- 1Department of Bone and Joint Surgery, Peking University Shenzhen Hospital, Shenzhen, China
- 2National and Local Joint Engineering Research Center of Orthopaedic Biomaterials, Peking University Shenzhen Hospital, Shenzhen, China
- 3Department of Ultrasonography, Peking University Shenzhen Hospital, Shenzhen, China
Objectives: The aim of this systematic review was to summarize the available literature on gut microbiome (GMB) and osteoarthritis (OA), analyze the correlation between GMB and OA, and explore potential underlying mechanisms.
Methods: A systematic search of the PubMed, Embase, Cochrane, and Web of Science with the keywords “Gut Microbiome” and “Osteoarthritis” was conducted to identify the human and animal studies exploring the association between GMB and OA. The retrieval time range was from the database inception to July 31, 2022. Studies reported the other arthritic diseases without OA, reviews, and studies focused on the microbiome in other parts of the body with OA, such as oral or skin, were excluded. The included studies were mainly reviewed for GMB composition, OA severity, inflammatory factors, and intestinal permeability.
Results: There were 31 studies published met the inclusion criteria and were analyzed, including 10 human studies and 21 animal studies. Human and animal studies have reached a consistent conclusion that GMB dysbiosis could aggravate OA. In addition, several studies have found that alterations of GMB composition can increase intestinal permeability and serum levels of inflammatory factors, while regulating GMB can alleviate the changes. Owing to the susceptibility of GMB to internal and external environments, genetics, and geography, the included studies were not consistent in GMB composition analysis.
Conclusion: There is a lack of high-quality studies evaluating the effects of GMB on OA. Available evidence indicated that GMB dysbiosis aggravated OA through activating the immune response and subsequent induction of inflammation. Future studies should focus on more prospective, cohort studies combined with multi-omics to further clarify the correlation.
1 Introduction
Osteoarthritis (OA) is the most common musculoskeletal disease characterized by progressive articular cartilage loss, osteophyte formation, subchondral bone remodeling and joint inflammation (1, 2). OA is the main cause of joint pain and periarticular muscle weakness, followed by loss of function, increased disability, reduced performance of activities of daily living, and reduced health-related quality of life (3, 4). The aging global population and high incidence of obesity and joint injuries have increased the socioeconomic burden of OA, with an estimated 250 million people affected worldwide (1, 5, 6). Based on different exposure factors, OA is considered to be a collection of multiple phenotypes, each with specific pathophysiological and clinical features, such as metabolic OA, traumatic OA, and aging-related OA (7–9). These exposures alone or in concert contribute to the complex cross-talk between mechanical, biochemical, and cellular factors that ultimately lead to OA. Although OA is a typical joint degenerative disease, the role of inflammatory factors in its occurrence and development has attracted the attention of researchers. OA is characterized by chronic and low-grade inflammation, primarily mediated by the innate immune system (10, 11). As the pathogenesis of OA has not been completely elucidated, there is no recognized therapeutic target for advanced OA except pain management or joint replacement.
A previous large-scale study estimated that the human gut microbiome (GMB) contains approximately 35,000 bacterial species, of which 90% are members of the phyla Firmicutes and Bacteroidetes (12–14). GMB and its metabolites are closely related to host growth and development, immunity, and longevity (15), and perform a series of functions, such as maintaining metabolic homeostasis, developing and maturing the immune system, fighting infection, and producing neurotransmitters (16). Under physiological conditions, GMB can maintain a state of balance with the host to achieve symbiosis. However, this symbiosis can be disrupted and is termed GMB dysbiosis (17). Mancabelli et al. (18) conducted a meta-analysis of GMB in pre-agricultural and modern societies and showed that the modernization process altered the overall composition of human GMB by increasing and/or decreasing certain microbiota. According to previous studies, GMB has been closely associated with various pathological conditions, including obesity, diabetes, neurodegenerative diseases, cancer, and musculoskeletal diseases (19, 20). Based on the relationship between GMB and OA, experts have speculated that GMB dysbiosis may be closely related to the occurrence and development of OA (21–23); however, no consistent conclusion has been reached. In this systematic review, we aim to methodically summarize and analyze the latest evidence on GMB and OA in human and animal studies, systematically elaborate the “Gut-Joint” axis, and explore the underlying mechanisms by which GMB dysbiosis contributes to the progression of OA. With future research expected to deepen understanding of the correlation between GMB and OA, GMB dysbiosis may be a new target for the prevention or early treatment of OA.
2 Materials and methods
This systematic review of studies exploring the association between GMB and OA was conducted based on the method recommended by the Preferred Reporting Items for Systematic Review and Meta-Analyses (PRISMA) guidelines (2020) (Supplementary PRISMA 2020 Checklist) (24). It was registered on International Platform of Registered Systematic Review and Meta-analysis Protocols (INPLASY). The registration number is INPLASY202330039 and the protocol is available in full on inplasy.com (https://inplasy.com/inplasy-2023-3-0039/).
2.1 Search strategy and study selection
From database inception to July 31, 2022, we searched the PubMed, Embase, Cochrane, and Web of Science databases with the keywords “Gut Microbiome” and “Osteoarthritis” (Supplementary retrieval strategy). Inclusion criteria were human and animal studies exploring the association between GMB and OA. Exclusion criteria were arthritic diseases of other types, such as rheumatoid arthritis (RA), ankylosing spondylitis (AS); review; microbiome in other parts of the body with OA, such as oral or skin. Studies were screened by the order of duplication, title, abstract, and full text. In the process of analysis, if any literature was omitted, it was manually searched and included. The literature was screened by two independent researchers (Liu and Li), strictly adhering to uniform criteria. Any questions on selection of studies during the literature search were negotiated with each other or evaluated by a third party.
2.2 Study evaluation
Three reviewers (Liu, Li, and Xu) evaluated the studies independently. Following full text analysis, the selection of eligible studies was completed with the fourth reviewer (Wang). Any disagreements were discussed and reasons for inclusion or exclusion were shared.
2.3 Risk of bias evaluation
The quality of human studies was evaluated by Agency for Healthcare Research and Quality (AHRQ) scale on five aspects: selection bias, performance bias, attrition bias, detection bias, and reporting bias (25). The quality of animal studies was evaluated by the CAMARADES checklist on seven aspects: sample size calculation, random allocation, blinded evaluation of outcomes, appropriate animal model, animal welfare, peer review, and conflict of interest declaration (26). Three researchers (Liu, Li, Xu) evaluated the studies independently. Any questions were negotiated with each other or evaluated by a fourth researcher (Wang). The AHRQ evaluation scale scored study components as low risk, high risk, and unclear. Items from the CAMARADES checklist that were mentioned in a study were marked as “Yes”, otherwise were marked as “No”.
2.4 Data extraction and analysis
All data from the studies were extracted and evaluated in the same way. Extracted data included: author information, study design, evaluation methods, indicators correlated with GMB and OA, and the main findings. Considering that GMB dysbiosis could affect the intestinal mucosal barrier and inflammatory response, the indicators related to inflammation and intestinal permeability were also extracted.
3 Results
3.1 Study selection
A total of 6470 references were retrieved, including 732 in PubMed, 108 in Cochrane, 1459 in Embase, and 4171 in Web of Science. After sequential screening in order of duplication, title, abstract, and full text, 27 studies remained. The full-text assessment of excluded studies was shown in Supplementary Table 1. In the process of literature analysis, 4 studies were searched manually. Therefore, 31 studies were included in the final analysis—10 human studies (Table 1) and 21 animal studies (Table 2). The detailed literature retrieval process is shown in Figure 1.
