- 1State Key Laboratory of Esophageal Cancer Prevention & Treatment, School of Basic Medical Sciences, Academy of Medical Sciences, Zhengzhou University, Zhengzhou, China
- 2Centre for Cancer Biomarkers & Biotherapeutics, Barts Cancer Institute, Queen Mary University of London, London, United Kingdom
Cancer immunotherapy (CIT) has emerged as an exciting new pillar of cancer treatment. Although benefits have been achieved in individual patients, the overall response rate is still not satisfactory. To address this, an ideal preclinical animal model for evaluating CIT is urgently needed. Syrian hamsters present similar features to humans with regard to their anatomy, physiology, and pathology. Notably, the histological features and pathological progression of tumors and the complexity of the tumor microenvironment are equivalent to the human scenario. This article reviews the current tumor models in Syrian hamster and the latest progress in their application to development of tumor treatments including immune checkpoint inhibitors, cytokines, adoptive cell therapy, cancer vaccines, and oncolytic viruses. This progress strongly advocates Syrian hamster as an ideal animal model for development and assessment of CIT for human cancer treatments. Additionally, the challenges of the Syrian hamster as an animal model for CIT are also discussed.
1 Introduction
With increasing incidence and mortality, cancer is a major public health problem worldwide and is a major obstacle to increasing life expectancy (1, 2). Cancer immunotherapy (CIT) works by stimulating or re-invigorating the body’s immune system to directly attack cancer cells (3). In recent years, the number of approved CIT drugs has increased dramatically and it has become a mainstay cancer therapy alongside traditional therapeutic options. Despite the widespread application of CIT in human cancers, only a minority of patients benefit from these therapies (4). Therefore, there is an urgent need for an ideal animal model that can accurately reflect the complexity of the immunosuppressive human tumor microenvironment to predict the immune response and efficacy of immunotherapy, promoting the development of CIT and reducing the failure rate of clinical trials.
Syrian hamster (Mesocricetus auratus) belongs to the Cricitinae family of hamsters and is commonly known as the golden hamster. These animals originated in Syria and are naturally found in the arid, temperate southeast Europe and Asia Minor (5). Syrian hamsters currently used as laboratory animals originated from a litter captured in 1930 (5, 6). There is mounting evidence that the Syrian hamster is highly similar to humans in anatomy, physiology, and pathology (7–9). Their similarity is especially valuable in terms of the similarity of pathophysiology, i.e the occurrence, development, symptoms, pathology, and outcomes of disease (10). Syrian hamsters have a short reproductive cycle, are docile, and are easy to raise and handle (8, 11).
It has been reported that the human granulocyte-macrophage colony-stimulating factor (GM-CSF) is immunologically functional in hamsters (12) and recently we reported that human interleukin (IL)-12 was biologically active towards the immune cells of Syrian hamsters (13). The human cytokines IL-21 and IL-2 have also demonstrated immunological activity in hamsters (14, 15). The Syrian hamster macrophage migration inhibitory factor (MIF) is structurally and functionally similar to humans and significantly enhanced tumor growth and promoted tumor-associated angiogenesis in Syrian hamster tumor models (16). An animal model with an intact and functional immune system is indispensable for the development and characterization of cancer immunotherapy efficacy and potential toxicities, therefore, the Syrian hamster is the preferred animal model in many fields of medical research. In this review, we discuss the current landscape of the Syrian hamster tumor models, the promises and challenges of the Syrian hamster as a model for evaluating cancer immunotherapy.
2 Syrian hamster tumor models
2.1 Syngeneic tumor models
Syngeneic tumor models are the most used preclinical models for evaluating cancer immunotherapy and refer to the transplantation of in vitro cultured tumor cell lines into immunocompetent animals (Figure 1A), typically including subcutaneous, intraperitoneal disseminated and orthotopic tumor models. In recent years, Syrian hamsters have been widely used in preclinical research as tumor models. The Syrian hamster model can better simulate the development of human cancer and reproduce the genomic heterogeneity of human cancer and the complexity of its microenvironment (8, 17).
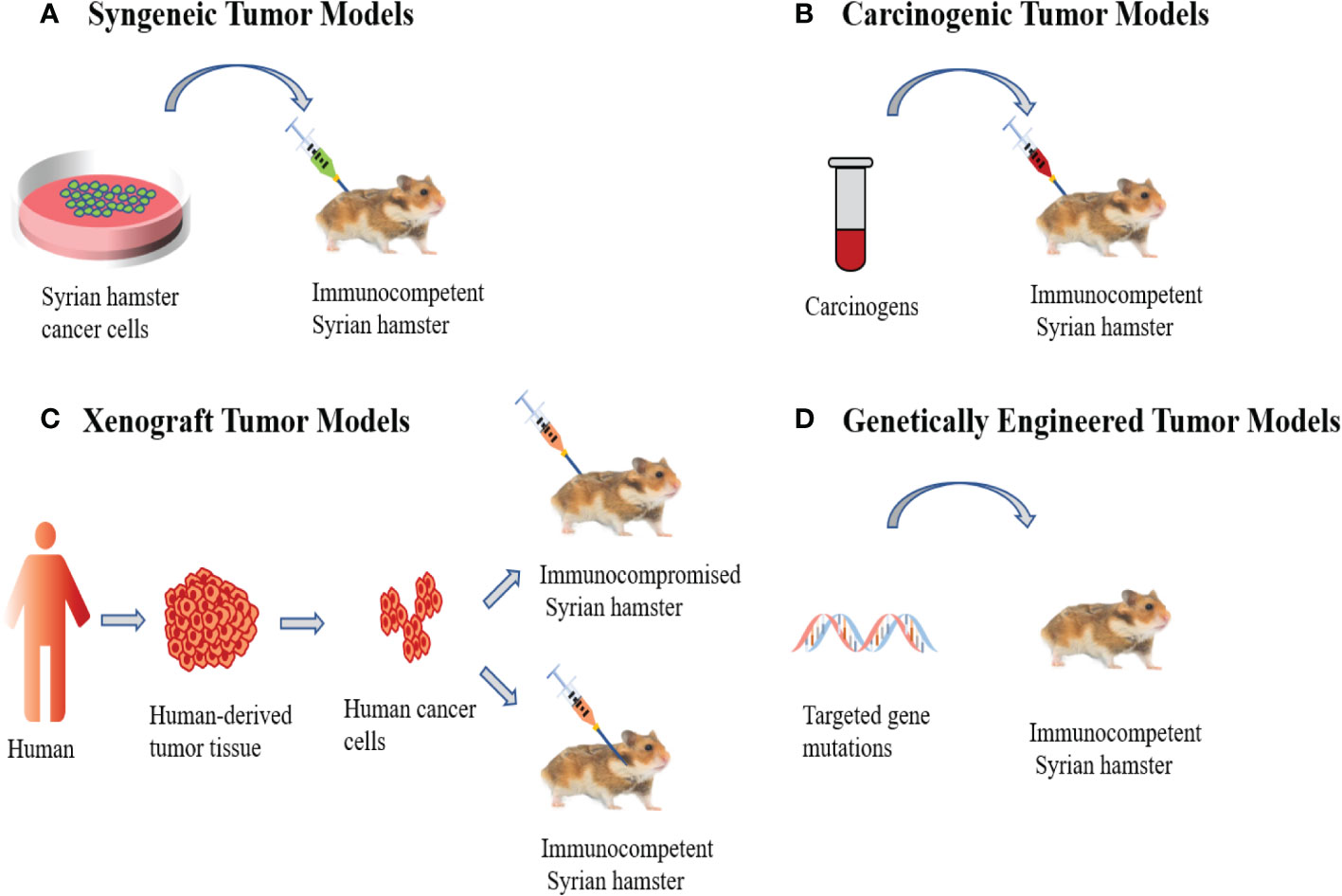
Figure 1 Syrian Hamster Tumor Models. (A) Syngeneic tumor models involve the transplantation of in vitro cultured Syrian hamster cancer cells into immunocompetent Syrian hamsters. (B) Carcinogenic tumor models are induced by carcinogens, which allows tumors to undergo de novo formation. (C) Human xenograft tumor models utilize human cancer cells to inject into immunocompromised Syrian hamsters or the cheek pouches of immunocompetent Syrian hamsters. (D) Genetically engineered models are the application of genetic engineering techniques to modify hamster genes to induce cancer development.
