- Department of Occupational Health and Occupational Disease, College of Public Health, Zhengzhou University, Zhengzhou, Henan, China
Fibrosis is a pathological tissue repair activity in which many myofibroblasts are activated and extracellular matrix are excessively accumulated, leading to the formation of permanent scars and finally organ failure. A variety of organs, including the lung, liver, kidney, heart, and skin, can undergo fibrosis under the stimulation of various exogenous or endogenous pathogenic factors. At present, the pathogenesis of fibrosis is still not fully elucidated, but it is known that the immune system plays a key role in the initiation and progression of fibrosis. Immune checkpoint molecules are key regulators to maintain immune tolerance and homeostasis, among which the programmed cell death protein 1/programmed death ligand 1 (PD-1/PD-L1) axis has attracted much attention. The exciting achievements of tumor immunotherapy targeting PD-1/PD-L1 provide new insights into its use as a therapeutic target for other diseases. In recent years, the role of PD-1/PD-L1 axis in fibrosis has been preliminarily explored, further confirming the close relationship among PD-1/PD-L1 signaling, immune regulation, and fibrosis. This review discusses the structure, expression, function, and regulatory mechanism of PD-1 and PD-L1, and summarizes the research progress of PD-1/PD-L1 signaling in fibrotic diseases.
1 Introduction
Fibrosis is a pathological process in which fibrous connective tissue (mainly collagen) accumulates excessively around inflamed or damaged tissues to form permanent scar structures, affecting organ function and eventually leading to death (1). Collagen deposition in fibrosis is necessary for biological activities such as wound healing and tissue repair. Under physiological conditions, collagen deposition is finely regulated by a complex molecular network to ensure the effective operation of tissue repair. When the tissue damage is too severe or occurs continuously and repeatedly, the tissue repair program is disturbed and causes a progressive irreversible fibrotic response, which eventually leads to organ fibrosis (2). Thus, fibrosis is an end-stage manifestation of an uncontrolled pathological tissue repair response. Studies have shown that fibrosis occurs in almost all types of tissues in the human body (3). Although the fibrotic response of different organs may show phased adaptive changes under different pathological conditions, long-term tissue damage will eventually lead to substantial scar formation and fibrotic lesions, which further suggests that fibrosis is closely related to human diseases (4). The incidence of fibrosis diseases in various organs is increasing, but the specific therapeutic strategies targeting the fibrogenesis process are particularly lacking. Therefore, it is of great significance to further clarify the molecular mechanism of different types of fibrotic diseases, reveal the general rules of fibrotic response, and screen specific targets for improving the ability to cure fibrotic diseases.
Programmed cell death protein 1 (PD-1) and programmed death ligand 1 (PD-L1) are the key immunosuppressive signals to maintain central and peripheral immune tolerance, inhibit autoimmune responses, and regulate immune homeostasis (5). PD-1/PD-L1 axis plays an important role in T cell response, which can inhibit T cell activation, proliferation, cytokine secretion, induce T cell apoptosis, and exhaustion, thereby inhibiting T cell immune effector function (6, 7). Given this, the PD-1/PD-L1 axis has been widely concerned in a variety of tumors. The expression of PD-L1 on the surface of tumor cells is generally up-regulated, which binds to PD-1 on the surface of T cells to inhibit the anti-tumor immunity of T cells, thereby achieving immune escape (8). Based on this, immune checkpoint inhibitors targeting PD-1/PD-L1 signaling have made remarkable achievements in the clinical treatment of a variety of tumors, which has significantly improved the ability of tumor immunotherapy. The antitumor mechanism of PD-1/PD-L1 inhibitors is to limit immunosuppressive signals, restore cytotoxic T-cell function, and achieve tumor clearance (9). The success of PD-1/PD-L1 blockade therapy in tumors also provides certain enlightenment to the basic and clinical research of other immune-related diseases. It has been found that PD-1/PD-L1 axis is involved in the occurrence and development of autoimmune diseases, chronic infection, sepsis, cardiovascular diseases, neurodegenerative diseases, and fibrosis diseases (6, 7, 10). With the deepening of PD-1/PD-L1 research, in addition to T lymphocytes, more and more immune cell functions have been confirmed to be regulated by PD-1/PD-L1 signaling, including monocytes, dendritic cells, natural killer cells, and B cells (11, 12). It can be seen that PD-1/PD-L1 signaling pathway plays a crucial role in maintaining immune homeostasis and is closely related to human diseases. In recent years, the role of PD-1/PD-L1 axis in organ fibrosis has also been confirmed. Given the close relationship between the PD-1/PD-L1 axis, immune regulation, and fibrosis, in this review, we discuss the structure, expression, function, and regulatory mechanism of PD-1 and PD-L1, and summarize the research of PD-1/PD-L1 axis in fibrotic diseases of lung, liver and other organs. We hope to provide new ideas for further understanding the immunological mechanism of fibrosis and constructing PD-1/PD-L1-based immunotherapy strategies for fibrotic disease.
2 The pathogenesis of fibrosis
A variety of endogenous and exogenous factors can initiate the body’s fibrotic response, such as genetic factors, autoimmune inflammation, chronic infection, repeated exogenous toxic exposure, obesity, hypertension, and diabetes (13). Although the pathogenic factors of fibrosis are different in different organs and different disease backgrounds, the fibrosis process can be divided into four main stages based on pathological characteristics. First, tissue injury caused by endogenous or exogenous factors initiates the inflammatory response; Second, immune cells from peripheral blood and peripheral lymphoid tissue are massively recruited to the injured site. Third, activation of key fibrotic effector cells, myofibroblasts; Fourth, the excessive production and deposition of extracellular matrix components eventually lead to organ failure (4). The above series of physiological and pathological processes are comprehensive manifestations of the interaction of a variety of cells, molecules, and signaling pathways (Figure 1). The pathological response to fibrosis and the physiological response to tissue repair have similar biological mechanisms in essence. When the tissue damage signal cannot be eliminated in time, the protective tissue repair process gradually changes to the harmful fibrogenesis process (14). The cells involved in the fibrotic response mainly include inflammatory cells (such as macrophages, monocytes, neutrophils, and T lymphocytes), epithelial cells, endothelial cells, and fibroblasts (4, 15, 16). Myofibroblasts are the key effector cells that mediate fibrotic responses. They can secrete large amounts of ECM components, possess enhanced proliferation, migration, and contraction abilities, and are resistant to apoptosis (17). Fibroblasts are the major precursors of myofibroblasts, which can be activated and transdifferentiated into myofibroblasts by pro-fibrotic factors such as transforming growth factor β1 (TGF-β1). In addition, epithelial cells, endothelial cells, pericytes, fibrocytes, and even macrophages can convert into myofibroblasts under certain conditions and participate in the fibrotic response (18, 19). With the progress of fibrosis, myofibroblasts produce and release excessive ECM, which leads to scar formation and affects tissue structure. The contractile characteristics of myofibroblasts also cause deformation of tissue parenchyma and eventually lead to structural and functional abnormalities of tissues and organs. The main components of ECM proteins in fibrotic scar are type I and type III collagen, fibronectin, and laminin, which are highly conserved in different tissues (4). ECM not only affects tissue structure and cell interaction, but also directly regulates cell proliferation, differentiation, metabolism, and migration (20, 21). In summary, myofibroblasts and their secreted ECM components play a key role in the fibrotic response. Based on this, a large number of studies have focused on elucidating the molecular mechanism of transdifferentiation of various cells into myofibroblasts, and TGF-β1-mediated signaling pathway has been proven to be a key driver of fibrosis response (22). In addition, a variety of cytokines such as interleukin 4 (IL-4), IL-6, IL-13; Chemokines such as monocyte chemoattractant protein-1 (MCP-1); Growth factors such as vascular endothelial growth factor (VEGF) and platelet-derived growth factor (PDGF); And other mediators such as reactive oxygen species (ROS) and lipids are involved in the regulation of fibrosis (16, 23).
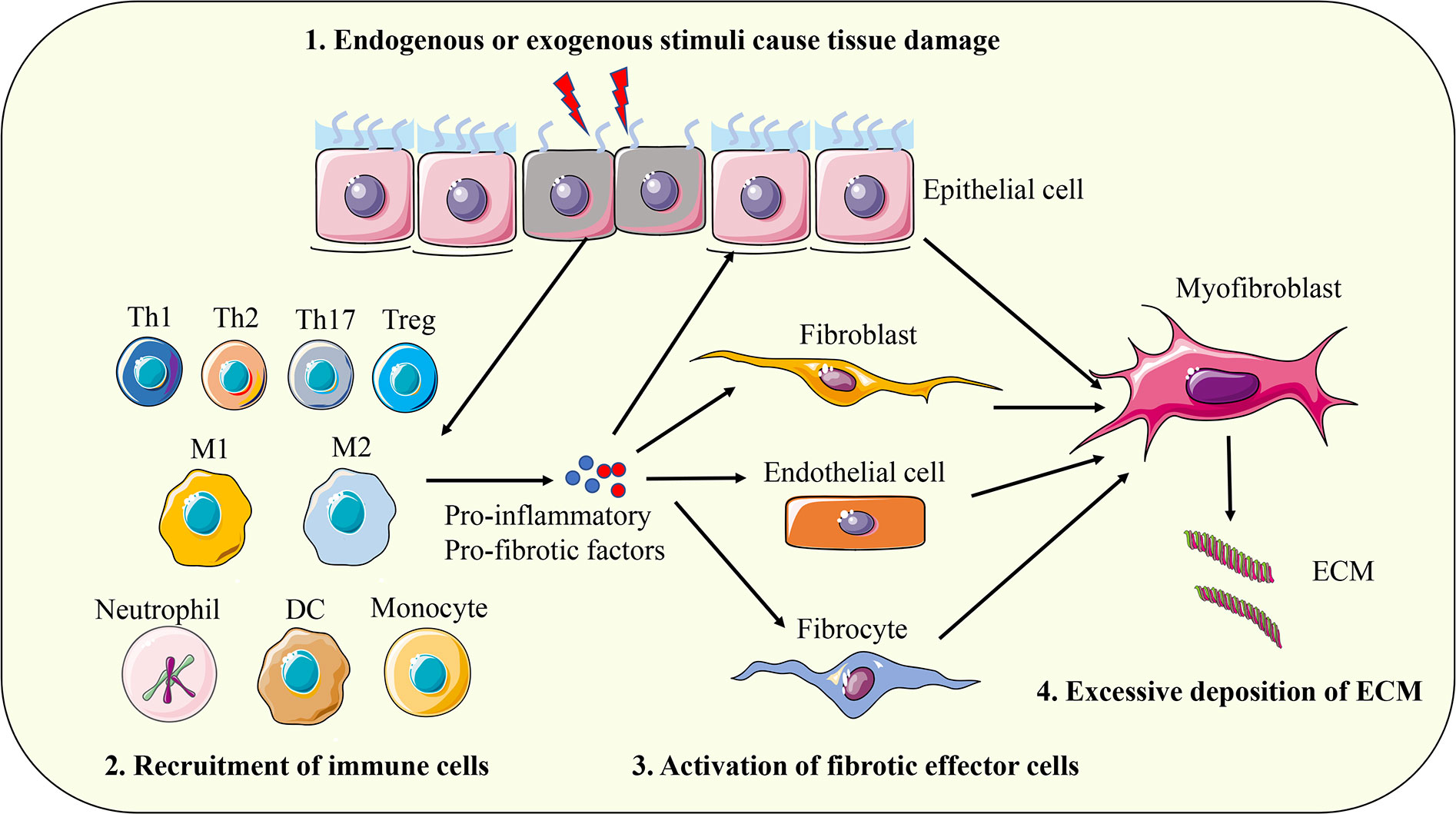
Figure 1 The pathogenesis of fibrosis. First, tissue injury caused by endogenous or exogenous factors initiates the inflammatory response; Second, immune cells from peripheral blood and peripheral lymphoid tissue are massively recruited to the injured site. Third, activation of key fibrotic effector cells, myofibroblasts; Fourth, the excessive production and deposition of extracellular matrix components eventually leads to organ failure. Th, T helper cell; Treg, regulatory T cell; M1, classically activated macrophages; M2, alternative activated macrophages; DC, dendritic cell; ECM, extracellular matrix.
3 Immunological regulation of fibrosis
The immune system plays a key regulatory role in various fibrotic diseases. Both acute and persistent chronic inflammation can initiate fibrosis, and the occurrence of inflammation involves a variety of innate and adaptive immune cells (16, 24). Under inflammation conditions, damaged cells can release a series of chemokines to recruit circulating monocytes and neutrophils to the site of tissue injury, where monocytes can further differentiate into macrophages to remove foreign microorganisms, particles, damaged cells, and cell debris, thereby promoting tissue repair and maintaining immune homeostasis. In the process of removing the risk factors that cause tissue damage, macrophages and neutrophils are also accompanied by the release of a series of active substances that are toxic to normal cells, such as reactive oxygen species, reactive nitrogen species, and pro-inflammatory cytokines. Therefore, when the risk factors are eliminated and these immune cells and cytokines cannot be reduced in time, it will lead to further deterioration of tissue damage, thus activating the fibrosis response (2, 14, 25). The high plasticity of macrophages enables them to acquire unique phenotypes and functions in different environments. Classically activated macrophages (M1) are mainly induced by proinflammatory cytokines such as interferon-γ (IFN-γ) and mediate inflammatory and anti-tumor immune responses. Alternatively activated macrophages (M2) are mainly induced by Th2 cytokines such as IL-4 and participate in immunosuppressive and profibrotic responses (26). In addition, DC, eosinophils, basophils, and mast cells have also been confirmed to participate in liver, lung, and kidney fibrosis (2). In addition to the innate immune cells mentioned above, the role of adaptive immune T lymphocytes in fibrosis has also been extensively studied. Existing evidence shows that T helper 1 (Th1) cells, Th2, Th17, and regulatory T cell (Treg) can play opposite roles in promoting or inhibiting fibrosis progression in different organs and different immune microenvironments (16, 27). Th1 cytokine IFN-γ can not only inhibit the differentiation of Th2 cells but also block the pro-fibrotic signal mediated by TGF-β1, thus exerting a significant anti-fibrotic effect (28, 29). However, another important Th1 cytokine tumor necrosis factor α (TNF-α) has been shown to have pro-fibrotic effects in a variety of animal models (30, 31). As for Th2, they are generally considered to release IL-4, IL-5, and IL-13, which have pro-fibrotic effects. For example, IL-13 can not only induce the expression and activation of key pro-fibrosis factor TGF-β1 but also directly promote the activation of fibroblasts (32, 33). However, in the acute tubular cell necrosis model, IL-4 can inhibit renal tubulointerstitial fibrosis by promoting tissue regeneration (34). IL-17A released by Th17 cells can up-regulate the expression of TGF-β1 and directly act on fibroblasts to promote their transformation into myofibroblasts (35). However, low dose of IL-17A could prevent and reverse diabetic renal fibrosis (36). Treg cells secrete two important cytokines, IL-10 and TGF-β1, which also play a complex role in fibrosis. TGF-β1 is a potent pro-fibrotic factor that can induce the transdifferentiation of fibroblasts, epithelial cells, and endothelial cells into myofibroblasts. Therefore, Treg can secrete large amounts of TGF-β1 to mediate the pro-fibrotic response (37). IL-10 is an important immunosuppressive factor, which can reduce tissue damage by inhibiting inflammatory response, thus playing an anti-fibrosis role (38). Therefore, T cell-mediated immune regulation plays a pivotal role in fibrosis, and its effector function is closely related to the state of cell polarization and the characteristics of immune microenvironment. In summary, the fibrosis response is precisely regulated pathophysiological processes involving a variety of immune cells and corresponding mediators. In-depth exploration of the immunological regulation mechanism can provide theoretical basis for further elucidating the pathogenesis of fibrosis.
4 Basis of the PD-1/PD-L1 axis
4.1 Structure of PD-1 and PD-L1 protein
PD-1, also known as CD279, is encoded by the PDCD1 gene located on human chromosome 2 (39). PD-1 is a type I transmembrane protein belonging to the CD28 immunoglobulin superfamily, which is composed of an IgV-like extracellular domain, a transmembrane domain, and a cytoplasmic domain. The cytoplasmic tail of PD-1 contains two key tyrosine signaling motifs, termed the immunoreceptor tyrosine inhibitory motif (ITIM) and the immunoreceptor tyrosine switch motif (ITSM), that mediate downstream signaling (40, 41).
PD-L1, also known as CD274 or B7-H1, is encoded by the PDCDL1 gene located on human chromosome 9, which belongs to the B7 immunoglobulin superfamily (42). As the main ligand of the PD-1 receptor, PD-L1 is also a typical type I transmembrane protein, containing extracellular IgV-like and IgC-like domains, a hydrophobic transmembrane domain, and a cytosolic domain consisting of 30 amino acids. However, in contrast to PD-1, the cytoplasmic tail of PD-L1 does not contain classical signal transduction motifs (5, 43).
4.2 Expression of PD-1 and PD-L1
Under physiological conditions, the expression of PD-1 and PD-L1 is an important signal to maintain immune tolerance and homeostasis. Under pathological conditions such as inflammation, autoimmune response, and cancer, the expression of PD-1 and PD-L1 can be induced by various factors such as cytokines, growth factors, and gene mutations to participate in disease progression.
PD-1 is mainly expressed in a variety of immune cells, including activated T cells, B cells, NK cells, macrophages, DCs, and monocytes (5, 7). Among the T cell subtypes, exhausted T cells, Treg cells, follicular helper T cells, follicular regulatory T cells, and memory T cells were identified to express PD-1, and high expression of PD-1 was an important feature of exhausted T cells (6). PD-1 is also a hallmark of effector T cells because it is not expressed by resting T cells and is dramatically upregulated when T cell receptor (TCR) mediated T-cell activation signals are activated in response to antigenic peptides (44). Hence, the positive signal mediated by the binding of antigen peptides-MHC complex to TCR is the most important signal to induce PD-1 expression in T cells. PD-1 expression is rapidly downregulated when T-cell activation signals are abrogated, otherwise it remains at high levels (45). In addition to TCR signaling, cytokines such as IL-10 and TGF-β can also induce the expression of PD-1 (46, 47).
PD-L1 is widely expressed in a variety of hematopoietic cells such as T cells, B cells, macrophages, DC, monocytes, mesenchymal stem cells, non-hematopoietic cells such as epithelial cells, endothelial cells, fibroblasts, keratinocytes, and various of cancer cells (12, 30, 48). Multiple proinflammatory factors such as IFN-γ can induce PD-L1 expression, and thus PD-L1 expression is usually associated with persistent inflammatory responses (49). In tumor immunity, IFN-γ secreted by tumor-infiltrating T cells induces the up-regulation of PD-L1 expression in tumor cells, thereby promoting the formation of immunosuppressive microenvironment, which may be one of the key mechanisms of tumor adaptive resistance.
4.3 PD-1/PD-L1 interaction
The engagement of the PD-1 receptor on the surface of T cells to PD-L1 ligands expressed on the surface of antigen presentation cells (APCs) or tumor cells will cause the conformational change of PD-1, leading to the phosphorylation of tyrosine in the ITIM and ITSM signal motifs in the intracellular domain of PD-1. Phosphorylated PD-1 recruits protein tyrosine phosphatases such as Src homology region 2 domain-containing phosphatase 1/2 (SHP1/2), and SHP1/2 could induce the dephosphorylation of TCR and CD28 downstream signal kinases such as Zeta-chain-associated protein kinase (ZAP70) and phosphoinositide 3-kinase/serine-threonine kinase 1 (PI3K/AKT). In turn, T cell activation signals mediated by ZAP70 and PKC such as protein kinase C (PKC), extracellular signal regulated kinase (ERK), phospholipase Cγ (PLCγ), and RAS will be blocked (Figure 2). Eventually, the above signaling cascade leads to T cell activation and proliferation inhibition, exhaustion, Treg cell differentiation, apoptosis initiation, cytokine production reduction, and metabolic changes (50–53). Some studies have shown that compared with TCR signaling, the PD-1 pathway may affect T cell activation mainly by interfering with CD28 costimulatory signaling (54).
