- Department of Emergency Medicine, University of Nebraska Medical Center, Omaha, NE, United States
Responding to tissue injury, skeletal muscles undergo the tissue destruction and reconstruction accompanied with inflammation. The immune system recognizes the molecules released from or exposed on the damaged tissue. In the local minor tissue damage, tissue-resident macrophages sequester pro-inflammatory debris to prevent initiation of inflammation. In most cases of the skeletal muscle injury, however, a cascade of inflammation will be initiated through activation of local macrophages and mast cells and recruitment of immune cells from blood circulation to the injured site by recongnization of damage-associated molecular patterns (DAMPs) and activated complement system. During the inflammation, macrophages and neutrophils scavenge the tissue debris to release inflammatory cytokines and the latter stimulates myoblast fusion and vascularization to promote injured muscle repair. On the other hand, an abundance of released inflammatory cytokines and chemokines causes the profound hyper-inflammation and mobilization of immune cells to trigger a vicious cycle and lead to the cytokine storm. The cytokine storm results in the elevation of cytolytic and cytotoxic molecules and reactive oxygen species (ROS) in the damaged muscle to aggravates the tissue injury, including the healthy bystander tissue. Severe inflammation in the skeletal muscle can lead to rhabdomyolysis and cause sepsis-like systemic inflammation response syndrome (SIRS) and remote organ damage. Therefore, understanding more details on the involvement of inflammatory factors and immune cells in the skeletal muscle damage and repair can provide the new precise therapeutic strategies, including attenuation of the muscle damage and promotion of the muscle repair.
1 Introduction
Infection or tissue injury elicits a series of rapid innate immune responses required to eliminate infectious agents or damaged tissues, named as septic or sterile inflammation respectively. It is host defensive reaction to remove the invaders or clean damaged tissues for wound healing. However, uncontrolled inflammation possibly results in the tissue damage, even to a danger situation.
Responding to tissue injury, skeletal muscles undergo tissue destruction and reconstruction. According to the cellular and molecular events, there are five interrelated and time-dependent phases, including degeneration-necrosis, inflammation, regeneration, maturation/remodeling, and functional recovery (1). The sterile inflammation, as a result of trauma, typically occurs in the absence of any microorganism (2, 3). Similar to microbially induced inflammation, the sterile inflammation is marked by the recruitment of neutrophils and macrophages and the production of pro-inflammatory cytokines and chemokines, notably tumor necrosis factor (TNF) and interleukin-1 (IL-1). The inflammatory response could play central roles in bridging initial responses to muscle injury and timely muscle injury reparation (4) or triggering a vicious cycle to exaggerate the tissue damage (5, 6). Therefore, understanding the pathophysiological process of the sterile inflammation and controlling the sterile inflammation attack are very important for local tissue repair when the tissue is less regenerative capacity and prevention of remote organ damage.
2 Triggers of inflammation in the damaged skeletal muscle
2.1 Damage-associated molecular patterns promote inflammation
In addition to the exogenous signal that can be introduced into the body, the immune system can also sense danger molecules released from damaged or stressed tissues. Thus, the immune system can discriminate not only ‘self from non-self’ but also ‘healthy from damaged self’ (7). These danger molecules are intracellularly sequestered and are therefore hidden from recognition by the immune system under normal physiological conditions. They can be released in response to a variety of tissue trauma resulted from burns, cold, chemical insults, radiation, oxygen deprivation, nutrient depletion, auto-immune tissue destruction, tumors, and xenobiotics (7). An initial traumatic insult disrupts macrobarries such as the skin, and microbarriers such as cell membranes, which causes the release of multiple danger molecules. These endogenous danger molecules released from damaged or dying cells are termed as damage-associated molecular patterns (DAMPs), including high-mobility group box 1 (HMGB1), S100 proteins, heat shock proteins (HSPs), histones, mitochondrial DNA (mtDNA), and ATP (Figure 1) (6). They can be recognized by the innate immune system and are considered as key inducers of sterile inflammation following the tissue damage (8, 9).
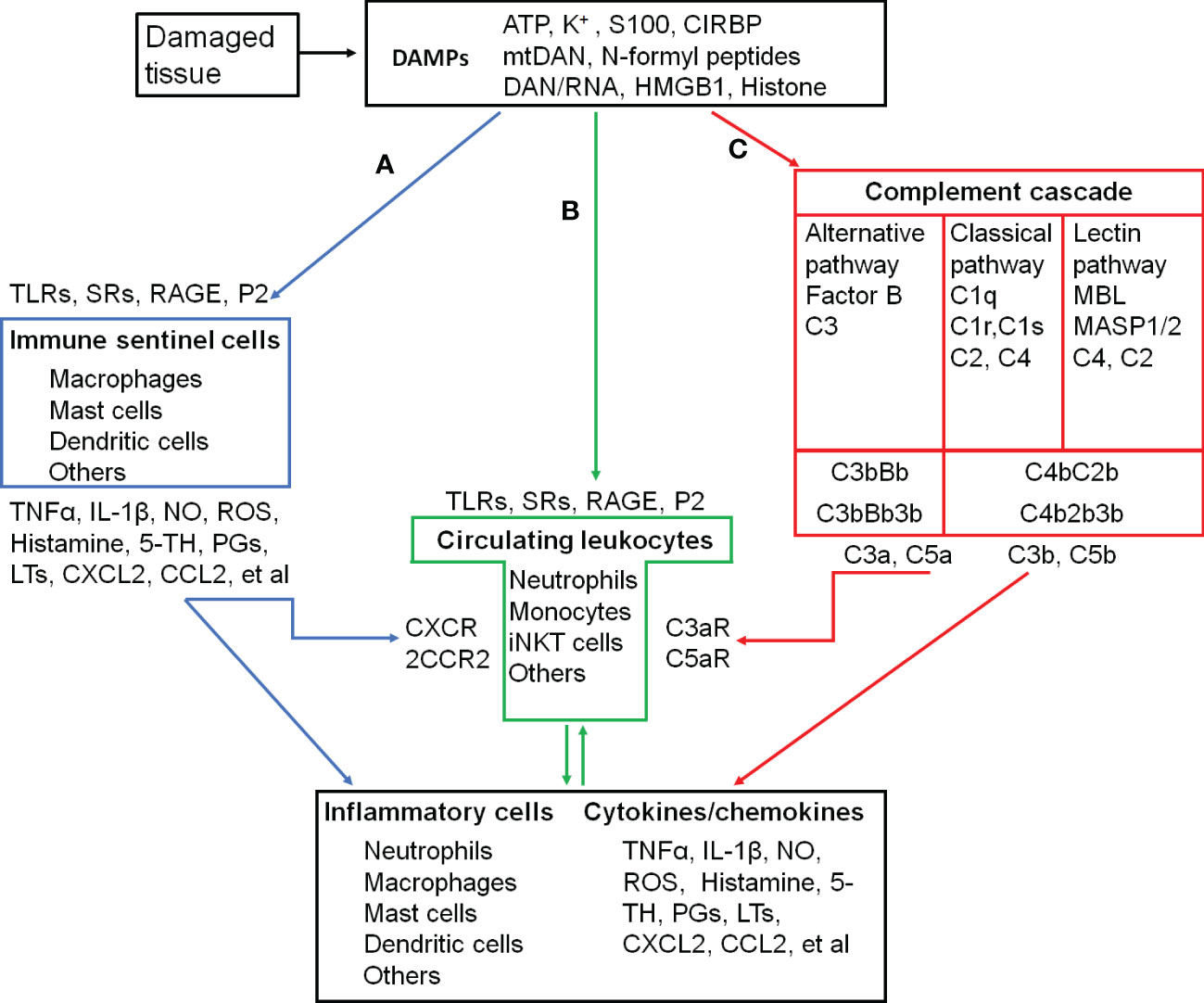
Figure 1 Inflammatory activation and its triggers in the skeletal muscle. (A-C), three pathways that activate inflammatory cells. (A), proteolytic cascades of complements are triggered by the damaged tissue and released C3a and C5a recruit and activate circulating leukocytes. (B), DAMPs released into circulation are recongnized by circulating leukocytes, which are recruted to and activated in the injured site. (C), damaged tissue-activated local immune sentinel cells release cytokines and chemokines to recruit and activate circulating leukocytes. ATP, Adenosine triphospate; CCL2, C-C motif chemokine ligand 2/Monocyte chemoattractant protein-1 (MCP-1); CCR2, C-C motif chemokine receptor; CIRBP, Cold-inducible RNA-binding protein; CXCL2, C-X-C motif chemokine ligand 2/macrophage inflammatory protein 2-alpha (MIP2-α); CXCR2, C-X-C motif chemokine receptor 2; DAMPs, Damage-associated molecular patterns; HMGB1, High mobility group box-1; IL-1β, interleukin-1β; iNKT cells, Invariant natural killer T cells. LTs, Leukotrienes; MBL, Mannose-binding lectin; MASP1/2, MBL-associated serine protease-1/2; mtDNA, mitochodrial DNA; NO, Nitric oxide; PGs, Prostaglandins; P2, Purinergic preceptors (P2Rs); S100, S100 protein; RAGE, Receptor for advanced glycation endproducts; ROS, Reactive oxygen species; SRs, Scavenger receptors; 5-TH, Serotonin; TLRs, Toll-like receptors; TNFα, Tumor necrosis factor α; C1 (2, 3, 4, 5), complement component 1 (2, 3, 4, 5); C1q, C1 complex componet– recognicition molecular C1q; C1r and C1s, C1 complex componet—tetrameric protease complex C1R2S2; C3bBb and C4b2b, C3 convertase; C2b, smaller fragment of C2 cleaved by C1s; C3a and C3b, two fragments of cleaved C3; C4b, Complement component C4b; C4bC2b, C3 convertase; C3bBb3b and C4b2b3b, C5 convertase; C5a and C5b, two fragments of cleaved C5; C3aR, C3a receptor; C5aR, C5a receptor; Factor B, Complement factor B; Bb, Fragment of complement factor B.
During the cellular stress or injury, DAMPs can be released into the extracellular environment and blood circulation from damaged cells and are recognized by pattern recognition receptors (PRRs), such as Toll-like receptors (TLRs) and scavenger receptors (SRs), or non-PRRs, such as the receptor for advanced glycation end-products (RAGE) and purinergic receptors expressed on immune cells (8, 9) (Figure 1). After migrating through the vessel wall from the blood stream mediated by endothelial selectins and leukocyte integrins, leukocytes exit to interstitial space following a chemokine gradient where these chemokines are main ligands for C-X-C motif chemokine receptor 2 (CXCR2, a major chemokine receptor expressed in neutrophils and other immune cells) (8, 9). As the first recruited leukocytes, neutrophils are activated after their migration to the injury site along a gradient of DAMPs (8, 9). The proinflammatory monocytes, including monocytes with high expression of C-C chemokine receptor type 2 (CCR2high) and with low levels of C-X3-C motif chemokine receptor (CX3CR1low), successively transmigrate from blood stream in a CCR2-dependent manner, undergo in situ reprogramming into CCR2low and CX3CR1high alternative monocytes, and enter the injury site following the DAMP gradient. The in-situ reprogramming of monocytes depends on interleukin-4 (IL-4) and interleukin-10 (IL-10) produced by invariant natural killer T (iNKT) cells (9). The leukocyte recognition of DAMPs through PPRs or non-PPRs activates the downstream signaling through the adaptor proteins. For example, TLR2 and TLR4 on the leukocytes can be recognized by intracellular proteins HMGB1, HSPs, and histone released from the damaged tissue. They activate mitogen-activated protein kinases (MAPKs) and inhibitor of nuclear factor kappa B (IκB) kinase (IKK) to increase the production of the inflammatory cytokines from subsequently activated leukocytes through the activation of the transcription factors activator protein 1 (AP-1) and nuclear factor κB (NFκB), respectively (6, 8–11).
When the skeletal muscle damage occurs, the integrity of myofibers and other cells is severely compromised, and the plasmalemma permeability is alternated with uncontrolled ionic flux and the loss of a proper architecture (1). DAMPs released into the interstitial space and systemic circulation (12, 13) interact with PRRs or no-PRRs to promote inflammation (14). As pro-inflammatory mediators, specific DAMPs released from the skeletal muscle, including HMGB1 (15–17), ATP (18, 19), and mitochondrial DNA (20, 21), induce the secretion of pro-inflammatory cytokines and chemokines to trigger inflammation through TLR4/RAGE, P2X7R, and TLR9 on infiltrating/tissue-resident macrophages and neutrophils.
2.2 Complements promote inflammation
The complement is a system of more than 40 proteins in the plasma (soluble) and on cell surfaces (membrane-bound proteins). A number of complement proteins are proteases and widely distributed throughout body fluids and tissues without adverse effects. Activation of complements produces proinflammatory molecules, such as C3a and C5a to stimulate the inflammatory response (Figure 1) (22).
Complements are activated by three different recognition pathways (classical, alternative, and lectin), all of which lead to sequential enzyme activation, protein cleavage, and function-enabling protein conformational changes. Among these cascades, complement component 3 (C3) is the central molecule to the complement activation. These three pathways of the complement activation converge at the point of cleavage of C3 with generation of biologically active products, C3a and C3b.
The classical pathway is often referred to as antibody-dependent pathway because it is strongly initiated by binding complement component 1q (C1q) to the fragment crystallizable domain (Fc) of immunoglobulin M (IgM) or immunoglobulin G (IgG) clusters via the pattern recognition molecule (PRM) C1q subcomponent. However, C1q can activate complements by recognizing distinct structures on damaged cells directly or through endogenous substances, such as hyperphosphorylated tau (23, 24). C1q binding to damaged cells induces autoactivation of C1 complex to cleave complement component 4 (C4) and complement component 2 (C2) to form the C3 convertase (C4b2b).
