- 1Epidemiology Doctorate, Facultad Nacional de Salud Pública, Universidad de Antioquia, Medellín, Colombia
- 2Department of Medical Microbiology & Infectious Diseases, University of Manitoba, Winnipeg, MB, Canada
- 3Departments of Internal Medicine and Community Health Sciences, University of Manitoba, Winnipeg, MB, Canada
- 4Facultad de Medicina, Universidad Pontificia Bolivariana, Medellín, Colombia
- 5Grupo de Investigación en Salud Pública, Universidad Pontificia Bolivariana, Medellín, Colombia
- 6Grupo Investigador de Problemas en Enfermedades Infecciosas (GRIPE), Facultad de Medicina, Universidad de Antioquia, Medellín, Colombia
- 7JC Wilt Infectious Diseases Research Centre, Public Health Agency of Canada, Winnipeg, MB, Canada
Introduction: The risk of progression to tuberculosis disease is highest within the first year after M. tuberculosis infection (TBI). We hypothesize that people with newly acquired TBI have a unique cytokine/chemokine profile that could be used as a potential biomarker.
Methods: We evaluated socio-demographic variables and 18 cytokines/chemokines in plasma samples from a cohort of people deprived of liberty (PDL) in two Colombian prisons: 47 people diagnosed with pulmonary TB, 24 with new TBI, and 47 non-infected individuals. We performed a multinomial regression to identify the immune parameters that differentiate the groups.
Results: The concentration of immune parameters changed over time and was affected by the time of incarceration. The concentration of sCD14, IL-18 and IP-10 differed between individuals with new TBI and short and long times of incarceration. Among people with short incarceration, high concentrations of MIP-3α were associated with a higher risk of a new TBI, and higher concentrations of Eotaxin were associated with a lower risk of a new TBI. Higher concentrations of sCD14 and TNF-α were associated with a higher risk of TB disease, and higher concentrations of IL-18 and MCP-1 were associated with a lower risk of TB disease.
Conclusions: There were cytokines/chemokines associated with new TBI and TB disease. However, the concentration of immune mediators varies by the time of incarceration among people with new TBI. Further studies should evaluate the changes of these and other cytokines/chemokines over time to understand the immune mechanisms across the spectrum of TB.
1 Introduction
To reduce the burden of Tuberculosis (TB) worldwide, two key components will be necessary: 1) prevention of new TB infection (TBI) and 2) prevention of the progression from TBI to TB disease (1). The global prevalence of TBI is 24.8% (95% CI: 19.7-30.0%) and 21.2% (95% CI: 17.9-24.4%) based on the results of the interferon-gamma release assays (IGRAs) and tuberculin skin test (TST), respectively (2). Prevalence among people who are at higher risk for TBI, including people deprived of liberty (PDL), is generally higher than the community at large in the same geographic location and can be as high as 88.8% (3).
Control of the progression to TB disease relies on accurate TBI diagnosis. However, this is one of the significant challenges in the path toward TB elimination. The main limitations of current tests (TST and IGRAs) are their inability to distinguish between TBI and TB disease or to predict TB progression to active disease (4–8). Despite numerous articles reporting immune markers associated with TBI and TB disease, there is high heterogeneity in the design, participant selection, sample processing (including the stimulation protocols), measured markers and analysis that limits comparing study results (9).
TB disease occurs most frequently among people newly infected with TB, mainly within the first year of acquiring mycobacterial infection (10). Identifying and prioritizing those with new TBI within the first year of mycobacterial infection to offer TBI treatment could be an effective measure to decrease TB transmission.
This study focused on incarcerated population because of the high risk of exposure to TB and progress to TB disease (11). We analyzed data and samples collected from two prisons where we have previously shown high rates of TB infection and disease (12, 13). This study aimed to determine the plasma concentration of 18 cytokines/chemokines associated with the presence of new Mycobacterium tuberculosis (MTB) infection and compare it with the concentration among people with pulmonary TB diagnosis and exposed but uninfected people.
2 Methods
2.1 Ethics statement
Approval for the study was obtained from the Ethics Committees of the Universidad Pontificia Bolivariana and the University of Manitoba. The Instituto Nacional Penitenciario y Carcelario (INPEC) and the director of each prison approved the project. In all cases, written consent forms were explained and signed in the presence of two witnesses (always people deprived of liberty -PDL).
2.2 Study design, settings, and population
This cohort study was conducted in Colombia between September 2016 and December 2018 in two medium and high-security men’s prisons. According to the inclusion criteria, the cohort included 124 PDL with a negative two-step TST at enrolment (Supplementary Material 1). Complete information related to the protocol, procedures, eligibility, recruitment, follow-up and epidemiological data for this cohort study has been published elsewhere (13).
During the cohort study, the TBI incidence rates varied between 2,402.88 cases per 100,000 person-months (95% CI 1,364.62 - 4,231.10) in PDL with short time of incarceration to 419.66 issues per 100,000 person-months (95% CI 225.80 - 779.95) in individuals with a long time of incarceration (13). For this reason, the cohort was divided into two subgroups: 64 “PDL with short incarceration” for those who were enrolled in follow-up upon incarceration or within the first three months of incarceration, and 60 “PDL with long incarceration” for people who started their follow-up after one year or more of imprisonment.
The primary outcome in the cohort was a new TBI (documented TST conversion). In the end, there were 25 new TBIs among 124 people with a negative two-step TST at enrolment. Thirteen out of 25 were people with short incarceration, and 12/25 new TBIs were people with long incarceration. Ninety-nine individuals remained TST negative after the follow-up. Supplementary Material 1 has information about the new TBI diagnosis criteria.
In addition, we included 51 of 88 (57.95%) people with a new pulmonary TB diagnosis during the study period. The prison healthcare system performed the microbiological diagnoses, and we also collected sputum samples to confirm Mycobacterium tuberculosis. People were invited to participate in the study after the TB diagnosis. Supplementary Material 1 describes the eligibility criteria and the TB diagnosis.
2.3 Procedures
2.3.1 Data collection
The socio-demographic data were collected from all individuals at baseline: age; history and time of prior incarceration; use of drugs (inhaled, injected, or smoked), smoking, and alcohol consumption; comorbidities (chronic obstructive pulmonary disease, diabetes, chronic kidney disease, HIV, and any other immunosuppressive condition); contact with a person diagnosed with TB disease (outside and inside the prison); history of prior TB, including date of the last episode, and outcome; weight and height. To determine previous exposure to the BCG vaccine, the field team sought the presence of the BCG scar.
2.3.2 Blood sample collection
All PDL included in the study provided blood samples at baseline and every three months during their follow-up. The samples were collected in sodium heparin tubes, and plasma was separated and stored at -80°C until processing.
We processed 148 samples as follows: a) 58 samples of PDL with a new TBI (21 samples at baseline, 18 at pre-conversion [3 months before TST conversion], and 19 at the time of TST conversion); b) 43 samples of PDL of the last follow–up available among people that remain TST negative and had the longest follow-ups; c) and 47 samples at baseline of people diagnosed with TB disease (Figure 1). We excluded 4/51 participants diagnosed with TB because 3 had HIV co-infection, and one did not have a plasma sample at baseline.
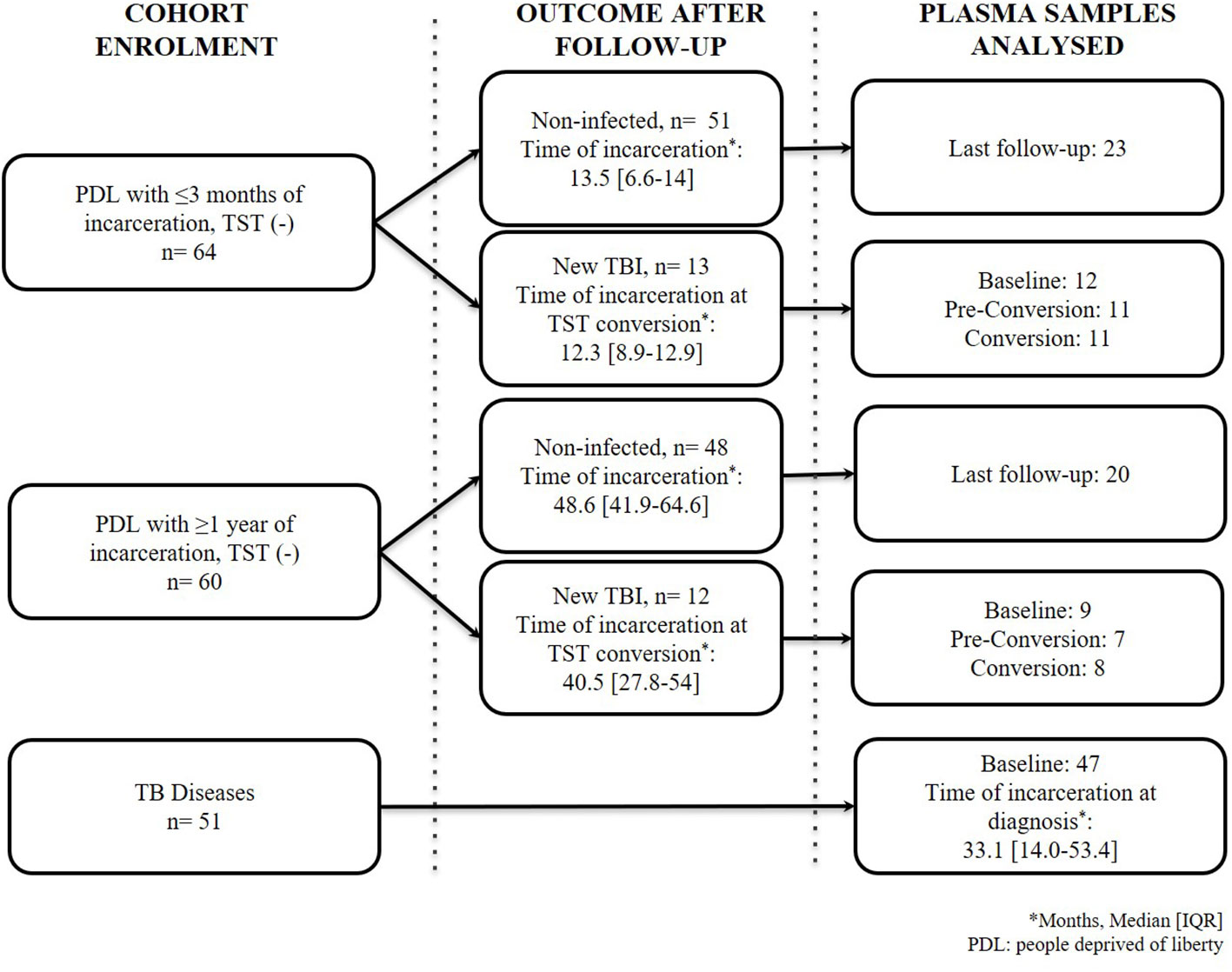
Figure 1 Flow chart of people included in the study. TB: tuberculosis; TST: tuberculin skin test. New TBI [people with negative two-step TST that became positive during follow-up were divided into TBI with short incarceration (they had ≤3 months of incarceration at enrolment) and TBI with long incarceration (they had ≥1 year of incarceration at enrolment)].
