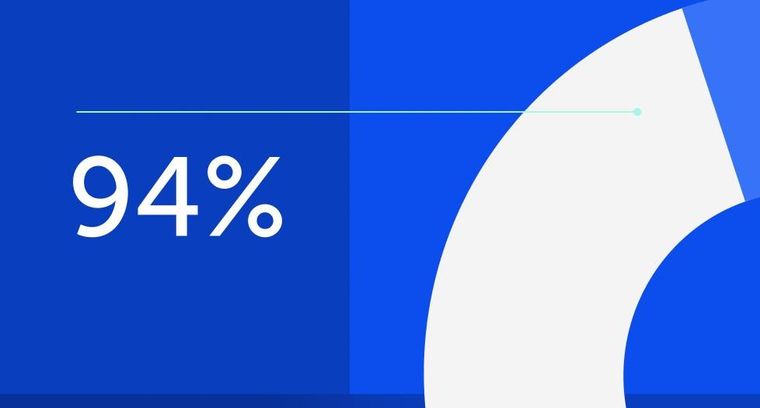
94% of researchers rate our articles as excellent or good
Learn more about the work of our research integrity team to safeguard the quality of each article we publish.
Find out more
ORIGINAL RESEARCH article
Front. Immunol., 08 March 2023
Sec. Mucosal Immunity
Volume 14 - 2023 | https://doi.org/10.3389/fimmu.2023.1140714
This article is part of the Research TopicIgA and Mucosal Immunity in Vaccinology and in Protection from InfectionView all 9 articles
Introduction: Current approved COVID-19 vaccines, notably mRNA and adenoviral vectored technologies, still fail to fully protect against infection and transmission of various SARS-CoV-2 variants. The mucosal immunity at the upper respiratory tract represents the first line of defense against respiratory viruses such as SARS-CoV-2 and is thus critical to develop vaccine blocking human-to-human transmission.
Methods: We measured systemic and mucosal Immunoglobulin A (IgA) response in serum and saliva from 133 healthcare workers from Percy teaching military hospital following a mild infection (SARS-CoV-2 Wuhan strain, n=58) or not infected (n=75), and after SARS-CoV-2 vaccination (Vaxzevria®/Astrazeneca and/or Comirnaty®/Pfizer).
Results: While serum anti-SARS-CoV-2 Spike IgA response lasted up to 16 months post-infection, IgA response in saliva had mostly fallen to baseline level at 6 months post-infection. Vaccination could reactivate the mucosal response generated by prior infection, but failed to induce a significant mucosal IgA response by itself. Early post-COVID-19 serum anti-Spike-NTD IgA titer correlated with seroneutralization titers. Interestingly, its saliva counterpart positively correlated with persistent smell and taste disorders more than one year after mild COVID-19.
Discussion: As breakthrough infections have been correlated with IgA levels, other vaccine platforms inducing a better mucosal immunity are needed to control COVID-19 infection in the future. Our results encourage further studies to explore the prognosis potential of anti-Spike-NTD IgA in saliva at predicting persistent smell and taste disorders.
Protection against infection and mitigation of human-to-human transmission are key factors to block the spread of respiratory viruses, like influenza virus and SARS-CoV-2 (1, 2). These goals designed by herd immunity have not yet been met by current SARS-CoV-2 approved vaccines (3, 4). Systemic vaccination by mRNA and adenovirus-vectored vaccines has shown a great potential in crisis management but fails to induce a prolonged protection against infection and human-to-human transmission by the subsequent variants (5), even though they could reduce the period of transmission by a faster control of viral replication (6). There is a need for second generation vaccines that may broaden the immune response against multiple variants, induce a long-lasting memory response and protect more efficiently against transmission and breakthrough infections, ideally by imprinting a strong mucosal immune response in the upper respiratory tract, as described in recent preclinical studies (7–9).
The protection of the respiratory tract is particularly complex since it is provided by the integrity of the epithelial barriers, a protective mucus layer propelled by cilia, antimicrobial peptides and innate immune cells as well as by an adaptive immune response initiated at the inductive sites of nasopharynx-associated-lymphoid tissue (NALT) (10). The local microbiota has also been described as a modulator of the immune response (11). Polymeric immunoglobulins A (IgA) are specific soluble mediators of the adaptive immune response. While IgA are monomeric in human serum, they are produced locally mostly under dimeric form in mucosal tissues and are released with the secretory component at the mucosal lumen as secretory IgA (SIgA). Monomeric Ig are also present in mucosal secretions following passive transport from blood compartment, with a majority of IgG and fewer IgA (12). Natural polyreactive IgA with cross-reactivity and low affinity have also been described in the lumen of mucosal surfaces (13). In contrast to nasal or bronchoalveolar samples, saliva is an easy-to-access biofluid where specific SARS-CoV-2 IgA have been detected (14–16), providing information about the mucosal response by harboring a significant population of IgA-secreting plasma cells.
Another major health issue of the COVID-19 pandemic is the persistence of long-lasting clinical disorders (so-called long COVID or post-acute sequelae of COVID-19) for a substantial proportion of SARS-CoV-2-infected people, with heterogeneity due to geographic location, SARS-CoV-2 variants and studied population (17, 18). The clinical presentation is highly diverse, involving several organs and systems. Smell and taste disorders were particularly frequent since the initial phase of the COVID-19 pandemic (19, 20), with quantitative (hypo-, hyper-, anosmia or ageusia) or qualitative (dys-, phantosmia or phantageusia) alterations. Persistent olfactory disorders have been notified from 10% to more than 50% according to SARS-CoV-2 variants (18, 19), and can be recovered in a few months. Nevertheless, recent data described less than 40% people with a complete recovery after 2 years, and 7.5% displaying no improvement (21). The pathophysiology of long COVID remains poorly understood and may include viral persistence or delayed clearance, autoimmunity and tissue damages due to inflammation. Recent studies have also highlighted the persistence of the humoral response (22, 23). The contribution of the mucosal humoral compartment has started to be investigated to potentially identify prognosis markers (24).
In this study, we therefore examined the anti-SARS-CoV-2 IgA response over more than one-year in serum and saliva from a cohort of 133 healthcare workers suffering from a mild COVID-19 (SARS-CoV-2 Wuhan strain, n=58, the COVID+ group), and non-infected controls (n=75, the COVID- group). We analyzed the antigen specificity of the response against the spike immune-dominant target and its subdomains (Spike (S), Spike receptor binding domain (RBD), Spike N terminal domain (NTD)) and the nucleoprotein (N). We evaluated the impact of pre-exposure to other human coronaviruses (HCoV-NL63, HCoV-229E, HCoV-OC43, HCoV-HKU1) and the reactivation of mucosal immunity after systemic intramuscular vaccination. Post-infection anti-SARS-CoV-2 Spike IgA response in serum was sustained up to 16 months, whereas IgA response in saliva was back to its baseline level after 6 months. We observed that vaccination reactivated prior mucosal immune response generated by infection, while vaccine alone failed to induce significant IgA titers in saliva. Finally, the initial levels of serum anti-Spike N-terminal domain (NTD) IgA were found to positively correlate with seroneutralization efficacy, whereas their salivary counterpart positively correlated with the persistence of smell disorders or taste disorders more than one year after acute COVID-19.
The main characteristics of the cohort are represented in Table 1. In the total of 400 volunteers achieving the totality of the longitudinal follow-up, we selected 133 individuals (75 naive individuals and 58 previously-infected individuals), based on the certainty of the diagnosis at enrollment, the absence of a new COVID-19 infection during the longitudinal follow-up, and the quality/quantity of saliva samples (Supplementary Method and Supplementary Figure S1).
Table 1 Main characteristics of naive individuals (COVID-) and previously infected individuals (COVID+) at the initial visit (V1).