Mannan-binding lectin (MBL) is a central recognition molecule in the lectin pathway. As the pattern recognition molecules binding to oligosaccharide structures on the surface of microorganisms, MBL, ficolins, and collectins assemble together and activate the MBL-associated serine proteases (MASP1/2). Once activated, MASP1/2 cleaves C4 and C2 to form the C3 cleaving enzyme-C4b2b. The lectin pathway is also triggered by released DAMPs, such as ATP (25) and cytoskeletal proteins (26), or unmasked sugars and neo-antigens (27) from damaged cells that can be recognized by and bind to MBL to initiates phagocytosis. The studies in MBL-deficient mice have demonstrated the impaired removal of damaged cells (26).
In the classical and lectin pathways, C3 convertase (C4b2b) sequentially cleaves multiple C3 proteins into C3a and C3b. Some of the C3b are associated with the C4b2b to form complement component 5 (C5) convertase (C4b2b3b) to cleave C5 into C5a and C5b (28).
The complement activation in the alternative pathway is initiated when spontaneously cleaved C3b directly attaches to a permissive/acceptor surface on the pathogen or damaged tissue (29, 30). Cleavage of inactive C3 protein can be spontaneously hydrolyzed into the functional fragments C3a and C3b at low level. Upon hydrolysis, the C3 protein undergoes a dramatic structural change that exposes a binding site for complement factor B to form C3bBb (a C3 cleaving enzyme complex) and C3bBb3b (an alternative C5 cleaving enzyme) (28).
Enzymatic cleavage of C5 to C5a initiates the terminal complement cascade, leading to polymerization of complement component 9 (C9) and insertion of membrane attack complex into cell membranes to lysis their targets. C3a and C5a, as potent proinflammatory mediators, recruit neutrophils, macrophages, mast cells, basophils, and lymphocytes to the injury site and promote inflammatory factor expression through C3a and C5a receptors in these immune cells (22, 31–33). C3b and C5b covalently attaches to pattern recognition molecules on the cell membrane to provide the opsonic signal to phagocytes for ingestion (24, 34, 35). During the physiological condition, complement activation with the low level of C3b deposition facilitates elimination of foreign and altered host cells (such as clearance of apoptotic cells) without the release of dangerous signals (32, 33, 36).
Skeletal muscles can produce complement components, include C1q, C1r, C1s, C2 and C4 (37). Complement activation is detected in damaged skeletal muscles from animals and human patients (38, 39). At the very early stage of skeletal muscle injury, the complement system is activated by its contacts with tissue intracellular components (40). The activated complements then recruit immune cells to cause inflammation. When complement activation is inhibited, the invasion of neutrophils and macrophages to the skeletal muscle is attenuated (41, 42) and muscle pathology is ameliorated (38).
2.3 Muscle-resident macrophages and mast cells promote inflammation
In the injury site, sentinel cells of the immune system (such as mast cells, macrophages, dendritic cells, innate lymphoid cells, and basophils) and non-immune system (such as endothelial cells) sense and react to DAMPs to produce proinflammatory cytokines (e.g., TNF-α and IL-1β), vasoactive amines (e.g., histamine and serotonin), nitric oxide (NO), ROS, neuropeptides, and arachidonic acid metabolites (e.g., prostaglandins and leukotrienes), which promote inflammatory responses through the recruitment of more neutrophiles and monocytes (Figure 1) (43–48).
Unlike monocyte-derived macrophages released from bone marrow and recruited to tissues during the injury with CCR2 activation (49), tissue-resident macrophages originate from the yolk sac and fetal liver during development and persist in many tissues via self-renewal. Tissue-resident macrophages express a wide array of receptors for the recogniztion of DAMPs, such as Toll-like receptors, nucleotide oligomerization domain (NOD)-like receptors, retinoic-acid inducible gene I (RIG-I) family, lectins, and scavenger receptors (50). Cells with these receptors act as local responders to the tissue damage and rapidly sense the death of individual cells. After initial recognition of the tissue damage, tissue-resident macrophages release inflammatory cytokines (TNF, IL-1, IL-6, IL-8, and IL-12) and chemokines (CXCL1, CXCL2, and CXCL5) (51) to drive the influx of inflammatory leukocytes, classically neutrophils and monocytes, from blood to the injured muscle (52).
Mast cells are located in the connective tissue that contacts close with the external environment. They are thought to play a pivotal role in allergy. IgE is thought to have a central role in the activation of mast cells through cross-linking of its high-affinity receptors (FcεIRs), whereas non-IgE-mediated activation of mast cells has been regarded as potentially important factor in the initation and amplification of acute inflammatory responses induced by tissue injury (53–55). DAMPs released from injured tissues, such as ATP (56) and IL-33 (45, 57), are recognized by mast cells via their receptors (P2X and P2Y receptors for ATP, ST2 receptor for IL-33), and then recognized DAMPs increase intracellular Ca2+ and activate mast cell degranulation. C3a and C5a, two complement components, can stimulate mast cell migration and degranulation via C3aRs and C5aRs (58, 59). The main contents in mast cell granules include histamine, heparin, serotonin, proteases, proteoglycans, cathepsin G, and cytokines (60, 61). Many of these mediators can induce inflammation and vasodilatation (55). The early disruption of the myofiber membrane elicits the accumulation and activation of muscle-resident mast cells. Activated mast cells subsequently degranulate and release inflammatory mediators (i.e. TNFα, IL-1, and histamine) to promote further immune cell recruitments (62, 63).
3 Inflammation promotes injured muscle regeneration
The damaged skeletal muscle has the intrinsic capacity to regenerate and repair itself through myogenesis with the satellite stem cell activation triggered by damaged myofiber-derived factors (64). Activated satellite stem cells undergo proliferation and differentiation, which eventually fuse together or combine with damaged fibers to reconstitute the fiber integrity and function (65). Upon the tissue injury, infiltrating macrophages engulf and digest dead cells and cellular debris via phagocytosis, which causes a phenotypic change of macrophages to become healing macrophages for the regulation of inflammation, myoblast fusion and growth, fibrosis, vascularization, and final return to homeostasis (Figure 2) (66, 67).
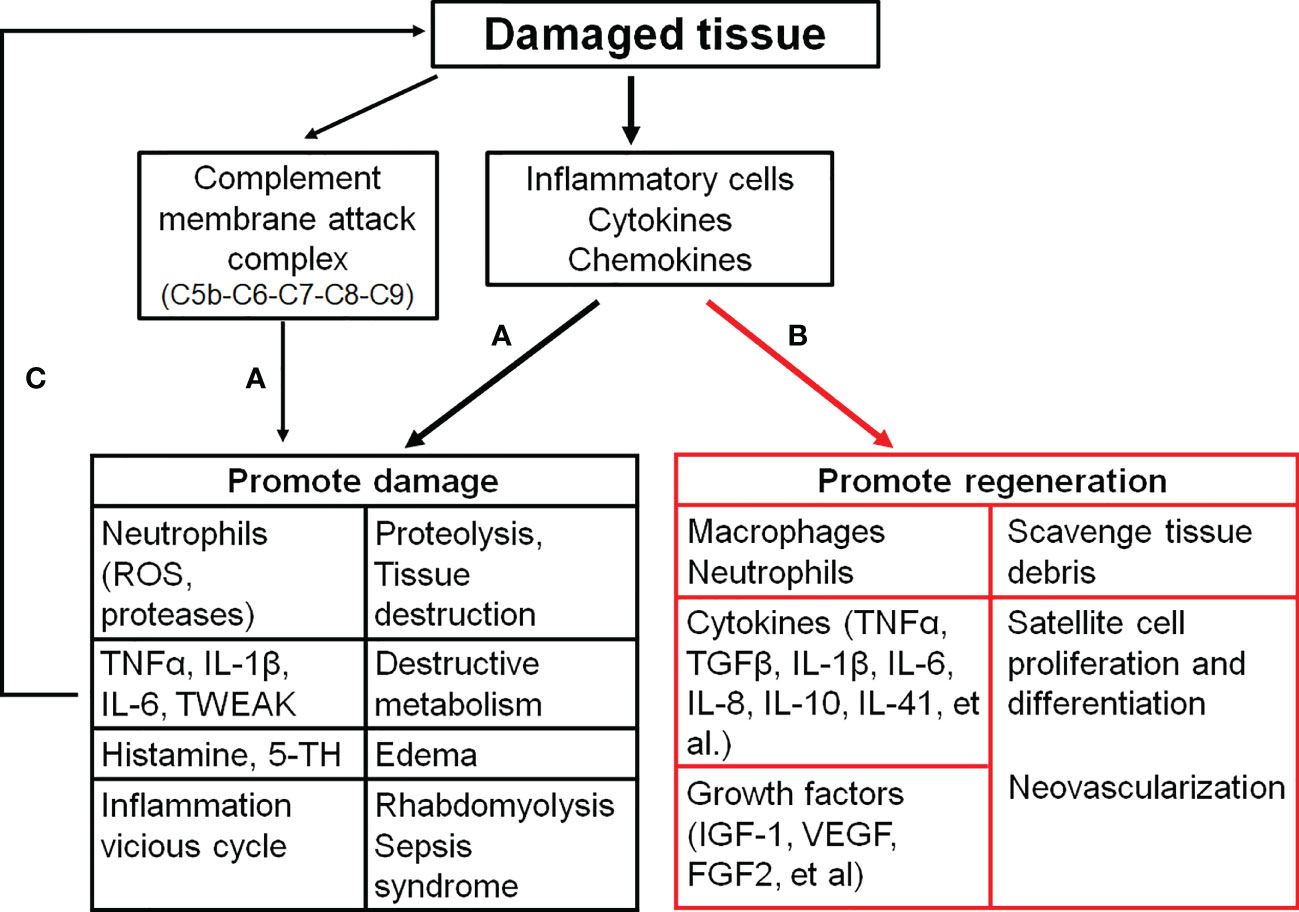
Figure 2 The effects of inflammatory activation on the skeletal muscle. (A), inflammation distorys tissues; (B), inflammation promtes muscle repair; (C), a vicious cyle of the tissue damage and inflammation. C5b (6, 7, 8, 9), complement component 5b (6, 7, 8, 9); FGF2, Fibroblast growth factor 2; IGF-1, Insulin-like growth factor 1; IL-1β (6, 8, 10, 41), interleukin-1β (6, 8, 10, 41); ROS, Reactive oxygen species; TGFβ, Tranformaing growth factor-β; 5-TH, Serotonin; TNFα, Tumor necrosis factor α; TWEAK, TNF-like weak inducer of apoptosis; VEGF, Vascular endothelial growth factor.
3.1 Skeletal muscle damage triggers the inflammation to scavenge muscle debris
Necrotic myofibers may act as either atrophic factors to repress myoblast growth or physical barriers to prevent myoblast fusion. Engulfment of dead cells by phagocytes is a key event that ensures an efficient skeletal muscle regeneration to start the repair process and end the pro-inflammatory response (68). Most clearence of tissue debris is performed by macrophages and neutrophils through phagocytosis when damaged cells expose “find-me” and “eat-me” signals released from intracellular contents or appeared on their membranes (69, 70). These professional phagocytes are attracted by these specific “find-me” signals released by damaged cells. Then the multiple receptors on the cell membrane of phagocytes recognize phosphatidylserine (a key “eat-me” signal) exposed on the surface of damaged cells to capture damaged cells and the lysosomes in the phagocytes efficiently digest and decompose the internalized materials through phospholipases and hydrolases (71, 72).
As the professional phagocytes, macrophages perform their critical functions to scavenge debris for skeletal muscle repair. Several types of scavenger receptors are found in macrophages to bind with and internalize a variety of ligands, including endogenous proteins and pathogens, and modulate macrophage activation (73, 74). Macrophage scavenger receptor class A (SRA) binds with HMGB1 to eliminate HMGB1 from interstitial space by internalization, and then activates TLR4 to stimulate cytokine production (75). Additionally, elimination of exposed cellular components is a process to resolve inflammation (49, 68). The phagocytosis of muscle cell debris induces a switch from pro-inflammatory macrophages (M1) to anti-inflammatory macrophages (M2) (49, 68, 76) to stimulate myogenesis and fiber growth (77). Insufficient infiltration of macrophages or phagocytosis of necrotic fibers partially impairs myogenesis (78). Deletion of macrophage scavenger receptors decreases macrophage phagocytic activity on myoblast debris, and blocks the transition of macrophage phenotypes from M1 to M2, which delays muscle regeneration (79).
3.2 Inflammation-related cytokines promote muscle regeneration
Immune cells release a large number of cytokines, such as TNF-α, IL-1β, IL-6 and TGFβ, and growth factors. These cytokines can stimulate expansion of the muscle stem cells to promote repair (80).
TNF-α and IL-1β are mainly produced by macrophages. Both TNF-α and IL-1β directly activate the production of IL-6 in multiple cell types, including macrophages, T cells, and myofibers (81, 82). TNF-α and IL-1β induce the proliferation of cultured myoblast cells by similar mechanisms, whereas they regulate the transitory phase of myoblast differentiation through other mechanisms. Hight levels of TNF-α and IL-6 stimulate myoblast proliferation via STAT3 signaling, while they inhibit subsequent myoblast differentiation through NF-κB (p50/p65)-mediated degradation and destabilization of myogenic regulatory factors, including myoblast determination protein 1 (MyoD, a transcription factor that induces cell cycle arrest for the regulation of muscle cell differentiation) and myogenin (MyoG, a transcription factor that regulates myocyte fusion to induce myogenesis) (83). IL-1β decreases the level of myostatin, a negative regulator of muscle growth and regeneration to trigger myoblast proliferation (81). On the other hand, low level of TNF-α and IL-6 is necessary to facilitate later stages of myogenesis, because TNF-α and IL-6 at a low level stimulate myoblast differentiation and fusion through p38MAPK and the alternative NF-κB (p52/ReIB) pathway (81, 83). Ablation of TNF-α or IL-6 displays poor muscle regeneration (4).
