- Department of Infectious Diseases, College of Veterinary Medicine, University of Georgia, Athens, GA, United States
Bordetella pertussis (Bp) is the highly transmissible etiologic agent of pertussis, a severe respiratory disease that causes particularly high morbidity and mortality in infants and young children. Commonly known as “whooping cough,” pertussis is one of the least controlled vaccine-preventable diseases worldwide with several countries experiencing recent periods of resurgence despite broad immunization coverage. While current acellular vaccines prevent severe disease in most cases, the immunity they confer wanes rapidly and does not prevent sub clinical infection or transmission of the bacterium to new and vulnerable hosts. The recent resurgence has prompted new efforts to generate robust immunity to Bp in the upper respiratory mucosa, from which colonization and transmission originate. Problematically, these initiatives have been partially hindered by research limitations in both human and animal models as well as potent immunomodulation by Bp. Here, we consider our incomplete understanding of the complex host-pathogen dynamics occurring in the upper airway to propose new directions and methods that may address critical gaps in research. We also consider recent evidence that supports the development of novel vaccines specifically designed to generate robust mucosal immune responses capable of limiting upper respiratory colonization to finally halt the ongoing circulation of Bordetella pertussis.
Introduction
Bordetella pertussis (Bp) is the highly transmissible etiologic agent of pertussis, a severe respiratory disease that causes particularly high morbidity and mortality in infants and young children (1–3). Patients with pertussis disease classically exhibit bouts of intense paroxysmal coughing which may be accompanied by post-tussive vomiting, bronchopneumonia, pulmonary hypertension, hypoxia, and in severe cases, brain damage or death (4). Problematically, whooping cough remains one of the least controlled vaccine-preventable diseases worldwide with several countries experiencing recent periods of resurgence (5). Increased incidence has been correlated with a transition from whole cell pertussis (wP) vaccines to acellular pertussis (aP) vaccines composed of 1-5 detoxified antigens including pertussis toxin, pertactin, filamentous hemagglutinin, and fimbriae 2&3 (6–9). While aP vaccines are highly successful in preventing severe lower respiratory (LR) disease in most cases, the Th-2 skewed immunity they confer wanes rapidly and fails to prevent sub-clinical upper respiratory (UR) mucosal infection and transmission to new hosts (9, 10).
These observations are compounded by the rapid emergence and expansion of “vaccine escape mutant” strains deficient in pertactin, the only antigen included in aP vaccines capable of generating bactericidal antibodies against Bp (7, 8, 11–14). It is speculated that targeted loss of this surface-bound antigen, but not other aP components, may grant a fitness advantage as it evades anti-PRN bactericidal antibodies to facilitate persistence within the UR tract of aP vaccinated hosts (8). This is further supported by the observation that pertactin-deficient mutants are emerging from a diversity of Bp lineages, which have now risen to dominance in many countries with broad aP vaccine coverage. Together these observations have prompted new research initiatives aimed at generating sterilizing mucosal immunity in the nasal cavity to prevent initial colonization and limit the ongoing circulation of Bp within vaccinated populations.
Of note, the issue of sub-clinical infection and transmission amongst vaccinated individuals is not limited to Bp. Generating sterilizing immunity in the nasopharynx is a common challenge against many respiratory pathogens including pandemic SARS-CoV-2, Mycobacterium tuberculosis, influenza, respiratory syncytial virus, and others (15–18). Each of these pathogens can infect, persist, and often transmit from the nasopharynx; a mucosal site that continuously encounters foreign antigen. To do this, these pathogens must bypass specialized nasopharyngeal tissue equipped with mucus layers saturated with antimicrobial peptides, beating cilia, a sea of competitive microflora, innate responders such as neutrophils & macrophages, as well as inductor and effector sites replete with dendritic cells, T cells, B cells, and secretory IgA (19–22).
In humans, these complex defensive networks are largely evaded or modulated by Bp long enough to facilitate growth and transmission to new hosts (19, 23–30). Increased reports of asymptomatic or sub-clinical cases of Bp infection provides clinical evidence of this ability in populations with broad vaccine coverage which warrants new research initiatives and the development of targeted mucosal vaccine strategies. To develop vaccines capable of generating sustained protective immunity in the UR mucosa, it is critical that we first understand how these pathogens modulate or evade the host immune response in the nasopharynx and similarly determine which mucosal immune factors are involved in eventually controlling and clearing infection.
Much of our understanding of host-pathogen interactions in Bp infections has been derived from conventional animal models aimed at characterizing (and preventing) the most severe forms of pertussis disease (19). These efforts have been instrumental in understanding the systemic and pulmonary immune responses to Bp, enabling the development of vaccines capable of preventing severe life-threatening pertussis. However, despite Bp primarily residing within the upper respiratory mucosa, there is comparatively little published work that investigates the specific local mucosal immune response to Bp relative to pulmonary or systemic responses (31). Excitingly, the recent development of several novel murine and baboon models has begun to allow us to study the critical mechanistic distinctions between UR and LR responses, and elucidate factors involved in colonization, persistence, transmission, and immunity in Bp infections. Although much more arduous and expensive, controlled human infection models have the potential to verify results observed in precursory animal models and expand our understanding of the human-specific response to Bp.
Here we consider our incomplete understanding of the complex host-pathogen dynamics occurring in the upper airway to highlight and propose new directions that may address these critical gaps in research. We also consider recent evidence that supports the development of intranasal vaccines specifically designed to generate sustained mucosal immune responses capable of limiting upper respiratory colonization and the ongoing circulation of Bordetella pertussis.