At enrollment (Visit 1 – V1), the median onset of symptoms was 1.5 months (Table 1) and specific anti-SARS-CoV-2 Spike IgA were present in the serum from almost all previously infected individuals (n=56/58) (Figure 1A). The positive threshold of each test was determined by the Youden index (ROC curves in Supplementary Figure S2). The IgA antibodies targeted preferentially the Spike/RBD (n=54/58) (Figure 1B) than the Spike/NTD (n=48/58) (Figure 1C), and the nucleocapsid-N (n=48/58) (Figure 1D). Six months later (Visit 2 – V2), the IgA signal significantly decreased, regardless of the targeted antigen, as compared to V1 (p<0,001) (Figure 1). Especially, the mean titer of anti-N IgA fell under the positive threshold at 6 months. Hypothesizing a linear evolution of the log(IgA signal) over time between V1 and V2, the modeling using linear mixed models found negative slopes (Supplementary Table S1) that corresponded to a daily decrease of IgA concentration of 2.70 ‰ for S, 2.78 ‰ for Spike/RBD, 3.95 ‰ for Spike/NTD and 6.52 ‰ for N. Between 6 and 12 months following the inclusion (V2 and V3 respectively), anti-N IgA had a tendency to decrease (Figure 1D), with a negative modeling slope corresponding to a daily decrease of 0.21 ‰, whereas we observed a stabilization of IgA titers against all other targets. The general anti-SARS-CoV-2 serological response (anti-N and anti-Spike/RBD total Ig and seroneutralization titers) from previously infected individuals is shown in Figure 2. The Spike/RBD total Ig response (Figure 2A) increased over time (p<0.001), whereas the anti-N total Ig response (Figure 2B) decreased (p<0.001). The seroneutralization capacity of the serum against the BetaCoV/France/IDF0372/2020 strain was maintained up to 16 months post-infection (Figure 2C).
Figure 1 Serum anti-SARS-CoV-2 IgA kinetics and specificity up to 16 months after COVID-19 acute infection and without immunization. Serum anti-SARS-CoV2 IgA (UA/ml) were quantified on a MesoQuickPlex SQ120 using the V-PLEX COVID-19 Coronavirus Panel 2 IgA kit, from naive (COVID-, blue) and previously infected (COVID+, red) individuals at enrollment (V1, boxplots on the left), and its kinetic of decrease (mean slope in red) for symptomatic COVID-19+ individuals up to 16 months after the acute infection (timeline evolution of the signal on the right). (A) Serology IgA titers against the whole Spike. (B) Serology IgA titers against the Spike/RBD. (C) Serology IgA titers against the Spike/NTD. (D) Serology IgA titers against the Nucleocapsid. The red dashed line corresponded to the positivity threshold, determined by the quantitative variable maximizing both sensitivity and specificity (i.e. Youden index). Wilcoxon-rank sum test: *** p<0.001. Linear mixed models were used to calculate the mean slope between each visit (red line).
Figure 2 Serum anti-SARS-CoV-2 total Immunoglobulins (Ig) and seroneutralization titers at each visit and without immunization for previously infected individuals. (A) Titers of total Ig targeting the Spike/RBD and (B) the Nucleocapsid in serum from previously infected individuals are represented at enrollment (V1), 6 months (V2) and 12 months (V3) later. (C) Seroneutralization titers from previously infected individuals at V1, V2 and V3. The positivity thresholds are represented with a red dashed line. Linear regression slopes from (A, B) were significantly different from zero with p < 0.001.
Seroneutralization titers at V1 positively correlated with serum anti-Spike/NTD IgA (Spearman, rho=0.32, p=0.012) (Supplementary Figure S3A). More than 6 months post-infection (V2), seroneutralization titers correlated with serum anti-Spike/NTD IgA (Spearman, rho=0.5, p=0.013) and anti-Spike IgA (Spearman, rho=0.32, p=0.03) (Supplementary Figure S3B). More than one year after COVID-19 infection (V3) and without any vaccination, seroneutralization titers still correlated with serum anti-Spike IgA (Spearman, rho=0.3, p=0.02) (Supplementary Figure S3C).
Overall, we observed in our cohort an induction of high serological titers of anti-SARS-CoV-2 Spike IgA that positively correlated with seroneutralization titers, and maintained for up to 16 months post-infection despite a first decline after 6 months.
At enrollment (V1), specific anti-SARS-CoV-2 IgA were also detected in saliva from infected individuals, as compared to controls individuals (Figure 3). These antibodies targeted the total Spike (p<0.001, Figure 3A), preferentially Spike/RBD (p=0.002, Figure 3B) than Spike/NTD (p=0.001, Figure 3C), but not the nucleocapsid (p=0.43, Figure 3D). The representation of paired serum and saliva anti-SARS-CoV-2 Spike IgA underlined the greater variability of the salivary response, as compared to the signal in serum that is more homogenous (Supplementary Figure S4A). The intensity of anti-Sars-CoV-2 Spike IgA signal in saliva positively correlated with the number of reported symptoms at the initial visit (Pearson, R2 = 0.09, p=0.03) but did not explain by itself the whole clinical variability (Supplementary Figure S4B). Also, it was not predictive of the prevalence of acute smell and taste disorders. During the first six months, the IgA level in saliva significantly decreased against all targets (p<0,04) (Figure 3). The modelling using linear mixed models found negative slopes that corresponded to a daily decrease of IgA concentration of 1.16 ‰ for S, 0.89 ‰ for Spike/RBD and 0.90 ‰ for Spike/NTD. Between 6 and 12 months, a similar decrease was observed against these targets (Supplementary Table S1).
Figure 3 Saliva anti-SARS-CoV-2 IgA kinetics and specificity up to 16 months after COVID-19 acute infection and without immunization. Saliva anti-SARS-CoV2 IgA (UA/ml) were quantified on a MesoQuickPlex SQ120 using the V-PLEX COVID-19 Coronavirus Panel 2 IgA kit, from naive (COVID-, blue) and previously infected (COVID+, red) individuals at enrollment (V1, boxplots on the left), and its kinetic of decrease (mean slope in red) for symptomatic infected individuals up to 16 months after the acute infection (timeline evolution of the signal on the right). (A) Salivary IgA titers against the whole Spike. (B) Salivary IgA titers against the Spike/RBD. (C) Salivary IgA titers against the Spike/NTD. (D) Salivary IgA titers against the Nucleocapsid. The red dashed line corresponded to the positivity threshold, determined by the quantitative variable maximizing both sensitivity and specificity (i.e. Youden index). Wilcoxon-rank sum test: ns, not significant, ** p<0.01, *** p<0.001. Linear mixed models were used to calculate the mean slope between each visit (red line).
So, a specific anti-SARS-CoV-2 Spike salivary IgA response was noted shortly after infection, but weak, variable and with a continuous decrease over the time. This was in contrast with anti-N antibodies for which the detected signal did not differ between naive and previously infected individuals.
In order to evaluate the impact of human coronaviruses pre-exposure on the level of anti-SARS-CoV-2 response and estimate the cross-reactivity in vitro, we quantified IgA in serum and saliva against the Spike protein from two alpha-coronaviruses (hCoV-229E, hCoV-NL63) and three beta-coronaviruses (hCoV-OC43, hCoV-HKU1, SARS-CoV-1) at V1. No previous exposure to SARS-CoV-1 was reported by any participant of the cohort. First, we observed in the COVID-19-negative group a similar range of detected signal against the four hCoV, with slightly higher responses against hCoV-OC43 and hCoV-229E compared to hCoV-NL63 and hCoV-HKU1 in serum and saliva (Figure 4A). IgA directed against SARS-CoV-1 or SARS-CoV-2 were weakly detected, which was obvious in the absence of exposure to the viruses in this group and may reflect non-specific response/background noise, or low level of in vitro cross-reactivity between human coronaviruses.
Figure 4 Serum and saliva anti-Spike IgA titers against human coronaviruses. (A) Normalized log-10 transformed serology titers of anti-Spike IgA (log10 UA/ml) in saliva (left) and serum (right) from naive (COVID-, blue) and previously infected (COVID+, red) individuals measured by MSD technology at enrollment (V1). IgA specificity corresponds to the Spike proteins from human coronaviruses (hCoV) 229E, HKU1, NL63, OC43, SARS-CoV-1 and SARS-CoV-2. Kruskal-Wallis test: ns, not significant; * p<0.05; *** p<0.001. (B) Correlation matrices in saliva (left) and serum (right) at V1 showing significant positive correlations between alpha-coronaviruses (hCoV 229E and NL63), beta-coronaviruses (hCoV OC43, HKU1, SARS-CoV-1, SARS-CoV-2), as well as SARS-CoV-2 antigen specificity (N, Spike, Spike/RBD, Spike/NTD) in previously infected individuals. Only significant correlations (Spearman, p<0.05) are represented on the matrices. The color and size of the dots (scale next to the graph) indicate the degree of correlation (Spearman, rho) between the different parameters (small to large indicating low to high correlation).
