AbE129K (2% mortality up to 22% mortality with 500 ng/cm2 PxHsp70; p<0.0006) mutants that showed a subtle increase in toxicity in the same experimental conditions (Figure 2B), suggesting that PxHsp70 assists Cry1Ab binding to midgut receptors.
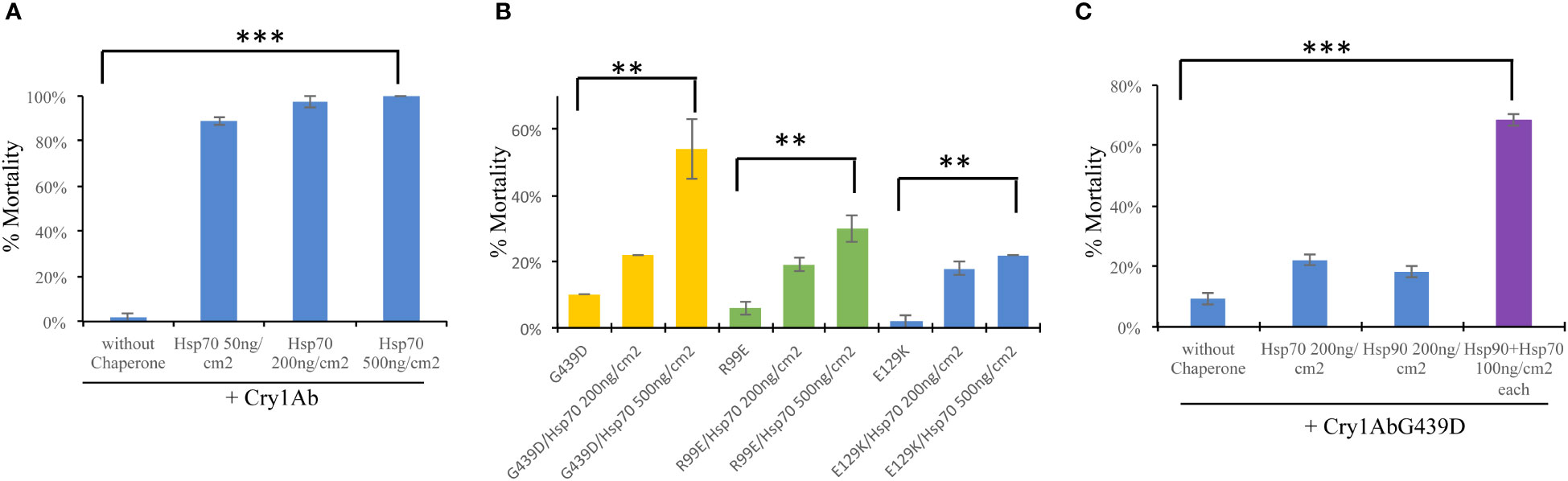
Figure 2 PxHsp70 and PxHsp90 recovers toxicity of Cry1AbG439D against Plutella xylostella. (A) Effect of PxHsp70 on Cry1Ab toxicity. Percentage of mortality (%) of P. xylostella larvae treated with 2.5 ng/cm2 of Cry1Ab in the presence of 50, 200 or 500 ng/cm2 of PxHsp70 chaperon (***p> 0.0001). (B) Effect of PxHsp70 on the toxicity of non-toxic Cry1Ab mutants affected in different steps of the mode of action. Percentage of mortality (%) of P. xylostella larvae treated with 25 ng/cm2 of Cry1AbG439D (affected in receptor binding), Cry1AbR99E (affected in oligomerization) or Cry1AbE129K (affected in oligomer membrane insertion) assayedin the presence of different concentrations of PxHsp70 (200 and 500 ng/cm2). P values indicated statistically significant differences: **p> 0.0024 with Cry1AbG439D; **p> 0.0016 with Cry1AbR99E, and **p> 0.0006 with Cry1AbE129K mutant. (C) Effect of both chaperones, PxHsp70 and PxHsp90, on the toxicity of Cry1AbG439D mutant (affected in receptor binding). Percentage of mortality (%) of P. xylostella larvae treated with 25 ng/cm2 of Cry1AbG439D, mutant toxin in the presence of different concentrations of PxHsp70 (200 ng/cm2), PxHsp90 (200 ng/cm2) or a mixture of both chaperones (100 ng/cm2 of each chaperone) (P values indicated statistically significant differences: ***p<0.0001). Data with standard deviations represent means of three treatments using 24 larvae per treatment in each repetition.