Infiltrating macrophages recruited via CCR2 produce insulin-like growth factor-1 (IGF-I) in the injured muscle to stimulate muscle regeneration (84, 85). Meteorin-like protein (Metrnl/IL-41), identified as a myokine/cytokine, is secreted by the skeletal muscle (86) or activated macrophages (87). Metrnl/IL-41 promotes macrophage differentiation to an anti-inflammatory phenotype (M2) and induces IGF-1 production in M2 macrophages through activation of signal transducer and activator of transcription (STAT) proteins, which has a direct effect on the proliferation of primary muscle satellite cells (88). Macrophage-specific Metrnl/IL-41 knockout impairs muscle repair (88). M2 macrophages secret TGFβ to promote myogenesis through stimulating myogenic precursor cell commitment into differentiated myocytes and the formation of mature myotubes (89).
HMGB1 is also displays the regenerative character. These contrasting effects of HMGB1 depend on the redox state of cysteine residues (90). HMGB1 contains three cysteines (C23, C45, C106), which can be reduced or oxidized. If all cysteines are oxidized, HMGB1 has no known proinflammatory activity. The oxidation of the C23 and C45 residues leads to the formation of an intramolecular disulfide bond (dsHMGB1). Both dsHMGB1 and full reduced HMGB1 (frHMGB1) have the migrating function of macrophages. The dsHMGB1 is a proinflammatory cytokine to polarize macrophages toward pro-inflammatory phenotype (M1) through binding to RAGE/TLR4. The frHMGB1 induces distinct macrophages polarization phenotypes (90, 91). The frHMGB1 forms a heterocomplex with CXCL12 and activates CXCR4 expressed on stem cells to promote muscle regeneration and repair after acute muscle injury (92, 93). Compared to an HMGB1-RAGE/TLR4-axis in immune cells as a proinflammatory signaling pathway for the impairment of skeletal muscle function, the HMGB1-CXCL12-CXCR4 signaling pathway in stem cells promotes tissue regeneration in chronic inflammation diseases (16, 19, 93). The oxidation of HMGB1 cysteines can switch its extracellular activities from the orchestration of tissue regeneration to the exacerbation of inflammation. Pharmacological treatment with an engineered nonoxidizable variant of HMGB1 reduces inflammation and fibrosis, and improves muscle regeneration and functional performance (94). Additionally, studies with RAGE knockout or defective TLR4 demonstrate that HMGB1 binding to RAGE/TRL4 in the stem cells is also important to stimulate quiescent stem cell proliferation and differentiation, and further promotes muscle regeneration and neovascularization after the muscle is destroyed (15, 95–97). These systemically genetic modifications possibly impair the response of immunocytes to the tissue injury or other unknown signaling to reduce the cell proliferation and differentiation. TLR4 deficient mice developed into severe muscle injury (96), mild inflammation with low TNF-α and scarce macrophage infiltration, and poor muscle regeneration (98). RAGE is not expressed in the adult skeletal muscle, while it is transiently expressed in activated, proliferated, and differentiated satellite cells in injured muscles. The RAGE signaling represses Pax7 transcription in satellite cells through upregulation of MyoG, thereby accelerating muscle regeneration (myocyte fusion) and limiting satellite cell self-renewal (97). Satellite cells from RAGE knockout mice not only lack a high level of some cytokines (TNFα, MCP-1, IL- 6) in response to in vivo ischemia and in vitro stimuli with HMGB1 (99), but also exhibit the increase in basal satellite cells and delayed regeneration (myocyte fusion) of injured muscles (97).
3.3 Inflammation related cytokines promote neovascularization in skeletal muscle
Neo-angiogenesis is also necessary to establish a new vascular network for muscle repair. Both macrophages and mast cells contribute vascular regeneration (100, 101). In damaged skeletal muscles, endothelial-derived progenitors can contribute to neo-angiogenesis or fibrosis through the generation of mesenchymal/fibrogenic cells. The polarized macrophages affect the fate of endothelial progenitors during muscle regeneration after an acute injury. Experiments performed by Zordan et al. have demonstrated that the vast majority of endothelial-derived cells contributes to the formation of a new capillary network with macrophage infiltration (102). When circulating monocytes and infiltrating macrophages are depleted, angiogenesis and myogenesis are delayed with leading to a persistent fibrosis (102). Vascular endothelial growth factor (VEGF) signaling has a crucial role in this transformation. When the muscle trauma results in the disruption of blood flow and reduction of oxygen, hypoxia inducible factor (HIF) is elevated in the injured muscle, which induces the production and release of VEGF from macrophages to bind to VEGF receptors (VEGFR) expressed in endothelial cells for the proliferation, migration, and survival of endothelial cells (103, 104). Depletion of the macrophage recruitment reduces the VEGF production and impairs angiogenesis and skeletal muscle regeneration (102, 105). Macrophage-derived VEGF is also crucial to re-establishment of the neuromuscular junction (106). Other pro-angiogenic factors produced by macrophages, including fibroblast growth factor 2 (FGF2), IL-8, IGF-1, and IL-10 (101), also improve tissue repair. Additionally, macrophages stimulate myogenesis/angiogenesis coupling to orchestrate muscle regeneration through secreted osteopontin (107) and oncostatin M production (108).
Local mast cells are also associated with arteriogenesis and formation of collateral circulation in the skeletal muscle after ischemic injury (100, 109). In patients with peripheral arterial disease, or animal models with femoral artery ligation, mast cells are activated (62, 100, 109, 110). Activation of mast cells increases the proliferation of vascular endothelin cells and smooth muscle cells to promote neovascularization (100, 109). Treatment with cromolyn, a mast cell stabilizer, prevents the mast cell-induced arteriogenesis. Mast cells could directly contribute to vascular remodeling and vascular cell proliferation through the increase in matrix metalloproteinases’ (MMPs) activities and monocyte responses as well as supplement of growth-promoting factors, including VEGF FGF2, and platelet-derived growth factor BB (PDGF-BB) (109).
4 Inflammation aggravates muscle injury
As discussed above, inflammation is involved in skeletal muscle regeneration and has beneficial on muscle healing. However, a number of pro-inflammatory cytokines/chemokines also contribute to the pathogenesis of skeletal muscle injuries (Figure 2) (111). Therefore, anti-inflammatory modalities are commonly used for the treatment of various musculoskeletal injuries (112). Treatment with dexamethasone, a potent anti-inflammatory drug, protects the skeletal muscle from ischemia/reperfusion injuries through the inhibition of inflammation (113–117). Dexamethasone attenuates the alterations in microvascular function, edema, and necrosis of muscle fibers, and improves the muscle contractile function (113–117).
4.1 Inflammatory response aggravates muscle injury
After trauma, local and recruited immune cells are activated in the injured site. Activated lymphocytes, macrophages, and neutrophils contain radical forming enzymes in their intracellular granules to generate ROS. ROS can further increase tissue injuries and in turn enhance the immune responses to the tissue damage (118–120). Macrophages are rich in diverse growth factors and cytokines as well as ROS (121, 122). Therefore, macrophages play the opposite roles in the skeletal muscle to injure muscle cells or stimulate muscle regeneration. Pro-inflammatory cytokines released from activated phagocytes have been found to accelerate muscle protein degradation in patients with trauma (123, 124). Recently, Shang et al. reported that macrophages appear to compete with satellite cells for binding with glutamine to impede muscle regeneration (125). A macrophage-specific knockout of glutamate dehydrogenase inhibits the glutamine utilization in macrophages and improves earlier restoration of muscle functional capacity (80, 125).
Unlike macrophages, neutrophiles mainly release proteases to degrade cellular debris produced by the damaged tissue (126). As a part of neutrophil activation, neutrophils lead to proteolysis and removal of debris, high concentration of proteases, or other cytolytic and cytotoxic molecules released from neutrophils. These neutrophil-caused events can damage skeletal muscles and other healthy bystander tissues (48). Over-activation of neutrophils lyses the cell membrane (127) and results in the muscle damage (128). As one type of mediators, neutrophil-derived ROS are capable of direct lysis of the muscle membrane (129–131). Additionally, oxidative stress exacerbates the inflammatory responses and enhances the formation of fibrotic scar tissues after the skeletal muscle injury (132). Inhibition of the neutrophil infiltration attenuates the muscle damage (133, 134).
Mast Cells also play a prominent role in the ischemia/reperfusion-mediated cytotoxic injury in the skeletal muscle. Mast cell granules contain a number of mast cell-specific proteases, including tryptases, chymases, and mast cell carboxypeptidase A (MC-CPA) (135). These mast cell proteases are expressed at exceptionally high levels and kept in a fully active form. At the blood reperfusion, complement molecules, C3a and C5a, cause mast cell degranulation through activation of G-protein-coupled receptors (GPCR) on the mast cell surface. Additionally, increased ROS production also activates the intracellular signaling pathways to stimulate mast cell degranulation (60). When mast cells undergo degranulation, large amounts of enzymatically active proteases are thus released into the extracellular space to result in the tissue damage (136, 137). Drugs that target mast cells and their mediators (138), genetical deficiency in mast cells (138–140), or direct knockout of mast cell proteases (141) reduce the skeletal muscle ischemia-reperfusion injury accompanied with the attenuation of remote lung injury.
Since pro-inflammatory cytokines are found to accelerate the muscle protein degradation (142), lots of inflammatory mediators involved in the skeletal muscle injury have been reported (83, 141, 143–145). Although these cytokines modulate myofiber function and execute pleiotropic roles in the functional recovery of the skeletal muscle, they disrupt healing and exacerbate the muscle dysfunction when they form an aberrant downstream signaling pathway (21). Accompanied with the initiation of inflammation, HMGB1, amplifies the tissue damage and lethality through the HMGB1/RAGE axis (146). As a co-receptor of HMGB1 for the TLR activation, macrophage scavenger receptor A (SRA) mediates HMGB1 internalization (75) and interaction with TLR4 (147, 148) to exaggerate inflammatory responses. SRA-mediated influx of lipids through macrophage-modified lipoprotein uptake is thought to be involved in the formation of foam cells (149). In Duchenne muscular dystrophy (DMD), the inflammatory HMGB1-TLR4 axis promotes the dystrophic muscle pathological process and destroys dystrophic muscle fibers (16, 94). Ablation of TLR4 or inhibition of HMGB1 binding to TLR4 attenuates inflammation and improves the muscle histopathology and muscle force generation (16, 94).
TNF-α and IL-1β limit cell differentiation events and lead to muscle wasting (81, 150–152). Although high levels of TNF-α and IL-1β stimulate myoblast proliferation, they decrease the production of irisin, an important myokine that can stimulate myogenesis and muscle growth (81). Additionally, TNF-α inhibits myoblast differentiation through the degradation of myogenic regulatory factors (MyoD and MyoG) and downregulation of osteonectin, a secreted protein involved in the differentiation of pulp cells during the development and repair (81). TNF‐α blockade reduces TNF-α-associated tissue degradation and positively regulates the restauration of skeletal muscles upon injuries (153).
Complements not only activate inflammation but also aggravate the tissue injury (154). Complement activation converges at the point of cleavage of complement component 3 (C3) with the generation of biologically active products (C3a and C3b). C3a recruits neutrophils and macrophages to the injury site, while C3b activates the remainder of the complement cascade (C5-C9) to lead to the formation of membrane attack complex (MAC). MAC leads to the pore formation in the skeletal muscle membrane (38). Loss of the membrane integrity results in the release of intracellular contents, such as DAMPs. Then a severe and sudden reaction of the complement cascade against DAMPs could lead to the hyper-inflammation and tissue damage (155). Inactivation of complements or complement knockout reduces the invasion and activation of neutrophils and macrophages, and attenuates vascular damage (156, 157), muscle injury (38, 145, 158, 159) and edma (41, 159) in the damaged skeltal muscle. Additionally, inhibition of complements reduces remote pulmonary injuries secondary to tourniquet-induced skeletal muscle damage (157, 159).
4.2 The vicious cycle of tissue damage and inflammation-exaggerated muscle injury
After the muscle damage, intracellular DAMPs are released into interstitial space and the complement system and local macrophages/mast cells are activated. All of these local inflammatory events promote immune cell recruitment and activation with the high level production and release of inflammatory factors to trigger a vicious cycle of the tissue damage and inflammation (Figure 2) (5, 6).
Upon the tissue injury, released DAMPs activate the immune system to produce proinflammatory cytokines. As a specific DAMP and a necrotic marker for the immune system, HMGB1 initiates the proinflammatory signaling pathways and stimulates the immune cell activation (160). Activated immune cells also release HMGB1 (13, 161). Once the tissue damage becomes a prolonged event and the tissue repair fails, HMGB1 released by necrotic tissues and immune cells induces the second wave of inflammatory responses (162) or chronic inflammation (163, 164). Continuous inflammation can contribute to the development of various inflammatory diseases. Inflammatory diseases, in turn stimulate the secretion of DAMPs, thus establishing a vicious cycle of DAMPs production and inflammation (6, 162, 165). Continuously chronic inflammation results in muscle loss and atrophy (166).
In the alternative pathway of complement activation, C3 convertase initially cleaves C3 to C3a and C3b fragments. Then C3b binds with factor B to form C3bBb, a C3 convertase, to further cleave C3, which results in a positive feedback amplification loop (167) to produce a large amount of C3a and C5a fragments and promote the inflammatory responses, such as neutrophil recruitment and activation. When neutrophils are activated, they in turn activate the alternative complement pathway for the release of C5a fragments and the latter further amplifies neutrophil proinflammatory responses with another positive feedback loop (168). A severe reaction of the complement cascade can lead to the hyper-inflammation (30, 169) and tissue damage (29), or chronic inflammation (24).