At V1, there were significantly higher signals against hCoV-HKU1 in the saliva (p=0.04) and SARS-CoV-1 in the serum (p<0.001) in the COVID-19+ group than in the COVID-19-negative group. The rest of the signals were unchanged by the COVID-19 status. As these viruses are two beta-coronaviruses belonging to a closer specie, it may be explained by some cross-reactivity in vitro. The detected signal against SARS-CoV-2 Spike after infection in this group reached the same level of response observed against the other hCoV in serum and saliva. Then we looked for correlations between individual responses and noticed that in serum (Figure 4B, right panel, Spearman; Supplementary Figure S5, Pearson) beta-coronaviruses responses correlated together, as well as alpha-coronaviruses, independently of SARS-CoV-2 response. In saliva, the pattern showed a larger correlation between coronaviruses genera (Figure 4B, left panel, Spearman; Supplementary Figure S6, Pearson).
Thus, we identified some cross-reactivity in vitro of anti-SARS-CoV-2 Spike IgA against different human coronaviruses. But this phenomenon did not interfere with specific SARS-CoV-2 IgA response that reached the same range of hCoV IgA titers after infection.
At 12 months of the follow-up, the vaccination campaign had already started for healthcare workers, which were a priority population. The vaccine campaign started at the end of December 2020 and a high proportion of the cohort already got vaccinated. At V3, individuals ranged from 0 to 2 doses of vaccine (Table 2) due to limited supplies, updating of vaccine recommendations and vaccine hesitancy. Two vaccination schedules were conducted at this time (Table 3), with 54 individuals having received first an mRNA vaccine (Pfizer-BioNTech, Comirnaty®) and 32 individuals having received first a ChAd-vectored vaccine (Astrazeneca, Vaxzevria®). Few people (n=14) received a heterologous prime-boost with Vaxzevria® followed by Comirnaty® 12 weeks apart (as compared to 4 weeks apart for 2 doses of Comirnaty®). The remaining vaccinated individuals got two doses of Comirnaty® (n=37). At V3 and without vaccination, previously infected individuals did not have any more detectable circulating anti-N IgA whereas anti-Spike (including Spike/RBD and Spike/NTD) IgA were still present (Figure 1). Both vaccination schedule induced significant titers of anti-SARS-CoV-2 Spike IgA in serum (Supplementary Figure S7), in naive and in previously infected individuals. The different group comparisons with the level of significance are represented in Figure 5. Not surprisingly, the specificity of the response was restricted to the Spike, including Spike/RBD and Spike/NTD, and did not extend to the nucleocapsid, an antigen absent from all vaccines. Nevertheless, even after 2 doses, IgA levels in the serum from COVID-19-negative individuals did not reach the level of response observed in previously infected individuals after one or two doses (p<0.001).
Table 2 Vaccination status of naive individuals (COVID-) and previously infected individuals (COVID+) at the last visit (V3).
Table 3 Vaccine schedule of naive (COVID-) and previously infected individuals (COVID+) at the last visit (V3).
Figure 5 Impact of the number of vaccine doses on serum anti-SARS-CoV-2 IgA titers in the presence (red) or absence (blue) of a previous COVID-19 infection. (A) Serology IgA titers against the whole Spike. (B) Serology IgA titers against the Spike/RBD. (C) Serology IgA titers against the Spike/NTD. (D) Serology IgA titers against the Nucleocapsid. Two-Way ANOVA tests with Tukey post’hoc test: ns, not significant; * p<0.05; ** p<0.001; *** p<0.001.
In saliva, we observed significantly higher titers of anti-Spike IgA in individuals previously infected by COVID-19 after one dose (p=0.02) or two doses (p=0.003) of vaccine (Figure 6A). There was no difference between both vaccination schedules (Supplementary Figure S8). Nevertheless, the variability of the delay between the last vaccine dose did not allow a robust comparison and more subjects would be necessary to confirm it. Conversely, the vaccine alone in naive individuals (one or two doses) did not induce specific salivary anti-Spike IgA. Similar results were observed for SARS-CoV-2 Spike/RBD (Figure 6B) and Spike/NTD-specific IgA (Figure 6C). As observed in serum, the specificity of the saliva response was restricted to the Spike and did not extend to the nucleocapsid (Figure 6D). The anti-Spike IgA signals in the COVID-19-positive group after vaccination (one dose, V3) were highly correlated with the V1 post-COVID-19 response (Adj R2 = 0.735, p<0.001) (Figure 7A). Moreover, the slope of the linear regression between serum and saliva after immunization (one or two doses) was highly different in naive (Adj R2 = 0.31, slope = 1, p<0.001) and previously infected individuals (Adj R2 = 0.44, slope = 0.45, p<0.001) (Figure 7B). These analyses were not affected by the removal of two individuals with extremely high IgA levels in saliva and serum (data not shown), and supported the hypothesis that immunization reactivated previously-induced mucosal immunity.
Figure 6 Impact of the number of vaccine doses on saliva anti-SARS-CoV-2 IgA titers in the presence (red) or absence (blue) of a previous COVID-19 infection. (A) Salivary IgA titers against the whole Spike. (B) Salivary IgA titers against the Spike/RBD. (C) Salivary IgA titers against the Spike/NTD. (D) Salivary IgA titers against the Nucleocapsid. Two-Way ANOVA tests with Tukey post’hoc test: ns, not significant; * p<0.05; ** p<0.001; *** p<0.001.
Figure 7 SARS-CoV-2 vaccination reactivated mucosal immunity in previously infected individuals. (A) Salivary anti-Spike IgA post-immunization (Visit 3) positively correlated with salivary anti-Spike IgA post-infection (Visit 1) in previously infected individuals (Pearson correlation, R2 = 0.735, p<0.001). (B) After immunization, the correlation between saliva and serum anti-Spike IgA was well differentiated in naive (COVID-, blue) and previously infected (COVID+, red) individuals, underlining the reactivation of mucosal immunity in previously infected individuals only: COVID+ group (Adj R2 = 0.44, slope = 0.45); COVID- group (Adj R2 = 0.31, slope = 1), p<0.001.
After vaccination, seroneutralization titers (Supplementary Figures S9, S3D) highly correlated with serum anti-Spike/NTD IgA level (Spearman, rho=0.92, p=0.0001), saliva anti-Spike/NTD IgA level (Spearman, rho=0.84, p=0.003), serum anti-Spike IgA (rho=0.86, p=0.0001) and with a lower correlation with serum anti-Spike/RBD IgA (Spearman, rho=0.36, p=0.005). The post-vaccine titers of anti-SARS-CoV-2 Spike IgA in serum and saliva did not modify the initial level of anti-NL63 and 229E hCoV Spike IgA signals (Supplementary Figures S10, S11), but highly increased anti-SARS-CoV-1 Spike signal and slightly anti-OC43 and HKU1 anti-Spike signals (beta-coronaviruses) in serum, confirming that the correlation between human coronaviruses may interfere with the serodiagnosis shortly after vaccination.
Altogether, these results highly suggested that vaccination with mRNA and ChAd-vectored vaccines reactivated mucosal immunity in previously infected COVID-19 individuals but was not sufficient to induce an effective mucosal response in naive individuals.
During the longitudinal follow-up of the cohort, the clinical status was evaluated at each visit. An important proportion of COVID-19 infected individuals had a significant persistence of symptoms after one year (43.5%), especially taste disorders, including dys-, hypo- and ageusia (n=38/102 in the cohort, n=10/58 in our study) and smell disorders, including dys-, hypo- and anosmia (n=42/102 in the cohort, n=13/58 in our study) (Supplementary Figure S12). We compared anti-SARS-CoV-2 IgA response in serum and saliva after infection from individuals with smell (Figure 8A) or taste disorders (Figure 8B) persisting less or more than one year after the acute infection with SARS-CoV2 ancestor Wuhan strain. We found out that patients with smell and taste disorders persisting more than one year had higher titers of anti-SARS-CoV-2 IgA in saliva at enrollment (V1), significantly targeting the Spike/NTD (taste disorders, p=0.02; taste disorders, p=0.04) but not in serum. As Spike-NTD specificity was positively correlated with higher seroneutralization titers, it suggests that it could be an IgA target of clinical relevance
Figure 8 Persistent taste and smell disorders more than one year after infection are associated with higher initial titers of anti-Spike NTD IgA. (A) Normalized log-10 transformed serology titers of anti-SARS-CoV-2 IgA (log10 UA/ml) in saliva (left) and serum (right) measured at visit 1 by MSD technology from individuals suffering from taste disorders persistent for less (brown, n=28) or more (orange, n=10) than one year. (B) Normalized log-10 transformed serology titers of anti-SARS-CoV-2 IgA (log10 UA/ml) in saliva (left) and serum (right) measured at visit 1 by MSD technology from individuals suffering from smell disorders persistent for less (brown, n=27) or more (orange, n=15) than one year. Antigen specificity corresponds to the Nucleocapsid (N), Spike (S), Spike-receptor binding domain (S_RBD) and Spike-N-terminal domain (S_NTD). Kruskal-Wallis test: ns, not significant; * p<0.05.