To further analyze the effect of PxHsp70 on Cry1AbG439D protoxin, the binding of Cry1AbG439D protoxin to a M. sexta cadherin fragment CR12 was analyzed in the presence or absence of PxHsp70 by ELISA binding assays. This CR12 fragment contains a Cry1Ab binding site (18). Figure 3A shows that PxHsp70 bound similarly to Cry1Ab, Cry1AbR99E, Cry1AbE129K or Cry1AbG439D (p> 0.0001). Figure 3B shows that Cry1AbG439D (100 nM) did not recover substantial binding to CR12 in the presence of PxHsp70 (750 nM) or PxHsp90 (750 nM) under these experimental conditions (Figure 3B). However, in the presence of both chaperones, PxHsp70 and PxHsp90 (375 nM of each chaperone), the Cry1AbG439D mutant recover significant binding to CR12, higher than that observed with PxHsp70 or PxHsp90 alone (p<0.0002) (Figure 3B), suggesting that these two chaperones act cooperatively in assisting the binding of Cry1Ab to the CR12 receptor. Since both PxHsp70 and PxHsp90 act cooperatively in enhancing binding of Cry1AbG439D protoxin to the CR12 cadherin fragment, we analyzed the effect of both chaperons in the toxicity of Cry1AbG439D to P. xylostella larvae. Figure 2C shows that in the presence of both chaperons (100 ng/cm2 of each chaperone) Cry1AbG439D recover higher toxicity (from 9% mortality to 68% mortality; p<0.0001) compared with the effect observed with PxHsp70 (from 9% mortality to 22% mortality with 200 ng/cm2; p<0.0005) or PxHsp90 (from 9% mortality to 18% mortality with 200 ng/cm2; p<0.0001). These results show that both Hsp70 and Hsp90 act cooperatively to recover binding of Cry1AbG439D mutant to CR12 and to increase toxicity of this Cry1AbG439D mutant against P. xylostella larvae.
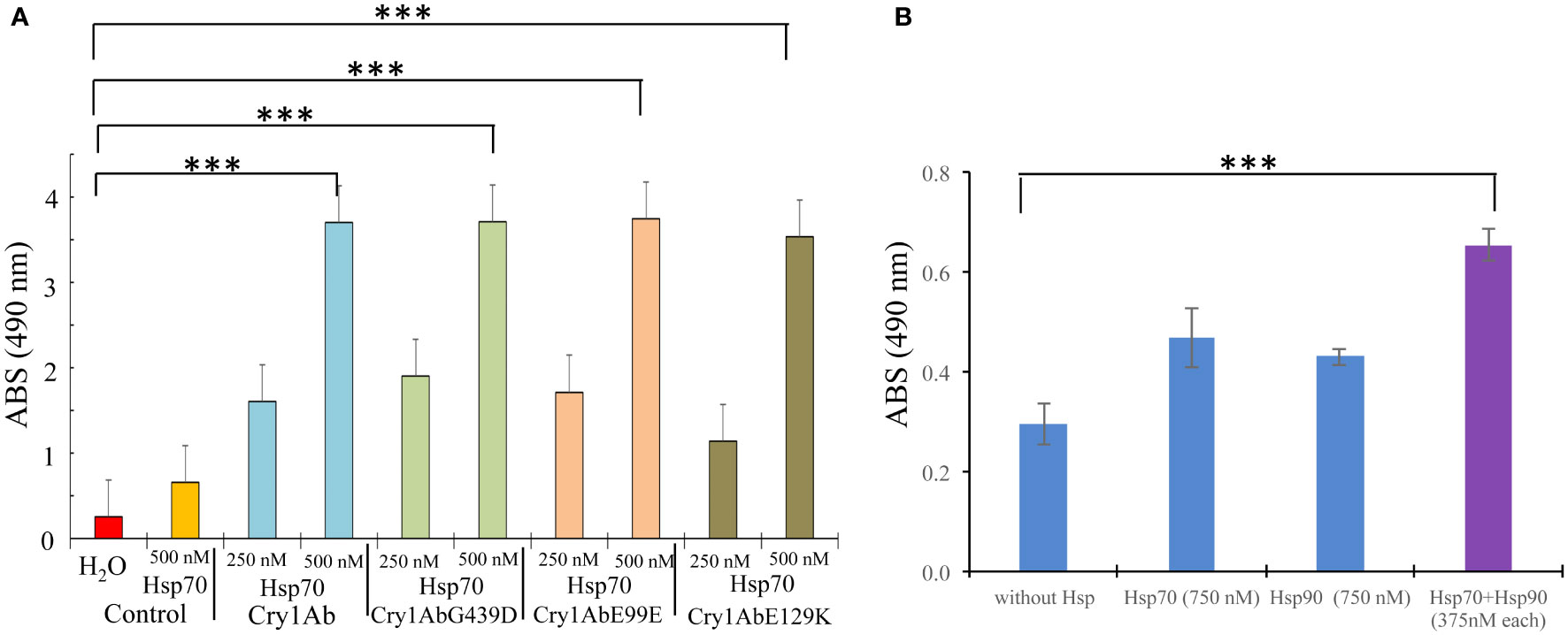
Figure 3 PxHsp70 and PxHsp90 assist Cry1Ab protoxin in binding to CAD receptor fragment. (A) ELISA binding assays of Cry1Ab, Cry1AbG439D, Cry1AbR99E or Cry1AbE129K protoxins to PxHsp70. ELISA plates were coated with 0.5 μg of each protoxin and after washing unbound proteins, the plates were incubated with