Mast cell degranulation and macrophage activation can release proinflammatory cytokines (such as TNF-α, IL-1, histamine) (170, 171) to recruit more mast cells, neutrophiles and other immune cells. Then these recruited immune cells further release more proinflammatory cetokines (172). As the results, more immune cells infiltrate to the injured site following chemokine gradient to further promote inflammation, especially for neutrophil congregation in which a large number of neutrophils are recruited and collectively move toward the injured site in a very directed manner, i.e., prior activation of one or more ‘‘leading’’ neutrophils secretes leukotriene B4 (LTB4) and ATP. These molecules are recognized by A3 receptors (A3Rs) and LTB receptors (LTB4Rs) expressed on following neutrophils for the further neutrophil recruitment. This neutrophil–neutrophil signaling results in an autocorrelated behavior described as neutrophil swarming (9, 48, 173).
The muscle necrosis together with inflammation results in the accumulation of substantial amounts of fluid to raise the intracompartmental pressure in the affected limbs. The high level of the intracompartmental pressure provokes the additional damage and leads to more muscle necrosis, even limb amputation (174–177).
Not all responses to the muscle injury form a vicious cycle. Marcophages also suppress inflammation and autoimmunity in response to self-antigens caused during homeostasis (46). In the local minor injury, tissue-resident macrophages rapidly sense the death of individual cells and extend membrane processes that physiologically sequester pro-inflammatory debris to prevent initiation of the feedforward chemoattractant signaling cascade for the formation of neutrophil swarms (48). In the acute inflammation, tissue-resident macrophages release IL-10 to inhibit neutrophil scrolling and migration into the tissue and subsquently attenaute the inflammation (178).
5 Exaggerated inflammation causes remote organ damage
A local inflammation in the injured skeletal muscle can become more systemic and lead to the tissue damage and edema at distant sites (such as lungs). The decreased microperfusion of blood and tissue hypoxia due to the lung damage could cause more tissue damage (5).
5.1 Rhabdomyolysis
In the skeletal muscle, the damage initially occurs as “tears” in the membrane, which destroys the integrity of the sarcolemma and reduces the connection of the muscle membrane to cytoskeleton (179). Lack of the connection with cytoskeleton causes myofibers to be fragile and more sensitive to damage (180). This type of the damage can be exacerbated by the inflammatory response, leading to myofiber necrosis rather than repair (170).
Disintegration and necrosis of the skeletal muscle result in rapid breakdown of skeletal muscle fibers and release of muscular cell constituents into the extracellular fluid and circulation (12, 174), which is referred to as rhabdomyolysis. Rhabdomyolysis is usually caused by the direct muscle injury, such as trauma, and inflammation results in an additional injury and promotes rhabdomyolysis (181–183). Acute kidney injury (AKI) is one of the most severe complications after the occurrence of rhabdomyolysis and happens in 33-50% of patients with rhabdomyolysis (184) because of the toxicity of myoglobin to kidney tubular cells (182, 185). Previous studies also reported that severe acute inflammatory myosis without trauma triggers rhabdomyolysis with acute kidney injury (181, 186–188). These studies confirm that severe muscle inflammation contributes to the tissue damage and organ dysfunction (182). Another serious complication of rhabdomyolysis is severe hyperkalemia and the latter causes cardiac arrhythmia and arrest (189–191).
5.2 Severe inflammation in skeletal muscle results sepsis like syndrome
Thirty years ago, microbial pathogens were thought to cause the clinical sepsis syndrome and the relationship between the circulating mediators of inflammation and post-injury sepsis could not be imaged (192, 193). Now we understand that sepsis is fundamentally an inflammatory disease, and even infectious pathogens are not detectable in about one third of patients displayed clinical signs of sepsis (194), although sepsis is traditionally defined as life-threatening organ dysfunction caused by dysregulated host responses to infection (193). Sepsis is the consequence of exaggerated immune responses and widespread inflammation in the body to generate cytokine storm and results in life-threatening organ dysfunction (193, 195, 196).
Inflammatory cytokines are synthesized at the site of tissue injury where the sterile DAMPs released from wound sites activate innate immune cells (192). After severe tissue damage initiates massive activation of inflammatory mediators, the activated inflammatory mediators release into the bloodstream. Massive inflammatory mediators in the bloodstream result in systemic inflammation and multiple organ failure and death (12, 197), named sepsis-like systemic inflammation response syndrome (SIRS) (198).
In damaged skeletal muscles, proinflammatory cytokines, including IL-1β and TNFα, are significantly elevated (115, 116). As the early mediators of endotoxemia, IL-1β and TNFα mainly released by macrophages from the injured site into circulation cause septic shock and multiple organ injuries. Their antibodies have been used to prevent the organs against the lethal damage in mice suffered systemic inflammation (199–204). Additionally, IL-1β and TNFα stimulate the release of HMGB1, a late mediator of endotoxemia, and exaggerate the inflammatory damage (13). In a tourniquet-induced mouse hindlimb ischemia-reperfusion model, complement inhibition or neutrophil depletion attenuates remote organ injuries in the lung and liver (205), which confirms that local muscle injury results in the systemic inflammation and remote organ damage.
DAMPs are key inducers of systemic sterile inflammation. As a late mediator of endotoxin lethality, HMGB1 is secreted by activated monocytes and macrophages, or passively released from the damaged skeletal muscle into circulation (12, 13). In the human sepsis, the serum HMGB1 is increased, especially in non-survivors (13). Furubeppu, et al. have demonstrated that bilateral hindlimb ischemia not only induces severe muscle damage, but also significantly causes the elevation of serum HMGB1 levels and animal death (12). Treatment with anti-HMGB1 antibodies markedly improves animal survival (12, 13). Macrophage scavenger receptor A (SRA) mediates HMGB1 internalization (75) and interaction with TLR4 (147, 148) to enhance the development of the pro-inflammatory phenotype and mediate the morbidity and mortality of sepsis/septic shock, whereas the deletion of SRA or inhibition of SRA interaction with HMGB1 ameliorates sepsis/septic shock (147, 148).
Mitochondria and its components are another source for circulating DAMPs to activate systemic inflammation (192). The mitochondrial genome (mtDNA) contains CpG DNA repeats and also codes for formylated peptides. Unmethylated ‘CpG’ repeats existed in the mitochondrial DNA confer the affinity for innate immune cells with TLR9, and formylated peptides bind to formyl peptide receptor-1 to activate human polymorphonuclear neutrophils (PMN) through promoting Ca2+ influx and phosphorylation of mitogen-activated protein kinases (MAPKs), thus leading to PMN migration and degranulation (198). Intravenous injection of crude mitochondrial preparations causes neutrophil-mediated attack on the lung (198).
In addition to the involvement of neutrophil-mediated organ injury (198), the systemic inflammation also initiates clotting (206) to reduce blood flow into limbs and vital organs. Poor circulation leads to the organ failure and even animal death. Furthermore, clinical investigations and animal studies found that the systemic inflammation increases protein degradation and suppresses protein synthesis in the skeletal muscle, leading to an amplified net catabolism (143, 207).
6 Conclusion
Sterile inflammation is a host defensive reaction to scavenge damaged tissues for wound healing. The outcome is influenced by the magnitude of the inflammatory response whether the inflammatory process has an overall beneficial or detrimental effect on muscle function, and how to balance the beneficial and detrimental effect should be highlighted in the clinical practice (Figure 3). The inflammatory response consists of hormonal metabolic and immunological components and the extent correlates with the magnitude of the tissue injury (208). For tissue microlesions, tissue-resident macrophages sequester the damage through extending membrane processes to prevent initiation of inflammation (48). The macrophage activation can create a favorable microenvironment to release inflammatory cytokines for damaged tissue repair. This process is very useful for cells with the ability of regeneration. For other tissues without the regenerative capacity, inflammatory cytokines promote the fibrosis, such as in the heart. Given the skeletal muscle intrinsic capacity for regeneration and the benefit of inflammation on muscle repair, inflammation has less side effects on muscle recovery. However, inflammation also impairs muscle homeostasis in the patients with poor muscle stem cell pool, such as patient with peripheral arterial disease (209). Furthermore, severe tissue damage can cause systemic inflammation response syndrome and life-threatening organ dysfunction (12), especially, multiple remote organ damage, such as in the lung, heart, and kidney. To focus on this point, anti-inflammation could be a life-saving strategy. Sometimes, amputation rather than attempts at revascularization is the most prudent course to limit the toxic products from the damaged limb into the systemic circulation (210).
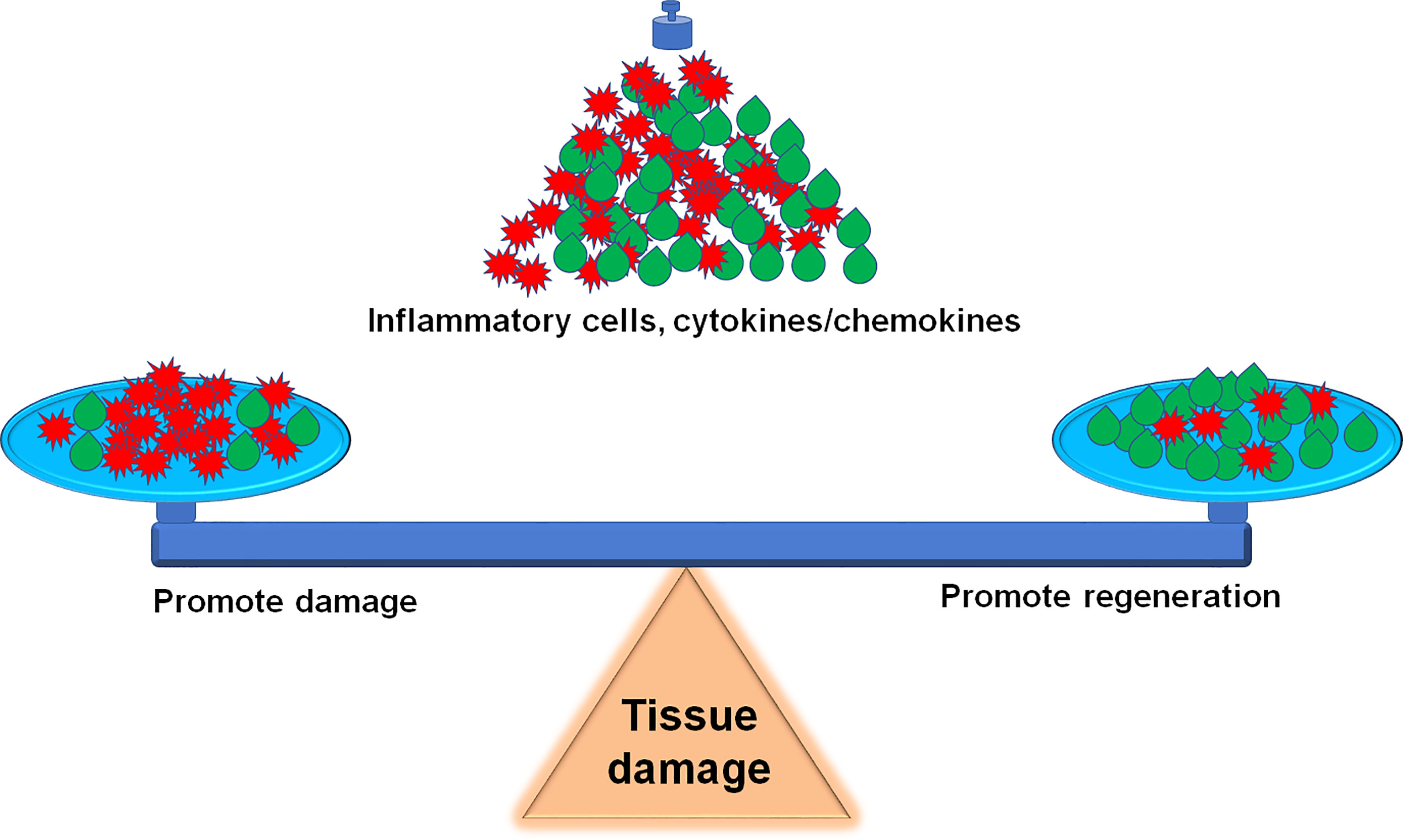
Figure 3 Inflammation balance in skeletal muscle damage and repair. How to accurately balance the beneficial and detrimental effect of inflammation during the skeletal muscle damage and repair is a challenge for precise therapeutic strategies.
We updated the information about the inflammation balance in the skeletal muscle damage and repair in this review. It is a great challenge to balance the beneficial and detrimental effect of inflammation during the skeletal muscle damage and repair (Figure 3). Following the development of innovative techniques, including epigenetics, transcriptomics, single-cell RNA sequence (scRNA-seq) and proteomics, etc., more details on the involvement of inflammatory factors and immune cells in the skeletal muscle damage and repair can be further explored. For example, scRNA-seq analysis provides a new benchmark reference resource to examine the muscle tissue heterogeneity and identify potential targets for accurate therapy. Using scRNAseq analysis, Pang, et al. demonstrate that elevation of cell cycle genes in the specific monocyte/macrophages promotes inflammation and impairs skin wound healing (211). The use of scRNA-seq analysis resolves the cellular diversity of human muscles, including four types of stromal cells, five types of vascular cells, and two subpopulations of muscle stem cells (212). The proportion of different cell-types and cell-subtypes relates to age, sex, and the pathophysiology of muscle diseases (213). Krasniewski, et al. report eleven clusters of distinct macrophages in the mouse skeletal muscle, measured by scRNAseq analysis and also demonstrate that the enriched gene expression programs link to reparative, proinflammatory, phagocytic, proliferative, and senescence-associated functions (214). Therefore, the development of these new techniques will advance the new precise therapeutic strategies, including attenuation of the muscle damage and promotion of the muscle repair.