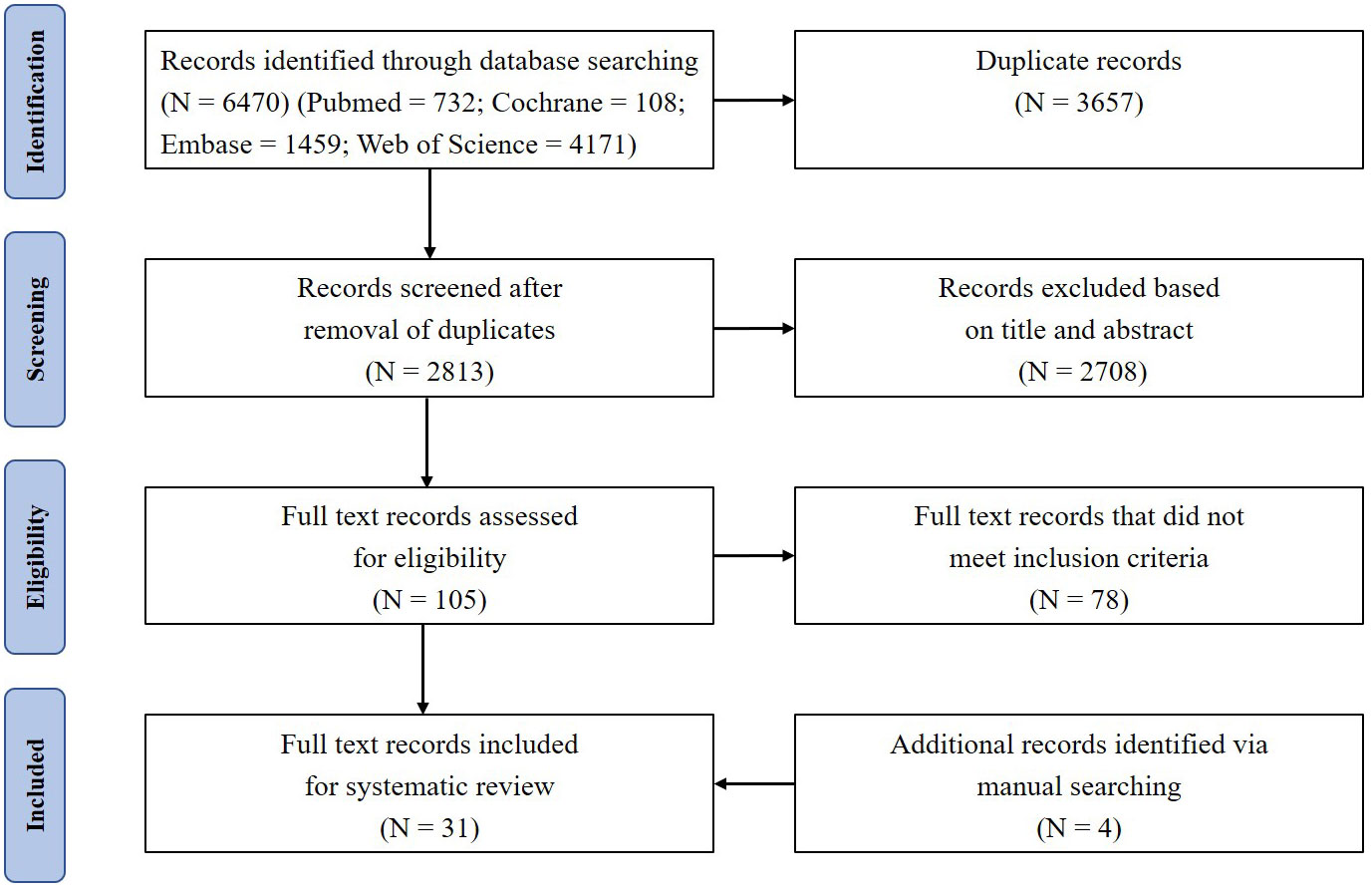
Figure 1 PRISMA flow diagram depicts the search results and selection of included studies in this systematic review.
3.2 Basic characteristics of included studies
The human studies comprised six observational studies (27–30, 32, 35), a two-sample Mendelian randomization (MR) study (36), and three randomized controlled trials (RCTs) (31, 33, 34). The observational studies were conducted to compare OA patients with normal controls or RA patients to identify differences in GMB composition and function. The MR study initially found the causal relationship between GMB and OA through large-scale genome-wide association studies (GWAS) summary statistics. The RCTs reported that supplemented with green-lipped mussels (GLM) (31), glucosamine sulfate (GS), Streptococcus thermophilus (TCI633) (33), and Lactobacillus casei Shirota (34) can significantly alleviate the symptoms of OA by regulating GMB. The basic characteristics of participants in human studies was shown in Supplementary Table 2. The animal studies included three non-intervention experiments (37, 55, 56), seven experiments about disturbing GMB to exacerbate OA (21, 38, 40, 41, 46, 48, 49), and 11 experiments about regulating GMB dysbiosis to alleviate OA (39, 42–44; 45, 47, 50–54). The risk of bias was evaluated for each study (Figures 2, 3; Supplementary Table 3, 4). Among the human studies, only one (31) had all five items as low risk, while the remaining eight studies had high risks and/or unclear, especially for attrition bias, which was not clearly indicated in the studies. The sample size and peer review declaration were not included in all of the animal studies. All of the animal experiments were conducted using currently recognized and established animal models, and the process was in line with animal ethics requirements.
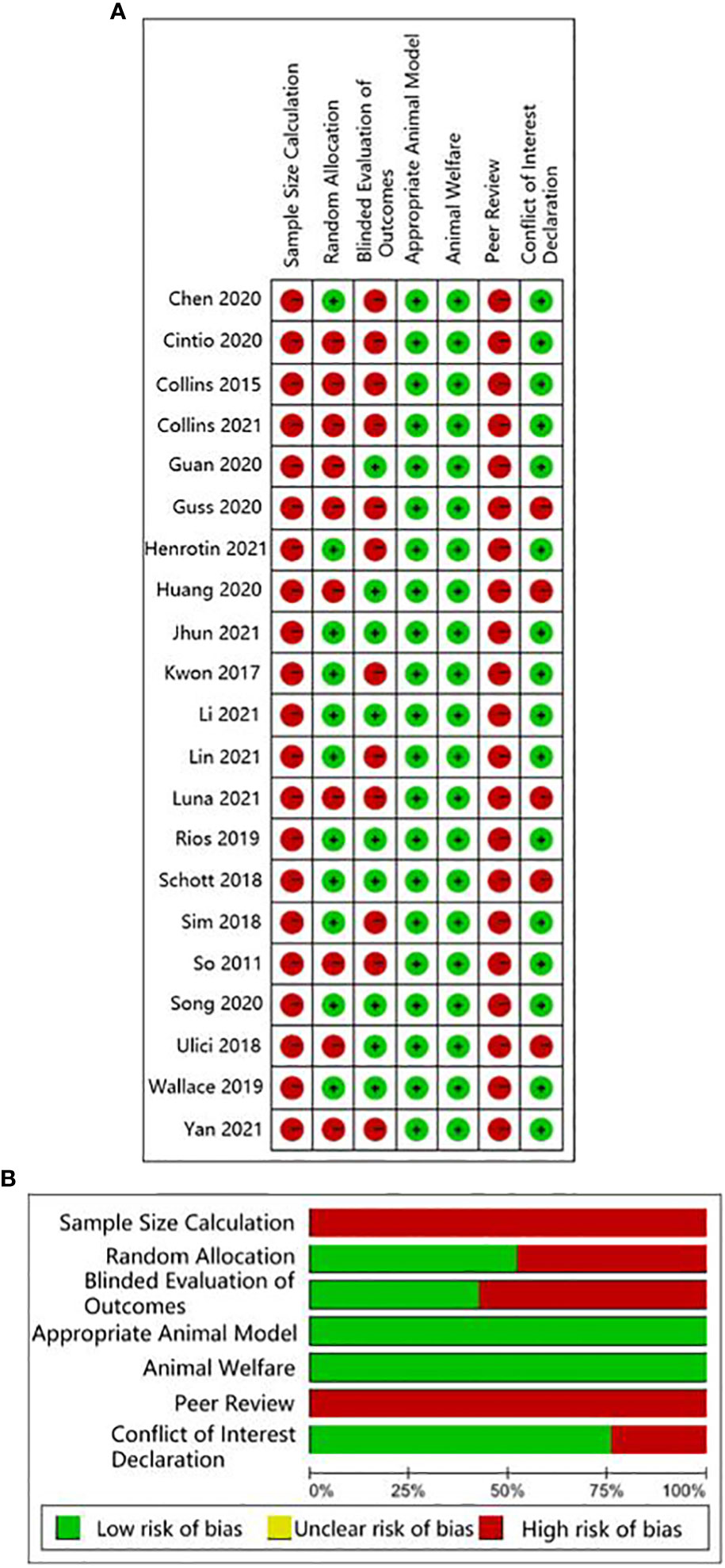
Figure 3 CAMARADES evaluation for animal experiments. (A) Traffic-lights plots; (B) Weighted bar plots.
3.3 Human studies: Observational studies reporting the association between GMB and OA
Wei et al. (27) found a significant correlation between β-diversity and symptomatic hand OA. Patients with symptomatic hand OA showed higher relative abundance of Bilophila and Desulfovibrio, and lower relative abundance of Roseburia, compared with controls. Among functional pathways, those for amino acid, carbohydrate, and lipid metabolism were markedly different between the two groups. Ramasamy et al. (28) showed that the abundance of Peptococcus, Shimwellia, Propionibacterium, Intestinimonas, and Parvimonas in knee OA was significantly higher compared with that in healthy controls. In addition, vitamin D deficiency had an effect on GMB composition, mainly reflected in Parabacteroides, Butyricimonas, and Gordonibacter dominating in knee OA with vitamin D deficiency, while Peptococcus, Intestimonas, Delftia, and Oribacterium were predominant in knee OA without vitamin D deficiency. Lee et al. (29) compared the GMB between RA and OA and found no difference in diversity, but the ratio of Bacteroidetes/Firmicutes (B/F) was lower in the RA group. In addition, the abundance of Lactobacilli and Prevotella was higher, while that of Bacteroides and Bifidobacterium was lower in the RA group compared with those of the OA group. Chen et al. (30) reported that the richness and diversity of GMB in OA patients were significantly reduced, and this was predominantly reflected by the decreased abundance of Bifidobacterium longum and Faecalibacterium prausnitzii and increased abundance of Clostridium spp. Functional prediction analysis revealed that tryptophan degradation, glutamine degradation, tyrosine degradation, propionate production, and acetate to acetyl-CoA were significantly enriched in the OA group, while triacylglycerol degradation, glycerol degradation, pentose phosphate pathway, acetyl-CoA to acetate, fructose degradation, and glycolysis were significantly enriched in the healthy control group. Boer et al. (32) reported a positive correlation between the abundance of Streptococcus and knee pain and synovial inflammation based on the largest cohort study to date (Rotterdam Study), which was verified in another independent cohort study (Lifelines DEEP Study). Wang et al. (35) detected decreasing diversity and richness in overweight patients with OA, with significant differences in 9 phyla and 87 genera, including Gemmiger, Klebsiella, Akkermansia, Bacteroides, Prevotella, Alistipes, and Parabacteroides.