Intramuscular vaccine-induced serum IgG protects against severe pulmonary disease but does not control Bp in the nasal cavity
While the cause of pertussis resurgence is multifactorial, it is well-appreciated that waning immunity and flawed aP vaccine-induced immunity are involved. Problematically, vaccine-induced protection and antibody titers roughly correlate, but neither is sustained indefinitely. Prior data from several human studies have demonstrated that peripheral blood anti-pertussis immunoglobulin G (IgG) titers decay rapidly within the first-year post-vaccination and continue to decline at slower rates thereafter (32–37). However, it remains unclear at what threshold antibody-titer decay results in increased susceptibility to pertussis disease. Although it is currently believed that high anti-pertussis IgG titers contribute to prevention of severe pulmonary disease, the mechanisms of protection remain poorly understood, and appear to vary in different regions of the respiratory tract (10, 19, 22, 38–41).
In contrast to protection observed in the LR tract, vaccine-induced IgG responses are less effective in controlling UR mucosal Bp infection. This has been repeatedly observed from conventional murine models, as high antibody titers were detected in aP vaccinated animals with no reduction in CFU in the nasal cavity, resulting in prolonged carriage (19, 21). This was similarly reported in the aP-primed baboon model, which demonstrated persistent colonization beyond 35-days post-challenge despite high serum IgG titers against all five aP antigens. Interestingly, the lack of IgG-mediated protection is not unique to aP vaccine-induced immunity and was also reported from wP primed baboons with modest improvement in clearance to 19/21 days post-challenge (10). Together these observations suggest aP- and wP-primed IgG responses fail to rapidly control Bp from the nasopharynx, resulting in extended periods of colonization and transmissibility.
Historically, anti-pertussis IgG titers have been the most accessible indicator for immunity in both clinical and research settings. However, the dual observation that both wP and aP vaccine-induced IgG titers correlate with reduced incidence of severe pulmonary disease but fail to prevent sub-clinical infection complicates our ability to use anti-pertussis IgG as a reliable indicator for UR protection. The disconnect between high IgG titers and persistent nasopharyngeal infection may be partially attributed to pertussis toxin-specific mechanisms that block serum-mediated clearance by delaying the recruitment of neutrophils, among other immunomodulatory abilities detailed in sections below (26). These data collectively suggest that serum IgG titers alone are not a sufficient indicator for protective immunity against Bp, and that other, localized mechanisms of protection should be evaluated.
It is well appreciated that convalescent immunity following a course of primary infection with Bp generates humoral and cellular immune responses that are critical in generating more effective protection against secondary infection in both the UR and LR tracts (31). It is probable that a portion of this protective immune response in the LR is mediated by IgG, and evidence supporting its role in reducing morbidity and mortality associated with clinical disease warrants continued research into anti-pertussis IgG responses. However, when evaluating IgG responses to new candidate antigens for improved vaccines, it is critical that we differentiate between bactericidal antibodies directed against surface-bound factors which facilitate clearance, and neutralizing antibodies which protect against toxin-induced severe disease (11).
The controversial role of secretory IgA against Bp
Immunoglobulin A (IgA) is produced in response to natural infection, but not from current intramuscular aP or wP vaccines. However, conflicting reports regarding the role of IgA in infection with Bp have confounded our ability to correlate protection with this critical component of mucosal immunity. Anti-pertussis IgA appears to reduce adherence of Bp to ciliated epithelium in vitro, which could provide some level of protection against initial colonization and/or spread through the respiratory tract (39). In addition, human anti-pertussis IgA has been shown to effectively bind FcαRI on polymorphonuclear leukocytes to stimulate phagocytosis in vitro, indicating that IgA can be effective in facilitating bacterial clearance, despite IgA being traditionally considered a poor complement activator relative to IgM and IgG (22, 42). However, IgA-deficient mice were previously reported to be indistinguishable from wild type mice in controlling primary or secondary Bp infection, suggesting IgA plays at most a modest and/or redundant role in immunity to Bp (41).
The strongest data to argue a protective role for IgA against Bp was reported from experiments investigating a recent live-attenuated vaccine candidate, BPZE1. Designed to be administered intranasally, BPZE1 is reported to generate a robust secretory IgA response which appears to correlate with protection against challenge with Bp. Importantly, BPZE1-vaccinated IgA-/- mice had significantly higher colonization on days 7 and 21 post-challenge relative to vaccinated wildtype mice (43). These data indicate that removal of IgA resulted in significantly impaired protection generated by a candidate intranasal vaccine. Importantly, baboons vaccinated with BPZE1 also generated protective immunity with detectable Bp-specific serum IgA, however UR mucosal sIgA was not directly evaluated (44).
The observation of sIgA production following intranasal delivery of commercial pertussis vaccines has prompted new investigations into the merits of intranasal vs. intramuscular vaccination approaches. In a recent study, mice intranasally vaccinated with aP vaccines generated similar levels of protection and serum IgG levels relative to intramuscular vaccination and significantly higher IgA titers in the nasal cavity by day 9 post Bp challenge (45, 46). A small human study also observed strong mucosal and systemic immune responses following intranasal wP vaccination (over 4 doses), which is a positive indicator for applicability of this concept in human immunization (47).
These examples suggest that intranasal vaccination and/or infection are able to generate protective sIgA against Bp via a site-specific mechanism. It is likely that intranasal but not intramuscular delivery facilitates uptake by nasal microfold (M) cells required for translocation into nasopharynx-associate lymphoid tissue (NALT) (48). This inductive tissue is critical for the generation of mucosal immunity against other pathogens, and NALT-targeted immunization has been shown to induce both mucosal and systemic immunity. Importantly, sIgA production is specifically stimulated in mucosal effector sites, where dendritic cell-activated T-cells specifically induce clonal expansion of IgA+ B cells (Figure 1). The resulting IgA+ B cells and plasmablasts induced by nasal immunization express CCR10 and α4β1-integrin, which together facilitate migration to respiratory tissues that express the corresponding receptors CCL28 and VCAM1, respectively (48–50).