It has been reported that the hamster peritoneal omentum-associated lymphoid tissue is anatomically equivalent to the human milky spots, which are mainly composed of macrophages and are closely related to the spread of cancer cells (18, 19) The Syrian hamster cheek pouch lacks an intact lymphatic drainage pathway and is considered immune privileged, which supports the long-term survival of transplanted foreign tissue without immunological rejection (5). It was previously reported that human myofibrosarcoma (MFS-l) and melanoticmelanoma (ME-l) transplanted into the cheek pouch of Syrian hamsters were fully sensitive to colchicine stimulation throughout growth and that this sensitivity persisted after multiple consecutive transplants, thus providing support for the hamster-human tumor system as a tool to evaluate human antitumor agents (20). The Syrian hamster pancreatic cancer cell line HaP-T1 has been established and exhibits tumorigenicity on syngeneic Syrian hamsters, and histologically showed obvious epithelial characteristics similar to human pancreatic cancer. Further studies have shown that hamster N-terminal Sonic hedgehog (SHH) is 99% identical to human, while N-SHH promotes desmoplasia in human pancreatic cancer (21, 22). Researchers have also established the Syrian hamster pancreatic carcinoma cell line SHPC6, which when injected into peritoneal cavity, forms disseminated tumor nodules and nodules attached to the pancreas, faithfully simulating patients with advanced pancreatic cancer metastasis (23). Additionally, the syngeneic Syrian hamster glioma orthotopic model showed hypercellularity and necrotic areas, consistent with the characteristics of human glioma (24). At present, the Syrian hamster syngeneic tumor models used for research include pancreatic cancer (25–27), oral cancer (28), gallbladder cancer (29), glioma (24), kidney cancer (23, 30), liver cancer (23), lung cancer (23) and breast cancer (31). However, the challenge with all syngeneic tumor models is that they cannot reflect the multi-stage process of carcinogenesis due to the direct engraftment of malignant cells, therefore, these models are not suitable for the evaluation of the application of anti-tumor immunotherapy in the early stages of tumorigenesis (32).
2.2 Carcinogenic tumor models
Oncogenic tumor models are primarily caused by carcinogens (Figure 1B). The instability of the genome allows tumors to undergo de novo formation in the microenvironment. This produces great genomic complexity and accurately reflects the occurrence and development of tumors in humans (33). It has been reported that N-nitroso-bis(2-oxopropyl) amine (BOP) can induce pancreatic ductal adenocarcinoma in Syrian hamsters and its histology, morphology, biology, pathology, and genetics are similar to humans. For example, its development from ductal cells, local invasion, distant metastasis, cachexia, and point mutations in the K-ras gene (34–37). In addition, studies have shown that the Syrian hamster cholangiocarcinoma model induced by the combination of Clonorchis Sinensis infection and dimethyl nitrosamine resulted in chronic inflammation, bile duct injury and atypical bile duct hyperplasia, with progression to cholangiocarcinoma, histopathologically similar to human liver fluke infection-associated cholangiocarcinoma (38–40). The chemically induced Syrian hamster cheek pouch carcinogenesis model can accurately reflect a series of common processes seen in human oral precancerous lesions and carcinogenesis, including mutations and expression changes of oncogenes and tumor suppressor genes. Histologically, the cells showed varying degrees of atypia and differentiation, similar to those seen in human squamous cell carcinoma (41–46). Researchers used 9,10-dimethyl-1,2-benzthracene (DMBA) to induce the formation of cheek pocket squamous cell carcinoma in Syrian hamsters and established the Syrian hamster cheek pocket squamous cell carcinoma cell line. Subsequently, the cell lines were transplanted into the subcutaneous and cheek pouch of hamsters and treated with the oncolytic adenovirus HAdV5 exhibiting significant antitumor effects (28). In addition, it has been reported that chemical carcinogens could induce lung cancer in Syrian hamsters (47–52), and the N-nitrosobis (2-oxopropyl) amine (BOP) induced Syrian hamster lung cancer model faithfully mimics human small cell and non-small cell lung cancer (NSCLC) (47, 51, 52). Histopathologically, it shows a subtype of adenocarcinoma, which is very similar to the type of adenocarcinoma of human non-small cell lung cancer (NSCLC), and the common feature with human lung cancer is that tumor tissues overexpress cyclooxygenase-2 (COX-2) and nuclear factor kappa B (NF-kappaB), which are important factors in the pathogenesis of lung cancer (44). Compared with mice and rats, the streptozotocin-induced Syrian hamster hepatocellular carcinoma tumor model has the advantage of high incidence and short latency, making it an ideal animal model for assessing hepatocarcinogenesis (53). Researchers have also used N-methyl-N-nitrosourea (MNU) to induce hamster breast cancer, and histopathological analysis showed that this animal model resembled human high-grade poorly differentiated breast adenocarcinoma. At the same time, they established the first immortalization of a Syrian hamster breast cancer cell line HMAM5, with a high tumorigenic rate in female hamsters (31). Additional carcinogen-induced cancers in Syrian hamsters include kidney cancer (51, 54, 55), cholangiocellular tumors (52), endometrial adenocarcinoma (56) and laryngeal cancer (57).
2.3 Xenograft tumor models
Human xenograft tumor models are established by transplantation of human-derived tumor cell lines or tumor tissues into immunocompromised Syrian hamsters (Figure 1C). Recently, we created a new pancreatic cancer xenograft model using IL2RG gene knockout Syrian hamsters (named ZZU001) with IL-2, IL-4, IL-7, IL-9, IL-15, and IL- 21 receptor function deficiencies, resulting in a significantly decrease in T lymphocyte activity and non-functional B and NK cells, producing a severely immunocompromised state (8, 11). Subcutaneous and orthotopic transplantation of a panel of human pancreatic cancer (PC) cell lines into ZZU001 Syrian hamsters and immunodeficient mice demonstrated that only in ZZU001 did multi-organ metastases similar to those seen in human pancreatic cancer develop. PC tissue from Syrian hamsters exhibited desmoplastic reactions in both stromal and epithelial-to-mesenchymal transition phenotypes, whereas PC tissues from immunodeficient mice did not show this feature. In addition, we transplanted an established human esophageal cancer (EC) cell line (SBRC-EC01) into immunodeficient ZZU001 Syrian hamsters, resulting in a subcutaneous tumor with a pathological structure similar to the primary tumor, but SBRC-EC01 has no tumorigenicity in B-NDG mice and BALB/c nude mice. This suggests the Syrian hamster is a very valuable animal model for studying treatment responses in human PC and EC (8).
Most commonly, human tumor models are established by subcutaneous inoculation of human tumor cells into immunodeficient mice, however, in these models, the histological features of the primary tumor are not reflected (17) The cheek pouches of hamsters possess immune-privileged sites that allow human tumor transplantation to form a distinctive xenograft tumor model. Sakakibara et al. (58) showed that when human malignant tumor tissue-derived cell lines were transplanted into the cheek pouches of Syrian hamsters, the histology of these tumors was consistent with the primary tumor. This was not reflected when the same model was established in mice (59). In addition, serial transplantation of human lymphomas in neonatal hamsters has also been reported, a model that may be a practical system for the study of immunotherapeutic agents, however, no similar reports have been reported in neonatal mice (60, 61). The establishment of human osteosarcoma model in newborn Syrian hamsters has also been reported (62). In conclusion, although xenograft mouse models have become common models for evaluating tumor progression and the efficacy of cancer drugs, they do not faithfully reflect human tumor progression. However, the immune-deficient Syrian hamster model ZZU001 can be used to better simulate human tumors and show the histological and pathological characteristics of human tumors.
2.4 Genetically engineered tumor models
Genetically engineered models involve the application of genetic engineering techniques to modify hamster genes to induce cancer development (Figure 1D). Currently, hamster transgenic models mainly include KCNQ1 knockout, TP53 knockout and IL2RG knockout as discussed above (8, 63, 64). KCNQ1 is a gene encoding a potassium channel that is widely expressed in human and rodent tissues, and KCNQ1 functions as a tumor suppressor gene in the gastrointestinal tract of both humans and mice (65). Li et al. created the first genetically engineered KCNQ1 knockout hamster cancer model by CRISPR/Cas9 genetic engineering technology, and the homozygous KCNQ1 knockout hamsters showed severe physical discomfort response at day 70. 85.7% of the hamsters had visible cancers at necropsy, including T-cell lymphoma, plasma cell tumors, hemangiosarcomas, and suspected myeloid leukemia, and developed multi-organ infiltrates (63). In addition, Miao et al. used CRISPR/Cas9 genetic engineering technology to generate TP53 knockout hamsters. In TP53 homozygous mutant hamsters, lymphomas, hemangiosarcomas, and myeloid leukemias were the predominant types of cancer developed, while TP53 heterozygous hamsters mainly developed lymphomas (64). Of note, in contrast with TP53 homozygous KO mice, hamsters developed carcinomas in several epithelial tissues that were not observed in TP53 KO mice. TP53-deficient acute myeloid leukemia (AML) in human is highly malignant, has a low response rate to chemotherapy, and has a mediate overall survival of only 5-10 months (66), so there is an urgent need for effective animal models to develop new treatments. Most importantly, TP53-deficient hamsters can develop acute myelogenous leukemia, however, TP53-deficient mice generally do not develop acute myelogenous leukemia (64). Thus, hamsters can be used to evaluate new therapeutic modalities for TP53-deficient acute myeloid leukemia. Recently, Miao et al. established a novel SHARPIN knockout (KO) Syrian hamster using the CRISPR/Cas9 system, and eosinophil infiltration in esophagus and other organs was observed in SHARPIN KO transgenic hamsters, which may represent early symptoms of human eosinophilic esophagitis (EoE). Therefore, it is expected that SHARPIN KO transgenic hamsters will encapsulate the syndrome of human autoinflammatory diseases (67).
