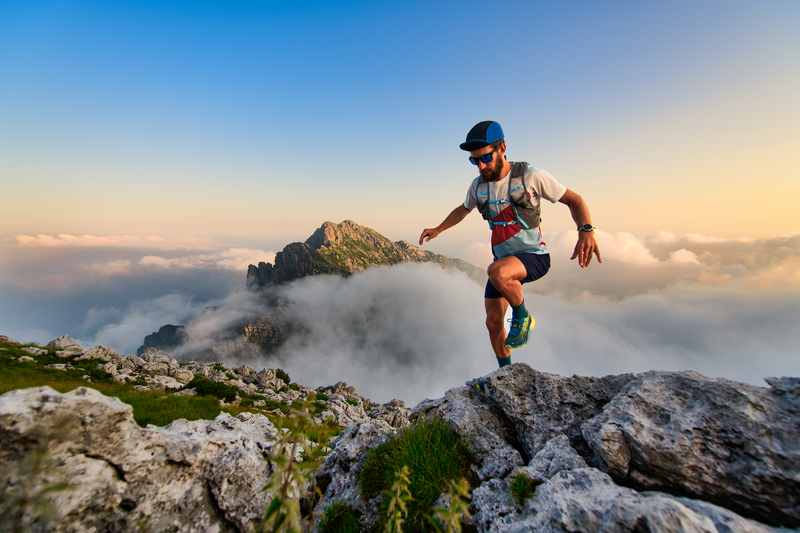
95% of researchers rate our articles as excellent or good
Learn more about the work of our research integrity team to safeguard the quality of each article we publish.
Find out more
REVIEW article
Front. Immunol. , 19 April 2023
Sec. Autoimmune and Autoinflammatory Disorders : Autoimmune Disorders
Volume 14 - 2023 | https://doi.org/10.3389/fimmu.2023.1148359
This article is part of the Research Topic Cutaneous Immunology View all 8 articles
Alopecia areata (AA) is a non-scarring hair loss disorder caused by autoimmunity. The immune collapse of the hair follicle, where interferon-gamma (IFN-γ) and CD8+ T cells accumulate, is a key factor in AA. However, the exact functional mechanism remains unclear. Therefore, AA treatment has poor efficacy maintenance and high relapse rate after drug withdrawal. Recent studies show that immune-related cells and molecules affect AA. These cells communicate through autocrine and paracrine signals. Various cytokines, chemokines and growth factors mediate this crosstalk. In addition, adipose-derived stem cells (ADSCs), gut microbiota, hair follicle melanocytes, non-coding RNAs and specific regulatory factors have crucial roles in intercellular communication without a clear cause, suggesting potential new targets for AA therapy. This review discusses the latest research on the possible pathogenesis and therapeutic targets of AA.
Alopecia areata (AA) is a non-scarring hair loss disorder that affects about 2% of the population, regardless of race, gender, or age (1, 2). The symptoms and signs of AA vary depending on the severity of the condition, from patchy hair loss to diffuse hair involvement on the scalp or the whole body (3, 4). Most AA patients experience unpredictable relapses and remissions (5). Studies show that it lowers the quality of life of patients and may lead to emotional disorders such as depression and anxiety (6–8). Further associations between AA and certain inflammatory and metabolic diseases have been observed, increasing the probability of developing them (9, 10); however, causality remains to be established and the exact pathogenesis of AA is still to be discovered. The pathogenesis of AA is driven by immune factors, as the current mainstream view suggests (11). Genetic factors may also contribute. Variants in PRDX5 and STX17, genes expressed in the hair follicle, can impair its immune privilege and induce autoimmune responses leading to hair loss (12). A recent study linked mutations in KRT82 to the loss of hair follicle immune privilege and CD8+ T cell infiltration (13). These mutations may contribute to the loss of immune privilege of the hair follicle, which may be a contributing factor of AA development (14, 15). Current conventional treatments and immunobiological therapies do not provide the durable effect or permanent reversal of AA (16, 17). Therefore, it is essential to understand AA pathogenesis comprehensively and identify new therapeutic targets. CD8+ T cells have been considered the culprit in AA (18), but with the development of cellular and molecular biology, it was discovered that they are not the only driver of AA. In addition, studies have revealed that numerous unconventional, such as gamma delta (γδ) T cells, interact with CD8+ T cells and influence AA pathogenesis (19, 20), as shown in Figure 1A. Simultaneously, ADSCs, gut microbiota, hair follicle melanocytes, and non-coding RNA may contribute to the development of AA. However, the causal relationship between these factors and AA is unclear. This review will provide an overview of the relevant studies.
Figure 1 Immune responses involved by associated cells around AA hair follicles. (A) presents that the cells involved in the immune response of AA hair follicles in anagen primarily include γδ T cells, TRM cells, Treg cells, Tol DCs, iNKT 10 cells, ADSCs, and melanocytes. When immune privilege collapses and self-antigens are exposed, AA is produced and has various effects, including activating iNKT10 cells, γδ T cells, and TRM cells in the vascular plexus around the hair follicle and suppressing Tol DCs, Treg cells, ADSCs, and melanocytes. Tol DC and Treg cells can mutually activate. (B) indicates that TCR on γδ T cells can bind to CD1d and MICA/B in hair follicle epithelial cells, and NKG2D on its surface can bind to CD8+ T cells, release IFN-γ, and activate CD8+ T cells. TRM can release CXCL9 and IFN-γ and can express CD69 and CD103. Treg cells can express FOXP3, CD25, and CD4, produce IL-2, IL-10, and TGF-β, suppress CD8+ T cells, inhibit the autoantigen production on hair follicle epithelial cells, and activate Tol DCs. Tol DC can release retinoic acid, TGF-β, and IL-10, promote Treg cells and inhibit CD8+ T cells. iNKT 10 cells can release IL-10 to inhibit CD8+ T cells, and ADSCs can release IL-4 and IL-5 to inhibit CD8+ T cells. Hair follicle melanocytes can bind to CD8+ T cells via melanin-associated antigens and be attacked by T cells. Hair follicle epithelial cells can bind to CD8+ T cells and activate CD8+ T cells to release INF-γ. Created with Biorender.com.
According to different receptor types, T cells can be classified into two subtypes: αβ T and γδ T cells (21). γδ T cells constitute fewer than 5% of the population and are uncommon in the T cell compartment of the blood and secondary lymphoid organs. Still, they are abundant in the skin epithelial tissues (22). Antigen-presenting cells (APCs) detect antigens with the help of the major histocompatibility complex (MHC) molecules on their surfaces. Unlike most human T cells that are MHC-restricted, γδ T cells do not need MHC activation to recognize antigens, as they are major players in innate immune responses along with inflammasomes and INF γ (23–25). Additionally, γδ T cells produce cytokines in the periphery and can be recruited to the lesion where they accumulate (26). This process occurs more rapidly than αβ T cell activation. The ability of γδ T cells to get activated without specific T cell receptor (TCR) ligands renders them potent early inflammasome inducers (27, 28). Depending on their cytokine production, γδ T could be classified into three subsets: IL-17 producers, IFN-γ producers, and IL-4 producers (29). High levels of IFN-γ can trigger skin-related autoimmune disorders via its expression. A recent study revealed that γδ T cells could also express natural killer group 2D (NKG2D) (30). AA patients’ hair follicles highly express CD1d and human major histocompatibility complex class I chain-related gene A (MICA), which can bind to MHC-I and NKG2D receptors and promote AA occurrence (31, 32), as shown in Figure 1B.
The researchers compared the number and activation state of T cells in the lesional and non-lesional scalps of AA patients to the scalps of healthy controls. Uchida et al. (33) indicated that the number of γδ T cells was significantly higher in the scalp of AA patients than in healthy controls. γδ T cells promote inflammation, resulting in high NKG2D and IFN-γ expressions. They discovered that γδ T cells increased in both AA non-lesional and lesional scalps, primarily in and around the hair follicle, presenting that γδ T cells play a role in the early development of AA and are agonists for the onset of AA, thereby facilitating the identification of future treatment targets. Additionally, their research discovered (34) that human γδ T cells have a physiological stress sentinel role, wherein their hyperactivity can promote autoimmunity against stressed HF overexpressing CD1d and MICA, which is essential for the early onset of AA. Therefore, inhibiting γδ T cells in the hair follicle could be a proper neoadjuvant method for treating acute AA.
Research on γδT cells has shown promise in the treatment of various diseases. Targeting immune checkpoints around tumors can improve the function of γδT cells within the tumor microenvironment, leading to enhanced proliferation, activation and cytotoxicity (35). Recent studies have also identified CFTR, a regulator of chloride and ion channels primarily studied in epithelial cells and expressed on γδ T cells, as a negative regulator of IFN-γ production and antitumor immunity (36). Both genetic overexpression and pharmacological activation of CFTR can reduce IFN-γ release by peripheral γδ T cells. Thus, targeting γδ T cells around hair follicles may hold significant potential for treating of AA.