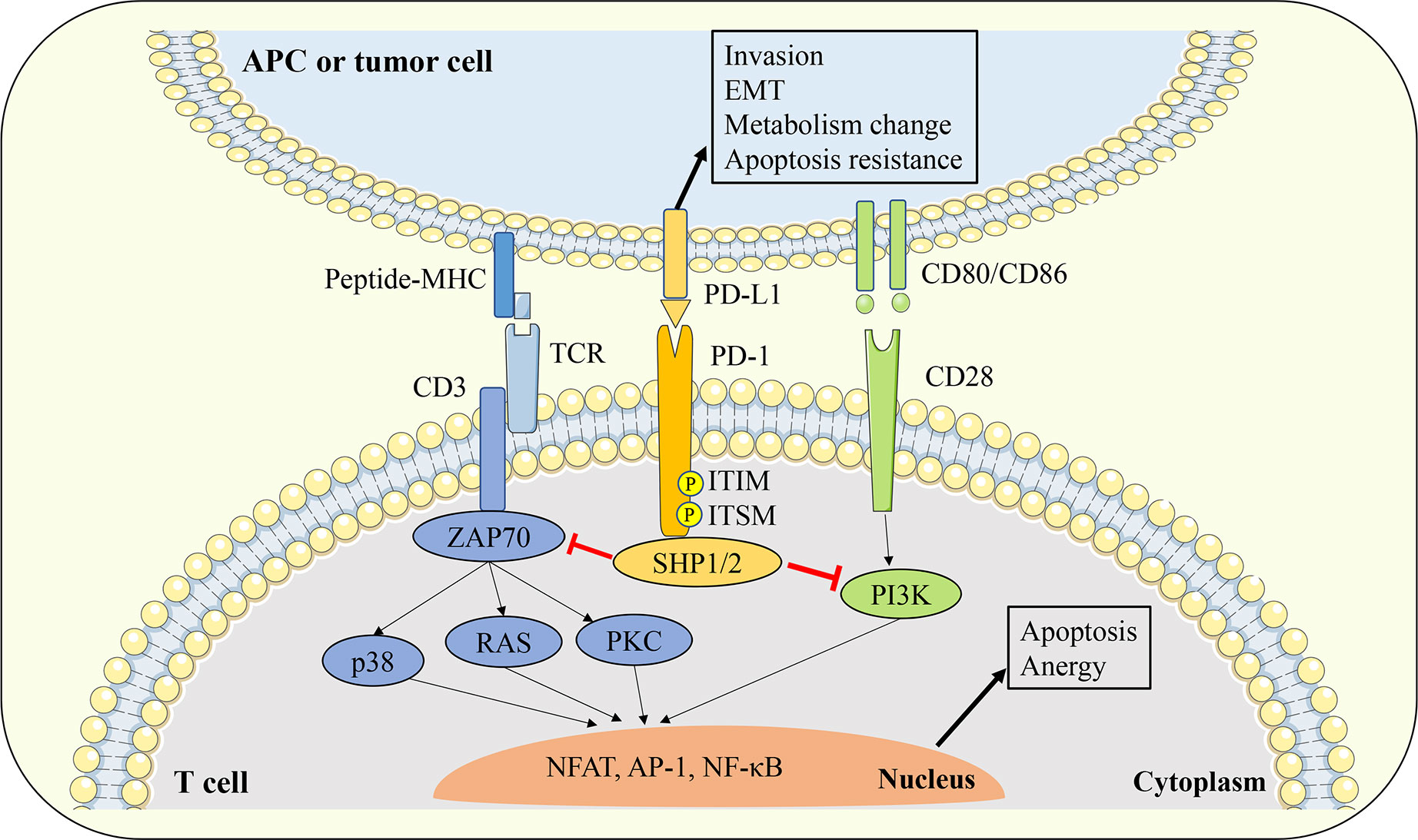
Figure 2 Interaction between PD-1 and PD-L1. The engagement of PD-1 receptor on the surface of T cells to PD-L1 ligands expressed on the surface of APCs or tumor cells will cause the conformational change of PD-1, leading to the phosphorylation of tyrosine in the ITIM and ITSM signal motifs in the intracellular domain of PD-1. Phosphorylated PD-1 recruits protein tyrosine phosphatases such as SHP1/2, and SHP1/2 could inhibit the downstream TCR and CD28 signal cascade. Thereby leads to T cell activation and proliferation inhibition, exhaustion, Treg cell differentiation, apoptosis initiation, cytokine production reduction, and metabolic changes. On the other hand, PD-L1 can also transmit biological signals to PD-L1 expressing cells and affect tumor cell invasion, EMT and apoptosis resistance. APC, antigen presentation cell; EMT, epithelial-mesenchymal transition; TCR, T cell receptor; ZAP70, zeta-chain-associated protein kinase; PKC, protein kinase C; SHP1/2, Src homology region 2 domain-containing phosphatase 1/2; ITIM, immunoreceptor tyrosine based inhibitory motif; ITSM, immunoreceptor tyrosine based switch motif;PI3K, phosphoinositide 3-kinase/serine-threonine kinase 1; NFAT, nuclear factor of activated T cells; AP-1, activator protein 1; NF-κB, nuclear factor kappa-light-chain-enhancer of activated B cells.
Due to the lack of classical signaling motifs in the intracellular domain of PD-L1 protein, the study of PD-1/PD-L1 interaction usually focuses on the signal transduction process in PD-1 expressing cells, but less attention is paid to the intracellular signaling mediated by PD-L1. However, it has been shown that the engagement of PD-1 and PD-L1 can also transmit biological signals to PD-L1-expressing cells and affect their functions. For example, stimulation of tumor cells with PD-1 recombinant proteins can activate PD-L1 signaling and enhance the apoptosis resistance of tumor cells (55). In an in vitro model lacking T cells, blocking PD-L1 signaling in tumor cells with PD-L1 antibody significantly affected their glucose metabolic activity, thereby inhibiting cell viability (56).
4.4 Non-cytomembrane PD-1 and PD-L1
In addition to the classical membrane-bound form, studies have confirmed that PD-1 and PD-L1 can also be distributed in extracellular or intracellular regions in a non-membrane-bound form (57). Extracellular PD-1 and PD-L1 are mainly present in various body fluids in soluble form or located on the surface of exosome membrane. Soluble PD-1 (sPD-1) or soluble PD-L1 (sPD-L1) is generated by alternative splicing of mRNA or membrane protein proteolysis, while exosomal PD-1 (Exo-PD-1) or exosomal PD-L1 (Exo-PD-L1) is released by the fusion of multivesicular bodies with the cell membrane. Intracellular PD-L1 can be distributed in the cytoplasm or nucleus and exert unique signal transduction functions depending on different mechanisms (58) (Figure 3).
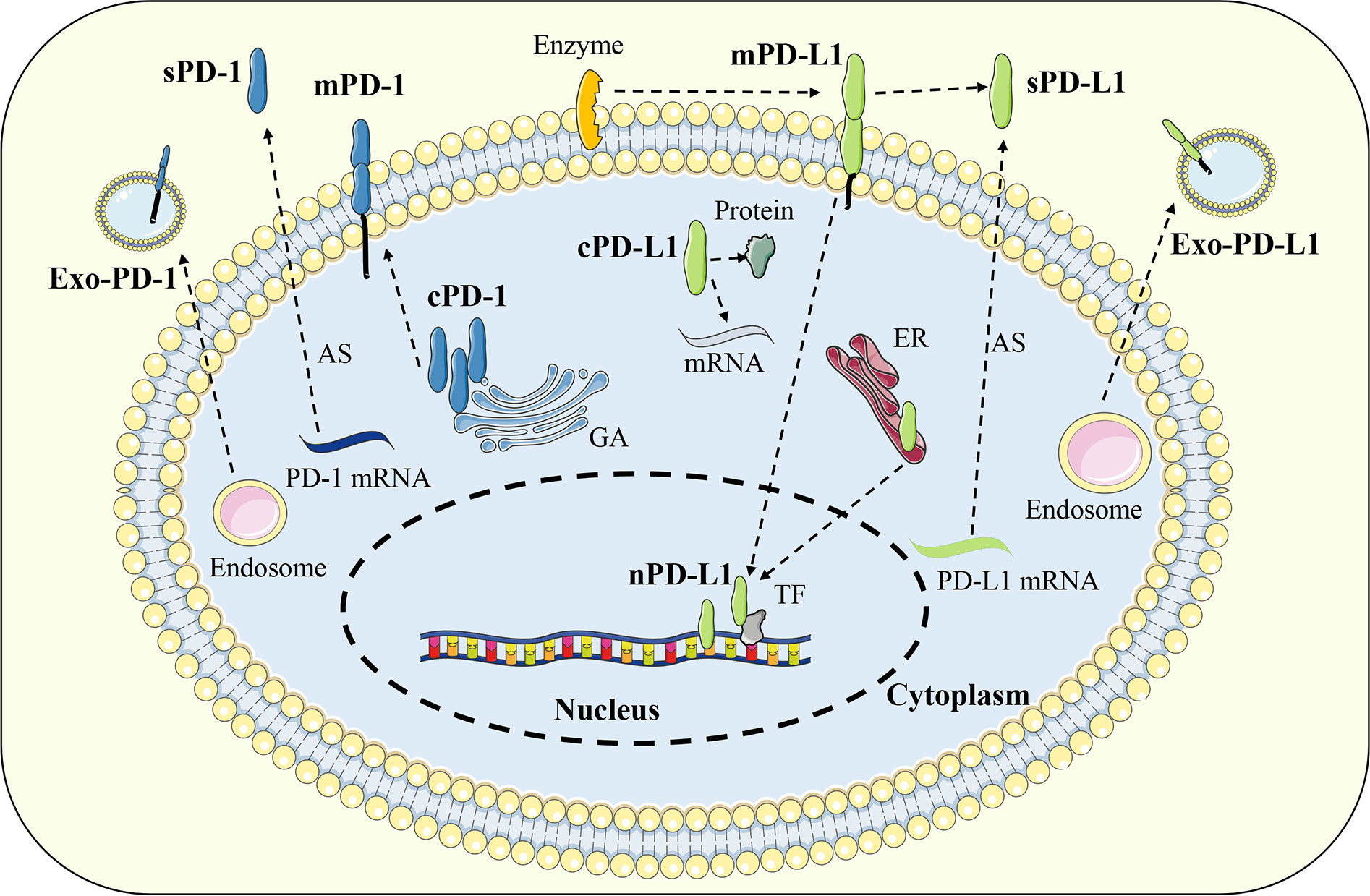
Figure 3 Distribution of PD-1 and PD-L1. In addition to the classical membrane-bound form, PD-1 and PD-L1 can also be distributed in extracellular or intracellular regions in a non-membrane-bound form. Extracellular PD-1 and PD-L1 are mainly present in soluble form or located on the surface of exosome. Intracellular PD-L1 can be distributed in the cytoplasm or nucleus while PD-1 was found only in the cytoplasm. Exo-PD-1/PD-L1, exosomal PD-1/PD-L1; sPD-1/PD-L1, soluble PD-1/PD-L1; mPD-1/PD-L1, membrane PD-1/PD-L1; cPD-1/PD-L1, cytoplasmic PD-1/PD-L1; nPD-L1, nuclear PD-L1; AS, alternative splicing; GA, golgi apparatus; ER, endoplasmic reticulum; TF, transcription factor.
4.4.1 Extracellular PD-1 and PD-L1
Extracellular PD-1 and PD-L1 mainly include free soluble forms or exosomal membrane-bound forms, which can be distributed throughout the body with blood circulation. Studies have shown that some soluble and exosomal forms of PD-1 and PD-L1 maintain intact immune activity and can bind to their cell membrane receptors/ligands to exert immunomodulatory functions (59). In immune checkpoint blockade therapy, extracellular PD-1 and PD-L1 can act as molecular decoys to specifically bind to therapeutic antibodies, thereby affecting efficacy (60). In addition, due to the wide distribution and easy detection of extracellular soluble and exosomal PD-1 and PD-L1, they have great value as biomarkers for disease diagnosis or prognosis evaluation (57).
sPD-1 and sPD-L1 are generated by alternative splicing of mRNA during transcription or proteolysis of cell membrane PD-1 and PD-L1, lacking typical transmembrane domains (61, 62). The PDCD1 gene contains five exons, which are responsible for encoding the extracellular, transmembrane, and intracellular structures of the PD-1 protein. Among them, the transmembrane domain of PD-1 is encoded by exon 3, and its selective deletion leads to the production of sPD-1. When only exon 3 is missing, sPD-1 has an intact extracellular domain and can specifically bind to PD-L1 ligand (5, 63). For PD-L1, the amplification of the endogenous retroelement LINE-2A in the intron of the PDCDL1 gene will lead to the deletion of the transmembrane and signaling domains of PD-L1 protein, thereby inducing the production of sPD-L1. Moreover, this type of sPD-L1 cannot activate the PD-1 signal but instead acts as a molecular decoy to antagonize the immunosuppressive activity of membrane PD-L1 (64). Other studies have reported that a variety of endogenous metalloproteinases are involved in the proteolysis of membrane PD-L1, resulting in the production of sPD-L1. For example, matrix metalloproteinase 9 (MMP9) and MMP13 catalyze the degradation of PD-L1 and PD-L2 on the surface of fibroblasts, inducing the production of sPD-L1 and reducing the ability of fibroblasts to activate the PD-1 signaling pathway, thereby indirectly promote T cell immunity (65). Thus, the biological activity that sPD-1 and sPD-L1 possess depends on how it is generated. When they contain an intact extracellular domain, they can specifically bind to the corresponding receptor or ligand to mediate immune regulation. Among them, the function of sPD-L1 is similar to that of membrane-bound PD-L1, which activates inhibitory immune signals by binding to PD-1 (59). sPD-L1 can be released by a variety of PD-L1-positive tumor cells to inhibit the effector function of T cells. Combined with the finding that sPD-L1 levels are significantly increased in the serum of cancer patients, it is suggested that sPD-L1 may be an important mediator for tumor cells to achieve immune escape (60, 66). Compared with PD-L1-mediated immunosuppressive effect, sPD-1 can prevent the activation of PD-1 signal by competitive binding to PD-L1, and indirectly enhance the anti-tumor immune effect (67). In melanoma, it has been found that intravenous injection of a plasmid encoding the extracellular domain of PD-1 can block the PD-1/PD-L1 signaling pathway and inhibit the lung metastasis and drug resistance of tumor cells (68). In conclusion, sPD-1 and sPD-L1 have a wide range of sources and diverse functions, and their immunomodulatory mechanism and clinical application value worth to be further exploring.
Exo-PD-1 and Exo-PD-L1 are localized on the exosome membrane, maintain the same protein structure as their cell membrane form, and are secreted to the outside of the cell with the release of exosomes (69). Many tumor cells, including lung cancer, prostate cancer, breast cancer, and melanoma, can release PD-L1-expressing exosomes, which prevent the activation of effector T cells by binding to PD-1 and promote the formation of a suppressive tumor immune microenvironment (58). Inhibition of genes related to exosome synthesis and release, such as N-SMase2 and Rab27a, can reduce the level of exosome PD-L1 by blocking the release of exosomes, and significantly improve the efficacy of anti-PD-L1 immunotherapy (70). The level of Exo-PD-L1 can be significantly upregulated in response to the stimulation of inflammatory factors such as IFN-γ (69). Therefore, it can be used to evaluate the response rate and efficacy of immune checkpoint inhibitor therapy. The high level of Exo-PD-L1 in the peripheral blood of patients before treatment may reflect the severe exhaustion of T cells, suggesting that it is difficult to ensure the efficacy of immune checkpoint inhibitors. If the level of Exo-PD-L1 in the peripheral blood of patients increases after treatment, it often indicates the recovery of T cell immune response and the restart of anti-tumor immunity (71, 72). Compared with sPD-L1, Exo-PD-L1 can be co-expressed with MHC molecules and thus has a more significant immunosuppressive ability (73). Due to the stability of exosomes, Exo-PD-L1 can not only regulate the local immune microenvironment but also enter the blood circulation to coordinate systemic immune homeostasis (74). In contrast to PD-L1, Exo-PD-1 was identified only in T cell-derived exosomes. Activated T cells can release exosomes carrying PD-1, which can bind to the cell surface or Exo-PD-L1 as a molecule decoy and induce PD-L1 internalization through clathrin-mediated endocytosis, thereby preventing the activation of PD-1 signaling and indirectly inhibiting PD-L1-mediated immunosuppression (75). In addition, T cell-derived Exo-PD-1 may be used to evaluate the clinical response rate of metastatic melanoma to ipilimumab treatment (76). In conclusion, Exo-PD-1 and Exo-PD-L1 can not only be used as therapeutic targets but also be applied to evaluate the clinical response rate and efficacy of immune checkpoint blockade.
4.4.2 Intracellular PD-1 and PD-L1
Intracellular PD-L1 could be located in the cytoplasm or nucleus. Nuclear PD-L1 (nPD-L1) can regulate the transcription procedure by directly binding to DNA or transcription factors, and then affect cell activities (77). Currently, nPD-L1 expression has been found in many types of tumor cells. In non-small cell lung cancer, nPD-L1 can directly bind to the transcription factor SP1 and upregulate the expression of growth arrest-specific 6 (GAS6). GAS6 promotes tumor cell proliferation through the downstream MerTK signaling pathway (78). In addition, nPD-L1 can also activate inflammatory pathways and promote the presentation of tumor antigens, which may be related to the high response rate of PD-1/PD-L1 blockade in tumors with high levels of PD-L1. At present, the formation mechanism of nPD-L1 is not clear. At first, it was thought to originate from the endocytosis of membrane PD-L1. However, subsequent studies have found that nPD-L1 contains more complex glycosylation groups than membrane PD-L1, suggesting that part of PD-L1 may undergo highly glycosylated reactions after translation in the endoplasmic reticulum and Golgi apparatus and then be directly transported to the nucleus (79). Cytoplasmic PD-L1 (cPD-L1) has also been confirmed to exist in tumor cells such as the lung, kidney, and pancreas, as well as non-tumor cells such as macrophages, fibroblasts, and epithelial cells (80). In tumor cells, PD-L1 can rely on signal transducer and activator of transcription 3 (STAT3) signaling pathway to accumulate in the cytoplasm. Furthermore, cPD-L1 affects the anti-tumor effect of resveratrol by preventing the nuclear translocation of cytochrome c oxidase subunit II (COX2) (81, 82). In addition, similar to the DNA-binding properties of nPD-L1, cPD-L1 can also bind to mRNA to maintain its stability and facilitate the translation program. For example, Tu et al. found that cPD-L1 can act as an RNA-binding protein to regulate the mRNA stability of NBS1 and BRCA1, which are associated with DNA damage and radiation resistance in some tumor cells (83). Thus, in contrast to classical immunosuppressive signals mediated by membrane PD-L1, intracellular PD-L1 can directly participate in transcriptional or translational processes and regulate tumor cell survival.