different concentrations of PxHsp70, and revealed with anti-His antibody as described in methods. P values indicated statistically significant differences: ***p> 0.0001. (B) ELISA binding assays of Cry1AbG439D protoxin (100 nM) and the mixtures of Cry1AbG439D protoxin (100 nM):PxHsp70 (750 nM), Cry1AbG439D protoxin (100 nM):PxHsp90 (750 nM) or Cry1AbG439D protoxin (100nM):PxHsp70/PxHsp90 (375 nM/375 nM)) to CAD CR12. After washing, the toxin bound to the CR12 was revealed with anti-Cry1Ab antibody as described in methods. P values indicated statistically significant differences: ***p> 0.0002.
3.4 Hsp70 and Hsp90 act cooperatively in countering resistance to Cry1Ac insecticidal protein
High resistance to Cry toxins in different insects has been linked to mutations affecting the expression of Cry toxins receptors (6). It was previously described that the resistance of NO-QAGE P. xylostella population, that is highly resistant to Cry1Ac toxin (> 1000-fold resistance), was linked to a 30 bp deletion on the ABCC2 gene, a functional Cry1Ac receptor (24). Therefore, we analyzed if PxHsp70 and PxHsp90 could counteract resistance to Cry1Ac toxin in the NO-QAGE strain. The 3rd instar larvae of NO-QAGE strain were fed with Cry1Ac (25 ng/cm2) along with 250 or 500 ng of PxHsp70 or PxHsp90 or the mixture of the two chaperones (250 ng/cm2 of each chaperone). Figure 4 shows that 500 ng/cm2 PxHsp70 had no significant effect on the Cry1Ac mortality to the NO-QAGE strain (19% mortality of Cry1Ac with or without PxHsp70), in contrast to 500 ng/cm2 PxPHsp90 that showed significant increase in mortality of Cry1Ac (from 19% mortality to 48% mortality; p< 0.0001). Finally, when 250 ng/cm2 of both chaperones were fed together along with the Cry1Ac toxin to the NO-QAGE strain a significant increase in mortality was observed (from 19% mortality to 84% mortality in the presence of both chaperones; p<0.0001). These results show that PxHsp70 and PxHsp90 act cooperatively to counteract insect resistance to Cry toxins (Figure 4).