3 Cancer immunotherapy in the Syrian hamster model
Cancer Immunotherapy (CIT) was the breakthrough of the year 2013 (68). More than a dozen immunotherapies have been approved for cancer treatment to date, however, there are many more immunotherapies in preclinical trials and there is an urgent need for suitable preclinical models to effectively assess their suitability. CIT methods currently evaluated in Syrian hamsters include immune checkpoint inhibitors (14, 69), cytokines (13, 70–72), adoptive cell therapy (15, 60, 73), cancer vaccines (19, 74) and oncolytic viruses (14, 26, 29, 75). A summary of Syrian hamster models used for evaluation of CIT is presented in Table 1.
3.1 Immune checkpoint inhibitors
Immune checkpoint inhibitors (ICI) are by far the most thoroughly studied and clinically advanced form of immunotherapy. PD-1/PD-L1 and CTLA-4 are the two most common immune checkpoint inhibitor targets and several ICI drugs have been approved by the FDA for cancer treatment. Although ICI produces more durable responses than conventional chemoradiation, response rates remain low in many cancers (3, 96), a situation that may be improved by the application of more suitable animal models during pre-clinical testing of these agents.
It has been reported that in a Syrian hamster PC subcutaneous tumor model, αPD-1 (anti-mouse) combined with oncolytic adenovirus (AdV) co-expressing IL-12, GM-CSF, and RLX (Relaxin), which can inhibit the synthesis of extracellular matrix (ECM) and degrade the overexpressed ECM in tumor tissue, to allow full dispersion of the therapeutics,exhibited stronger tumor inhibition compared with the αPD-1 group (69). In the Syrian hamster orthotopic PC model, αPD-1 combined with oncolytic AdV significantly inhibited the growth of primary and metastatic tumors without ascites formation, whereas the αPD-1 group exhibits extensive tumor metastasis and ascites formation. Further studies showed that application of oncolytic Ad/IL12/GM-RLX enhanced penetration of α-PD1 into tumor tissues, and enhanced T cell activation and infiltration into tumors (69). Both the Syrian hamster orthotopic and intraperitoneal dissemination models can faithfully mimic the characteristics of human PC and like human PC, the Syrian hamster PC models are insensitive to ICI. However, combination therapy sensitized PC to αPD-1 treatment and enhanced antitumor effects.
3.2 Cytokines
Cytokines were the first type of immunotherapy to be used in the clinic and have a history of over 40 years of clinical use. IFNα and IL-2 have been approved for the treatment of malignant tumors (97, 98). Cytokines are key mediators of cell-to-cell communication in the tumor microenvironment (TME) and dysregulation of cytokine expression is present in all human cancers (99). Cytokine therapy refers to the direct stimulation of immune cell growth and activity by injected cytokines. Former commonly used cytokines treatments involved mono-therapeutic use of interferons, interleukins, or granulocyte-macrophage colony-stimulating factor (GM-CSF) (3). However, studies have shown that, except for a few cancers, such as melanoma and renal cell carcinoma, single cytokine therapy in clinical trials is not satisfactory (99) and testing of multiple agents in suitable pre-clinical models is necessary for future application of cytokine therapy.
Interestingly, it appears that the similarities between hamster and human disease pathology extend to the immune system and many human cytokines are functional in the Syrian hamster. Parviainen et al. (70) reported that human GM-CSF has immunological functions in immunocompetent Syrian hamsters. In the Syrian hamster PC model, oncolytic Vaccinia virus expressing human GM-CSF can enhance specific anti-tumor immune responses by recruitment and activation of natural killer cells, monocytes, and granulocytes to enhance the anti-tumor effect. However, human GM-CSF is not functional in mice. We reported that human IL-12 stimulated the proliferation of hamster, but not murine peripheral blood mononuclear cells and induced the expression of interferon-γ and tumor necrosis factor-alpha. At the same time, we constructed an oncolytic AdV expressing non-secreting IL-12 to achieve local low-dose release of IL-12, solving the problems of short half-life and high systemic toxicity associated with IL-12. Using the Syrian hamster PC peritoneal dissemination model and orthotopic tumor models, we demonstrated a good anti-tumor effect and prolonged survival with no toxic side effects observed (13). We also demonstrated that the oncolytic Vaccinia virus (VV) expressing human IL-21 prolongs survival in an intraperitoneal dissemination hamster model of pancreatic cancer (14). Joao Manuel Santos et al. (73) demonstrated that oncolytic AdV expressing human IL-2 achieved the local release of IL-2 in hamster pancreatic cancer, improved the efficacy of adoptive cell therapy, and significantly enhanced the infiltration of CD8+ T cells into the tumor microenvironment, while avoiding systemic toxicity caused by a intravenous delivery of IL-2. In addition, it was reported that human IFN-α is also immunologically functional in hamsters and prolongs survival in a hamster lymphosarcoma model (71, 72). Thus, many human cytokines are biologically active in Syrian hamsters, suggesting this model as a highly suitable immunocompetent model for direct assessment of the role of human cytokines in antitumor activity. This model will also provide robust toxicity information regarding the side-effects of cytokine treatment that can only be determined in mice by use of the murine cytokine counterparts.
3.3 Adoptive cell therapy
Adoptive cell therapy (ACT) mainly utilizes tumor-infiltrating lymphocyte (TIL)-derived T cells or genetically engineered T cells to express tumor-recognition receptors, resulting in durable anti-tumor responses (100). TIL-based ACT relies on (i) non-myeloablative lymphoid exhaustion, (ii) tumor-specific T cells isolated from tumor tissues, expanded in vitro, and injected into the host, and (iii) IL-2 administration after TIL infusion (100). Currently, TIL therapy has a durable antitumor effect in melanoma patients (101), but to make TIL therapy widely available, many researchers have improved the efficacy and safety of ACT through combination therapy strategies.
Mikko Siurala et al. (60) reported for the first time that tumor-infiltrating lymphocytes (TILs) from Syrian hamsters combined with oncolytic AdV enhanced tumor cell killing ability in vitro, and oncolytic adenovirus enhanced the efficacy of TIL therapy. However, systemic administration of high doses of IL-2 can lead to severe systemic toxicity and even death (73). It has been reported that using ACT to treat hamster PC, the local high-dose release of IL-2 achieved by co-treatment with Ad5/3-E2F-d24-hIL2 avoided toxicity associated with systemic administration. More interestingly, Ad5/3-E2F-d24-hIL2 increased CD8+ T cells in the tumor microenvironment compared with systemic IL-2 administration. Therefore, Syrian hamster models have demonstrated that in ACT therapy, local release of IL-2 can effectively replace systemic administration and reduce toxicity associated with this form of therapy (73). The Syrian hamster is crucial for this modelling as they not only have immune responses that are reflective of the human scenario and support human IL-2 functions, but critically hamster models are supportive of AdV replication, a feature not shared by murine models. The first step in ACT therapy is host lymphoid depletion, but this can lead to severe cytopenia, systemic inflammatory responses, and damage to vital organs (15, 100). In order to overcome this problem, João Manuel Santos et al. (15) used a hamster PC model to demonstrate that oncolytic AdV armed with human IL-2 and human tumor necrosis factor alpha (TNF-α) could achieve antitumor effects without lymphoid depletion. Indeed, compared with lymphoid depletion, hamsters receiving oncolytic adenovirus and TILs had a significantly longer survival time and repeated AdVtreatment resulted in durable antitumor effects. The mechanism for this is postulated as dependent on AdV-induced upregulation of major histocompatibility complex (MHC) class II in draining lymph nodes, which effectively stimulates CD4+ T cells. These data suggest that the Syrian hamster provides a reliable preclinical animal model for ACT, accurately modeling the toxicity of treatments with human cytokines and that they are critical models for evaluating the ACT combination with oncolytic AdV therapy. Data obtained from Syrian hamster models provide more relevant and reliable evidence for the clinical translation of this therapeutic strategy.