Author contributions
HT and Y-LL contributed to drafting the work and revising it critically for important intellectual content. Both authors contributed to the article and approved the submitted version.
Funding
This study was supported by the National Institute of General Medical Sciences, National Institutes of Health, USA (R01GM-145736 to Y-LL).
Conflict of interest
The authors declare that the research was conducted in the absence of any commercial or financial relationships that could be construed as a potential conflict of interest.
Publisher’s note
All claims expressed in this article are solely those of the authors and do not necessarily represent those of their affiliated organizations, or those of the publisher, the editors and the reviewers. Any product that may be evaluated in this article, or claim that may be made by its manufacturer, is not guaranteed or endorsed by the publisher.
References
1. Forcina L, Cosentino M, Musaro A. Mechanisms regulating muscle regeneration: Insights into the interrelated and time-dependent phases of tissue healing. Cells (2020) 9:1297. doi: 10.3390/cells9051297
2. Chen GY, Nunez G. Sterile inflammation: Sensing and reacting to damage. Nat Rev Immunol (2010) 10:826–37. doi: 10.1038/nri2873
3. Eltzschig HK, Eckle T. Ischemia and reperfusion–from mechanism to translation. Nat Med (2011) 17:1391–401. doi: 10.1038/nm.2507
4. Yang W, Hu P. Skeletal muscle regeneration is modulated by inflammation. J Orthop Translat (2018) 13:25–32. doi: 10.1016/j.jot.2018.01.002
5. Pierce A, Pittet JF. Inflammatory response to trauma: Implications for coagulation and resuscitation. Curr Opin Anaesthesiol (2014) 27:246–52. doi: 10.1097/ACO.0000000000000047
6. Roh JS, Sohn DH. Damage-associated molecular patterns in inflammatory diseases. Immune Netw (2018) 18:e27. doi: 10.4110/in.2018.18.e27
7. Zhang X, Mosser DM. Macrophage activation by endogenous danger signals. J Pathol (2008) 214:161–78. doi: 10.1002/path.2284
8. Gong T, Liu L, Jiang W, Zhou R. Damp-sensing receptors in sterile inflammation and inflammatory diseases. Nat Rev Immunol (2020) 20:95–112. doi: 10.1038/s41577-019-0215-7
9. Zindel J, Kubes P. DAMPs, PAMPs, and LAMPs in immunity and sterile inflammation. Annu Rev Pathol (2020) 15:493–518. doi: 10.1146/annurev-pathmechdis-012419-032847
10. Venereau E, Ceriotti C, Bianchi ME. DAMPs from cell death to new life. Front Immunol (2015) 6:422. doi: 10.3389/fimmu.2015.00422
11. Scaffidi P, Misteli T, Bianchi ME. Release of chromatin protein HMGB1 by necrotic cells triggers inflammation. Nature (2002) 418:191–5. doi: 10.1038/nature00858
12. Furubeppu H, Ito T, Kakuuchi M, Yasuda T, Kamikokuryo C, Yamada S, et al. Differential regulation of damage-associated molecular pattern release in a mouse model of skeletal muscle ischemia/reperfusion injury. Front Immunol (2021) 12:628822. doi: 10.3389/fimmu.2021.628822
13. Wang H, Bloom O, Zhang M, Vishnubhakat JM, Ombrellino M, Che J, et al. HMG-1 as a late mediator of endotoxin lethality in mice. Science (1999) 285:248–51. doi: 10.1126/science.285.5425.248
14. Rayavarapu S, Coley W, Kinder TB, Nagaraju K. Idiopathic inflammatory myopathies: Pathogenic mechanisms of muscle weakness. Skelet Muscle (2013) 3:13. doi: 10.1186/2044-5040-3-13
15. Sachdev U, Cui X, Tzeng E. HMGB1 and TLR4 mediate skeletal muscle recovery in a murine model of hindlimb ischemia. J Vasc Surg (2013) 58:460–9. doi: 10.1016/j.jvs.2012.11.071
16. Giordano C, Mojumdar K, Liang F, Lemaire C, Li T, Richardson J, et al. Toll-like receptor 4 ablation in mdx mice reveals innate immunity as a therapeutic target in duchenne muscular dystrophy. Hum Mol Genet (2015) 24:2147–62. doi: 10.1093/hmg/ddu735
17. Venereau E, Casalgrandi M, Schiraldi M, Antoine DJ, Cattaneo A, De Marchis F, et al. Mutually exclusive redox forms of HMGB1 promote cell recruitment or proinflammatory cytokine release. J Exp Med (2012) 209:1519–28. doi: 10.1084/jem.20120189
18. Panicucci C, Raffaghello L, Bruzzone S, Baratto S, Principi E, Minetti C, et al. eATP/P2X7R axis: An orchestrated pathway triggering inflammasome activation in muscle diseases. Int J Mol Sci (2020) 21:5963. doi: 10.3390/ijms21175963
19. Muth IE, Zschuntzsch J, Kleinschnitz K, Wrede A, Gerhardt E, Balcarek P, et al. HMGB1 and RAGE in skeletal muscle inflammation: Implications for protein accumulation in inclusion body myositis. Exp Neurol (2015) 271:189–97. doi: 10.1016/j.expneurol.2015.05.023
20. Rodriguez-Nuevo A, Diaz-Ramos A, Noguera E, Diaz-Saez F, Duran X, Munoz JP, et al. Mitochondrial DAN and TLR9 drive muscle inflammation upon Ppa1 deficiency. EMBO J (2018) 37:e96553. doi: 10.15252/embj.201796553
21. Qualls AE, Southern WM, Call JA. Mitochondria-cytokine crosstalk following skeletal muscle injury and disuse: A mini-review. Am J Physiol Cell Physiol (2021) 320:C681–C8. doi: 10.1152/ajpcell.00462.2020
22. Dunkelberger JR, Song WC. Complement and its role in innate and adaptive immune responses. Cell Res (2010) 20:34–50. doi: 10.1038/cr.2009.139
23. Ricklin D, Hajishengallis G, Yang K, Lambris JD. Complement: A key system for immune surveillance and homeostasis. Nat Immunol (2010) 11:785–97. doi: 10.1038/ni.1923
24. Schartz ND, Tenner AJ. The good, the bad, and the opportunities of the complement system in neurodegenerative disease. J Neuroinflamm (2020) 17:354. doi: 10.1186/s12974-020-02024-8
25. Ratajczak MZ, Adamiak M, Kucia M, Tse W, Ratajczak J, Wiktor-Jedrzejczak W. The emerging link between the complement cascade and purinergic signaling in stress hematopoiesis. Front Immunol (2018) 9:1295. doi: 10.3389/fimmu.2018.01295
26. Beltrame MH, Catarino SJ, Goeldner I, Boldt AB, de Messias-Reason IJ. The lectin pathway of complement and rheumatic heart disease. Front Pediatr (2014) 2:148. doi: 10.3389/fped.2014.00148
27. Ip WK, Takahashi K, Ezekowitz RA, Stuart LM. Mannose-binding lectin and innate immunity. Immunol Rev (2009) 230:9–21. doi: 10.1111/j.1600-065X.2009.00789.x
28. Reis ES, Mastellos DC, Ricklin D, Mantovani A, Lambris JD. Complement in cancer: Untangling an intricate relationship. Nat Rev Immunol (2018) 18:5–18. doi: 10.1038/nri.2017.97
29. Thurman JM, Ljubanovic D, Edelstein CL, Gilkeson GS, Holers VM. Lack of a functional alternative complement pathway ameliorates ischemic acute renal failure in mice. J Immunol (2003) 170:1517–23. doi: 10.4049/jimmunol.170.3.1517
30. Banda NK, Thurman JM, Kraus D, Wood A, Carroll MC, Arend WP, et al. Alternative complement pathway activation is essential for inflammation and joint destruction in the passive transfer model of collagen-induced arthritis. J Immunol (2006) 177:1904–12. doi: 10.4049/jimmunol.177.3.1904
31. Ruan CC, Gao PJ. Role of complement-related inflammation and vascular dysfunction in hypertension. Hypertension (2019) 73:965–71. doi: 10.1161/HYPERTENSIONAHA.118.11210
32. Merle NS, Noe R, Halbwachs-Mecarelli L, Fremeaux-Bacchi V, Roumenina LT. Complement system part II: Role in immunity. Front Immunol (2015) 6:257. doi: 10.3389/fimmu.2015.00257
33. Merle NS, Church SE, Fremeaux-Bacchi V, Roumenina LT. Complement system part I - molecular mechanisms of activation and regulation. Front Immunol (2015) 6:262. doi: 10.3389/fimmu.2015.00262
34. Acharya D, Li XRL, Heineman RE, Harrison RE. Complement receptor-mediated phagocytosis induces proinflammatory cytokine production in murine macrophages. Front Immunol (2019) 10:3049. doi: 10.3389/fimmu.2019.03049
35. Scieszka JF, Maggiora LL, Wright SD, Cho MJ. Role of complements C3 and C5 in the phagocytosis of liposomes by human neutrophils. Pharm Res (1991) 8:65–9. doi: 10.1023/a:1015830306839
36. Bosurgi L, Manfredi AA, Rovere-Querini P. Macrophages in injured skeletal muscle: A perpetuum mobile causing and limiting fibrosis, prompting or restricting resolution and regeneration. Front Immunol (2011) 2:62. doi: 10.3389/fimmu.2011.00062
37. Legoedec J, Gasque P, Jeanne JF, Scotte M, Fontaine M. Complement classical pathway expression by human skeletal myoblasts. vitro Mol Immunol (1997) 34:735–41. doi: 10.1016/s0161-5890(97)00093-x
38. Han R, Frett EM, Levy JR, Rader EP, Lueck JD, Bansal D, et al. Genetic ablation of complement C3 attenuates muscle pathology in dysferlin-deficient mice. J Clin Invest (2010) 120:4366–74. doi: 10.1172/JCI42390
39. Dufaux B, Order U. Complement activation after prolonged exercise. Clin Chim Acta (1989) 179:45–9. doi: 10.1016/0009-8981(89)90021-1
40. Linder E, Lehto VP, Stenman S. Activation of complement by cytoskeletal intermediate filaments. Nature (1979) 278:176–8. doi: 10.1038/278176a0
41. Frenette J, Cai B, Tidball JG. Complement activation promotes muscle inflammation during modified muscle use. Am J Pathol (2000) 156:2103–10. doi: 10.1016/S0002-9440(10)65081-X
42. Wang HA, Lee JD, Lee KM, Woodruff TM, Noakes PG. Complement C5a-C5aR1 signalling drives skeletal muscle macrophage recruitment in the hSOD1G93A mouse model of amyotrophic lateral sclerosis. Skelet Muscle (2017) 7:10. doi: 10.1186/s13395-017-0128-8
43. He Z, Ma C, Yu T, Song J, Leng J, Gu X, et al. Activation mechanisms and multifaceted effects of mast cells in ischemia reperfusion injury. Exp Cell Res (2019) 376:227–35. doi: 10.1016/j.yexcr.2019.01.022
44. Frangogiannis NG, Lindsey ML, Michael LH, Youker KA, Bressler RB, Mendoza LH, et al. Resident cardiac mast cells degranulate and release preformed TNF-alpha, initiating the cytokine cascade in experimental canine myocardial ischemia/reperfusion. Circulation (1998) 98:699–710.6. doi: 10.1161/01.cir.98.7.699
45. Enoksson M, Lyberg K, Moller-Westerberg C, Fallon PG, Nilsson G, Lunderius-Andersson C. Mast cells as sensors of cell injury through IL-33 recognition. J Immunol (2011) 186:2523–8. doi: 10.4049/jimmunol.1003383
46. Wynn TA, Chawla A, Pollard JW. Macrophage biology in development, homeostasis and disease. Nature (2013) 496:445–55. doi: 10.1038/nature12034
47. Davies LC, Taylor PR. Tissue-resident macrophages: Then and now. Immunology (2015) 144:541–8. doi: 10.1111/imm.12451
48. Uderhardt S, Martins AJ, Tsang JS, Lammermann T, Germain RN. Resident macrophages cloak tissue microlesions to prevent neutrophil-driven inflammatory damage. Cell (2019) 177:541–55.e17. doi: 10.1016/j.cell.2019.02.028
49. Watanabe S, Alexander M, Misharin AV, Budinger GRS. The role of macrophages in the resolution of inflammation. J Clin Invest (2019) 129:2619–28. doi: 10.1172/JCI124615
50. Davies LC, Jenkins SJ, Allen JE, Taylor PR. Tissue-resident macrophages. Nat Immunol (2013) 14:986–95. doi: 10.1038/ni.2705
51. Arango Duque G, Descoteaux A. Macrophage cytokines: Involvement in immunity and infectious diseases. Front Immunol (2014) 5:491. doi: 10.3389/fimmu.2014.00491
52. Brigitte M, Schilte C, Plonquet A, Baba-Amer Y, Henri A, Charlier C, et al. Muscle resident macrophages control the immune cell reaction in a mouse model of notexin-induced myoinjury. Arthritis Rheum (2010) 62:268–79. doi: 10.1002/art.27183
53. Metcalfe DD, Baram D, Mekori YA. Mast cells. Physiol Rev (1997) 77:1033–79. doi: 10.1152/physrev.1997.77.4.1033