TRM cells are long-lived lymphocytes that remain in tissues, particularly in the skin, making it an ideal location for rapidly responding to infection (37). They are essential immunological components that provide the organism with an immediate and precise response to pathogen reinfection or antigen re-exposure of peripheral tissues and are involved in early inflammation (38, 39). TRM cells can continuously monitor and inspect tissues. When suspected antigens and pathogens are discovered, TRM cells are activated (40, 41). It can mediate the immunological responses of other immune cells and tissue (42). Additionally, it may produce cytokines, such as IFN-γ (43), and release cytotoxic substances, such as granzyme B and perforin (44). TRM cells are crucial in tissue differentiation and the early period of an inflammatory immune response. Tissue-specific signals influence its differentiation (45). TRM cells are predominantly generated by T cell-mediated immunological responses (46). Simultaneously, TRM cells can recirculate memory T cells through the blood and lymphoid organs (47). However, the number of TRM cells decreases during recirculation. Recent research has demonstrated that TRM cells can also be created during autoimmunity. TRM-mediated autoimmunity is extremely devastating because autoantigens cannot be eliminated and continue to stimulate self-reactive TRM cells (48).
NKG2D+ and CD8+ T cells are responsible for the advancement of AA, inducing a collapse of immunological response by activating T cells and dendritic cells and causing perifollicular T cells to produce IFN-γ, CXCL9, and CXCL10 (5), as shown in Figure 1B. Duca et al. (49) discovered that an AA scalp had more CD103+CD69+ TRM cells than a non-lesional scalp via TCR sequencing of CD8 T cells. Notably, Janus kinase inhibitors (JAKi)-treated AA patients are susceptible to relapse during the off-drug interval. A study revealed a rise in CD103+CD69+ TRM cells in patients who relapsed (50). This indicates that TRM cells may be associated with AA and might be a significant aspect of understanding why relapse arises in JAKi-based therapy for AA, making it a good target for more research.
Recent studies have highlighted the potential role of reducing perifollicular TRM cells in the treatment of AA. Fatty acid-binding proteins 4 and 5 (FABP4 and FABP5) have been shown to play critical roles in maintaining the lifespan and function of TRM cells. Deficiency in these proteins impairs the uptake of exogenous free fatty acids by TRM cells and reduces their long-term survival in vivo, without affecting central memory T cells in lymph nodes (51). Additionally, research has demonstrated that tissue-resident memory T helper 17 (TRM17) cell proliferation and retention in skin requires interleukin-23 (IL-23), and blocking IL-23 can inhibit TRM cell proliferation. This method has already been applied to the treatment of psoriasis and holds potential for future use in AA (52).
Treg cells are immunosuppressive cells that inhibit the immune response of other cells and serve as the principal regulators of self-tolerance (53). Its absence or aberrant function results in the onset of autoimmune disorders (54). Treg cells are a subset of T cells with a cellular profile that express FOXP3, CD25, and CD4 (55). Dendritic cells, specifically tolerogenic dendritic cells, may limit adaptive immunity activation by promoting Treg cell differentiation into suppressive subtypes (56). According to a study, transferring Treg cells from skin-draining lymph nodes of mice with normal hair to AA-affected ones reduced the development of generalized AA and site-specific alopecia in the latter but did not promote hair regrowth (57). The study discovered that the efficacy of IL-2 in treating of AA in mice is limited. However, several studies have demonstrated that modest doses of IL-2 injected subcutaneously into AA patients recruit CD4+CD25+FOXP3+ Treg and induce effective hair regeneration (58). This may be because the AA process in humans and mice differs. There is evidence that Treg cells can directly influence hair follicular circulation in AA mice models. Hamed et al. discovered that a lack of Treg cells around the hair bulb leads to a reduced transition from telogen to the anagen phase, reduced hair regeneration, and reduced follicular stem cell differentiation, with an increase in the proportion of the telogen phase (59). A study exhibited that CD80CD86 double-negative C57BL/6 mice are prone to decreased CD4+FOXP3+ Treg cells, causing spontaneous AA (60). Additionally, studies have confirmed a decrease in FOXP3+CD39+ Treg cells in the peripheral blood of AA patients (61). However, studies have revealed that TGF-β secreted by Treg cells is higher in scalp and blood samples of AA patients than in healthy controls, and IL-17 is also elevated, as shown in Figure 1B. The researchers speculate that Treg cells are more inclined to differentiate into IL-17a-producing cells (62). Phase 2a randomized clinical research revealed dupilumab’s efficacy in treating of AA patients (63–65). It has been claimed that dupilumab stimulates hair development by enhancing Treg function, which is decreased in typical Th2 inflammation (66, 67). Although the mechanism by which Treg cells inhibit AA remains unclear, in the AA mouse model, stimulation of myeloid-derived suppressor cells exosomes (MDSC-Exo) to increase Treg cells in vivo can reduce lymphocyte proliferation and activity around the hair follicle and promote hair growth (68), indicating that Treg cells are effective therapeutic targets.
Dendritic cells are well-known immunologically presenting cells, and immature dendritic cells predominantly produce immunomodulatory factors to mediate central and peripheral immune responses (69). Tol DCs are a subtype of dendritic cells that promote Treg cell activity, reduce Th1 and Th2 inflammation, and inhibit effector T cells (70). Tol DCs may acquire tolerance to various immune cells implicated in autoimmunity through contact-dependent interactions and pleiotropic cytokines and metabolite production that may treat autoimmune illnesses (71, 72). Therapies based on the injection of Tol DCs exhibited promising results as alternatives to immunomodulators in some chronic inflammatory diseases and organ transplantation (73, 74) because Tol DCs can reduce autoimmune responses without spreading widespread immunosuppression. Tol DCs may be generated for therapeutic applications by adopting dendritic cell vaccination protocols. According to a survey (75), dendritic cells were cultured with drug resistance-inducing medicines (rapamycin or dexamethasone) and cytokines (transforming growth factor-beta (TGF-β) and interleukin-10 (IL-10)) to develop a drug-resistant phenotype in vitro, as shown in Figure 1B. It is efficient to reintroduce these “induced” Tol DCs into the body. Epigenetic indicators, like DNA methylation and histone modifications, after translation, may also be used to influence cell phenotype and function, either directly or through various studies highlighting the significance of numerous epigenetic mechanisms in the establishment of DC tolerance. Tol DC is generated during in vitro differentiation of human monocytes (76, 77). Prostaglandin E2 (PGE2) has altered the ability of Tol DC to suppress CD8+ T cell proliferation in vitro by inducing DNA Methyltransferase 3A (DNMT3A) upregulation, thereby mediating immunogenic gene methylation and silencing (78). Depending on the induction method, these cells exhibit an intermediate phenotype with traits derived from immature and activated adult dendritic cells, including alterations in migratory activity, anti-inflammatory cytokine production, and the kind of T cell-induced tolerance response (79, 80). These DCs must be loaded with disease-specific autoantigens to develop therapeutic DCs that target specific autoimmune disorders (81). It is now being utilized in phase I and II clinical studies for illnesses, such as type I diabetes and multiple sclerosis, where it has demonstrated encouraging outcomes (82).
Given the abundance of Tol DCs within the skin, epigenetic modifications and therapies targeting epigenetic mechanisms may offer novel avenues for modulating Tol DC adaptability in AA. Additionally, further investigation into hair follicle-specific antigens present in AA, such as keratins and melanocyte antigens, may prove fruitful. However, it should be noted that no studies to date have reported on the use of Tol DCs loaded with autoantigens for the treatment of AA.
The association between AA and CD8+NKG2D+ effector T cell drive has been well-documented. However, recent studies have revealed that iNKT cells express NKG2D receptors and rapidly release cytokines in response to early stimulation, possibly making them necessary for Th cell polarization by creating a cytokine environment for conventional Th cells (83). iNKT cells are CD1d-restricted, lipid-reactive T cells with predominantly immunomodulatory properties to produce cytokines and chemokines and modulate other immune cells (84). Additionally, iNKT cells can provide cytotoxic immune responses via cytotoxic granules and death receptor pathways (85). In arthritis, some iNKT are promoted while others are suppressed. These findings suggest that iNKT-mediated regulation in autoimmune diseases is dependent, at least in part, on their ability to regulate macrophage inflammatory profiles (86).
