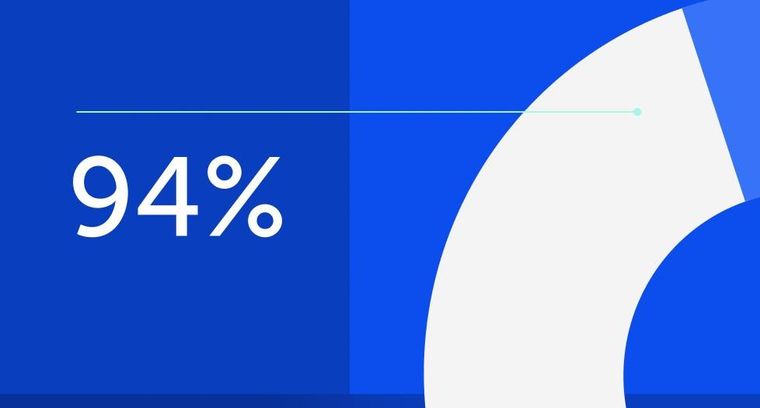
94% of researchers rate our articles as excellent or good
Learn more about the work of our research integrity team to safeguard the quality of each article we publish.
Find out more
REVIEW article
Front. Immunol., 09 March 2023
Sec. Inflammation
Volume 14 - 2023 | https://doi.org/10.3389/fimmu.2023.1123853
This article is part of the Research TopicCrosstalk between Peripheral and Local Immune Response in the Pathophysiology of Stroke and Neurodegeneration DiseasesView all 30 articles
Glioma is a mixed solid tumor composed of neoplastic and non-neoplastic components. Glioma-associated macrophages and microglia (GAMs) are crucial elements of the glioma tumor microenvironment (TME), regulating tumor growth, invasion, and recurrence. GAMs are also profoundly influenced by glioma cells. Recent studies have revealed the intricate relationship between TME and GAMs. In this updated review, we provide an overview of the interaction between glioma TME and GAMs based on previous studies. We also summarize a series of immunotherapies targeting GAMs, including clinical trials and preclinical studies. Specifically, we discuss the origin of microglia in the central nervous system and the recruitment of GAMs in the glioma background. We also cover the mechanisms through which GAMs regulate various processes associated with glioma development, such as invasiveness, angiogenesis, immunosuppression, recurrence, etc. Overall, GAMs play a significant role in the tumor biology of glioma, and a better understanding of the interaction between GAMs and glioma could catalyze the development of new and effective immunotherapies for this deadly malignancy.
Glioblastoma (GBM), also known as IV grade glioma, is the most common and malignant neoplasm in the adult central nervous system (CNS). Despite surgical resection, targeted radiotherapy, combined chemotherapy, and newer developed treatments like tumor-treating fields (TTF), the prognosis of GBM patients remains very poor (1). The median survival time of adult GBM patients is less than 15 months with traditional treatment and less than 20 months with TTF treatment (1, 2). This poor prognosis is attributed to the highly aggressive nature of GBM, which is characterized by progressive growth, diffuse invasiveness, and frequent resistance to chemotherapy (1). Besides the inability to completely resect the tumor body due to invasive growth of the tumor and cell heterogeneity of the GBM stem cells (GSCs) in the glioma, tumor-associated immune cells significantly contribute to the high malignancy and proliferation of GBM (3). These immune cells as well as other noncancerous cells such as normal and reactive astrocytes, GSCs, fibroblasts, vascular pericytes, and endothelial cells form the tumor microenvironment (TME), which assists tumor development by releasing various cytokines, chemokines, growth factors, and other hormones (4–6). In recent years, researchers have conducted comprehensive studies on TME using genomics, proteomics, and other technologies.
Although many types of immune cells within the lymphoid lineages have been detected in the TME, glioma-associated macrophages and microglia (GAMs) are the predominant immune population in the solid GBM, comprising up to one-third of the tumor mass (7–9). This is primarily due to the critical role resident macrophages and microglia play in the innate immunity of the brain, an organ known for its immune privilege (9). Recently, T-cell-based immunotherapies have demonstrated curative potential in several non-intracranial malignancies, such as B-cell acute lymphoblastic leukemia and advanced renal-cell carcinoma (10–12). However, although T-cells can infiltrate the tumor body and surrounding areas of the GBM, the application of T-cell rejuvenation strategies for GBM has produced contradictory results due to the low number of T-cells and the lack of key stimulators (13). Although immune checkpoint blockade regimens including cytotoxic T lymphocyte antigen 4 and programmed cell death 1 (PD-1) have been employed, they have not demonstrated a significant improvement in the survival time of GBM patients (13, 14). Dominant GAMs in GBM mediate low levels of proinflammatory factors and a lack of key T-cell costimulatory factors, leading to a weak response state of T-cells in GBM (15). Additionally, the presence of GAMs in GBM is a known indicator of poor prognosis (8, 9), as GAMs are biased toward M2 polarization, which promotes heterogeneous differentiation, diffusion growth, and tumor recurrence (7, 16). Nonetheless, GAMs possess a feature of plasticity, which highlights the potential of developing new therapeutic methods based on their metabolism and genome regulation. In this review, we will summarize the origin of GAMs, their relationship with GBM, and recently developed immunotherapies targeting GAMs.
Microglia are a critical innate immune component in the CNS and represent 10-15% of all glial cells (17). Due to their phagocytic activity and origin with peripheral myeloid cells, microglia are considered the tissue resident macrophages of the CNS (17, 18). In the physiological state, the number of macrophages in the CNS is much less than that of microglia (19), and they are mainly distributed in the perivascular space, meninges, and organs surrounding the ventricles and choroid plexus, and are rare in brain parenchyma (19). These macrophage populations are highly heterogeneous and can be replaced to a certain extent. In pathological conditions such as injury, infection, degenerative diseases, and tumors, microglia, and macrophages display different polarization states and express specific markers (19, 20).