We followed SARS-CoV-2 IgA response in serum and saliva one year after a COVID-19 outbreak (SARS-CoV-2 Wuhan strain) in healthcare workers. By exploring its antigen specificity and kinetics, we aimed at better understanding the induction and persistence of mucosal immunity after infection and vaccination. We showed different kinetics and level of anti-Spike IgA in serum and saliva after infection, the first being sustainable up to 16 months after a first decline, the second being lost after 6 months. The antigen specificity of anti SARS-CoV-2 IgA unveiled the Spike/NTD as an important target, with positive correlations between serum anti-Spike/NTD IgA and titers of neutralizing antibodies, and saliva initial anti-Spike/NTD IgA levels and long-term persistence of smell and taste disorders. Vaccination with mRNA or Adenovirus-vectored vaccines reactivated a mucosal response in previously infected individuals but failed to induce a significant mucosal response in naive individuals, as already observed on a more limited cohort (25). As breakthrough infections have been correlated with IgA levels (16, 26), IgA production may be a key point to address the issue of controlling the infection locally and preventing the transmission from vaccinated individuals. Most efficient vaccine platforms or route of administration inducing mucosal immunity are needed in the future for COVID-19 control, as already shown in preclinical studies (7, 9).
We used saliva IgA as a surrogate marker of mucosal immunity (10). Indeed, two major antibody classes operate in this fluid: secretory IgA and IgG. Most IgG in saliva are derived from serum whereas IgA are mostly synthesized by plasma cells in salivary glands, reflecting a local immune response that is generated independently from systemic IgA (12, 27). Saliva constitutes an interesting fluid offering an insight into the mucosal immune response (28), and a window into the plasma due to vascular leakage from the gingival crevicular epithelium (29, 30). Saliva is easier to sample and more accepted than nasal swab or nasal epithelial lining fluid. Previous studies on respiratory viruses, like influenza virus have already confirmed that saliva is good proxy at deciphering mucosal immunity (31–33).
The kinetics and antigen specificity of anti-SARS-CoV-2 IgA in our study underlined the sustainability of anti-Spike IgA response in serum over 16 months, after a first decline. This sustainability of the IgA response has already been described over 115 days post-infection with a rapid decline of IgA and IgM responses after a peak at days 16-30 (15), and for up to 8 months (34) and one year (35, 36). We were not able to catch the peak response in our cohort but we confirmed the decline between enrollment (early time post-onset of symptoms) and 6 months later, and the stability between 6 and 12 months. Early SARS-CoV-2 humoral response were shown to be dominated by IgA antibodies that contributed greatly to virus neutralization, in serum and saliva (37). By studying the antigen-specificity of the Spike response, we identified Spike/NTD as a specific IgA target that correlated with seroneutralization titers. A shift of the humoral response toward the Spike/NTD domain had previously been described after the second boost, despite the fact that some Omicron sublineages displayed several NTD mutations (38). Monoclonal antibodies targeting the Spike/NTD have shown in previous studies their ability to inhibit cell-to-cell fusion, to activate effector functions and to protect Syrian hamsters from SARS-CoV-2 challenge (39). Although, serum IgA titers have been correlated with the severity of the disease (40, 41). In our cohort of healthcare workers, the acute symptoms were mainly mild and could not been associated with the anti-Spike/NTD IgA titers. In saliva, IgA mainly targeted the Spike protein (including RBD and NTD specificity), with low titers constantly declining over the year of the follow-up. In previous studies, salivary IgG in mild-COVID-19 were detectable for up to 9 months post-recovery, whereas salivary IgA were short-lived. But salivary dimeric IgA were 15 times more potent than IgA monomers in a pseudoneutralization assay (42).
In our cohort, we observed slightly higher serum IgA titers against hCoV-229E and hCoV-0C43 than hCoV-NL63 and hCoV-HKU1. This description was similarly observed in another cohort using a bead-based multiplexed assay (43) and may reflect previous exposure in the life to these different circulating human coronaviruses (hCoVs). We noticed cross-reactivity inside hCoVs genera in serum, and more broadly between genera in saliva. Cross-reactive antibodies to hCoVs have been already reported in serum, against beta-hCoVs preferentially (44). The differences observed between correlations in serum and saliva were not surprising, as salivary IgA are generated locally and independently of systemic IgA. Mucosal immunity could favor the acquisition of a broader antigenic specificity than systemic immunity. We showed after infection that the level of anti-SARS-CoV-2 IgA response reached the range observed for the other hCoVs, and that SARS-CoV-2 vaccine slightly boosted the beta-coronaviruses response in serum, as already described previously (45). We could not associate the initial signal level of anti-hCoVs IgA with any clinical protection as our cohort included only mild COVID-19 individuals, nor vaccine efficacy as we did not monitor breakthrough infections. Nevertheless, initial anti-hCOV IgA titers did not correlate with seroneutralization titers. It seemed that the observed in vitro cross-reactive activity did not influence the SARS-CoV-2-spike specific IgA response after infection or vaccination. Our results are consistent with previous studies demonstrating the lack of SARS-CoV-2 neutralizing activity in pre-pandemic sera (46) and the absence of correlation with protection and severity of COVID-19 (45). Nevertheless, other studies support the hypothesis that previous hCoV exposure may influence (positively or negatively) the outcome of COVID-19 (47–50).
After vaccination, we confirmed that previously infected individuals had higher titers of IgA in serum than naive individuals and only naive people benefited from a booster effect of the second dose (51–53). Notably, even after two doses, IgA titers from naive individuals did not reach the level of response of previously infected individuals. In our cohort, only anti-Spike/NTD IgA specificity in serum was associated with neutralizing antibodies after infection. After vaccination, Spike, Spike/NTD and Spike/RBD positively correlated with neutralizing antibodies, as observed in other cohorts (52), but we also noticed a positive correlation with Spike/NTD in saliva. In previous studies, a detectable neutralizing activity in saliva observed 2 to 4 weeks post-vaccination was described low and transient with a rapid decline (16, 54). The observed correlations between Spike/NTD IgA specificity and neutralizing antibody titers are not functional proofs of their neutralization capacity as we did not deplete other Ig for the seroneutralization assay, in particular IgG the main neutralizing isotype, but may help to understand the pathophysiology. The ratio of saliva/serum IgA clearly showed two differential profiles according to previous COVID-19 status. Salivary IgA were not induced after vaccination in naive individuals. Conversely, salivary IgA were highly reactivated after vaccination in individuals previously exposed to SARS-CoV-2, and did not mirror serum IgA, suggesting that systemic vaccination could reactivate previously acquired mucosal immunity. This observation emphasized the importance of the first encounter of the antigen that imprints the immune response. Immune imprinting is a phenomenon whereby initial exposure to an antigen effectively primes lymphocyte memory to determine the eventual destination of an activated T cell, and the generation of local tissue-immunity, but limits the development of a different pattern of response that would be necessary to protect against a variant or another strain of the same pathogen after a secondary encounter of the antigen. It is known for viruses like influenza that the mode of initial exposure to a virus or a vaccine affects both the strength of the response and the breadth of the imprint (55, 56).
Lastly, we noticed that individuals suffering from persistent taste or smell disorders more than one year after a mild COVID-19 had higher initial titers of saliva anti-SARS-CoV-2 IgA targeting more specifically the Spike/NTD than individuals who recovered before one year. This initial response correlated also positively with the number of COVID-19 reported symptoms, but was not indicative of the initial prevalence of smell and taste disorders. After vaccination, there was no more difference between the 2 groups. Persisting olfactory and gustatory dysfunctions were commonly reported, and probably underestimated (57). We could not correlate the level of the Spike/NTD-specific antibody response with the initial viral load as the outbreak took place very early, in March 2020, before semi-quantitative molecular diagnosis tools were deployed at our hospital. Studies in animals associated these symptoms with viral persistence and inflammation in the olfactory epithelium (58), but the underlying mechanisms of these persistent dysfunctions are still debated (19). Recent studies have started to explore the involvement of humoral responses in systemic (59, 60) and mucosal compartments (24). In accordance with their results, we did not observe a major difference in serum IgA regarding the duration of olfactory symptoms. Saussez et al. showed that persistent olfactory outcomes at 2 months were associated with lower saliva and nasal IgG1, without any modification of mucosal IgA (24). However, they did not investigate the level of specific anti-Spike/NTD IgA. Our observation was done on few subjects and needs to be confirmed in other cohorts. If confirmed, further studies will be needed to explore the underlying mechanism. It would also help physicians to predict the outcome of smell and taste disorders in order to address rapidly individuals at risk to otorhinolaryngology specialists.