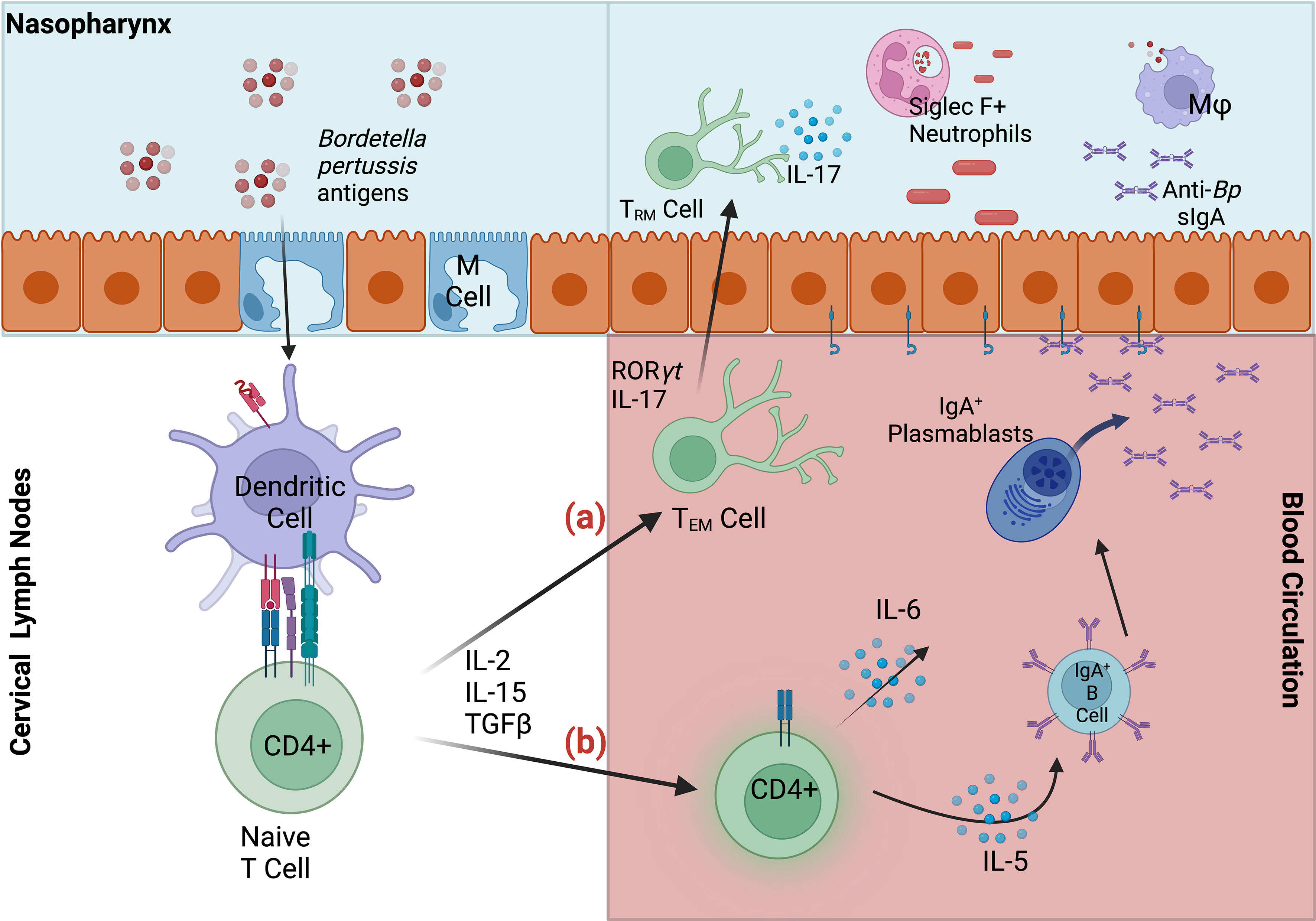
Figure 1 Summary of mucosal responses to Bordetella pertussis in nasopharynx-associated lymphoid tissue (NALT). Bp antigens are transported via microfold (M) cells into the NALT, where dendritic cells capture antigen, migrate to the lymph nodes and stimulate naïve CD4+ T cells. (A) Activated effector CD4+ T cells may then migrate to nasal tissue, where they are maintained as TRM. Upon secondary infection IL-17+ TRM expand and recruit Siglec F+ neutrophils to the nasopharynx, facilitating rapid clearance (B) Effector CD4+ T cells also induce maturation of IgA-committed B cells, which migrate to the cervical lymph nodes, and to their effector site (nasal cavity). IgA+ B cells and plasmablasts mature into plasma cells in response to cytokines IL-5 and IL-6. Dimeric IgA, secreted by differentiated plasma cells, bind polymeric Ig receptors, and are released into infected respiratory tissue as secretory IgA. Anti-Bp sIgA, along with macrophages (Mφ) and Siglec F+ neutrophils contribute to the clearance of Bp in the upper respiratory tract.
In sum, conflicting reports of IgA-mediated protection against Bp warrant additional investigations into sIgA and intranasal vaccination strategies that deliver antigen directly to the site of infection (50). However, linking sIgA responses with sustained protection may present an additional challenge for future vaccine applications aimed at eliciting this response given the faster decline of IgA relative to IgG titers following diagnosis of clinical (symptomatic) pertussis (42, 51).
New vaccines must generate robust Th1/Th17 responses to enable clearance of Bordetella pertussis from the nasopharynx
The inability of aP vaccines to generate robust immunity in the nasal mucosa has sparked a critical need to better understand the immune response to Bp at this initial site of infection, in contrast to pneumonic responses which are relatively well-characterized. To date, cellular responses specific to Bp in the nasal mucosa have received the most attention, however, research initiatives investigating this topic are relatively recent, and much remains unknown.