2.3.3 Cytokines and chemokine selection and detection
The cytokines/chemokines quantified in the study were selected based on: 1) a systematic review we conducted to identify the relevant cytokines/chemokines associated with TBI (14), 2) published reviews about the pathophysiology of M. tuberculosis infection (15–17), 3) results from animal models (guinea pig, macaques and mice) (15, 16, 18–20), 4) immune response to intracellular bacteria (21, 22), 5) M. tuberculosis pathway (23) (available at: http://www.genome.jp/kegg/pathway.html) and 6) our previous results among people diagnosed with TB disease (24).
Commercial multiplex and single bead-based fluorescent assay kits were used to quantify 18 cytokines/chemokines of interest from plasma samples as follows: Macrophage Inflammatory Protein 3α (MIP-3α/CCL20), Human Cytokine/Chemokine magnetic Panel III, Milliplex® Map kit); Interleukin 18 (IL-18), Human IL-18 Singleplex Magnetic Bead kit, Milliplex® Map kit; soluble CD14 (sCD14), Human Cardiovascular Disease (CVD) Panel 6 Magnetic Bead kit, Milliplex® Map kit; Eotaxin 1 (CCL11), Interferon gamma (INF-γ), Interleukin 5 (IL-5), Interleukin 6 (IL-6), Interleukin 10 (IL-10), Interleukin IL-12 p40 homodimer (IL-12[p40]), Interleukin 13 (IL-13), Interleukin 15 (IL-15), Interleukin (IL-17), Interleukin-1 receptor antagonist (IL-1RA), human interferon-inducible protein 10 (CXCL10/IP-10), monocyte chemoattractant protein-1 (CCL2/MCP-1), macrophage inflammatory protein 1α (CCL3/MIP-1α), macrophage inflammatory protein 1β (CCL4/MIP-1β), Tumor necrosis factor alpha (TNF-α), Human Cytokine/Chemokine magnetic Bead Panel, Milliplex® Map kit, Millipore Corporation, Billeria, MA, USA.
The assays were performed according to the manufacturer’s instructions, using 25 µl of plasma per sample (5 µl to run the sCD14 assay) and overnight incubation. Standards were reconstituted and serially diluted to generate standard curves. Two controls with low and high concentrations, provided by the commercial kit, were included in each assay and considered positive controls for the experiment. Results were analyzed in the BioPlex-200 instrument (Bio-Rad, Mississauga, Canada), reported as mean fluorescence intensity, and converted to pg/ml or ng/ml using the BioPlex® Manager version 6.0 (Bio-Rad, Mississauga, ON).
To control for potential biases, all specimens were analyzed, blinded to the clinical status, and longitudinal samples were performed by the same person and analyzed on the same plate. Samples with values outside the standard curve range were assigned a value of one-half of the lower limit of detection (LOD divided by 2) in pg/mL.
2.4 Analysis
We used descriptive statistics (median [IQR] and n [%]) to report cytokines/chemokines and socio-demographic variables, and chi-squared and Kruskal Wallis tests to evaluate differences between groups. The primary outcome of this study was a new TBI stratified by short and long incarceration.
In individuals with new TBI, we compared the concentration of the immune mediators at baseline, three months before the TST conversion (pre-conversion point) and TST conversion. Wilcoxon test was used to compare the changes in cytokines/chemokines over time. In addition, we used the Mann-Whitney test to compare each follow-up between individuals with a new TBI with short and long times of incarceration.
Then, we compared plasma concentration of cytokines/chemokines in people with new TBI with short and long incarceration to non-infected individuals and people diagnosed with pulmonary TB using the Kruskal-Wallis test. We used multinomial logistic regression to determine the association between each cytokine/chemokine and new TBI with short or long incarceration compared to TB disease and non-infected groups. All cytokines/chemokines were log-transformed to adjust for skewness before the multivariable analysis. Variables included in the final regression model were selected using the biological plausibility criteria, a manual backward elimination method. We adjusted the model by age, BCG scar, contact with a person diagnosed with TB and drug use. All models were adjusted by cluster effect (15 different courtyards in the two prisons).
All analyses were done using STATA® version 14. A two-tailed p-value <0.05 was considered significant. Considering the multiple comparisons were made when we evaluated the cytokine concentration between the groups, a p-value <0.01 was considered significant, applying the Bonferroni correction.
3 Results
3.1 Study participants
The median time of TST conversion (new TBI) among the short incarceration group was 12.3 months, and in the new TBI with long incarceration group was 40.5 months (Figure 1). The majority of new TBIs occurred in one prison (75%).
There was no significant difference between groups regarding the mean age (Table 1). Ten individuals in the study had a body mass index ≤18.5 kg/m2, and 9 of them had a TB diagnosis. Individuals diagnosed with TB reported higher consumption of smoked drugs (61.7%) and inhaled drugs (34%) compared to the other groups (p ≤ 0.001). There was no difference in the frequency of alcohol consumption and tobacco use (p>0.051) (Table 1). Having contact with a person diagnosed with TB was reported in 8.3% of the new TBI group, 34% of people diagnosed with TB, and 19.2% in the non-infected group. Table 1 reports socio-demographic information at baseline.
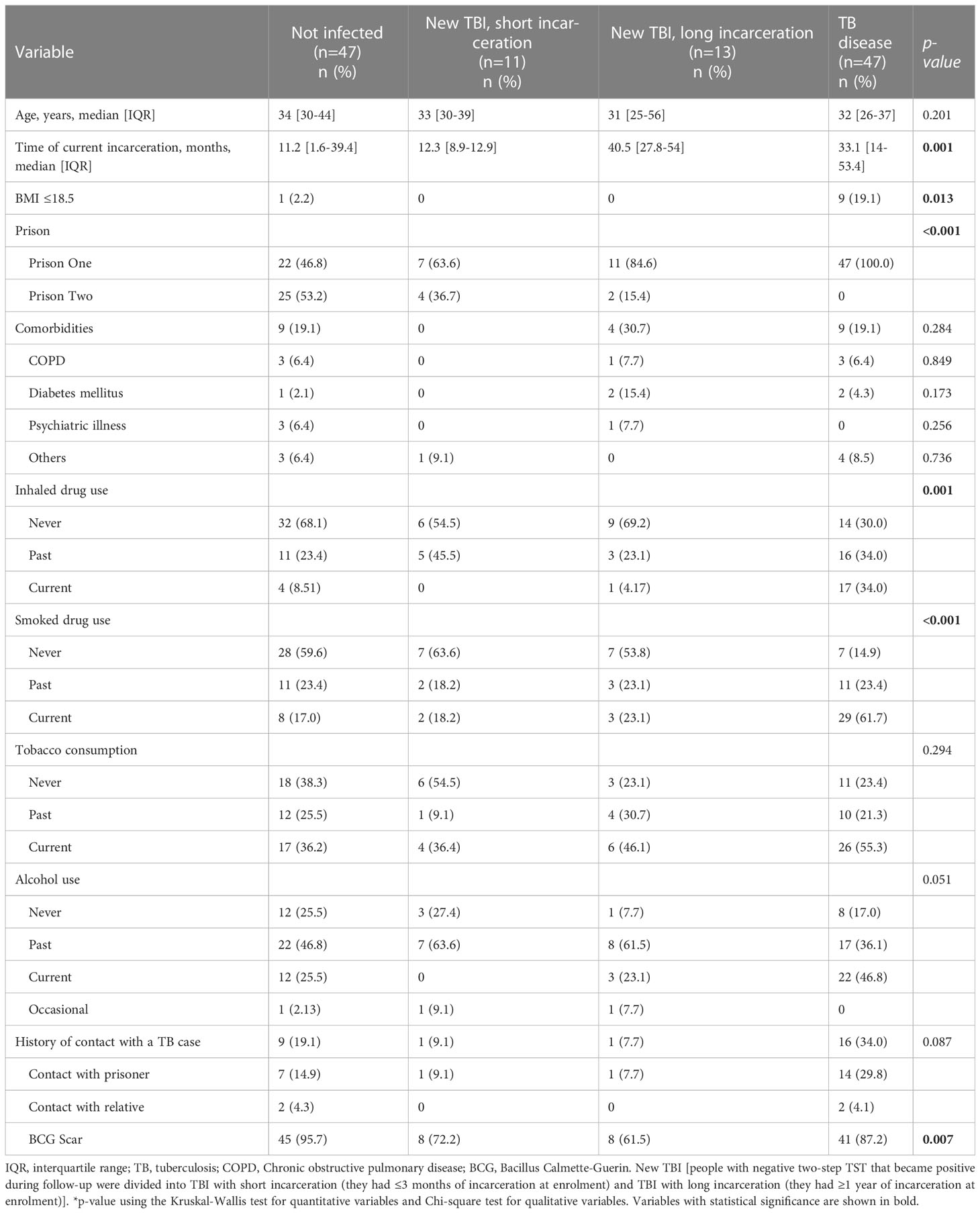
Table 1 Baseline characteristics of study participants diagnosed with a new TBI (with short and long incarceration), TB disease, and non-infected people.