3.4 Human studies: MR study reporting the causal relationship between GMB and OA
Yu et al. (36) firstly reported the causal relationship between GMB and OA through a two-sample MR approach based on GWAS summary statistics. Methanobacteriaceae, Desulfovibrionales, and Ruminiclostridium 5 were identified as being negatively related to the risk of knee OA, while Ruminococcaceae UCG003 and Enterorhabdus were negatively related to the risk of hip OA. In addition, 49 Gene Ontology (GO) biological processes were related to knee OA, such as regulations of melanocyte, pigment cell, and multicellular organism process. Three GO biological processes were associated with hip OA, including regulation of membrane potential, response to ammonium ion, and positive regulation of voltage-gated potassium channel activity.
3.5 Human studies: Clinical trials on the regulation of GMB to alleviate OA
Coulson et al. (31) observed the therapeutic effects of green-lipped mussels (GLM) and glucosamine sulfate (GS) in patients with OA. The results showed that the abundance of Clostridium and Staphylococcus decreased, while Lactobacillus, Streptococcus, and Eubacterium increased in the two groups. Specially, Bifidobacterium increased in the GLM group, while Enterococcus and yeast decreased in the GLM group, and Bacteroides decreased in the GS group, while Yeasts and Coliforms decreased in the GS group. At the end of the study, symptoms of OA and gastrointestinal manifestations significantly improved in both groups. Streptococcus thermophilus (TCI633), recently identified from human milk, is a bacterium that produces hyaluronic acid (HA) in the gastrointestinal tract. Lyu et al. (33) found that TCI633 contributed to improving serum collagen type II C-telopeptide (SCTX-II) and C-reactive protein (CRP), and alleviating OA progression. Lei et al. (34) investigated the effects of Lactobacillus casei Shirota (LcS) on patients with knee OA. In this double-blind, placebo-controlled trial, 537 patients with knee OA were randomly assigned to drink skimmed milk with LcS or placebo daily for 6 months. At the end of the study, patients in the LcS group exhibited significant improvements in Western Ontario and McMaster Universities Osteoarthritis Index (WOMAC) and visual analog scale (VAS) scores compared with those in the placebo group. C-reactive protein (CRP) levels were also significantly lower in patients receiving LcS compared with those in the placebo group. There was a strong linear correlation between CRP level and WOMAC and VAS scores.
3.6 Animal studies: Non-intervention studies reporting the association between GMB and OA
Yan et al. (37) examined the association between GMB and OA in spontaneously OA rhesus macaque and healthy rhesus macaque. GMB diversity was decreased in the OA group but the difference was not significant. The abundance of Lactobacillus significantly increased, while that of Prevotella and Ruminococcus decreased in the OA group. Mollicutes, Tenericutes, Coprobacillus, and Faecalitalea may be biomarkers of OA in monkeys. F/B was higher in the OA group compared with that in the control group but the difference was not statistically significant. In functional prediction analysis, 20 KEGG orthology (KO) pathways were different between the two groups, including 11 pathways that were significantly enriched in the OA group (dominated by Zinc/Manganese Transport System Permease protein and Cyclopropane-Fatty Acyl-Phospholipid synthase), and 9 pathways enriched in the healthy control group. Cintio et al. (55) compared CRP, vitamin B12, and folate in blood samples, along with the GMB, from dogs with chronic arthritis disease (AD) and healthy dogs. Compared with healthy dogs, the AD group had higher CRP and lower vitamin B12 and folate. GMB sequencing showed that the relative abundance of Megamonas was higher in the AD group, while those of Paraprevotellaceae, Porphyromonadaceae, and Mogibacteriaceae were lower. Wallace et al. (56) compared the effects of inactivity and exercise on OA and GMB in Hartley guinea pigs. The prevalence of knee OA was similar between the sedentary and exercise groups, but cartilage lesions were more severe in the sedentary group. In addition, the content of aggrecan in cartilage of the sedentary group was significantly lower than that of the exercise group. However, there were no significant differences between the two groups in cartilage thickness, collagen amount, muscle mass, subcutaneous fat, and GMB composition.
3.7 Animal studies: Intervention studies about GMB dysbiosis exacerbating OA progression
Huang et al. (38) found that germ-free (GF) mice without fecal transplantation had the lowest histological OA severity, while those transplanted with feces from OA patients with metabolic syndrome had the highest histological OA severity, levels of inflammatory biomarkers (IL-1β, IL-6, and MIP-1α), and intestinal mucosal permeability. High abundances of Fusobacterium and Faecalibacterium and a low abundance of Ruminococcaceae were strongly associated with OA severity and inflammatory markers. Guss et al. (40) investigated the effects of metabolic syndrome, obesity, and GMB on OA induced by cyclic compressive load. The study found that after 6 weeks of loading, the high-fat diet group had the most severe cartilage damage. Toll-like receptor-5 deficient (TLR5KO) mice with metabolic syndrome had cartilage damage similar to WT mice, which was alleviated by antibiotics. Collins et al. (41) found that the Modified Mankin score of high-fat diet mice was significantly higher than that of normal diet mice, and the serum lipopolysaccharide (LPS) was also significantly increased. Analysis of GMB showed a strong correlation between the abundance of Lactobacillus spp. and Methanobrevibacter spp. and Modified Mankin score. Ulici et al. (21) reported that GF mice had lower cartilage damage compared with specific-pathogen free (SPF) mice, especially among younger mice. Simultaneously, the osteophyte and serum LPS binding protein (LBP) levels of the younger GF mice were significantly lower compared with those of the SPF mice, but no significant difference in synovitis score and serum LPS was found. In addition, GMB composition was influenced by the severity of cartilage damage among SPF mice. Collins et al. (46) found that a high-fat diet (HFD) significantly increased LPS levels in synovial fluid and reduced the B/F. Nine new significant associations were also identified between GMB and OA severity. Guan et al. (48) used antibiotics to disturb GMB in mice of different genders and conducted destabilization of the medial meniscus (DMM) to establish a mouse model of OA. They found that the Osteoarthritis Research Society International (OARSI) score of female and male mice was significantly decreased after antibiotic treatment, as well as the serum levels of IL-6, TNF-α, calcium ion, and MMP-13. Luna et al. (49) performed cyclic loading to induce OA in mice and investigated the effects of HFD-induced obesity on cartilage damage and GMB. The study did not find an effect of obesity on cartilage damage, only a significant change in GMB composition was observed.
3.8 Animal studies: Intervention studies about regulating GMB to alleviate OA
Schott et al. (39) found that B/F and the abundance of Actinobacteria decreased, while the proinflammatory bacteria, dominated by Peptostreptococcaceae and Peptococcaceae, significantly increased in obese mice. Oligofructose supplementation could reverse obesity-induced GMB changes, reduce IL-12 and MCP-1, and increase IL-10. Moreover, oligofructose could also inhibit macrophage migration to the synovium and exert a chondroprotective effect by reducing the expression of Runx2, Mmp13, and COL X. Rios et al. (42) found that knee joint damage caused by a HFD was closely related to metabolic changes in mice, while prebiotics, a fiber diet, and aerobic exercise could alleviate the severity of cartilage damage. In addition, prebiotics and fiber supplementation could prevent the increase of LPS and ameliorate GMB dysbiosis in HFD-induced mice. So et al. (2010) fed mice with Lactobacillus casei and found that this treatment effectively improved pain, cartilage destruction, and lymphocytic infiltration, and reduced the proinflammatory cytokines (IL-1β, IL-2, IL-6, IL-12, IL17, TNF-α, IFN-γ) and MMPs (MMP1, MMP3, and MMP13). Tyndallized Clostridium butyricum (ID-CBT5101) could also inhibit inflammatory and bone metabolic markers (COX-2, IL-6, LTB4, COMP, MMP-2, MMP-3, MMP-9, MMP-13, TIMP-1, and TIMP-2), exerting a protective effect on cartilage (44). Kwon et al. (45) reported a therapeutic effect of probiotic complex, rosavin, and zinc on OA, mainly reflected in reductions in proinflammatory cytokines and catabolic factors accompanied by increases in anti-inflammatory cytokines and anabolic factors. Li et al. (47) reported that exercise could improve GMB diversity decreases, inflammation, and cartilage damage induced by HFD. Feeding with Lactobacillus paracasei subsp. paracasei M5 (M5) and chondroitin sulfate (CS) effectively prevented cartilage destruction and type II collagen degradation, and reduced the serum and sub-patellar fat pad leptin caused by HFD (50). Song et al. also found that there was a strong predictive relationship between Ruminococcaceae_UCG-014, Streptococcus, Bacteroides, and OA. Chen et al. (51) investigated the effect of moxibustion on knee cartilage injury and inflammation induced by monosodium iodoacetate (MIA). The moxibustion treatment significantly improved cartilage injury and reduced inflammatory factors in serum and cartilage. In addition, moxibustion had a regulatory effect on GMB composition. Lactobacillus rhamnosus, Streptococcus thermophilus (TCI633), and Bifidobacterium longum CBi0703 were all proved to exert a protective effect on cartilage through reducing inflammatory cytokines and catabolic factors in OA models (52–54); however, none of these studies elaborated on the changes of GMB.