Compared with PD-L1, there is a lack of research on intracellular PD-1, and only a few pieces of research on T cells have confirmed the existence of intracellular PD-1. Studies have shown that the localization of PD-1 in T cells changes with their phenotypic switching. Among them, PD-1 expression was upregulated and distributed on the cell surface during T cell activation, while the expression of PD-1 in resting Treg cells was mainly localized intracellularly. When the TCR signal of Treg cells was activated, intracellular PD-1 could translocate to the cell membrane, thereby mediating immune regulation (84). In addition, in activated T cells, PD-1 is also distributed near the Golgi apparatus, but not in endosomes and lysosomes, which may be related to the intracellular trafficking of PD-1 synthesis (85). In the peripheral blood of patients with advanced melanoma, PD-1 accumulates in the cytoplasm of circulating T lymphocytes, which can supplement the loss of PD-1 on T cell membrane and mediate tumor immune escape by affecting the T cell function (86). Taken together, the main function of intracellular PD-1 may be as a reservoir of cell membrane PD-1 that compensates for the upregulation of cell membrane PD-1 levels under specific circumstances.
5 Regulation of PD-1 and PD-L1 expression
The expression of PD-1 and PD-L1 is exquisitely regulated in different tissues, cell types, stimulation signals, and immune microenvironments by distinct mechanisms. At the mRNA or protein level, it can be divided into transcriptional regulation, post-transcriptional regulation, epigenetic regulation, and post-translational modification (Figure 4). The synergistic operation of the above regulatory mechanisms maintains the dynamic balance of synthesis, modification, degradation, and stability of PD-1 and PD-L1 mRNA and protein, thereby ensuring the operation of PD-1/PD-L1 signaling pathway.
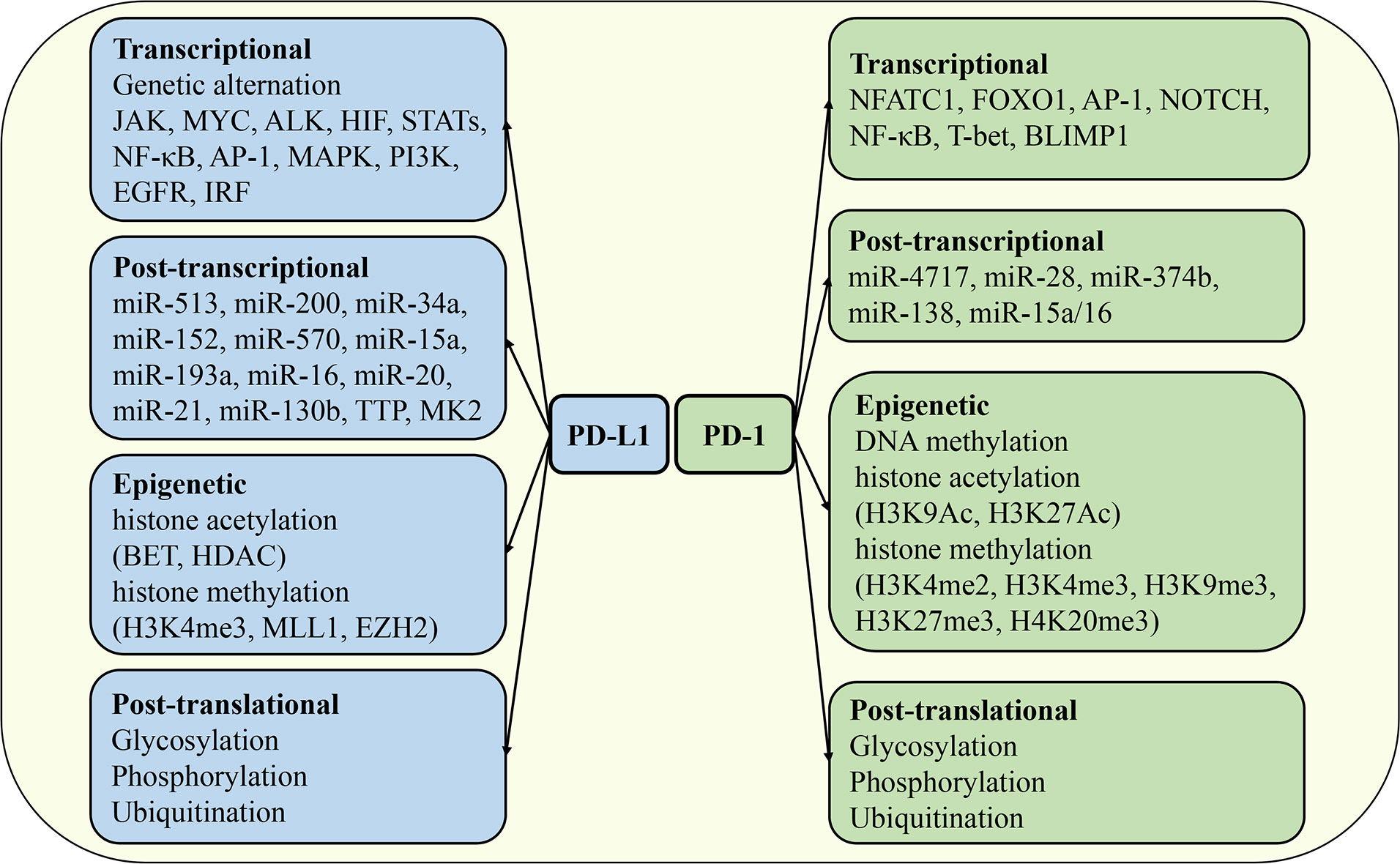
Figure 4 Regulation of PD-1 and PD-L1 expression. The mechanisms regulating the expression of PD-1 and PD-L1 include transcriptional regulation, post-transcriptional regulation, epigenetic regulation, and post-translational modification. JAK, Janus kinase; HIF, hypoxia-inducible factor; STAT, signal transducer and activator of transcription; MAPK, mitogen-activated protein kinases; EGFR, epidermal growth factor receptor; IRF, interferon response factor; FOXO1, forkhead box O1; BLIMP1, B lymphocyte-induced maturation protein 1; TTP, tristetraprolin; BET, bromodomain and extra-terminal domain; HDAC, histone deacetylases; MLL1, mixed lineage leukemia protein 1; EZH2, enhancer of zeste homolog 2.
5.1 Transcriptional regulation of PD-1 and PD-L1
Genomic alterations, oncogene activation, and inflammatory cytokines can promote PD-L1 expression mediated by a variety of transcription factors. First, genomic alterations can directly or indirectly promote PD-L1 expression, which is closely related to high levels of PD-L1 in tumors. For example, gene rearrangement changes such as amplification and translocation of the PDCDL1 gene can directly activate the transcriptional program of PD-L1 (87, 88). In addition, when the 3’UTR region of PDCDL1 is mutated, the expression of PD-L1 protein will be significantly enhanced (89). Moreover, Janus kinase 2 (JAK2), which regulates the expression of PD-L1, is located on the same chromosome as PDCDL1, and the mutation of JAK family genes can indirectly upregulate the mRNA level of PD-L1 (90). Second, a variety of carcinoma signal and transcription factors such as MYC, ALK, hypoxia-inducing factor 1/2 (HIF-1/2), STAT3, nuclear factor kappa-light-chain-enhancer of activated B cells (NF-κB), activator protein 1 (AP-1), mitogen-activated protein kinases (MAPK), PI3K and epidermal growth factor receptor (EGFR) were involved in the regulation of PD-L1 expression. Among them, the key oncogene MYC is involved in a variety of tumorigenesis processes. Studies have shown that MYC can directly bind to the promoter of the PDCDL1 gene to activate the transcription of PD-L1 (91). ALK signaling is associated with gene translocation and amplification, and the NPM-ALK fusion protein can activate STAT3 and further bind to the promoter of the PDCDL1 gene to drive the expression of PD-L1 (92). HIF is a key regulator of tumor angiogenesis and metastasis, and there are hypoxia response elements that can be recognized by HIF in the promoter region of the PDCDL1 gene (93). NF-κB signaling pathway is involved in the regulation of inflammatory response, and its subunit RELA can also bind to the promoter of PDCDL1 to promote PD-L1 transcription (94). Finally, various cytokines regulate PD-L1 expression through distinct signal transduction pathways. IFN-γ is the first cytokine found to activate PD-L1 expression and affect anti-tumor immune responses. IFN-γ is mainly derived from activated T cells, which activate the classical JAK/STAT signaling pathway by binding to IFNGR1/2, further inducing the expression of interferon response factor (IRF) and initiating a series of downstream transcriptional programs. It has now been confirmed that IRF1 plays a key role in IFN-γ induced PD-L1 expression (95). In addition, TNF-α, IL-17, IL-1β, IL-10, IL-4, IL-6 and IL-27 derived from various immune cells have been reported to induce the expression of PD-L1 in various immune and non-immune cells under different conditions (49). In addition, TGF-β, as a pleiotropic cytokine, also regulates PD-L1 expression and exhibits cellular and environmental specificity. For example, TGF-β induces PD-L1 expression in DCs cultured in vitro but inhibits PD-L1 expression in monocytes (96, 97).
Research on the regulation of PD-1 expression has mainly focused on T cells. At present, several transcription factors have been identified to regulate PD-1 expression on T cells, including the nuclear factor of activated T cells 1 (NFATC1), forkhead box O1 (FOXO1), AP-1, NOTCH, NF-κB, T-bet, and B lymphocyte-induced maturation protein (BLIMP1). During T cell activation, TCR signaling activates the transcription factor NFATC1 through the calcineurin pathway. Nuclear translocation of NFATC1 directly binds to the cis-regulatory elements of the PDCD1 gene to initiate the PD-1 transcriptional program. Hence, both calcineurin and NFAT inhibitors could inhibit the expression of PD-1 in T cells (98). During chronic viral infection, antigen-specific CD8+ T cells gradually transform to an exhausted phenotype and highly express PD-1, which is closely related to the persistent expression of FOXO1. Moreover, specific knockout of FOXO1 in CD8+ T cells significantly enhances its antiviral activity (99). In the tumor microenvironment, tumor-infiltrating exhausted T cells also express high levels of PD-1, but AP-1 rather than FOXO1 is the major transcription factor involved in the regulation of PD-1 expression. c-FOS subunit of AP-1 family can target the AP-1 binding site in the promoter of PDCD1 to promote the transcription of PD-1 (100). In addition to T cells, a few studies have focused on the transcriptional regulation of PD-1 expression in other immune cells. In macrophage activation, Toll-like receptors mediate the activation of the classical NF-κB signaling, and the NF-κB complex further specifically binds to the p65 binding site on the PDCD1 gene to initiate the transcription of PD-1. In B cells, NFATC1 and NF-κB have also been shown to be involved in the transcriptional regulation of PD-1 (101). In addition to the above-mentioned transcription factors and related signals that promote PD-1 expression, a few transcription factors have been found to inhibit the transcriptional activity of PD-1. BLIMP1 is an important regulator of CD8+ T cell differentiation and a key transcription factor that inhibits PD-1 expression. Mechanistically, BLIMP1 not only directly binds to the downstream sequence of the PDCD1 gene to inhibit the transcription of PD-1, but also indirectly inhibits the expression of PD-1 by downregulating the expression of PD-1 transcriptional activator NFATC1 (102). However, under the condition of chronic infection, although BLIMP1 is highly expressed in exhausted CD8+ T cells, its inhibitory effect on PD-1 transcription is abnormal, and the transcription factor T-bet plays a compensatory inhibitory role of PD-1 expression to maintain the function of T cells (103).
5.2 Post-transcriptional regulation of PD-1 and PD-L1
MicroRNAs (miRNAs) mediate mRNA degradation or translational repression by specifically recognizing and binding the 3’UTR sequence of mRNA, which is an important mechanism of post-transcriptional regulation. Studies have shown that PD-L1 expression is directly or indirectly regulated by a variety of miRNAs. Among them, miR-513 is the first miRNA identified to specifically regulate PD-L1 expression, which can directly bind to the 3’UTR sequence of PD-L1 mRNA to inhibit its translation. Interestingly, the expression of miR-513 was inhibited by inflammatory signaling of IFN-γ, a key activator of PD-L1, while overexpression of miR-513 antagonized IFN-γ induced PD-L1 expression (104). In addition, miR-200 blocked the epithelial-mesenchymal transition (EMT) of tumor cells and limited tumor metastasis by targeted inhibition of PD-L1 expression. Zinc finger E-box binding homeobox 1 (ZEB1), a key transcription factor regulating EMT, inhibits the expression of miR-200, so miR-200 is usually at a low level in the tumor microenvironment (105). In addition to miR-513 and miR-200, a variety of miRNAs such as miR-34a, miR-152, miR-570, miR-15a, miR-193a, and miR-16 have been found to directly inhibit the expression of PD-L1 in non-small cell lung cancer, gastric cancer, melanoma, and pancreatic cancer (106). Besides, miR-20, miR-21 and miR-130b could indirectly upregulate the expression of PD-L1 by interfering with protein phosphatase and tensin homolog (PTEN), an inhibitory regulator of PD-L1 (107). Moreover, some kinases are also involved in regulating the mRNA stability and translational program of PD-L1. Tristetraprolin (TTP) interferes with the mRNA stability of PD-L1 by binding to AU-rich elements in the 3’UTR region of the PDCDL1 gene, while MK2 kinase of MEK signaling pathway can inhibit the expression of TTP indirectly enhance the mRNA stability of PD-L1 and prolong its half-life (108).
PD-1 expression is also regulated by many miRNAs under different conditions. miR-4717 can directly bind to the 3’UTR region of PD-1 mRNA and downregulate its expression to enhance T cell-mediated anti-hepatitis B virus immune response (109). In melanoma, miR-28 inhibits the expression of PD-1 and reverses the T cell exhaustion phenotype, thereby enhancing the release of T cell cytokines such as IL-2 and TNF-α (110). Further studies showed that tumor cells could specifically block the inhibitory effect of miR-28 on PD-1 expression by secreting exosomes carrying CircRNA-002178 (111). In addition, miR-374b and miR-138 could also directly inhibit the translation program of PD-1 mRNA (112, 113). Similar to PD-L1, miRNA can also indirectly regulate PD-1 by controlling the expression of its upstream regulators. In glioma, miR-15a/16 depletion activated tumor-infiltrating CD8+ T cells via the mammalian target of rapamycin (mTOR) signaling pathway, as indicated by the downregulation of PD-1 expression and the increase of the anti-tumor factor IFN-γ production in T cells (114).
5.3 Epigenetic regulation of PD-1 and PD-L1
Epigenetic regulation is also an important mechanism affecting the expression of PD-1 and PD-L1. Histone acetylation and methylation are common epigenetic modifications to regulate PD-L1 expression. Histone acetylation of the PDCDL1 gene enhances the recruitment and binding of bromodomain and extra-terminal domain (BET) proteins, thereby promoting the transcription of PD-L1. The expression of PD-L1 was significantly downregulated when the interaction between BET protein and PDCDL1 gene was blocked, while the inhibition of histone deacetylases (HDAC) activity increased the expression of PD-L1 by maintaining the acetylation modification of PDCDL1 gene (115, 116). In addition, tri-methylation of histone H3 on lysine 4 (H3K4me3) has been reported to be associated with PD-L1 expression. The expression of methyltransferase enhancer of zeste homolog 2 (EZH2) was positively correlated with the level of PD-L1 in lung adenocarcinoma. Moreover, the histone methyltransferase mixed lineage leukemia protein 1 (MLL1) directly binds to the promoter of PDCDL1 gene and catalyzes H3K4 methylation to upregulate the expression of PD-L1 (117, 118).
PD-1 expression is regulated by DNA methylation and histone modifications. During T cell activation, the PD-1 gene locus shows dynamic DNA methylation characteristics, and the DNA methylation level is negatively correlated with PD-1 expression (119). The Pdcd1 gene of naive CD8+ T cells showed a high level of DNA methylation. In acute lymphocytic choriomeningitis virus (LCMV) infection, the DNA methylation level of the Pdcd1 gene was decreased with the activation of antigen-specific CD8+ T cells, accompanied by the upregulation of PD-1 expression. When the infection resolves, DNA methylation in memory T cells climbs to high levels (119). In experimental autoimmune encephalomyelitis, the PD-1 gene promoter region of autoreactive CD4+ T cells was also epigenetically modified (120). The histone modifications that regulate PD-1 expression mainly include methylation and acetylation, which can be divided into promoting or inhibiting modifications. During the activation of CD8+ T cells in vitro, the expression of PD-1 was up-regulated with the level of active histone modification markers H3K9Ac, H3K27Ac, H3K4me2, and H3K4me3 (102). When the activation of T cells was blocked and the expression of PD-1 was decreased, the repressive histone modification markers H3K9me3, H3K27me3, and H4K20me3 were significantly increased, while the active histone modification markers were decreased (102).
5.4 Post-translational regulation of PD-1 and PD-L1
Post-translational modification is the final procedure to regulate the expression level, protein stability, protein translocation, and protein-protein interaction of PD-1 and PD-L1, which directly affects the immune regulatory function mediated by the PD-1/PD-L1 axis. Under different conditions, PD-1 and PD-L1 proteins can undergo post-translational modification reactions such as glycosylation, phosphorylation, and ubiquitination mediated by different factors.
Glycosylation is the most common post-translational modification in mammalian cells. The heavily N-linked glycosylation of the PD-L1 protein significantly increases its stability and prolongs its half-life by about 3-fold by resisting glycogen synthase kinase 3β (GSK3β) mediated 26S proteasome degradation (121). Inhibition of PD-L1 glycosylation in tumor cells can enhance T cell-mediated anti-tumor immune response in breast cancer, suggesting that glycosylation is related to PD-L1 mediated tumorigenic effect (122). Similar to PD-L1, the extracellular IgV domain of PD-1 protein also shows a high degree of N-linked glycosylation, and the structural stability of glycosylated PD-1 protein is significantly increased (123). In addition, a time-dependent increase in glycosylated PD-1 levels was accompanied by a decrease in unglycosylated PD-1 levels during T cell activation (124). Glycosylation modification also affects the interaction between PD-1 and PD-L1. Several in vitro and in vivo studies have confirmed that interfering with glycosylation modification of PD-1 or PD-L1 will weaken their binding ability, thereby affecting the immunosuppressive effect of PD-1/PD-L1 axis (124, 125).
Phosphorylation is another important post-translational modification. When PD-L1 binds to PD-1, tyrosine phosphorylation of the PD-1 intracellular domain is the basis for initiating downstream inhibitory signals. Phosphorylated PD-1 blocks TCR and CD28 mediated T cell activation signals such as ZAP70, PI3K/AKT, and RAS/MEK/ERK by recruitment of SHP phosphatase (50, 53, 126). At present, the mechanism of PD-1 intracellular phosphorylation and SHP recruitment is not clear, but targeted inhibition of its interaction has been confirmed to enhance T cell-mediated anti-tumor immune response, suggesting the potential possibility of PD-1 phosphorylation as a target for immunotherapy (127). In contrast to phosphorylation in the intracellular domain of PD-1, multiple sites in the extracellular domain of PD-L1 can be phosphorylated. For example, GSK3β induces the phosphorylation of PD-L1 at serine (S184) and threonine (T180) sites and promotes the phosphorylated PD-L1 to the E3 ubiquitin ligase β-transducin repeat-containing protein (β-TrCP), which eventually leads to the degradation of PD-L1 (121). Moreover, phosphorylation of PD-L1 at the serine site (S195) by AMPK kinase induces abnormal PD-L1 glycosylation, which prevents PD-L1 from being transported to the Golgi apparatus for degradation in the endoplasmic reticulum (128).