According to Th cell nomenclature, iNKT cells are categorized as iNKT1, iNKT2, iNKT10, and iNKT17 based on cytokine (87). In chronic inflammation, iNKT10 cells, primarily in adipose tissue, express the transcription factor E4BP4 and release IL-10, as shown in Figure 1B. Chen et al. activated adipose tissue iNKT10 cells by injecting GalCer, a traditional activator of iNKT cells, subcutaneously into animals fed a high-fat diet, boosting M2 macrophage polarisation and alleviating chronic inflammation in obese adipose tissue (88). Ghraieb et al. demonstrated that the agonist-GalCer could block AA in iNKT10 cells using a humanized mouse model of AA, allowing their expansion to produce IL-10 and activate memory iNKT cells, thereby demonstrating the protective effect of iNKT cells against AA in vivo (89). Targeting iNKT cells as a treatment for autoimmune diseases such as type 1 diabetes has been shown to have the potential to help determine the state or severity of an immunological disease (90, 91).
ADSCs, mesenchymal stem cells (MSCs) in the stromal vascular fraction (SVF) of adipose tissue, are more abundant in adipose tissue, including subcutaneous adipose tissue, than in MSCs of other origins and are an integral part of regenerative medicine (92). These growth factors influence neighboring cell’s activity and play a crucial role in neovascularization (93). Therefore, injecting autologous fat into the scalp before hair transplantation can enhance blood vessel distribution in the scalp and stimulate hair development (94). In addition to their potential applications in tissue repair and regeneration, ADSCs have substantial immunomodulatory properties, such as regulating inflammatory or autoimmune diseases, such as arthritis, colitis, and other autoimmune disorders (95–97). Several studies have demonstrated that their internal extracellular vesicles (EVs) play an essential role. Jafarinia et al. targeted human ADSCs, ADSCs-EVs, and placebo via tail vein injection in a mouse model of induced experimental autoimmune encephalomyelitis. They demonstrated that intravenous ADSC-EV enhanced the anti-inflammatory capacity of T cells by decreasing their proliferative capacity and leukocyte infiltration (98). A recent study revealed that in an in vitro model of neuropathy, ADSCs boost anti-inflammatory effects and IL-4 and IL-5 expressions, thereby dampening the immune system (99), as shown in Figure 1B. ADSCs have regulated intracellular signaling pathways in adjacent cells and protect the organism by secreting substantial quantities of cytokines, growth factors, and antioxidant substances into their milieu (100). However, the exact mechanism remains unknown, and numerous studies suggest that ADSCs are crucial for preventing cell death caused by oxidative stress (101). ADSCs can improve the anti-aging treatment of senescent cells and animal models of early aging by accelerating the autophagy of mitochondria, eliminating intracellular reactive oxygen species (ROS), and improving mitochondrial quality (102).
Additionally, ADSCs treatment can improve hair loss by promoting mitochondrial division. Anderi et al. established the efficacy and safety of the treatment by injecting 4–4.7×106 autologous ADSVCs into the scalps of AA patients. After three and six months of ADSVCs treatment, all patients had hair regrowth, enhanced hair growth, and a decreased tension test, demonstrating the treatment’s efficacy and safety (103). A randomized, double-blind, controlled clinical trial using adipose‐derived stem cell constituent extract (ADSC-CE) topical solution has the potential to serve as an alternative therapy method for hair regrowth in AGA patients, enhancing hair density and thickness while preserving appropriate treatment safety (104). It cannot be denied that it could prevent AA, although its exact mechanism of action is unknown.
In clinical practice, most AA patients demonstrate an unexpected phenomenon: white hair in the lesions of balding patients does not fall out. However, almost all black hair has vanished. This is particularly true for early-stage AA sufferers (105). AA patients exhibit a significant reduction in melanocytes (106). Since melanocyte production and melanin synthesis in mature hair follicles occur primarily during the anagen phase, a reduction in melanocytes in the hair follicle may be associated with the early onset of AA.
Bertolini et al. examined lesion sections from balding patients using immunohistochemistry. They discovered that CD8+ T cells recognized and attacked the autoantigen presented by follicular melanocytes, providing further evidence that autoantigens are present in follicular melanocytes of balding patients (107). AA has been associated with melanocyte-specific antigens that have yet to be identified. In healthy populations, melanin antigens, such as tyrosinase, MAGE-A3, Melan-A/MART-1, gp100, and NY-ESO-1 may elicit an immune response against CD8+ T cells, but they also protect against the formation of melanoma and are persistently overexpressed in humans (108), as shown in Figure 1B. In vitiligo, dendritic cells transfer antigenic proteins from normal or stressed melanocytes, such as gp100, which are then recognized by invading T lymphocytes and cause melanocyte death. However, it is unknown what causes melanocytes to perish. The most commonly recognized explanation is that oxidative stress induces an immune response that results in melanocyte death. Melanocyte death may occur via apoptosis, autophagy, autophagic cell death, or ferroptosis when oxidative stress causes high levels of ROS, thereby leading to molecular and organelle dysfunction (109). Kang et al. discovered that oxidative stress could cause apoptosis, which is exacerbated by abnormal calcium accumulation in the mitochondria, and that melanocytes experience mitochondria-dependent apoptosis when oxidative stress increases the expression of transient receptor potential cation channel subfamily M member 2 (TRPM2), allowing a higher calcium influx and consequent cell death (110). Oxidative stress has been connected with melanocyte apoptosis. A meta-analysis indicated that the oxidative marker levels were significantly higher in severe AA patients than in mild to moderate AA patients (111). Multiple studies suggest that oxidative stress may play a substantial role in the pathogenesis of age-associated macular degeneration and melanocyte death (112–114). The AA treatment may include using antioxidant supplements to alleviate oxidative stress or antioxidants in combination with other therapeutic strategies to boost melanocyte oxidative capacity. Several studies (115–117) have demonstrated that antioxidant supplements, such as Yucca, polyunsaturated fatty acids, and carotenoids, preserve melanocytes and improve the therapeutic efficacy of phototherapy in vitiligo patients.
The gut microbiota is essential for maintaining healthy immune function, regulating Treg cell activity, and monitoring the processing and presentation of antigens (118). Immune-related problems, especially autoimmune diseases, may develop if the gut microbial ecology is imbalanced due to poor food, excessive use of antibiotics, or incompetent breastfeeding (119). Gut microorganisms can regulate specific immune cells and growth factors. Certain probiotics, such as bifidobacteria and segmented filamentous bacteria, can control T-helper 17 cells (120). Bacteroides fragilis surface polysaccharide A binds to toll-like receptor 2 on dendritic cells, inducing T reg cells to generate the anti-inflammatory cytokine IL-10 and promoting immunological tolerance (121). Clostridium spp., especially those belonging to clusters IV, XIVa, and XVIII, stimulate CD4+FOXP3+ Treg cells by producing short-chain fatty acids (SCFAs) (122). Although no microbial medications currently target a specific disease, microbial-based methods for preventing or curing inflammatory autoimmune disorders provide new potential. Most of the metabolites made by microbes in the intestine are SCFAs (123). They are formed when intestinal flora consumes and ferments soluble fibers and oligosaccharides. The intestinal epithelial cells readily absorb them. They are an essential energy source for the bacteria and cells that live in and line the gut. SCFAs control chromatin’s structure in T lymphocytes’ nucleus, making gene products more active (124), as shown in Figure 2.
Figure 2 Gut microbiota and AA. The dysregulation of gut microbiota can reduce the SCFA, stimulating the release of TNF-α, IFN-γ, and IL-1β, while suppressing Treg cells and the release of IL-10 and TGF-β, thereby fostering the AA process. Created with Biorender.com.
Binding of SCFAs to G protein-coupled receptor 43 (GPR43) has been shown to be essential for the resolution of inflammatory responses (125). While SCFAs exert systemic effects following absorption, local application may be more relevant in the context of AA. Indeed, enemas containing SCFAs have demonstrated efficacy in a subset of patients with distal ulcerative colitis (126). Furthermore, topical application of SCFAs has been shown to promote induction of tissue plasminogen activator in airway epithelial cells via binding to G protein-coupled receptors, reducing fibrin deposition and alleviating chronic sinusitis (127). These findings suggest that topical application of SCFAs to scalp hair follicles may hold promise for the treatment of AA.
MicroRNAs (miRNAs) and long non-coding RNAs (lncRNAs) are the most common non-coding RNAs. Various potential miRNAs, lncRNAs, and regulatory proteins have been identified in autoimmune disorders (128). Upregulated miRNAs include miR-210 (129), miR-1246 (130), miR-17 (131), miR-34a (132), miR-101 (133), miR-27b (133), miR-142-3p (134), miR-142-5p (134), and miR-150 (134), while downregulated miRNAs include miR-30b (135), miR-103 (133), miR-2355-3p (133), and miR-186-5p (134) that stimulate their target protein expressions, as shown in Table 1. This mismatched miRNA-target interaction causes abnormal T cell activation (134), melanosome autophagy (137), and angiogenesis inhibition (138), thereby damaging hair follicles and contributing to AA. According to the bioinformatics results of Sheng et al., NONHSAT011665-RPS26-has-miR-186-5p may play a role in the control of AA (139). Qi et al. demonstrated that novel treatment for AA might lie in RP11-251G23.5 and RP11-231E19, which may play an important role in the etiology of AA through modulation of the cytokine-cytokine receptor interaction pathway (140). Eomesodermin (Eomes) may play a major role in AA by regulating immune cell infiltration and keratin-forming cell activity, indicating that they are a possible therapeutic target for AA (141). Several JAJ-STAT pathway components downstream of the cytokine-containing chain (known to increase the activity and survival of IFN-producing cytotoxic T cells) were upregulated in human and mouse AA skin. Systemic injection of JAK inhibitors reduced AA development in a mouse model (142, 143). Several open-label phase II clinical studies have demonstrated that oral JAK inhibitor treatment significantly promotes hair regrowth and improves AA with assured safety and dependability (144, 145).