T cell-mediated immunity has long been reported to play a central role in the control and clearance of Bp from the respiratory tract. Specifically, proinflammatory Th1 and Th17 responses have been shown to be critical for protection against colonization with Bp (50, 52). Problematically, aP vaccines primarily induce a Th2-skewed response, which is not sufficient to protect the UR tract from subsequent infection (31, 50, 52). Additionally, aP vaccines fail to generate tissue-resident memory T cells (TRM), which are typically maintained in respiratory tissue and respond rapidly to secondary encounter with Bp (53).
Natural infection and intranasal vaccination generate TRM that are protective in the upper respiratory tract (Figure 1). Specifically, IL-17 secreting CD4+ TRM induce rapid neutrophilic recruitment to the nasopharynx in response to secondary challenge, which has been shown to greatly contribute to the opsonization and control of Bp (52–54). Following secondary infection, a cascade of pro-inflammatory cytokines including IL-1β, TNFα, IL-17A (from TRM), IL-17C (from epithelial cells), IL-6, and IFNγ recruit additional phagocytes to the site of infection including dendritic cells and macrophages which ultimately clear the infection via opsonization-enhanced phagocytosis (Figure 2). Interestingly, IL-17+ TRM have been shown to recruit a unique subset of Siglec F+ neutrophils, which have been recently described to primarily reside within the nasal mucosa and exhibit an activated phenotype with increased NETosis (55, 56).
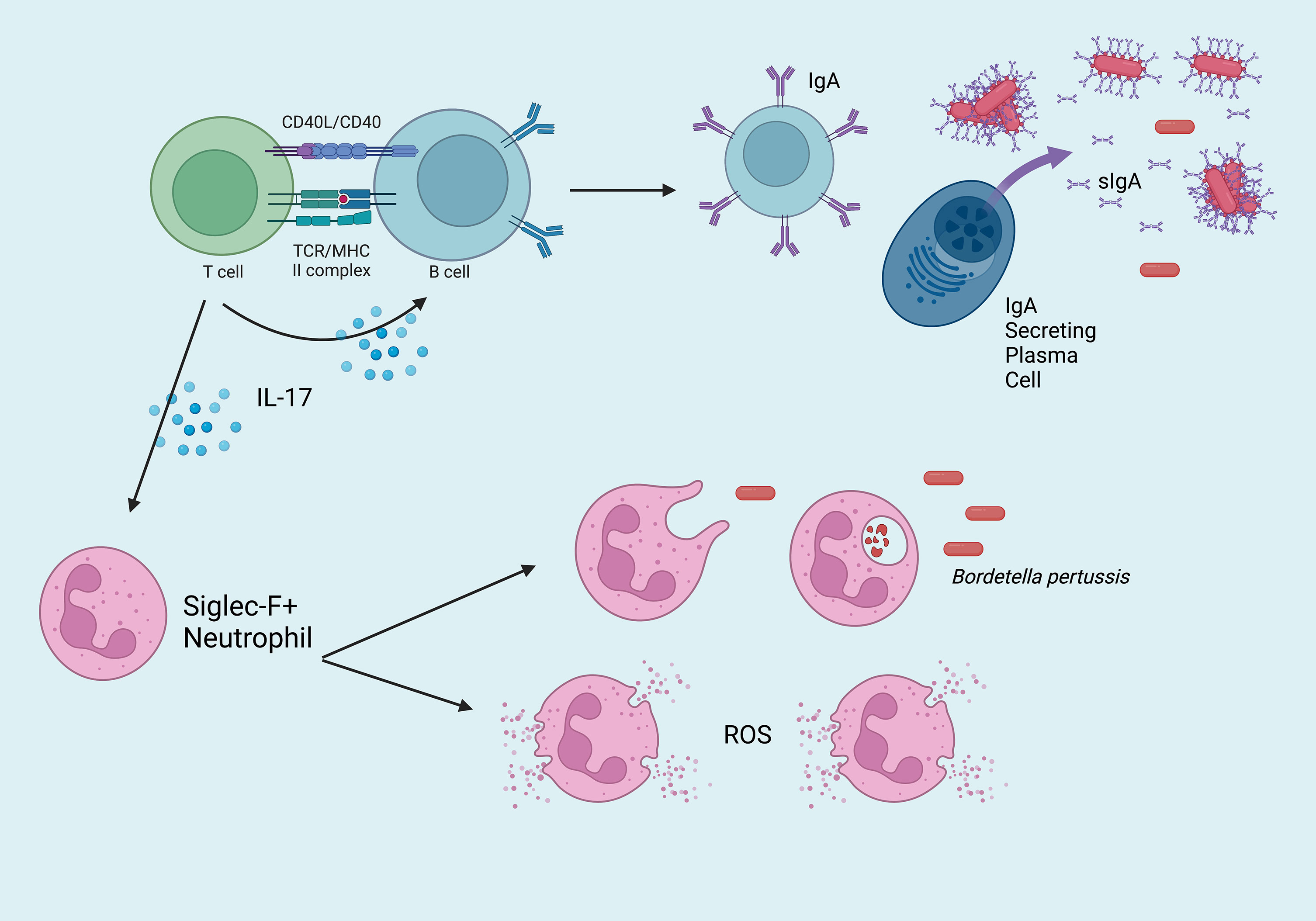
Figure 2 Overview of the critical mucosal-specific factors in cell-mediated immunity to Bordetella pertussis in the nasopharynx. Effector CD4+ T cells activate IgA+ B cells, which mature into dimeric IgA-secreting plasma cells. Once transported across the epithelium, sIgA binds Bp, which may bind and enable clearance by phagocytes. IL-17+ TRM recruit Siglec F+ neutrophils to the site of infection, which eliminate Bp via phagocytosis or NETosis (ROS = reactive oxygen species).