Ubiquitination is a major post-translational modification that mediates the degradation of PD-1 and PD-L1 proteins. As mentioned above, aberrant PD-L1 glycosylation induces its degradation in the ER, and HRD1 plays an important role in this process as an E3 ubiquitin ligase (128). Interestingly, β-TrCP is involved in the degradation of unglycosylated PD-L1 as a substrate (121). In addition, the ubiquitin ligase STIP1 homology and U-Box-containing protein 1 (STUB1) are also involved in the degradation of membrane-bound PD-L1, while CKLF-like MARVEL transmembrane domain-containing protein 6 (CMTM6) blocked the interaction between STUB1 and PD-L1 to inhibit the degradation of PD-L1 (129). For PD-1, F-box protein 38 (FBXO38) is an important ubiquitin ligase mediating the ubiquitination of PD-1, which can specifically induce the degradation of PD-1 by the proteasome. Knockdown of FBXO38 significantly promoted tumor growth, and its expression was significantly reduced in multiple tumors (130). In addition, two ubiquitin ligases, Casitas B lineage lymphoma (c-Cbl) and kelch-like family member 22 (KLHL22), have also been reported to be involved in regulating the ubiquitination degradation of PD-1. Specifically, c-Cbl initiates the degradation program by binding to the cytoplasmic tail of PD-1, while KLHL22 mediates the ubiquitination degradation of PD-1 before its trafficking to the cell membrane (131, 132).
6 Functions of the PD-1/PD-L1 axis
The main function of the PD-1/PD-L1 axis is to limit the excessive activation of T cells to maintain immune tolerance, eliminate inflammatory responses, and prevent tissue damage. It is known that the biological processes of T cell proliferation, differentiation, cytokine secretion, effector function, immune memory, apoptosis, and exhaustion are regulated by PD-1/PD-L1 signaling. In addition, various cellular activities such as activation, phagocytosis, migration, invasion, and EMT of a variety of immune cells (such as B cells, macrophages, DC, NK cells) and non-immune cells (such as fibroblasts, epithelial cells, various tumor cells) have also been found to be regulated by PD-1/PD-L1 axis (Figure 5). Next, we mainly introduce the regulation of PD-1/PD-L1 axis on the function of fibrosis-related effector cells such as T cells, macrophages, epithelial cells, and fibroblasts.
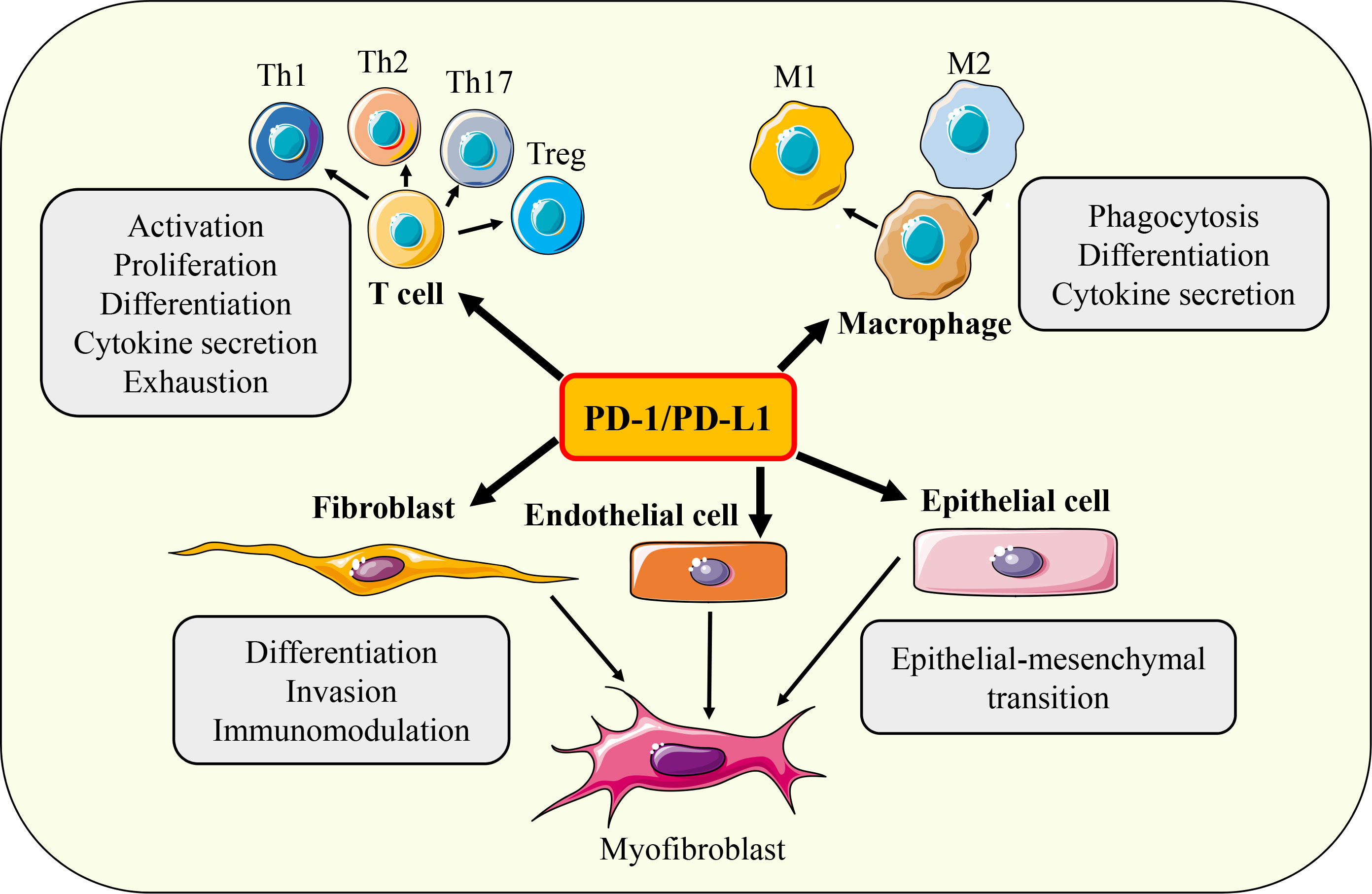
Figure 5 Functions of PD-1/PD-L1 axis. Under different conditions, PD-1/ PD-L1 axis can regulate the activation, proliferation, differentiation, cytokine secretion, exhaustion, phagocytosis, invasion, migration and EMT of T cells, macrophages, fibroblasts, epithelial cells and endothelial cells respectively.
6.1 Regulation of T cell function by PD-1/PD-L1 axis
The activation of T cells depends on the synergy of two signals, the first signal comes from the binding of the antigen peptide-MHC molecule complex on the surface of APC to the T cell receptor, and the second signal comes from the binding of the co-stimulatory molecules CD80 (B7.1) and CD86 (B7.2) on APC to the T cell stimulatory immune checkpoint CD28. In the absence of the second signal, T cells cannot be fully activated and enter an anergic or tolerant state and are not immunocompetent (133). In the early stage of T cell activation, a large number of negative regulatory factors are upregulated to limit the excessive activation of T cells, which are called immune checkpoint molecules. Cytotoxic T-lymphocyte-associated protein 4 (CTLA-4) is the first identified immune checkpoint that limits T cell activation by competitively binding costimulatory molecules (CD80 and CD86) on the surface of APC to inhibit CD28 signaling (39). PD-1 is another important inhibitory immune checkpoint expressed on the surface of activated T cells. By combining with its ligands PD-L1 and PD-L2, PD-1 antagonizes T cell activation signals such as TCR and CD28 (5). PD-1/PD-L1 pathway plays an important role in regulating the biological processes of T cell activation, differentiation, proliferation, cytokine secretion, and apoptosis, and is a key signal to maintain T cell tolerance and homeostasis (134). Thus, loss of PD-1/PD-L1 signaling causes autoimmune disease, whereas hyperactivated PD-1 signaling leads to decreased immune surveillance. In the tumor microenvironment, tumor cells upregulate the expression of PD-L1 and engaged with PD-1 on the surface of T cells, further inducing the exhaustion of CD8+ T cells and weakening their tumor-killing ability, so as to achieve immune escape (135). Subsequent clinical trials have confirmed that targeted inhibition of the PD-1/PD-L1 signaling pathway can continuously activate anti-tumor immune response, inhibit tumor growth, and reduce the disease burden of patients. Therefore, in 2014, the US Food and Drug Administration approved pembrolizumab, the first monoclonal antibody targeting PD-1, for immunotherapy in patients with unresectable or metastatic melanoma (136). The application of immune checkpoint inhibitors has significantly improved the clinical therapeutic ability of many tumors, and further confirmed the key role of PD-1/PD-L1 signal in tumorigenesis. Subsequently, a variety of immune checkpoint inhibitors targeting PD-1/PD-L1 axis, such as nivolumab, atezolizumab, avelumab, and durvalumab, were approved for the treatment of non-small cell lung cancer, melanoma, renal cell carcinoma, bladder cancer, Hodgkin’s lymphoma, liver cancer, and gastric cancer (137). Although some patients are resistant to PD-1/PD-L1 inhibitors, the overall clinical efficacy is still remarkable. Therefore, the PD-1/PD-L1 axis is a key regulator of T-cell immunity. Targeted inhibition or activation of the PD-1/PD-L1 signaling pathway can improve the dysfunction of T cell-dependent adaptive immunity, restore immune homeostasis, and thus treat immune-related diseases.
6.2 Regulation of macrophage function by PD-1/PD-L1 axis
Macrophages are involved in complex physiological and pathological immune responses with their highly plastic and versatile immunomodulatory abilities. In the tumor microenvironment, tumor-associated macrophages (TAMs) can inhibit the anti-tumor effector function of T cells and enhance the immune escape ability of tumor cells by hijacking the PD-1/PD-L1 signaling pathway. On the other hand, the phenotype and function of TAMs are also precisely regulated by PD-1/PD-L1 signaling, underscoring the importance of the PD-1/PD-L1 axis for macrophage immunity. PD-1/PD-L1 signaling regulates macrophage immune responses through multiple pathways such as controlling macrophage phagocytosis, cytokine production, and polarization. Phagocytosis is a key immune effector function of macrophages. The expression of PD-1 in TAMs was significantly upregulated, and the volume of PD-1+ TAMs increased and appeared as foam cells. Further studies found that the phagocytosis ability of PD-1+ TAMs was significantly reduced while blocking PD-1/PD-L1 signaling could promote the phagocytosis of macrophages to tumor cells and enhance the macrophage dependent anti-tumor immune response (138, 139). Secretion of cytokines is one of the mechanisms by which macrophages exert immunomodulatory effects. In RAW264.7 macrophages, LPS-induced IL-12 production could be inhibited by PD-1 signaling, which was similar to the inhibition of PD-1 mediated T cell activation by recruiting SHP-2 phosphatase to inhibit the activation of JNK/PI3K/AKT pathway (140). In chronic hepatitis C virus infection, PD-1/PD-L1 signaling can also downregulate the expression of IL-12 by interfering with STAT1 pathway (141). The function of macrophages changes with their phenotypic switching. Among them, M1 macrophages mainly play a pro-inflammatory role, while M2 macrophages mainly play an immunosuppressive function. Studies have shown that TAMs usually upregulate M2 macrophage related markers such as CD206 and CD11c, accompanied by high expression of PD-1, suggesting that PD-1/PD-L1 signaling may be involved in M2 macrophage polarization (138). When PD-1 was specifically knocked out, macrophages were activated to the M1 type and secreted a variety of pro-inflammatory factors (139). In addition, PD-1/PD-L1 signaling can also interfere with the ability of activated macrophages to mediate adaptive immune cells by inhibiting the expression of costimulatory molecules CD80 and CD86 and MHC molecules on the surface of activated macrophages (140). In conclusion, PD-1/PD-L1 signaling regulates macrophage function through multiple pathways to maintain immune homeostasis.
6.3 Regulation of epithelial cell function by PD-1/PD-L1 axis
The transition of epithelial cells to mesenchymal cells (EMT), which in turn replenish the number of myofibroblasts, is a key cellular event in the fibrotic response. Meanwhile, the occurrence of EMT is also closely related to tumor metastasis. At present, the correlation between PD-L1 and EMT has been confirmed in a variety of tumors such as non-small cell lung cancer, breast cancer, and head and neck squamous cell carcinoma, and the existing evidence suggests that there may be bidirectional feedback regulation between PD-L1 and EMT (142). Clinical data show that PD-L1 is highly expressed in stromal cells and lowly expressed in epithelial cells in a variety of tumor tissues. Moreover, the level of PD-L1 was positively correlated with the expression of EMT markers such as Snail, ZEB1, Vimentin, and N-cadherin, and negatively correlated with the expression of epithelial marker E-cadherin (143, 144). The above evidence provides sufficient evidence for a link between PD-L1 and EMT, but cannot confirm the temporal relevance of their regulatory relationship. At this point, researchers have carried out a large number of studies to reveal this puzzle. The adaptor protein Crk plays an important role in promoting the expression of EMT markers ZEB1 and N-cadherin. When Crk was knocked out specifically, the expression of PD-L1 was significantly inhibited (145). In addition, MUC1 can induce EMT by activating the NF-κB/ZEB1 pathway, and the p65 subunit of NF-κB can directly bind to the promoter of PD-L1 to promote its transcription (146). TGF-β, as a key inducer of EMT, can also upregulate the expression of PD-L1 by activating PI3K/AKT and MEK/ERK signaling pathways (147). Together, these studies suggest that key signals regulating EMT can simultaneously affect PD-L1 expression. On the other hand, the upregulation of PD-L1 can also induce EMT. In glioblastoma, PD-L1 upregulates the expression of EMT markers N-cadherin and vimentin by activating transcription factors Slug and β-catenin (148). In addition, the expression of PD-L1 was correlated with the migration of renal cancer cells, and silencing of PD-L1 significantly inhibited the expression of EMT markers vimentin and F-actin. Overexpression of PD-L1 promotes EMT by activating transcription factor sterol-regulatory element binding protein 1 (SREBP1) (149). In conclusion, the expression of PD-L1 is closely related to the occurrence of EMT, and multiple signals that initiate the EMT program can promote the expression of PD-L1, while upregulated PD-L1 can also drive the EMT process.
6.4 Regulation of fibroblast function by PD-1/PD-L1 axis
In fibrotic response, fibroblasts are the main source of myofibroblasts. The activation, invasion, and immunoregulatory function of fibroblasts are also closely regulated by the PD-1/PD-L1 signaling pathway. TGF-β is a key effector to induce the transdifferentiation of fibroblasts into myofibroblasts. Many studies have confirmed that TGF-β can induce the upregulation of PD-L1 during the activation of fibroblasts, and PD-L1 mediated signaling is necessary for fibroblast activation. In primary human lung fibroblasts, TGF-β induces PD-L1 expression and promotes transdifferentiation depending on SMAD3, p38, and yes-associated protein/transcriptional co-activator with PDZ-binding motif (YAP/TAZ) signaling. After silencing PD-L1 by siRNA, the expression levels of α-SMA, collagen I, and fibronectin in fibroblasts were significantly decreased (150). Mechanically, PD-L1 acts as a cofactor and binds to the key transdifferentiation transcription factor SMAD3 to form a protein complex, and then directly activates the transcription of transdifferentiation genes such as α-SMA. On the other hand, PD-L1 is involved in mediating the transduction of GSK3β/β-catenin signaling to indirectly promote the activation of fibroblasts (150). Another study found that PD-L1 promoted TGF-β induced fibroblast transdifferentiation by impairing autophagy through activation of PI3K/AKT/mTOR signaling pathway, and inhibition of PD-L1 using monoclonal antibody prevented fibroblast activation by restoring autophagy activity (151). Therefore, PD-L1 may act as an intermediate to coordinate the activities of multiple signaling pathways related to transdifferentiation to regulate the activation of fibroblasts. In addition, PD-L1 expression is also a key factor in maintaining the invasive phenotype of fibroblasts. Invasive fibroblasts with high expression of PD-L1 in lung tissue of patients with idiopathic pulmonary fibrosis (IPF) are closely related to the progression of pulmonary fibrosis. Overexpression of PD-L1 in normal fibroblasts enhances their invasion and migration abilities, and injection of invasive fibroblasts into immunodeficient mice induces severe pulmonary fibrosis (152). In addition, fibroblasts can regulate T-cell immune responses by expressing PD-L1. In vitro studies have shown that TGF-β can not only upregulate the expression of PD-L1 on the cell membrane during the activation of fibroblasts, but also induce fibroblasts to secrete exosomes containing PD-L1, and PD-L1+ exosomes can inhibit the proliferation of T cells (153). In vivo studies have also found that the upregulation of PD-L1 on fibroblasts in the fibrotic lung can induce the exhaustion of T cells and promote the formation of immunosuppressive microenvironment (154). In conclusion, PD-1/PD-L1 signaling can directly or indirectly participate in the fibrotic response by regulating the activation of fibroblasts and their immunomodulatory capacity.
6.5 Regulation of endothelial cell function by PD-1/PD-L1 axis
Similar to epithelial cells, endothelial cells can directly transform into myofibroblasts under specific stimuli or, by modulating immune responses, participate in fibrosis. However, only a few studies have focused on the role of endothelial cell-mediated PD-1/PD-L1 signaling in fibrosis. In radiation therapy induced pulmonary fibrosis, PD-L1+ endothelial cells co-express TGF-β. Bintrafusp alfa, a bifunctional inhibitor of PD-L1 and TGF-β, significantly ameliorated radiation therapy induced pulmonary fibrosis (155). These results tentatively suggest that endothelial cell mediated PD-L1 signaling is profibrotic, they can induce an immunosuppressive microenvironment while directly activating a profibrotic response by upregulating the expression of PD-L1 and TGF-β. However, in pulmonary arterial hypertension (PH) associated fibrosis, the maintenance of PD-L1 expression in right ventricular vascular endothelial cells by Treg is important for the inhibition of fibrosis. Once Treg was depleted, the expression of PD-L1 in endothelial cells was downregulated, and the inflammation and fibrosis of the right ventricle were significantly aggravated. Moreover, blockade of PD-1/PD-L1 signaling could offset the protective effect of Treg on PH-induced fibrosis (156). These evidences suggest that Treg can suppress inflammation and fibrosis by inducing PD-L1 expression in endothelial cells. Interestingly, murine vascular endothelial cells can induce allogeneic Treg generation in a PD-L1-dependent manner, and these induced Tregs can inhibit the proliferation of alloreactive T cells (157). In many cases, Tregs promote fibrosis progression by releasing immunosuppressive and profibrotic factors such as IL-10 and TGF-β, which suggests that endothelial cells can indirectly promote fibrosis by inducing Treg activation through PD-L1 signaling. In a murine heart allograft model, blockade of Lymphotoxin-Beta Receptor downregulated PD-L1 expression in vascular endothelial cells and induced cardiac inflammation and fibrosis (158). This further indicates that the expression of PD-L1 in endothelial cells is important for the maintenance of immune tolerance. In the early stage of lung injury induced by SARS-CoV-2, pulmonary venules and capillaries have obvious proliferation, dilatation, and distortion, accompanied by a significant upregulation of PD-L1 expression in endothelial cells. With the aggravation of infection, lung tissue injury gradually develops to fibrosis, while endothelial cells still express high levels of PD-L1 (159). This also suggests that PD-L1 signaling in endothelial cells is associated with fibrosis development, but the molecular mechanisms still need to be further elucidated. Taken together, endothelial cell-mediated PD-1/PD-L1 signaling is also an important regulator of fibrogenesis, and its specific role may be organ or microenvironment determined.