Ethical approval of the cohort study “Immuno-Covid Percy” was given by the Committee for Protection of Persons engaged in clinical research (CPP 20.05.25) and was registered in clinicaltrials.gov (NCT04408001). A written consent was obtained from all participants prior enrollment. The participants did not receive any compensation. Blood and saliva samples were anonymized, handled aliquoted and stored at appropriate temperature (-20°C for the saliva and the serum after blood centrifugation).
The cohort follow-up started after a major outbreak among healthcare workers at the military teaching hospital Percy (HIA Percy) at the end of February 2020. The study population was constituted of hospital workers, including caregivers but also administrative staff. Subjects with a severe form of COVID-19 or immune disorders were excluded. At the initial visit (V1, from day 8 to day 118 after the onset of symptoms), volunteers were included, examined and followed at 6 months (V2, from day 193 to 305 post-onset of symptoms) and 12 months later (V3, from day 375 to day 479 post-onset of symptoms, ie. up to 16 months after infection). At each consultation, blood and saliva were sampled and the volunteers completed a medical form (Supplementary Method). Convalescents (COVID+ group) and uninfected individuals (COVID- group) were defined on their SARS-CoV-2 genome detection (PCR) and/or total immunoglobulin serology (ELISA) against different SARS-CoV-2 Spike and Spike/RBD (Supplementary Method).
In order to confirm the serum status of each participant, total immunoglobulin reactivity of human serum samples was tested against SARS-CoV-2 N and RBD at each sampling timepoint (V1, V2, V3) using the Elecsys® electrochemiluminescence double-antigen sandwich assay performed on Cobas e601. The Elecsys® anti-SARS-CoV-2 Spike RBD is a quantitative immunoassay. According to the manufacturer, a corrective factor (1.029) was applied to convert the results obtained in units per mL to WHO International Standards for anti-SARS-CoV-2 antibodies in BAU/mL (Binding Arbitrary Units per mL). For undiluted serum, SARS-CoV-2 RBD antibodies measuring range covered from 0.4 BAU/mL to 257 BAU/mL and values higher than 0.8 BAU/mL were defined as positive. For sera whose values were below the detection limits, automatized dilutions (1/100e and 1/10 000e) provided by the supplier were used. The Elecsys® anti-SARS-CoV-2 Nucleocapsid assay provided a qualitative detection of antibodies using a recombinant nucleocapsid protein. The result of SARS-CoV-2 N antibodies was automatically computed in a cutoff index, with values ≥1.0 interpreted as reactive corresponding to a positive result. Since these immunoassays were not available at the beginning of the study, retrospective dosing on frozen serum aliquots (maximum thrice thawed) were performed. Independent serum samples were tested to confirm the lack of long-term effect of freezing (more than 6 months) on the measuring results of these immunoassays (data not shown).
A total of 400 volunteers achieved the totality of the longitudinal follow-up, including 249 individuals (62.3%) remaining uninfected and 151 individuals (37.8%) infected by SARS-CoV-2 (Supplementary Figure S1). For IgA detection in serum and saliva, we selected 75 naive individuals and 58 previously-infected individuals, based on the certainty of the diagnosis at enrollment (Table 1), including PCR and ELISA/ECLIA serology, the absence of a new COVID-19 infection during the longitudinal follow-up, and the quality/quantity of saliva samples (compliance to one-hour fasting before sampling, including non-smoking) (Supplementary Figure S1).
Vero cells (ATCC CCL-81) were grown in DMEM medium (Gibco Cat. No. 31966021, ThermoFisher Scientific, Waltham, MA, USA). At confluence, the Vero cells were harvested and subsequently seeded at 1.5 × 105/mL in 96-well plates in order to reach confluence after 72 h at 37°C in 5% CO2. Serial dilutions of stock viruses were made in the infection medium [DMEM supplemented with 50 U/mL penicillin, 50 mg/mL streptomycin (Gibco Cat. No. 15070063, ThermoFisher Scientific) and TPCK-trypsin (Sigma Cat. No. T1426) at a final concentration of 1 µg/mL]. For the neutralization test, SARS-CoV-2 (BetaCoV/France/IDF0372/2020 strain) from clade 19A was isolated by the National Centre (CNR) for Respiratory Viruses (Institut Pasteur), as described previously (61). The BetaCoV/France/IDF0372/2020 strain was supplied by CNR for Respiratory Viruses hosted by Institut Pasteur, headed by Pr. Sylvie van der Werf. The human sample from which the BetaCoV/France/IDF0372/2020 strain was isolated was provided by Dr. X. Lescure and Pr. Y. Yazdanpanah from Bichat Hospital, Paris. Moreover, the BetaCoV/France/IDF0372/2020 strain was supplied through the European Virus Archive goes Global (Evag) platform, a project that has received funding from the European Union’s Horizon 2020 research and innovation program under Grant No. 653316.
Briefly, 50 µL of 10-fold serial dilutions of SARS-CoV-2 were inoculated into eight replicate wells. The 96-well microplates were incubated at 37°C in a 5% CO2 atmosphere, and the cytopathic effect was checked 5 days after inoculation. The virus titer was calculated according to the method of Reed and Muench (62).
At each sampling timepoint (V1, V2, V3), the presence of neutralizing antibodies was sought by a seroneutralization assay performed on Vero cells using the Institut Pasteur SARS-CoV-2 reference BetaCoV/France/IDF0372/2020 strain, in a BSL3 facility. Neutralizing antibodies (Nab) tests were performed in flat-bottomed microtitre plates (96 wells), 3 days after seeding the Vero cells. Two-fold serial dilutions of inactivated sera, starting at a 1/40 dilution, were mixed with an equal volume of SARS-CoV-2 (100 TCID50/50 µL), and incubated for 1 h at 37°C. One hundred microlitres of the serum–virus mixture and 100 µL of infection medium were inoculated in each well (four wells per dilution), and the plates were incubated for 5 days at 37°C in 5% CO2. The cytopathic effect was checked 5 days after inoculation. Neutralization titers were expressed as the inverse of the final dilution of serum that neutralized 50% of the inoculated wells. As no cytopathic effects due to serum cytotoxicity were observed at a dilution of 1/40, a neutralization titer of 40 was considered as positive.
At each sampling timepoint (V1, V2, V3), IgA reactivity of human serum and salivary samples was quantified against SARS-CoV-2 Spike (soluble ectodomain with T4 trimerization domain; C-terminal Strep-Tag and His-Tag), Spike/RBD (R319-F541 of the Wuhan ancestral SARS-CoV-2 Spike sequence; C-terminal His-Tag), Spike/NTD (Q14-L303 of the Wuhan ancestral SARS-CoV-2 Spike sequence; C-terminal Strep-Tag and His-Tag) and Nucleocapsid (Full length Nucleocapsid; C-terminal His-Tag), and the Spike of other human coronaviruses (SARS-CoV-1, alpha HCOV-NL63, alpha HCOV-229E, beta HCOV-OC43 and beta HCOV-HKU1, soluble ectodomain with T4 trimerization domain; C-terminal Strep-Tag and His-Tag) on a MesoQuickPlex SQ120 (Mesoscale discovery – MSD) using the V-PLEX COVID-19 Coronavirus Panel 2 IgA kit (MSD, K15371U) and according to the manufacturer’s protocol. Briefly, after a blocking step, wells from a 96-well plate were washed and incubated during 2 hours at 22˚C with diluted human serum samples (1: 5,000) or diluted human saliva samples (1:25). After three washing steps, bound antibodies were labelled with SULFO-TAG™ anti-human IgA antibody during 1 hour at 22°C. After three washing steps, read buffer was added and plates were read using MSD QuickPlex SQ120 electrochemiluminescent detection system. Samples out of range were up diluted and retested. Data were processed using MSD’s Discovery Workbench version 4.0 and quantification was reported in Arbitrary Units/mL (AU/mL) based on a reference standard curve included in the assay. To assess assay precision and inter-assay variability, three serological controls, containing known concentrations of human IgA against the panel targets’, were run in duplicate on each plate. The technical coefficient of variation (CV) of controls and the individual CV of negatives samples were calculated following the equation (σ = standard deviation of calculated IgA-concentration, m = mean of calculated IgA-concentration). The positive threshold of each test was determined by the quantitative variable maximizing both sensitivity and specificity (i.e. Youden index). The ROC curves are found in Supplementary Figure S2.