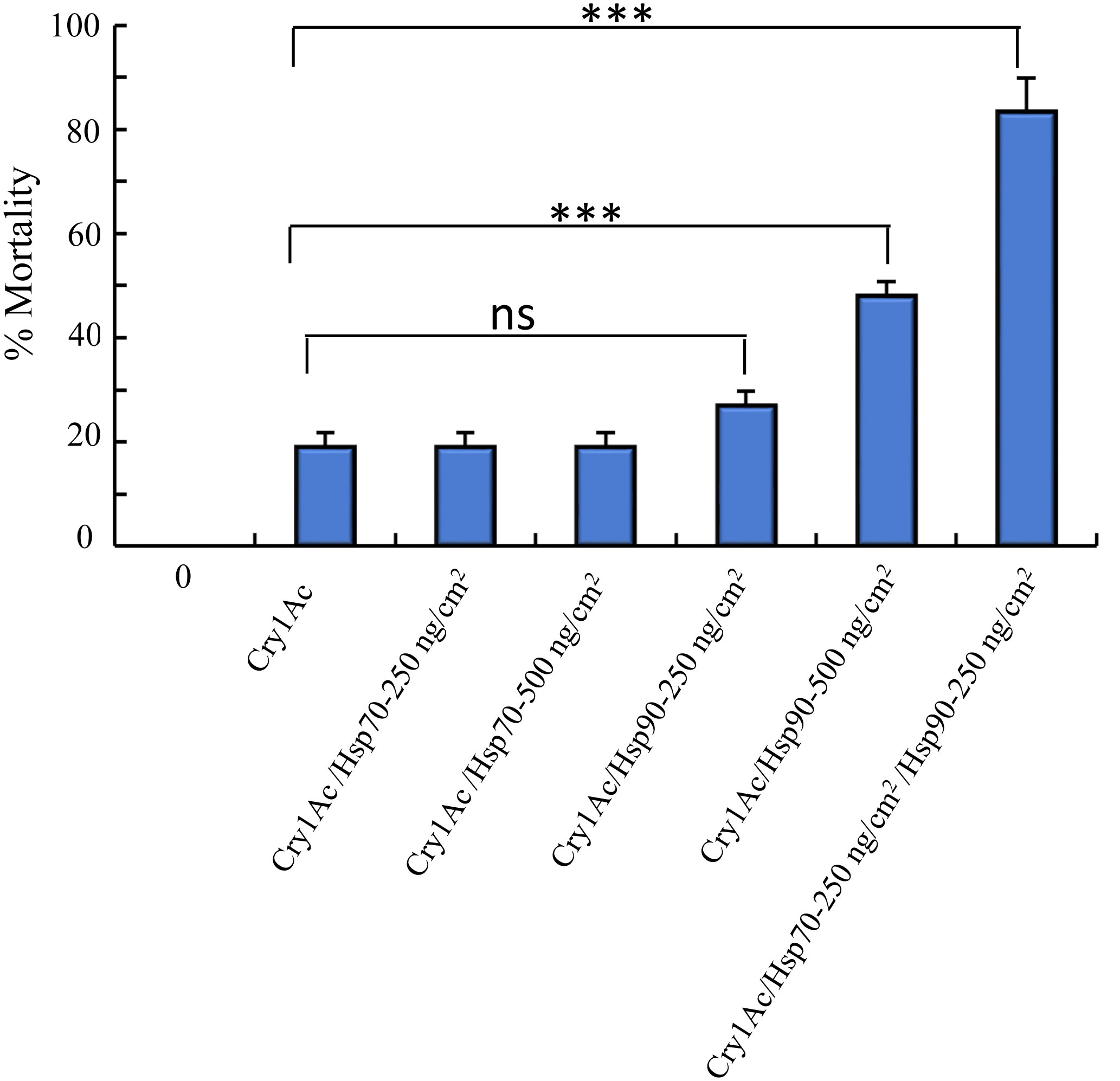
Figure 4 PxHsp70 and PxHsp90 recovers Cry1Ac toxicity against Plutella xylostella NO-QAGE Cry1Ac resistant strain. Percentage of mortality of Cry1Ac protoxin (25 ng/cm2) against P. xylostella NO-QAGE strain in the presence of different concentrations of PxHsp70 (250 or 500 ng/cm2), PxHsp90 (250 or 500 ng/cm2) or Pxhsp70+PxHsp90 (250 + 250 ng/cm2). Data with standard deviations represent means of three treatments using 24 larvae per treatment in each repetition. P values indicated statistically significant differences: ***p> 0.0001; ns, not significant difference.