Recent studies have shed light on potential mechanisms for inhibiting the occurrence and development of AA. Activation of immune tolerance cells such as Treg cells and Tol DCs, along with inhibition of immune enhancement cells such as γδ T cells, TRM cells, and iNKT cells may play a role in preventing AA. Additionally, ADSCs and SCFAs from Gut microbiome can improve the inflammatory manifestations of AA, and patients may benefit more with its topical application. Reducing exposure of hair follicle melanocytes’ antigens to CD8+ T cells may also be effective; loading melanocyte-associated antigens through Tol DCs could be a potential approach. Furthermore, noncoding RNAs and regulatory proteins can release small molecules or cytokines such as growth factors or their receptors, opening up new avenues for AA treatment.
WX, SW, BX, and XS contributed significantly to the analysis and manuscript preparation; WX wrote the manuscript; SW, BX, and XS helped perform the analysis with constructive discussions. All authors contributed to the article and approved the submitted version.
This work was supported by Basic Public Welfare Research Project of Zhejiang (No.LY23H110001); Science and Technology Major Project of Zhejiang Province and the State Administration of Traditional Chinese Medicine (No. GZY-ZJ-KJ-2023035); Health Science and Technology Major Project of Hangzhou (No. Z20220040).
The authors declare that the research was conducted in the absence of any commercial or financial relationships that could be construed as a potential conflict of interest.
All claims expressed in this article are solely those of the authors and do not necessarily represent those of their affiliated organizations, or those of the publisher, the editors and the reviewers. Any product that may be evaluated in this article, or claim that may be made by its manufacturer, is not guaranteed or endorsed by the publisher.
1. Strazzulla LC, Wang EHC, Avila L, Lo Sicco K, Brinster N, Christiano AM, et al. Alopecia areata: disease characteristics, clinical evaluation, and new perspectives on pathogenesis. J Am Acad Dermatol (2018) 78:1–12. doi: 10.1016/j.jaad.2017.04.1141
2. Lee HH, Gwillim E, Patel KR, Hua T, Rastogi S, Ibler E, et al. Epidemiology of alopecia areata, ophiasis, totalis, and universalis: a systematic review and meta-analysis. J Am Acad Dermatol (2020) 82:675–82. doi: 10.1016/j.jaad.2019.08.032
3. Meah N, Wall D, York K, Bhoyrul B, Bokhari L, Asz-Sigall D, et al. The alopecia areata consensus of experts (ACE) study part II: results of an international expert opinion on diagnosis and laboratory evaluation for alopecia areata. J Am Acad Dermatol (2021) 84:1594–601. doi: 10.1016/j.jaad.2020.09.028
4. King BA, Mesinkovska NA, Craiglow B, Kindred C, Ko J, McMichael A, et al. Development of the alopecia areata scale for clinical use: results of an academic-industry collaborative effort. J Am Acad Dermatol (2022) 86:359–64. doi: 10.1016/j.jaad.2021.08.043
5. Lee S, Kim BJ, Lee YB, Lee W-S. Hair regrowth outcomes of contact immunotherapy for patients with alopecia areata: a systematic review and meta-analysis. JAMA Dermatol (2018) 154:1145–51. doi: 10.1001/jamadermatol.2018.2312
6. Okhovat J-P, Marks DH, Manatis-Lornell A, Hagigeorges D, Locascio JJ, Senna MM. Association between alopecia areata, anxiety, and depression: a systematic review and meta-analysis. J Am Acad Dermatol (2019), S0190-9622(19)30890-4. doi: 10.1016/j.jaad.2019.05.086
7. Toussi A, Barton VR, Le ST, Agbai ON, Kiuru M. Psychosocial and psychiatric comorbidities and health-related quality of life in alopecia areata: a systematic review. J Am Acad Dermatol (2021) 85:162–75. doi: 10.1016/j.jaad.2020.06.047
8. Dai Y-X, Tai Y-H, Chen C-C, Chang Y-T, Chen T-J, Chen M-H. Bidirectional association between alopecia areata and major depressive disorder among probands and unaffected siblings: a nationwide population-based study. J Am Acad Dermatol (2020) 82:1131–7. doi: 10.1016/j.jaad.2019.11.064
9. Conic RRZ, Chu S, Tamashunas NL, Damiani G, Bergfeld W. Prevalence of cardiac and metabolic diseases among patients with alopecia areata. J Eur Acad Dermatol Venereol (2021) 35:e128–9. doi: 10.1111/jdv.16864
10. Conic RZ, Tamashunas NL, Damiani G, Fabbrocini G, Cantelli M, Young Dermatologists Italian Network, et al. Comorbidities in pediatric alopecia areata. J Eur Acad Dermatol Venereol (2020) 34:2898–901. doi: 10.1111/jdv.16727
11. Shin J-W, Kang T, Lee JS, Kang MJ, Huh C-H, Kim M-S, et al. Time-dependent risk of acute myocardial infarction in patients with alopecia areata in Korea. JAMA Dermatol (2020) 156:763–71. doi: 10.1001/jamadermatol.2020.1133
12. Petukhova L, Duvic M, Hordinsky M, Norris D, Price V, Shimomura Y, et al. Genome-wide association study in alopecia areata implicates both innate and adaptive immunity. Nature (2010) 466:113–7. doi: 10.1038/nature09114
13. Erjavec SO, Gelfman S, Abdelaziz AR, Lee EY, Monga I, Alkelai A, et al. Whole exome sequencing in alopecia areata identifies rare variants in KRT82. Nat Commun (2022) 13:800. doi: 10.1038/s41467-022-28343-3
14. Zhou C, Li X, Wang C, Zhang J. Alopecia areata: an update on etiopathogenesis, diagnosis, and management. Clin Rev Allergy Immunol (2021) 61:403–23. doi: 10.1007/s12016-021-08883-0
15. Gilhar A, Laufer-Britva R, Keren A, Paus R. Frontiers in alopecia areata pathobiology research. J Allergy Clin Immunol (2019) 144:1478–89. doi: 10.1016/j.jaci.2019.08.035
16. Messenger A, Harries M. Baricitinib in alopecia areata. N Engl J Med (2022) 386:1751–2. doi: 10.1056/NEJMe2203440
17. Strazzulla LC, Wang EHC, Avila L, Lo Sicco K, Brinster N, Christiano AM, et al. Alopecia areata: an appraisal of new treatment approaches and overview of current therapies. J Am Acad Dermatol (2018) 78:15–24. doi: 10.1016/j.jaad.2017.04.1142
18. Xing L, Dai Z, Jabbari A, Cerise JE, Higgins CA, Gong W, et al. Alopecia areata is driven by cytotoxic T lymphocytes and is reversed by JAK inhibition. Nat Med (2014) 20:1043–9. doi: 10.1038/nm.3645
19. Gela A, Murphy M, Rodo M, Hadley K, Hanekom WA, Boom WH, et al. Effects of BCG vaccination on donor unrestricted T cells in two prospective cohort studies. EBioMedicine (2022) 76:103839. doi: 10.1016/j.ebiom.2022.103839
20. Genardi S, Visvabharathy L, Cao L, Morgun E, Cui Y, Qi C, et al. Type II natural killer T cells contribute to protection against systemic methicillin-resistant staphylococcus aureus infection. Front Immunol (2020) 11:610010. doi: 10.3389/fimmu.2020.610010
21. Gebru YA, Gupta H, Kim HS, Eom JA, Kwon GH, Park E, et al. T Cell subsets and natural killer cells in the pathogenesis of nonalcoholic fatty liver disease. Int J Mol Sci (2021) 22:12190. doi: 10.3390/ijms222212190
22. Handgretinger R, Schilbach K. The potential role of γδ T cells after allogeneic HCT for leukemia. Blood (2018) 131:1063–72. doi: 10.1182/blood-2017-08-752162
23. Bafor EE, Valencia JC, Young HA. Double negative T regulatory cells: an emerging paradigm shift in reproductive immune tolerance? Front Immunol (2022) 13:886645. doi: 10.3389/fimmu.2022.886645