IL-10, IL-12, IL-13, IL-15, IL-17A, IL-1RA, IL-5, IL-6 and MIP-1α had more than 40% of the results below the lower detection limit. We did not see consistent trends of these results within and between groups or by the follow-up time. Therefore, these cytokines/chemokines were excluded from the analysis and results.
3.2 The concentration of cytokines/chemokines among the new TBI group is affected by the time of incarceration
Supplementary Material S2 reports the median concentrations of all immune parameters at baseline, pre-conversion and TST conversion by short and long incarceration among the new TBI group.
Our results showed that there were no differences in the concentrations of MIP-3α (Figure 2A), Eotaxin (Figure 2B), INF-γ (Figure 2D) and MIP-1β (Figure 2F). We found higher concentrations of sCD14 at baseline compared to pre-conversion and TST conversion points; the change was more evident in PDL with long incarceration (Figure 2C). PDL with short incarceration showed increased plasma levels of IL-18 at baseline and pre-conversion, compared to the time of TST conversion (Figure 2E). MCP-1 showed an increased concentration over time, with a higher concentration at the time of TST conversion (Figure 2G). We also found that in PDL with short incarceration, IP-10 decreased three months before TST conversion (Figure 2H).
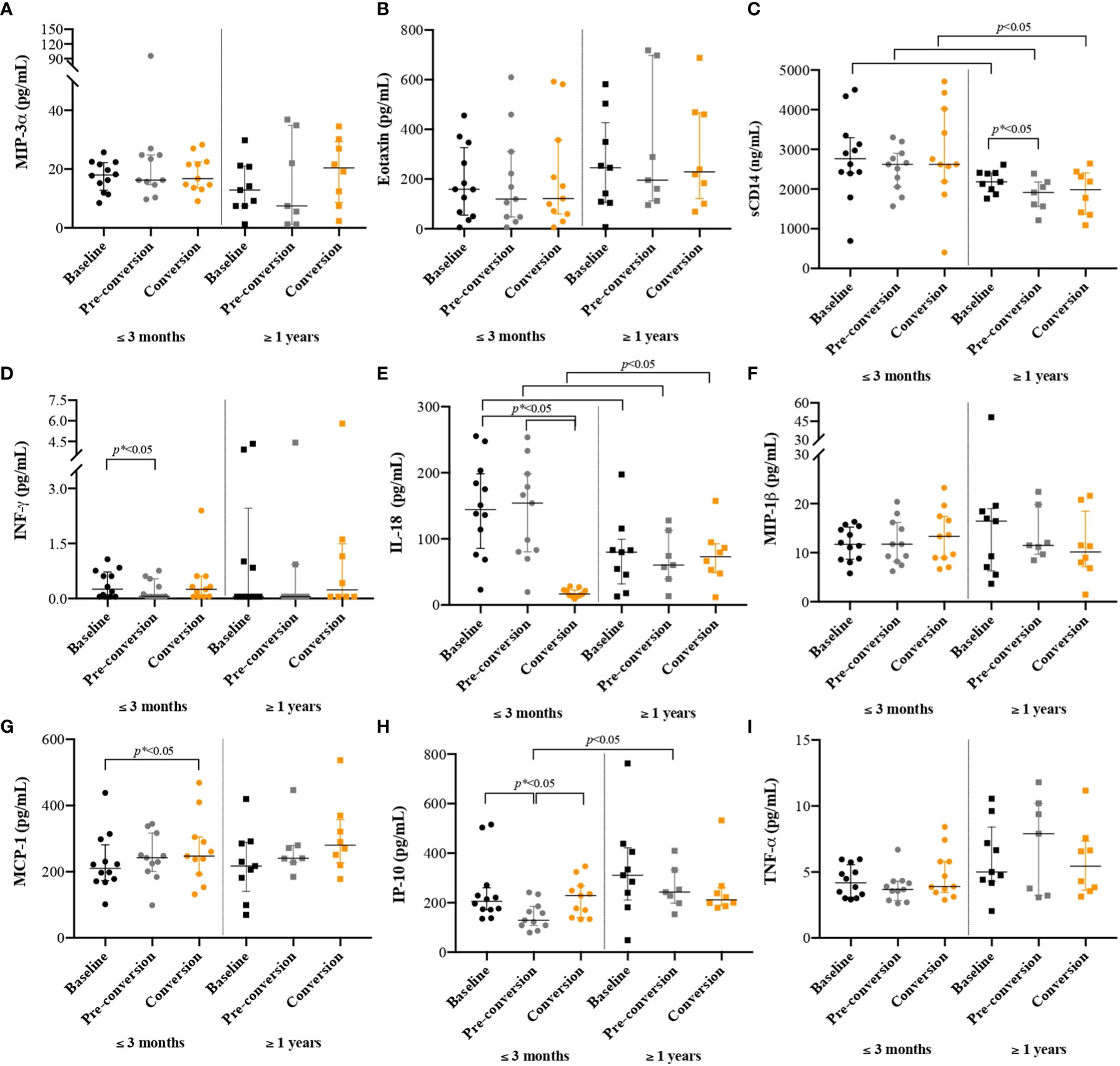
Figure 2 Cytokines/chemokines concentrations among people with new latent tuberculosis infection at baseline, pre-conversion, and TST conversion, by the time of incarceration. New TBI [people with negative two-step TST that became positive during follow-up were divided into new TBI with short incarceration (they had ≤3 months of incarceration at enrolment) and new TBI with long incarceration (they had ≥1 year of incarceration at enrolment)]. Values are reported in pg/ml for all cytokines/chemokines, except for sCD14 where results are reported in ng/ml. Boxplots are median and interquartile range. (A) MIP-3α; (B) Eotaxin; (C) sCD14; (ng/ml) (D) INF-γ; (E) IL-18; (F) MIP-1β; (G) MCP-1; (H) IP-10; (I) TNF-α.
The concentrations of sCD14 (Figure 2C) and IL-18 (Figure 2E) were higher among people with new TBI and short incarceration compared to those with long incarceration (p< 0.05). The concentration of IP-10 (Figure 2H) was higher in people with long incarceration.
3.3 Cytokines/chemokines concentrations are different between groups
We found higher concentrations of sCD14 and TNF-α among people diagnosed with TB, MIP-3α among people with new TBI and short incarceration, and IL-18 and MCP-1 among people with new TBI and long incarceration (Table 2). We did not find differences in the concentration of Eotaxin, INF-γ, MIP-1β, and IP-10 among the groups (Figures 3B, 3D, 3F, 3H).