Microglia derive from hematopoietic precursor cells of the yolk sac during early embryonic development (17, 21), and Runt related transcription factor-1 (Runx-1) and colony stimulating factor-1(CSF-1) play critical roles in their development (21). Due to their powerful phagocytic function, microglia can engulf abnormal entities in the CNS, such as tumor cells, necrotic cell fragments, and pathogens (21). In addition, microglia perform immune regulatory functions, interact with neurons and glial cells, and promote angiogenesis (22). Therefore, microglia not only effectively respond to CNS damage, infection, and mutation, but also play a critical role in the development and homeostatic maintenance of brain (21, 22). Microglia rapidly shift from a ramified resting state to an amoeboid-like activated state and release reactive oxygen species (ROS), proinflammatory cytokines, and chemokines in response to exogenous and endogenous stimuli, such as infection and injury (23). This effect is mainly achieved through pattern recognition receptors (PRRs) expressed in microglia, which recognize pathogen-associated molecular patterns (PAMPs) and damage-associated molecular patterns (DAMPs) (24, 25). Recent studies have also shown that microglia play an important role in synaptic formation in the mature brain (26), serving as intermediates for information exchange through hemichannels and gap junctions with neurons and other glial cells (26). Moreover, during the development of primary tumors, activated microglia can kill tumor cells by secreting proinflammatory cytokines and other factors (9). However, with tumor progression, the phenotype and function of microglia change, causing damage to normal neural structures and promoting tumor growth (9, 27). This topic will be discussed in detail later.
Due to the lack of reliable in vivo and in vitro experimental models of microglia, the investigation of microglia in the context of tumors remains limited. Alongside primary microglia cells extracted from experimental animals or human brains, immobilized murine (BV-2) and human (HMO6) microglia cell lines have been developed (28). Nevertheless, it is crucial to note that there exist differences between the primary brain-derived microglia and the immortalized BV-2 cell line at the transcriptional level (29). Recent advancements in flow sorting technology and transcriptome sequencing, several specific marker molecules of primary microglia have been identified. For example, some transcription factors, including Rhox5, E2f6, Hoxc6, and Ppargc1b are exclusively expressed in microglia (30, 31). Furthermore, certain membrane proteins, Lrp8 and Lpcat3, which are associated with lipid metabolism, and ion transporters, like Slco4a1 and Slc30a5, are unique to microglia compared with macrophages (30, 31). Potential markers that distinguish CNS-derived microglia and BMDMs are systematically summarized in Table 1.
Brain-infiltrating bone marrow-derived macrophages (BMDMs) originate from hematopoietic stem cells (32) and infiltrate the brain parenchyma in large numbers after the development of GBM due to the destruction of blood-brain barrier and release of multiple chemokines by the tumor tissue (33, 34). They are mainly located in perivascular and necrotic regions to address the ischemic regions of the tumor (33). Although microglia and infiltrating BMDMs have distinct origins, they perform similar immune regulatory functions and express several common markers, such as ionized calcium-binding adapter molecule 1 (IBA1), CD11b, CD68, CX3C chemokine receptor 1 (CX3CR1) (9, 35, 36). Among these markers, IBA-1 and CX3CR1 were thought to be specific to microglia, however, subsequent studies confirmed that they were also expressed by BMDMs (22, 37). In contrast, major histocompatibility complex (MHC) class II and subsequent Sall1 are specific to microglia and can be used to differentiate between microglia and macrophages in the brain parenchyma (22, 38). In addition, transmembrane protein 119 (TMEM119) is a recently discovered marker with high specificity for microglia that can distinguish microglia and macrophages in both human and mouse models (35, 39). CD49D is specifically expressed by BMDMs infiltrating malignant brain tumors in mice and humans, but not by microglia (33). C-C chemokine receptor-2 (CCR2) was previously considered as another specific marker to differentiate macrophages in TME, but subsequent studies found that CCR2 is also expressed by microglia, particularly those activated by interaction with glioma cells (40). Previously, CD45 has been used to distinguish between resident microglia (CD45low) and infiltrating macrophages (CD45high) (41), and in combination with CD11b, microglia and macrophages in rodent and human GBM can be defined as CD11b+/CD45low and CD11b+/CD45high populations, respectively (42). However, CD45 expression increases in microglia activated in the TME, which adds complicates the identification of microglia and macrophages in tumors (42). To date, no lineage-specific markers have been developed to accurately distinguish these two cell populations, which has made it challenging to assess the specific role of each cell type in tumor development.
Mills et al. were the first to define macrophages as M1 polarization type for pro-inflammatory (antitumor) and M2 polarization type for anti-inflammatory (protumor), referring to the dichotomy of CD4+ T cell function into T helper (Th) 1 and Th2 lineages (43). Ponomarev et al. subsequently verified the polarization of microglia in the rodent CNS (44). Numerous CNS pathological events, such as tumor occurrence, injury, microbial infection, and degenerative diseases, can cause the polarization of microglia/macrophages (33, 45–47). For instance, microglia, which account for the majority of GBM, can be polarized into the M1 phenotype under the stimulation of lipopolysaccharides (LPS) or interferon-γ (IFN-γ) (13). M1 type microglia reactively express co-stimulatory molecules such as tumor necrosis factor-alpha (TNF-α), interleukin-6 (IL-6), and chemokine (C-X-C motif) ligand 10 (CXCL10), and high-level of MHC class II, which endows them with the function of antigen presentation (13). In the context of glioma, M1 microglia play an anti-tumorigenic role through antigen presentation mediated adaptive immunity response (phagocytosis of tumorigenic cells) and secretion of proinflammatory factors (8, 48). Inflammatory factors such as TNF-α further recruit peripheral macrophages into the tumor (49). M1 microglia/macrophages have the classic activated phenotype, and their activated receptors and functions are relatively clear. The polarization of M2 microglia and macrophages is strictly regulated and presents a highly dynamic state, often followed by M1 polarization (49, 50). Type II inflammatory factors such as IL-4, IL-10, and IL-13 generally mediate M2 polarization, which can prevent further tissue damage by secreting multiple anti-inflammatory factors to downregulate the inflammatory response (51, 52). In the context of glioma, M2 microglia promote tumor development (53). M2-polarized microglia/macrophages are differentiated by the co-expression of surface markers such as CD163, CD204, CD206 as well as arginase (53). We have a systematic list of markers for M1 and M2 microglia/macrophages in Table 1. M2 microglia/macrophages can be further divided into several subtypes (M2a, M2b, M2c) based on their expression of different transcription factors, effector functions, and secretion of cytokines and chemokines (54). M2a and M2b microglia/macrophages are responsible for Th2 activation and immunoregulation (55, 56), while the M2c subtype attenuates inflammatory response, promotes matrix deposition and tissue remodeling, significantly promoting tumor development in the neoplastic context (57–59).