3.4 Therapeutic cancer vaccines
Therapeutic cancer vaccines are designed to induce tumor regression and eradicate cancer cells by boosting a patient’s immune response and developing durable antitumor memory immune responses (102). Dendritic cells (DCs) are the most functional antigen-presenting cells (APCs) in the body and play a key role in coordinating the innate and adaptive immune responses against the tumor (103). In 2010, the first therapeutic cancer vaccine (SiPuleucel-T) was approved by the FDA, which improved overall survival for patients with refractory prostate cancer (104, 105).
To understand the potential for a DC vaccine in hamster PC, Akiyama et al. (74) reported that hamster bone marrow (BM)-derived DC (BM-DC) stimulated with hamster tumor cell lysate had an obvious anti-tumor effect on hamster subcutaneous PC. Further studies showed that tumor lysate-pulsed hamster DC could enhance activity of tumor-specific cytotoxic T lymphocytes (CTL). Akiyama et al. (19) also reported that in the hamster PC intraperitoneal dissemination tumor model, tumor lysate-treated DCs significantly inhibited the growth of orthotopic tumor cells and the formation of ascites. Further studies showed that the antitumor effect of tumor lysate-pulsed DCs was mainly due to the activation and expansion of tumor-specific CTLs. Given the lack of success in development of successful therapeutic cancer vaccines to date, the Syrian hamster tumor model should be considered for the development and testing of these regimes as it can better demonstrate the complexity of human cancer and thus has many advantages over mouse models.
3.5 Oncolytic virus therapy
Oncolytic viral therapy is a promising strategy for CIT. Naturally tumor-tropic viruses, or viruses genetically modified for tumor tropism are used to generate anti-tumor activity. The mechanisms by with oncolytic viruses achieve this are (i) selective replication in and killing of tumor cells, (ii) release of tumor-related antigens to induce a systemic anti-tumor immune response, and (iii) induction of potent anti-tumor immune responses via infiltration and activation of innate and adaptive immune cells (106). While the prospects for oncolytic viral therapy are positive, with 4 licensed treatments to date, animal models to evaluate these vectors have significant limitations. Oncolytic AdV was the first approved oncolytic virus, licensed in 2005 in China for treatment of refractory head and neck cancer. Since then, AdV has been intensively studied as an oncolytic agent as their genome and biology are well understood, safe, and easily manipulated. However, replication of AdV is species-selective and immunocompetent mouse models are not supportive of AdV replication. Therefore, the evaluation of oncolytic AdV vectors is often performed in immunodeficient mice with human xenograft tumors that do support virus replication. This limits the useful information that is produced through in vivo experimentation, as these models cannot adequately assess the safety of viral vectors and their role in host immune responses. Furthermore, as human AdV replicates poorly in normal and tumor tissues of mice, off target effects are difficult to determine (19, 77, 107).
The Syrian hamster is both immunocompetent and allows human AdV replication in normal and tumor tissues, and many research papers have detailed the usefulness of Syrian hamster models for investigation on oncolytic AdV (9, 13, 23–26, 28, 76–80, 82, 84–87, 90–95, 108–111). Phillips et al. (24) established the first immunocompetent Syrian hamster glioma model that is oncolytic AdV replication-permissive, which is novel platform for studying the interactions between the host immune system, tumors, and oncolytic adenoviral therapy. Further studies showed that T-cell infiltration in the tumor microenvironment was significantly increased after treatment with the oncolytic AdV Delta-24-RGD and significant prolongation of survival was achieved compared to the control group (24). Moreover, it was reported that in the Syrian hamster xenograft human esophageal tumor model, AdV-TD-nsIL12 showed strong anti-tumor effects and inhibited tumor cell proliferation and reduced microvascular density in tumor tissue (17). In addition to its advantages for testing oncolytic AdV, the immune response elicited following VV virus infection in Syrian hamsters has been shown to be partly mediated by natural killer cells in a similar manner to human infection, and this phenomenon is not seen in mice (112), suggesting the Syrian hamster as a suitable model for assessing the immune competence of the oncolytic VV (8, 9, 14, 70, 83, 88). The immune-competent Syrian hamster has also been used to evaluate oncolytic Herpes Simplex Virus (HSV) (29, 89), Reovirus (75), and Newcastle disease virus (NDV) (26), thus providing reliable preclinical studies for the development of oncolytic virus drugs. Nakano et al. (29) demonstrated that the antitumor ability of HSV G207 in athymic-free mice was significantly lower than that in immunocompetent Syrian hamsters, indicating that T-cell-mediated immune response played an important role in the local and systemic anti-tumor effects of G207. This demonstrates the necessity of using animal models with intact immune responses to evaluate oncolytic virus therapy. Interestingly, a novel therapeutic regime to eradicate established tumors by sequential use of two different oncolytic viruses was demonstrated in immunocompetent Syrian hamster PC (HPD-1NR) and kidney cancer (HaK) models (9). Sequential administration of low-dose oncolytic adenovirus and Vaccinia virus exhibited stronger antitumor effects compared to the reverse combination or single agent therapy. Further studies showed that AdV-VV treatment increased tumor-infiltrating lymphocytes and induced a higher tumor-specific immune response (9). Therefore, the immunocompetent Syrian hamster is an appropriate animal model to evaluate the combined treatment strategy of oncolytic AdV and VV as well as other oncolytic viruses.
4 Conclusions and prospects
The number of cancer immunotherapy drugs approved by the FDA has been increasing and cancer immunotherapy is an exciting new paradigm in cancer therapy. An ideal preclinical animal model is urgently needed to evaluate cancer immunotherapy. The US Food and Drug Administration indicates a suitable model is similar to humans in disease onset, progression, symptoms, pathology, and pathophysiology. The Syrian hamster complies with this requirement and immunocompetent hosts can accurately mimic the human scenario in terms of immune responses to disease states and pathogens. Indeed, we and others have recently reported its relevance over mouse models for modelling of SARS-CoV2 infection and disease (113). The Syrian hamster tumor model is more representative of the occurrence and development of human tumors and faithfully recapitulates the complexity of the tumor microenvironment (23, 41). Many human cytokines, including GM-CSF, IL-21, and IL-12, have immunological functions in the hamster (13, 14, 70) and this model is also favored for the examination of species-selective oncolytic viruses such as AdV, which replicate poorly in immunocompetent mice (88).
Challenges do remain with the use of the Syrian hamster model. For example, there are fewer syngeneic tumor cell lines and genetically engineered cancer models available compared with mice and a lack of specific immune reagents can hamper in-depth investigation of immune responses and cytokine changes in the TME after CIT. Moreover, there is currently no humanized Syrian hamster model. We and others are working hard to overcome these constraints, building banks of Syrian hamster tumor cell lines, transgenic hamster models, and developing research reagents to assess immune functions accurately. Thus, we believe that soon, the Syrian hamster will become a widely-used preclinical animal model that accurately simulates various human disease states. This will allow a more accelerated, refined analysis of CIT that can be confidently applied to clinical practice in a more timely manner.
Author contributions
Conceptualization, YJ and YHW. Writing, original draft preparation, YJ and YRW. Writing, review and editing, NL, LD and YHW. Supervision, YHW and PW. Project administration, YHW. Funding acquisition, YHW and PW. All authors contributed to the article and approved the submitted version.
Funding
This project was supported by the National Natural Science Foundation of China (81872486), the National Key R&D program of China (2019YFC1316101), the Natural Science Foundation of Henan Province for Excellent Young Scholars (202300410360) and the UK Medical Research Council (MR/V006053/1) to LD and YHW.
Conflict of interest
The authors declare that the research was conducted in the absence of any commercial or financial relationships that could be construed as a potential conflict of interest.
Publisher’s note
All claims expressed in this article are solely those of the authors and do not necessarily represent those of their affiliated organizations, or those of the publisher, the editors and the reviewers. Any product that may be evaluated in this article, or claim that may be made by its manufacturer, is not guaranteed or endorsed by the publisher.