54. Yu Y, Blokhuis BR, Garssen J, Redegeld FA. Non-IgE mediated mast cell activation. Eur J Pharmacol (2016) 778:33–43. doi: 10.1016/j.ejphar.2015.07.017
55. Parrella E, Porrini V, Benarese M, Pizzi M. The role of mast cells in stroke. Cells (2019) 8:437. doi: 10.3390/cells8050437
56. Bulanova E, Bulfone-Paus S. P2 receptor-mediated signaling in mast cell biology. Purinergic Signal (2010) 6:3–17. doi: 10.1007/s11302-009-9173-z
57. Lunderius-Andersson C, Enoksson M, Nilsson G. Mast cells respond to cell injury through the recognition of IL-33. Front Immunol (2012) 3:82. doi: 10.3389/fimmu.2012.00082
58. Hartmann K, Henz BM, Kruger-Krasagakes S, Kohl J, Burger R, Guhl S, et al. C3a and C5a stimulate chemotaxis of human mast cells. Blood (1997) 89:2863–70. doi: 10.1182/blood.V89.8.2863
59. Ali H. Regulation of human mast cell and basophil function by anaphylatoxins C3a and C5a. Immunol Lett (2010) 128:36–45. doi: 10.1016/j.imlet.2009.10.007
60. Krystel-Whittemore M, Dileepan KN, Wood JG. Mast cell: A multi-functional master cell. Front Immunol (2015) 6:620. doi: 10.3389/fimmu.2015.00620
61. Solimando AG, Desantis V, Ribatti D. Mast cells and interleukins. Int J Mol Sci (2022) 23:14004. doi: 10.3390/ijms232214004
62. Lefaucheur JP, Gjata B, Sebille A. Factors inducing mast cell accumulation in skeletal muscle. Neuropathol Appl Neurobiol (1996) 22:248–55. doi: 10.1111/j.1365-2990.1996.tb00901.x
63. Gorospe JR, Nishikawa BK, Hoffman EP. Recruitment of mast cells to muscle after mild damage. J Neurol Sci (1996) 135:10–7. doi: 10.1016/0022-510x(95)00255-z
64. Tsuchiya Y, Kitajima Y, Masumoto H, Ono Y. Damaged myofiber-derived metabolic enzymes act as activators of muscle satellite cells. Stem Cell Rep (2020) 15:926–40. doi: 10.1016/j.stemcr.2020.08.002
65. Dumont NA, Bentzinger CF, Sincennes MC, Rudnicki MA. Satellite cells and skeletal muscle regeneration. Compr Physiol (2015) 5:1027–59. doi: 10.1002/cphy.c140068
66. Al-Zaeed N, Budai Z, Szondy Z, Sarang Z. TAM kinase signaling is indispensable for proper skeletal muscle regeneration in mice. Cell Death Dis (2021) 12:611. doi: 10.1038/s41419-021-03892-5
67. Panci G, Chazaud B. Inflammation during post-injury skeletal muscle regeneration. Semin Cell Dev Biol (2021) 119:32–8. doi: 10.1016/j.semcdb.2021.05.031
68. Juban G, Chazaud B. Efferocytosis during skeletal muscle regeneration. Cells (2021) 10:3267. doi: 10.3390/cells10123267
69. Westman J, Grinstein S, Marques PE. Phagocytosis of necrotic debris at sites of injury and inflammation. Front Immunol (2019) 10:3030. doi: 10.3389/fimmu.2019.03030
70. Zitvogel L, Kepp O, Kroemer G. Decoding cell death signals in inflammation and immunity. Cell (2010) 140:798–804. doi: 10.1016/j.cell.2010.02.015
71. Arai S, Miyazaki T. A scavenging system against internal pathogens promoted by the circulating protein apoptosis inhibitor of macrophage (AIM). Semin Immunopathol (2018) 40:567–75. doi: 10.1007/s00281-018-0717-6
72. Hirayama D, Iida T, Nakase H. The phagocytic function of macrophage-enforcing innate immunity and tissue homeostasis. Int J Mol Sci (2018) 19:92. doi: 10.3390/ijms19010092
73. Zani IA, Stephen SL, Mughal NA, Russell D, Homer-Vanniasinkam S, Wheatcroft SB, et al. Scavenger receptor structure and function in health and disease. Cells (2015) 4:178–201. doi: 10.3390/cells4020178
74. Goh JW, Tan YS, Dodds AW, Reid KB, Lu J. The class a macrophage scavenger receptor type I (SR-AI) recognizes complement iC3b and mediates NF-κB activation. Protein Cell (2010) 1:174–87. doi: 10.1007/s13238-010-0020-3
75. Komai K, Shichita T, Ito M, Kanamori M, Chikuma S, Yoshimura A. Role of scavenger receptors as damage-associated molecular pattern receptors in toll-like receptor activation. Int Immunol (2017) 29:59–70. doi: 10.1093/intimm/dxx010
76. Sciorati C, Rigamonti E, Manfredi AA, Rovere-Querini P. Cell death, clearance and immunity in the skeletal muscle. Cell Death Differ (2016) 23:927–37. doi: 10.1038/cdd.2015.171
77. Arnold L, Henry A, Poron F, Baba-Amer Y, van Rooijen N, Plonquet A, et al. Inflammatory monocytes recruited after skeletal muscle injury switch into antiinflammatory macrophages to support myogenesis. J Exp Med (2007) 204:1057–69. doi: 10.1084/jem.20070075
78. Dort J, Fabre P, Molina T, Dumont NA. Macrophages are key regulators of stem cells during skeletal muscle regeneration and diseases. Stem Cells Int (2019) 2019:4761427. doi: 10.1155/2019/4761427
79. Zhang J, Qu C, Li T, Cui W, Wang X, Du J. Phagocytosis mediated by scavenger receptor class bi promotes macrophage transition during skeletal muscle regeneration. J Biol Chem (2019) 294:15672–85. doi: 10.1074/jbc.RA119.008795
80. Schilling JD. Macrophages fuel skeletal muscle regeneration. Immunometabolism (2021) 3:e210013. doi: 10.20900/immunometab20210013
81. Alvarez AM, DeOcesano-Pereira C, Teixeira C, Moreira V. IL-1β and TNF-α modulation of proliferated and committed myoblasts: IL-6 and COX-2-derived prostaglandins as key actors in the mechanisms involved. Cells (2020) 9:2005. doi: 10.3390/cells9092005
82. Gallucci S, Provenzano C, Mazzarelli P, Scuderi F, Bartoccioni E. Myoblasts produce IL-6 in response to inflammatory stimuli. Int Immunol (1998) 10:267–73. doi: 10.1093/intimm/10.3.267
83. Howard EE, Pasiakos SM, Blesso CN, Fussell MA, Rodriguez NR. Divergent roles of inflammation in skeletal muscle recovery from injury. Front Physiol (2020) 11:87. doi: 10.3389/fphys.2020.00087
84. Lu H, Huang D, Saederup N, Charo IF, Ransohoff RM, Zhou L. Macrophages recruited via CCR2 produce insulin-like growth factor-1 to repair acute skeletal muscle injury. FASEB J (2011) 25:358–69. doi: 10.1096/fj.10-171579
85. Hammers DW, Rybalko V, Merscham-Banda M, Hsieh PL, Suggs LJ, Farrar RP. Anti-inflammatory macrophages improve skeletal muscle recovery from ischemia-reperfusion. J Appl Physiol (1985) (2015) 118:1067–74. doi: 10.1152/japplphysiol.00313.2014
86. Rao RR, Long JZ, White JP, Svensson KJ, Lou J, Lokurkar I, et al. Meteorin-like is a hormone that regulates immune-adipose interactions to increase beige fat thermogenesis. Cell (2014) 157:1279–91. doi: 10.1016/j.cell.2014.03.065
87. Ushach I, Arrevillaga-Boni G, Heller GN, Pone E, Hernandez-Ruiz M, Catalan-Dibene J, et al. Meteorin-like/meteorin-β is a novel immunoregulatory cytokine associated with inflammation. J Immunol (2018) 201:3669–76. doi: 10.4049/jimmunol.1800435
88. Baht GS, Bareja A, Lee DE, Rao RR, Huang R, Huebner JL, et al. Meteorin-like facilitates skeletal muscle repair through a Stat3/IGF-1 mechanism. Nat Metab (2020) 2:278–89. doi: 10.1038/s42255-020-0184-y
89. Saclier M, Yacoub-Youssef H, Mackey AL, Arnold L, Ardjoune H, Magnan M, et al. Differentially activated macrophages orchestrate myogenic precursor cell fate during human skeletal muscle regeneration. Stem Cells (2013) 31:384–96. doi: 10.1002/stem.1288
90. Salo H, Qu H, Mitsiou D, Aucott H, Han J, Zhang XM, et al. Disulfide and fully reduced HMGB1 induce different macrophage polarization and migration patterns. Biomolecules (2021) 11:800. doi: 10.3390/biom11060800
91. Ferrara M, Chialli G, Ferreira LM, Ruggieri E, Careccia G, Preti A, et al. Oxidation of HMGB1 is a dynamically regulated process in physiological and pathological conditions. Front Immunol (2020) 11:1122. doi: 10.3389/fimmu.2020.01122
92. Lee G, Espirito Santo AI, Zwingenberger S, Cai L, Vogl T, Feldmann M, et al. Fully reduced HMGB1 accelerates the regeneration of multiple tissues by transitioning stem cells to GAlert. Proc Natl Acad Sci USA (2018) 115:E4463–E72. doi: 10.1073/pnas.1802893115
93. Tirone M, Tran NL, Ceriotti C, Gorzanelli A, Canepari M, Bottinelli R, et al. High mobility group box 1 orchestrates tissue regeneration via CXCR4. J Exp Med (2018) 215:303–18. doi: 10.1084/jem.20160217
94. Careccia G, Saclier M, Tirone M, Ruggieri E, Principi E, Raffaghello L, et al. Rebalancing expression of HMGB1 redox isoforms to counteract muscular dystrophy. Sci Transl Med (2021) 13:eaay8416. doi: 10.1126/scitranslmed.aay8416
95. De Mori R, Straino S, Di Carlo A, Mangoni A, Pompilio G, Palumbo R, et al. Multiple effects of high mobility group box protein 1 in skeletal muscle regeneration. Arterioscler Thromb Vasc Biol (2007) 27:2377–83. doi: 10.1161/ATVBAHA.107.153429
96. Paiva-Oliveira EL, Ferreira da Silva R, Correa Leite PE, Cogo JC, Quirico-Santos T, Lagrota-Candido J. TLR4 signaling protects from excessive muscular damage induced by bothrops jararacussu snake venom. Toxicon (2012) 60:1396–403. doi: 10.1016/j.toxicon.2012.10.003
97. Riuzzi F, Sorci G, Sagheddu R, Donato R. HMGB1-RAGE regulates muscle satellite cell homeostasis through p38-MAPK- and myogenin-dependent repression of Pax7 transcription. J Cell Sci (2012) 125:1440–54. doi: 10.1242/jcs.092163
98. Paiva-Oliveira EL, da Silva RF, Bellio M, Quirico-Santos T, Lagrota-Candido J. Pattern of cardiotoxin-induced muscle remodeling in distinct TLR-4 deficient mouse strains. Histochem Cell Biol (2017) 148:49–60. doi: 10.1007/s00418-017-1556-6
99. Hansen L, Joseph G, Valdivia A, Taylor WR. Satellite cell expression of RAGE (receptor for advanced glycation end products) is important for collateral vessel formation. J Am Heart Assoc (2021) 10:e022127. doi: 10.1161/JAHA.120.022127
100. Bot I, Velden DV, Bouwman M, Kroner MJ, Kuiper J, Quax PHA, et al. Local mast cell activation promotes neovascularization. Cells (2020) 9:701. doi: 10.3390/cells9030701
101. Hong H, Tian XY. The role of macrophages in vascular repair and regeneration after ischemic injury. Int J Mol Sci (2020) 21:6328. doi: 10.3390/ijms21176328
102. Zordan P, Rigamonti E, Freudenberg K, Conti V, Azzoni E, Rovere-Querini P, et al. Macrophages commit postnatal endothelium-derived progenitors to angiogenesis and restrict endothelial to mesenchymal transition during muscle regeneration. Cell Death Dis (2014) 5:e1031. doi: 10.1038/cddis.2013.558
103. Ramakrishnan S, Anand V, Roy S. Vascular endothelial growth factor signaling in hypoxia and inflammation. J Neuroimmun Pharmacol (2014) 9:142–60. doi: 10.1007/s11481-014-9531-7
104. Olsson AK, Dimberg A, Kreuger J, Claesson-Welsh L. VEGF receptor signalling - in control of vascular function. Nat Rev Mol Cell Biol (2006) 7:359–71. doi: 10.1038/nrm1911
105. Ochoa O, Sun D, Reyes-Reyna SM, Waite LL, Michalek JE, McManus LM, et al. Delayed angiogenesis and VEGF production in CCR2-/- mice during impaired skeletal muscle regeneration. Am J Physiol Regul Integr Comp Physiol (2007) 293:R651–61. doi: 10.1152/ajpregu.00069.2007
106. Lu CY, Santosa KB, Jablonka-Shariff A, Vannucci B, Fuchs A, Turnbull I, et al. Macrophage-derived vascular endothelial growth factor-a is integral to neuromuscular junction reinnervation after nerve injury. J Neurosci (2020) 40:9602–16. doi: 10.1523/JNEUROSCI.1736-20.2020
107. Rowe GC, Raghuram S, Jang C, Nagy JA, Patten IS, Goyal A, et al. PGC-1α induces SPP1 to activate macrophages and orchestrate functional angiogenesis in skeletal muscle. Circ Res (2014) 115:504–17. doi: 10.1161/CIRCRESAHA.115.303829
108. Latroche C, Weiss-Gayet M, Muller L, Gitiaux C, Leblanc P, Liot S, et al. Coupling between myogenesis and angiogenesis during skeletal muscle regeneration is stimulated by restorative macrophages. Stem Cell Rep (2017) 9:2018–33. doi: 10.1016/j.stemcr.2017.10.027