4 Discussion
Bt hijacked an important cellular function for enhancing its infection capability, making use of insect cellular chaperones for enhancing Cry toxicity and for lowering the evolution of insect resistance to Cry toxins. In the case of different virus, it has been shown that Hsp90 assists certain viral proteins at different stages of infection (25), while in the case of the cholera toxin, Hsp90 assists the translocation of the catalytic subunit to the cytoplasm where it affects cAMP cellular levels (26) indicating that different microbial pathogens make use of cellular chaperones for their own benefit.
Previous work showed that that lowering down Hsp90 expression by RNAi in the mosquito Aedes aegypti resulted in a tolerant phenotype to Cry11Aa toxin (27). This result suggested that Hsp90 was somehow involved in the toxicity pathway of Cry toxins. Regarding the mechanism involved on the enhancement of Cry toxicity induced by Hsp90 action, previous work showed that PxHsp90 assists Cry1Ab or Cry1Ac toxins by protecting these protoxins from degradation by larval midgut proteases and also by enhancing binding of Cry1Ab to cadherin receptor (14). Here we show that PxHsp70 also protected Cry1Ab protoxin from degradation by larval gut proteases. However, PxHsp70 had no effect on binding of Cry1AbG439D to a CAD CR12 fragment receptor. We showed here that both chaperones act together since the binding of Cry1AbG439D to a cadherin fragment CR12 was much higher when both chaperones were used together with the Cry1AbG439D mutant in contrast when the mutant protein was treated with only PxHsp70 or PxHsp90. As described previously Hsp70 is consider a co-chaperone of Hsp90 since Hsp70 assist unfolded intermediates by recognizing stretches of hydrophobic residues to fold them into their native state, while Hsp90 assist later stages of folding or activation (15, 16). Thus, it is possible that the alkaline gut conditions of the larvae, partially unfold the Cry protoxins enhancing their degradation by gut proteases and limiting their binding to larval brush border membrane proteins.
Since PxHsp70 and PxHsp90 act cooperatively to recover the toxicity of Cry1AbG439D affected in receptor binding, we explored the possibility that these chaperones could recover the toxicity of Cry1Ac to a P. xylostella Cry1Ac resistant strain, NO-QAGE, whose resistance has been shown to be linked to a mutation in the ABCC2 receptor gene (24). Here we show that both chaperones, PxHsp70 and PxHsp90 act cooperatively to recover the toxicity of Cry1Ac to the NO-QAGE strain. This result suggests that the insect chaperones assist Cry1Ac toxin to bind to other midgut proteins, besides ABCC2, recovering its toxicity. Although we have not identified the gut proteins involved in binding of Cry1Ac in the NO-QAGE strain in the presence of both chaperones it is possible that ABCC3 could be involved, since it has been shown that ABCC2 and ABCC3, orthologous proteins, have redundant roles in Cry1Ac toxicity in P. xylostella (28). Another possibility is that increased binding to cadherin may be involved, since we have shown that both PxHsp90 or PxHsp70 enhances binding of Cry1Ab protoxin to M. sexta cadherin (Figure 3B) (14).
Both Hsp70 and Hsp90 are intracellular proteins that have important roles in maintaining the cellular proteome homeostasis (15, 16). However, Cry toxins exert their toxicity by forming pores in the cellular membrane. It was recently shown that the mosquitocidal Cry11Aa toxin is internalized inside the midgut cells, although it is not clear if its internalization is related to toxicity (29). Since PxHsp70 and PxHsp90 protect Cry protoxins from gut proteases and also enhance binding to membrane gut proteins it is likely that their effect on Cry toxicity is exerted outside the cell. Cry toxins burst insect cells by osmotic shock and both Hsp70 and Hsp90 are abundant cellular proteins (15, 16, 30), thus it is possible that the insect chaperones are released, along with other proteins, into the gut lumen when cells are burst by osmotic shock, facilitating their interaction with the Cry toxins outside the cells. Bacterial infection can enhance chaperones expression as a result of cell stress (31). Thus, Bt took advantage of stress responses in arthropods to enhance its toxicity and to counter resistance to the Bt Cry toxins. In any case, the effect of insect chaperones in increasing Cry toxicity in susceptible and in Cry-resistant insects could have important biotechnological applications.