Recent single-cell sequencing studies on GBM suggest that individual cells can express genes promoting inflammation (M1) and genes promoting immunosuppression (M2) (60, 61). These findings challenge the precision of the conventional M1/M2 typing of GBMs and suggest that the polarization may be a highly continuous process in the context of GBM, where GAMs are highly plastic (8). Furthermore, there are likely unclearly defined GAM subtypes having important specific functions in human and rodent GBM. For example, the nonpolarized M0 subtype may have been overlooked to some extent, even though it represents a weakened M2 subtype (62). Accurate classification of GAMs is critical for guiding the development of therapeutic drugs, but it is challenging to establish a systematic microglia/macrophage classification system. Thus, the classical subtype classification model still holds important reference value.
Glioma-associated microglia/macrophages (GAMs) are not passive bystanders in the tumor microenvironment, but actively evolve with tumors through feedback and feedforward mechanisms (Figure 1). While most of the mechanisms involved are feedback regulation mechanisms, some feedforward mechanisms also play important roles in the recruitment of GAMs by glioma cells. For instance, glioma cells can secrete versican, which promotes the activation of microglia through the Toll-like receptor 2 (TLR2) signaling pathway (63, 64). Some researchers even propose the hypothesis that the immune system does not recognize malignant tumor cells as invaders in the CNS, but rather helps them infiltrate and grow (65). These effects are largely due to the recruitment of microglia/macrophages by tumor cells. After being polarized into a tumor-promoting M2 phenotype, GAMs assist tumor development by promoting invasive growth, inducing angiogenesis, interacting with GSCs, and mediating the formation of an inhibitory TME (9).
Figure 1 A schematic diagram outlining the interaction between glioma cells and glioma-associated macrophages and microglia (GAMs). Under the action of various cytokines and chemokines secreted by tumor cells, resident microglia, and peripheral blood-derived macrophages are recruited into the tumor parenchyma. Activated GAMs interact with tumor cells to promote glioma growth and invasion through various mechanisms such as angiogenesis promotion, GSCs proliferation, epithelial-mesenchymal transformation, and tumorigenic immune regulation.
A large number of previous studies have demonstrated that glioma cells secrete different chemokines, which serve as chemoattractants and mediate the recruitment of GAMs. These chemokines include monocyte chemoattractant protein (MCP)-1 (alternative name: C-C motif ligand 2 (CCL2)) (66), MCP-3 (67), stroma-derived factor (SDF)-1 (alternative name: CXCL12) (68), lysyl oxidase (LOX) (69), macrophage colony-stimulating factor (M-CSF) (70), and glial cell–derived neurotrophic factor (GDNF) (71). The role of MCP-1 in GAMs has been verified by various in vivo and in vitro studies, where the MCP-1 expression level is highly correlated with the grade of glioma (66). A recent study indicated that the cooperation between β- Catenin and MCP-1 may be responsible for the rapid and highly heterogeneous growth of Isocitrate dehydrogenase wildtype GBM (72). M-CSF is another important chemokine, which not only promotes the mobility of GAMs but also mediates the M2 polarization of GAMs (73). Gliomas having a phosphatase and tensin homolog (PTEN) deletion highly express LOX, which activates the β 1 integral/proline-rich tyrosine kinase 2 pathway in GAMs, aiding their recruitment (69).
In recent years, researchers have suggested alternative factors that might impact the recruitment of GAMs and M2 polarization, such as peritumoral hypoxia microenvironment-induced factors and GSCs (74). Hypoxia may be the most critical regulatory factor for the recruitment of GAMs, as a large portion of the glioma is hypoxic and harbors large numbers of M2 polarization-type GAMs. Recent studies suggest that hypoxia may affect the expression pattern of chemokines, especially in perivascular niches. For example, Guo et al. revealed that TGF-α mediated upregulation of periostin (POSTN) in the peritumoral region of the glioma significantly promotes the recruitment of GAMs (75). Another recent study showed that arginine methyltransferases (PRMTs) enhance the recruitment of GAMs by enhancing hypoxia-inducible factor-1 mediated hypoxia, thus promoting glioma progression (76). Specific chemokines secreted by GSCs may recruit certain GAMs subtypes. For example, POSTN secreted by GSCs specifically recruits M2 GAMs through integrin αvβ3 signaling pathway (53). In addition, some cytokines with undefined functions have also been reported to participate in the recruitment of GAMs. For example, dual function cytokine IL-33, secreted at the nucleus of gliomas, was recently demonstrated to recruit and activate circulating and resident innate immune cells (77).