Decades of immunology research has demonstrated that the absence or removal of a single component of the immune system can result in deleterious effects to protective immunity. Notably, intramuscular aP vaccines fail to stimulate or generate multiple components of both humoral (IgA) and cellular (IL-17+ TRM, Siglec F+ neutrophils) immunity that are critical in the mucosal response to respiratory pathogens. However, the observation that intranasal administration of aP vaccines and candidate vaccines like BPZE1 are able to generate these responses suggest we have much to learn from continued investigations in this area (31).
Disrupting immunomodulation to inform vaccine-development
An additional barrier to our understanding of the response to Bp in the nasopharynx is immunomodulation. Like many well-adapted pathogens, Bp has evolved complex mechanisms to modulate the host immune system to its advantage in active infection and can reinfect convalescent hosts after immunity wanes. These abilities are well-accepted to be multifactorial, with several known virulence factors having unique impacts on diverse aspects of the host immune response to include the suppression of neutrophilic inflammation, decreased cytokine signaling from multiple cell types, complement evasion, and suppression of serum antibody responses (23–30).
To highlight a few examples: pertactin has been reported to modulate the secretion of pro-inflammatory cytokines (TNFα, IL-6, IL-8, G-CSF) and may downregulate host genes associated with cell death (30). Adenylate cyclase toxin targets Cd11b-positive professional phagocytes, including dendritic cells, macrophages, and neutrophils by forming cation-selective pores to permeabilize the cell membrane, while also impairing dendritic cell maturation and cytokine secretion (25). Pertussis toxin disrupts G protein-coupled receptor signaling and inhibits the early recruitment of macrophages and neutrophils (24, 26). B. pertussis also evades phagocyte-mediated killing by surviving within macrophages, an ability that is shared by many other bacteria with immunomodulatory abilities (57).
Future vaccine candidates, particularly live-attenuated, may benefit from removal of these immunomodulatory factors to boost immunity. Their formulations could be supplemented with detoxified versions of these proteins if their antigens are critical in Bp immunity, such as pertussis toxin. It is likely that immunomodulation is particularly important in mucosal immunity to Bp, as the tightly controlled inflammatory responses in the nasopharynx may be further reduced to enable persistence and extend the window of transmissibility (19, 58–61).
Disrupting immunomodulation in animal models may be informative as a tool to evaluate which aspects of the host response are actively suppressed by Bp (62). This idea is supported by recent work published in animal models of Bordetella bronchiseptica (Bb) infection, where deletion of a regulator of immunomodulators (btrS) generated robust sterilizing immunity and identified eosinophils as unexpected contributors in pulmonary responses to Bb (63).
Concluding remarks
In an era of widespread resurgence, imperfect waning immunity, and rapid expansion of vaccine-escape mutants, research initiatives have ambitiously shifted toward generating complete protection in the nasal mucosa. Accomplishing this goal will require an improved understanding of the complex immunobiology of upper respiratory infection with Bp, and the development of vaccines that prime IgG, IgA, and Th1/Th-17 responses in the respiratory mucosa. Excitingly, recent investigations into intranasal vaccination support our ability to develop next-generation vaccines to generate robust sterilizing mucosal and systemic immunity sufficient to reduce carriage and the ongoing circulation of Bp in vaccinated populations.
Data availability statement
The original contributions presented in the study are included in the article/supplementary material. Further inquiries can be directed to the corresponding author.
Author contributions
AC and MC conceived the perspective. AC and EH wrote the manuscript. MC generated the figures. All authors contributed to the article and approved the submitted version.
Funding
This work was supported by grants AI156293 and AI159347 of the National Institutes of Health to EH. The funders had no role in study design, data collection, and interpretation, or the decision to submit the work for publication.
Conflict of interest
The authors declare that the research was conducted in the absence of any commercial or financial relationships that could be construed as a potential conflict of interest.
Publisher’s note
All claims expressed in this article are solely those of the authors and do not necessarily represent those of their affiliated organizations, or those of the publisher, the editors and the reviewers. Any product that may be evaluated in this article, or claim that may be made by its manufacturer, is not guaranteed or endorsed by the publisher.