Data availability statement
The original contributions presented in the study are included in the article/supplementary material. Further inquiries can be directed to the corresponding author.
Author contributions
BG-G performed toxicity assays against Px NO-QAGE strain and binding analysis to CAD receptor, TS performed toxicity assays of Cry1Ab and Cry1Ab mutants and binding of Hsp70 to Cry1Ab mutants. SC performed analysis of Cry1Ab protoxin stability in the presence of Hsp70. NN prepare recombinant proteins from E. coli. AB planned experiments, prepare figures and wrote the manuscript. MS planned experiments, prepare figures and wrote the manuscript. All authors contributed to the article and approved the submitted version.
Funding
This work was funded by CONACyT grant no. No. A1-S-12053 and also by CORTEVA agriscience.
Acknowledgments
To Lizbeth Cabrera and Jorge Sanchez for technical assistance.
Conflict of interest
BG-G, AB and MS are holders of patent: Enhancing Bacillus thuringiensis Cry insecticidal activity with a chaperone. Data submission September 16, 2016 patent application US Provisional 62/395768. PCT/IB2017/055416.
The remaining authors declare that the research was conducted in the absence of any commercial or financial relationships that could be construed as a potential conflict of interest.
Publisher’s note
All claims expressed in this article are solely those of the authors and do not necessarily represent those of their affiliated organizations, or those of the publisher, the editors and the reviewers. Any product that may be evaluated in this article, or claim that may be made by its manufacturer, is not guaranteed or endorsed by the publisher.
References
1. Bravo A, Likitvivatanavong S, Gill SS, Soberón M. Bacillus thuringiensis: A story of a successful bioinsecticide. Insect Biochem Mol Biol (2011) 41:423–31. doi: 10.1016/j.ibmb.2011.02.006
2. Crickmore N, Berry C, Panneerselvam S, Mishra R, Connor TR, Bonning BCA. Structure-based nomenclature for Bacillus thuringiensis and other bacteria-derived pesticidal proteins. J Invertebr Pathol (2021) 186:107438. doi: 10.1016/j.jip.2020.107438
3. Sanahuja G, Banakar R, Twyman RM, Capell T, Christou P. Bacillus thuringiensis: A century of research, development and commercial applications. Plant Biotechnol J (2011) 9:283–300. doi: 10.1111/j.1467-7652.2011.00595.x
4. Pardo-López L, Soberón M, Bravo A. Bacillus thuringiensis Insecticidal three-domain cry toxins: mode of action, insect resistance and consequences for crop protection. FEMS Microbiol Rev (2013) 37:3–22. doi: 10.1111/j.1574-6976.2012.00341.x
5. Tabashnik BE, Carrière Y. Surge in insect resistance to transgenic crops and prospects for sustainability. Nat Biotechnol (2017) 35:926–35. doi: 10.1038/nbt.3974
6. Jurat-Fuentes JL, Heckel DG, Ferré J. Mechanisms of resistance to insecticidal proteins produced by Bacillus thuringiensis. Ann Rev Entomol (2021) 66:121–40. doi: 10.1146/annurev-ento-052620-073348
7. Bravo A, Gómez I, Porta H, Garcia-Gómez BI, Rodriguez-Almazan C, Pardo L, et al. Evolution of Bacillus thuringiensis cry toxins insecticidal activity. Microbial Biotechnol (2013) 6:17–26. doi: 10.1111/j.1751-7915.2012.00342.x