The tumor-promoting effects of GAMs have been demonstrated in organotypic brain tumor-slice cultures as well as several in vivo models (78–80). Co-culture of glioma cells with microglia extracted from mouse brain significantly increased glioma cell migration, while the deprivation of microglia significantly promoted tumor growth and invasion (78, 79). A range of anti-inflammatory and pro-tumoral factors secreted by GAMs have been identified as playing important roles in glioma cell invasion, including transforming growth factor beta (TGF-β), epidermal growth factor (EGF), IL-6, IL-1β, stress-inducible protein-1 (STI-1), matrix metallopeptidase-2 (MMP-2), and MMP-9 (27). Among these, MMP-2 and MMP-9 are important effector molecules that enhance glioma cell invasiveness by breaking down extracellular matrix (ECM) components such as collagen and elastin. MMP-2 expression is positively correlated with glioma invasiveness and poor patient prognosis (81). TGF-β, an inhibitory immune regulatory factor, is the most widely explored factor in the mechanism of invasive growth of glioma. TGF- β released from GAMs has been demonstrated to promote the secretion of MMP-2 and MMP-9, resulting in enhanced GBM invasiveness (82). Recent studies have revealed that MMP-2 may be an important downstream molecule of CCL5 in promoting the migratory and invasive activities of GBM (83). MMP-9 has also been reported to be an effector molecule in the tumorigenic infiltration of GBM mediated by GAMs (84). GBM released CCL2 has been reported to upregulate IL-6 expression, which is responsible for GBM invasiveness in a TLR4-dependent fashion (85). The expression level of IL-6 is highly correlated with the pathologies of GBM patients (86). EGF is considered a promoter of GAM-mediated tumor invasiveness, as it is not detectable in the supernatant or cell lysate of glioma cells cultured separately (70). EGF primarily binds to the surface of GBMs to promote tumor invasion. Amplification of the EGFR gene and its truncation mutant are present in over half of primary GBM and are indicative of highly aggressive tumors (87). It should be noted that the regulatory pathways discussed above do not strictly follow the effect of GAMs on GBM in the context of GBM. Many regulatory factors can also be released by tumor cells and influence GAMs.
Angiogenesis is a critical factor in the growth and progression of glioma. GAMs extracted from GL261 gliomas have been shown to release a plethora of proangiogenic molecules, including vascular endothelial growth factor (VEGF) and CXCL2 (88). Depletion of resident microglia significantly reduces tumor vessel count, and a high spatial correlation between GAMs and tumor neovascularization has been reported (88). For instance, specific GAMs that are absent in normal brain tissue were directly detected in the perivascular niche (merged with CD31+ vessels) (89). Furthermore, studies have not only verified the direct contact effect of GAMs with blood vessels around and inside the tumor using allografted mice but have also detected elevated levels of various angiogenesis-inducing factors, such as VEGF, VEGFR1, CCR2, CXCR4, CCL2/5, and CXCL2/10/14 (14, 90–93). Recent research has confirmed the strong correlation between GAMs and tumor blood vessels in GBM patients and observed the restructuring of the blood vessel architecture, indicating the potential of anti-angiogenic therapy in the treatment of GBM (88). Consistent with this, VEGF receptor (VEGFR) blocker Sunitinib and VEGF inhibitor Bevacizumab have been shown to promote survival by reducing tumor angiogenesis in the mouse GBM model (94).
The immune-escape mechanism of GBM relies on two main factors: the intrinsic characteristics of neoplastic cells and the immunosuppressive TME mediated by GAMs. Glioma cells are difficult to identify by the immune due to their downregulation of human leukocyte antigen (HLA) molecules that cover the surface specific tumor antigen molecules (95). However, the immunosuppressive TME has been recognized as a more significant contributor to promoting tumor immune evasion, facilitating its growth, invasion, and recurrence (78, 79). GAMs, especially the type M2 subtype, are considered major contributors to TME due to their secretion of type II immune factors such as TGF-β, IL-4, and IL-10 (4, 6). These cytokines upregulate transcription factors such as signal transducer and activator of transcription 3 (STAT3) in glioma cells, which subsequently triggers tumorigenic immune responses (27). STAT3 plays a vital role in the interaction between tumor cells and GAMs, and its activation significantly enhances tumor-promoting immune regulation while inhibiting tumor-killing immune response (96, 97). Several studies have demonstrated that glioma cells secrete S100 calcium binding protein B (S100B), which promotes M2 polarization of GAMs through the receptor for advanced glycation end products (RAGE)-STAT3 signaling pathway (98). Additionally, other growth factors secreted by type M2 GAMs, such as platelet derived growth factor (PDGF), EGF, and fibroblast growth factor-2 (FGF-2), have been reported to activate STAT3, promoting GBM progression (99–101). In vitro studies have shown that conditioned medium derived from glioma cells can activate the STAT3 pathway of microglia, promoting their polarization towards the M2 subtype and secretion of IL-6 and IL-10 (102).
A recent study found that blocking the breakdown of interferon gamma inducible protein 16 (IFI16) with drugs can activate the STAT3 signaling pathway (103). This pathway is crucial for GBM progression because it promotes cell proliferation, invasion, and regulation of the TME. Moreover, high levels of phosphorylated-STAT3 (pSTAT3) are associated with more severe gliomas and poorer patient outcomes (104–106). This is likely because pSTAT3 can suppress the immune system, leading to greater resistance to standard cancer treatments and an increased risk of tumor recurrence (105, 106).
GCSs are a group of cells that are resistant to chemotherapy and contribute to tumor growth and recurrence (107–109). They share stem cell-like characteristics, which enable them to regenerate and differentiate into different cell types (108, 109). This allows them to continuously generate new tumor cells at the site of the tumor. GSCs are similar to NSCs in terms of their phenotypic characteristics. For example, they can form neurospheres in vitro and express common markers such as nestin, Sox2, and Musashi-1 (110, 111).