109. Chillo O, Kleinert EC, Lautz T, Lasch M, Pagel JI, Heun Y, et al. Perivascular mast cells govern shear stress-induced arteriogenesis by orchestrating leukocyte function. Cell Rep (2016) 16:2197–207. doi: 10.1016/j.celrep.2016.07.040
110. Shi GP, Bot I, Kovanen PT. Mast cells in human and experimental cardiometabolic diseases. Nat Rev Cardiol (2015) 12:643–58. doi: 10.1038/nrcardio.2015.117
111. Lutz J, Thurmel K, Heemann U. Anti-inflammatory treatment strategies for ischemia/reperfusion injury in transplantation. J Inflammation (Lond) (2010) 7:27. doi: 10.1186/1476-9255-7-27
112. Duchesne E, Dufresne SS, Dumont NA. Impact of inflammation and anti-inflammatory modalities on skeletal muscle healing: From fundamental research to the clinic. Phys Ther (2017) 97:807–17. doi: 10.1093/ptj/pzx056
113. Breitbart GB, Dillon PK, Suval WD, Padberg FT Jr., FitzPatrick M, Duran WN. Dexamethasone attenuates microvascular ischemia-reperfusion injury in the rat cremaster muscle. Microvasc Res (1989) 38:155–63. doi: 10.1016/0026-2862(89)90024-1
114. Chen LE, Silver WP, Seaber AV, Korompilias AV, Urbaniak JR. Effects of dexamethasone on the contractile function of reperfused skeletal muscle. Microsurgery (1996) 17:313–20. doi: 10.1002/(SICI)1098-2752(1996)17:6<313::AID-MICR5>3.0.CO;2-I
115. Corrick RM, Tu H, Zhang D, Barksdale AN, Muelleman RL, Wadman MC, et al. Dexamethasone protects against tourniquet-induced acute ischemia-reperfusion injury in mouse hindlimb. Front Physiol (2018) 9:244. doi: 10.3389/fphys.2018.00244
116. Tu H, Zhang D, Wadman MC, Li YL. Dexamethasone ameliorates recovery process of neuromuscular junctions after tourniquet-induced ischemia-reperfusion injuries in mouse hindlimb. Eur J Pharmacol (2020) 883:173364. doi: 10.1016/j.ejphar.2020.173364
117. Zhang D, Wang D, Pipinos II, Muelleman RL, Li YL. Dexamethasone promotes long-term functional recovery of neuromuscular junction in a murine model of tourniquet-induced ischaemia-reperfusion. Acta Physiol (Oxf) (2017) 219:453–64. doi: 10.1111/apha.12737
118. Queme LF, Ross JL, Jankowski MP. Peripheral mechanisms of ischemic myalgia. Front Cell Neurosci (2017) 11:419. doi: 10.3389/fncel.2017.00419
119. Mittal M, Siddiqui MR, Tran K, Reddy SP, Malik AB. Reactive oxygen species in inflammation and tissue injury. Antioxid Redox Signal (2014) 20:1126–67. doi: 10.1089/ars.2012.5149
120. Nguyen HX, Tidball JG. Interactions between neutrophils and macrophages promote macrophage killing of rat muscle cells in vitro. J Physiol (2003) 547:125–32. doi: 10.1113/jphysiol.2002.031450
121. Canton M, Sanchez-Rodriguez R, Spera I, Venegas FC, Favia M, Viola A, et al. Reactive oxygen species in macrophages: Sources and targets. Front Immunol (2021) 12:734229. doi: 10.3389/fimmu.2021.734229
122. Wynn TA, Vannella KM. Macrophages in tissue repair, regeneration, and fibrosis. Immunity (2016) 44:450–62. doi: 10.1016/j.immuni.2016.02.015
123. Baracos V, Rodemann HP, Dinarello CA, Goldberg AL. Stimulation of muscle protein degradation and prostaglandin E2 release by leukocytic pyrogen (interleukin-1). a mechanism for the increased degradation of muscle proteins during fever. N Engl J Med (1983) 308:553–8. doi: 10.1056/NEJM198303103081002
124. Clowes GH Jr., George BC, Villee CA Jr., Saravis CA. Muscle proteolysis induced by a circulating peptide in patients with sepsis or trauma. N Engl J Med (1983) 308:545–52. doi: 10.1056/NEJM198303103081001
125. Shang M, Cappellesso F, Amorim R, Serneels J, Virga F, Eelen G, et al. Macrophage-derived glutamine boosts satellite cells and muscle regeneration. Nature (2020) 587:626–31. doi: 10.1038/s41586-020-2857-9
126. Tidball JG. Inflammatory processes in muscle injury and repair. Am J Physiol Regul Integr Comp Physiol (2005) 288:R345–53. doi: 10.1152/ajpregu.00454.2004
127. Nguyen HX, Lusis AJ, Tidball JG. Null mutation of myeloperoxidase in mice prevents mechanical activation of neutrophil lysis of muscle cell membranes in vitro and in vivo. J Physiol (2005) 565:403–13. doi: 10.1113/jphysiol.2005.085506
128. Dumont N, Bouchard P, Frenette J. Neutrophil-induced skeletal muscle damage: A calculated and controlled response following hindlimb unloading and reloading. Am J Physiol Regul Integr Comp Physiol (2008) 295:R1831–8. doi: 10.1152/ajpregu.90318.2008
129. Nguyen HX, Tidball JG. Null mutation of gp91phox reduces muscle membrane lysis during muscle inflammation in mice. J Physiol (2003) 553:833–41. doi: 10.1113/jphysiol.2003.051912
130. Judge AR, Dodd SL. Oxidative damage to skeletal muscle following an acute bout of contractile claudication. Atherosclerosis (2003) 171:219–24. doi: 10.1016/j.atherosclerosis.2003.08.022
131. Smith JK, Grisham MB, Granger DN, Korthuis RJ. Free radical defense mechanisms and neutrophil infiltration in postischemic skeletal muscle. Am J Physiol (1989) 256:H789–93. doi: 10.1152/ajpheart.1989.256.3.H789
132. Ghaly A, Marsh DR. Ischaemia-reperfusion modulates inflammation and fibrosis of skeletal muscle after contusion injury. Int J Exp Pathol (2010) 91:244–55.E. doi: 10.1111/j.1365-2613.2010.00708.x
133. Judge AR, Dodd SL. Xanthine oxidase and activated neutrophils cause oxidative damage to skeletal muscle after contractile claudication. Am J Physiol Heart Circ Physiol (2004) 286:H252–6. doi: 10.1152/ajpheart.00684.2003
134. Walden DL, McCutchan HJ, Enquist EG, Schwappach JR, Shanley PF, Reiss OK, et al. Neutrophils accumulate and contribute to skeletal muscle dysfunction after ischemia-reperfusion. Am J Physiol (1990) 259:H1809–12. doi: 10.1152/ajpheart.1990.259.6.H1809
135. Pejler G, Abrink M, Ringvall M, Wernersson S. Mast cell proteases. Adv Immunol (2007) 95:167–255. doi: 10.1016/S0065-2776(07)95006-3
136. Pejler G, Ronnberg E, Waern I, Wernersson S. Mast cell proteases: Multifaceted regulators of inflammatory disease. Blood (2010) 115:4981–90. doi: 10.1182/blood-2010-01-257287
137. Yang MQ, Ma YY, Ding J, Li JY. The role of mast cells in ischemia and reperfusion injury. Inflammation Res (2014) 63:899–905. doi: 10.1007/s00011-014-0763-z
138. Lazarus B, Messina A, Barker JE, Hurley JV, Romeo R, Morrison WA, et al. The role of mast cells in ischaemia-reperfusion injury in murine skeletal muscle. J Pathol (2000) 191:443–8. doi: 10.1002/1096-9896(2000)9999:9999<::AID-PATH666>3.0.CO;2-L
139. Mukundan C, Gurish MF, Austen KF, Hechtman HB, Friend DS. Mast cell mediation of muscle and pulmonary injury following hindlimb ischemia-reperfusion. J Histochem Cytochem (2001) 49:1055–6. doi: 10.1177/002215540104900813
140. Bortolotto SK, Morrison WA, Han X, Messina A. Mast cells play a pivotal role in ischaemia reperfusion injury to skeletal muscles. Lab Invest (2004) 84:1103–11. doi: 10.1038/labinvest.3700126
141. Abonia JP, Friend DS, Austen WG Jr., Moore FD Jr., Carroll MC, Chan R, et al. Mast cell protease 5 mediates ischemia-reperfusion injury of mouse skeletal muscle. J Immunol (2005) 174:7285–91. doi: 10.4049/jimmunol.174.11.7285
142. Beisel WR. Mediators of fever and muscle proteolysis. N Engl J Med (1983) 308:586–7. doi: 10.1056/NEJM198303103081009
143. Deger SM, Hung AM, Gamboa JL, Siew ED, Ellis CD, Booker C, et al. Systemic inflammation is associated with exaggerated skeletal muscle protein catabolism in maintenance hemodialysis patients. JCI Insight (2017) 2:e95185. doi: 10.1172/jci.insight.95185
144. Kaizu Y, Ohkawa S, Odamaki M, Ikegaya N, Hibi I, Miyaji K, et al. Association between inflammatory mediators and muscle mass in long-term hemodialysis patients. Am J Kidney Dis (2003) 42:295–302. doi: 10.1016/s0272-6386(03)00654-1
145. Morrison TE, Fraser RJ, Smith PN, Mahalingam S, Heise MT. Complement contributes to inflammatory tissue destruction in a mouse model of ross river virus-induced disease. J Virol (2007) 81:5132–43. doi: 10.1128/JVI.02799-06
146. Huebener P, Pradere JP, Hernandez C, Gwak GY, Caviglia JM, Mu X, et al. The HMGB1/RAGE axis triggers neutrophil-mediated injury amplification following necrosis. J Clin Invest (2015) 125:539–50. doi: 10.1172/JCI76887
147. Ozment TR, Ha T, Breuel KF, Ford TR, Ferguson DA, Kalbfleisch J, et al. Scavenger receptor class a plays a central role in mediating mortality and the development of the pro-inflammatory phenotype in polymicrobial sepsis. PloS Pathog (2012) 8:e1002967. doi: 10.1371/journal.ppat.1002967
148. Drummond R, Cauvi DM, Hawisher D, Song D, Nino DF, Coimbra R, et al. Deletion of scavenger receptor a gene in mice resulted in protection from septic shock and modulation of TLR4 signaling in isolated peritoneal macrophages. Innate Immun (2013) 19:30–41. doi: 10.1177/1753425912449548
149. de Winther MP, van Dijk KW, Havekes LM, Hofker MH. Macrophage scavenger receptor class a: A multifunctional receptor in atherosclerosis. Arterioscler Thromb Vasc Biol (2000) 20:290–7. doi: 10.1161/01.atv.20.2.290
150. Reid MB, Li YP. Tumor necrosis factor-alpha and muscle wasting: A cellular perspective. Respir Res (2001) 2:269–72. doi: 10.1186/rr67
151. Bhatnagar S, Panguluri SK, Gupta SK, Dahiya S, Lundy RF, Kumar A. Tumor necrosis factor-α regulates distinct molecular pathways and gene networks in cultured skeletal muscle cells. PloS One (2010) 5:e13262. doi: 10.1371/journal.pone.0013262
152. Li W, Moylan JS, Chambers MA, Smith J, Reid MB. Interleukin-1 stimulates catabolism in C2C12 myotubes. Am J Physiol Cell Physiol (2009) 297:C706–14. doi: 10.1152/ajpcell.00626.2008
153. Stratos I, Behrendt AK, Anselm C, Gonzalez A, Mittlmeier T, Vollmar B. Inhibition of TNF-α restores muscle force, inhibits inflammation, and reduces apoptosis of traumatized skeletal muscles. Cells (2022) 11:2397. doi: 10.3390/cells11152397
154. Chan RK, Ibrahim SI, Verna N, Carroll M, Moore FD Jr., Hechtman HB. Ischaemia-reperfusion is an event triggered by immune complexes and complement. Br J Surg (2003) 90:1470–8. doi: 10.1002/bjs.4408
156. Weiser MR, Williams JP, Moore FD Jr., Kobzik L, Ma M, Hechtman HB, et al. Reperfusion injury of ischemic skeletal muscle is mediated by natural antibody and complement. J Exp Med (1996) 183:2343–8. doi: 10.1084/jem.183.5.2343
157. Lindsay TF, Hill J, Ortiz F, Rudolph A, Valeri CR, Hechtman HB, et al. Blockade of complement activation prevents local and pulmonary albumin leak after lower torso ischemia-reperfusion. Ann Surg (1992) 216:677–83. doi: 10.1097/00000658-199212000-00010
158. Kyriakides C, Austen W Jr., Wang Y, Favuzza J, Kobzik L, Moore FD Jr., et al. Skeletal muscle reperfusion injury is mediated by neutrophils and the complement membrane attack complex. Am J Physiol (1999) 277:C1263–8. doi: 10.1152/ajpcell.1999.277.6.C1263
159. Chan RK, Ibrahim SI, Takahashi K, Kwon E, McCormack M, Ezekowitz A, et al. The differing roles of the classical and mannose-binding lectin complement pathways in the events following skeletal muscle ischemia-reperfusion. J Immunol (2006) 177:8080–5. doi: 10.4049/jimmunol.177.11.8080
160. Ulloa L, Messmer D. High-mobility group box 1 (HMGB1) protein: Friend and foe. Cytokine Growth Factor Rev (2006) 17:189–201. doi: 10.1016/j.cytogfr.2006.01.003