References
1. Siegel RL, Miller KD, Fuchs HE, Jemal A. Cancer statistics, 2021. CA: Cancer J Clin (2021) 71(1):7–33. doi: 10.3322/caac.21654
2. Sung H, Ferlay J, Siegel RL, Laversanne M, Soerjomataram I, Jemal A, et al. Global cancer statistics 2020: Globocan estimates of incidence and mortality worldwide for 36 cancers in 185 countries. CA: Cancer J Clin (2021) 71(3):209–49. doi: 10.3322/caac.21660
3. Riley RS, June CH, Langer R, Mitchell MJ. Delivery technologies for cancer immunotherapy. Nat Rev Drug Discovery (2019) 18(3):175–96. doi: 10.1038/s41573-018-0006-z
4. Hegde PS, Chen DS. Top 10 challenges in cancer immunotherapy. Immunity (2020) 52(1):17–35. doi: 10.1016/j.immuni.2019.12.011
5. Miedel EL, Hankenson FC. Biology and diseases of hamsters: Laboratory animal medicine. (2015), 209–45. doi: 10.1016/B978-0-12-409527-4.00005-5
6. Mohr U. The Syrian golden hamster as a model in cancer research. Prog Exp tumor Res (1979) 24:245–52. doi: 10.1159/000402101
7. Miao J, Ying B, Li R, Tollefson AE, Spencer JF, Wold WSM, et al. Characterization of an n-terminal non-core domain of Rag1 gene disrupted Syrian hamster model generated by crispr Cas9. Viruses (2018) 10(5):243. doi: 10.3390/v10050243
8. Miao JX, Wang JY, Li HZ, Guo HR, Dunmall LSC, Zhang ZX, et al. Promising xenograft animal model recapitulating the features of human pancreatic cancer. World J Gastroenterol (2020) 26(32):4802–16. doi: 10.3748/wjg.v26.i32.4802
9. Tysome JR, Li X, Wang S, Wang P, Gao D, Du P, et al. A novel therapeutic regimen to eradicate established solid tumors with an effective induction of tumor-specific immunity. Clin Cancer Res an Off J Am Assoc Cancer Res (2012) 18(24):6679–89. doi: 10.1158/1078-0432.Ccr-12-0979
10. Warner BM, Safronetz D, Kobinger GP. Syrian Hamsters as a small animal model for emerging infectious diseases: Advances in immunologic methods. Adv Exp Med Biol (2017) 972:87–101. doi: 10.1007/5584_2016_135
11. Li R, Ying B, Liu Y, Spencer JF, Miao J, Tollefson AE, et al. Generation and characterization of an I L2rg knockout Syrian hamster model for xscid and hadv-C6 infection in immunocompromised patients. Dis Models Mech (2020) 13(8):dmm044602. doi: 10.1242/dmm.044602
12. Cho SA, Park JH, Seok SH, Juhn JH, Kim SJ, Ji HJ, et al. Effect of granulocyte macrophage-colony stimulating factor (Gm-csf) on 5-Fu-Induced ulcerative mucositis in hamster buccal pouches. Exp toxicologic Pathol Off J Gesellschaft fur Toxikologische Pathologie (2006) 57(4):321–8. doi: 10.1016/j.etp.2005.09.006
13. Wang P, Li X, Wang J, Gao D, Li Y, Li H, et al. Re-designing interleukin-12 to enhance its safety and potential as an anti-tumor immunotherapeutic agent. Nat Commun (2017) 8(1):1395. doi: 10.1038/s41467-017-01385-8
14. Marelli G, Chard Dunmall LS, Yuan M, Di Gioia C, Miao J, Cheng Z, et al. A systemically deliverable vaccinia virus with increased capacity for intertumoral and intratumoral spread effectively treats pancreatic cancer. J immunotherapy Cancer (2021) 9(1):e001624. doi: 10.1136/jitc-2020-001624
15. Santos JM, Cervera-Carrascon V, Havunen R, Zafar S, Siurala M, Sorsa S, et al. Adenovirus coding for interleukin-2 and tumor necrosis factor alpha replaces lymphodepleting chemotherapy in adoptive T cell therapy. Mol Ther J Am Soc Gene Ther (2018) 26(9):2243–54. doi: 10.1016/j.ymthe.2018.06.001
16. Suresh V, Sundaram R, Dash P, Sabat SC, Mohapatra D, Mohanty S, et al. Macrophage migration inhibitory factor of Syrian golden hamster shares structural and functional similarity with human counterpart and promotes pancreatic cancer. Sci Rep (2019) 9(1):15507. doi: 10.1038/s41598-019-51947-7
17. Zhang Z, Zhang C, Miao J, Wang Z, Wang Z, Cheng Z, et al. A tumor-targeted replicating oncolytic adenovirus ad-Td-Nsil12 as a promising therapeutic agent for human esophageal squamous cell carcinoma. Cells (2020) 9(11):2438. doi: 10.3390/cells9112438
18. Shimotsuma M, Shields JW, Simpson-Morgan MW, Sakuyama A, Shirasu M, Hagiwara A, et al. Morpho-physiological function and role of omental milky spots as omentum-associated lymphoid tissue (Oalt) in the peritoneal cavity. Lymphology (1993) 26(2):90–101.
19. Takigawa Y, Akiyama Y, Maruyama K, Sugiyama K, Uchida E, Kosuge T, et al. Antitumor effect induced by dendritic cell (Dc)-based immunotherapy against peritoneal dissemination of the hamster pancreatic cancer. Cancer Lett (2004) 215(2):179–86. doi: 10.1016/j.canlet.2004.07.007
20. Cardinalli G, Cardinali G, Handler AH. Effect of colchicine on human tumours transplanted in cheek pouch of Syrian hamster. Nature (1964) 203:90–2. doi: 10.1038/203090b0
21. Saito S, Nishimura N, Kubota Y, Yamazaki K, Shibuya T, Sasaki H. Establishment and characterization of a cultured cell line derived from nitrosamine-induced pancreatic ductal adenocarcinoma in Syrian golden hamsters. Gastroenterologia Japonica (1988) 23(2):183–94. doi: 10.1007/bf02799031
22. Suklabaidya S, Das B, Ali SA, Jain S, Swaminathan S, Mohanty AK, et al. Characterization and use of Hapt1-derived homologous tumors as a preclinical model to evaluate therapeutic efficacy of drugs against pancreatic tumor desmoplasia. Oncotarget (2016) 7(27):41825–42. doi: 10.18632/oncotarget.9729
23. Spencer JF, Sagartz JE, Wold WS, Toth K. New pancreatic carcinoma model for studying oncolytic adenoviruses in the permissive Syrian hamster. Cancer Gene Ther (2009) 16(12):912–22. doi: 10.1038/cgt.2009.36
24. Phillips LM, Li S, Gumin J, Daou M, Ledbetter D, Yang J, et al. An immune-competent, replication-permissive Syrian hamster glioma model for evaluating delta-24-Rgd oncolytic adenovirus. Neuro-oncology (2021) 23(11):1911–21. doi: 10.1093/neuonc/noab128
25. LaRocca CJ, Han J, Gavrikova T, Armstrong L, Oliveira AR, Shanley R, et al. Oncolytic adenovirus expressing interferon alpha in a syngeneic Syrian hamster model for the treatment of pancreatic cancer. Surgery (2015) 157(5):888–98. doi: 10.1016/j.surg.2015.01.006
26. Nistal-Villan E, Bunuales M, Poutou J, Gonzalez-Aparicio M, Bravo-Perez C, Quetglas JI, et al. Enhanced therapeutic effect using sequential administration of antigenically distinct oncolytic viruses expressing oncostatin m in a Syrian hamster orthotopic pancreatic cancer model. Mol Cancer (2015) 14:210. doi: 10.1186/s12943-015-0479-x
27. Hara H, Kobayashi A, Yoshida K, Ohashi M, Ohnami S, Uchida E, et al. Local interferon-alpha gene therapy elicits systemic immunity in a syngeneic pancreatic cancer model in hamster. Cancer Sci (2007) 98(3):455–63. doi: 10.1111/j.1349-7006.2007.00408.x
28. Vijayalingam S, Kuppusamy M, Subramanian T, Strebeck FF, West CL, Varvares M, et al. Evaluation of apoptogenic adenovirus type 5 oncolytic vectors in a Syrian hamster head and neck cancer model. Cancer Gene Ther (2014) 21(6):228–37. doi: 10.1038/cgt.2014.22
29. Nakano K, Todo T, Chijiiwa K, Tanaka M. Therapeutic efficacy of G207, a conditionally replicating herpes simplex virus type 1 mutant, for gallbladder carcinoma in immunocompetent hamsters. Mol Ther J Am Soc Gene Ther (2001) 3(4):431–7. doi: 10.1006/mthe.2001.0303
30. Shashkova EV, Spencer JF, Wold WS, Doronin K. Targeting interferon-alpha increases antitumor efficacy and reduces hepatotoxicity of E1a-mutated spread-enhanced oncolytic adenovirus. Mol Ther J Am Soc Gene Ther (2007) 15(3):598–607. doi: 10.1038/sj.mt.6300064
31. Coburn MA, Brueggemann S, Bhatia S, Cheng B, Li BD, Li XL, et al. Establishment of a mammary carcinoma cell line from Syrian hamsters treated with n-Methyl-N-Nitrosourea. Cancer Lett (2011) 312(1):82–90. doi: 10.1016/j.canlet.2011.08.003
32. Greenberg NM, DeMayo F, Finegold MJ, Medina D, Tilley WD, Aspinall JO, et al. Prostate cancer in a transgenic mouse. Proc Natl Acad Sci United States America (1995) 92(8):3439–43. doi: 10.1073/pnas.92.8.3439
33. Hanahan D, Weinberg RA. Hallmarks of cancer: The next generation. Cell (2011) 144(5):646–74. doi: 10.1016/j.cell.2011.02.013
34. Pour P, Krüger FW, Althoff J, Cardesa A, Mohr U. Cancer of the pancreas induced in the Syrian golden hamster. Am J Pathol (1974) 76(2):349–58.