Statistical analyses were performed with R (version 4.2.5) using RStudio interface (version 2022.07.2 + 576). Graphical representations were made using ggplot2 package. Tests were performed in a bilateral way and p-values under 0.05 were considered statistically significant. A correction for multiple comparisons was applied using the Benjamini-Hochberg correction or Tukey HSD, resulting in a corrected p-value (false discovery rate) at or under 0.05. Nevertheless, we used a false discovery rate threshold of 0.2 for the association of persistent clinical symptoms with antigen-specific IgA titers in order to avoid missing potential important association due to the weaker number of subjects.
- Discrete independent variables and quantitative dependent:
Testing for group effect on a quantitative variable was performed with the following rank-based tests: Wilcoxon rank-sum test in unpaired two-group settings (comparing IgA concentration between naive and previously infected individuals); Kruskal-Wallis test with Dunn’s correction when comparing multiple unpaired groups (or two-way ANOVA when homoscedasticity and normality of residual was assessed); Friedman test with Nemenyi post Hoc test when comparing multiple paired groups (e.g. comparing concentrations at several timings) using the PMCMR plus package.
- Quantitative variables:
Linear mixed models were used to describe time effect on quantitative variables (lmer function, lme4 package). Following the Box Cox approach (powerTransform function, car package), concentrations were log10 transformed in these linear models to maximize linearity, homoscedasticity and normality of residuals. Simplification of complete models (free intercept and slope per individual for both time and period effects) to allow the model to converge led to models with free intercept per individual only. Slopes and intercepts were compared between conditions and periods using emmeans package. 95 percent profile confidence intervals for model estimates were extracted using the confint function, stats package.
The original contributions presented in the study are included in the article/Supplementary Material. Further inquiries can be directed to the corresponding authors.
Ethical approval of the cohort study “Immuno-Covid Percy” was given by the Comité de Protection des Personnes Ile de France VIII engaged in clinical research (92100 Boulogne-Billancourt, France, CPP 20 05 25) and was registered in clinicaltrials.gov (NCT04408001). The patients/participants provided their written informed consent to participate in this study.
Guillaume Andéol 1, Arnaud Avril 1, Céline Bonnet 2, Olivier Ferraris 1, Vincent Foissaud 2, Olivier Gorgé 1, Fréderic Iseni 1, Francis Klotz 2, Lionel Koch 1, Christine Leclere 2, Kelly Meneyrol 1, Mona Michaud 2,4, Céline Mizoule 2, Albane Moreau 2,4, Bruno Pats 2, Diane Riccobono 1, Clémence Rougeaux 1, Patrick Sandor 1,3, Brian Tervil 4, Emilie Tessier 1, Anthony Vacher 1, Philippe Vest 2, Clarisse Vigne 1, Yordanka Yordanova 2. 1. Institut de Recherche Biomédicale de Armées, 1 place du Général Valérie André, 91223 Brétigny-sur-Orge, France. 2. Hôpital d’Instruction des Armées Percy, 101 avenue Henri Barbusse, 92140 Clamart, France. 3. Direction de la médecine des forces, 60 boulevard du Général Martial Valin, 75509 Paris Cedex 15, France. 4. Centre Borelli UMR 9010/Université Paris-Saclay, ENS Paris-Saclay, CNRS, SSA, Université de Paris Cité, INSERM; 4, avenue des Sciences, 91190 Gif-sur-Yvette, France.
Conceptualization, AT, J-NT, DR; methodology, JD, AG, LC, AF, HT, FJ, CH, EB-D, MM, AT, Percy ImmunoCovid Group; software, JD, AT, MM; statistical analysis, JD, AT, MM; resources, AT, MM; data curation, JD, AT; writing—original draft preparation, JD, AT, MM; writing—review and editing, JD, AT, MM, J-NT, EB-D, AF; visualization, JD, MM, AT; supervision, MM, AT. All authors contributed to the article and approved the submitted version.
This work was supported by the Service de Santé des Armées, and the Agence Innovation Défense (projet ONADAP).
The authors thank the healthcare workers from HIA Percy who massively enrolled in the cohort and gave their time for clinical research during the difficult period of the initial wave of the COVID-19 outbreak. The authors also thank the Percy ImmunoCovid group volunteers that were not directly involved in this work but largely helped to manage the cohort (Nassima Airapetian, Cyril Badaut, Marie Baruteau, Marie De Laage, Amir Dib, Corinne Jamet, Quentin Laborde, Carole Leclercq, Tiphaine Le Lièvre de la Morinière, Pierre-Yves Masse, Karine Michaud, Alice Nicolai, Amandine Steiger), all the team of the department of laboratory medicine who performed serological analysis (particularly Chrystelle Patole, Stéphanie Coppet, Pauline Chauvin) and PCR testing, and all the nurses of HIA Percy (especially from the aeromedical center, the neurophysiology and the anesthesia departments) who realized the blood samples. The authors are grateful to Mesoscale Discovery® for a free of charge loan of a Meso QuickPlex SQ 120 (namely, Fatima Lamraoui for administrative support and Xavier Huet for scientific and technical support) and to Catherine Drogou for her advice on saliva sample management. Finally, the authors address a special acknowledgment to Dr Thépenier for the statistical analyses on R software, its precious help to manage the cohort and for the critical revision of the manuscript.
The authors declare that the research was conducted in the absence of any commercial or financial relationships that could be construed as a potential conflict of interest.
All claims expressed in this article are solely those of the authors and do not necessarily represent those of their affiliated organizations, or those of the publisher, the editors and the reviewers. Any product that may be evaluated in this article, or claim that may be made by its manufacturer, is not guaranteed or endorsed by the publisher.
The Supplementary Material for this article can be found online at: https://www.frontiersin.org/articles/10.3389/fimmu.2023.1140714/full#supplementary-material
1. Kuster SP, Shah PS, Coleman BL, Lam PP, Tong A, Wormsbecker A, et al. Incidence of influenza in healthy adults and healthcare workers: A systematic review and meta-analysis. PLoS One (2011) 6(10):e26239. doi: 10.1371/journal.pone.0026239
2. Vargese SS, Dev SS, Soman AS, Kurian N, Varghese VA, Mathew E. Exposure risk and COVID-19 infection among frontline health-care workers: A single tertiary care centre experience. Clin Epidemiol Glob Health (2022) 13:100933. doi: 10.1016/j.cegh.2021.100933
3. Hsu L, Wisplinghoff H, Kossow A, Hurrass J, Wiesmuller GA, Grune B, et al. Limited protection against SARS-CoV-2 infection and virus transmission after mRNA vaccination. J Infect (2022) 84(1):94–118. doi: 10.1016/j.jinf.2021.06.023
4. Maugey N, Lefebvre T, Tournier JN, Neulat-Ripoll F, Chapus C, Grandperret V, et al. Vaccine efficacy against the SARS-CoV-2 delta variant during a COVID-19 outbreak aboard a military ship. BMJ Mil Health (2022) 29:e002082. doi: 10.1136/bmjmilitary-2022-002082
5. Mura M, Simon F, Pommier de Santi V, Tangy F, Tournier JN. Role and limits of COVID-19 vaccines in the delicate transition from pandemic mitigation to endemic control. Vaccines (Basel) (2022) 10(9):1555. doi: 10.3390/vaccines10091555
6. Puhach O, Meyer B, Eckerle I. SARS-CoV-2 viral load and shedding kinetics. Nat Rev Microbiol (2022) 21(3):147–61. doi: 10.1038/s41579-022-00822-w