161. Tang D, Shi Y, Kang R, Li T, Xiao W, Wang H, et al. Hydrogen peroxide stimulates macrophages and monocytes to actively release HMGB1. J Leukoc Biol (2007) 81:741–7. doi: 10.1189/jlb.0806540
162. Palmai-Pallag T, Bachrati CZ. Inflammation-induced DNA damage and damage-induced inflammation: A vicious cycle. Microbes Infect (2014) 16:822–32. doi: 10.1016/j.micinf.2014.10.001
163. Bellussi LM, Cocca S, Chen L, Passali FM, Sarafoleanu C, Passali D. Rhinosinusal inflammation and high mobility group box 1 protein: A new target for therapy. ORL J Otorhinolaryngol Relat Spec (2016) 78:77–85. doi: 10.1159/000443481
164. Wittemann B, Neuer G, Michels H, Truckenbrodt H, Bautz FA. Autoantibodies to nonhistone chromosomal proteins HMG-1 and HMG-2 in sera of patients with juvenile rheumatoid arthritis. Arthritis Rheum (1990) 33:1378–83. doi: 10.1002/art.1780330910
165. Liu Y, Zhuang GB, Zhou XZ. HMBG1 as a driver of inflammatory and immune processes in the pathogenesis of ocular diseases. J Ophthalmol (2018) 2018:5195290. doi: 10.1155/2018/5195290
166. Londhe P, Guttridge DC. Inflammation induced loss of skeletal muscle. Bone (2015) 80:131–42. doi: 10.1016/j.bone.2015.03.015
167. Noris M, Remuzzi G. Overview of complement activation and regulation. Semin Nephrol (2013) 33:479–92. doi: 10.1016/j.semnephrol.2013.08.001
168. Camous L, Roumenina L, Bigot S, Brachemi S, Fremeaux-Bacchi V, Lesavre P, et al. Complement alternative pathway acts as a positive feedback amplification of neutrophil activation. Blood (2011) 117:1340–9. doi: 10.1182/blood-2010-05-283564
169. Pickering MC, Cook HT, Warren J, Bygrave AE, Moss J, Walport MJ, et al. Uncontrolled C3 activation causes membranoproliferative glomerulonephritis in mice deficient in complement factor h. Nat Genet (2002) 31:424–8. doi: 10.1038/ng912
170. Radley HG, Grounds MD. Cromolyn administration (to block mast cell degranulation) reduces necrosis of dystrophic muscle in mdx mice. Neurobiol Dis (2006) 23:387–97. doi: 10.1016/j.nbd.2006.03.016
171. Gordon JR, Galli SJ. Mast cells as a source of both preformed and immunologically inducible TNF-alpha/cachectin. Nature (1990) 346:274–6. doi: 10.1038/346274a0
172. Fielding RA, Manfredi TJ, Ding W, Fiatarone MA, Evans WJ, Cannon JG. Acute phase response in exercise. III. neutrophil and IL-1 beta accumulation in skeletal muscle. Am J Physiol (1993) 265:R166–72. doi: 10.1152/ajpregu.1993.265.1.R166
173. Lammermann T, Afonso PV, Angermann BR, Wang JM, Kastenmuller W, Parent CA, et al. Neutrophil swarms require LTB4 and integrins at sites of cell death. vivo Nat (2013) 498:371–5. doi: 10.1038/nature12175
174. Vanholder R, Sever MS, Erek E, Lameire N. Rhabdomyolysis. J Am Soc Nephrol (2000) 11:1553–61. doi: 10.1681/ASN.V1181553
175. Mabvuure NT, Malahias M, Hindocha S, Khan W, Juma A. Acute compartment syndrome of the limbs: Current concepts and management. Open Orthop J (2012) 6:535–43. doi: 10.2174/1874325001206010535
176. Gourgiotis S, Villias C, Germanos S, Foukas A, Ridolfini MP. Acute limb compartment syndrome: A review. J Surg Educ (2007) 64:178–86. doi: 10.1016/j.jsurg.2007.03.006
177. Via AG, Oliva F, Spoliti M, Maffulli N. Acute compartment syndrome. Muscles Ligaments Tendons J (2015) 5:18–22.
178. Ajuebor MN, Das AM, Virag L, Flower RJ, Szabo C, Perretti M. Role of resident peritoneal macrophages and mast cells in chemokine production and neutrophil migration in acute inflammation: Evidence for an inhibitory loop involving endogenous IL-10. J Immunol (1999) 162:1685–91. doi: 10.4049/jimmunol.162.3.1685
179. Lovering RM, De Deyne PG. Contractile function, sarcolemma integrity, and the loss of dystrophin after skeletal muscle eccentric contraction-induced injury. Am J Physiol Cell Physiol (2004) 286:C230–8. doi: 10.1152/ajpcell.00199.2003
180. Duan D, Goemans N, Takeda S, Mercuri E, Aartsma-Rus A. Duchenne muscular dystrophy. Nat Rev Dis Primers (2021) 7:13. doi: 10.1038/s41572-021-00248-3
181. Hamel Y, Mamoune A, Mauvais FX, Habarou F, Lallement L, Romero NB, et al. Acute rhabdomyolysis and inflammation. J Inherit Metab Dis (2015) 38:621–8. doi: 10.1007/s10545-015-9827-7
182. Bosch X, Poch E, Grau JM. Rhabdomyolysis and acute kidney injury. N Engl J Med (2009) 361:62–72. doi: 10.1056/NEJMra0801327
183. Torres PA, Helmstetter JA, Kaye AM, Kaye AD. Rhabdomyolysis: pathogenesis, diagnosis, and treatment. Ochsner J (2015) 15:58–69.
184. Lima RS, da Silva Junior GB, Liborio AB, Daher EDF. Acute kidney injury due to rhabdomyolysis. Saudi J Kidney Dis Transpl (2008) 19:721–9.
185. Petejova N, Martinek A. Acute kidney injury due to rhabdomyolysis and renal replacement therapy: A critical review. Crit Care (2014) 18:224. doi: 10.1186/cc13897
186. Cassim F, Soni AJ, Murphy S. Severe acute inflammatory myositis and rhabdomyolysis in paediatric SARS-CoV-2-associated MIS-c (multisystem inflammatory syndrome in children). BMJ Case Rep (2021) 14:e243112. doi: 10.1136/bcr-2021-243112
187. Shah SV, Reddy K. Rhabdomyolysis with acute renal failure triggered by the seasonal flu vaccination in a patient taking simvastatin. BMJ Case Rep (2010) 2010:bcr1120092485. doi: 10.1136/bcr.11.2009.2485
188. Acharya S, Shukla S, Mahajan SN, Diwan SK. Acute dengue myositis with rhabdomyolysis and acute renal failure. Ann Indian Acad Neurol (2010) 13:221–2. doi: 10.4103/0972-2327.70882
189. Chatzizisis YS, Misirli G, Hatzitolios AI, Giannoglou GD. The syndrome of rhabdomyolysis: Complications and treatment. Eur J Intern Med (2008) 19:568–74. doi: 10.1016/j.ejim.2007.06.037
190. Rosenberry C, Stone F, Kalbfleisch K. Rhabdomyolysis-induced severe hyperkalemia. West J Emerg Med (2009) 10:302.
191. Park SE, Kim DY, Park ES. Hyperkalemia in a patient with rhabdomyolysis and compartment syndrome -a case report-. Korean J Anesthesiol (2010) 59(Suppl):S37–40. doi: 10.4097/kjae.2010.59.S.S37
192. Hauser CJ, Otterbein LE. Danger signals from mitochondrial damps in trauma and post-injury sepsis. Eur J Trauma Emerg Surg (2018) 44:317–24. doi: 10.1007/s00068-018-0963-2
193. Mas-Celis F, Olea-Lopez J, Parroquin-Maldonado JA. Sepsis in trauma: A deadly complication. Arch Med Res (2021) 52:808–16. doi: 10.1016/j.arcmed.2021.10.007
194. Nedeva C, Menassa J, Puthalakath H. Sepsis: Inflammation is a necessary evil. Front Cell Dev Biol (2019) 7:108. doi: 10.3389/fcell.2019.00108
195. Denning NL, Aziz M, Gurien SD, Wang P. DAMPs and NETs in sepsis. Front Immunol (2019) 10:2536. doi: 10.3389/fimmu.2019.02536
196. Kumar V. Toll-like receptors in sepsis-associated cytokine storm and their endogenous negative regulators as future immunomodulatory targets. Int Immunopharmacol (2020) 89:107087. doi: 10.1016/j.intimp.2020.107087
197. Goris RJ, te Boekhorst TP, Nuytinck JK, Gimbrere JS. Multiple-organ failure. generalized autodestructive inflammation? Arch Surg (1985) 120:1109–15. doi: 10.1001/archsurg.1985.01390340007001
198. Zhang Q, Raoof M, Chen Y, Sumi Y, Sursal T, Junger W, et al. Circulating mitochondrial damps cause inflammatory responses to injury. Nature (2010) 464:104–7. doi: 10.1038/nature08780
199. Moldawer LL. Biology of proinflammatory cytokines and their antagonists. Crit Care Med (1994) 22:S3–7.
200. Tracey KJ, Beutler B, Lowry SF, Merryweather J, Wolpe S, Milsark IW, et al. Shock and tissue injury induced by recombinant human cachectin. Science (1986) 234:470–4. doi: 10.1126/science.3764421
201. Morrison DC, Ryan JL. Endotoxins and disease mechanisms. Annu Rev Med (1987) 38:417–32. doi: 10.1146/annurev.me.38.020187.002221
202. Tracey KJ, Fong Y, Hesse DG, Manogue KR, Lee AT, Kuo GC, et al. Anti-cachectin/TNF monoclonal antibodies prevent septic shock during lethal bacteraemia. Nature (1987) 330:662–4. doi: 10.1038/330662a0
203. Tracey KJ, Lowry SF, Fahey TJ 3rd, Albert JD, Fong Y, Hesse D, et al. Cachectin/tumor necrosis factor induces lethal shock and stress hormone responses in the dog. Surg Gynecol Obstet (1987) 164:415–22.
204. Tracey KJ, Lowry SF, Cerami A. Cachectin: A hormone that triggers acute shock and chronic cachexia. J Infect Dis (1988) 157:413–20. doi: 10.1093/infdis/157.3.413
205. Kyriakides C, Austen WG Jr., Wang Y, Favuzza J, Moore FD Jr., Hechtman HB. Neutrophil mediated remote organ injury after lower torso ischemia and reperfusion is selectin and complement dependent. J Trauma (2000) 48:32–8. doi: 10.1097/00005373-200001000-00006
206. Esmon CT. The interactions between inflammation and coagulation. Br J Haematol (2005) 131:417–30. doi: 10.1111/j.1365-2141.2005.05753.x
207. Ho TL, Tang CH, Chang SL, Tsai CH, Chen HT, Su CM. HMGB1 promotes in vitro and in vivo skeletal muscle atrophy through an IL-18-dependent mechanism. Cells (2022) 11:3936. doi: 10.3390/cells11233936
208. Brochner AC, Toft P. Pathophysiology of the systemic inflammatory response after major accidental trauma. Scand J Trauma Resusc Emerg Med (2009) 17:43. doi: 10.1186/1757-7241-17-43
209. Hart CA, Tsui J, Khan K, Ho TK, Shiwen X, Ponticos M, et al. Critical limb ischaemia in adult human skeletal muscle increases satellite cell proliferation but not differentiation. Surg Sci (2015) 6:198–207. doi: 10.4236/ss.2015.65031
210. Blaisdell FW. The pathophysiology of skeletal muscle ischemia and the reperfusion syndrome: A review. Cardiovasc Surg (2002) 10:620–30. doi: 10.1016/s0967-2109(02)00070-4
211. Pang J, Maienschein-Cline M, Koh TJ. Enhanced proliferation of Ly6c(+) monocytes/macrophages contributes to chronic inflammation in skin wounds of diabetic mice. J Immunol (2021) 206:621–30. doi: 10.4049/jimmunol.2000935
212. De Micheli AJ, Spector JA, Elemento O, Cosgrove BD. A reference single-cell transcriptomic atlas of human skeletal muscle tissue reveals bifurcated muscle stem cell populations. Skelet Muscle (2020) 10:19. doi: 10.1186/s13395-020-00236-3
213. Rubenstein AB, Smith GR, Raue U, Begue G, Minchev K, Ruf-Zamojski F, et al. Single-cell transcriptional profiles in human skeletal muscle. Sci Rep (2020) 10(1):229. doi: 10.1038/s41598-019-57110-6
Keywords: complements, damage-associated molecular patterns (DAMP), immune cell, inflammation, sepsis, skeletal muscle
Citation: Tu H and Li Y-L (2023) Inflammation balance in skeletal muscle damage and repair. Front. Immunol. 14:1133355. doi: 10.3389/fimmu.2023.1133355
Received: 28 December 2022; Accepted: 12 January 2023;
Published: 26 January 2023.
Edited by:
Guo-Chang Fan, University of Cincinnati, United StatesReviewed by:
Jingbo Pang, University of Illinois at Chicago, United StatesYifan Li, University of South Dakota, United States
Copyright © 2023 Tu and Li. This is an open-access article distributed under the terms of the Creative Commons Attribution License (CC BY). The use, distribution or reproduction in other forums is permitted, provided the original author(s) and the copyright owner(s) are credited and that the original publication in this journal is cited, in accordance with accepted academic practice. No use, distribution or reproduction is permitted which does not comply with these terms.
*Correspondence: Yu-Long Li, eXVsb25nbGlAdW5tYy5lZHU=