4 Discussion
The causal relationship between OA and GMB has not yet been identified owing to the lack of corresponding research. Though Yu et al. (36) found a causal relationship between several microbiota and OA in a two-sample MR study based on the GWAS database, this conclusion was not supported by direct evidence. The consistent view among the studies is that the association between OA and GMB may be related to systemic or local LPS and subsequent elevation of other proinflammatory factors, such as TNF-a, IL-1b, IL-6, and CRP. These components activate the immune system and induce an inflammatory cascade. In the studies included in this systematic review, the human subjects have different geographical and ethnic backgrounds, and the experimental animals also have different genetic backgrounds. These variables can significantly affect GMB composition, leading to significant differences in the consistency of the studies. In addition, animal models of OA often use changes in diet or surgery to induce joint instability because it is difficult to develop OA spontaneously. This indicates that there is no accurate correspondence between humans and animal models, and there are even differences among various animal models. Considering the complexity of GMB composition and susceptibility to internal and external environments, it is challenging to identify a specific microorganism directly associated with OA.
It is well known that obesity and metabolic syndrome are risk factors for OA in addition to negatively affecting the GMB. According to animal studies, GMB changes have the potential to lower systemic inflammation and preserve cartilage damage in OA models. There is evidence that prebiotics or probiotics have an immunomodulatory effect that can reduce systemic inflammation and cartilage damage, as well as changes associated with OA in animals. There are limited human studies on this topic. Observational studies showed that blood LPS and LBP levels were associated with macrophage activation in the knee synovium and the severity of OA. Pain symptoms were associated with loss of GMB diversity and abundance of Streptococcus (32). According to these findings, blood toxemia or differences in GMB may contribute to OA pathogenesis. Supplementation with LcS, GLM and GS was found to alleviate OA symptoms in small clinical trials (31, 34). Nonetheless, these trials lack standardized formulations, have small sample sizes, inconsistent results, and the mechanisms mediating these outcomes remain unclear. It is necessary to conduct larger and more carefully planned randomized controlled trials before making any conclusions about the efficacy of such supplements.
Joints are traditionally considered sterile, but in recent years there have been studies that found bacterial DNA in articular cartilage and synovium. Zhao et al. (57) performed 16S rRNA gene sequencing to evaluate bacterial DNA in 16 synovial and 42 synovial fluid samples from 58 patients with OA. There were abundant and diverse bacterial DNA in these clinical samples, with Porphyromonas and Bacteroides prevalent in all 58 patients. The synovial tissue of patie
nts with OA was mainly composed of Bacteroides, Megacoccus, Haemophilus, Porphyromonas, and Streptococcus, while the synovial fluid was predominantly composed of Bacteroides. This study confirmed the presence of bacterial DNA in synovial and synovial fluid samples from OA lesions and revealed a potential correlation between bacterial DNA and lesion severity. Dunn and colleagues (58) found significant differences in the bacterial species present in human hip and knee joints. Cartilage was obtained under aseptic conditions from patients with primary knee OA and hip OA who underwent joint replacement, and from 30 fresh cadavers of similar sex, age, and body mass index (BMI) without OA. Deep sequencing of all samples showed that there were 63 different bacterial flora branches between the OA group and the control group. The OA group was dominated by Proteus, while the control group was dominated by Actinomycetes and Clostridium. Similarly, there were 46 branch differences in the composition of bacteria between knee and hip joints, among which hip OA was dominated by Proteus, while knee OA was dominated by Actinomycetes.
Although bacterial DNA has been found in cartilage specimens of humans and animals, no study has accurately identified where this bacterial DNA is stored: in the subchondral bone marrow, deep cartilage blood vessels, cartilage matrix, or chondrocytes. Furthermore, the process or mechanism by which these bacterial DNA or viable bacteria reach the cartilage remains unclear (58). Articular cartilage has no vessels, nerves, or lymphatic vessels, thus most of its nutrients are supplied from synovial fluid and subchondral bone marrow. The microstructure of cartilage matrix limits substance transport through pore size, charge, and molecular configuration (59). The average pore size of extracellular matrix (ECM) is estimated to be approximately 6.0 nm, whereas most bacteria have diameters ranging from 100 to 4000 nm, and bacterial spores have diameters of approximately 100 nm (60). Consequently, bacteria cannot penetrate the surface of cartilage and enter chondrocytes unless the surface is damaged by erosion. The same is true of bacterial DNA. In addition, bacterial DNA is negatively charged, and only positively charged substances can accumulate in the cartilage matrix; even neutral substances cannot enter chondrocytes (61). This phenomenon applies to most bacteria, since both Gram-positive and Gram-negative bacteria have negatively charged cell walls. In contrast, calcified cartilage, due to its increased permeability, allows passage of small solute molecules (62). During OA, there are more blood vessels and thickened tube diameters in calcified cartilage zones, which allows commensal bacterial components of the blood to transfer to calcified cartilage and subchondral bone, and even to enter normal chondrocytes through tiny cracks in the tide mark (63, 64) (Figure 3). Microorganisms from the gut may be stored in the subchondral bone for a long time and remain in a latent state, because the microbiota in the subchondral bone can also undergo anaerobic metabolism, which is consistent with the situation in the gut (65). Concurrently, commensal bacteria in the gut have antianabolic effects, which may inhibit osteoblasts and promote osteoclast formation through local interference with insulin-like growth factor-1 signaling, leading to bone loss (66). This transient invasion by microbiota can occur before birth; for example, Proteus, the predominant bacteria found in the blood and cartilage of healthy people, is part of the microbiota in the normal placenta (67).
The normal intestinal barrier acts as a mechanical barrier, chemical barrier, immune barrier, and biological barrier. Once barrier function is impaired, intestinal contents including GMB and its metabolites and immune cells will leak into the circulatory system, followed by chronic inflammation (68). Based on evidence of microbial components in the gut, blood, and joints, Tsai et al. (69) hypothesized that a “leaky” gut allows microbial-associated molecular patterns (MAMPs) correlated with dysregulation of immune signaling and promotion of inflammation to migrate from the gut to the joints, leading to OA. GMB dysbiosis can disrupt the structure and function of the intestinal barrier, and increase the porosity through several mechanisms (70). Outer membrane vesicles (OMVs), produced by pathogenic bacteria and commensal Gram-negative bacteria during the regulation of bacterial membrane proteins, have diameters ranging from 20 nm to 250 nm (71). Based on the in vitro activity of OMVs, Kaparakis-Liaskos and Ferrero (72) indicated that pathogens may use OMVs to disrupt the integrity of the mucosal epithelium and allow MAMPs to enter the submucosa. MAMPs could directly interact with various immune cells, including neutrophils, macrophages, and dendritic cells, contributing to pathological changes in the intestinal wall (Figure 4).
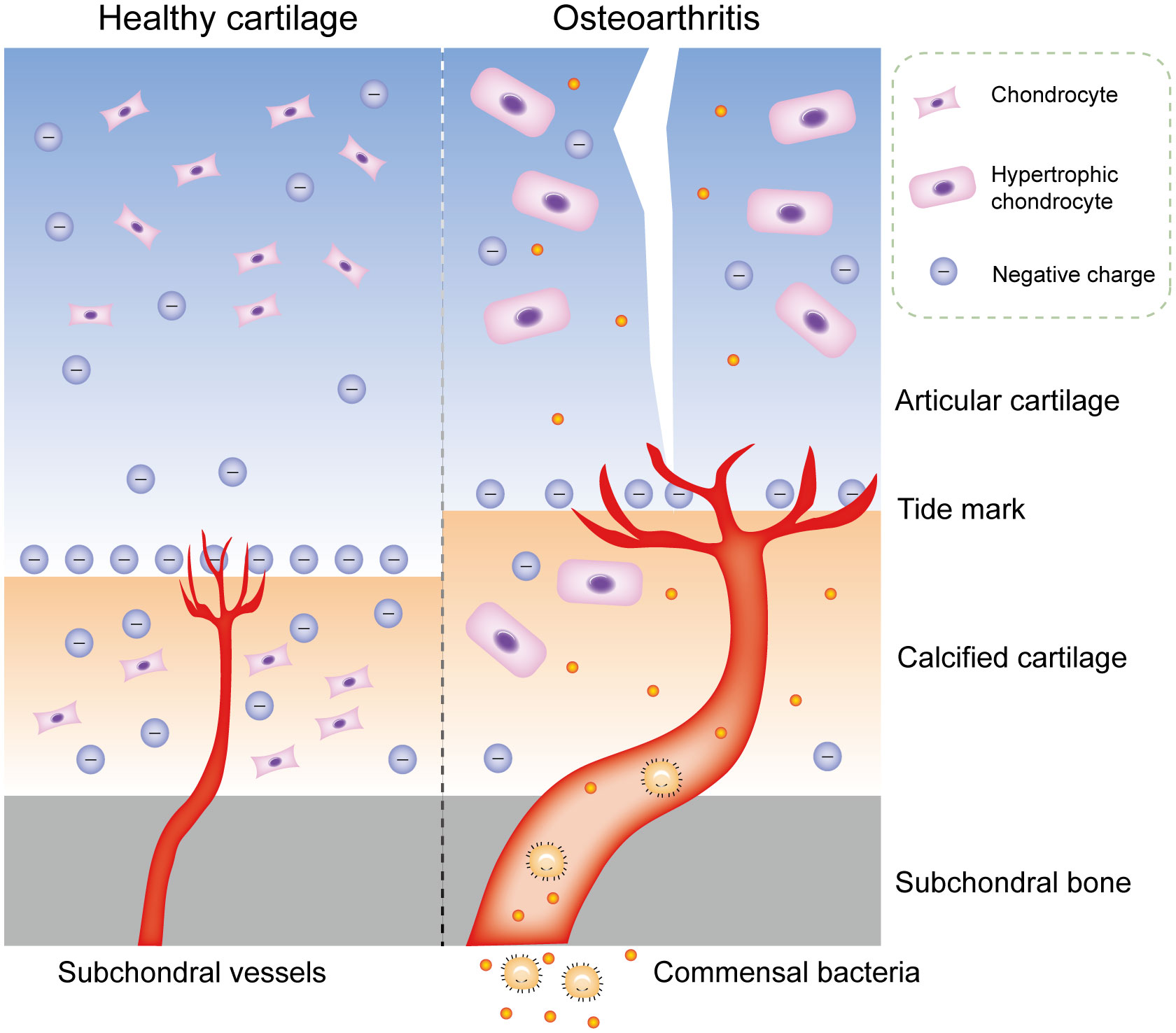
Figure 4 Commensal bacterial components may transfer to cartilage in OA. Physiologically, the microstructure of cartilage matrix limits substance transport through pore size, charge, and molecular configuration. During OA, there are more blood vessels and thickened tube diameters in calcified cartilage zones, which allows commensal bacterial components of the blood to transfer to calcified cartilage and subchondral bone, and even to enter normal chondrocytes through tiny cracks in the tide mark. OA, osteoarthritis.