References
1. Roush SW, Murphy T. v., Basket MM, Iskander JK, Moran JS, Seward JF, et al. Historical comparisons of morbidity and mortality for vaccine-preventable diseases in the united states. J Am Med Assoc (2007) 298(18):2155–63. doi: 10.1001/jama.298.18.2155
2. Jackson DW, Rohani P. Perplexities of pertussis: Recent global epidemiological trends and their potential causes. Epidemiol Infect. (2014) 142(4):672–84. doi: 10.1017/S0950268812003093
3. Black RE, Cousens S, Johnson HL, Lawn JE, Rudan I, Bassani DG, et al. Global, regional, and national causes of child mortality in 2008: a systematic analysis. Lancet (2010) 375:1969–87. doi: 10.1016/S0140-6736(10)60549-1
4. Hiramatsu Y, Suzuki K, Nishida T, Onoda N, Satoh T, Akira S, et al. The mechanism of pertussis cough revealed by the mouse-coughing model. mBio (2022) 13(2):e0319721. doi: 10.1128/mbio.03197-21
5. Frenkel LD. The global burden of vaccine-preventable infectious diseases in children less than 5 years of age: Implications for COVID-19 vaccination. how can we do better? Allergy Asthma Proc (2021) 42(5):378–85. doi: 10.2500/aap.2021.42.210065
6. Saso A, Kampmann B, Roetynck S. Vaccine-induced cellular immunity against bordetella pertussis: Harnessing lessons from animal and human studies to improve design and testing of novel pertussis vaccines. Vaccines (2021) 9(8). doi: 10.3390/vaccines9080877
7. Martin SW, Pawloski L, Williams M, Weening K, Debolt C, Qin X, et al. Pertactin-negative bordetella pertussis strains: Evidence for a possible selective advantage. Clin Infect Dis (2015) 60(2):223–7. doi: 10.1093/cid/ciu788
8. Ma L, Caulfield A, Dewan KK, Harvill ET. Pertactin-deficient bordetella pertussis, vaccine-driven evolution, and reemergence of pertussis. Emerging Infect Dis (2021) 27(6):1561–6. doi: 10.3201/eid2706.203850
9. Locht C. Pertussis: Where did we go wrong and what can we do about it? J Infect. (2016) 72. doi: 10.1016/j.jinf.2016.04.020
10. Warfel JM, Zimmerman LI, Merkel TJ. (2014). Acellular pertussis vaccines protect against disease but fail to prevent infection and transmission in a nonhuman primate model, in: Proceedings of the National Academy of Sciences of the United States of America. doi: 10.1073/pnas.1314688110
11. Lesne E, Cavell BE, Freire-Martin I, Persaud R, Alexander F, Taylor S, et al. Acellular pertussis vaccines induce anti-pertactin bactericidal antibodies which drives the emergence of pertactin-negative strains. Front Microbiol (2020) 11:2108. doi: 10.3389/fmicb.2020.02108
12. Pawloski LC, Queenan AM, Cassiday PK, Lynch AS, Harrison MJ, Shang W, et al. Prevalence and molecular characterization of pertactin-deficient bordetella pertussis in the united states. Clin Vaccine Immunol (2014) 21(2):119–25. doi: 10.1128/CVI.00717-13
13. Barkoff AM, Mertsola J, Pierard D, Dalby T, Hoegh SV, Guillot S, et al. Pertactin-deficient bordetella pertussis isolates: Evidence of increased circulation in Europe, 1998 to 2015. Eurosurveillance (2019) 24(7):1700832. doi: 10.2807/1560-7917.ES.2019.24.7.1700832
14. Safarchi A, Octavia S, Luu LDW, Tay CY, Sintchenko V, Wood N, et al. Pertactin negative bordetella pertussis demonstrates higher fitness under vaccine selection pressure in a mixed infection model. Vaccine (2015) 33(46):6277–81. doi: 10.1016/j.vaccine.2015.09.064
15. Petukhova G, Naikhin A, Chirkova T, Donina S, Korenkov D, Rudenko L. Comparative studies of local antibody and cellular immune responses to influenza infection and vaccination with live attenuated reassortant influenza vaccine (LAIV) utilizing a mouse nasal-associated lymphoid tissue (NALT) separation method. Vaccine (2009) 27(19):2580–7. doi: 10.1016/J.VACCINE.2009.02.035
16. Barnes MVC, Openshaw PJM, Thwaites RS. Mucosal immune responses to respiratory syncytial virus. Cells (2022) 11:1153. doi: 10.3390/cells11071153
17. Gallo O, Locatello LG, Mazzoni A, Novelli L, Annunziato F. The central role of the nasal microenvironment in the transmission, modulation, and clinical progression of SARS-CoV-2 infection. Mucosal Immunol 2020 14:2 (2020) 14(2):305–16. doi: 10.1038/s41385-020-00359-2
18. Li W, Deng G, Li M, Liu X, Wang Y. Roles of mucosal immunity against mycobacterium tuberculosis infection. Tuberculosis Res Treat (2012) 2012. doi: 10.1155/2012/791728
19. Soumana IH, Linz B, Dewan KK, Sarr D, Gestal MC, Howard LK, et al. Modeling immune evasion and vaccine limitations by targeted nasopharyngeal bordetella pertussis inoculation in mice. Emerging Infect Dis (2021) 27(8):2107–16. doi: 10.3201/eid2708.203566
20. Mettelman RC, Allen EK, Thomas PG. Mucosal immune responses to infection and vaccination in the respiratory tract. Immunity (2022) 55(5):749–80. doi: 10.1016/j.immuni.2022.04.013
21. Holmgren J, Czerkinsky C. Mucosal immunity and vaccines. Nat Med (2005) 11(Suppl 4):S45–53. doi: 10.1038/nm1213
22. Hellwig SMM, van Spriel AB, Schellekens JFP, Mooi FR, van de Winkel JGJ. Immunoglobulin a-mediated protection against bordetella pertussis infection. Infect. Immun (2001) 69(8):4846–50. doi: 10.1128/IAI.69.8.4846-4850.2001
23. Carbonetti NH, Artamonova GV, Andreasen C, Dudley E, Mays RM, Worthington ZEV. Suppression of serum antibody responses by pertussis toxin after respiratory tract colonization by bordetella pertussis and identification of an immunodominant lipoprotein. Infect. Immun (2004) 72(6):3350–8. doi: 10.1128/IAI.72.6.3350-3358.2004