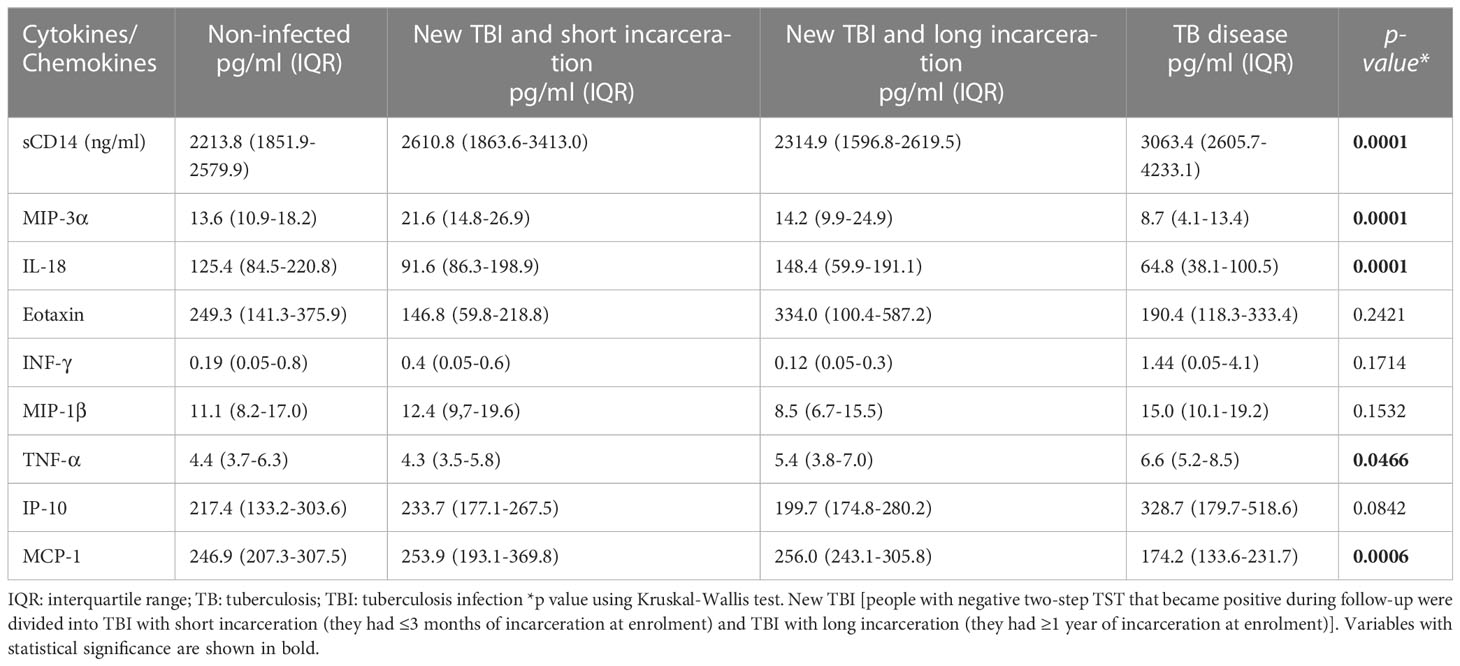
Table 2 The concentration cytokine/chemokine and differences between the groups (new TBI [with short and long incarceration], TB disease, and non-infected).
The concentration of sCD14 was higher in the TB disease group compared to non-infected individuals and new TBI with long incarceration group (Figure 3C). TNF-α was higher in the TB disease group compared to non-infected individuals (Figure 3I). MIP-3α was higher in the new TBI with short incarceration compared to the TB disease group (Figure 3A). MCP-1 was higher in the new TBI with long incarceration compared to the TB disease group (Figure 3G). IL-18 (Figure 3E) and MCP-1 (Figure 3G) concentrations were lower in the TB disease group compared to non-infected individuals.
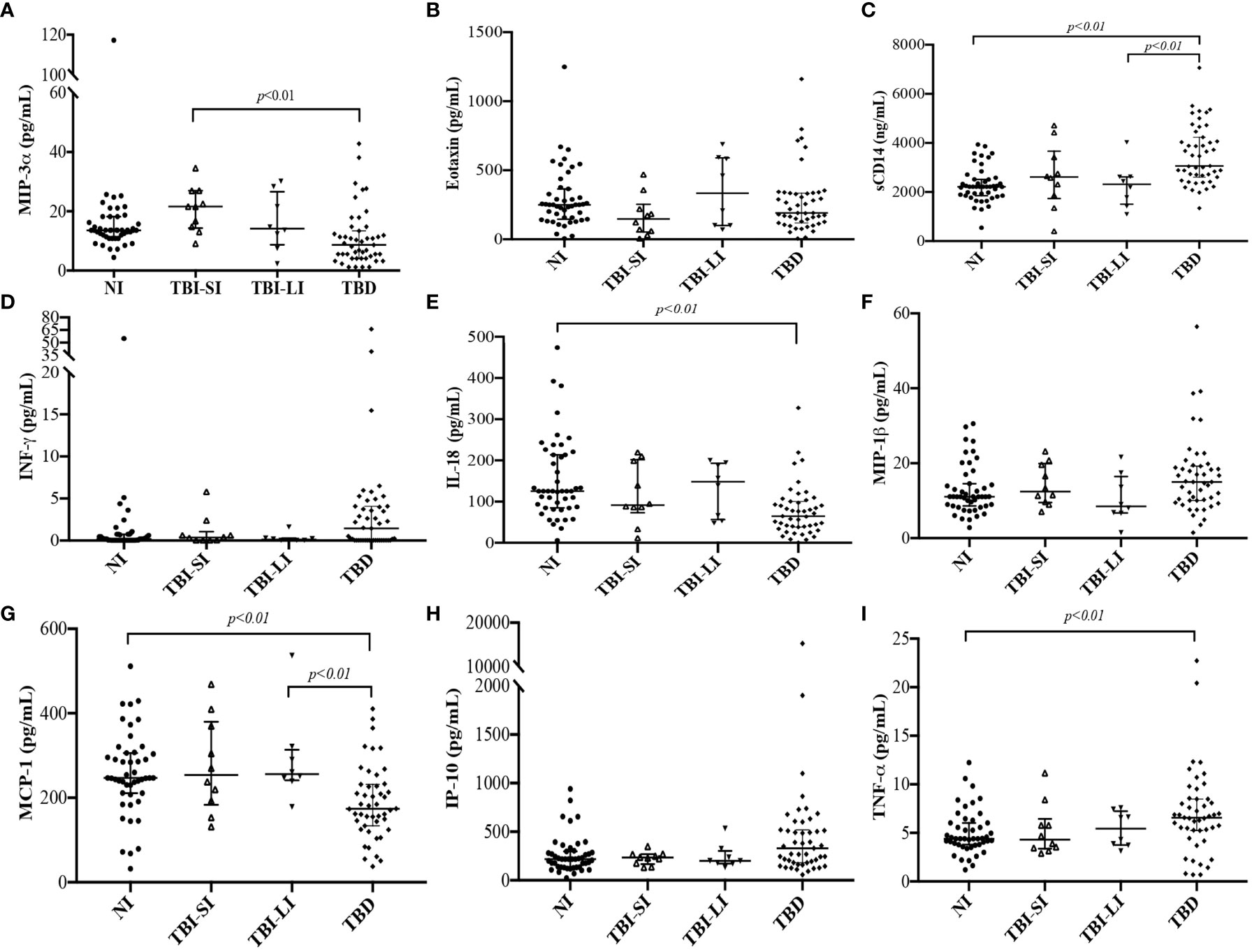
Figure 3 Immune parameters concentrations among people who converted the TST (new TBI with short incarceration [n= 11] and TBI with long incarceration [n= 13]), people diagnosed with TB disease (n=47), and non-infected people (n=47). New TBI [people with negative two-step TST that became positive during follow-up were divided into new TBI with short incarceration (they had ≤3 months of incarceration at enrolment) and new TBI with long incarceration (they had ≥1 year of incarceration at enrolment)]. (A) MIP-3α; (B) Eotaxin; (C) sCD14 (ng/ml); (D) INF-γ; (E) IL-18; (F) MIP-1β; (G) MCP-1; (H) IP-10; (I) TNF-α. Values reported in pg/ml for all cytokines, except for sCD14 reported in ng/ml. Boxplots are median and interquartile range. p-value using the Mann-Whitney U test and adjusted by multiple comparisons.
3.4 There are cytokines/chemokines associated with a new TBI and TB disease
In the multivariable analysis, only having a BCG scar was associated with decreased risk of a new TBI in people with short incarceration. The other socio-demographic variables (age, history of having contact with a person diagnosed with TB, and drug use) were not associated with new TBI or TB disease. Although having a BMI of less than 18 kg/m2 and alcohol consumption were significant factors, the multinomial model did not converge when we included those variables due to the low number of outcomes in the ‘yes’ category.
In the final multinomial model (Table 3), we identified that higher concentrations of MIP-3α were associated with a higher risk of a new TBI. The presence of a BCG scar and higher concentrations of Eotaxin were associated with a lower risk of a new TBI among people with short incarceration compared to non-infected individuals.