GSCs have low mitotic activity, which makes them resistant to therapies directed at actively dividing cells, such as temozolomide (TMZ)-based chemotherapy, and enables them to cause tumor recurrence (112, 113). They also have low expression of molecules that present tumor antigens to CD8+ T cells, and immune checkpoint pathways are often activated in glioma cells (114). These mechanisms help tumor cells evade immune surveillance. GSCs interact with various components in TME to maintain their drug resistance and tumorigenicity. They are located in specific niches where they are protected from therapy exposure and niche-specific factors (95, 115, 116). GAMs mainly accumulate in perivascular and perinecrotic hypoxic niches, where they initiate interactions with SGCs to affect glioma prognosis (74, 95). GSCs secrete chemokines (such as VEGF, CCL2, CCL5, and CCL7), to recruit GAMs to tumor mass and induce M2 type polarization of GAMs, aiding their transformation and proliferation by building a tumorigenic TME. Apart from GSCs-secreted chemokines, GSCs also exhibit higher levels of neurotensin than those in non-GSC glioma cells (27, 117). In vitro studies have shown that GSCs recruit GAMs more effectively than glioma cell lines. Moreover, a positive correlation between GAMs and GSCs has also been reported (118). These findings emphasize the importance of GSCs for GAMs recruitment.
Conversely, GAMs have been shown to play a role in promoting GSCs (119–122). Research by Wang et al. demonstrated that IL-6, which is secreted by microglia, can function as a growth factor for GSCs and enhance their biological function (122). It is important to note that only GAMs, not the naïve resident microglia, can promote GSC amplification (123). Interestingly, microglia from healthy individuals can even suppress glioma growth by expressing IL-8 and MCP-1 (123). In general, GAMs and GSCs interact extensively in glioma, jointly regulating tumor progression, recurrence, and drug resistance.
The rapid development of new tumor treatment technologies and a deeper understanding of CNS have led to significant progress in immunotherapy for glioma (124). Some of these advancements have already undergone clinical trials, such as dendritic cell vaccine (DC Vax-L), chimeric antigen receptor T cell therapy (CAR-T), and immune checkpoint inhibitors (1–3, 125, 126). As highlighted earlier, GAMs play a critical role in glioma progression, invasion, recurrence, and drug resistance, making them a promising intervention target for glioma immunotherapy. The basic concept of GAMs targeted immunotherapy involves inhibiting the recruitment and infiltration of GAMs, inhibiting the polarization of the M2 phenotype, or eliminating the M2 phenotype and promoting the transformation of the M2 and M0 phenotypes into M1 through reprogramming and other means, thereby restoring their tumor-killing effect. Table 2 summarizes some representative immunotherapy approaches targeting GAMs.
Several antibiotics and inflammatory cytokines/substances have been reported to regulate the origin and activation of GAMs, particularly microglia, to exert effective anti-tumor effects. For instance, minocycline, a broad-spectrum tetracycline antibiotic, has been shown to reduce microglia activation and infiltration by mitigating matrix degradation, thereby exhibiting anti-tumor effects (64). Similarly, several preclinical studies have demonstrated that amphotericin B, a polyene antifungal drug, can inhibit glioma development by activating M1 GAMs (123). Researchers found that treatment with amphotericin B promotes M1 polarization of GAMs by inducing TLR signaling pathways and significantly prolongs the survival time in the mouse model of glioma (123, 144). Furthermore, IFN-γ, IL-12, LPS, and oligodeoxynucleotides containing CpG motifs (CpGODN) have been shown to increase the M1 polarization of GAMs, leading to the elimination of tumor progression in vivo (138–141). Recently, a phase II clinical trial for recurrent GBM has been conducted using combined immunotherapy including IL-12 gene-regulated therapy (130).
CSF-1 (M-CSF)/CSF-1R signal is considered a key factor in GAMs recruitment and M2 polarization. Therefore, researchers have explored drugs targeting this pathway. Treatment with the anti-CSF-1R antibody pexidartinib (PLX3397) significantly reduces GL261-associated GAM infiltration and inhibits M2 polarization, thereby inhibiting glioma growth (135). PLX3397 treatment significantly prolonged the survival time of mice with glioma. However, in the phase II clinical trial, PLX3397 as a monotherapy failed to affect recurrent GBM patients (128), mainly due to drug resistance. This highlights the need for combination therapy. Similarly, the application of another anti-CSF-1R antibody, Emactuzumab (RG7155), also failed to achieve the therapeutic effect, likely due to reactive overproduction of IL-4 by glioma cells (129). In addition, treatment with CSF1R inhibitors such as AFS98 and BLZ945 increased the expression of M2 GAM markers, but they did not inhibit glioma growth as single agents (137).
SDF-1 (CXCL12) is another factor crucial in GAMs recruitment, especially under normoxic conditions. The Food and Drug Administration (FDA)-approved drug for the treatment of multiple myeloma and lymphoma, SDF-1 inhibitor Plerixafor, is currently being evaluated in two clinical trials for its potential therapeutic effect against glioma (132, 133). As mentioned earlier, STAT3 is the central transcription factor mediating the M2 polarization of GAMs. WP1066 has been reported to inhibit the growth and recurrence of glioma by suppressing the protein synthesis of STAT3 (142). John de Groot et al., in their recently concluded Phase I clinical trial of WP1066, have determined the maximum allowable dose of WP1066 to be 8 mg/kg (134).
Microglia/macrophages exhibit strong phagocytic activity. However, in glioma, the phagocytosis of GAMs is greatly reduced, due to the high expression of CD47 along with signal regulatory protein alpha (SIRPa) on the surface of GAMs (145, 146). Therefore, a therapeutic approach involving a blockade of this signaling pathway using anti CD47 antibody to restore the phagocytic activity of GAMs has been proposed (145, 146). Recently, Zhou et al. have developed a novel nano-capsule loaded with anti-CD47 antibodies, which could prove to be useful in testing the anti-tumor potential of this therapeutic approach (143).
Recent studies have shown that olfactomedin Like 3 (OLFML3) exhibits an anti-glioma effect by regulating GAMs infiltration under the influence of the biological clock, and a positive correlation between the survival of GBM patients and the expression level of OLFML3 has been reported (147).