35. Li J, Qin D, Knobloch TJ, Tsai MD, Weghorst CM, Melvin WS, et al. Expression and characterization of Syrian golden hamster P16, a homologue of human tumor suppressor P16 Ink4a. Biochem Biophys Res Commun (2003) 304(2):241–7. doi: 10.1016/s0006-291x(03)00577-1
36. Tsutsumi M, Kondoh S, Noguchi O, Horiguchi K, Kobayashi E, Okita S, et al. K-Ras gene mutation in early ductal lesions induced in a rapid production model for pancreatic carcinomas in Syrian hamsters. Japanese J Cancer Res Gann (1993) 84(11):1101–5. doi: 10.1111/j.1349-7006.1993.tb02807.x
37. Takahashi M, Hori M, Mutoh M, Wakabayashi K, Nakagama H. Experimental animal models of pancreatic carcinogenesis for prevention studies and their relevance to human disease. Cancers (2011) 3(1):582–602. doi: 10.3390/cancers3010582
38. Lee JH, Rim HJ, Sell S. Heterogeneity of the "Oval-cell" response in the hamster liver during cholangiocarcinogenesis following clonorchis sinensis infection and dimethylnitrosamine treatment. J Hepatol (1997) 26(6):1313–23. doi: 10.1016/s0168-8278(97)80467-9
39. Pinlaor S, Hiraku Y, Ma N, Yongvanit P, Semba R, Oikawa S, et al. Mechanism of no-mediated oxidative and nitrative DNA damage in hamsters infected with opisthorchis viverrini: A model of inflammation-mediated carcinogenesis. Nitric Oxide Biol Chem (2004) 11(2):175–83. doi: 10.1016/j.niox.2004.08.004
40. Yoon BI, Kim YH, Yi JY, Kang MS, Jang JJ, Joo KH, et al. Expression of thioredoxin during progression of hamster and human cholangiocarcinoma. Cancer Sci (2010) 101(1):281–8. doi: 10.1111/j.1349-7006.2009.01353.x
41. Vairaktaris E, Spyridonidou S, Papakosta V, Vylliotis A, Lazaris A, Perrea D, et al. The hamster model of sequential oral oncogenesis. Oral Oncol (2008) 44(4):315–24. doi: 10.1016/j.oraloncology.2007.08.015
42. Gimenez-Conti IB, Slaga TJ. The hamster cheek pouch carcinogenesis model. J Cell Biochem Supplement (1993) 17f:83–90. doi: 10.1002/jcb.240531012
43. Chang KW, Sarraj S, Lin SC, Tsai PI, Solt D. P53 expression, P53 and ha-ras mutation and telomerase activation during nitrosamine-mediated hamster pouch carcinogenesis. Carcinogenesis (2000) 21(7):1441–51. doi: 10.1093/carcin/21.7.1441
44. Sumida T, Hamakawa H, Sogawa K, Bao Y, Zen H, Sugita A, et al. Telomerase activation and cell proliferation during 7,12-Dimethylbenz[a]Anthracene-Induced hamster cheek pouch carcinogenesis. Mol carcinogenesis (1999) 25(3):164–8. doi: 10.1002/(sici)1098-2744(199907)25:3<164::aid-mc2>3.0.co;2-5
45. Chen YK, Huse SS, Lin LM. Differential expression of P53, P63 and P73 protein and mrna for dmba-induced hamster buccal-pouch squamous-cell carcinomas. Int J Exp Pathol (2004) 85(2):97–104. doi: 10.1111/j.0959-9673.2004.0374.x
46. Monti-Hughes A, Aromando RF, Pérez MA, Schwint AE, Itoiz ME. The hamster cheek pouch model for field cancerization studies. Periodontology 2000 (2015) 67(1):292–311. doi: 10.1111/prd.12066
47. Vegeler RC, Yip-Schneider MT, Ralstin M, Wu H, Crooks PA, Neelakantan S, et al. Effect of celecoxib and novel agent lc-1 in a hamster model of lung cancer. J Surg Res (2007) 143(1):169–76. doi: 10.1016/j.jss.2007.08.007
48. Schuller HM, Becker KL, Witschi HP. An animal model for neuroendocrine lung cancer. Carcinogenesis (1988) 9(2):293–6. doi: 10.1093/carcin/9.2.293
49. Ishinishi N, Yamamoto A, Hisanaga A, Inamasu T. Tumorigenicity of arsenic trioxide to the lung in Syrian golden hamsters by intermittent instillations. Cancer Lett (1983) 21(2):141–7. doi: 10.1016/0304-3835(83)90200-8
50. Rehm S, Takahashi M, Ward JM, Singh G, Katyal SL, Henneman JR. Immunohistochemical demonstration of Clara cell antigen in lung tumors of bronchiolar origin induced by n-nitrosodiethylamine in Syrian golden hamsters. Am J Pathol (1989) 134(1):79–87.
51. Furukawa F, Nishikawa A, Imazawa T, Kasahara K, Takahashi M. Enhancing effects of quinacrine on development of hepatopancreatic lesions in n-Nitrosobis(2-Oxopropyl)Amine-Initiated hamsters. Japanese J Cancer Res Gann (1998) 89(2):131–6. doi: 10.1111/j.1349-7006.1998.tb00540.x
52. Son HY, Nishikawa A, Furukawa F, Lee IS, Ikeda T, Miyauchi M, et al. Modifying effects of 4-phenylbutyl isothiocyanate on n-Nitrosobis(2-Oxopropyl)Amine-Induced tumorigenesis in hamsters. Cancer Lett (2000) 160(2):141–7. doi: 10.1016/s0304-3835(00)00570-x
53. Bell RH Jr., Hye RJ, Miyai K. Streptozotocin-induced liver tumors in the Syrian hamster. Carcinogenesis (1984) 5(10):1235–8. doi: 10.1093/carcin/5.10.1235
54. Li JJ, Gonzalez A, Banerjee S, Banerjee SK, Li SA. Estrogen carcinogenesis in the hamster kidney: Role of cytotoxicity and cell proliferation. Environ Health Perspect (1993) 101 Suppl 5(Suppl 5):259–64. doi: 10.1289/ehp.93101s5259
55. Zurawa-Janicka D, Kobiela J, Stefaniak T, Wozniak A, Narkiewicz J, Wozniak M, et al. Changes in expression of serine proteases Htra1 and Htra2 during estrogen-induced oxidative stress and nephrocarcinogenesis in Male Syrian hamster. Acta Biochim Polonica (2008) 55(1):9–19. doi: 10.18388/abp.2008_3123
56. Leavitt WW, Evans RW, Hendry WJ 3rd. Etiology of des-induced uterine tumors in the Syrian hamster. Adv Exp Med Biol (1981) 138:63–86. doi: 10.1007/978-1-4615-7192-6_4
57. Homburger F, Bernfeld P. Cigarette smoke-induced laryngeal cancer: A model for bronchogenic carcinoma in humans. New Engl J Med (1979) 300(15):862. doi: 10.1056/nejm197904123001517
58. Sakakibara K, Oota K. Heterotransplantation of cell lines derived from human malignant neoplasms into golden hamsters treated with antithymocyte serum. Japanese J Exp Med (1975) 45(6):501–14.