24. Andreasen C, Carbonetti NH. Pertussis toxin inhibits early chemokine production to delay neutrophil recruitment in response to bordetella pertussis respiratory tract infection in mice. Infect. Immun (2008) 76(11):5139–48. doi: 10.1128/IAI.00895-08
25. Henderson MW, Inatsuka CS, Sheets AJ, Williams CL, Benaron DJ, Donato GM, et al. Contribution of bordetella filamentous hemagglutinin and adenylate cyclase toxin to suppression and evasion of interleukin-17-mediated inflammation. Infect. Immun (2012) 80(6):2061–75. doi: 10.1128/IAI.00148-12
26. Kirimanjeswara GS, Agosto LM, Kennett MJ, Bjornstad ON, Harvill ET. Pertussis toxin inhibits neutrophil recruitment to delay antibody-mediated clearance of bordetella pertussis. J Clin Invest (2005) 115(12):3594–6301. doi: 10.1172/JCI24609
27. Gestal MC, Whitesides LT, Harvill ET. Integrated signaling pathways mediate bordetella immunomodulation, persistence, and transmission. Trends Microbiol (2019) 27(2):118–30. doi: 10.1016/j.tim.2018.09.010
28. Valdez HA, Oviedo JM, Gorgojo JP, Lamberti Y, Rodriguez ME. Bordetella pertussis modulates human macrophage defense gene expression. Pathog Dis (2016) 74(6). doi: 10.1093/femspd/ftw073
29. Gorgojo J, Scharrig E, Gómez RM, Harvill ET, Rodríguez ME. Bordetella parapertussis circumvents neutrophil extracellular bactericidal mechanisms. PloS One (2017) 12(1). doi: 10.1371/journal.pone.0169936
30. Hovingh ES, Mariman R, Solans L, Hijdra D, Hamstra HJ, Jongerius I, et al. Bordetella pertussis pertactin knock-out strains reveal immunomodulatory properties of this virulence factor article. Emerging Microbes Infect. (2018) 7(1):39. doi: 10.1038/s41426-018-0039-8
31. Solans L, Locht C. The role of mucosal immunity in pertussis. Front Immunol (2019) 10:3068(JAN). doi: 10.3389/fimmu.2018.03068
32. Le T, Cherry JD, Chang SJ, Knoll MD, Lee ML, Barenkamp S, et al. Immune responses and antibody decay after immunization of adolescents and adults with an acellular pertussis vaccine: The APERT study. J Infect Dis (2004) 190(3):535–44. doi: 10.1086/422035
33. Tomovici A, Barreto L, Zickler P, Meekison W, Noya F, Voloshen T, et al. Humoral immunity 10 years after booster immunization with an adolescent and adult formulation combined tetanus, diphtheria, and 5-component acellular pertussis vaccine. Vaccine (2012) 30(16):2647–53. doi: 10.1016/j.vaccine.2012.02.013
34. Taranger J, Trollfors B, Lagergård T, Sundh V, Bryla DA, Schneerson R, et al. Correlation between pertussis toxin IgG antibodies in postvaccination sera and subsequent protection against pertussis. J Infect Dis (2000) 181(3):1010–3. doi: 10.1086/315318
35. van Twillert I, Han WGH, van Els CACM. Waning and aging of cellular immunity to bordetella pertussis. Pathog Dis (2015) 73(8). doi: 10.1093/femspd/ftv071
36. Munoz FM, Bond NH, Maccato M, Pinell P, Hammill HA, Swamy GK, et al. Safety and immunogenicity of tetanus diphtheria and acellular pertussis (Tdap) immunization during pregnancy in mothers and infants: A randomized clinical trial. JAMA (2014) 311(17):1760–9. doi: 10.1001/jama.2014.3633
37. Raya BA, Srugo I, Kessel A, Peterman M, Vaknin A, Bamberger E. The decline of pertussis-specific antibodies after tetanus, diphtheria, and acellular pertussis immunization in late pregnancy. J Infect Dis (2015) 212(12):1869–73. doi: 10.1093/infdis/jiv324
38. Mills KHG, Ryan M, Ryan E, Mahon BP. A murine model in which protection correlates with pertussis vaccine efficacy in children reveals complementary roles for humoral and cell- mediated immunity in protection against bordetella pertussis. Infect. Immun (1998) 66(2):594–602. doi: 10.1128/iai.66.2.594-602.1998
39. Tuomanen EI, Zapiain LA, Galvan P, Hewlett EL. Characterization of antibody inhibiting adherence of bordetella pertussis to human respiratory epithelial cells. J Clin Microbiol (1984) 20(2):167–70. doi: 10.1128/jcm.20.2.167-170.1984
40. Kirimanjeswara GS, Mann PB, Harvill ET. Role of antibodies in immunity to bordetella infections. Infect. Immun (2003) 71(4):1719–24. doi: 10.1128/IAI.71.4.1719-1724.2003
41. Wolfe DN, Kirimanjeswara GS, Goebel EM, Harvill ET. Comparative role of immunoglobulin a in protective immunity against the bordetellae. Infect. Immun (2007) 75(9):4416–22. doi: 10.1128/IAI.00412f-07
42. van Twillert I, Marinović AAB, Kuipers B, van Gaans-Van Den Brink JAM, Sanders EAM, van Els CACM. Impact of age and vaccination history on long-term serological responses after symptomatic b. pertussis infection, a high dimensional data analysis. Sci Rep (2017) 7. doi: 10.1038/srep40328
43. Solans L, Debrie AS, Borkner L, Aguiló N, Thiriard A, Coutte L, et al. IL-17-dependent SIgA-mediated protection against nasal bordetella pertussis infection by live attenuated BPZE1 vaccine. Mucosal Immunol (2018) 11(6):1753–62. doi: 10.1038/s41385-018-0073-9
44. Locht C, Papin JF, Lecher S, Debrie AS, Thalen M, Solovay K, et al. Live attenuated pertussis vaccine BPZE1 protects baboons against bordetella pertussis disease and infection. J Infect Dis (2017) 216(1):117–24. doi: 10.1093/infdis/jix254
45. Boehm DT, Wolf MA, Hall JM, Wong TY, Sen-Kilic E, Basinger HD, et al. Intranasal acellular pertussis vaccine provides mucosal immunity and protects mice from bordetella pertussis. NPJ Vaccines (2019) 4(1). doi: 10.1038/s41541-019-0136-2