Although researchers have extensively studied the origin, evolution, recruitment, and other mechanisms of microglia/macrophages, the role of GAMs in TME is still poorly understood. This is because the underlying mechanisms do not act in isolation in the tumor context. Glioma cells and various components of the TME form a complex interaction network. Although novel therapies are being developed, GBM recurrence remains a challenge. A deeper understanding of various aspects of GAM biology may provide useful insights for the development of effective strategies for glioma immunotherapy. Moreover, understanding the role of the TME in the development and evolution of tumorigenic cells from a broader perspective, rather than limiting the focus to glioma cells and their transformation, would provide a holistic perspective. For example, the niche of GSCs, which influences tumor diversity and drug resistance, is of great research significance. In the future, the combined treatment with chemotherapy drugs as well as monotherapies involving immunotherapeutic strategies may prove useful in improving the life quality of glioma patients.
Conceived and designed the review: CYX. Wrote and revise the paper: CL and NW. All authors contributed to the article and approved the submitted version.
The authors declare that the research was conducted in the absence of any commercial or financial relationships that could be construed as a potential conflict of interest.
All claims expressed in this article are solely those of the authors and do not necessarily represent those of their affiliated organizations, or those of the publisher, the editors and the reviewers. Any product that may be evaluated in this article, or claim that may be made by its manufacturer, is not guaranteed or endorsed by the publisher.
1. Omuro A, DeAngelis LM. Glioblastoma and other malignant gliomas: A clinical review. Jama (2013) 310(17):1842–50. doi: 10.1001/jama.2013.280319
2. Thakkar JP, Dolecek TA, Horbinski C, Ostrom QT, Lightner DD, Barnholtz-Sloan JS, et al. Epidemiologic and molecular prognostic review of glioblastoma. Cancer epidemiol Biomarkers Prev (2014) 23(10):1985–96. doi: 10.1158/1055-9965.Epi-14-0275
3. Lah TT, Novak M, Breznik B. Brain malignancies: Glioblastoma and brain metastases. Semin Cancer Biol (2020) 60:262–73. doi: 10.1016/j.semcancer.2019.10.010
4. Dapash M, Hou D, Castro B, Lee-Chang C, Lesniak MS. The interplay between glioblastoma and its microenvironment. Cells (2021) 10(9). doi: 10.3390/cells10092257
5. Huang S, Song Z, Zhang T, He X, Huang K, Zhang Q, et al. Identification of immune cell infiltration and immune-related genes in the tumor microenvironment of glioblastomas. Front Immunol (2020) 11:585034. doi: 10.3389/fimmu.2020.585034
6. Yekula A, Yekula A, Muralidharan K, Kang K, Carter BS, Balaj L. Extracellular vesicles in glioblastoma tumor microenvironment. Front Immunol (2019) 10:3137. doi: 10.3389/fimmu.2019.03137
7. Vinnakota K, Hu F, Ku MC, Georgieva PB, Szulzewsky F, Pohlmann A, et al. Toll-like receptor 2 mediates Microglia/Brain macrophage Mt1-mmp expression and glioma expansion. Neuro-oncology (2013) 15(11):1457–68. doi: 10.1093/neuonc/not115
8. Szulzewsky F, Pelz A, Feng X, Synowitz M, Markovic D, Langmann T, et al. Glioma-associated Microglia/Macrophages display an expression profile different from M1 and M2 polarization and highly express gpnmb and Spp1. PloS One (2015) 10(2):e0116644. doi: 10.1371/journal.pone.0116644
9. Roesch S, Rapp C, Dettling S, Herold-Mende C. When immune cells turn bad-Tumor-Associated Microglia/Macrophages in glioma. Int J Mol Sci (2018) 19(2). doi: 10.3390/ijms19020436
10. Parker KR, Migliorini D, Perkey E, Yost KE, Bhaduri A, Bagga P, et al. Single-cell analyses identify brain mural cells expressing Cd19 as potential off-tumor targets for car-T immunotherapies. Cell (2020) 183(1):126–42.e17. doi: 10.1016/j.cell.2020.08.022
11. Davila ML, Riviere I, Wang X, Bartido S, Park J, Curran K, et al. Efficacy and toxicity management of 19-28z car T cell therapy in b cell acute lymphoblastic leukemia. Sci Trans Med (2014) 6(224):224ra25. doi: 10.1126/scitranslmed.3008226
12. Xu Y, Miller CP, Warren EH, Tykodi SS. Current status of antigen-specific T-cell immunotherapy for advanced renal-cell carcinoma. Hum Vaccines immunotherapeutics (2021) 17(7):1882–96. doi: 10.1080/21645515.2020.1870846
13. Wei J, Chen P, Gupta P, Ott M, Zamler D, Kassab C, et al. Immune biology of glioma-associated macrophages and microglia: Functional and therapeutic implications. Neuro-oncology (2020) 22(2):180–94. doi: 10.1093/neuonc/noz212
14. Richard SA. The pivotal immunoregulatory functions of microglia and macrophages in glioma pathogenesis and therapy. J Oncol (2022) 2022:8903482. doi: 10.1155/2022/8903482
15. Sahin A, Sanchez C, Bullain S, Waterman P, Weissleder R, Carter BS. Development of third generation anti-egfrviii chimeric T cells and egfrviii-expressing artificial antigen presenting cells for adoptive cell therapy for glioma. PloS One (2018) 13(7):e0199414. doi: 10.1371/journal.pone.0199414