MAMPs can affect systemic and local inflammation by activating the immune system, which could accelerate OA progression (58, 73–75). The inflammation cascade in the host is predominantly dependent on pattern recognition receptors, especially Toll-like receptors (TLRs) (76, 77). MAMPs are the most common ligands for these receptors and include LPS, peptidoglycan, flagellin, and bacterial DNA, which could be carried by OMVs through the mucosal epithelium into submucosa, triggering a proinflammatory response in resident immune cells. The inflammatory factors are delivered to the joints to stimulate innate immune receptors in cartilage and synovium, thereby inducing a cascade of inflammatory reactions (78–80). LPS activates an immune response by binding to the CD14-LBP-TLR4-MD-2, resulting in increased levels of activated NF-κB, followed by the release of inflammatory factors such as TNF-α, IL-1β, IL-6, RANKL, and IL-8 (81). Activation of the inflammatory response can promote catabolism, reduce anabolism, and enhance NF-κB activation, culminating in secondary inflammation of the joints (82, 83). Dendritic cells are key antigen presenting cells mediating innate and adaptive immune responses, which can induce the initiation and differentiation of naive CD4+T cells into effector cells (84). In addition, Li et al. (85) elucidated the role of T cells in OA, especially T helper (Th) cells such as Th1, Th17, and regulatory T cells (Treg). T cells are clearly present in OA and produce catabolic cytokines that stimulate proteases to disrupt cartilage matrix, which accelerates OA progression by modulating the adaptive immune system (86). GMB dysbiosis determines the differentiation of primitive CD4+ T cells into effector T cells or Treg cells. The balance between Treg cells and effector T cells is critical for maintaining immune homeostasis; disorder of this balance could lead to systemic or local inflammation, including in the joints (87) (Figure 5).
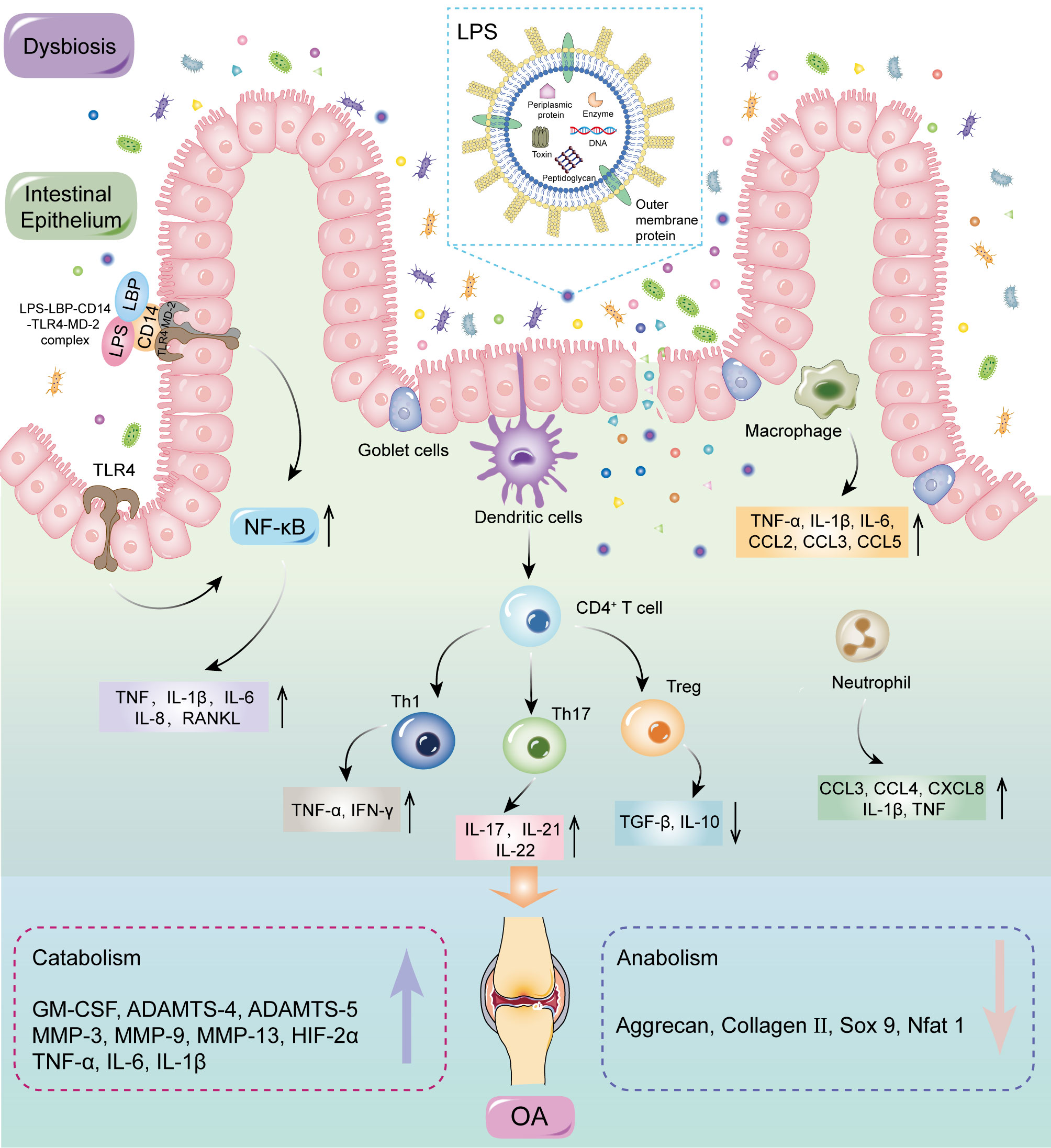
Figure 5 MAMPs may induce inflammation by activating the immune system. GMB dysbiosis can disrupt the structure and function of intestinal barrier, therefore allowing MAMPs to enter the submucosa. MAMPs could directly interact with various immune cells, including neutrophils, macrophages, and dendritic cells. Dendritic cells can induce the initiation and differentiation of naive CD4+T cells into effector cells. Activation of the inflammatory response can promote catabolism, reduce anabolism, and enhance NF-κB activation, culminating in secondary inflammation of the joints. LPS, lipopolysaccharide; LBP, LPS binding protein; CD 14, cluster of differentiation 14; TLRs, Toll-like receptors; MD-2, Myeloid differentiation protein-2; MAMPs, microbial-associated molecular patterns; NF-κB, nuclear factor kappa-B; TNF, tumor necrosis factor; IL, interleukin; CCL, CC-chemokine ligand; IFN, interferon; CXCL, CXC-chemokine ligand; GM-CSF, granulocyte–macrophage colony-stimulating factor; TGF, transforming growth factor; Th, T helper; Treg, regulatory T; MMP, matrix metallopeptidase; HIF, hypoxia inducible factor; SOX 9, SRY-Box transcription factor 9; Nfat, nuclear factor of activated T cells.
5 Conclusions and future perspectives
The development of high-throughput sequencing technology and metagenomic analysis has facilitated the accurate study of GMB. In this review, we systematically summarized the current evidence supporting the “Gut-Joint” axis hypothesis. However, owing to limitations in the quantity and quality of studies, the causal role of GMB in OA has not yet been concluded. Furthermore, identification of specific microbiome (s) closely related to OA progression is challenging. Nevertheless, combined with the relevant human and animal studies, it can be concluded that there is a correlation between GMB dysbiosis and OA progression, which may be related to activation of the immune response and subsequent induction of inflammation.