7 PD-1/PD-L1 axis in organ fibrosis
Fibrosis is a pathological response finely regulated by the immune system, and its occurrence and development involve the participation of various immune cells and various immune regulatory factors. Studies have shown that PD-1/PD-L1 signaling mediates fibrotic pathological responses by regulating T cell immunity, fibroblast activation, and epithelial EMT. However, the clinical and experimental data on PD-1/PD-L1 axis in fibrotic diseases are still limited.
7.1 PD-1/PD-L1 axis in pulmonary fibrosis
At present, the research on PD-1/PD-L1 axis in pulmonary fibrosis mainly focuses on IPF and bleomycin-induced experimental pulmonary fibrosis. First, PD-1/PD-L1 signaling modulates pulmonary fibrosis by regulating T cell function. The expression of PD-1 in lung tissue and peripheral blood T cells of patients with IPF is significantly increased, while the level of PD-L1 has no significant change compared with healthy controls (160). The proliferation of PD-1+CD4+ T cells was significantly reduced, but their ability to secrete pro-fibrotic factors such as IL-17A and TGF-β was significantly increased, which could induce collagen I production from lung fibroblasts in vitro. Notably, pro-fibrotic T cells were mainly Th17 subtypes, which were induced by the PD-1/STAT3 pathway. In addition, PD-1 knockout or anti-PD-L1 antibodies significantly inhibited bleomycin-induced pulmonary fibrosis (161). The above evidence suggests that PD-1 signaling induces the activation of T cells with a profibrotic phenotype that plays an important role in the pathogenesis of IPF.
Second, PD-L1 expression correlates with the immunomodulatory capacity of lung fibroblasts. As a member of the AP-1 family, JUN is an important transcription factor regulating cell growth and proliferation, and excessive activation of JUN can induce spontaneous pulmonary fibrosis. In JUN-induced pulmonary fibrosis, the high expression of JUN in fibroblasts induces the upregulation of PD-L1 and CD47 by regulating chromatin remodeling and enhancing DNA accessibility and promoting the release of pro-fibrotic factor IL-6 (154). Activated fibroblasts with high expression of PD-L1 and CD47 can further interact with T cells and macrophages in lung tissue, induce the exhaustion of T cells and inhibit the clearance of myofibroblasts by macrophages, thereby promoting the formation of an inhibitory immune microenvironment (154). Combined blockade of PD-L1, CD47, and IL-6 signaling can significantly inhibit bleomycin-induced pulmonary fibrosis in mice by activating adaptive immune response and macrophage phagocytic activity, suggesting that PD-L1 mediated pro-fibrotic response may be the common mechanism of pulmonary fibrosis induced by different factors (154). Another in vitro study found that TGF-β induced PD-L1 expression on the cell surface and exosomes of multiple human and mouse derived fibroblasts by activating the classical SMAD2/3 and the non-classical YAP/TAZ pathways, and TGF-β-activated fibroblasts derived exosomes can inhibit the proliferation of T cells (153). These results suggest that PD-L1 can mediate the immunomodulatory function of fibroblasts to promote fibrosis.
Third, PD-L1 directly regulates the activation of fibroblasts and their profibrotic phenotype. Invasive fibroblasts derived from the lung tissues of IPF patients highly express PD-L1, which is closely related to the progression of pulmonary fibrosis (152). Overexpression of PD-L1 in normal fibroblasts significantly enhanced their invasion and migration abilities, indicating that PD-L1 expression is a key factor in acquiring the invasive phenotype of fibroblasts. Mechanistically, the expression of PD-L1 and the invasive ability of invasive fibroblasts is regulated by p53 and focal adhesion kinase (FAK) signals. Injection of invasive fibroblasts into immunodeficient mice via the tail vein can induce severe pulmonary fibrosis, and blockade of PD-1/PD-L1 signaling can inhibit pulmonary fibrosis (152). Relevant in vitro studies have shown that silencing PD-L1 can inhibit TGF-β induced transdifferentiation of pulmonary fibroblasts and reduce their migration ability (150). In particular, the expression of PD-L1 in fibroblasts is dependent on TGF-β mediated activation of SMAD3 and p38 signaling. Upregulated PD-L1 acts as a SMAD3 cofactor to initiate transcription of transdifferentiation related gene α-SMA, and activates GSK3β/β-catenin signaling pathway to promote transdifferentiation (150). In addition, anti-PD-L1 antibodies can restore the impaired autophagy activity of fibroblasts under the stimulation of TGF-β and inhibit their transdifferentiation by inhibiting the PI3K/AKT/mTOR pathway (151). Thus, PD-L1 can regulate the fibrotic response by directly controlling the activation of key fibrotic effector cells, fibroblasts.
Fourth, sPD-L1 may be an important profibrotic factor. In clinical studies of IPF, researchers found that plasma levels of sPD-L1 were significantly higher in IPF patients than in healthy controls, and that sPD-L1 levels tended to increase with the progression of fibrosis (162, 163). As mentioned above, certain forms of sPD-L1 are fully immunocompetent and can directly activate PD-1 signaling to exert immunosuppressive function. Thus, sPD-L1 originating from fibrotic lung tissue may be transmitted distally through the circulation, thereby creating a systemic immunosuppressive environment for fibrosis progression. However, further preclinical in vivo studies are needed to confirm this hypothesis. In addition, which effector cells are responsible for the large amount of secreted sPD-L1 also needs to be elucidated.
Fifth, interactions between the lung microbiome and PD-1/PD-L1 signaling may also influence the progression of pulmonary fibrosis. Although it has been considered that the lung is sterile, a large number of evidences have shown that local lung microbiome integrity is important for maintaining lung homeostasis (164). In addition, the abnormal diversity and composition of the lung microbiome are associated with a variety of lung diseases (165). During the formation stage of lung microbiome in neonatal mice, changes in respiratory microbial subsets can lead to up-regulation of PD-L1 expression in lung CD11b+ DC and induce the generation of Helios− Treg, thereby protecting neonatal mice from airway hyperresponsiveness to allergens (166). In lung cancer, Lee et al. found that the microbial species in bronchoalveolar lavage fluid of cancer patients were correlated with the PD-L1 level. Veillonella dispar was identified in the group with high PD-L1 expression, and Neisseria was mainly in the group with low PD-L1 expression (167). Furthermore, Kumanogoh et al. found that the presence of the lung microbiome is critical for supporting patient sensitivity to anti-PD-1 therapy (168). The above evidence suggests that there is a reciprocal regulatory relationship between PD-1/PD-L1 axis and the lung microbiome, and their interaction is of great significance for the homeostasis of the lung immune microenvironment, but its effect on pulmonary fibrosis still needs to be further explored.
Based on the above evidence, PD-1/PD-L1 signaling appears to promote the progression of pulmonary fibrosis. Similar to our view, Jiang et al. in their recent review of the role of PD-1/PD-L1 axis in IPF concluded that the PD-1/PD-L1 pathway mainly plays a profibrotic role (169). However, PD-1/PD-L1 signaling may also prevent fibrosis development by correcting the dysregulated pulmonary immunity. Resting mesenchymal stem cells (MSCs) express a low level of PD-L1, and when they interact with activated T cells, they can significantly upregulate the expression of PD-L1 to inhibit inflammatory response, suggesting that MSCs can rely on PD-1/PD-L1 axis to exert their immunomodulatory function (160). In a humanized mouse model, intravenous administration of human MSCs inhibited pathological T cell infiltration and proinflammatory cytokine production in mouse lung tissue and attenuated bleomycin-induced pulmonary fibrosis, while PD-L1 monoclonal antibody significantly interfered with these therapeutic effects (160). In other words, MSCs inhibited the inflammatory response in lung tissue by activating the PD-1/PD-L1 signaling pathway, thereby reducing fibrosis. This also suggests the theoretical feasibility of activating PD-1/PD-L1 signaling to inhibit inflammatory response for the treatment of pulmonary fibrosis.
In silicotic fibrosis induced by silica exposure, a few studies have begun to focus on the role of PD-1/PD-L1 pathway. Two clinical studies of silicosis patients conducted in China and Brazil obtained similar results. The expression of PD-1 on CD4+ and CD8+ T cells and the expression of PD-L1 and PD-L2 on CD14+ monocytes in peripheral blood of silicosis patients are significantly lower than those of healthy people, and the downregulation of PD-1 may be related to the single nucleotide polymorphism of PDCD1 gene (170, 171). However, our previous experimental study on silicosis found that the overall levels of PD-1 and PD-L1 were significantly increased in the lung tissues, spleen, and lymph nodes of silicosis mice (172). In-depth analysis of T cell subtypes found that the expression of PD-1 in CD8+ T cells was upregulated in the peripheral blood of early silicosis mice, but downregulated in the lung tissue. The expression of PD-1 in CD4+ T cells was significantly increased in lung tissues of early and late silicosis, but there was no significant change in peripheral blood (172). Based on this, we further blocked the PD-1/PD-L1 pathway in an experimental silicosis model, and the results showed that small molecule inhibitors of PD-1/PD-L1 could significantly reduce silicosis fibrosis (172). Hence, PD-1/PD-L1 signaling is also involved in the pathogenesis of silicosis, but the mechanism needs to be further studied in vivo and in vitro.
In conclusion, PD-1/PD-L1 axis plays a key regulatory role in the pathogenesis of pulmonary fibrosis induced by different factors, and its effects is dominated by pathogenic factors, fibrosis stage and immune cell types (Table 1). The wide expression of PD-1 and PD-L1 identifies its versatile immunomodulatory ability, which can participate in pulmonary fibrosis by regulating a variety of biological activities such as T cell differentiation and cytokine secretion, fibroblast activation, and the interaction between various fibrotic effector cells. Overall, PD-1/PD-L1 axis is mainly pro-fibrotic, but it can also inhibit fibrosis progression through specific mechanisms. Targeting the PD-1/PD-L1 pathway may be a new direction for immunotherapy of pulmonary fibrosis in the future. However, the unique role of PD-1/PD-L1 axis in different scenarios of pulmonary fibrosis should be further explored.
7.2 PD-1/PD-L1 axis in liver fibrosis
PD-1/PD-L1 axis is also involved in liver fibrosis, liver cancer-related fibrosis, and cirrhosis. In hepatitis B virus (HCV) infection-induced liver fibrosis, the expression of PD-1 and PD-L1 in liver tissue is significantly increased, which together with other immune checkpoint molecules such as CTLA-4, T cell immunoglobulin and mucin domain-containing protein 3 (TIM-3) and lymphocyte activation gene 3 (LAG3), promotes the formation of immunosuppressive microenvironment in liver (173). In addition, there is a positive correlation between the levels of PD-1 and PD-L1 and the severity of liver fibrosis, suggesting that PD-1/PD-L1 signaling may promote liver fibrosis. Another clinical study also found that serum sPD-1 levels in patients with HCV infection gradually increased with the severity of liver fibrosis, and was significantly higher than that in healthy people (174). Experimental studies have shown that Golgi membrane protein 1 (GOLM1) is significantly upregulated in carbon tetrachloride (CCL4) induced mouse liver fibrosis, and GOML1 induces PD-L1 expression and promotes fibrosis by activating EGFR/AKT/STAT3 signaling pathway (175). The aggressive fibrotic response further induces a suppressive immune microenvironment, thereby accelerating hepatocellular carcinoma (HCC) development. Therefore, targeted inhibition of PD-L1 can enhance anti-tumor and anti-fibrotic immune responses by reducing M2 macrophages and myeloid-derived suppressor cells in the fibrotic immunosuppressive microenvironment, and increasing the number of effector CD8+ T cells in liver fibrosis induced HCC (175). In liver cirrhosis, the expression of PD-1 upregulated in liver, peripheral blood, and spleen T cells, and the blockage of liver macrophages phagocytosis mediated by PD-1/PD-L1 pathway may be involved in the pathogenesis of liver cirrhosis (176). The above research evidence collectively proves that PD-1/PD-L1 signaling promotes the development of liver fibrosis, but other studies have found that indirect activation of PD-L1 signaling can prevent liver fibrosis. In CCL4-induced liver fibrosis in mice, kinsenoside can inhibit the activation and maturation of DCs by targeting the PI3K/AKT/FoxO1 signaling pathway and upregulating the expression of PD-L1 in DCs while inhibiting the release of IL-12 (177). Expression of PD-L1 on the surface of DCs interacts with CD8+ T cells to inhibit their activation, while the reduction of IL-12 leads to the blockade of hepatic stellate cell activation and ECM release, thereby reducing liver fibrosis (177). In summary, similar to pulmonary fibrosis, PD-1/PD-L1 axis also plays an important role in the pathogenesis of liver fibrosis. Notably, PD-1/PD-L1 axis mediated immunomodulation can regulate the development of liver fibrosis by reprogramming the function of liver macrophages, DC, and T cells. Similar to pulmonary fibrosis, the massive proliferation of myofibroblasts is the direct cause of the aggravation of liver fibrosis. However, the role of PD-1/PD-L1 signaling in the transdifferentiation of hepatic stellate cells into myofibroblasts has been poorly studied, so this is the focus of further attention, and the mechanism needs to be further elucidated.
7.3 PD-1/PD-L1 axis in tubulointerstitial nephritis and renal fibrosis
Tubulointerstitial nephritis (TIN) is one of the major causes of acute kidney injury and may eventually lead to chronic kidney disease such as renal fibrosis. Although not very common, there have been some case reports of TIN and renal fibrosis development during the treatment of PD-1 or PD-L1 inhibitors against a variety of cancers (178). In a clinical application of Nivolumab in the treatment of recurrent gastric cancer, the patient developed an apparent acute granulomatous TIN. Renal biopsy showed that the main lesions occurred in the renal tubular and interstitial areas, and renal tubular atrophy with granulomatous changes was the typical feature. Notably, PD-L1 was highly expressed by both macrophages in the granulomatous tissue and degenerated renal tubular epithelial cells (179). Moreover, TIN also occurs in patients with metastatic malignant melanoma treated with CTLA-4 and PD-1 inhibitors. Histological examination revealed inflammatory cell infiltration, edema and fibrosis in the renal interstitium. Among the infiltrated cells, CD3+ T cells and CD163+ macrophages were predominant, which highly expressed PD-L1 and were negative for PD-1 (180). This suggests that T cells and macrophages play an important role in renal fibrosis caused by PD-1/PD-L1 signaling imbalance. In addition, in the treatment of metastatic renal cell carcinoma with atezolizumab, both CD4+ and CD8+ T cells are key players in TIN pathogenesis (181). The above clinical data have fully proved that PD-1/PD-L1 axis is related to TIN and renal fibrosis, while other experimental evidence may partly explain its mechanism. Renal tubular epithelial cells (TEC) are a special type of APC in the kidney, which constitutively express MHC-II molecules and weakly express PD-L1 under physiological conditions (182, 183). When stimulated by pro-inflammatory factors such as IFN-γ, the expression of PD-L1 in TEC was increased in a dose-dependent manner. Moreover, blocking PD-1/PD-L1 signaling in IFN-γ-activated TEC and T cell co-culture system could relieve the inhibitory effect of TEC on Th1 and Th2 cytokine production (182). Interestingly, TGF-β, a crucial profibrotic factor, downregulated PD-L1 expression in TEC and enhanced their ability to activate CD8+ T cells (184). This suggests that TGF-β may promote TIN generation by regulating the interaction between TEC and T cells, and then induces renal fibrosis. Mechanistically, PD-1/PD-L1 axis may mediate TIN and renal fibrosis from the following aspects. First, under the use of immune checkpoint inhibitors, the immune system, especially T cells, is overactivated, which leads to the initiation of inflammatory response, resulting in adverse effects. Thus, TIN and even renal fibrosis induced by PD-1 or PD-L1 inhibitors may represent an autoimmune response. Second, the physiological expression of PD-L1 in renal tubular epithelial cells is important for the maintenance of immune tolerance in renal interstitium. The application of PD-1 or PD-L1 inhibitors disrupts renal interstitial immune homeostasis, which leads to T cell overactivation and ultimately TIN. In the interaction between renal tubular epithelial cells and T lymphocytes, TEC can present antigens to initiate T cell activation, but on the other hand, it can inhibit T cell activation by binding PD-L1 to the PD-1 receptor on the surface of T cells. Third, it is worth noting that in TIN induced by PD-1 or PD-L1 inhibitors, patients are usually exposed to some drugs with nephrotoxicity such as proton pump inhibitors and non-steroidal anti-inflammatory drugs before receiving immune checkpoint inhibitor therapy. This suggests that PD-1 or PD-L1 inhibitors may have reactivated drug-specific effector T-cell clones in the resting state. In conclusion, the PD-1/PD-L1 axis is important for the pathogenesis of renal fibrosis.
7.4 PD-1/PD-L1 axis in other fibrosis
PD-1/PD-L1 axis is also involved in other fibrotic diseases with relatively low incidence. In systemic sclerosis associated fibrosis, the PD-1 level of Treg and γδT cells in peripheral blood of patients were significantly increased, and PD-1 co-expressed with another inhibitory immune checkpoint T cell immunoreceptor with Ig and ITIM domains (TIGIT) (185). In addition, compared with TIGIT and TIM-3, PD-1 plays a major role in the secretion of peripheral T cell cytokines in patients with systemic sclerosis (185). In upper airway tracheal stenosis associated fibrosis, PD-1 and PD-L1 expression were significantly upregulated in cricotracheal resection tissues of patients with iatrogenic laryngotracheal stenosis and idiopathic subglottic stenosis, and co-expressed with CD4 in peritracheal epithelial cells (186). In oral squamous cell carcinoma, the expression of PD-1 and PD-L1 in tumor tissues is significantly increased, and it is further increased when combined with oral mucosal fibrosis, and the level of PD-L1 is positively correlated with the occurrence of fibrosis (187). In PH-associated vascular fibrosis, the expression of PD-L1 is significantly increased and mediated by JAK/STAT1 pathway. Upregulation of PD-L1 can further activate pyroptosis of pulmonary artery smooth muscle cells and promote vascular fibrosis, eventually leading to PH (188). It can be concluded that immune and non-immune regulatory signals mediated by PD-1/PD-L1 pathway are involved in various fibrotic diseases.
8 Conclusions
In this review, we summarize the research progress of the PD-1/PD-L1 axis and discuss its potential role in fibrotic diseases. PD-1 and PD-L1 are important immune checkpoint molecules to maintain immune homeostasis, which are widely expressed in immune cells such as T cells, B cells, macrophages, monocytes, DC, and NK cells, and non-immune cells such as epithelial cells, fibroblasts, and endothelial cells. They are distributed in different forms on the cell membrane, cytoplasm, nucleus, and even extracellular, and exert diverse immunomodulatory functions. The expression of PD-1 and PD-L1 can be activated by a variety of pro-oncogene, inflammatory factor, and growth factor signals, which are regulated by transcription factors, kinases, and miRNAs at different levels including transcriptional, post-transcriptional, epigenetic, translational and post-translational. It has been found that PD-1/PD-L1 axis depends on different mechanisms to regulate a variety of important cellular activities such as T cell activation, proliferation, differentiation, cytokine secretion, apoptosis, macrophage phagocytosis, fibroblast migration and invasion, and tissue repair of MSCs. In addition, the dysregulation of PD-1 and PD-L1 expression has been confirmed in autoimmune diseases, cancers, chronic infections, and other chronic diseases. Importantly, immunomodulatory effects mediated by PD-1/PD-L1 pathway participate in a variety of physiological and pathological immune responses and are essential for immune system homeostasis. Given the promising role of PD-1/PD-L1 axis in biomedicine, its potential as a diagnostic biomarker and therapeutic target for various diseases has been greatly explored. The remarkable achievements of immune checkpoint inhibitors targeting the PD-1/PD-L1 pathway in the clinical tumor immunotherapy have attracted great attention to immune checkpoint blockade therapy, and also laid a foundation for its further application in the treatment of other immune-related diseases.