16. Pyonteck SM, Akkari L, Schuhmacher AJ, Bowman RL, Sevenich L, Quail DF, et al. Csf-1r inhibition alters macrophage polarization and blocks glioma progression. Nat Med (2013) 19(10):1264–72. doi: 10.1038/nm.3337
17. Milligan CE, Cunningham TJ, Levitt P. Differential immunochemical markers reveal the normal distribution of brain macrophages and microglia in the developing rat brain. J Comp Neurol (1991) 314(1):125–35. doi: 10.1002/cne.903140112
18. McLaurin J, Almazan G, Williams K, Antel JP. Immortalization and characterization of rat microglial cells. Neuropathol Appl Neurobiol (1995) 21(4):302–11. doi: 10.1111/j.1365-2990.1995.tb01064.x
19. Li Q, Barres BA. Microglia and macrophages in brain homeostasis and disease. Nat Rev Immunol (2018) 18(4):225–42. doi: 10.1038/nri.2017.125
20. Yang L, Zhou Y, Jia H, Qi Y, Tu S, Shao A. Affective immunology: The crosstalk between microglia and astrocytes plays key role? Front Immunol (2020) 11:1818. doi: 10.3389/fimmu.2020.01818
21. Ginhoux F, Greter M, Leboeuf M, Nandi S, See P, Gokhan S, et al. Fate mapping analysis reveals that adult microglia derive from primitive macrophages. Science (2010) 330(6005):841–5. doi: 10.1126/science.1194637
22. Harry GJ. Microglia during development and aging. Pharmacol Ther (2013) 139(3):313–26. doi: 10.1016/j.pharmthera.2013.04.013
23. Fetler L, Amigorena S. Neuroscience. brain under surveillance: The microglia patrol. Science (2005) 309(5733):392–3. doi: 10.1126/science.1114852
24. Prionisti I, Bühler LH, Walker PR, Jolivet RB. Harnessing microglia and macrophages for the treatment of glioblastoma. Front Pharmacol (2019) 10:506. doi: 10.3389/fphar.2019.00506
25. RodrÍguez E, Schetters STT, van Kooyk Y. The tumour glyco-code as a novel immune checkpoint for immunotherapy. Nat Rev Immunol (2018) 18(3):204–11. doi: 10.1038/nri.2018.3
26. Kim HJ, Cho MH, Shim WH, Kim JK, Jeon EY, Kim DH, et al. Deficient autophagy in microglia impairs synaptic pruning and causes social behavioral defects. Mol Psychiatry (2017) 22(11):1576–84. doi: 10.1038/mp.2016.103
27. Chaudhary R, Morris RJ, Steinson E. The multifactorial roles of microglia and macrophages in the maintenance and progression of glioblastoma. J Neuroimmunol (2021) 357:577633. doi: 10.1016/j.jneuroim.2021.577633
28. Blasi E, Barluzzi R, Bocchini V, Mazzolla R, Bistoni F. Immortalization of murine microglial cells by a V-Raf/V-Myc carrying retrovirus. J Neuroimmunol (1990) 27(2-3):229–37. doi: 10.1016/0165-5728(90)90073-v
29. Horvath RJ, Nutile-McMenemy N, Alkaitis MS, Deleo JA. Differential migration, lps-induced cytokine, chemokine, and no expression in immortalized bv-2 and hapi cell lines and primary microglial cultures. J neurochemistry (2008) 107(2):557–69. doi: 10.1111/j.1471-4159.2008.05633.x
30. Beutner C, Linnartz-Gerlach B, Schmidt SV, Beyer M, Mallmann MR, Staratschek-Jox A, et al. Unique transcriptome signature of mouse microglia. Glia (2013) 61(9):1429–42. doi: 10.1002/glia.22524
31. Lannes N, Eppler E, Etemad S, Yotovski P, Filgueira L. Microglia at center stage: A comprehensive review about the versatile and unique residential macrophages of the central nervous system. Oncotarget (2017) 8(69):114393–413. doi: 10.18632/oncotarget.23106
32. Rutherford MS, Schook LB. Differential immunocompetence of macrophages derived using macrophage or granulocyte-macrophage colony-stimulating factor. J leukocyte Biol (1992) 51(1):69–76. doi: 10.1002/jlb.51.1.69
33. Bowman RL, Klemm F, Akkari L, Pyonteck SM, Sevenich L, Quail DF, et al. Macrophage ontogeny underlies differences in tumor-specific education in brain malignancies. Cell Rep (2016) 17(9):2445–59. doi: 10.1016/j.celrep.2016.10.052
34. Guldner IH, Wang Q, Yang L, Golomb SM, Zhao Z, Lopez JA, et al. Cns-native myeloid cells drive immune suppression in the brain metastatic niche through Cxcl10. Cell (2020) 183(5):1234–48.e25. doi: 10.1016/j.cell.2020.09.064
35. Bennett ML, Bennett FC, Liddelow SA, Ajami B, Zamanian JL, Fernhoff NB, et al. New tools for studying microglia in the mouse and human cns. Proc Natl Acad Sci U.S.A. (2016) 113(12):E1738–46. doi: 10.1073/pnas.1525528113
36. Sousa C, Biber K, Michelucci A. Cellular and molecular characterization of microglia: A unique immune cell population. Front Immunol (2017) 8:198. doi: 10.3389/fimmu.2017.00198
37. Mecca C, Giambanco I, Donato R, Arcuri C. Microglia and aging: The role of the Trem2-Dap12 and Cx3cl1-Cx3cr1 axes. Int J Mol Sci (2018) 19(1). doi: 10.3390/ijms19010318
38. Buttgereit A, Lelios I, Yu X, Vrohlings M, Krakoski NR, Gautier EL, et al. Sall1 is a transcriptional regulator defining microglia identity and function. Nat Immunol (2016) 17(12):1397–406. doi: 10.1038/ni.3585
39. Kaiser T, Feng G. Tmem119-egfp and Tmem119-Creert2 transgenic mice for labeling and manipulating microglia. eNeuro (2019) 6(4). doi: 10.1523/eneuro.0448-18.2019