8. Evdokimov AG, Moshiri F, Sturman EJ, Rydel TJ, Zheng M, Seale JW, et al. Structure of the full-length insecticidal protein Cry1Ac reveals intriguing details of toxin packaging into in vivo formed crystals. Prot Sci (2014) 23:1491–7. doi: 10.1002/pro.2536
9. Gómez I, Sanchez J, Muñoz-Garay C, Matus V, Gill SS, Soberón M, et al. Bacillus thuringiensis Cry1A toxins are versatile-proteins with multiple modes of action: two distinct pre-pores are involved in toxicity. Biochem J (2014) 459:383–96. doi: 10.1042/BJ20131408
10. Peña A, Grande R, Sánchez J, Tabashnik BE, Bravo A, Soberón M, et al. The c-terminal protoxin domain of Bacillus thuringiensis Cry1Ab has a functional role in binding to GPI-anchored receptors in the insect midgut. J Biol Chem (2018) 293:20263–72. doi: 10.1074/jbc.RA118.005101
11. Tabashnik BE, Zhang M, Fabrick JA, Wu Y, Gao M, Huang F, et al. Dual mode of action of bt proteins: protoxin efficacy against resistant insects. Sci Rep (2015) 5:15107. doi: 10.1038/srep15107
12. Chen LZ, Liang GM, Zhang J, Wu KM, Guo YY, Rector BG. Proteomic analysis of novel Cry1Ac binding proteins in Helicoverpa armigera (Hübner). Arch Ins Biochem Physiol (2010) 73:61–73. doi: 10.1002/arch.20340
13. Xu L, Ferry N, Wang Z, Zhang J, Edwards MG, Gatehouse AM, et al. A proteomic approach to study the mechanism of tolerance to bt toxins in Ostrinia furnicalis larvae selected for resistance to Cry1Ab. Transgenic Res (2013) 22:1155–66. doi: 10.1007/s11248-013-9718-3
14. Garcia-Gómez BI, Cano SN, Zagal EE, Dantán-Gonzalez E, Bravo A, Soberón M. Insect Hsp90 chaperone assist Bacillus thuringiensis cry toxicity by enhancing protoxin binding to receptor and by protecting protoxin from gut protease degradation. mBio (2019) 10:e02775–19. doi: 10.1128/mBio.02775-19
15. Mayer MP. Hsp70 chaperone dynamics and molecular mechanism. Trends Biochem Sci (2013) 38:507–14. doi: 10.1016/j.tibs.2013.08.001
16. Karagöz GE, Rüdiger SGD. Hsp90 interaction with clients. Trends Biochem Sci (2015) 40:117–25. doi: 10.1016/j.tibs.2014.12.002
17. Pacheco S, Gómez I, Arenas I, Saab-Rincon G, Rodríguez-Almazán C, Gill SS, Bravo A, Soberón M. Domain II loop 3 of Bacillus thuringiensis Cry1Ab toxin is involved in a “ping pong” binding mechanism with Manduca sexta aminopetidase-n and cadherin receptors. J Biol Chem (2009) 284:32750–7. doi: 10.1074/jbc.M109.024968
18. Soberón M, Pardo-López L, López I, Gómez I, Tabashnik B, Bravo A. Engineering modified bt toxins to counter insect resistance. Science (2007) 318:1640–2. doi: 10.1126/science.1146453
19. Queitsch C, Sangster TD, Lindquist S. Hsp90 as capacitor of phenotypic variation. Nature (2002) 417:618–24. doi: 10.1038/nature749
20. Jarosz DF, Lindquist S. Hsp90 and environmental stress transform the adaptive value of natural genetic variation. Science (2010) 330:1820–4. doi: 10.1126/science.1195487
21. Torres-Quintero M, Gómez I, Pacheco S, Sánchez J, Flores H, Osuna J, et al. Engineering Bacillus thuringiensis Cyt1Aa toxin specificity from dipteran to lepidopteran toxicity. Sci Rep (2018) 8:4989. doi: 10.1038/s41598-018-22740-9
22. Jiménez-Juárez A, Muñoz-Garay C, Gómez I, Saab-Rincon G, Damian-Alamazo JY, Gill SS, et al. Bacillus thuringiensis Cry1Ab mutants affecting oligomer formation are non-toxic to Manduca sexta larvae. J Biol Chem (2007) 282:21222–9. doi: 10.1074/jbc.M701314200