24. Velikkakam T, Gollob KJ, Dutra WO. Double-negative T cells: setting the stage for disease control or progression. Immunology (2022) 165:371–85. doi: 10.1111/imm.13441
25. Hashimoto K, Yamada Y, Sekiguchi K, Mori S, Matsumoto T. NLRP3 inflammasome activation contributes to development of alopecia areata in C3H/HeJ mice. Exp Dermatol (2022) 31:133–42. doi: 10.1111/exd.14432
26. Ribot JC, Lopes N, Silva-Santos B. γδ T cells in tissue physiology and surveillance. Nat Rev Immunol (2021) 21:221–32. doi: 10.1038/s41577-020-00452-4
27. Castro CD, Boughter CT, Broughton AE, Ramesh A, Adams EJ. Diversity in recognition and function of human γδ T cells. Immunol Rev (2020) 298:134–52. doi: 10.1111/imr.12930
28. Herrmann T, Karunakaran MM, Fichtner AS. A glance over the fence: using phylogeny and species comparison for a better understanding of antigen recognition by human γδ T-cells. Immunol Rev (2020) 298:218–36. doi: 10.1111/imr.12919
29. Narayan K, Sylvia KE, Malhotra N, Yin CC, Martens G, Vallerskog T, et al. Intrathymic programming of effector fates in three molecularly distinct γδ T cell subtypes. Nat Immunol (2012) 13:511–8. doi: 10.1038/ni.2247
30. Kabelitz D, Serrano R, Kouakanou L, Peters C, Kalyan S. Cancer immunotherapy with γδ T cells: many paths ahead of us. Cell Mol Immunol (2020) 17:925–39. doi: 10.1038/s41423-020-0504-x
31. Rajabi F, Drake LA, Senna MM, Rezaei N. Alopecia areata: a review of disease pathogenesis. Br J Dermatol (2018) 179:1033–48. doi: 10.1111/bjd.16808
32. Gálvez NMS, Bohmwald K, Pacheco GA, Andrade CA, Carreño LJ, Kalergis AM. Type I natural killer T cells as key regulators of the immune response to infectious diseases. Clin Microbiol Rev (2021) 34:e00232–20. doi: 10.1128/CMR.00232-20
33. Uchida Y, Gherardini J, Schulte-Mecklenbeck A, Alam M, Chéret J, Rossi A, et al. Pro-inflammatory Vδ1+T-cells infiltrates are present in and around the hair bulbs of non-lesional and lesional alopecia areata hair follicles. J Dermatol Sci (2020) 100:129–38. doi: 10.1016/j.jdermsci.2020.09.001
34. Uchida Y, Gherardini J, Pappelbaum K, Chéret J, Schulte-Mecklenbeck A, Gross CC, et al. Resident human dermal γδT-cells operate as stress-sentinels: lessons from the hair follicle. J Autoimmun (2021) 124:102711. doi: 10.1016/j.jaut.2021.102711
35. Gao Z, Bai Y, Lin A, Jiang A, Zhou C, Cheng Q, et al. Gamma delta T-cell-based immune checkpoint therapy: attractive candidate for antitumor treatment. Mol Cancer (2023) 22:31. doi: 10.1186/s12943-023-01722-0
36. Duan Y, Li G, Xu M, Qi X, Deng M, Lin X, et al. CFTR is a negative regulator of γδ T cell IFN-γ production and antitumor immunity. Cell Mol Immunol (2021) 18:1934–44. doi: 10.1038/s41423-020-0499-3
37. Scott P. Long-lived skin-resident memory T cells contribute to concomitant immunity in cutaneous leishmaniasis. Cold Spring Harb Perspect Biol (2020) 12:a038059. doi: 10.1101/cshperspect.a038059
38. Yuan R, Yu J, Jiao Z, Li J, Wu F, Yan R, et al. The roles of tissue-resident memory T cells in lung diseases. Front Immunol (2021) 12:710375. doi: 10.3389/fimmu.2021.710375
39. Tokura Y, Phadungsaksawasdi P, Kurihara K, Fujiyama T, Honda T. Pathophysiology of skin resident memory T cells. Front Immunol (2020) 11:618897. doi: 10.3389/fimmu.2020.618897
40. Masopust D, Soerens AG. Tissue-resident T cells and other resident leukocytes. Annu Rev Immunol (2019) 37:521–46. doi: 10.1146/annurev-immunol-042617-053214
41. Chen L, Wei B, Di D-L. A narrative review of tissue-resident memory T cells and their role in immune surveillance and COVID-19. Eur Rev Med Pharmacol Sci (2022) 26:4486–96. doi: 10.26355/eurrev_202206_29088
42. Byrne A, Savas P, Sant S, Li R, Virassamy B, Luen SJ, et al. Tissue-resident memory T cells in breast cancer control and immunotherapy responses. Nat Rev Clin Oncol (2020) 17:341–8. doi: 10.1038/s41571-020-0333-y
43. Frisoli ML, Essien K, Harris JE. Vitiligo: mechanisms of pathogenesis and treatment. Annu Rev Immunol (2020) 38:621–48. doi: 10.1146/annurev-immunol-100919-023531
44. Behr FM, Chuwonpad A, Stark R, van Gisbergen KPJM. Armed and ready: transcriptional regulation of tissue-resident memory CD8 T cells. Front Immunol (2018) 9:1770. doi: 10.3389/fimmu.2018.01770
45. Parga-Vidal L, van Aalderen MC, Stark R, van Gisbergen KPJM. Tissue-resident memory T cells in the urogenital tract. Nat Rev Nephrol (2022) 18:209–23. doi: 10.1038/s41581-021-00525-0
46. Barreto de Albuquerque J, Mueller C, Gungor B. Tissue-resident T cells in chronic relapsing-remitting intestinal disorders. Cells (2021) 10:1882. doi: 10.3390/cells10081882
47. Ho AW, Kupper TS. T Cells and the skin: from protective immunity to inflammatory skin disorders. Nat Rev Immunol (2019) 19:490–502. doi: 10.1038/s41577-019-0162-3
48. Molodtsov A, Turk MJ. Tissue resident CD8 memory T cell responses in cancer and autoimmunity. Front Immunol (2018) 9:2810. doi: 10.3389/fimmu.2018.02810
49. Del Duca E, Ruano Ruiz J, Pavel AB, Sanyal RD, Song T, Gay-Mimbrera J, et al. Frontal fibrosing alopecia shows robust T helper 1 and janus kinase 3 skewing. Br J Dermatol (2020) 183:1083–93. doi: 10.1111/bjd.19040
50. Ryan GE, Harris JE, Richmond JM. Resident memory T cells in autoimmune skin diseases. Front Immunol (2021) 12:652191. doi: 10.3389/fimmu.2021.652191
51. Pan Y, Tian T, Park CO, Lofftus SY, Mei S, Liu X, et al. Survival of tissue-resident memory T cells requires exogenous lipid uptake and metabolism. Nature (2017) 543:252–6. doi: 10.1038/nature21379
52. Whitley SK, Li M, Kashem SW, Hirai T, Igyártó BZ, Knizner K, et al. Local IL-23 is required for proliferation and retention of skin-resident memory TH17 cells. Sci Immunol (2022) 7:eabq3254. doi: 10.1126/sciimmunol.abq3254
53. Lucca LE, Dominguez-Villar M. Modulation of regulatory T cell function and stability by co-inhibitory receptors. Nat Rev Immunol (2020) 20:680–93. doi: 10.1038/s41577-020-0296-3
54. Wing JB, Tanaka A, Sakaguchi S. Human FOXP3+ regulatory T cell heterogeneity and function in autoimmunity and cancer. Immunity (2019) 50:302–16. doi: 10.1016/j.immuni.2019.01.020
55. Martin-Moreno PL, Tripathi S, Chandraker A. Regulatory T cells and kidney transplantation. Clin J Am Soc Nephrol (2018) 13:1760–4. doi: 10.2215/CJN.01750218
56. Tang R, Acharya N, Subramanian A, Purohit V, Tabaka M, Hou Y, et al. Tim-3 adapter protein Bat3 acts as an endogenous regulator of tolerogenic dendritic cell function. Sci Immunol (2022) 7:eabm0631. doi: 10.1126/sciimmunol.abm0631
57. McElwee KJ, Freyschmidt-Paul P, Hoffmann R, Kissling S, Hummel S, Vitacolonna M, et al. Transfer of CD8(+) cells induces localized hair loss whereas CD4(+)/CD25(-) cells promote systemic alopecia areata and CD4(+)/CD25(+) cells blockade disease onset in the C3H/HeJ mouse model. J Invest Dermatol (2005) 124:947–57. doi: 10.1111/j.0022-202X.2005.23692.x
58. Castela E, Le Duff F, Butori C, Ticchioni M, Hofman P, Bahadoran P, et al. Effects of low-dose recombinant interleukin 2 to promote T-regulatory cells in alopecia areata. JAMA Dermatol (2014) 150:748–51. doi: 10.1001/jamadermatol.2014.504