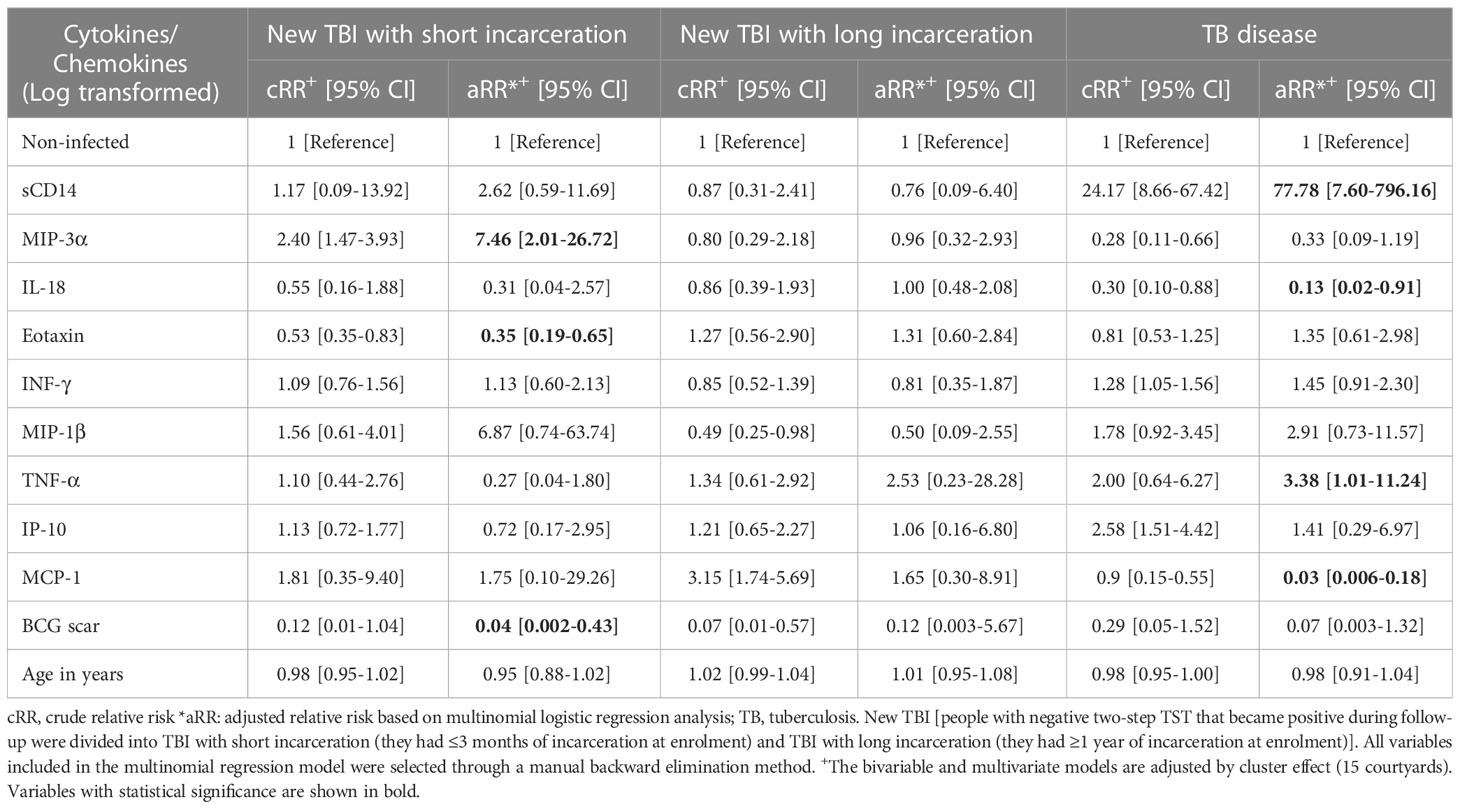
Table 3 Cytokines/chemokines associated with new TBI with short or long incarceration, TB disease compared to non-infected people in a multinomial regression model.
Higher plasma concentrations of sCD14 and TNF-α were associated with an increased risk of TB disease. Finally, we found that higher concentrations of IL-18 and MCP-1 were associated with a lower risk of TB disease compared to non-infected individuals (Table 3).
4 Discussion
The main results of our study were: 1) the concentration of immune mediators in persons with a new TBI varies according to the time of incarceration (short or long incarceration); and 2) Among people with short incarceration, high concentrations of MIP-3α were associated with a higher risk of a new TBI, and higher concentrations of Eotaxin was associated with a lower risk of a new TBI. Higher concentrations of sCD14 and TNF-α were associated with a higher risk of TB disease, and higher concentrations of IL-18 and MCP-1 were associated with a lower risk of TB disease.
In this study, immune mediators’ concentration varied among people newly infected by the time of incarceration. Levels of sCD14 and IL-18 were increased in individuals with short incarceration and IP-10 in those with long incarceration. These variations between people could occur for several reasons: 1) early exposure to M. tuberculosis can induce increased macrophage activation, leading to elevated sCD14 concentration. 2) Continuously exposed individuals (≥1 year in prison) could eliminate the bacteria repeatedly, owing to an effective immune response. 3) Other factors associated with prison entry include stress, physical activity, or dietary patterns. Several studies have documented changes in the production of pro-inflammatory cytokines when people are under a chronic stressful situation (25), variable levels of physical activity/exercise (26), or when a stress-generating situation is compounded by an infectious process, such as influenza vaccination (27). People deprived of liberty are exposed to acute and chronic stressors, given the social and safety conditions that prevail in prisons in which they live or the changes associated with freedom deprivation (28). In other words- the inflammatory milieu induced by environmental factors related to imprisonment may alter the immune response, thereby increasing the risk of MTB infection (29).
We found that increased circulating levels of MIP-3α were associated with a higher risk of having new TBI after short incarceration. The MIP-3α/CCL20 is a dendritic cell, T cell, B cell and monocyte chemoattractant involved in lymphocyte homeostasis and trafficking, cell proliferation and activation (21, 30, 31). Rivero-Lezcano et al. reported that the expression of MIP-3α increased up to 39-fold when monocytes from healthy donors were infected with M. tuberculosis (32), and Lee et al. showed that MIP-3α was up-regulated in PBMC and bronchoalveolar lavage fluids from people diagnosed with TB compared to healthy controls after in vitro stimulation with the 30 kDa antigen (Ag) of M. tuberculosis (33). MIP-3α concentration in serum/plasma samples is an attractive target for further evaluation in newly acquired MTB infection studies among people with documented recent exposure. In our research, PDL with a new TBI and with short incarceration had lower circulating levels of Eotaxin. Eotaxin has been reported as inhibited after in vitro infection by M. tuberculosis (34), decreased in people diagnosed with TB disease, and with increasing levels after anti-TB treatment (35).
After adjusting for the other co-variables, individuals with elevated concentrations of MCP-1, a potent chemotactic factor for monocytes (36), had a lower risk of TB disease. In a model of macaques previously vaccinated with BCG, the production of β-chemokine MCP-1 increased in lung lesions of animals after five weeks of infection with MTB (37). These immune molecules are mainly associated with the recruitment of monocytes/macrophages, in keeping with the observation that animals had a higher frequency of macrophages (CD14+ CD68+) in peripheral blood three weeks post-infection with MTB (37). In this case, the increase in cells and β-chemokines may be a sign of the recruitment of these cells from peripheral blood to the site of infection in the lung. Our results show that the concentrations of MCP-1 were lower among the TB disease group compared to non-infected individuals; however, most reports about this chemokine have shown increased concentration among people diagnosed with TB (38, 39). Results may be different because of stimulation with mycobacterial antigens or the absence of stimulation in whole blood samples. Increased concentrations of MCP-1 have been reported in stimulated samples from individuals diagnosed with active TB, but higher concentrations in un-stimulated samples among non-infected individuals compared to individuals diagnosed with TB (40). Some additional explanations for those differences between the studies are the ancestry of the population, the specimen (serum, whole blood, or supernatants of cultured PBMC), and the presence of genetic polymorphisms regulating IL-18 or MCP-1 production (41) in the populations, which could alter the final structure, concentration, or function of the protein.
We also found that higher concentrations of sCD14 and TNF-α were associated with a higher risk of TB disease. These immune mediators are highly involved in monocyte/macrophage activation and trafficking pathways (42, 43). Macrophages are the main niche for the growth and survival of M. tuberculosis (44); likewise, macrophages are the most important cells for infection control in animal and human models and critical cells in the host response during active disease (44–46) trying to limit the systemic spread of mycobacteria (47). Soluble CD14, whose membrane-bound portion is highly expressed in these cells, is a mediator of macrophage activation and serves as a receptor for mycobacterial lipoarabinomannan (43). It has been reported among people diagnosed with TB disease (48, 49), with or without concurrent HIV infection (47, 50), and individuals with diabetes mellitus (51). A published article by Lawn SD et al. suggests that sCD14 might increase the concentration of TNF-α due to the high load of mycobacterial antigens among people living with HIV and co-infected with TB disease (47). In our study, people diagnosed with TB had higher plasma concentrations of TNF-α, presumably contributing to cell recruitment, the production of other pro-inflammatory cytokines, and apoptosis of MTB-infected cells (52). Similar to our study, TNF-α has been reported to be increased in adults (49, 53–56) and children (57) diagnosed with TB disease.
Our findings show lower concentrations of IL-18 and MCP-1 among the TB group compared to non-infected individuals. Other researchers have shown that M. tuberculosis-stimulated culture supernatants from people diagnosed with TB have lower concentrations of IL-18 compared to those from healthy TST converters (58). IL-18 has an important function in TB as a pro-inflammatory cytokine (59); it plays an important role in the T-cell-helper type 1 (Th1) response, primarily by its ability to induce IFN-γ production in T cells and natural killer (NK) cells (60) and in combination with IL-12 triggers the antimicrobial protein cathelicidin and autophagy, resulting in inhibition of intracellular mycobacteria in macrophages and lung epithelial cells (61). Still, studies in humans are not conclusive. For example, Yamada G et al. (62) showed that increased serum IL-18 concentrations were associated with TB disease compared to healthy individuals (including TST converters).
Future studies are needed to validate and complement our cytokine and chemokine findings.
We did not find associations between IP-10, IL-17, IL-10, and a new TBI (the first one was not different between groups, and the latter two had concentrations below the lower detection limit), contrary to other publications (63, 64). This discrepancy may be attributed to the measurement of immune mediators after mycobacterial antigen and mitogen stimulation in prior studies (53, 56, 65–75), in contrast to the unstimulated measurements in our study. In the case of IL-17, there are six members in the IL-17 family, including IL-17A. IL-17B, IL-17C, IL-17D, IL-17E, and IL-17F (76, 77). Our study only measured Il-17A. Therefore, it is possible that other not measured subunits, such as IL-17F, were increased in plasma samples. In the same way, IL-17 is described as essential in the lung during recent infection (60). Still, perhaps blood sampling in the context of recent infection may have contributed to the reduced systemic level of IL-17.