46. Hall JM, Bitzer GJ, DeJong MA, Kang J, Wong TY, Wolf MA, et al. Mucosal immunization with DTaP confers protection against bordetella pertussis infection and cough in sprague-dawley rats. Infect. Immun (2021) 89(12). doi: 10.1128/IAI.00346-21
47. Berstad AKH, Holst J, Frøholm LO, Haugen IL, Wedege E, Oftung F, et al. A nasal whole-cell pertussis vaccine induces specific systemic and cross-reactive mucosal antibody responses in human volunteers. J Med Microbiol (2000) 49(2):157–63. doi: 10.1099/0022-1317-49-2-157
48. Date Y, Ebisawa M, Fukuda S, Shima H, Obata Y, Takahashi D, et al. NALT m cells are important for immune induction for the common mucosal immune system. Int Immunol (2017) 29(10):471–8. doi: 10.1093/intimm/dxx064
49. Kiyono H, Fukuyama S. Nalt-versus peyer’s-patch-mediated mucosal immunity. Nat Rev Immunol (2004) 4(9):699–710. doi: 10.1038/nri1439
50. Dubois V, Locht C. Mucosal immunization against pertussis: Lessons from the past and perspectives. Front Immunol (2021) 12:701285. doi: 10.3389/fimmu.2021.701285
51. Marcellini V, Mortari EP, Fedele G, Gesualdo F, Pandolfi E, Midulla F, et al. Protection against pertussis in humans correlates to elevated serum antibodies and memory b cells. Front Immunol (2017) 8:1158(SEP). doi: 10.3389/fimmu.2017.01158
52. Allen AC, Wilk MM, Misiak A, Borkner L, Murphy D, Mills KHG. Sustained protective immunity against bordetella pertussis nasal colonization by intranasal immunization with a vaccine-adjuvant combination that induces IL-17-secreting T RM cells. Mucosal Immunol (2018) 11(6):1763–76. doi: 10.1038/s41385-018-0080-x
53. Wilk MM, Borkner L, Misiak A, Curham L, Allen AC, Mills KHG. Immunization with whole cell but not acellular pertussis vaccines primes CD4 TRM cells that sustain protective immunity against nasal colonization with bordetella pertussis. Emerging Microbes Infect. (2019) 8(1):169–85. doi: 10.1080/22221751.2018.1564630
54. Wilk MM, Mills KHG. CD4 TRM cells following infection and immunization: Implications for more effective vaccine design. Front Immunol (2018) 9:1860(AUG). doi: 10.3389/fimmu.2018.01860
55. Borkner L, Curham LM, Wilk MM, Moran B, Mills KHG. IL-17 mediates protective immunity against nasal infection with bordetella pertussis by mobilizing neutrophils, especially siglec-f+ neutrophils. Mucosal Immunol (2021) 14(5):1183–202. doi: 10.1038/s41385-021-00407-5
56. Matsui M, Nagakubo D, Satooka H, Hirata T. A novel siglec-f+ neutrophil subset in the mouse nasal mucosa exhibits an activated phenotype and is increased in an allergic rhinitis model. Biochem Biophys Res Commun (2020) 526(3):599–606. doi: 10.1016/j.bbrc.2020.03.122
57. Gestal MC, Howard LK, Dewan K, Johnson HM, Barbier M, Bryant C, et al. Enhancement of immune response against bordetella spp. by disrupting immunomodulation. Sci Rep (2019) 9(1). doi: 10.1038/s41598-019-56652-z
58. Holubova J, Stanek O, Juhasz A, Hamidou Soumana I, Makovicky P, Sebo P. The fim and FhaB adhesins play a crucial role in nasal cavity infection and bordetella pertussis transmission in a novel mouse catarrhal infection model. PloS Pathog (2022) 18(4):e1010402. doi: 10.1371/journal.ppat.1010402
59. Soumana IH, Dewan KK, Linz B, Rivera I, Ma L, Howard LK, et al. Modeling the catarrhal stage of bordetella pertussis upper respiratory tract infections in mice. DMM Dis Models Mech (2022) 15(5). doi: 10.1242/dmm.049266
60. Naninck T, Contreras V, Coutte L, Langlois S, Hébert-Ribon A, Pelletier M, et al. Intranasal inoculation with bordetella pertussis confers protection without inducing classical whooping cough in baboons. Curr Res Microbial Sci (2021) 2. doi: 10.1016/j.crmicr.2021.100072
61. Scanlon KM, Snyder YG, Skerry C, Carbonetti NH. Fatal pertussis in the neonatal mouse model is associated with pertussis toxin-mediated pathology beyond the airways. Infect. Immun (2017) 85(11). doi: 10.1128/IAI.00355-17
62. Gestal MC, Johnson HM, Harvill ET. Immunomodulation as a novel strategy for prevention and treatment of bordetella spp. infections. Front Immunol (2019) 10:2869. doi: 10.3389/fimmu.2019.02869
Keywords: pertussis, whooping cough, mucosal immunity, sterilizing immunity, animal models, waning immunity, pulmonary disease
Citation: Caulfield AD, Callender M and Harvill ET (2023) Generating enhanced mucosal immunity against Bordetella pertussis: current challenges and new directions. Front. Immunol. 14:1126107. doi: 10.3389/fimmu.2023.1126107
Received: 17 December 2022; Accepted: 08 February 2023;
Published: 21 February 2023.
Edited by:
Marcela Pasetti, University of Maryland, United StatesReviewed by:
Elena Mitsi, Department of Paediatrics, University of Oxford, United KingdomCopyright © 2023 Caulfield, Callender and Harvill. This is an open-access article distributed under the terms of the Creative Commons Attribution License (CC BY). The use, distribution or reproduction in other forums is permitted, provided the original author(s) and the copyright owner(s) are credited and that the original publication in this journal is cited, in accordance with accepted academic practice. No use, distribution or reproduction is permitted which does not comply with these terms.
*Correspondence: Amanda D. Caulfield, YW1hbmRhLmNhdWxmaWVsZEB1Z2EuZWR1