59. Hamed FN, Åstrand A, Bertolini M, Rossi A, Maleki-Dizaji A, Messenger AG, et al. Alopecia areata patients show deficiency of FOXP3+CD39+ T regulatory cells and clonotypic restriction of treg TCRβ-chain, which highlights the immunopathological aspect of the disease. PloS One (2019) 14:e0210308. doi: 10.1371/journal.pone.0210308
60. Bae J-H, Hwang W-S, Jang Y-J, Lee Y-H, Jang D-E, Kim J-S, et al. CD80CD86 deficiency disrupts regulatory CD4+FoxP3+T cell homoeostasis and induces autoimmune-like alopecia. Exp Dermatol (2017) 26:1053–9. doi: 10.1111/exd.13371
61. Salcido-Ochoa F, Tsang J, Tam P, Falk K, Rotzschke O. Regulatory T cells in transplantation: does extracellular adenosine triphosphate metabolism through CD39 play a crucial role? Transplant Rev (Orlando) (2010) 24:52–66. doi: 10.1016/j.trre.2010.01.002
62. Loh S-H, Moon H-N, Lew B-L, Sim W-Y. Role of T helper 17 cells and T regulatory cells in alopecia areata: comparison of lesion and serum cytokine between controls and patients. J Eur Acad Dermatol Venereol (2018) 32:1028–33. doi: 10.1111/jdv.14775
63. Guttman-Yassky E, Renert-Yuval Y, Bares J, Chima M, Hawkes JE, Gilleaudeau P, et al. Phase 2a randomized clinical trial of dupilumab (anti-IL-4Rα) for alopecia areata patients. Allergy (2022) 77:897–906. doi: 10.1111/all.15071
64. Renert-Yuval Y, Pavel AB, Del Duca E, Facheris P, Pagan AD, Bose S, et al. Scalp biomarkers during dupilumab treatment support Th2 pathway pathogenicity in alopecia areata. Allergy (2023) 78:1047–59. doi: 10.1111/all.15561
65. Renert-Yuval Y, Guttman-Yassky E. Reply to: “Phase 2a randomized clinical trial of dupilumab (anti-IL-4Rα) for alopecia areata patients”. Allergy (2022) 77:2269–70. doi: 10.1111/all.15285
66. Marks DH, Mesinkovska N, Senna MM. Cause or cure? review of dupilumab and alopecia areata. J Am Acad Dermatol (2023) 88:651–3. doi: 10.1016/j.jaad.2019.06.010
67. Maher E, Martiniuk F, Levis W. Correspondence to: phase 2a randomized clinical trial of dupilumab (anti-IL-4Rα) for alopecia areata patients. Allergy (2022) 77:1073–4. doi: 10.1111/all.15185
68. Frontiers Production Office. Erratum: immunoregulatory effects of myeloid-derived suppressor cell exosomes in mouse model of autoimmune alopecia areata. Front Immunol (2021) 12:832980. doi: 10.3389/fimmu.2021.832980
69. Munson PV, Adamik J, Butterfield LH. Immunomodulatory impact of α-fetoprotein. Trends Immunol (2022) 43:438–48. doi: 10.1016/j.it.2022.04.001
70. Jonuleit H, Schmitt E, Steinbrink K, Enk AH. Dendritic cells as a tool to induce anergic and regulatory T cells. Trends Immunol (2001) 22:394–400. doi: 10.1016/s1471-4906(01)01952-4
71. Fan X, Men R, Huang C, Shen M, Wang T, Ghnewa Y, et al. Critical roles of conventional dendritic cells in autoimmune hepatitis via autophagy regulation. Cell Death Dis (2020) 11:23. doi: 10.1038/s41419-019-2217-6
72. Phillips BE, Garciafigueroa Y, Engman C, Liu W, Wang Y, Lakomy RJ, et al. Arrest in the progression of type 1 diabetes at the mid-stage of insulitic autoimmunity using an autoantigen-decorated all-trans retinoic acid and transforming growth factor beta-1 single microparticle formulation. Front Immunol (2021) 12:586220. doi: 10.3389/fimmu.2021.586220
73. Suuring M, Moreau A. Regulatory macrophages and tolerogenic dendritic cells in myeloid regulatory cell-based therapies. Int J Mol Sci (2021) 22:7970. doi: 10.3390/ijms22157970
74. Hossein-Khannazer N, Torabi S, Hosseinzadeh R, Shahrokh S, Asadzadeh Aghdaei H, Memarnejadian A, et al. Novel cell-based therapies in inflammatory bowel diseases: the established concept, promising results. Hum Cell (2021) 34:1289–300. doi: 10.1007/s13577-021-00560-w
75. Zhang X, Chen X, Guo Y, Gao G, Wang D, Wu Y, et al. Dual gate-controlled therapeutics for overcoming bacterium-induced drug resistance and potentiating cancer immunotherapy. Angewandte Chemie Int Edition (2021) 60:14013–21. doi: 10.1002/anie.202102059
76. Morante-Palacios O, Fondelli F, Ballestar E, Martínez-Cáceres EM. Tolerogenic dendritic cells in autoimmunity and inflammatory diseases. Trends Immunol (2021) 42:59–75. doi: 10.1016/j.it.2020.11.001
77. Carty F, Corbett JM, Cunha JPMCM, Reading JL, Tree TIM, Ting AE, et al. Multipotent adult progenitor cells suppress T cell activation in In Vivo models of homeostatic proliferation in a prostaglandin E2-dependent manner. Front Immunol (2018) 9:645. doi: 10.3389/fimmu.2018.00645
78. Ching MM, Reader J, Fulton AM. Eicosanoids in cancer: prostaglandin E2 receptor 4 in cancer therapeutics and immunotherapy. Front Pharmacol (2020) 11:819. doi: 10.3389/fphar.2020.00819
79. Odobasic D, Oudin V, Ito K, Gan P-Y, Kitching AR, Holdsworth SR. Tolerogenic dendritic cells attenuate experimental autoimmune antimyeloperoxidase glomerulonephritis. J Am Soc Nephrol (2019) 30:2140–57. doi: 10.1681/ASN.2019030236
80. Benito-Villalvilla C, Pérez-Diego M, Angelina A, Kisand K, Rebane A, Subiza JL, et al. Allergoid-mannan conjugates reprogram monocytes into tolerogenic dendritic cells via epigenetic and metabolic rewiring. J Allergy Clin Immunol (2022) 149:212–222.e9. doi: 10.1016/j.jaci.2021.06.012
81. Cifuentes-Rius A, Desai A, Yuen D, Johnston APR, Voelcker NH. Inducing immune tolerance with dendritic cell-targeting nanomedicines. Nat Nanotechnol (2021) 16:37–46. doi: 10.1038/s41565-020-00810-2
82. Raposo CJ, Cserny JD, Serena G, Chow JN, Cho P, Liu H, et al. Engineered RBCs encapsulating antigen induce multi-modal antigen-specific tolerance and protect against type 1 diabetes. Front Immunol (2022) 13:869669. doi: 10.3389/fimmu.2022.869669
83. Cruz MS, Loureiro JP, Oliveira MJ, Macedo MF. The iNKT cell-macrophage axis in homeostasis and disease. Int J Mol Sci (2022) 23:1640. doi: 10.3390/ijms23031640
84. Tuomela K, Ambrose AR, Davis DM. Escaping death: how cancer cells and infected cells resist cell-mediated cytotoxicity. Front Immunol (2022) 13:867098. doi: 10.3389/fimmu.2022.867098
85. Yu Z, Liu W, He Y, Sun M, Yu J, Jiao X, et al. HLA-A2.1-restricted ECM1-derived epitope LA through DC cross-activation priming CD8+ T and NK cells: a novel therapeutic tumour vaccine. J Hematol Oncol (2021) 14:71. doi: 10.1186/s13045-021-01081-7
86. Zarobkiewicz MK, Morawska I, Michalski A, Roliński J, Bojarska-Junak A. NKT and NKT-like cells in autoimmune neuroinflammatory diseases-multiple sclerosis, myasthenia gravis and Guillain-barre syndrome. Int J Mol Sci (2021) 22:9520. doi: 10.3390/ijms22179520
87. Yarosz EL, Chang C-H, Kumar A. Metabolism in invariant natural killer T cells: an overview. Immunometabolism (2021) 3:e210010. doi: 10.20900/immunometab20210010
88. Chen D, Zhao H, Gao X, Chen S, Liu H, Zhang J, et al. Subcutaneous administration of α-GalCer activates iNKT10 cells to promote M2 macrophage polarization and ameliorates chronic inflammation of obese adipose tissue. Int Immunopharmacol (2019) 77:105948. doi: 10.1016/j.intimp.2019.105948
89. Ghraieb A, Keren A, Ginzburg A, Ullmann Y, Schrum AG, Paus R, et al. iNKT cells ameliorate human autoimmunity: lessons from alopecia areata. J Autoimmun (2018) 91:61–72. doi: 10.1016/j.jaut.2018.04.001
90. Erkers T, Xie BJ, Kenyon LJ, Smith B, Rieck M, Jensen KP, et al. High-parametric evaluation of human invariant natural killer T cells to delineate heterogeneity in allo- and autoimmunity. Blood (2020) 135:814–25. doi: 10.1182/blood.2019001903