Another reason for discrepant results may be that individuals included in other longitudinal studies were evaluated with only one TST administration, and those with a negative TST may represent a false negative result (61). In our previous studies, a second administration of TST identified an additional 11.6% positive TST individuals (78).
Our study’s most crucial distinguishing feature is the study design (cohort). In cross-sectional studies, the duration an individual has had TBI cannot be quantified. The main limitation of a cross-sectional approach is that human and primate studies have demonstrated there is a spectrum of TB stages (5, 6, 79, 80), on which there is little published work (4, 6), and that potentially could alter the concentration of the immune parameters according to the stage of the infection. The cohort design allowed us to quantify the concentration of cytokines at a temporal point close to the time of infection (new infection) and identify individuals in whom infection occurred recently. Borgstrom WE et al., using mathematical models and CD4+ T-cell flow-cytometry data, showed that the most specific prediction of recent TBI was a high proliferative CD4+ response to CFP-10 and PPD, and a low response to ESAT-6 at ≤1 month after exposure (81).
Our study has other strengths: rigorous selection criteria among people diagnosed with TB, including only those with microbiological confirmation and with less than 15 days of treatment, rule out of booster effect, and rigorous monitoring and follow-up every three months to all uninfected participants.
The main limitation of this study is that some bacterial factors may modify the response to cytokines, such as the virulence of some strains; however, in people with TBI, it is not feasible to isolate the mycobacterium, and therefore the role of MTB strain cannot be assessed yet.
5 Conclusion
Our study found that immune markers vary according to the time of incarceration. Among people with short incarceration, high concentrations of MIP-3α were associated with a higher risk of a new TBI, and higher concentrations of Eotaxin were associated with a lower risk of a new TBI. Higher concentrations of sCD14 and TNF-α were associated with a higher risk of TB disease, and higher concentrations of IL-18 and MCP-1 were associated with a lower risk of TB disease. It is necessary to have more cohort studies that evaluate the changes over time of these and other cytokines/chemokines to understand the immune mechanisms across the spectrum of TB.
Data availability statement
The raw data supporting the conclusions of this article will be made available by the authors, without undue reservation.
Ethics statement
The studies involving human participants were reviewed and approved by Ethics Committees of the Universidad Pontificia Bolivariana and the University of Manitoba. The patients/participants provided their written informed consent to participate in this study.
Author contributions
Substantial contributions to the conception or design of the work: MH, YK, ZR. Acquisition of data: MH. Analysis and interpretation of data: MH, YK, LL, DM, ZR. Drafting the article and revising it critically for important intellectual content: MH, YK, LV, PM, ZR. Final approval of the version to be published: MH, YK, LV, LL, DM, PM, ZR. Agreement to be accountable for all aspects of the work in ensuring that questions related to the accuracy or integrity of any part of the work are appropriately investigated and resolved: MH, YK, LL, DM, LV, PM, ZR. Principal investigator and funding acquisition: ZVR. All authors contributed to the article and approved the submitted version.
Funding
This article (the design of the study, the collection, analysis, and interpretation of data) was funded by The Administrative Department of Science, Technology and Innovation (Colciencias), as part of the research project entitled “Host gene expression profile used to identify latent TB infection and the transition to active disease - Perfil de la expresión génica del hospedero para identificar tuberculosis latente y la transición a enfermedad activa”, grant number: 121071249878. And the project “Pro-inflammatory patterns of cytokine/chemokine associated with latent tuberculosis in people deprived of liberty (PDL),” grant number: 639B-06/16-55, funded by Universidad Pontificia Bolivariana. The Administrative Department of Science, Technology and Innovation (Colciencias), scholarship program #647 in Colombia, and Emerging Leaders of Americas Program, 2018, a scholarship from the Government of Canada support the Ph.D. student. This manuscript was also supported, in part, by the Canada Research Chairs Program for ZR. Award number: 950-232963.
Acknowledgments
The authors are grateful to all of the PDL who accepted to participate in the study, to INPEC (Instituto Nacional Penitenciario y Carcelario de Colombia), and to the director of each prison and all personnel working there for their support in performing the study.
Conflict of interest
The authors declare that the research was conducted in the absence of any commercial or financial relationships that could be construed as a potential conflict of interest.
Publisher’s note
All claims expressed in this article are solely those of the authors and do not necessarily represent those of their affiliated organizations, or those of the publisher, the editors and the reviewers. Any product that may be evaluated in this article, or claim that may be made by its manufacturer, is not guaranteed or endorsed by the publisher.
Supplementary material
The Supplementary Material for this article can be found online at: https://www.frontiersin.org/articles/10.3389/fimmu.2023.1129398/full#supplementary-material
References
1. Matteelli A, Sulis G, Capone S, D’Ambrosio L, Migliori GB, Getahun H. Tuberculosis elimination and the challenge of latent tuberculosis. Presse Médicale (2017) 46:e13–21. doi: 10.1016/j.lpm.2017.01.015
2. Cohen A, Mathiasen VD, Schön T, Wejse C. The global prevalence of latent tuberculosis: a systematic review and meta-analysis. Eur Respir J (2019) 54:13–21. doi: 10.1183/13993003.00655-2019
3. Al-Darraji HAA, Kamarulzaman A, Altice FL. Latent tuberculosis infection in a Malaysian prison: implications for a comprehensive integrated control program in prisons. BMC Public Health (2014) 14:22. doi: 10.1186/1471-2458-14-22
4. Behr MA, Edelstein PH, Ramakrishnan L. Is mycobacterium tuberculosis infection life long? BMJ (2019) 367:l5770. doi: 10.1136/bmj.l5770
5. 3rd CEB, Boshoff HI, Dartois V, Dick T, Ehrt S, Flynn J, et al. The spectrum of latent tuberculosis: rethinking the biology and intervention strategies. Nat Rev Microbiol (2009) 7:nrmicro2236. doi: 10.1038/nrmicro2236
6. Lin PL, Flynn JL. The end of the binary era: revisiting the spectrum of tuberculosis. J Immunol Baltim Md 1950 (2018) 201:2541–8. doi: 10.4049/jimmunol.1800993
7. Schnappinger D, Ehrt S. A broader spectrum of tuberculosis. Nat Med (2016) 22:1076–7. doi: 10.1038/nm.4186
8. Kestler B, Tyler SK. Latent tuberculosis testing through the ages: the search for a sleeping killer. Am J Physiol - Lung Cell Mol Physiol (2022) 322:L412–9. doi: 10.1152/ajplung.00217.2021
9. Sudbury EL, Clifford V, Messina NL, Song R, Curtis N. Mycobacterium tuberculosis-specific cytokine biomarkers to differentiate active TB and LTBI: a systematic review. J Infect (2020) 81:873–81. doi: 10.1016/j.jinf.2020.09.032
10. Reichler MR, Khan A, Sterling TR, Zhao H, Moran J, McAuley J, et al. Risk and timing of tuberculosis among close contacts of persons with infectious tuberculosis. J Infect Dis (2018) 218:1000–8. doi: 10.1093/infdis/jiy265
11. Cords O, Martinez L, Warren JL, O’Marr JM, Walter KS, Cohen T, et al. Incidence and prevalence of tuberculosis in incarcerated populations: a systematic review and meta-analysis. Lancet Public Health (2021) 6:e300–8. doi: 10.1016/S2468-2667(21)00025-6
12. Arroyave L, Keynan Y, López L, Marin D, Arbeláez MP, Rueda ZV. Negative latent tuberculosis at time of incarceration: identifying a very high-risk group for infection. Epidemiol Infect (2017) 145:2491–9. doi: 10.1017/S0950268817001558
13. Herrera M, Keynan Y, López L, Marín D, Arroyave L, Arbeláez MP, et al. Incidence and risk factors associated with latent tuberculosis infection and pulmonary tuberculosis among people deprived of liberty in Colombian prisons. Am J Trop Med Hyg (2021) 106:66–74. doi: 10.4269/ajtmh.20-0307
14. Herrera M, Vera C, Keynan Y, Rueda ZV. Gaps in study design for immune parameter research for latent tuberculosis infection: a systematic review. J Immunol Res (2020) 2020:8074183. doi: 10.1155/2020/8074183
15. Etna MP, Giacomini E, Severa M, Coccia EM. Pro- and anti-inflammatory cytokines in tuberculosis: a two-edged sword in TB pathogenesis. Semin Immunol (2014) 26:543–51. doi: 10.1016/j.smim.2014.09.011
16. Ahmad S. Pathogenesis, immunology, and diagnosis of latent mycobacterium tuberculosis infection. J Immunol Res (2010) 2011:e814943. doi: 10.1155/2011/814943
17. Simmons JD, Stein CM, Seshadri C, Campo M, Alter G, Fortune S, et al. Immunological mechanisms of human resistance to persistent mycobacterium tuberculosis infection. Nat Rev Immunol (2018) 18:575–89. doi: 10.1038/s41577-018-0025-3
18. Zuñiga J, Torres-García D, Santos-Mendoza T, Rodriguez-Reyna TS, Granados J, Yunis EJ. Cellular and humoral mechanisms involved in the control of tuberculosis. Clin Dev Immunol (2012) 2012:193923. doi: 10.1155/2012/193923
19. Lyadova IV, Panteleev AV. Th1 and Th17 cells in tuberculosis: protection, pathology, and biomarkers. Mediators Inflammation (2015) 2015:1–13. doi: 10.1155/2015/854507
20. Lin PL, Flynn JL. Understanding latent tuberculosis: a moving target. J Immunol Baltim Md 1950 (2010) 185:15–22. doi: 10.4049/jimmunol.0903856
21. Cellular and molecular immunology . Available at: https://www.elsevier.com/books/cellular-and-molecular-immunology/abbas/978-0-323-47978-3 (Accessed October 31, 2017).