7. Langel SN, Johnson S, Martinez CI, Tedjakusuma SN, Peinovich N, Dora EG, et al. Adenovirus type 5 SARS-CoV-2 vaccines delivered orally or intranasally reduced disease severity and transmission in a hamster model. Sci Transl Med (2022) 14(658):eabn6868. doi: 10.1126/scitranslmed.abn6868
8. Lapuente D, Fuchs J, Willar J, Vieira Antao A, Eberlein V, Uhlig N, et al. Protective mucosal immunity against SARS-CoV-2 after heterologous systemic prime-mucosal boost immunization. Nat Commun (2021) 12(1):6871. doi: 10.1038/s41467-021-27063-4
9. Mao T, Israelow B, Pena-Hernandez MA, Suberi A, Zhou L, Luyten S, et al. Unadjuvanted intranasal spike vaccine elicits protective mucosal immunity against sarbecoviruses. Science (2022) 378(6622):eabo2523. doi: 10.1126/science.abo2523
10. Yaugel-Novoa M, Bourlet T, Paul S. Role of the humoral immune response during COVID-19: guilty or not guilty? Mucosal Immunol (2022) 15:1170–80. doi: 10.1038/s41385-022-00569-w
11. Smith N, Goncalves P, Charbit B, Grzelak L, Beretta M, Planchais C, et al. Distinct systemic and mucosal immune responses during acute SARS-CoV-2 infection. Nat Immunol (2021) 22(11):1428–39. doi: 10.1038/s41590-021-01028-7
12. Brandtzaeg P. Secretory immunity with special reference to the oral cavity. J Oral Microbiol (2013) 5. doi: 10.3402/jom.v5i0.20401
13. Bunker JJ, Erickson SA, Flynn TM, Henry C, Koval JC, Meisel M, et al. Natural polyreactive IgA antibodies coat the intestinal microbiota. Science (2017) 358(6361):eaan6619. doi: 10.1126/science.aan6619
14. Huang N, Perez P, Kato T, Mikami Y, Okuda K, Gilmore RC, et al. SARS-CoV-2 infection of the oral cavity and saliva. Nat Med (2021) 27(5):892–903. doi: 10.1038/s41591-021-01296-8
15. Isho B, Abe KT, Zuo M, Jamal AJ, Rathod B, Wang JH, et al. Persistence of serum and saliva antibody responses to SARS-CoV-2 spike antigens in COVID-19 patients. Sci Immunol (2020) 5(52):eabe5511. doi: 10.1126/sciimmunol.abe5511
16. Sheikh-Mohamed S, Isho B, Chao GYC, Zuo M, Cohen C, Lustig Y, et al. Systemic and mucosal IgA responses are variably induced in response to SARS-CoV-2 mRNA vaccination and are associated with protection against subsequent infection. Mucosal Immunol (2022) 15(5):799–808. doi: 10.1038/s41385-022-00511-0
17. Perlis RH, Santillana M, Ognyanova K, Safarpour A, Lunz Trujillo K, Simonson MD, et al. Prevalence and correlates of long COVID symptoms among US adults. JAMA Netw Open (2022) 5(10):e2238804. doi: 10.1001/jamanetworkopen.2022.38804
18. Spinicci M, Graziani L, Tilli M, Nkurunziza J, Vellere I, Borchi B, et al. Infection with SARS-CoV-2 variants is associated with different long COVID phenotypes. Viruses (2022) 14(11):2367. doi: 10.3390/v14112367
19. Butowt R, Bilinska K, von Bartheld CS. Olfactory dysfunction in COVID-19: new insights into the underlying mechanisms. Trends Neurosci (2022) 46(1):75–90. doi: 10.1016/j.tins.2022.11.003
20. Mendes Paranhos AC, Nazareth Dias AR, Machado da Silva LC, Vieira Hennemann Koury G, de Jesus Sousa E, Cerasi AJ Jr, et al. Sociodemographic characteristics and comorbidities of patients with long COVID and persistent olfactory dysfunction. JAMA Netw Open (2022) 5(9):e2230637. doi: 10.1001/jamanetworkopen.2022.30637
21. McWilliams MP, Coelho DH, Reiter ER, Costanzo RM. Recovery from covid-19 smell loss: Two-years of follow up. Am J Otolaryngol (2022) 43(5):103607. doi: 10.1016/j.amjoto.2022.103607
22. Klein J, Wood J, Jaycox J, Lu P, Dhodapkar RM, Gehlhausen JR, et al. Distinguishing features of long COVID identified through immune profiling. medRxiv (2022). doi: 10.1101/2022.08.09.22278592
23. Peghin M, De Martino M, Palese A, Gerussi V, Bontempo G, Graziano E, et al. Post-COVID-19 syndrome and humoral response association after 1 year in vaccinated and unvaccinated patients. Clin Microbiol Infect (2022) 28(8):1140–8. doi: 10.1016/j.cmi.2022.03.016
24. Saussez S, Sharma S, Thiriard A, Olislagers V, Vu Duc I, Le Bon SD, et al. Predictive factors of smell recovery in a clinical series of 288 coronavirus disease 2019 patients with olfactory dysfunction. Eur J Neurol (2021) 28(11):3702–11. doi: 10.1111/ene.14994
25. Sano K, Bhavsar D, Singh G, Floda D, Srivastava K, Gleason C, et al. SARS-CoV-2 vaccination induces mucosal antibody responses in previously infected individuals. Nat Commun (2022) 13(1):5135. doi: 10.1038/s41467-022-32389-8
26. Havervall S, Marking U, Svensson J, Greilert-Norin N, Bacchus P, Nilsson P, et al. Anti-spike mucosal IgA protection against SARS-CoV-2 omicron infection. N Engl J Med (2022) 387(14):1333–6. doi: 10.1056/NEJMc2209651
27. Russell MW, Moldoveanu Z, Ogra PL, Mestecky J. Mucosal immunity in COVID-19: A neglected but critical aspect of SARS-CoV-2 infection. Front Immunol (2020) 11:611337. doi: 10.3389/fimmu.2020.611337
28. Guerra ENS, de Castro VT, Amorim Dos Santos J, Acevedo AC, Chardin H. Saliva is suitable for SARS-CoV-2 antibodies detection after vaccination: A rapid systematic review. Front Immunol (2022) 13:1006040. doi: 10.3389/fimmu.2022.1006040
29. Hettegger P, Huber J, Passecker K, Soldo R, Kegler U, Nohammer C, et al. High similarity of IgG antibody profiles in blood and saliva opens opportunities for saliva based serology. PLoS One (2019) 14(6):e0218456. doi: 10.1371/journal.pone.0218456
30. Seneviratne CJ, Balan P, de Alwis R, Udawatte NS, Herath T, Toh JZN, et al. BNT162b2 mRNA vaccine-induced immune response in oral fluids and serum. Int Dent J (2022) S0020-6539(22)00225-8. doi: 10.1016/j.identj.2022.09.005
31. Tada A, Senpuku H. The impact of oral health on respiratory viral infection. Dent J (Basel) (2021) 9(4):43. doi: 10.3390/dj9040043
32. Tsunetsugu-Yokota Y, Ito S, Adachi Y, Onodera T, Kageyama T, Takahashi Y. Saliva as a useful tool for evaluating upper mucosal antibody response to influenza. PLoS One (2022) 17(2):e0263419. doi: 10.1371/journal.pone.0263419
33. Moldoveanu Z, Clements ML, Prince SJ, Murphy BR, Mestecky J. Human immune responses to influenza virus vaccines administered by systemic or mucosal routes. Vaccine. (1995) 13(11):1006–12. doi: 10.1016/0264-410X(95)00016-T
34. Dan JM, Mateus J, Kato Y, Hastie KM, Yu ED, Faliti CE, et al. Immunological memory to SARS-CoV-2 assessed for up to 8 months after infection. Science (2021) 371(6529):eabf4063. doi: 10.1126/science.abf4063
35. Chansaenroj J, Yorsaeng R, Puenpa J, Wanlapakorn N, Chirathaworn C, Sudhinaraset N, et al. Long-term persistence of severe acute respiratory syndrome coronavirus 2 (SARS-CoV-2) spike protein-specific and neutralizing antibodies in recovered COVID-19 patients. PLoS One (2022) 17(4):e0267102. doi: 10.1371/journal.pone.0267102
36. Shi D, Weng T, Wu J, Dai C, Luo R, Chen K, et al. Dynamic characteristic analysis of antibodies in patients with COVID-19: A 13-month study. Front Immunol (2021) 12:708184. doi: 10.3389/fimmu.2021.708184
37. Sterlin D, Mathian A, Miyara M, Mohr A, Anna F, Claer L, et al. IgA dominates the early neutralizing antibody response to SARS-CoV-2. Sci Transl Med (2021) 13(577):eabd2223. doi: 10.1126/scitranslmed.abd2223
38. Wang J, Deng C, Liu M, Liu Y, Li L, Huang Z, et al. A fourth dose of the inactivated SARS-CoV-2 vaccine redistributes humoral immunity to the n-terminal domain. Nat Commun (2022) 13(1):6866. doi: 10.1038/s41467-022-34633-7
39. McCallum M, De Marco A, Lempp FA, Tortorici MA, Pinto D, Walls AC, et al. N-terminal domain antigenic mapping reveals a site of vulnerability for SARS-CoV-2. Cell. (2021) 184(9):2332–47.e16. doi: 10.1016/j.cell.2021.03.028