91. Van Kaer L, Wu L. Therapeutic potential of invariant natural killer T cells in autoimmunity. Front Immunol (2018) 9:519. doi: 10.3389/fimmu.2018.00519
92. Bacakova L, Zarubova J, Travnickova M, Musilkova J, Pajorova J, Slepicka P, et al. Stem cells: their source, potency and use in regenerative therapies with focus on adipose-derived stem cells - a review. Biotechnol Adv (2018) 36:1111–26. doi: 10.1016/j.biotechadv.2018.03.011
93. Li X, Xie X, Lian W, Shi R, Han S, Zhang H, et al. Exosomes from adipose-derived stem cells overexpressing Nrf2 accelerate cutaneous wound healing by promoting vascularization in a diabetic foot ulcer rat model. Exp Mol Med (2018) 50:1–14. doi: 10.1038/s12276-018-0058-5
94. Kim SJ, Kim MJ, Lee YJ, Lee JC, Kim JH, Kim DH, et al. Innovative method of alopecia treatment by autologous adipose-derived SVF. Stem Cell Res Ther (2021) 12:486. doi: 10.1186/s13287-021-02557-6
95. Huldani H, Abdalkareem Jasim S, Olegovich Bokov D, Abdelbasset WK, Nader Shalaby M, Thangavelu L, et al. Application of extracellular vesicles derived from mesenchymal stem cells as potential therapeutic tools in autoimmune and rheumatic diseases. Int Immunopharmacol (2022) 106:108634. doi: 10.1016/j.intimp.2022.108634
96. Lu L, Dai C, Zhang Z, Du H, Li S, Ye P, et al. Treatment of knee osteoarthritis with intra-articular injection of autologous adipose-derived mesenchymal progenitor cells: a prospective, randomized, double-blind, active-controlled, phase IIb clinical trial. Stem Cell Res Ther (2019) 10:143. doi: 10.1186/s13287-019-1248-3
97. Yuan Y, Ni S, Zhuge A, Li L, Li B. Adipose-derived mesenchymal stem cells reprogram M1 macrophage metabolism via PHD2/HIF-1α pathway in colitis mice. Front Immunol (2022) 13:859806. doi: 10.3389/fimmu.2022.859806
98. Jafarinia M, Alsahebfosoul F, Salehi H, Eskandari N, Azimzadeh M, Mahmoodi M, et al. Therapeutic effects of extracellular vesicles from human adipose-derived mesenchymal stem cells on chronic experimental autoimmune encephalomyelitis. J Cell Physiol (2020) 235:8779–90. doi: 10.1002/jcp.29721
99. Angeloni C, Gatti M, Prata C, Hrelia S, Maraldi T. Role of mesenchymal stem cells in counteracting oxidative stress–related neurodegeneration. Int J Mol Sci (2020) 21:3299. doi: 10.3390/ijms21093299
100. Chen X, Yan L, Guo Z, Chen Z, Chen Y, Li M, et al. Adipose-derived mesenchymal stem cells promote the survival of fat grafts via crosstalk between the Nrf2 and TLR4 pathways. Cell Death Dis (2016) 7:e2369. doi: 10.1038/cddis.2016.261
101. Nosalski R, Guzik TJ. Perivascular adipose tissue inflammation in vascular disease. Br J Pharmacol (2017) 174:3496–513. doi: 10.1111/bph.13705
102. Li T, Zhou L, Fan M, Chen Z, Yan L, Lu H, et al. Human umbilical cord-derived mesenchymal stem cells ameliorate skin aging of nude mice through autophagy-mediated anti-senescent mechanism. Stem Cell Rev Rep (2022) 18:2088–103. doi: 10.1007/s12015-022-10418-9
103. Anderi R, Makdissy N, Azar A, Rizk F, Hamade A. Cellular therapy with human autologous adipose-derived adult cells of stromal vascular fraction for alopecia areata. Stem Cell Res Ther (2018) 9:141. doi: 10.1186/s13287-018-0889-y
104. Tak YJ, Lee SY, Cho AR, Kim YS. A randomized, double-blind, vehicle-controlled clinical study of hair regeneration using adipose-derived stem cell constituent extract in androgenetic alopecia. Stem Cells Transl Med (2020) 9:839–49. doi: 10.1002/sctm.19-0410
105. Xie B, Sun J, Song X. Hair follicle melanocytes initiate autoimmunity in alopecia areata: a trigger point. Clin Rev Allergy Immunol (2022) 63:417–30. doi: 10.1007/s12016-022-08954-w
106. Speeckaert R, Belpaire A, Speeckaert M, van Geel N. The delicate relation between melanocytes and skin immunity: a game of hide and seek. Pigment Cell Melanoma Res (2022) 35:392–407. doi: 10.1111/pcmr.13037
107. Bertolini M, Rossi A, Paus R. Cover image: are melanocyte-associated peptides the elusive autoantigens in alopecia areata? Br J Dermatol (2017) 176:1106. doi: 10.1111/bjd.15288
108. Przybyla A, Lehmann AA, Zhang T, Mackiewicz J, Galus Ł, Kirchenbaum GA, et al. Functional T cell reactivity to melanocyte antigens is lost during the progression of malignant melanoma, but is restored by immunization. Cancers (Basel) (2021) 13:E223. doi: 10.3390/cancers13020223
109. Chen J, Li S, Li C. Mechanisms of melanocyte death in vitiligo. Med Res Rev (2021) 41:1138–66. doi: 10.1002/med.21754
110. Kang P, Zhang W, Chen X, Yi X, Song P, Chang Y, et al. TRPM2 mediates mitochondria-dependent apoptosis of melanocytes under oxidative stress. Free Radic Biol Med (2018) 126:259–68. doi: 10.1016/j.freeradbiomed.2018.08.022
111. Acharya P, Mathur MC. Oxidative stress in alopecia areata: a systematic review and meta-analysis. Int J Dermatol (2020) 59:434–40. doi: 10.1111/ijd.14753
112. Nandi A, Yan L-J, Jana CK, Das N. Role of catalase in oxidative stress- and age-associated degenerative diseases. Oxid Med Cell Longev (2019) 2019:9613090. doi: 10.1155/2019/9613090
113. Kang HY, Lee JW, Papaccio F, Bellei B, Picardo M. Alterations of the pigmentation system in the aging process. Pigment Cell Melanoma Res (2021) 34:800–13. doi: 10.1111/pcmr.12994
114. Kim JH, Kim M-M. Corrigendum to “The relationship between melanin production and lipofuscin formation in tyrosinase gene knockout melanocytes using CRISPR/Cas9 system”. Life Sci (2022) 289:120225. doi: 10.1016/j.lfs.2021.120225
115. Farjadmand F, Karimpour-Razkenari E, Nabavi SM, Ardekani MRS, Saeedi M. Plant polyphenols: natural and potent UV-protective agents for the prevention and treatment of skin disorders. Mini Rev Med Chem (2021) 21:576–85. doi: 10.2174/1389557520666201109121246
116. Balić A, Vlašić D, Žužul K, Marinović B, Bukvić Mokos Z. Omega-3 versus omega-6 polyunsaturated fatty acids in the prevention and treatment of inflammatory skin diseases. Int J Mol Sci (2020) 21:E741. doi: 10.3390/ijms21030741
117. Shakhbazova A, Wu H, Chambers CJ, Sivamani RK. A systematic review of nutrition, supplement, and herbal-based adjunctive therapies for vitiligo. J Altern Complement Med (2021) 27:294–311. doi: 10.1089/acm.2020.0292
118. Aghamajidi A, Maleki Vareki S. The effect of the gut microbiota on systemic and anti-tumor immunity and response to systemic therapy against cancer. Cancers (Basel) (2022) 14:3563. doi: 10.3390/cancers14153563
119. Fan L, Chen J, Pan L, Xin X, Geng B, Yang L, et al. Alterations of gut microbiome, metabolome and lipidome in takayasu arteritis. Arthritis Rheumatol (2023) 75:266–78. doi: 10.1002/art.42331
120. Beurel E, Medina-Rodriguez EM, Jope RS. Targeting the adaptive immune system in depression: focus on T helper 17 cells. Pharmacol Rev (2022) 74:373–86. doi: 10.1124/pharmrev.120.000256
121. Ramakrishna C, Kujawski M, Chu H, Li L, Mazmanian SK, Cantin EM. Bacteroides fragilis polysaccharide a induces IL-10 secreting b and T cells that prevent viral encephalitis. Nat Commun (2019) 10:2153. doi: 10.1038/s41467-019-09884-6