22. Bennett JE, Dolin R, Bennett JE. Enfermedades infecciosas. Principios y práctica. 9th Edition. Chapter 6 Cell-Mediated Defense Against Infection. (2020) Pag 69–72.
23. Kanehisa M, Furumichi M, Tanabe M, Sato Y, Morishima K. KEGG: new perspectives on genomes, pathways, diseases and drugs. Nucleic Acids Res (2017) 45:D353–61. doi: 10.1093/nar/gkw1092
24. Keynan Y, Trajtman A, McLaren P, Aguilar Y, Velez L, Rueda ZV. Inflammatory cytokine and gene expression patterns correlate with etiologic agent causing pneumonia among patients with advanced HIV in medellin, Colombia. J Clin Virol (2016) 82:S93. doi: 10.1016/j.jcv.2016.08.186
25. Tian R, Hou G, Li D, Yuan T-F. A possible change process of inflammatory cytokines in the prolonged chronic stress and its ultimate implications for health. Sci World J (2014) 2014:780616. doi: 10.1155/2014/780616
26. Zhou X, Fragala MS, McElhaney JE, Kuchel GA. Conceptual and methodological issues relevant to cytokine and inflammatory marker measurements in clinical research. Curr Opin Clin Nutr Metab Care (2010) 13:541–7. doi: 10.1097/MCO.0b013e32833cf3bc
27. Sribanditmongkol V, Neal JL, Patrick TE, Szalacha LA, McCarthy DO. Effect of perceived stress on cytokine production in healthy college students. West J Nurs Res (2015) 37:481–93. doi: 10.1177/0193945914545658
28. Lauren C. Porter. being “on point”: exploring the strees-related experiences of incarceration. Soc Ment Health (2018) 9:1–17. doi: 10.1177/2156869318771439
29. Tabung FK, Birmann BM, Epstein MM, Martínez-Maza O, Breen EC, Wu K, et al. Influence of dietary patterns on plasma soluble CD14, a surrogate marker of gut barrier dysfunction. Curr Dev Nutr (2017) 1:1–11. doi: 10.3945/cdn.117.001396
30. Baba T, Mukaida N. Role of macrophage inflammatory protein (MIP)-1α/CCL3 in leukemogenesis. Mol Cell Oncol (2014) 1:1–5. doi: 10.4161/mco.29899
31. Aziz N, Detels R, Chang LC, Butch AW. Macrophage inflammatory protein-3 alpha (MIP-3α)/CCL20 in HIV-1-Infected individuals. J AIDS Clin Res (2016) 7:1–14. doi: 10.4172/2155-6113.1000587
32. Rivero-Lezcano OM, González-Cortés C, Reyes-Ruvalcaba D, Diez-Tascón C. CCL20 is overexpressed in mycobacterium tuberculosis-infected monocytes and inhibits the production of reactive oxygen species (ROS). Clin Exp Immunol (2010) 162:289–97. doi: 10.1111/j.1365-2249.2010.04168.x
33. Lee J-S, Lee J-Y, Son JW, Oh J-H, Shin D-M, Yuk J-M, et al. Expression and regulation of the CC-chemokine ligand 20 during human tuberculosis. Scand J Immunol (2008) 67:77–85. doi: 10.1111/j.1365-3083.2007.02040.x
34. Riffo-Vasquez Y, Coates ARM, Page CP, Spina D. Mycobacterium tuberculosis chaperonin 60.1 inhibits leukocyte diapedesis in a murine model of allergic lung inflammation. Am J Respir Cell Mol Biol (2012) 47:245–52. doi: 10.1165/rcmb.2011-0412OC
35. Yang Q, Cai Y, Zhao W, Wu F, Zhang M, Luo K, et al. IP-10 and MIG are compartmentalized at the site of disease during pleural and meningeal tuberculosis and are decreased after antituberculosis treatment. Clin Vaccine Immunol (2014) 21:1635–44. doi: 10.1128/CVI.00499-14
36. Deshmane SL, Kremlev S, Amini S, Sawaya BE. Monocyte chemoattractant protein-1 (MCP-1): an overview. J Interferon Cytokine Res (2009) 29:313–26. doi: 10.1089/jir.2008.0027
37. Dutta NK, McLachlan J, Mehra S, Kaushal D. Humoral and lung immune responses to mycobacterium tuberculosis infection in a primate model of protection. Trials Vaccinol (2014) 3:47–51. doi: 10.1016/j.trivac.2014.02.001
38. Wang Y, Li H, Bao H, Jin Y, Liu X, Wu X, et al. Auxiliary diagnostic value of monocyte chemoattractant protein-1 of whole blood in active tuberculosis. Int J Clin Exp Med (2015) 8:9454–61.
39. Suzukawa M, Akashi S, Nagai H, Nagase H, Nakamura H, Matsui H, et al. Combined analysis of IFN-γ, IL-2, IL-5, IL-10, IL-1RA and MCP-1 in QFT supernatant is useful for distinguishing active tuberculosis from latent infection. PloS One (2016) 11:e0152483. doi: 10.1371/journal.pone.0152483
40. Ruhwald M, Bjerregaard-Andersen M, Rabna P, Eugen-Olsen J, Ravn P. IP-10, MCP-1, MCP-2, MCP-3, and IL-1RA hold promise as biomarkers for infection with m. tuberculosis in a whole blood based T-cell assay. BMC Res Notes (2009) 2:19. doi: 10.1186/1756-0500-2-19
41. Zhang Y, Zhang J, Zeng L, Huang H, Yang M, Fu X, et al. The -2518A/G polymorphism in the MCP-1 gene and tuberculosis risk: a meta-analysis. PloS One (2012) 7:e38918. doi: 10.1371/journal.pone.0038918
42. Menten P, Wuyts A, Van Damme J. Macrophage inflammatory protein-1. Cytokine Growth Factor Rev (2002) 13:455–81. doi: 10.1016/S1359-6101(02)00045-X
43. Yu W, Soprana E, Cosentino G, Volta M, Lichenstein HS, Viale G, et al. Soluble CD14(1-152) confers responsiveness to both lipoarabinomannan and lipopolysaccharide in a novel HL-60 cell bioassay. J Immunol Baltim Md 1950 (1998) 161:4244–51. doi: 10.4049/jimmunol.161.8.4244
44. Guirado E, Schlesinger LS, Kaplan G. Macrophages in tuberculosis: friend or foe. Semin Immunopathol (2013) 35:563–83. doi: 10.1007/s00281-013-0388-2
45. Marino S, Cilfone NA, Mattila JT, Linderman JJ, Flynn JL, Kirschner DE. Macrophage polarization drives granuloma outcome during mycobacterium tuberculosis infection. Infect Immun (2015) 83:324–38. doi: 10.1128/IAI.02494-14
46. Flynn J, Chan J, Lin P. Macrophages and control of granulomatous inflammation in tuberculosis. Mucosal Immunol (2011) 4:271–8. doi: 10.1038/mi.2011.14
47. Lawn SD, Labeta MO, Arias M, Acheampong JW, Griffin GE. Elevated serum concentrations of soluble CD14 in HIV– and HIV+ patients with tuberculosis in Africa: prolonged elevation during anti-tuberculosis treatment. Clin Exp Immunol (2000) 120:483–7. doi: 10.1046/j.1365-2249.2000.01246.x
48. Ayaslioglu E, Kalpaklioglu F, Kavut AB, Erturk A, Capan N, Birben E. The role of CD14 gene promoter polymorphism in tuberculosis susceptibility. J Microbiol Immunol Infect (2013) 46:158–63. doi: 10.1016/j.jmii.2012.05.008
49. Zambuzi FA, Cardoso-Silva PM, Espindola MS, Soares LS, Galvão-Lima LJ, Brauer VS, et al. Identification of promising plasma immune biomarkers to differentiate active pulmonary tuberculosis. Cytokine (2016) 88:99–107. doi: 10.1016/j.cyto.2016.08.030
50. Wyndham-Thomas C, Corbière V, Selis E, Payen M-C, Goffard J-C, Van Vooren J-P, et al. Immune activation by mycobacterium tuberculosis in HIV-infected and -uninfected subjects. J Acquir Immune Defic Syndr 1999 (2017) 74:103–11. doi: 10.1097/QAI.0000000000001157
51. Kumar NP, Moideen K, Bhootra Y, Nancy A, Viswanathan V, Shruthi BS, et al. Elevated circulating levels of monocyte activation markers among tuberculosis patients with diabetes co-morbidity. Immunology (2019) 156:249–58. doi: 10.1111/imm.13023
52. Lin PL, Plessner HL, Voitenok NN, Flynn JL. Tumor necrosis factor and tuberculosis. J Investig Dermatol Symp Proc (2007) 12(1):22–5. doi: 10.1038/sj.jidsymp.5650027
53. Won E-J, Choi J-H, Cho Y-N, Jin H-M, Kee HJ, Park Y-W, et al. Biomarkers for discrimination between latent tuberculosis infection and active tuberculosis disease. J Infect (2017) 74:281–93. doi: 10.1016/j.jinf.2016.11.010
54. Belay M, Legesse M, Mihret A, Bekele Y, Ottenhoff THM, Franken KLMC, et al. Pro- and anti-inflammatory cytokines against Rv2031 are elevated during latent tuberculosis: a study in cohorts of tuberculosis patients, household contacts and community controls in an endemic setting. PloS One (2015) 10:e0124134. doi: 10.1371/journal.pone.0124134