40. Carsetti R, Zaffina S, Piano Mortari E, Terreri S, Corrente F, Capponi C, et al. Different innate and adaptive immune responses to SARS-CoV-2 infection of asymptomatic, mild, and severe cases. Front Immunol (2020) 11:610300. doi: 10.3389/fimmu.2020.610300
41. Cervia C, Nilsson J, Zurbuchen Y, Valaperti A, Schreiner J, Wolfensberger A, et al. Systemic and mucosal antibody responses specific to SARS-CoV-2 during mild versus severe COVID-19. J Allergy Clin Immunol (2021) 147(2):545–57.e9. doi: 10.1016/j.jaci.2020.10.040
42. Wang Z, Lorenzi JCC, Muecksch F, Finkin S, Viant C, Gaebler C, et al. Enhanced SARS-CoV-2 neutralization by dimeric IgA. Sci Transl Med (2021) 13(577):eabf1555. doi: 10.1126/scitranslmed.abf1555
43. Becker M, Strengert M, Junker D, Kaiser PD, Kerrinnes T, Traenkle B, et al. Exploring beyond clinical routine SARS-CoV-2 serology using MultiCoV-ab to evaluate endemic coronavirus cross-reactivity. Nat Commun (2021) 12(1):1152. doi: 10.1038/s41467-021-20973-3
44. Narowski TM, Raphel K, Adams LE, Huang J, Vielot NA, Jadi R, et al. SARS-CoV-2 mRNA vaccine induces robust specific and cross-reactive IgG and unequal neutralizing antibodies in naive and previously infected people. Cell Rep (2022) 38(5):110336. doi: 10.1016/j.celrep.2022.110336
45. Anderson EM, Goodwin EC, Verma A, Arevalo CP, Bolton MJ, Weirick ME, et al. Seasonal human coronavirus antibodies are boosted upon SARS-CoV-2 infection but not associated with protection. Cell. (2021) 184(7):1858–64.e10. doi: 10.1016/j.cell.2021.02.010
46. Poston D, Weisblum Y, Wise H, Templeton K, Jenks S, Hatziioannou T, et al. Absence of severe acute respiratory syndrome coronavirus 2 neutralizing activity in prepandemic sera from individuals with recent seasonal coronavirus infection. Clin Infect Dis (2021) 73(5):e1208–e11. doi: 10.1093/cid/ciaa1803
47. Galipeau Y, Siragam V, Laroche G, Marion E, Greig M, McGuinty M, et al. Relative ratios of human seasonal coronavirus antibodies predict the efficiency of cross-neutralization of SARS-CoV-2 spike binding to ACE2. EBioMedicine (2021) 74:103700. doi: 10.1016/j.ebiom.2021.103700
48. Lin CY, Wolf J, Brice DC, Sun Y, Locke M, Cherry S, et al. Pre-existing humoral immunity to human common cold coronaviruses negatively impacts the protective SARS-CoV-2 antibody response. Cell Host Microbe (2022) 30(1):83–96.e4. doi: 10.1016/j.chom.2021.12.005
49. Wratil PR, Schmacke NA, Karakoc B, Dulovic A, Junker D, Becker M, et al. Evidence for increased SARS-CoV-2 susceptibility and COVID-19 severity related to pre-existing immunity to seasonal coronaviruses. Cell Rep (2021) 37(13):110169. doi: 10.1016/j.celrep.2021.110169
50. Yu ED, Narowski TM, Wang E, Garrigan E, Mateus J, Frazier A, et al. Immunological memory to common cold coronaviruses assessed longitudinally over a three-year period pre-COVID19 pandemic. Cell Host Microbe (2022) 30(9):1269–78.e4. doi: 10.1016/j.chom.2022.07.012
51. Azzi L, Dalla Gasperina D, Veronesi G, Shallak M, Ietto G, Iovino D, et al. Mucosal immune response in BNT162b2 COVID-19 vaccine recipients. EBioMedicine. (2022) 75:103788. doi: 10.1016/j.ebiom.2021.103788
52. Chivu-Economescu M, Bleotu C, Grancea C, Chiriac D, Botezatu A, Iancu IV, et al. Kinetics and persistence of cellular and humoral immune responses to SARS-CoV-2 vaccine in healthcare workers with or without prior COVID-19. J Cell Mol Med (2022) 26(4):1293–305. doi: 10.1111/jcmm.17186
53. Zurac S, Nichita L, Mateescu B, Mogodici C, Bastian A, Popp C, et al. COVID19 vaccination and IgG and IgA antibody dynamics in healthcare workers. Mol Med Rep (2021) 24(2):578. doi: 10.3892/mmr.2021.12217
54. Garziano M, Utyro O, Poliseno M, Santantonio TA, Saulle I, Strizzi S, et al. Natural SARS-CoV-2 infection affects neutralizing activity in saliva of vaccinees. Front Immunol (2022) 13:820250. doi: 10.3389/fimmu.2022.820250
55. Knight M, Changrob S, Li L, Wilson PC. Imprinting, immunodominance, and other impediments to generating broad influenza immunity. Immunol Rev (2020) 296(1):191–204. doi: 10.1111/imr.12900
56. McCarthy KR, Von Holle TA, Sutherland LL, Oguin TH, Sempowski GD, Harrison SC, et al. Differential immune imprinting by influenza virus vaccination and infection in nonhuman primates. Proc Natl Acad Sci USA (2021) 118(23):e2026752118. doi: 10.1073/pnas.2026752118
57. Otte MS, Klussmann JP, Luers JC. Persisting olfactory dysfunction in patients after recovering from COVID-19. J Infect (2020) 81(3):e58. doi: 10.1016/j.jinf.2020.06.054
58. de Melo GD, Lazarini F, Levallois S, Hautefort C, Michel V, Larrous F, et al. COVID-19-related anosmia is associated with viral persistence and inflammation in human olfactory epithelium and brain infection in hamsters. Sci Transl Med (2021) 13(596):eabf8396. doi: 10.1126/scitranslmed.abf8396
59. Rank A, Tzortzini A, Kling E, Schmid C, Claus R, Loll E, et al. One year after mild COVID-19: The majority of patients maintain specific immunity, but one in four still suffer from long-term symptoms. J Clin Med (2021) 10(15):3305. doi: 10.3390/jcm10153305
60. Schambeck SE, Mateyka LM, Burrell T, Graf N, Brill I, Stark T, et al. Two-year follow-up on chemosensory dysfunction and adaptive immune response after infection with SARS-CoV-2 in a cohort of 44 healthcare workers. Life (Basel) (2022) 12(10):1556. doi: 10.3390/life12101556
61. Lescure FX, Bouadma L, Nguyen D, Parisey M, Wicky PH, Behillil S, et al. Clinical and virological data of the first cases of COVID-19 in Europe: a case series. Lancet Infect Dis (2020) 20(6):697–706. doi: 10.1016/S1473-3099(20)30200-0
Keywords: mucosal immunity, SARS-CoV2 vaccine, dysosmia, dysgeusia, IgA, Spike N-terminal domain, smell, taste
Citation: Denis J, Garnier A, Cheutin L, Ferrier A, Timera H, Jarjaval F, Hejl C, Billon-Denis E, Percy ImmunoCovid group, Ricard D, Tournier J-N, Trignol A and Mura M (2023) Long-term systemic and mucosal SARS-CoV-2 IgA response and its association with persistent smell and taste disorders. Front. Immunol. 14:1140714. doi: 10.3389/fimmu.2023.1140714
Received: 09 January 2023; Accepted: 21 February 2023;
Published: 08 March 2023.
Edited by:
Cinzia Milito, Sapienza University of Rome, ItalyReviewed by:
Zina Moldoveanu, University of Alabama at Birmingham, United StatesCopyright © 2023 Denis, Garnier, Cheutin, Ferrier, Timera, Jarjaval, Hejl, Billon-Denis, Percy ImmunoCovid group, Ricard, Tournier, Trignol and Mura. This is an open-access article distributed under the terms of the Creative Commons Attribution License (CC BY). The use, distribution or reproduction in other forums is permitted, provided the original author(s) and the copyright owner(s) are credited and that the original publication in this journal is cited, in accordance with accepted academic practice. No use, distribution or reproduction is permitted which does not comply with these terms.
*Correspondence: Marie Mura, bWFyaWUubXVyYUBkZWYuZ291di5mcg==; Aurélie Trignol, YXVyZWxpZS50cmlnbm9sQGRlZi5nb3V2LmZy
Disclaimer: All claims expressed in this article are solely those of the authors and do not necessarily represent those of their affiliated organizations, or those of the publisher, the editors and the reviewers. Any product that may be evaluated in this article or claim that may be made by its manufacturer is not guaranteed or endorsed by the publisher.
Research integrity at Frontiers
Learn more about the work of our research integrity team to safeguard the quality of each article we publish.