In recent years, preclinical and clinical studies have preliminarily confirmed that PD-1/PD-L1 signaling is involved in the progression of fibrotic diseases in various organs such as the lung, liver, and kidney. The main finding is that the PD-1/PD-L1 axis has a predominant profibrotic effect. Specifically, PD-1/PD-L1 pathway promotes fibrosis progression by inducing key fibrogenic processes such as macrophage polarization, T cell activation, and transdifferentiation of fibroblasts and epithelial cells. Meanwhile, high expression of PD-1 and PD-L1 has been found in fibrotic tissues of patients in clinical investigations. These evidences further emphasize the close relationship between PD-1/PD-L1 pathway and fibrosis response, and provides new ideas for elucidating the immunomodulatory mechanism of fibrotic diseases. On the other hand, this also suggests that PD-1/PD-L1 may serve as diagnostic and prognostic biomarkers and even potential therapeutic targets for fibrotic diseases. In murine models, blockade of the PD-1/PD-L1 pathway by gene knockout or monoclonal antibodies has been shown to alleviate fibrosis, which preliminarily identified this potential. With further exploration of this point, PD-1/PD-L1 inhibitors may be combined with currently approved anti-fibrosis drugs such as pirfenidone and nintedanib in the near future.
To further clarify the role of PD-1/PD-L1 axis in fibrosis and construct new immunotherapy strategies, it is necessary to fully understand the expression, activation, and regulation mechanisms of PD-1/PD-L1 signaling pathway in fibrosis. However, since the relevant research is still in the preliminary stage and the accumulation of evidence is not sufficient, there are still many important scientific issues that need to be further explored. First of all, the pathophysiology of many organ fibrosis types is comparable, yet each type also has its own distinctive features. Therefore, the general immunomodulatory and organ-specific functions of PD-1/PD-L1 signaling in various fibrosis conditions need to be interpreted separately. Secondly, the immunomodulatory effects of PD-1/PD-L1 axis have different performances in different cell types and different immune microenvironments, and fibrosis is a dynamic pathological process with multicellular and multifactorial interactions. Therefore, subsequent studies need to further explore the cell-specific functions of PD-1/PD-L1 signaling in different pathological stages of fibrosis. Thirdly, the initiation factors, regulatory mechanisms, and interactions of PD-1/PD-L1 signaling with other pathways in fibrotic diseases need to be further elucidated. Finally, in addition to PD-1 and PD-L1, inhibitory immune checkpoints such as TIM-3 and LAG3 have also been found to be involved in fibrotic diseases. As immunoregulatory factors with similar functions, whether the synergistic effects of other immune checkpoints should be further clarified when studying PD-1/PD-L1 signaling should be paid attention to.
All in all, PD-1/PD-L1 axis mediates key immunomodulatory and profibrotic signals in fibrosis response, comprehensive exploration and clarification of its specific functions and molecular mechanisms in fibrogenesis are expected to further elucidate the pathogenesis of fibrosis and build promising immunotherapy strategies for fibrotic diseases.
Author contributions
All authors listed have made a substantial, direct, and intellectual contribution to the work and approved it for publication.
Funding
This work is supported by the National Natural Science Foundation of China (no. U1904209).
Conflict of interest
The authors declare that the research was conducted in the absence of any commercial or financial relationships that could be construed as a potential conflict of interest.
Publisher’s note
All claims expressed in this article are solely those of the authors and do not necessarily represent those of their affiliated organizations, or those of the publisher, the editors and the reviewers. Any product that may be evaluated in this article, or claim that may be made by its manufacturer, is not guaranteed or endorsed by the publisher.
References
1. Henderson NC, Rieder F, Wynn TA. Fibrosis: from mechanisms to medicines. Nature (2020) 587(7835):555–66. doi: 10.1038/s41586-020-2938-9
2. Wynn TA, Ramalingam TR. Mechanisms of fibrosis: therapeutic translation for fibrotic disease. Nat Med (2012) 18(7):1028–40. doi: 10.1038/nm.2807
3. Klinkhammer BM, Floege J, Boor P. PDGF in organ fibrosis. Mol Aspects Med (2018) 62:44–62. doi: 10.1016/j.mam.2017.11.008
4. Rockey DC, Bell PD, Hill JA. Fibrosis–a common pathway to organ injury and failure. N Engl J Med (2015) 372(12):1138–49. doi: 10.1056/NEJMra1300575
5. Keir ME, Butte MJ, Freeman GJ, Sharpe AH. PD-1 and its ligands in tolerance and immunity. Annu Rev Immunol (2008) 26:677–704. doi: 10.1146/annurev.immunol.26.021607.090331
6. Sharpe AH, Pauken KE. The diverse functions of the PD1 inhibitory pathway. Nat Rev Immunol (2018) 18(3):153–67. doi: 10.1038/nri.2017.108
7. Qin W, Hu L, Zhang X, Jiang S, Li J, Zhang Z, et al. The diverse function of PD-1/PD-L pathway beyond Cancer. Front Immunol (2019) 10:2298. doi: 10.3389/fimmu.2019.02298
8. Dermani FK, Samadi P, Rahmani G, Kohlan AK, Najafi R. PD-1/PD-L1 immune checkpoint: potential target for cancer therapy. J Cell Physiol (2019) 234(2):1313–25. doi: 10.1002/jcp.27172
9. Budimir N, Thomas GD, Dolina JS, Salek-Ardakani S. Reversing T-cell exhaustion in cancer: lessons learned from PD-1/PD-L1 immune checkpoint Blockade. Cancer Immunol Res (2022) 10(2):146–53. doi: 10.1158/2326-6066.CIR-21-0515
10. Kuol N, Stojanovska L, Nurgali K, Apostolopoulos V. PD-1/PD-L1 in disease. Immunotherapy (2018) 10(2):149–60. doi: 10.2217/imt-2017-0120
11. Gianchecchi E, Delfino DV, Fierabracci A. Recent insights into the role of the PD-1/PD-L1 pathway in immunological tolerance and autoimmunity. Autoimmun Rev (2013) 12(11):1091–100. doi: 10.1016/j.autrev.2013.05.003
12. Cai J, Qi Q, Qian X, Han J, Zhu X, Zhang Q, et al. The role of PD-1/PD-L1 axis and macrophage in the progression and treatment of cancer. J Cancer Res Clin Oncol (2019) 145(6):1377–85. doi: 10.1007/s00432-019-02879-2
13. Wynn TA. Cellular and molecular mechanisms of fibrosis. J Pathol (2008) 214(2):199–210. doi: 10.1002/path.2277
14. Weiskirchen R, Weiskirchen S, Tacke F. Organ and tissue fibrosis: molecular signals, cellular mechanisms and translational implications. Mol Aspects Med (2019) 65:2–15. doi: 10.1016/j.mam.2018.06.003
15. Pakshir P, Hinz B. The big five in fibrosis: macrophages, myofibroblasts, matrix, mechanics, and miscommunication. Matrix Biol (2018) 68-69:81–93. doi: 10.1016/j.matbio.2018.01.019
16. Mack M. Inflammation and fibrosis. Matrix Biol (2018) 68-69:106–21. doi: 10.1016/j.matbio.2017.11.010
17. Pakshir P, Noskovicova N, Lodyga M, Son DO, Schuster R, Goodwin A, et al. The myofibroblast at a glance. J Cell Sci (2020) 133(13):jcs227900. doi: 10.1242/jcs.227900
18. Mack M, Yanagita M. Origin of myofibroblasts and cellular events triggering fibrosis. Kidney Int (2015) 87(2):297–307. doi: 10.1038/ki.2014.287
19. Kisseleva T. The origin of fibrogenic myofibroblasts in fibrotic liver. Hepatology (2017) 65(3):1039–43. doi: 10.1002/hep.28948
20. Herrera J, Henke CA, Bitterman PB. Extracellular matrix as a driver of progressive fibrosis. J Clin Invest (2018) 128(1):45–53. doi: 10.1172/JCI93557
21. Theocharis AD, Manou D, Karamanos NK. The extracellular matrix as a multitasking player in disease. FEBS J (2019) 286(15):2830–69. doi: 10.1111/febs.14818
22. Gyorfi AH, Matei AE, Distler JHW. Targeting TGF-beta signaling for the treatment of fibrosis. Matrix Biol (2018) 68-69:8–27. doi: 10.1016/j.matbio.2017.12.016
23. Luzina IG, Todd NW, Sundararajan S, Atamas SP. The cytokines of pulmonary fibrosis: much learned, much more to learn. Cytokine (2015) 74(1):88–100. doi: 10.1016/j.cyto.2014.11.008
24. Huang E, Peng N, Xiao F, Hu D, Wang X, Lu L. The roles of immune cells in the pathogenesis of Fibrosis. Int J Mol Sci (2020) 21(15):5203. doi: 10.3390/ijms21155203
25. Schaefer L. Decoding fibrosis: mechanisms and translational aspects. Matrix Biol (2018) 68-69:1–7. doi: 10.1016/j.matbio.2018.04.009
26. Wynn TA, Vannella KM. Macrophages in tissue repair, regeneration, and Fibrosis. Immunity (2016) 44(3):450–62. doi: 10.1016/j.immuni.2016.02.015
27. Zhang M, Zhang S. T Cells in fibrosis and fibrotic Diseases. Front Immunol (2020) 11:1142. doi: 10.3389/fimmu.2020.01142
28. Ulloa L, Doody J, Massague J. Inhibition of transforming growth factor-beta/SMAD signalling by the interferon-gamma/STAT pathway. Nature (1999) 397(6721):710–3. doi: 10.1038/17826
29. Zhang Y, Zhang Y, Gu W, Sun B. TH1/TH2 cell differentiation and molecular signals. Adv Exp Med Biol (2014) 841:15–44. doi: 10.1007/978-94-017-9487-9_2
30. Therrien FJ, Agharazii M, Lebel M, Lariviere R. Neutralization of tumor necrosis factor-alpha reduces renal fibrosis and hypertension in rats with renal failure. Am J Nephrol (2012) 36(2):151–61. doi: 10.1159/000340033
31. Osawa Y, Hoshi M, Yasuda I, Saibara T, Moriwaki H, Kozawa O. Tumor necrosis factor-alpha promotes cholestasis-induced liver fibrosis in the mouse through tissue inhibitor of metalloproteinase-1 production in hepatic stellate cells. PloS One (2013) 8(6):e65251. doi: 10.1371/journal.pone.0065251
32. Lee CG, Homer RJ, Zhu Z, Lanone S, Wang X, Koteliansky V, et al. Interleukin-13 induces tissue fibrosis by selectively stimulating and activating transforming growth factor beta(1). J Exp Med (2001) 194(6):809–21. doi: 10.1084/jem.194.6.809
33. Oriente A, Fedarko NS, Pacocha SE, Huang SK, Lichtenstein LM, Essayan DM. Interleukin-13 modulates collagen homeostasis in human skin and keloid fibroblasts. J Pharmacol Exp Ther (2000) 292(3):988–94.
34. Zhang MZ, Wang X, Wang Y, Niu A, Wang S, Zou C, et al. IL-4/IL-13-mediated polarization of renal macrophages/dendritic cells to an M2a phenotype is essential for recovery from acute kidney injury. Kidney Int (2017) 91(2):375–86. doi: 10.1016/j.kint.2016.08.020
35. Cortez DM, Feldman MD, Mummidi S, Valente AJ, Steffensen B, Vincenti M, et al. IL-17 stimulates MMP-1 expression in primary human cardiac fibroblasts via p38 MAPK- and ERK1/2-dependent C/EBP-beta , NF-kappaB, and AP-1 activation. Am J Physiol Heart Circ Physiol (2007) 293(6):H3356–3365. doi: 10.1152/ajpheart.00928.2007
36. Mohamed R, Jayakumar C, Chen F, Fulton D, Stepp D, Gansevoort RT, et al. Low-dose IL-17 therapy prevents and reverses diabetic nephropathy, metabolic syndrome, and associated organ Fibrosis. J Am Soc Nephrol (2016) 27(3):745–65. doi: 10.1681/ASN.2014111136
37. Liu F, Liu J, Weng D, Chen Y, Song L, He Q, et al. CD4+CD25+Foxp3+ regulatory T cells depletion may attenuate the development of silica-induced lung fibrosis in mice. PloS One (2010) 5(11):e15404. doi: 10.1371/journal.pone.0015404
38. Kitani A, Fuss I, Nakamura K, Kumaki F, Usui T, Strober W. Transforming growth factor (TGF)-beta1-producing regulatory T cells induce smad-mediated interleukin 10 secretion that facilitates coordinated immunoregulatory activity and amelioration of TGF-beta1-mediated fibrosis. J Exp Med (2003) 198(8):1179–88. doi: 10.1084/jem.20030917
39. Greenwald RJ, Freeman GJ, Sharpe AH. The B7 family revisited. Annu Rev Immunol (2005) 23:515–48. doi: 10.1146/annurev.immunol.23.021704.115611
40. Ishida Y, Agata Y, Shibahara K, Honjo T. Induced expression of PD-1, a novel member of the immunoglobulin gene superfamily, upon programmed cell death. EMBO J (1992) 11(11):3887–95. doi: 10.1002/j.1460-2075.1992.tb05481.x
41. Zhang X, Schwartz JC, Guo X, Bhatia S, Cao E, Lorenz M, et al. Structural and functional analysis of the costimulatory receptor programmed death-1. Immunity (2004) 20(3):337–47. doi: 10.1016/S1074-7613(04)00051-2
42. Dong H, Zhu G, Tamada K, Chen L. B7-H1, a third member of the B7 family, co-stimulates T-cell proliferation and interleukin-10 secretion. Nat Med (1999) 5(12):1365–9. doi: 10.1038/70932
43. Lin DY, Tanaka Y, Iwasaki M, Gittis AG, Su HP, Mikami B, et al. The PD-1/PD-L1 complex resembles the antigen-binding fv domains of antibodies and T cell receptors. Proc Natl Acad Sci U.S.A. (2008) 105(8):3011–6. doi: 10.1073/pnas.0712278105
44. Agata Y, Kawasaki A, Nishimura H, Ishida Y, Tsubata T, Yagita H, et al. Expression of the PD-1 antigen on the surface of stimulated mouse T and b lymphocytes. Int Immunol (1996) 8(5):765–72. doi: 10.1093/intimm/8.5.765
45. Barber DL, Wherry EJ, Masopust D, Zhu B, Allison JP, Sharpe AH, et al. Restoring function in exhausted CD8 T cells during chronic viral infection. Nature (2006) 439(7077):682–7. doi: 10.1038/nature04444
46. Sun Z, Fourcade J, Pagliano O, Chauvin JM, Sander C, Kirkwood JM, et al. IL10 and PD-1 cooperate to limit the activity of tumor-specific CD8+ T Cells. Cancer Res (2015) 75(8):1635–44. doi: 10.1158/0008-5472.CAN-14-3016
47. Park BV, Freeman ZT, Ghasemzadeh A, Chattergoon MA, Rutebemberwa A, Steigner J, et al. TGFbeta1-mediated SMAD3 enhances PD-1 expression on antigen-specific T cells in Cancer. Cancer Discovery (2016) 6(12):1366–81. doi: 10.1158/2159-8290.CD-15-1347
48. Yamazaki T, Akiba H, Iwai H, Matsuda H, Aoki M, Tanno Y, et al. Expression of programmed death 1 ligands by murine T cells and APC. J Immunol (2002) 169(10):5538–45. doi: 10.4049/jimmunol.169.10.5538
49. Sun C, Mezzadra R, Schumacher TN. Regulation and function of the PD-L1 Checkpoint. Immunity (2018) 48(3):434–52. doi: 10.1016/j.immuni.2018.03.014
50. Riley JL. PD-1 signaling in primary T cells. Immunol Rev (2009) 229(1):114–25. doi: 10.1111/j.1600-065X.2009.00767.x
51. Parry RV, Chemnitz JM, Frauwirth KA, Lanfranco AR, Braunstein I, Kobayashi SV, et al. CTLA-4 and PD-1 receptors inhibit T-cell activation by distinct mechanisms. Mol Cell Biol (2005) 25(21):9543–53. doi: 10.1128/MCB.25.21.9543-9553.2005
52. Yokosuka T, Takamatsu M, Kobayashi-Imanishi W, Hashimoto-Tane A, Azuma M, Saito T. Programmed cell death 1 forms negative costimulatory microclusters that directly inhibit T cell receptor signaling by recruiting phosphatase SHP2. J Exp Med (2012) 209(6):1201–17. doi: 10.1084/jem.20112741
53. Chemnitz JM, Parry RV, Nichols KE, June CH, Riley JL. SHP-1 and SHP-2 associate with immunoreceptor tyrosine-based switch motif of programmed death 1 upon primary human T cell stimulation, but only receptor ligation prevents T cell activation. J Immunol (2004) 173(2):945–54. doi: 10.4049/jimmunol.173.2.945
54. Hui E, Cheung J, Zhu J, Su X, Taylor MJ, Wallweber HA, et al. T Cell costimulatory receptor CD28 is a primary target for PD-1-mediated inhibition. Science (2017) 355(6332):1428–33. doi: 10.1126/science.aaf1292
55. Azuma T, Yao S, Zhu G, Flies AS, Flies SJ, Chen L. B7-H1 is a ubiquitous antiapoptotic receptor on cancer cells. Blood (2008) 111(7):3635–43. doi: 10.1182/blood-2007-11-123141
56. Chang CH, Qiu J, O'Sullivan D, Buck MD, Noguchi T, Curtis JD, et al. Metabolic competition in the tumor microenvironment is a driver of cancer Progression. Cell (2015) 162(6):1229–41. doi: 10.1016/j.cell.2015.08.016
57. Ying H, Zhang X, Duan Y, Lao M, Xu J, Yang H, et al. Non-cytomembrane PD-L1: an atypical target for cancer. Pharmacol Res (2021) 170:105741. doi: 10.1016/j.phrs.2021.105741
58. Xiong W, Gao Y, Wei W, Zhang J. Extracellular and nuclear PD-L1 in modulating cancer immunotherapy. Trends Cancer (2021) 7(9):837–46. doi: 10.1016/j.trecan.2021.03.003
59. Hassounah NB, Malladi VS, Huang Y, Freeman SS, Beauchamp EM, Koyama S, et al. Identification and characterization of an alternative cancer-derived PD-L1 splice variant. Cancer Immunol Immunother (2019) 68(3):407–20. doi: 10.1007/s00262-018-2284-z
60. Frigola X, Inman BA, Lohse CM, Krco CJ, Cheville JC, Thompson RH, et al. Identification of a soluble form of B7-H1 that retains immunosuppressive activity and is associated with aggressive renal cell carcinoma. Clin Cancer Res (2011) 17(7):1915–23. doi: 10.1158/1078-0432.CCR-10-0250
61. Zhang G, Hou J, Shi J, Yu G, Lu B, Zhang X. Soluble CD276 (B7-H3) is released from monocytes, dendritic cells and activated T cells and is detectable in normal human serum. Immunology (2008) 123(4):538–46. doi: 10.1111/j.1365-2567.2007.02723.x
62. Jeannin P, Magistrelli G, Aubry JP, Caron G, Gauchat JF, Renno T, et al. Soluble CD86 is a costimulatory molecule for human T lymphocytes. Immunity (2000) 13(3):303–12. doi: 10.1016/S1074-7613(00)00030-3
63. Nielsen C, Ohm-Laursen L, Barington T, Husby S, Lillevang ST. Alternative splice variants of the human PD-1 gene. Cell Immunol (2005) 235(2):109–16. doi: 10.1016/j.cellimm.2005.07.007
64. Ng KW, Attig J, Young GR, Ottina E, Papamichos SI, Kotsianidis I, et al. Soluble PD-L1 generated by endogenous retroelement exaptation is a receptor antagonist. Elife (2019) 8:e50256. doi: 10.7554/eLife.50256
65. Dezutter-Dambuyant C, Durand I, Alberti L, Bendriss-Vermare N, Valladeau-Guilemond J, Duc A, et al. A novel regulation of PD-1 ligands on mesenchymal stromal cells through MMP-mediated proteolytic cleavage. Oncoimmunology (2016) 5(3):e1091146. doi: 10.1080/2162402X.2015.1091146
66. Cheng S, Zheng J, Zhu J, Xie C, Zhang X, Han X, et al. PD-L1 gene polymorphism and high level of plasma soluble PD-L1 protein may be associated with non-small cell lung cancer. Int J Biol Markers (2015) 30(4):e364–368. doi: 10.5301/jbm.5000170
67. Song MY, Park SH, Nam HJ, Choi DH, Sung YC. Enhancement of vaccine-induced primary and memory CD8(+) T-cell responses by soluble PD-1. J Immunother (2011) 34(3):297–306. doi: 10.1097/CJI.0b013e318210ed0e
68. Geng H, Zhang GM, Xiao H, Yuan Y, Li D, Zhang H, et al. HSP70 vaccine in combination with gene therapy with plasmid DNA encoding sPD-1 overcomes immune resistance and suppresses the progression of pulmonary metastatic melanoma. Int J Cancer (2006) 118(11):2657–64. doi: 10.1002/ijc.21795
69. Chen G, Huang AC, Zhang W, Zhang G, Wu M, Xu W, et al. Exosomal PD-L1 contributes to immunosuppression and is associated with anti-PD-1 response. Nature (2018) 560(7718):382–6. doi: 10.1038/s41586-018-0392-8
70. Yang Y, Li CW, Chan LC, Wei Y, Hsu JM, Xia W, et al. Exosomal PD-L1 harbors active defense function to suppress T cell killing of breast cancer cells and promote tumor growth. Cell Res (2018) 28(8):862–4. doi: 10.1038/s41422-018-0060-4
71. Chen L, Deng H, Lu M, Xu B, Wang Q, Jiang J, et al. B7-H1 expression associates with tumor invasion and predicts patient's survival in human esophageal cancer. Int J Clin Exp Pathol (2014) 7(9):6015–23.