There is a need for more prospective, cohort studies to determine the exact causal connections between GMB dysbiosis and OA progression. It should be noted that this low degree systemic inflammation caused by GMB dysbiosis is not unique to OA, but also exists in other diseases, such as metabolic syndrome and cardiovascular disease. In this context, we speculate that GMB may have an effect on different OA subtypes. The development of multi-omics, such as metabolomics and transcriptomics, helps to link specific metabolites, genes or signaling pathways that contribute to the regulation of GMB. In addition, single-cell sequencing is expected to reveal the correlation between different cell subsets and GMB.
Author contributions
Conceptualization, SL and GL. Methodology, HX. Software, QW. Validation, SL, GL and HX. Formal analysis, YW. Figures, QY. Tables, AX. Writing—original draft preparation, SL and GL. Writing—review and editing, HX and FY. Supervision, JW and HZ. Project administration, JW. Funding acquisition, HZ. All authors contributed to the article and approved the submitted version.
Funding
This research was continuously funded by National Natural Science Foundation of China (No. 82172432; No. 82102568 and No. 82001319); National and Local Joint Engineering Research Center of Orthopaedic Biomaterials (No. XMHT20190204007); Shenzhen High-level Hospital Construction Fund, Shenzhen Key Medical Discipline Construction Fund (No. SZXK023); Shenzhen “San-Ming” Project of Medicine (No. SZSM201612092); Research and Development Projects of Shenzhen (No. KCXFZ20201221173411031;No. JCYJ20210324110214040; No. JCYJ20220531094214032; No. JCYJ20190809152409606); Guangdong Basic and Applied Basic Research Foundation (No. 2021A1515012586; No. 2021A1515220054; No. 2022B1515120046; No. 2022A1515220038; No. 2022A1515220111); the Scientific Research Foundation of PEKING UNIVERSITY SHENZHEN HOSPITAL (No. KYQD2021099).
Conflict of interest
The authors declare that the research was conducted in the absence of any commercial or financial relationships that could be construed as a potential conflict of interest.
Publisher’s note
All claims expressed in this article are solely those of the authors and do not necessarily represent those of their affiliated organizations, or those of the publisher, the editors and the reviewers. Any product that may be evaluated in this article, or claim that may be made by its manufacturer, is not guaranteed or endorsed by the publisher.
Supplementary material
The Supplementary Material for this article can be found online at: https://www.frontiersin.org/articles/10.3389/fimmu.2023.1150572/full#supplementary-material
References
1. Hunter DJ, Bierma-Zeinstra S. Osteoarthritis. Lancet. (2019) 393(10182):1745–59. doi: 10.1016/S0140-6736(19)30417-9
2. Wang L, He C. Nrf2-mediated anti-inflammatory polarization of macrophages as therapeutic targets for osteoarthritis. Front Immunol (2022) 13:967193. doi: 10.3389/fimmu.2022.967193
3. Deveza LA, Loeser RF. Is osteoarthritis one disease or a collection of many? Rheumatol (Oxford) (2018) 57(suppl_4):iv34–42. doi: 10.1093/rheumatology/kex417
4. de Sire A, Lippi L, Curci C, Calafiore D, Cisari C, Ammendolia A, et al. Effectiveness of combined treatment using physical exercise and ultrasound-guided radiofrequency ablation of genicular nerves in patients with knee osteoarthritis. Appl Sci (2021) 11:4338. doi: 10.3390/app11104338
5. GBD, Disease and Injury Incidence and Prevalence Collaborators. Global, regional, and national incidence, prevalence, and years lived with disability for 354 diseases and injuries for 195 countries and territories 1990-2017: a systematic analysis for the global burden of disease study 2017. Lancet (2017) 392(10159):1789–858. doi: 10.1016/S0140-6736(18)32279-7
6. Zhao X, Shah D, Gandhi K, Wei W, Dwibedi N, Webster L, et al. Clinical, humanistic, and economic burden of osteoarthritis among noninstitutionalized adults in the united states. Osteoarthritis Cartilage. (2019) 27(11):1618–26. doi: 10.1016/j.joca.2019.07.002
7. Berenbaum F, Wallace IJ, Lieberman DE, Felson DT. Modern-day environmental factors in the pathogenesis of osteoarthritis. Nat Rev Rheumatol (2018) 14(11):674–81. doi: 10.1038/s41584-018-0073-x
8. O’Neill TW, McCabe PS, McBeth J. Update on the epidemiology, risk factors and disease outcomes of osteoarthritis. Best Pract Res Clin Rheumatol (2018) 32(2):312–26. doi: 10.1016/j.berh.2018.10.007
9. Berenbaum F. Deep phenotyping of osteoarthritis: a step forward. Ann Rheumatol Dis (2019) 78(1):3–5. doi: 10.1136/annrheumdis-2018-213864
10. Robinson WH, Lepus CM, Wang Q, Raghu H, Mao R, Lindstrom TM, et al. Low-grade inflammation as a key mediator of the pathogenesis of osteoarthritis. Nat Rev Rheumatol (2016) 12(10):580–92. doi: 10.1038/nrrheum.2016.136
11. Guido G, Ausenda G, Iascone V, Chisari E. Gut permeability and osteoarthritis, towards a mechanistic understanding of the pathogenesis: a systematic review. Ann Med (2021) 53(1):2380–90. doi: 10.1080/07853890.2021.2014557
12. Frank DN, St Amand AL, Feldman RA, Boedeker EC, Harpaz N, Pace NR. Molecular-phylogenetic characterization of microbial community imbalances in human inflammatory bowel diseases. Proc Natl Acad Sci USA (2007) 104(34):13780–5. doi: 10.1073/pnas.0706625104
13. Jandhyala SM, Talukdar R, Subramanyam C, Vuyyuru H, Sasikala M, Nageshwar Reddy D. Role of the normal gut microbiota. World J Gastroenterol (2015) 21(29):8787–803. doi: 10.3748/wjg.v21.i29.8787
14. Rinninella E, Raoul P, Cintoni M, Franceschi F, Miggiano GAD, Gasbarrini A, et al. What is the healthy gut microbiota composition? a changing ecosystem across age, environment, diet, and diseases. Microorganisms. (2019) 7(1):14. doi: 10.3390/microorganisms7010014
15. Lee WJ, Hase K. Gut microbiota-generated metabolites in animal health and disease. Nat Chem Biol (2014) 10(6):416–24. doi: 10.1038/nchembio.1535
16. Adak A, Khan MR. An insight into gut microbiota and its functionalities. Cell Mol Life Sci (2019) 76(3):473–93. doi: 10.1007/s00018-018-2943-4
17. Fan Y, Pedersen O. Gut microbiota in human metabolic health and disease. Nat Rev Microbiol (2021) 19(1):55–71. doi: 10.1038/s41579-020-0433-9
18. Mancabelli L, Milani C, Lugli GA, Turroni F, Ferrario C, van Sinderen D, et al. Meta-analysis of the human gut microbiome from urbanized and pre-agricultural populations. Environ Microbiol (2017) 19(4):1379–90. doi: 10.1111/1462-2920.13692
19. Sanna S, van Zuydam NR, Mahajan A, Kurilshikov A, Vich Vila A, Võsa U, et al. Causal relationships among the gut microbiome, short-chain fatty acids and metabolic diseases. Nat Genet (2019) 51(4):600–5. doi: 10.1038/s41588-019-0350-x
20. Wu J, Wang S, Zheng B, Qiu X, Wang H, Chen L. Modulation of gut microbiota to enhance effect of checkpoint inhibitor immunotherapy. Front Immunol (2021) 12:669150. doi: 10.3389/fimmu.2021.669150
21. Ulici V, Kelley KL, Azcarate-Peril MA, Cleveland RJ, Sartor RB, Schwartz TA, et al. Osteoarthritis induced by destabilization of the medial meniscus is reduced in germ-free mice. Osteoarthritis Cartilage. (2018) 26(8):1098–109. doi: 10.1016/j.joca.2018.05.016
22. Biver E, Berenbaum F, Valdes AM, Araujo de Carvalho I, Bindels LB, Brandi ML, et al. Gut microbiota and osteoarthritis management: An expert consensus of the European society for clinical and economic aspects of osteoporosis, osteoarthritis and musculoskeletal diseases (ESCEO). Ageing Res Rev (2019) 55:100946. doi: 10.1016/j.arr.2019.100946
23. Kalinkovich A, Livshits G. A cross talk between dysbiosis and gut-associated immune system governs the development of inflammatory arthropathies. Semin Arthritis Rheumatol (2019) 49(3):474–84. doi: 10.1016/j.semarthrit.2019.05.007