55. Wei M, Wu ZY, Lin JH, Li Y, Qian ZX, Xie YQ, et al. Regulation network of serum cytokines induced by tuberculosis-specific antigens reveals biomarkers for tuberculosis diagnosis. Genet Mol Res GMR (2015) 14:17182–92. doi: 10.4238/2015.December.16.18
56. Jeong YH, Hur Y-G, Lee H, Kim S, Cho J-E, Chang J, et al. Discrimination between active and latent tuberculosis based on ratio of antigen-specific to mitogen-induced IP-10 production. J Clin Microbiol (2015) 53:504–10. doi: 10.1128/JCM.02758-14
57. Tebruegge M, Dutta B, Donath S, Ritz N, Forbes B, Camacho-Badilla K, et al. Mycobacteria-specific cytokine responses detect tuberculosis infection and distinguish latent from active tuberculosis. Am J Respir Crit Care Med (2015) 192:485–99. doi: 10.1164/rccm.201501-0059OC
58. Vankayalapati RR, Wizel B, Weis SE, Samten B, Girard WM, Barnes PF. Production of interleukin-18 in human tuberculosis. J Infect Dis (2000) 182:234–9. doi: 10.1086/315656
59. Schneider BE, Korbel D, Hagens K, Koch M, Raupach B, Enders J, et al. A role for IL-18 in protective immunity against mycobacterium tuberculosis. Eur J Immunol (2010) 40:396–405. doi: 10.1002/eji.200939583
60. Khader SA, Bell GK, Pearl JE, Fountain JJ, Rangel-Moreno J, Cilley GE, et al. IL-23 and IL-17 in the establishment of protective pulmonary CD4 + T cell responses after vaccination and during mycobacterium tuberculosis challenge. Nat Immunol (2007) 8:369–77. doi: 10.1038/ni1449
61. Menzies D. Interpretation of repeated tuberculin tests. boosting, conversion, and reversion. Am J Respir Crit Care Med (1999) 159:15–21. doi: 10.1164/ajrccm.159.1.9801120
62. Yamada G, Shijubo N, Shigehara K, Okamura H, Kurimoto M, Abe S. Increased levels of circulating interleukin-18 in patients with advanced tuberculosis. Am J Respir Crit Care Med (2000) 161:1786–9. doi: 10.1164/ajrccm.161.6.9911054
63. Buchwald UK, Adetifa IMO, Bottomley C, Owiafe PK, Donkor S, Bojang AL, et al. Broad adaptive immune responses to m. tuberculosis antigens precede TST conversion in tuberculosis exposed household contacts in a TB-endemic setting. PloS One (2014) 9:1–17. doi: 10.1371/journal.pone.0116268
64. Hussain R, Talat N, Shahid F, Dawood G. Biomarker changes associated with tuberculin skin test (TST) conversion: a two-year longitudinal follow-up study in exposed household contacts. PloS One (2009) 4:1–9. doi: 10.1371/journal.pone.0007444
65. Hur Y-G, Kang YA, Jang S-H, Hong JY, Kim A, Lee SA, et al. Adjunctive biomarkers for improving diagnosis of tuberculosis and monitoring therapeutic effects. J Infect (2015) 70:346–55. doi: 10.1016/j.jinf.2014.10.019
66. Kim K, Perera R, Tan DBA, Fernandez S, Seddiki N, Waring J, et al. Circulating mycobacterial-reactive CD4+ T cells with an immunosuppressive phenotype are higher in active tuberculosis than latent tuberculosis infection. Tuberc Edinb Scotl (2014) 94:494–501. doi: 10.1016/j.tube.2014.07.002
67. Hur Y-G, Gorak-Stolinska P, Ben-Smith A, Lalor MK, Chaguluka S, Dacombe R, et al. Combination of cytokine responses indicative of latent TB and active TB in Malawian adults. PloS One (2013) 8:1–10. doi: 10.1371/journal.pone.0079742
68. Hussain R, Kaleem A, Shahid F, Dojki M, Jamil B, Mehmood H, et al. Cytokine profiles using whole-blood assays can discriminate between tuberculosis patients and healthy endemic controls in a BCG-vaccinated population. J Immunol Methods (2002) 264:95–108. doi: 10.1016/S0022-1759(02)00092-3
69. Santin M, Morandeira-Rego F, Alcaide F, Rabuñal R, Anibarro L, Agüero-Balbín R, et al. Detection of interleukin-2 is not useful for distinguishing between latent and active tuberculosis in clinical practice: a prospective cohort study. Clin Microbiol Infect Off Publ Eur Soc Clin Microbiol Infect Dis (2016) 22:1007.e1–1007.e5. doi: 10.1016/j.cmi.2016.09.004
70. Wang S, Diao N, Lu C, Wu J, Gao Y, Chen J, et al. Evaluation of the diagnostic potential of IP-10 and IL-2 as biomarkers for the diagnosis of active and latent tuberculosis in a BCG-vaccinated population. PloS One (2012) 7:e51338. doi: 10.1371/journal.pone.0051338
71. Chegou NN, Black GF, Kidd M, van Helden PD, Walzl G. Host markers in quantiferon supernatants differentiate active TB from latent TB infection: preliminary report. BMC Pulm Med (2009) 9:21. doi: 10.1186/1471-2466-9-21
72. Sauzullo I, Mastroianni CM, Mengoni F, Ermocida A, Mascia C, Salotti A, et al. Long-term IFN-γ and IL-2 response for detection of latent tuberculosis infection in healthcare workers with discordant immunologic results. J Immunol Methods (2014) 414:51–7. doi: 10.1016/j.jim.2014.07.013
73. Anbarasu D, Raja CP, Raja A. Multiplex analysis of cytokines/chemokines as biomarkers that differentiate healthy contacts from tuberculosis patients in high endemic settings. Cytokine (2013) 61:747–54. doi: 10.1016/j.cyto.2012.12.031
74. Yao X, Liu Y, Liu Y, Liu W, Ye Z, Zheng C, et al. Multiplex analysis of plasma cytokines/chemokines showing different immune responses in active TB patients, latent TB infection and healthy participants. Tuberculosis (2017) 107:88–94. doi: 10.1016/j.tube.2017.07.013
75. Kamakia R, Kiazyk S, Waruk J, Meyers A, Ochanda J, Ball TB, et al. Potential biomarkers associated with discrimination between latent and active pulmonary tuberculosis. Int J Tuberc Lung Dis Off J Int Union Tuberc Lung Dis (2017) 21:278–85. doi: 10.5588/ijtld.16.0176
76. Jin W, Dong C. IL-17 cytokines in immunity and inflammation. Emerg Microbes Infect (2013) 2:e60. doi: 10.1038/emi.2013.58
77. Zenobia C, Hajishengallis G. Basic biology and role of interleukin-17 in immunity and inflammation. Periodontol 2000 (2015) 69:142–59. doi: 10.1111/prd.12083
78. Rueda ZV, Arroyave L, Marin D, López L, Keynan Y, Giraldo MR, et al. High prevalence and risk factors associated with latent tuberculous infection in two Colombian prisons. Int J Tuberc Lung Dis Off J Int Union Tuberc Lung Dis (2014) 18:1166–71. doi: 10.5588/ijtld.14.0179
79. Mehra S, Golden NA, Dutta NK, Midkiff CC, Alvarez X, Doyle LA, et al. Reactivation of latent tuberculosis in rhesus macaques by co-infection with simian immunodeficiency virus. J Med Primatol (2011) 40:233–43. doi: 10.1111/j.1600-0684.2011.00485.x
80. Kaushal D, Mehra S, Didier PJ, Lackner AA. The non-human primate model of tuberculosis. J Med Primatol (2012) 41:191–201. doi: 10.1111/j.1600-0684.2012.00536.x
Keywords: tuberculosis infection, TST conversion, Mycobacterium tuberculosis, cytokines, chemokines, tuberculosis
Citation: Herrera M, Keynan Y, Lopez L, Marín D, Vélez L, McLaren PJ and Rueda ZV (2023) Cytokine/chemokine profiles in people with recent infection by Mycobacterium tuberculosis. Front. Immunol. 14:1129398. doi: 10.3389/fimmu.2023.1129398
Received: 21 December 2022; Accepted: 26 April 2023;
Published: 16 May 2023.
Edited by:
Erwan Mortier, Centre National de la Recherche Scientifique (CNRS), FranceReviewed by:
Henrique Borges da Silva, Mayo Clinic Arizona, United StatesMarisa Benagiano, University of Florence, Italy
Copyright © 2023 Herrera, Keynan, Lopez, Marín, Vélez, McLaren and Rueda. This is an open-access article distributed under the terms of the Creative Commons Attribution License (CC BY). The use, distribution or reproduction in other forums is permitted, provided the original author(s) and the copyright owner(s) are credited and that the original publication in this journal is cited, in accordance with accepted academic practice. No use, distribution or reproduction is permitted which does not comply with these terms.
*Correspondence: Zulma Vanessa Rueda, enVsbWEucnVlZGFAdW1hbml0b2JhLmNh