72. Dong H, Strome SE, Salomao DR, Tamura H, Hirano F, Flies DB, et al. Tumor-associated B7-H1 promotes T-cell apoptosis: a potential mechanism of immune evasion. Nat Med (2002) 8(8):793–800. doi: 10.1038/nm730
73. Fan Y, Che X, Qu J, Hou K, Wen T, Li Z, et al. Exosomal PD-L1 retains immunosuppressive activity and is associated with gastric cancer Prognosis. Ann Surg Oncol (2019) 26(11):3745–55. doi: 10.1245/s10434-019-07431-7
74. Poggio M, Hu T, Pai CC, Chu B, Belair CD, Chang A, et al. Suppression of exosomal PD-L1 induces systemic anti-tumor immunity and Memory. Cell (2019) 177(2):414–427 e413. doi: 10.1016/j.cell.2019.02.016
75. Qiu Y, Yang Y, Yang R, Liu C, Hsu JM, Jiang Z, et al. Activated T cell-derived exosomal PD-1 attenuates PD-L1-induced immune dysfunction in triple-negative breast cancer. Oncogene (2021) 40(31):4992–5001. doi: 10.1038/s41388-021-01896-1
76. Tucci M, Passarelli A, Mannavola F, Stucci LS, Ascierto PA, Capone M, et al. Serum exosomes as predictors of clinical response to ipilimumab in metastatic melanoma. Oncoimmunology (2018) 7(2):e1387706. doi: 10.1080/2162402X.2017.1387706
77. Gao Y, Nihira NT, Bu X, Chu C, Zhang J, Kolodziejczyk A, et al. Acetylation-dependent regulation of PD-L1 nuclear translocation dictates the efficacy of anti-PD-1 immunotherapy. Nat Cell Biol (2020) 22(9):1064–75. doi: 10.1038/s41556-020-0562-4
78. Du W, Zhu J, Zeng Y, Liu T, Zhang Y, Cai T, et al. KPNB1-mediated nuclear translocation of PD-L1 promotes non-small cell lung cancer cell proliferation via the Gas6/MerTK signaling pathway. Cell Death Differ (2021) 28(4):1284–300. doi: 10.1038/s41418-020-00651-5
79. Yu J, Qin B, Moyer AM, Nowsheen S, Tu X, Dong H, et al. Regulation of sister chromatid cohesion by nuclear PD-L1. Cell Res (2020) 30(7):590–601. doi: 10.1038/s41422-020-0315-8
80. Parra ER, Villalobos P, Rodriguez-Canales J. The multiple faces of programmed cell death ligand 1 expression in malignant and nonmalignant Cells. Appl Immunohistochem Mol Morphol (2019) 27(4):287–94. doi: 10.1097/PAI.0000000000000602
81. Chin YT, Wei PL, Ho Y, Nana AW, Changou CA, Chen YR, et al. Thyroxine inhibits resveratrol-caused apoptosis by PD-L1 in ovarian cancer cells. Endocr Relat Cancer (2018) 25(5):533–45. doi: 10.1530/ERC-17-0376
82. Chen YR, Chen YS, Chin YT, Li ZL, Shih YJ, Yang YSH, et al. Thyroid hormone-induced expression of inflammatory cytokines interfere with resveratrol-induced anti-proliferation of oral cancer cells. Food Chem Toxicol (2019) 132:110693. doi: 10.1016/j.fct.2019.110693
83. Tu X, Qin B, Zhang Y, Zhang C, Kahila M, Nowsheen S, et al. PD-L1 (B7-H1) competes with the RNA exosome to regulate the DNA damage response and can be targeted to sensitize to radiation or Chemotherapy. Mol Cell (2019) 74(6):1215–1226 e1214. doi: 10.1016/j.molcel.2019.04.005
84. Raimondi G, Shufesky WJ, Tokita D, Morelli AE, Thomson AW. Regulated compartmentalization of programmed cell death-1 discriminates CD4+CD25+ resting regulatory T cells from activated T cells. J Immunol (2006) 176(5):2808–16. doi: 10.4049/jimmunol.176.5.2808
85. Pentcheva-Hoang T, Chen L, Pardoll DM, Allison JP. Programmed death-1 concentration at the immunological synapse is determined by ligand affinity and availability. Proc Natl Acad Sci U.S.A. (2007) 104(45):17765–70. doi: 10.1073/pnas.0708767104
86. Takahashi R, Sato Y, Kimishima M, Shiohara T, Ohyama M. Intracellular accumulation of PD-1 molecules in circulating T lymphocytes in advanced malignant melanoma: an implication for immune evasion mechanism. Int J Clin Oncol (2020) 25(10):1861–9. doi: 10.1007/s10147-020-01732-8
87. Cancer Genome Atlas Research Network. Comprehensive molecular characterization of gastric adenocarcinoma. Nature (2014) 513(7517):202–9. doi: 10.1038/nature13480
88. Ikeda S, Okamoto T, Okano S, Umemoto Y, Tagawa T, Morodomi Y, et al. PD-L1 is upregulated by simultaneous amplification of the PD-L1 and JAK2 genes in non-small cell lung Cancer. J Thorac Oncol (2016) 11(1):62–71. doi: 10.1016/j.jtho.2015.09.010
89. Kataoka K, Shiraishi Y, Takeda Y, Sakata S, Matsumoto M, Nagano S, et al. Aberrant PD-L1 expression through 3'-UTR disruption in multiple cancers. Nature (2016) 534(7607):402–6. doi: 10.1038/nature18294
90. Green MR, Monti S, Rodig SJ, Juszczynski P, Currie T, O'Donnell E, et al. Integrative analysis reveals selective 9p24.1 amplification, increased PD-1 ligand expression, and further induction via JAK2 in nodular sclerosing Hodgkin lymphoma and primary mediastinal large b-cell lymphoma. Blood (2010) 116(17):3268–77. doi: 10.1182/blood-2010-05-282780
91. Casey SC, Tong L, Li Y, Do R, Walz S, Fitzgerald KN, et al. MYC regulates the antitumor immune response through CD47 and PD-L1. Science (2016) 352(6282):227–31. doi: 10.1126/science.aac9935
92. Marzec M, Zhang Q, Goradia A, Raghunath PN, Liu X, Paessler M, et al. Oncogenic kinase NPM/ALK induces through STAT3 expression of immunosuppressive protein CD274 (PD-L1, B7-H1). Proc Natl Acad Sci USA (2008) 105(52):20852–7. doi: 10.1073/pnas.0810958105
93. Barsoum IB, Smallwood CA, Siemens DR, Graham CH. A mechanism of hypoxia-mediated escape from adaptive immunity in cancer cells. Cancer Res (2014) 74(3):665–74. doi: 10.1158/0008-5472.CAN-13-0992
94. Gowrishankar K, Gunatilake D, Gallagher SJ, Tiffen J, Rizos H, Hersey P. Inducible but not constitutive expression of PD-L1 in human melanoma cells is dependent on activation of NF-kappaB. PloS One (2015) 10(4):e0123410. doi: 10.1371/journal.pone.0123410
95. Lee SJ, Jang BC, Lee SW, Yang YI, Suh SI, Park YM, et al. Interferon regulatory factor-1 is prerequisite to the constitutive expression and IFN-gamma-induced upregulation of B7-H1 (CD274). FEBS Lett (2006) 580(3):755–62. doi: 10.1016/j.febslet.2005.12.093
96. Ou JN, Wiedeman AE, Stevens AM. TNF-alpha and TGF-beta counter-regulate PD-L1 expression on monocytes in systemic lupus erythematosus. Sci Rep (2012) 2:295. doi: 10.1038/srep00295
97. Song S, Yuan P, Wu H, Chen J, Fu J, Li P, et al. Dendritic cells with an increased PD-L1 by TGF-beta induce T cell anergy for the cytotoxicity of hepatocellular carcinoma cells. Int Immunopharmacol (2014) 20(1):117–23. doi: 10.1016/j.intimp.2014.02.027
98. Oestreich KJ, Yoon H, Ahmed R, Boss JM. NFATc1 regulates PD-1 expression upon T cell activation. J Immunol (2008) 181(7):4832–9. doi: 10.4049/jimmunol.181.7.4832
99. Staron MM, Gray SM, Marshall HD, Parish IA, Chen JH, Perry CJ, et al. The transcription factor FoxO1 sustains expression of the inhibitory receptor PD-1 and survival of antiviral CD8(+) T cells during chronic infection. Immunity (2014) 41(5):802–14. doi: 10.1016/j.immuni.2014.10.013
100. Xiao G, Deng A, Liu H, Ge G, Liu X. Activator protein 1 suppresses antitumor T-cell function via the induction of programmed death 1. Proc Natl Acad Sci U.S.A. (2012) 109(38):15419–24. doi: 10.1073/pnas.1206370109
101. Antonangeli F, Natalini A, Garassino MC, Sica A, Santoni A, Di Rosa F. Regulation of PD-L1 expression by NF-kappaB in Cancer. Front Immunol (2020) 11:584626. doi: 10.3389/fimmu.2020.584626
102. Lu P, Youngblood BA, Austin JW, Mohammed AU, Butler R, Ahmed R, et al. Blimp-1 represses CD8 T cell expression of PD-1 using a feed-forward transcriptional circuit during acute viral infection. J Exp Med (2014) 211(3):515–27. doi: 10.1084/jem.20130208
103. Kao C, Oestreich KJ, Paley MA, Crawford A, Angelosanto JM, Ali MA, et al. Transcription factor T-bet represses expression of the inhibitory receptor PD-1 and sustains virus-specific CD8+ T cell responses during chronic infection. Nat Immunol (2011) 12(7):663–71. doi: 10.1038/ni.2046
104. Gong AY, Zhou R, Hu G, Li X, Splinter PL, O'Hara SP, et al. MicroRNA-513 regulates B7-H1 translation and is involved in IFN-gamma-induced B7-H1 expression in cholangiocytes. J Immunol (2009) 182(3):1325–33. doi: 10.4049/jimmunol.182.3.1325
105. Chen L, Gibbons DL, Goswami S, Cortez MA, Ahn YH, Byers LA, et al. Metastasis is regulated via microRNA-200/ZEB1 axis control of tumour cell PD-L1 expression and intratumoral immunosuppression. Nat Commun (2014) 5:5241. doi: 10.1038/ncomms6241
106. Cha JH, Chan LC, Li CW, Hsu JL, Hung MC. Mechanisms controlling PD-L1 expression in Cancer. Mol Cell (2019) 76(3):359–70. doi: 10.1016/j.molcel.2019.09.030
107. Zhu J, Chen L, Zou L, Yang P, Wu R, Mao Y, et al. MiR-20b, -21, and -130b inhibit PTEN expression resulting in B7-H1 over-expression in advanced colorectal cancer. Hum Immunol (2014) 75(4):348–53. doi: 10.1016/j.humimm.2014.01.006
108. Coelho MA, de Carne Trecesson S, Rana S, Zecchin D, Moore C, Molina-Arcas M, et al. Oncogenic RAS signaling promotes tumor immunoresistance by stabilizing PD-L1 mRNA. Immunity (2017) 47(6):1083–1099 e1086. doi: 10.1016/j.immuni.2017.11.016
109. Zhang G, Li N, Li Z, Zhu Q, Li F, Yang C, et al. microRNA-4717 differentially interacts with its polymorphic target in the PD1 3' untranslated region: a mechanism for regulating PD-1 expression and function in HBV-associated liver diseases. Oncotarget (2015) 6(22):18933–44. doi: 10.18632/oncotarget.3662
110. Li Q, Johnston N, Zheng X, Wang H, Zhang X, Gao D, et al. miR-28 modulates exhaustive differentiation of T cells through silencing programmed cell death-1 and regulating cytokine secretion. Oncotarget (2016) 7(33):53735–50. doi: 10.18632/oncotarget.10731
111. Wang J, Zhao X, Wang Y, Ren F, Sun D, Yan Y, et al. circRNA-002178 act as a ceRNA to promote PDL1/PD1 expression in lung adenocarcinoma. Cell Death Dis (2020) 11(1):32. doi: 10.1038/s41419-020-2230-9
112. Huang F, Wang B, Zeng J, Sang S, Lei J, Lu Y. MicroRNA-374b inhibits liver cancer progression via down regulating programmed cell death-1 expression on cytokine-induced killer cells. Oncol Lett (2018) 15(4):4797–804. doi: 10.3892/ol.2018.7951
113. Wei J, Nduom EK, Kong LY, Hashimoto Y, Xu S, Gabrusiewicz K, et al. MiR-138 exerts anti-glioma efficacy by targeting immune checkpoints. Neuro Oncol (2016) 18(5):639–48. doi: 10.1093/neuonc/nov292
114. Yang J, Liu R, Deng Y, Qian J, Lu Z, Wang Y, et al. MiR-15a/16 deficiency enhances anti-tumor immunity of glioma-infiltrating CD8+ T cells through targeting mTOR. Int J Cancer (2017) 141(10):2082–92. doi: 10.1002/ijc.30912
115. Hogg SJ, Vervoort SJ, Deswal S, Ott CJ, Li J, Cluse LA, et al. BET-bromodomain inhibitors engage the host immune system and regulate expression of the immune checkpoint ligand PD-L1. Cell Rep (2017) 18(9):2162–74. doi: 10.1016/j.celrep.2017.02.011
116. Deng S, Hu Q, Zhang H, Yang F, Peng C, Huang C. HDAC3 inhibition upregulates PD-L1 expression in b-cell lymphomas and augments the efficacy of anti-PD-L1 Therapy. Mol Cancer Ther (2019) 18(5):900–8. doi: 10.1158/1535-7163.MCT-18-1068
117. Lu C, Paschall AV, Shi H, Savage N, Waller JL, Sabbatini ME, et al. The MLL1-H3K4me3 axis-mediated PD-L1 expression and pancreatic cancer immune Evasion. J Natl Cancer Inst (2017) 109(6):djw283. doi: 10.1093/jnci/djw283
118. Toyokawa G, Takada K, Tagawa T, Hamamoto R, Yamada Y, Shimokawa M, et al. A positive correlation between the EZH2 and PD-L1 expression in resected lung Adenocarcinomas. Ann Thorac Surg (2019) 107(2):393–400. doi: 10.1016/j.athoracsur.2018.08.056
119. Youngblood B, Oestreich KJ, Ha SJ, Duraiswamy J, Akondy RS, West EE, et al. Chronic virus infection enforces demethylation of the locus that encodes PD-1 in antigen-specific CD8(+) T cells. Immunity (2011) 35(3):400–12. doi: 10.1016/j.immuni.2011.06.015
120. McPherson RC, Konkel JE, Prendergast CT, Thomson JP, Ottaviano R, Leech MD, et al. Epigenetic modification of the PD-1 (Pdcd1) promoter in effector CD4(+) T cells tolerized by peptide immunotherapy. Elife (2014) 3:e03416. doi: 10.7554/eLife.03416
121. Li CW, Lim SO, Xia W, Lee HH, Chan LC, Kuo CW, et al. Glycosylation and stabilization of programmed death ligand-1 suppresses T-cell activity. Nat Commun (2016) 7:12632. doi: 10.1038/ncomms12632
122. Shao B, Li CW, Lim SO, Sun L, Lai YJ, Hou J, et al. Deglycosylation of PD-L1 by 2-deoxyglucose reverses PARP inhibitor-induced immunosuppression in triple-negative breast cancer. Am J Cancer Res (2018) 8(9):1837–46.