59. Wold WS, Toth K. Chapter three–Syrian hamster as an animal model to study oncolytic adenoviruses and to evaluate the efficacy of antiviral compounds. Adv Cancer Res (2012) 115:69–92. doi: 10.1016/b978-0-12-398342-8.00003-3
60. Siurala M, Vähä-Koskela M, Havunen R, Tähtinen S, Bramante S, Parviainen S, et al. Syngeneic Syrian hamster tumors feature tumor-infiltrating lymphocytes allowing adoptive cell therapy enhanced by oncolytic adenovirus in a replication permissive setting. Oncoimmunology (2016) 5(5):e1136046. doi: 10.1080/2162402x.2015.1136046
61. Adams RA, Flowers A, Sundeen R, Merk LP. Chemotherapy and immunotherapy of three human lymphomas serially transplantable in the neonatal Syrian hamster. Cancer (1972) 29(2):524–33. doi: 10.1002/1097-0142(197202)29:2<524::aid-cncr2820290244>3.0.co;2-s
62. Singh I, Tsang KY, Blakemore WS. A model for human osteosarcoma in hamsters. Clin orthopaedics related Res (1979) 144):305–10. doi: 10.1097/00003086-197910000-00053
63. Li R, Miao J, Tabaran AF, O'Sullivan MG, Anderson KJ, Scott PM, et al. A novel cancer syndrome caused by Kcnq1-deficiency in the golden Syrian hamster. J carcinogenesis (2018) 17:6. doi: 10.4103/jcar.JCar_5_18
64. Miao J, Li R, Wettere AJV, Guo H, Tabaran AF, O'Sullivan MG, et al. Cancer spectrum in Tp53-deficient golden Syrian hamsters: A new model for Li-fraumeni syndrome. J carcinogenesis (2021) 20:18. doi: 10.4103/jcar.jcar_18_21
65. Wang Z, Cormier RT. Golden Syrian hamster models for cancer research. Cells (2022) 11(15):2395. doi: 10.3390/cells11152395
66. Daver NG, Maiti A, Kadia TM, Vyas P, Majeti R, Wei AH, et al. Tp53-mutated myelodysplastic syndrome and acute myeloid leukemia: Biology, current therapy, and future directions. Cancer Discovery (2022) 12(11):2516–29. doi: 10.1158/2159-8290.Cd-22-0332
67. Miao J, Lan T, Guo H, Wang J, Zhang G, Wang Z, et al. Characterization of sharpin knockout Syrian hamsters developed using Crispr/Cas9 system. Anim Models Exp Med (2022). doi: 10.1002/ame2.12265
68. Couzin-Frankel J. Breakthrough of the year 2013. Cancer Immunother Sci (New York NY) (2013) 342(6165):1432–3. doi: 10.1126/science.342.6165.1432
69. Jung BK, Ko HY, Kang H, Hong J, Ahn HM, Na Y, et al. Relaxin-expressing oncolytic adenovirus induces remodeling of physical and immunological aspects of cold tumor to potentiate pd-1 blockade. J immunotherapy Cancer (2020) 8(2):e000763. doi: 10.1136/jitc-2020-000763
70. Parviainen S, Ahonen M, Diaconu I, Kipar A, Siurala M, Vähä-Koskela M, et al. Gmcsf-armed vaccinia virus induces an antitumor immune response. Int J Cancer (2015) 136(5):1065–72. doi: 10.1002/ijc.29068
71. Altrock BW, Fagin KD, Hockman HR, Fish EN, Goldstein L, Chang D, et al. Antiviral and antitumor effects of a human interferon analog, ifn-alpha con 1, assessed in hamsters. J Interferon Res (1986) 6(4):405–15. doi: 10.1089/jir.1986.6.405
72. Lee SH, Chiu H, Renton KW, Stebbing N. Modulation by a human interferon of antitumor effects of cyclophosphamide against a lymphosarcoma in hamsters. Biochem Pharmacol (1984) 33(21):3439–43. doi: 10.1016/0006-2952(84)90117-5
73. Santos JM, Havunen R, Siurala M, Cervera-Carrascon V, Tähtinen S, Sorsa S, et al. Adenoviral production of interleukin-2 at the tumor site removes the need for systemic postconditioning in adoptive cell therapy. Int J Cancer (2017) 141(7):1458–68. doi: 10.1002/ijc.30839
74. Akiyama Y, Maruyama K, Nara N, Hojo T, Cheng JY, Mori T, et al. Antitumor effects induced by dendritic cell-based immunotherapy against established pancreatic cancer in hamsters. Cancer Lett (2002) 184(1):37–47. doi: 10.1016/s0304-3835(02)00189-1
75. Himeno Y, Etoh T, Matsumoto T, Ohta M, Nishizono A, Kitano S. Efficacy of oncolytic reovirus against liver metastasis from pancreatic cancer in immunocompetent models. Int J Oncol (2005) 27(4):901–6. doi: 10.3892/ijo.27.4.901
76. Li X, Wang P, Li H, Du X, Liu M, Huang Q, et al. The efficacy of oncolytic adenovirus is mediated by T-cell responses against virus and tumor in Syrian hamster model. Clin Cancer Res an Off J Am Assoc Cancer Res (2017) 23(1):239–49. doi: 10.1158/1078-0432.Ccr-16-0477
77. Thomas MA, Spencer JF, La Regina MC, Dhar D, Tollefson AE, Toth K, et al. Syrian Hamster as a permissive immunocompetent animal model for the study of oncolytic adenovirus vectors. Cancer Res (2006) 66(3):1270–6. doi: 10.1158/0008-5472.Can-05-3497
78. Bazan-Peregrino M, Garcia-Carbonero R, Laquente B, Álvarez R, Mato-Berciano A, Gimenez-Alejandre M, et al. Vcn-01 disrupts pancreatic cancer stroma and exerts antitumor effects. J immunotherapy Cancer (2021) 9(11):e003254. doi: 10.1136/jitc-2021-003254
79. Mato-Berciano A, Morgado S, Maliandi MV, Farrera-Sal M, Gimenez-Alejandre M, Ginestà MM, et al. Oncolytic adenovirus with hyaluronidase activity that evades neutralizing antibodies: Vcn-11. J Controlled release Off J Controlled Release Soc (2021) 332:517–28. doi: 10.1016/j.jconrel.2021.02.035
80. Young BA, Spencer JF, Ying B, Toth K, Wold WS. The effects of radiation on antitumor efficacy of an oncolytic adenovirus vector in the Syrian hamster model. Cancer Gene Ther (2013) 20(9):531–7. doi: 10.1038/cgt.2013.50
81. Bortolanza S, Bunuales M, Alzuguren P, Lamas O, Aldabe R, Prieto J, et al. Deletion of the E3-6.7k/Gp19k region reduces the persistence of wild-type adenovirus in a permissive tumor model in Syrian hamsters. Cancer Gene Ther (2009) 16(9):703–12. doi: 10.1038/cgt.2009.12
82. Hasegawa N, Abei M, Yokoyama KK, Fukuda K, Seo E, Kawashima R, et al. Cyclophosphamide enhances antitumor efficacy of oncolytic adenovirus expressing uracil phosphoribosyltransferase (Uprt) in immunocompetent Syrian hamsters. Int J Cancer (2013) 133(6):1479–88. doi: 10.1002/ijc.28132
83. Bortolanza S, Alzuguren P, Buñuales M, Qian C, Prieto J, Hernandez-Alcoceba R. Human adenovirus replicates in immunocompetent models of pancreatic cancer in Syrian hamsters. Hum Gene Ther (2007) 18(8):681–90. doi: 10.1089/hum.2007.017
84. Bortolanza S, Bunuales M, Otano I, Gonzalez-Aseguinolaza G, Ortiz-de-Solorzano C, Perez D, et al. Treatment of pancreatic cancer with an oncolytic adenovirus expressing interleukin-12 in Syrian hamsters. Mol Ther J Am Soc Gene Ther (2009) 17(4):614–22. doi: 10.1038/mt.2009.9
85. Quixabeira DCA, Zafar S, Santos JM, Cervera-Carrascon V, Havunen R, Kudling TV, et al. Oncolytic adenovirus coding for a variant interleukin 2 (Vil-2) cytokine re-programs the tumor microenvironment and confers enhanced tumor control. Front Immunol (2021) 12:674400. doi: 10.3389/fimmu.2021.674400
86. Heiniö C, Havunen R, Santos J, de Lint K, Cervera-Carrascon V, Kanerva A, et al. Tnfa and Il2 encoding oncolytic adenovirus activates pathogen and danger-associated immunological signaling. Cells (2020) 9(4):798. doi: 10.3390/cells9040798
87. Bunuales M, Garcia-Aragoncillo E, Casado R, Quetglas JI, Hervas-Stubbs S, Bortolanza S, et al. Evaluation of monocytes as carriers for armed oncolytic adenoviruses in murine and Syrian hamster models of cancer. Hum Gene Ther (2012) 23(12):1258–68. doi: 10.1089/hum.2012.043