24. Page MJ, McKenzie JE, Bossuyt PM, Boutron I, Hoffmann TC, Mulrow CD, et al. The PRISMA 2020 statement: an updated guideline for reporting systematic reviews. BMJ (2021) 372:n71. doi: 10.1136/bmj.n71
25. Wang L, Sang B, Zheng Z. Risk of dementia or cognitive impairment in non-alcoholic fatty liver disease: A systematic review and meta-analysis. Front Aging Neurosci (2022) 14:985109. doi: 10.3389/fnagi.2022.985109
26. Laou E, Mavridis T, Papagiannakis N, Pais G, Chighine A, Chang J, et al. Blood biomarkers and metabolomic profiling for the early diagnosis of vancomycin-associated acute kidney injury: A systematic review and meta-analysis of experimental studies. J Pers. Med (2022) 12(9):1397. doi: 10.3390/jpm12091397
27. Wei J, Zhang C, Zhang Y, Zhang W, Doherty M, Yang T, et al. Association between gut microbiota and symptomatic hand osteoarthritis: data from the xiangya osteoarthritis study. Arthritis Rheumatol (2021) 73(9):1656–62. doi: 10.1002/art.41729
28. Ramasamy B, Magne F, Tripathy SK, Venugopal G, Mukherjee D, Balamurugan R. Association of gut microbiome and vitamin d deficiency in knee osteoarthritis patients: A pilot study. Nutrients. (2021) 13(4):1272. doi: 10.3390/nu13041272
29. Lee JY, Mannaa M, Kim Y, Kim J, Kim GT, Seo YS. Comparative analysis of fecal microbiota composition between rheumatoid arthritis and osteoarthritis patients. Genes (Basel). (2019) 10(10):748. doi: 10.3390/genes10100748
30. Chen J, Wang A, Wang Q. Dysbiosis of the gut microbiome is a risk factor for osteoarthritis in older female adults: a case control study. BMC Bioinf (2021) 22(1):2 99. doi: 10.1186/s12859-021-04199-0
31. Coulson S, Butt H, Vecchio P, Gramotnev H, Vitetta L. Green-lipped mussel extract (Perna canaliculus) and glucosamine sulphate in patients with knee osteoarthritis: therapeutic efficacy and effects on gastrointestinal microbiota profiles. Inflammopharmacology. (2013) 21(1):79–90. doi: 10.1007/s10787-012-0146-4
32. Boer CG, Radjabzadeh D, Medina-Gomez C, Garmaeva S, Schiphof D, Arp P, et al. Intestinal microbiome composition and its relation to joint pain and inflammation. Nat Commun (2019) 10(1):4881. doi: 10.1038/s41467-019-12873-4
33. Lyu JL, Wang TM, Chen YH, Chang ST, Wu MS, Lin YH, et al. Oral intake of streptococcus thermophilus improves knee osteoarthritis degeneration: A randomized, double-blind, placebo-controlled clinical study. Heliyon. (2020) 6(4):e03757. doi: 10.1016/j.heliyon.2020.e03757
34. Lei M, Guo C, Wang D, Zhang C, Hua L. The effect of probiotic lactobacillus casei shirota on knee osteoarthritis: a randomised double-blind, placebo-controlled clinical trial. Benef Microbes (2017) 8(5):697–703. doi: 10.3920/BM2016.0207
35. Wang Z, Zhu H, Jiang Q, Zhu YZ. The gut microbiome as non-invasive biomarkers for identifying overweight people at risk for osteoarthritis. Microb Pathog (2021) 157:104976. doi: 10.1016/j.micpath.2021.104976
36. Yu XH, Yang YQ, Cao RR, Bo L, Lei SF. The causal role of gut microbiota in development of osteoarthritis. Osteoarthritis Cartilage. (2021) 29(12):1741–50. doi: 10.1016/j.joca.2021.08.003
37. Yan Y, Yi X, Duan Y, Jiang B, Huang T, Inglis BM, et al. Alteration of the gut microbiota in rhesus monkey with spontaneous osteoarthritis. BMC Microbiol (2021) 21(1):328. doi: 10.1186/s12866-021-02390-0
38. Huang Z, Chen J, Li B, Zeng B, Chou CH, Zheng X, et al. Faecal microbiota transplantation from metabolically compromised human donors accelerates osteoarthritis in mice. Ann Rheum Dis (2020) 79(5):646–56. doi: 10.1136/annrheumdis-2019-216471
39. Schott EM, Farnsworth CW, Grier A, Lillis JA, Soniwala S, Dadourian GH, et al. Targeting the gut microbiome to treat the osteoarthritis of obesity. JCI Insight (2018) 3(8):e95997. doi: 10.1172/jci.insight.95997
40. Guss JD, Ziemian SN, Luna M, Sandoval TN, Holyoak DT, Guisado GG, et al. The effects of metabolic syndrome, obesity, and the gut microbiome on load-induced osteoarthritis. Osteoarthritis Cartilage (2019) 27(1):129–39. doi: 10.1016/j.joca.2018.07.020
41. Collins KH, Paul HA, Reimer RA, Seerattan RA, Hart DA, Herzog W. Relationship between inflammation, the gut microbiota, and metabolic osteoarthritis development: studies in a rat model. Osteoarthritis Cartilage. (2015) 23(11):1989–98. doi: 10.1016/j.joca.2015.03.014
42. Rios JL, Bomhof MR, Reimer RA, Hart DA, Collins KH, Herzog W. Protective effect of prebiotic and exercise intervention on knee health in a rat model of diet-induced obesity. Sci Rep (2019) 9(1):3893. doi: 10.1038/s41598-019-40601-x
43. So JS, Song MK, Kwon HK, Lee CG, Chae CS, Sahoo A, et al. Lactobacillus casei enhances type II collagen/glucosamine-mediated suppression of inflammatory responses in experimental osteoarthritis. Life Sci (2011) 88(7-8):358–66. doi: 10.1016/j.lfs.2010.12.013
44. Sim BY, Choi HJ, Kim MG, Jeong DG, Lee DG, Yoon JM, et al. Effects of ID-CBT5101 in preventing and alleviating osteoarthritis symptoms in a monosodium iodoacetate-induced rat model. J Microbiol Biotechnol (2018) 28(7):1199–208. doi: 10.4014/jmb.1803.03032
45. Kwon JY, Lee SH, Jhun J, Choi J, Jung K, Cho KH, et al. The combination of probiotic complex, rosavin, and zinc improves pain and cartilage destruction in an osteoarthritis rat model. J Med Food (2018) 21(4):364–71. doi: 10.1089/jmf.2017.4034
46. Collins KH, Schwartz DJ, Lenz KL, Harris CA, Guilak F. Taxonomic changes in the gut microbiota are associated with cartilage damage independent of adiposity, high fat diet, and joint injury. Sci Rep (2021) 11(1):14560. doi: 10.1038/s41598-021-94125-4
47. Li K, Liu A, Zong W, Dai L, Liu Y, Luo R, et al. Moderate exercise ameliorates osteoarthritis by reducing lipopolysaccharides from gut microbiota in mice. Saudi J Biol Sci (2021) 28(1):40–9. doi: 10.1016/j.sjbs.2020.08.027
48. Guan Z, Jia J, Zhang C, Sun T, Zhang W, Yuan W, et al. Gut microbiome dysbiosis alleviates the progression of osteoarthritis in mice. Clin Sci (Lond). (2020) 134(23):3159–74. doi: 10.1042/CS20201224
49. Luna M, Guss JD, Vasquez-Bolanos LS, Alepuz AJ, Dornevil S, Strong J, et al. Obesity and load-induced posttraumatic osteoarthritis in the absence of fracture or surgical trauma. J Orthop Res (2021) 39(5):1007–16. doi: 10.1002/jor.24799
50. Song W, Liu YG, Dong XH, Song C, Bai YY, Hu PP, et al. Lactobacillus M5 prevents osteoarthritis induced by a high-fat diet in mice. J Funct Foods. (2020) 72(11):104039. doi: 10.1016/j.jff.2020.104039
51. Chen Y, Lv J, Jia Y, Wang R, Zhang Z, Liu J, et al. Effect of moxibustion on the intestinal flora of rats with knee osteoarthritis induced by monosodium iodoacetate. Evid Based Complement Alternat Med (2020) 2020:3196427. doi: 10.1155/2020/3196427
52. Jhun J, Cho KH, Lee DH, Kwon JY, Woo JS, Kim J, et al. Oral administration of lactobacillus rhamnosus ameliorates the progression of osteoarthritis by inhibiting joint pain and inflammation. Cells. (2021) 10(5):1057. doi: 10.3390/cells10051057
53. Lin YY, Chen NF, Yang SN, Jean YH, Kuo HM, Chen PC, et al. Effects of streptococcus thermophilus on anterior cruciate ligament transection-induced early osteoarthritis in rats. Exp Ther Med (2021) 21(3):222. doi: 10.3892/etm.2021.9653
54. Henrotin Y, Patrier S, Pralus A, Roche M, Nivoliez A. Protective actions of oral administration of bifidobacterium longum CBi0703 in spontaneous osteoarthritis in dunkin Hartley Guinea pig model. Cartilage (2021) 13(2_suppl):1204S–13S. doi: 10.1177/1947603519841674
55. Cintio M, Scarsella E, Sgorlon S, Sandri M, Stefanon B. Gut microbiome of healthy and arthritic dogs. Vet Sci (2020) 7(3):92. doi: 10.3390/vetsci7030092