122. Quraishi MN, Shaheen W, Oo YH, Iqbal TH. Immunological mechanisms underpinning faecal microbiota transplantation for the treatment of inflammatory bowel disease. Clin Exp Immunol (2020) 199:24–38. doi: 10.1111/cei.13397
123. van der Hee B, Wells JM. Microbial regulation of host physiology by short-chain fatty acids. Trends Microbiol (2021) 29:700–12. doi: 10.1016/j.tim.2021.02.001
124. Kanda N, Hoashi T, Saeki H. The defect in regulatory T cells in psoriasis and therapeutic approaches. J Clin Med (2021) 10:3880. doi: 10.3390/jcm10173880
125. Maslowski KM, Vieira AT, Ng A, Kranich J, Sierro F, Yu D, et al. Regulation of inflammatory responses by gut microbiota and chemoattractant receptor GPR43. Nature (2009) 461:1282–6. doi: 10.1038/nature08530
126. Breuer RI, Soergel KH, Lashner BA, Christ ML, Hanauer SB, Vanagunas A, et al. Short chain fatty acid rectal irrigation for left-sided ulcerative colitis: a randomised, placebo controlled trial. Gut (1997) 40:485–91. doi: 10.1136/gut.40.4.485
127. Imoto Y, Kato A, Takabayashi T, Sakashita M, Norton JE, Suh LA, et al. Short-chain fatty acids induce tissue plasminogen activator in airway epithelial cells via GPR41&43. Clin Exp Allergy (2018) 48:544–54. doi: 10.1111/cea.13119
128. Khan AQ, Ahmad F, Raza SS, Zarif L, Siveen KS, Sher G, et al. Role of non-coding RNAs in the progression and resistance of cutaneous malignancies and autoimmune diseases. Semin Cancer Biol (2022) 83:208–26. doi: 10.1016/j.semcancer.2020.07.003
129. Zhao M, Wang L, Liang G, Zhang P, Deng X, Tang Q, et al. Up-regulation of microRNA-210 induces immune dysfunction via targeting FOXP3 in CD4(+) T cells of psoriasis vulgaris. Clin Immunol (2014) 150:22–30. doi: 10.1016/j.clim.2013.10.009
130. Liao J-M, Zhou X, Zhang Y, Lu H. MiR-1246: a new link of the p53 family with cancer and down syndrome. Cell Cycle (2012) 11:2624–30. doi: 10.4161/cc.20809
131. Faisal S, Toraih EA, Atef LM, Hassan R, Fouad MM, Al Ageeli E, et al. MicroRNA-17-92a-1 host gene (MIR17HG) expression signature and rs4284505 variant association with alopecia areata: a case–control study. Genes (Basel) (2022) 13:505. doi: 10.3390/genes13030505
132. Maher SA, Ismail NA, Toraih EA, Habib AH, Gouda NS, Gomaa AHA, et al. Hair follicle-related MicroRNA-34a serum expression and rs2666433A/G variant in patients with alopecia: a cross-sectional analysis. Biomolecules (2022) 12:602. doi: 10.3390/biom12050602
133. Conteduca G, Rossi A, Megiorni F, Parodi A, Ferrera F, Tardito S, et al. Single-nucleotide polymorphisms in 3′-untranslated region inducible costimulator gene and the important roles of miRNA in alopecia areata. Skin Health Dis (2021) 1:e34. doi: 10.1002/ski2.34
134. Paul S, Licona-Vázquez I, Serrano-Cano FI, Frías-Reid N, Pacheco-Dorantes C, Pathak S, et al. Current insight into the functions of microRNAs in common human hair loss disorders: a mini review. Hum Cell (2021) 34:1040–50. doi: 10.1007/s13577-021-00540-0
135. Tafazzoli A, Forstner AJ, Broadley D, Hofmann A, Redler S, Petukhova L, et al. Genome-wide MicroRNA analysis implicates miR-30b/d in the etiology of alopecia areata. J Invest Dermatol (2018) 138:549–56. doi: 10.1016/j.jid.2017.09.046
136. Sheng Y, Qi S, Hu R, Zhao J, Rui W, Miao Y, et al. Identification of blood microRNA alterations in patients with severe active alopecia areata. J Cell Biochem (2019) 120:14421–30. doi: 10.1002/jcb.28700
137. Wang X-Y, Guan X-H, Yu Z-P, Wu J, Huang Q-M, Deng K-Y, et al. Human amniotic stem cells-derived exosmal miR-181a-5p and miR-199a inhibit melanogenesis and promote melanosome degradation in skin hyperpigmentation, respectively. Stem Cell Res Ther (2021) 12:501. doi: 10.1186/s13287-021-02570-9
138. Goradel NH, Mohammadi N, Haghi-Aminjan H, Farhood B, Negahdari B, Sahebkar A. Regulation of tumor angiogenesis by microRNAs: state of the art. J Cell Physiol (2019) 234:1099–110. doi: 10.1002/jcp.27051
139. Sheng Y, Ma J, Zhao J, Qi S, Hu R, Yang Q. Differential expression patterns of specific long noncoding RNAs and competing endogenous RNA network in alopecia areata. J Cell Biochem (2019) 120:10737–47. doi: 10.1002/jcb.28365
140. Qi S, Sheng Y, Hu R, Xu F, Miao Y, Zhao J, et al. Genome-wide expression profiling of long non-coding RNAs and competing endogenous RNA networks in alopecia areata. Math Biosci Eng (2020) 18:696–711. doi: 10.3934/mbe.2021037
141. Yuan X, Tang Y, Zhao Z, Liu F, Shi W, Zhang Y, et al. Identification and verification of EOMEs regulated network in alopecia areata. Int Immunopharmacol (2020) 84:106544. doi: 10.1016/j.intimp.2020.106544
142. Nakanishi T, Mizutani K, Iida S, Matsushima Y, Umaoka A, Kondo M, et al. Janus kinase inhibitors ameliorated gastrointestinal amyloidosis and hypoalbuminemia in persistent dermatitis mouse model. Int J Mol Sci (2021) 23:28. doi: 10.3390/ijms23010028
143. Aung WW, Wang C, Xibei J, Horii M, Mizumaki K, Kano M, et al. Immunomodulating role of the JAKs inhibitor tofacitinib in a mouse model of bleomycin-induced scleroderma. J Dermatol Sci (2021) 101:174–84. doi: 10.1016/j.jdermsci.2020.12.007
144. King B, Mesinkovska N, Mirmirani P, Bruce S, Kempers S, Guttman-Yassky E, et al. Phase 2 randomized, dose-ranging trial of CTP-543, a selective janus kinase inhibitor, in moderate-to-severe alopecia areata. J Am Acad Dermatol (2022) 87:306–13. doi: 10.1016/j.jaad.2022.03.045
145. King B, Ko J, Forman S, Ohyama M, Mesinkovska N, Yu G, et al. Efficacy and safety of the oral janus kinase inhibitor baricitinib in the treatment of adults with alopecia areata: phase 2 results from a randomized controlled study. J Am Acad Dermatol (2021) 85:847–53. doi: 10.1016/j.jaad.2021.05.050
Keywords: alopecia areata, immune cells, interleukins, gut microbiota, therapeutic targets
Citation: Xu W, Wan S, Xie B and Song X (2023) Novel potential therapeutic targets of alopecia areata. Front. Immunol. 14:1148359. doi: 10.3389/fimmu.2023.1148359
Received: 20 January 2023; Accepted: 05 April 2023;
Published: 19 April 2023.
Edited by:
Antonio Ji-Xu, University of California, Davis, United StatesReviewed by:
Leszek Blicharz, Medical University of Warsaw, PolandCopyright © 2023 Xu, Wan, Xie and Song. This is an open-access article distributed under the terms of the Creative Commons Attribution License (CC BY). The use, distribution or reproduction in other forums is permitted, provided the original author(s) and the copyright owner(s) are credited and that the original publication in this journal is cited, in accordance with accepted academic practice. No use, distribution or reproduction is permitted which does not comply with these terms.
*Correspondence: Xiuzu Song, c29uZ3hpdXp1QHNpbmEuY29t
Disclaimer: All claims expressed in this article are solely those of the authors and do not necessarily represent those of their affiliated organizations, or those of the publisher, the editors and the reviewers. Any product that may be evaluated in this article or claim that may be made by its manufacturer is not guaranteed or endorsed by the publisher.
Research integrity at Frontiers
Learn more about the work of our research integrity team to safeguard the quality of each article we publish.