88. Ahmed J, Chard LS, Yuan M, Wang J, Howells A, Li Y, et al. A new oncolytic V accinia virus augments antitumor immune responses to prevent tumor recurrence and metastasis after surgery. J immunotherapy Cancer (2020) 8(1):e000415. doi: 10.1136/jitc-2019-000415
89. Nakano K, Todo T, Zhao G, Yamaguchi K, Kuroki S, Cohen JB, et al. Enhanced efficacy of conditionally replicating herpes simplex virus (G207) combined with 5-fluorouracil and surgical resection in peritoneal cancer dissemination models. J Gene Med (2005) 7(5):638–48. doi: 10.1002/jgm.700
90. Young BA, Spencer JF, Ying B, Tollefson AE, Toth K, Wold WS. The role of cyclophosphamide in enhancing antitumor efficacy of an adenovirus oncolytic vector in subcutaneous Syrian hamster tumors. Cancer Gene Ther (2013) 20(9):521–30. doi: 10.1038/cgt.2013.49
91. Dhar D, Toth K, Wold WS. Cycles of transient high-dose cyclophosphamide administration and intratumoral oncolytic adenovirus vector injection for long-term tumor suppression in Syrian hamsters. Cancer Gene Ther (2014) 21(4):171–8. doi: 10.1038/cgt.2014.13
92. Thomas MA, Spencer JF, Toth K, Sagartz JE, Phillips NJ, Wold WS. Immunosuppression enhances oncolytic adenovirus replication and antitumor efficacy in the Syrian hamster model. Mol Ther J Am Soc Gene Ther (2008) 16(10):1665–73. doi: 10.1038/mt.2008.162
93. Dhar D, Spencer JF, Toth K, Wold WS. Effect of preexisting immunity on oncolytic adenovirus vector ingn 007 antitumor efficacy in immunocompetent and immunosuppressed Syrian hamsters. J Virol (2009) 83(5):2130–9. doi: 10.1128/jvi.02127-08
94. Siurala M, Bramante S, Vassilev L, Hirvinen M, Parviainen S, Tähtinen S, et al. Oncolytic adenovirus and doxorubicin-based chemotherapy results in synergistic antitumor activity against soft-tissue sarcoma. Int J Cancer (2015) 136(4):945–54. doi: 10.1002/ijc.29048
95. Bramante S, Koski A, Kipar A, Diaconu I, Liikanen I, Hemminki O, et al. Serotype chimeric oncolytic adenovirus coding for gm-csf for treatment of sarcoma in rodents and humans. Int J Cancer (2014) 135(3):720–30. doi: 10.1002/ijc.28696
96. He X, Xu C. Immune checkpoint signaling and cancer immunotherapy. Cell Res (2020) 30(8):660–9. doi: 10.1038/s41422-020-0343-4
97. Quesada JR, Hersh EM, Manning J, Reuben J, Keating M, Schnipper E, et al. Treatment of hairy cell leukemia with recombinant alpha-interferon. Blood (1986) 68(2):493–7. doi: 10.1182/blood.V68.2.493.493
98. Rosenberg SA. Il-2: The first effective immunotherapy for human cancer. J Immunol (Baltimore Md 1950) (2014) 192(12):5451–8. doi: 10.4049/jimmunol.1490019
99. Propper DJ, Balkwill FR. Harnessing cytokines and chemokines for cancer therapy. Nat Rev Clin Oncol (2022) 19(4):237–53. doi: 10.1038/s41571-021-00588-9
100. Met Ö, Jensen KM, Chamberlain CA, Donia M, Svane IM. Principles of adoptive T cell therapy in cancer. Semin immunopathology (2019) 41(1):49–58. doi: 10.1007/s00281-018-0703-z
101. Rosenberg SA, Restifo NP. Adoptive cell transfer as personalized immunotherapy for human cancer. Sci (New York NY) (2015) 348(6230):62–8. doi: 10.1126/science.aaa4967
102. Saxena M, van der Burg SH, Melief CJM, Bhardwaj N. Therapeutic cancer vaccines. Nat Rev Cancer (2021) 21(6):360–78. doi: 10.1038/s41568-021-00346-0
103. Sabado RL, Balan S, Bhardwaj N. Dendritic cell-based immunotherapy. Cell Res (2017) 27(1):74–95. doi: 10.1038/cr.2016.157
104. Cheever MA, Higano CS. Provenge (Sipuleucel-T) in prostate cancer: The first fda-approved therapeutic cancer vaccine. Clin Cancer Res an Off J Am Assoc Cancer Res (2011) 17(11):3520–6. doi: 10.1158/1078-0432.Ccr-10-3126
105. Kantoff PW, Higano CS, Shore ND, Berger ER, Small EJ, Penson DF, et al. Sipuleucel-T immunotherapy for castration-resistant prostate cancer. New Engl J Med (2010) 363(5):411–22. doi: 10.1056/NEJMoa1001294
106. Kaufman HL, Kohlhapp FJ, Zloza A. Oncolytic viruses: A new class of immunotherapy drugs. Nat Rev Drug Discovery (2015) 14(9):642–62. doi: 10.1038/nrd4663
107. Thomas MA, Spencer JF, Wold WS. Use of the Syrian hamster as an animal model for oncolytic adenovirus vectors. Methods Mol Med (2007) 130:169–83. doi: 10.1385/1-59745-166-5:169
108. Puig-Saus C, Laborda E, Rodríguez-García A, Cascalló M, Moreno R, Alemany R. The combination of I-leader truncation and gemcitabine improves oncolytic adenovirus efficacy in an immunocompetent model. Cancer Gene Ther (2014) 21(2):68–73. doi: 10.1038/cgt.2013.85
109. Thaci B, Ahmed AU, Ulasov IV, Tobias AL, Han Y, Aboody KS, et al. Pharmacokinetic study of neural stem cell-based cell carrier for oncolytic virotherapy: Targeted delivery of the therapeutic payload in an orthotopic brain tumor model. Cancer Gene Ther (2012) 19(6):431–42. doi: 10.1038/cgt.2012.21
110. Kim KH, Ryan MJ, Estep JE, Miniard BM, Rudge TL, Peggins JO, et al. A new generation of serotype chimeric infectivity-enhanced conditionally replicative adenovirals: The safety profile of Ad5/3-Δ24 in advance of a phase I clinical trial in ovarian cancer patients. Hum Gene Ther (2011) 22(7):821–8. doi: 10.1089/hum.2010.180
111. Poutou J, Bunuales M, Gonzalez-Aparicio M, Garcia-Aragoncillo E, Quetglas JI, Casado R, et al. Safety and antitumor effect of oncolytic and helper-dependent adenoviruses expressing interleukin-12 variants in a hamster pancreatic cancer model. Gene Ther (2015) 22(9):696–706. doi: 10.1038/gt.2015.45
112. Nelles MJ, Duncan WR, Streilein JW. Immune response to acute virus infection in the Syrian hamster. ii. studies on the identity of virus-induced cytotoxic effector cells. J Immunol (Baltimore Md 1950) (1981) 126(1):214–8. doi: 10.4049/jimmunol.126.1.214
Keywords: Syrian hamster, tumor model, cancer immunotherapy, immune checkpoint inhibitor, cytokine, adoptive cell therapy, cancer vaccine, oncolytic virus
Citation: Jia Y, Wang Y, Dunmall LSC, Lemoine NR, Wang P and Wang Y (2023) Syrian hamster as an ideal animal model for evaluation of cancer immunotherapy. Front. Immunol. 14:1126969. doi: 10.3389/fimmu.2023.1126969
Received: 18 December 2022; Accepted: 09 February 2023;
Published: 27 February 2023.
Edited by:
An Coosemans, KU Leuven, BelgiumReviewed by:
Tibor Bakacs, Alfred Renyi Institute of Mathematics, HungaryJinxin Miao, Henan University of Traditional Chinese Medicine, China
Copyright © 2023 Jia, Wang, Dunmall, Lemoine, Wang and Wang. This is an open-access article distributed under the terms of the Creative Commons Attribution License (CC BY). The use, distribution or reproduction in other forums is permitted, provided the original author(s) and the copyright owner(s) are credited and that the original publication in this journal is cited, in accordance with accepted academic practice. No use, distribution or reproduction is permitted which does not comply with these terms.
*Correspondence: Yaohe Wang, eWFvaGUud2FuZ0BxbXVsLmFjLnVr; Pengju Wang, d2FuZ3BlbmdqdUB6enUuZWR1LmNu