56. Wallace IJ, Bendele AM, Riew G, Frank EH, Hung HH, Holowka NB, et al. Physical inactivity and knee osteoarthritis in guinea pigs. Osteoarthritis Cartilage. (2019) 27(11):1721–8. doi: 10.1016/j.joca.2019.07.005
57. Zhao Y, Chen B, Li S, Yang L, Zhu D, Wang Y, et al. Detection and characterization of bacterial nucleic acids in culture-negative synovial tissue and fluid samples from rheumatoid arthritis or osteoarthritis patients. Sci Rep (2018) 8(1):14305. doi: 10.1038/s41598-018-32675-w
58. Dunn CM, Velasco C, Rivas A, Andrews M, Garman C, Jacob PB, et al. Identification of cartilage microbial DNA signatures and associations with knee and hip osteoarthritis. Arthritis Rheumatol (2020) 72(7):1111–22. doi: 10.1002/art.41210
59. Sophia Fox AJ, Bedi A, Rodeo SA. The basic science of articular cartilage: structure, composition, and function. Sports Health (2009) 1(6):461–8. doi: 10.1177/1941738109350438
60. Mow VC, Ratcliffe A, Poole AR. Cartilage and diarthrodial joints as paradigms for hierarchical materials and structures. Biomaterials. (1992) 13(2):67–97. doi: 10.1016/0142-9612(92)90001-5
61. Sterner B, Harms M, Wöll S, Weigandt M, Windbergs M, Lehr CM. The effect of polymer size and charge of molecules on permeation through synovial membrane and accumulation in hyaline articular cartilage. Eur J Pharm Biopharm. (2016) 101:126–36. doi: 10.1016/j.ejpb.2016.02.004
62. Arkill KP, Winlove CP. Solute transport in the deep and calcified zones of articular cartilage. Osteoarthritis Cartilage. (2008) 16(6):708–14. doi: 10.1016/j.joca.2007.10.001
63. Gabner S, Häusler G, Böck P. Vascular canals in permanent hyaline cartilage: Development, corrosion of nonmineralized cartilage matrix, and removal of matrix degradation products. Anat Rec (Hoboken). (2017) 300(6):1067–82. doi: 10.1002/ar.23537
64. Wormstrand B, Østevik L, Ekman S, Olstad K. Septic Arthritis/Osteomyelitis may lead to osteochondrosis-like lesions in foals. Vet Pathol (2018) 55(5):693–702. doi: 10.1177/0300985818777786
65. Qiu B, Al K, Pena-Diaz AM, Athwal GS, Drosdowech D, Faber KJ, et al. Cutibacterium acnes and the shoulder microbiome. J Shoulder Elbow Surg (2018) 27(10):1734–9. doi: 10.1016/j.jse.2018.04.019
66. Novince CM, Whittow CR, Aartun JD, Hathaway JD, Poulides N, Chavez MB, et al. Commensal gut microbiota immunomodulatory actions in bone marrow and liver have catabolic effects on skeletal homeostasis in health. Sci Rep (2017) 7(1):5747. doi: 10.1038/s41598-017-06126-x
67. Mor G, Kwon JY. Trophoblast-microbiome interaction: a new paradigm on immune regulation. Am J Obstet Gynecol. (2015) 213(4 Suppl):S131–7. doi: 10.1016/j.ajog.2015.06.039
68. Lorenzo D, GianVincenzo Z, Carlo Luca R, Karan G, Jorge V, Roberto M, et al. Oral-gut microbiota and arthritis: Is there an evidence-based axis? J Clin Med (2019) 8(10). doi: 10.3390/jcm8101753
69. Tsai JC, Casteneda G, Lee A, Dereschuk K, Li WT, Chakladar J, et al. Identification and characterization of the intra-articular microbiome in the osteoarthritic knee. Int J Mol Sci (2020) 21(22):8618. doi: 10.3390/ijms21228618
70. Szychlinska MA, Di Rosa M, Castorina A, Mobasheri A, Musumeci G. A correlation between intestinal microbiota dysbiosis and osteoarthritis. Heliyon. (2019) 5(1):e01134. doi: 10.1016/j.heliyon.2019.e01134
71. Kulp A, Kuehn MJ. Biological functions and biogenesis of secreted bacterial outer membrane vesicles. Annu Rev Microbiol (2010) 64:163–84. doi: 10.1146/annurev.micro.091208.073413
72. Kaparakis-Liaskos M, Ferrero RL. Immune modulation by bacterial outer membrane vesicles. Nat Rev Immunol (2015) 15(6):375–87. doi: 10.1038/nri3837
73. Arend WP, Firestein GS. Pre-rheumatoid arthritis: predisposition and transition to clinical synovitis. Nat Rev Rheumatol (2012) 8(10):573–86. doi: 10.1038/nrrheum.2012.134
74. Huang ZY, Stabler T, Pei FX, Kraus VB. Both systemic and local lipopolysaccharide (LPS) burden are associated with knee OA severity and inflammation. Osteoarthritis Cartilage. (2016) 24(10):1769–75. doi: 10.1016/j.joca.2016.05.008
75. Liu Y, Ding W, Wang HL, Dai LL, Zong WH, Wang YZ, et al. Gut microbiota and obesity-associated osteoarthritis. Osteoarthritis Cartilage. (2019) 27(9):1257–65. doi: 10.1016/j.joca.2019.05.009
76. McPhee JB, Schertzer JD. Immunometabolism of obesity and diabetes: microbiota link compartmentalized immunity in the gut to metabolic tissue inflammation. Clin Sci (Lond). (2015) 129(12):1083–96. doi: 10.1042/CS20150431
77. Nagpal R, Kumar M, Yadav AK, Hemalatha R, Yadav H, Marotta. F, et al. Gut microbiota in health and disease: an overview focused on metabolic inflammation. Benef Microbes (2016) 7(2):181–94. doi: 10.3920/bm2015.0062
78. Huang Z, Kraus VB. Does lipopolysaccharide-mediated inflammation have a role in OA? Nat Rev Rheumatol (2016) 12(2):123–9. doi: 10.1038/nrrheum.2015.158
79. Clarke TB, Davis KM, Lysenko ES, Zhou AY, Yu Y, Weiser JN. Recognition of peptidoglycan from the microbiota by Nod1 enhances systemic innate immunity. Nat Med (2010) 16(2):228–31. doi: 10.1038/nm.2087
80. Hernandez CJ. The microbiome and bone and joint disease. Curr Rheumatol Rep (2017) 19(12):77. doi: 10.1007/s11926-017-0705-1
81. Nair A, Kanda V, Bush-Joseph C, Verma N, Chubinskaya S, Mikecz K, et al. Synovial fluid from patients with early osteoarthritis modulates fibroblast-like synoviocyte responses to toll-like receptor 4 and toll-like receptor 2 ligands via soluble CD14. Arthritis Rheumatol (2012) 64(7):2268–77. doi: 10.1002/art.34495
82. Kapoor M, Martel-Pelletier J, Lajeunesse D, Pelletier JP, Fahmi H. Role of proinflammatory cytokines in the pathophysiology of osteoarthritis. Nat Rev Rheumatol (2011) 7(1):33–42. doi: 10.1038/nrrheum.2010.196
83. Zhang M, Egan B, Wang J. Epigenetic mechanisms underlying the aberrant catabolic and anabolic activities of osteoarthritic chondrocytes. Int J Biochem Cell Biol (2015) 67:101–9. doi: 10.1016/j.biocel.2015.04.019
84. Wehr P, Purvis H, Law SC, Thomas R. Dendritic cells, T cells and their interaction in rheumatoid arthritis. Clin Exp Immunol (2019) 196(1):12–27. doi: 10.1111/cei.13256
85. Li YS, Luo W, Zhu SA, Lei GH. T Cells in osteoarthritis: Alterations and beyond. Front Immunol (2017) 8:356. doi: 10.3389/fimmu.2017.00356
86. Woodell-May JE, Sommerfeld SD. Role of inflammation and the immune system in the progression of osteoarthritis. J Orthop Res (2020) 38(2):253–7. doi: 10.1002/jor.24457
Keywords: gut microbiome, osteoarthritis, cartilage, inflammation, immune response
Citation: Liu S, Li G, Xu H, Wang Q, Wei Y, Yang Q, Xiong A, Yu F, Weng J and Zeng H (2023) “Cross-talk” between gut microbiome dysbiosis and osteoarthritis progression: a systematic review. Front. Immunol. 14:1150572. doi: 10.3389/fimmu.2023.1150572
Received: 26 January 2023; Accepted: 27 March 2023;
Published: 25 April 2023.
Edited by:
Tetyana Falalyeyeva, Taras Shevchenko National University of Kyiv, UkraineReviewed by:
Marco Invernizzi, University of Eastern Piedmont, ItalyPei Shang, Mayo Clinic, United States
Copyright © 2023 Liu, Li, Xu, Wang, Wei, Yang, Xiong, Yu, Weng and Zeng. This is an open-access article distributed under the terms of the Creative Commons Attribution License (CC BY). The use, distribution or reproduction in other forums is permitted, provided the original author(s) and the copyright owner(s) are credited and that the original publication in this journal is cited, in accordance with accepted academic practice. No use, distribution or reproduction is permitted which does not comply with these terms.
*Correspondence: Fei Yu, b3NjYXJmeXVAMTYzLmNvbQ==; Jian Weng, andlbmdAcGt1LmVkdS5jbg==; Hui Zeng, emVuZ2h1aUBwa3VzemguY29t
†These authors have contributed equally to this work and share first authorship