23. Rodríguez-Almazán CR, Zavala LE, Muñoz-Garay C, Jiménez-Juárez N, Pacheco S, Masson L, Soberón M, Bravo A, et al. Dominant negative mutants of Bacillus thuringiensis Cry1Ab toxin function as anti-toxins: demonstration of the role of oligomerization in toxicity. PloS One (2009) 4:e5545. doi: 10.1371/journal.pone.0005545
24. Baxter SW, Badenas-Perez FR, Morrison A, Vogel H, Crickmore N, Kain W, et al. Parallel evolution of bt toxin resistance in Lepidoptera. Genetics (2011) 189:675–9. doi: 10.1534/genetics.111.130971
25. Wang Y, Jin F, Wang R, Li F, Wu Y, Kitazato K, et al. HSP90: a promising broad-spectrum antiviral drug target. Arch Virol (2017) 162:3269–82. doi: 10.1007/s00705-017-3511-1
26. Burress H, Taylor M, Banerjee T, Tatulian SA, Teter K. Co- and post-translocation roles for HSP90 in cholera intoxication. J Biol Chem (2014) 289:33644–54. doi: 10.1074/jbc.M114.609800
27. Cancino-Rodezno A, Lozano L, Oppert C, Castro JI, Lanz-Mendoza H, Encarnación S, et al. Comparative proteomic analysis of Aedes aegypti larval midgut alter intoxication with Cry11Aa toxin from bacillus thuringiensis. PloS One (2012) 7:e37034. doi: 10.1371/journal.pone.0037034
28. Guo ZJ, Sun D, Kang S, Zhou JL, Gong LJ, Qin JY, et al. CRISPR/Cas9-mediated knockout of both the PxABCC2 and PxABCC3 genes confers high-level resistance to Bacillus thuringiensis Cry1Ac toxin in the diamondback moth, Plutella xylostella (L). Insect Biochem Mol Biol (2019) 107:31–8. doi: 10.1016/j.ibmb.2019.01.009
29. López-Molina S, do Nascimento NA, Neves Lobo Silva-Filha MA, Guerrero A, Sánchez J, Pacheco S, et al. In vivo Nanoscale analysis of the dynamic synergistic interaction of Bacillus thuringiensis Cry11Aa and Cyt1Aa toxins in aedes aegypti. PloS Pathog (2021) 176(1):e1009199. doi: 10.1371/journal.ppat.1009199
30. Luengo TN, Mayer MP, Rüdiger SGD. The Hsp70-Hsp90 chaperone cascade in protein folding. Trends Cell Biol (2019) 29:164–77. doi: 10.1016/j.tcb.2018.10.004
Keywords: heat shock proteins, chaperones, Bacillus thuringiensis, cry toxins, protoxins, toxin receptors, Insect resistance
Citation: García-Gomez BI, Sánchez TA, Cano SN, do Nascimento NA, Bravo A and Soberón M (2023) Insect chaperones Hsp70 and Hsp90 cooperatively enhance toxicity of Bacillus thuringiensis Cry1A toxins and counteract insect resistance. Front. Immunol. 14:1151943. doi: 10.3389/fimmu.2023.1151943
Received: 27 January 2023; Accepted: 11 April 2023;
Published: 20 April 2023.
Edited by:
Humberto Lanz-Mendoza, National Institute of Public Health (Mexico), MexicoReviewed by:
Jianming Chen, Minjiang University, ChinaRenjie Jiao, Guangzhou Medical University, China
Copyright © 2023 García-Gomez, Sánchez, Cano, do Nascimento, Bravo and Soberón. This is an open-access article distributed under the terms of the Creative Commons Attribution License (CC BY). The use, distribution or reproduction in other forums is permitted, provided the original author(s) and the copyright owner(s) are credited and that the original publication in this journal is cited, in accordance with accepted academic practice. No use, distribution or reproduction is permitted which does not comply with these terms.
*Correspondence: Mario Soberón, bWFyaW8uc29iZXJvbkBpYnQudW5hbS5teA==