123. Tan S, Zhang H, Chai Y, Song H, Tong Z, Wang Q, et al. An unexpected n-terminal loop in PD-1 dominates binding by nivolumab. Nat Commun (2017) 8:14369. doi: 10.1038/ncomms14369
124. Sun L, Li CW, Chung EM, Yang R, Kim YS, Park AH, et al. Targeting glycosylated PD-1 induces potent antitumor Immunity. Cancer Res (2020) 80(11):2298–310. doi: 10.1158/0008-5472.CAN-19-3133
125. Li CW, Lim SO, Chung EM, Kim YS, Park AH, Yao J, et al. Eradication of triple-negative breast cancer cells by targeting glycosylated PD-L1. Cancer Cell (2018) 33(2):187–201 e110. doi: 10.1016/j.ccell.2018.01.009
126. Sheppard KA, Fitz LJ, Lee JM, Benander C, George JA, Wooters J, et al. PD-1 inhibits T-cell receptor induced phosphorylation of the ZAP70/CD3zeta signalosome and downstream signaling to PKCtheta. FEBS Lett (2004) 574(1-3):37–41. doi: 10.1016/j.febslet.2004.07.083
127. Fernandes RA, Su L, Nishiga Y, Ren J, Bhuiyan AM, Cheng N, et al. Immune receptor inhibition through enforced phosphatase recruitment. Nature (2020) 586(7831):779–84. doi: 10.1038/s41586-020-2851-2
128. Cha JH, Yang WH, Xia W, Wei Y, Chan LC, Lim SO, et al. Metformin promotes antitumor immunity via endoplasmic-Reticulum-Associated degradation of PD-L1. Mol Cell (2018) 71(4):606–620 e607. doi: 10.1016/j.molcel.2018.07.030
129. Burr ML, Sparbier CE, Chan YC, Williamson JC, Woods K, Beavis PA, et al. CMTM6 maintains the expression of PD-L1 and regulates anti-tumour immunity. Nature (2017) 549(7670):101–5. doi: 10.1038/nature23643
130. Meng X, Liu X, Guo X, Jiang S, Chen T, Hu Z, et al. FBXO38 mediates PD-1 ubiquitination and regulates anti-tumour immunity of T cells. Nature (2018) 564(7734):130–5. doi: 10.1038/s41586-018-0756-0
131. Lyle C, Richards S, Yasuda K, Napoleon MA, Walker J, Arinze N, et al. C-cbl targets PD-1 in immune cells for proteasomal degradation and modulates colorectal tumor growth. Sci Rep (2019) 9(1):20257. doi: 10.1038/s41598-019-56208-1
132. Zhou XA, Zhou J, Zhao L, Yu G, Zhan J, Shi C, et al. KLHL22 maintains PD-1 homeostasis and prevents excessive T cell suppression. Proc Natl Acad Sci USA (2020) 117(45):28239–50. doi: 10.1073/pnas.2004570117
133. Schwartz RH. T Cell anergy. Annu Rev Immunol (2003) 21:305–34. doi: 10.1146/annurev.immunol.21.120601.141110
134. Fife BT, Bluestone JA. Control of peripheral T-cell tolerance and autoimmunity via the CTLA-4 and PD-1 pathways. Immunol Rev (2008) 224:166–82. doi: 10.1111/j.1600-065X.2008.00662.x
135. Farhood B, Najafi M, Mortezaee K. CD8(+) cytotoxic T lymphocytes in cancer immunotherapy: a review. J Cell Physiol (2019) 234(6):8509–21. doi: 10.1002/jcp.27782
136. Raedler LA. Keytruda (Pembrolizumab): first PD-1 inhibitor approved for previously treated unresectable or metastatic melanoma. Am Health Drug Benefits (2015) 8(Spec Feature):96–100.
137. Page DB, Postow MA, Callahan MK, Allison JP, Wolchok JD. Immune modulation in cancer with antibodies. Annu Rev Med (2014) 65:185–202. doi: 10.1146/annurev-med-092012-112807
138. Gordon SR, Maute RL, Dulken BW, Hutter G, George BM, McCracken MN, et al. PD-1 expression by tumour-associated macrophages inhibits phagocytosis and tumour immunity. Nature (2017) 545(7655):495–9. doi: 10.1038/nature22396
139. Chen W, Wang J, Jia L, Liu J, Tian Y. Attenuation of the programmed cell death-1 pathway increases the M1 polarization of macrophages induced by zymosan. Cell Death Dis (2016) 7(2):e2115. doi: 10.1038/cddis.2016.33
140. Cho HY, Choi EK, Lee SW, Jung KO, Seo SK, Choi IW, et al. Programmed death-1 receptor negatively regulates LPS-mediated IL-12 production and differentiation of murine macrophage RAW264.7 cells. Immunol Lett (2009) 127(1):39–47. doi: 10.1016/j.imlet.2009.08.011
141. Ma CJ, Ni L, Zhang Y, Zhang CL, Wu XY, Atia AN, et al. PD-1 negatively regulates interleukin-12 expression by limiting STAT-1 phosphorylation in monocytes/macrophages during chronic hepatitis c virus infection. Immunology (2011) 132(3):421–31. doi: 10.1111/j.1365-2567.2010.03382.x
142. Jiang Y, Zhan H. Communication between EMT and PD-L1 signaling: new insights into tumor immune evasion. Cancer Lett (2020) 468:72–81. doi: 10.1016/j.canlet.2019.10.013
143. Dongre A, Rashidian M, Reinhardt F, Bagnato A, Keckesova Z, Ploegh HL, et al. Epithelial-to-Mesenchymal transition contributes to immunosuppression in breast Carcinomas. Cancer Res (2017) 77(15):3982–9. doi: 10.1158/0008-5472.CAN-16-3292
144. Ueno T, Tsuchikawa T, Hatanaka KC, Hatanaka Y, Mitsuhashi T, Nakanishi Y, et al. Prognostic impact of programmed cell death ligand 1 (PD-L1) expression and its association with epithelial-mesenchymal transition in extrahepatic cholangiocarcinoma. Oncotarget (2018) 9(28):20034–47. doi: 10.18632/oncotarget.25050
145. Kumar S, Davra V, Obr AE, Geng K, Wood TL, De Lorenzo MS, et al. Crk adaptor protein promotes PD-L1 expression, EMT and immune evasion in a murine model of triple-negative breast cancer. Oncoimmunology (2017) 7(1):e1376155. doi: 10.1080/2162402X.2017.1376155
146. Bouillez A, Rajabi H, Jin C, Samur M, Tagde A, Alam M, et al. MUC1-c integrates PD-L1 induction with repression of immune effectors in non-small-cell lung cancer. Oncogene (2017) 36(28):4037–46. doi: 10.1038/onc.2017.47
147. Alsuliman A, Colak D, Al-Harazi O, Fitwi H, Tulbah A, Al-Tweigeri T, et al. Bidirectional crosstalk between PD-L1 expression and epithelial to mesenchymal transition: significance in claudin-low breast cancer cells. Mol Cancer (2015) 14:149. doi: 10.1186/s12943-015-0421-2
148. Qiu XY, Hu DX, Chen WQ, Chen RQ, Qian SR, Li CY, et al. PD-L1 confers glioblastoma multiforme malignancy via ras binding and Ras/Erk/EMT activation. Biochim Biophys Acta Mol Basis Dis (2018) 1864(5 Pt A):1754–69. doi: 10.1016/j.bbadis.2018.03.002
149. Wang Y, Wang H, Zhao Q, Xia Y, Hu X, Guo J. PD-L1 induces epithelial-to-mesenchymal transition via activating SREBP-1c in renal cell carcinoma. Med Oncol (2015) 32(8):212. doi: 10.1007/s12032-015-0655-2
150. Guo X, Sunil C, Adeyanju O, Parker A, Huang S, Ikebe M, et al. PD-L1 mediates lung fibroblast to myofibroblast transition through Smad3 and beta-catenin signaling pathways. Sci Rep (2022) 12(1):3053. doi: 10.1038/s41598-022-07044-3
151. Lu Y, Zhong W, Liu Y, Chen W, Zhang J, Zeng Z, et al. Anti-PD-L1 antibody alleviates pulmonary fibrosis by inducing autophagy via inhibition of the PI3K/Akt/mTOR pathway. Int Immunopharmacol (2022) 104:108504. doi: 10.1016/j.intimp.2021.108504
152. Geng Y, Liu X, Liang J, Habiel DM, Kulur V, Coelho AL, et al. PD-L1 on invasive fibroblasts drives fibrosis in a humanized model of idiopathic pulmonary fibrosis. JCI Insight (2019) 4(6):e125326. doi: 10.1172/jci.insight.125326
153. Kang JH, Jung MY, Choudhury M, Leof EB. Transforming growth factor beta induces fibroblasts to express and release the immunomodulatory protein PD-L1 into extracellular vesicles. FASEB J (2020) 34(2):2213–26. doi: 10.1096/fj.201902354R
154. Cui L, Chen SY, Lerbs T, Lee JW, Domizi P, Gordon S, et al. Activation of JUN in fibroblasts promotes pro-fibrotic programme and modulates protective immunity. Nat Commun (2020) 11(1):2795. doi: 10.1038/s41467-020-16466-4
155. Lan Y, Moustafa M, Knoll M, Xu C, Furkel J, Lazorchak A, et al. Simultaneous targeting of TGF-beta/PD-L1 synergizes with radiotherapy by reprogramming the tumor microenvironment to overcome immune evasion. Cancer Cell (2021) 39(10):1388–1403 e1310. doi: 10.1016/j.ccell.2021.08.008
156. Tamosiuniene R, Manouvakhova O, Mesange P, Saito T, Qian J, Sanyal M, et al. Dominant role for regulatory T cells in protecting females against pulmonary Hypertension. Circ Res (2018) 122(12):1689–702. doi: 10.1161/CIRCRESAHA.117.312058
157. Krupnick AS, Gelman AE, Barchet W, Richardson S, Kreisel FH, Turka LA, et al. Murine vascular endothelium activates and induces the generation of allogeneic CD4+25+Foxp3+ regulatory T cells. J Immunol (2005) 175(10):6265–70. doi: 10.4049/jimmunol.175.10.6265
158. Nakayama Y, Bromberg JS. Lymphotoxin-beta receptor blockade induces inflammation and fibrosis in tolerized cardiac allografts. Am J Transplant (2012) 12(9):2322–34. doi: 10.1111/j.1600-6143.2012.04090.x
159. Ravaglia C, Doglioni C, Chilosi M, Piciucchi S, Dubini A, Rossi G, et al. Clinical, radiological and pathological findings in patients with persistent lung disease following SARS-CoV-2 infection. Eur Respir J (2022) 60(4):2102411. doi: 10.1183/13993003.02411-2021
160. Ni K, Liu M, Zheng J, Wen L, Chen Q, Xiang Z, et al. PD-1/PD-L1 pathway mediates the alleviation of pulmonary fibrosis by human mesenchymal stem cells in humanized Mice. Am J Respir Cell Mol Biol (2018) 58(6):684–95. doi: 10.1165/rcmb.2017-0326OC
161. Celada LJ, Kropski JA, Herazo-Maya JD, Luo W, Creecy A, Abad AT, et al. PD-1 up-regulation on CD4(+) T cells promotes pulmonary fibrosis through STAT3-mediated IL-17A and TGF-beta1 production. Sci Transl Med (2018) 10(460):eaar8356. doi: 10.1126/scitranslmed.aar8356
162. Jovanovic D, Roksandic Milenkovic M, Kotur Stevuljevic J, Markovic J, Ceriman V, Kontic M, et al. Membrane PD-L1 expression and soluble PD-L1 plasma levels in idiopathic pulmonary fibrosis-a pilot study. J Thorac Dis (2018) 10(12):6660–9. doi: 10.21037/jtd.2018.11.16
163. Roksandic Milenkovic M, Klisic A, Ceriman V, Kotur Stevuljevic J, Savic Vujovic K, Mirkov D, et al. Oxidative stress and inflammation parameters-novel biomarkers for idiopathic pulmonary fibrosis. Eur Rev Med Pharmacol Sci (2022) 26(3):927–34. doi: 10.26355/eurrev_202202_28002
164. Shukla SD, Budden KF, Neal R, Hansbro PM. Microbiome effects on immunity, health and disease in the lung. Clin Transl Immunol (2017) 6(3):e133. doi: 10.1038/cti.2017.6
165. Amati F, Stainer A, Mantero M, Gramegna A, Simonetta E, Suigo G, et al. Lung microbiome in idiopathic pulmonary fibrosis and other interstitial lung Diseases. Int J Mol Sci (2022) 23(2):977. doi: 10.3390/ijms23020977
166. Gollwitzer ES, Saglani S, Trompette A, Yadava K, Sherburn R, McCoy KD, et al. Lung microbiota promotes tolerance to allergens in neonates via PD-L1. Nat Med (2014) 20(6):642–7. doi: 10.1038/nm.3568
167. Jang HJ, Choi JY, Kim K, Yong SH, Kim YW, Kim SY, et al. Relationship of the lung microbiome with PD-L1 expression and immunotherapy response in lung cancer. Respir Res (2021) 22(1):322. doi: 10.1186/s12931-021-01919-1
168. Masuhiro K, Tamiya M, Fujimoto K, Koyama S, Naito Y, Osa A, et al. Bronchoalveolar lavage fluid reveals factors contributing to the efficacy of PD-1 blockade in lung cancer. JCI Insight (2022) 7(9):e157915. doi: 10.1172/jci.insight.157915
169. Jiang A, Liu N, Wang J, Zheng X, Ren M, Zhang W, et al. The role of PD-1/PD-L1 axis in idiopathic pulmonary fibrosis: friend or foe? Front Immunol (2022) 13:1022228. doi: 10.3389/fimmu.2022.1022228
170. Rocha MC, Santos LM, Bagatin E, Cohen Tervaert JW, Damoiseaux JG, Lido AV, et al. Genetic polymorphisms and surface expression of CTLA-4 and PD-1 on T cells of silica-exposed workers. Int J Hyg Environ Health (2012) 215(6):562–9. doi: 10.1016/j.ijheh.2011.10.010
171. Qiu M, Chen Y, Ye Q. Downregulation of the PD-1/PD-Ls pathway in peripheral cells correlates with asbestosis severity. BMC Pulm Med (2021) 21(1):175. doi: 10.1186/s12890-021-01531-5
172. Zhao Y, Hao C, Li M, Qu Y, Guo Y, Deng X, et al. PD-1/PD-L1 inhibitor ameliorates silica-induced pulmonary fibrosis by maintaining systemic immune homeostasis. BioMed Pharmacother (2022) 148:112768. doi: 10.1016/j.biopha.2022.112768
173. Ke MY, Xu T, Fang Y, Ye YP, Li ZJ, Ren FG, et al. Liver fibrosis promotes immune escape in hepatocellular carcinoma via GOLM1-mediated PD-L1 upregulation. Cancer Lett (2021) 513:14–25. doi: 10.1016/j.canlet.2021.05.007
174. Zhou L, Li X, Huang X, Chen L, Gu L, Huang Y. Soluble programmed death-1 is a useful indicator for inflammatory and fibrosis severity in chronic hepatitis B. J Viral Hepat (2019) 26(7):795–802. doi: 10.1111/jvh.13055
175. Liu X, Zhou J, Wu H, Chen S, Zhang L, Tang W, et al. Fibrotic immune microenvironment remodeling mediates superior anti-tumor efficacy of a nano-PD-L1 trap in hepatocellular carcinoma. Mol Ther (2022) 31(1):119–33. doi: 10.1016/j.ymthe.2022.09.012
176. Huang N, Zhou R, Chen H, Zhang S, Li J, Wei W, et al. Splenic CD4(+) and CD8(+) T-cells highly expressed PD-1 and Tim-3 in cirrhotic patients with HCV infection and portal hypertension. Int J Immunopathol Pharmacol (2021) 35:20587384211061051. doi: 10.1177/20587384211061051
177. Xiang M, Liu T, Tian C, Ma K, Gou J, Huang R, et al. Kinsenoside attenuates liver fibro-inflammation by suppressing dendritic cells via the PI3K-AKT-FoxO1 pathway. Pharmacol Res (2022) 177:106092. doi: 10.1016/j.phrs.2022.106092
178. Palamaris K, Alexandris D, Stylianou K, Giatras I, Stofas A, Kaitatzoglou C, et al. Immune checkpoint inhibitors' associated renal toxicity: a series of 12 Cases. J Clin Med (2022) 11(16):4786. doi: 10.3390/jcm11164786
179. Nakatani Y, Kawakami H, Ichikawa M, Yamamoto S, Otsuka Y, Mashiko A, et al. Nivolumab-induced acute granulomatous tubulointerstitial nephritis in a patient with gastric cancer. Invest New Drugs (2018) 36(4):726–31. doi: 10.1007/s10637-018-0596-7
180. Tominaga K, Takeuchi K, Takakuma S, Sakamoto E, Hatanaka S, Kajimoto Y, et al. Immune checkpoint inhibitors associated granulomatous small vessel vasculitis accompanied with tubulointerstitial nephritis: a case report. BMC Nephrol (2023) 24(1):48. doi: 10.1186/s12882-023-03091-8
181. Xipell M, Victoria I, Hoffmann V, Villarreal J, Garcia-Herrera A, Reig O, et al. Acute tubulointerstitial nephritis associated with atezolizumab, an anti-programmed death-ligand 1 (pd-l1) antibody therapy. Oncoimmunology (2018) 7(7):e1445952. doi: 10.1080/2162402X.2018.1445952
182. Schoop R, Wahl P, Le Hir M, Heemann U, Wang M, Wuthrich RP. Suppressed T-cell activation by IFN-gamma-induced expression of PD-L1 on renal tubular epithelial cells. Nephrol Dial Transplant (2004) 19(11):2713–20. doi: 10.1093/ndt/gfh423
183. Hagerty DT, Allen PM. Processing and presentation of self and foreign antigens by the renal proximal tubule. J Immunol (1992) 148(8):2324–30. doi: 10.4049/jimmunol.148.8.2324
184. Starke A, Wuthrich RP, Waeckerle-Men Y. TGF-beta treatment modulates PD-L1 and CD40 expression in proximal renal tubular epithelial cells and enhances CD8 cytotoxic T-cell responses. Nephron Exp Nephrol (2007) 107(1):e22–29. doi: 10.1159/000106506
185. Fleury M, Belkina AC, Proctor EA, Zammitti C, Simms RW, Lauffenburger DA, et al. Increased expression and modulated regulatory activity of coinhibitory receptors PD-1, TIGIT, and TIM-3 in lymphocytes from patients with systemic Sclerosis. Arthritis Rheumatol (2018) 70(4):566–77. doi: 10.1002/art.40399
186. Davis RJ, Lina I, Ding D, Engle EL, Taube J, Gelbard A, et al. Increased expression of PD-1 and PD-L1 in patients with laryngotracheal Stenosis. Laryngoscope (2021) 131(5):967–74. doi: 10.1002/lary.28790
187. Quan H, Liu S, Shan Z, Liu Z, Chen T, Hu Y, et al. Differential expression of programmed death-1 and its ligand, programmed death ligand-1 in oral squamous cell carcinoma with and without oral submucous fibrosis. Arch Oral Biol (2020) 119:104916. doi: 10.1016/j.archoralbio.2020.104916
Keywords: PD-1, PD-L1, immune checkpoint, fibrosis, immunomodulatory
Citation: Zhao Y, Qu Y, Hao C and Yao W (2023) PD-1/PD-L1 axis in organ fibrosis. Front. Immunol. 14:1145682. doi: 10.3389/fimmu.2023.1145682
Received: 16 January 2023; Accepted: 09 May 2023;
Published: 19 May 2023.
Edited by:
Laura Patrussi, University of Siena, ItalyReviewed by:
Aimin Jiang, The First Affiliated Hospital of Xi’an Jiaotong University, ChinaBinnaz Handan Özdemir, Başkent University, Türkiye
Copyright © 2023 Zhao, Qu, Hao and Yao. This is an open-access article distributed under the terms of the Creative Commons Attribution License (CC BY). The use, distribution or reproduction in other forums is permitted, provided the original author(s) and the copyright owner(s) are credited and that the original publication in this journal is cited, in accordance with accepted academic practice. No use, distribution or reproduction is permitted which does not comply with these terms.
*Correspondence: Wu Yao, eWFvd3VAenp1LmVkdS5jbg==; Changfu Hao, aGFvY2hhbmdmdUAxMjYuY29t