40. Komiya H, Takeuchi H, Ogawa Y, Hatooka Y, Takahashi K, Katsumoto A, et al. Ccr2 is localized in microglia and neurons, as well as infiltrating monocytes, in the lumbar spinal cord of als mice. Mol Brain (2020) 13(1):64. doi: 10.1186/s13041-020-00607-3
41. Badie B, Schartner JM. Flow cytometric characterization of tumor-associated macrophages in experimental gliomas. Neurosurgery (2000) 46(4):957–61. doi: 10.1097/00006123-200004000-00035
42. Müller A, Brandenburg S, Turkowski K, Müller S, Vajkoczy P. Resident microglia, and not peripheral macrophages, are the main source of brain tumor mononuclear cells. Int J Cancer (2015) 137(2):278–88. doi: 10.1002/ijc.29379
43. Mills CD, Kincaid K, Alt JM, Heilman MJ, Hill AM. M-1/M-2 macrophages and the Th1/Th2 paradigm. J Immunol (2000) 164(12):6166–73. doi: 10.4049/jimmunol.164.12.6166
44. Ponomarev ED, Shriver LP, Maresz K, Dittel BN. Microglial cell activation and proliferation precedes the onset of cns autoimmunity. J Neurosci Res (2005) 81(3):374–89. doi: 10.1002/jnr.20488
45. Yang X, Xu S, Qian Y, Xiao Q. Resveratrol regulates microglia M1/M2 polarization Via pgc-1α in conditions of neuroinflammatory injury. Brain Behav Immun (2017) 64:162–72. doi: 10.1016/j.bbi.2017.03.003
46. Roodveldt C, Labrador-Garrido A, Gonzalez-Rey E, Lachaud CC, Guilliams T, Fernandez-Montesinos R, et al. Preconditioning of microglia by A-synuclein strongly affects the response induced by toll-like receptor (Tlr) stimulation. PloS One (2013) 8(11):e79160. doi: 10.1371/journal.pone.0079160
47. Venugopal D, Vishwakarma S, Kaur I, Samavedi S. Electrospun meshes intrinsically promote M2 polarization of microglia under hypoxia and offer protection from hypoxia-driven cell death. Biomed materials (Bristol England) (2021) 16(4). doi: 10.1088/1748-605X/ac0a91
48. Rao G, Latha K, Ott M, Sabbagh A, Marisetty A, Ling X, et al. Anti-Pd-1 induces M1 polarization in the glioma microenvironment and exerts therapeutic efficacy in the absence of Cd8 cytotoxic T cells. Clin Cancer Res (2020) 26(17):4699–712. doi: 10.1158/1078-0432.Ccr-19-4110
49. Zeiner PS, Preusse C, Golebiewska A, Zinke J, Iriondo A, Muller A, et al. Distribution and prognostic impact of Microglia/Macrophage subpopulations in gliomas. Brain Pathol (Zurich Switzerland) (2019) 29(4):513–29. doi: 10.1111/bpa.12690
50. Li J, Sun Y, Sun X, Zhao X, Ma Y, Wang Y, et al. Aeg-1 silencing attenuates M2-polarization of glioma-associated Microglia/Macrophages and sensitizes glioma cells to temozolomide. Sci Rep (2021) 11(1):17348. doi: 10.1038/s41598-021-96647-3
51. He Y, Gao Y, Zhang Q, Zhou G, Cao F, Yao S. Il-4 switches Microglia/Macrophage M1/M2 polarization and alleviates neurological damage by modulating the Jak1/Stat6 pathway following ich. Neuroscience (2020) 437:161–71. doi: 10.1016/j.neuroscience.2020.03.008
52. Tang Y, Le W. Differential roles of M1 and M2 microglia in neurodegenerative diseases. Mol Neurobiol (2016) 53(2):1181–94. doi: 10.1007/s12035-014-9070-5
53. Zhou W, Ke SQ, Huang Z, Flavahan W, Fang X, Paul J, et al. Periostin secreted by glioblastoma stem cells recruits M2 tumour-associated macrophages and promotes malignant growth. Nat Cell Biol (2015) 17(2):170–82. doi: 10.1038/ncb3090
54. Mantovani A, Sica A, Sozzani S, Allavena P, Vecchi A, Locati M. The chemokine system in diverse forms of macrophage activation and polarization. Trends Immunol (2004) 25(12):677–86. doi: 10.1016/j.it.2004.09.015
55. Lisi L, Stigliano E, Lauriola L, Navarra P, Dello Russo C. Proinflammatory-activated glioma cells induce a switch in microglial polarization and activation status, from a predominant M2b phenotype to a mixture of M1 and M2a/B polarized cells. ASN Neuro (2014) 6(3):171–83. doi: 10.1042/an20130045
56. Chhor V, Le Charpentier T, Lebon S, Oré MV, Celador IL, Josserand J, et al. Characterization of phenotype markers and neuronotoxic potential of polarised primary microglia in vitro. Brain Behav Immun (2013) 32:70–85. doi: 10.1016/j.bbi.2013.02.005
57. Mecha M, Yanguas-Casás N, Feliú A, Mestre L, Carrillo-Salinas FJ, Riecken K, et al. Involvement of Wnt7a in the role of M2c microglia in neural stem cell oligodendrogenesis. J Neuroinflamm (2020) 17(1):88. doi: 10.1186/s12974-020-01734-3
58. Mecha M, Feliú A, Carrillo-Salinas FJ, Rueda-Zubiaurre A, Ortega-Gutiérrez S, de Sola RG, et al. Endocannabinoids drive the acquisition of an alternative phenotype in microglia. Brain Behav Immun (2015) 49:233–45. doi: 10.1016/j.bbi.2015.06.002
59. Shen L, Zhou Y, He H, Chen W, Lenahan C, Li X, et al. Crosstalk between macrophages, T cells, and iron metabolism in tumor microenvironment. Oxid Med Cell Longevity (2021) 2021:8865791. doi: 10.1155/2021/8865791
60. Xiao Y, Wang Z, Zhao M, Deng Y, Yang M, Su G, et al. Single-cell transcriptomics revealed subtype-specific tumor immune microenvironments in human glioblastomas. Front Immunol (2022) 13:914236. doi: 10.3389/fimmu.2022.914236
61. Xuan W, Hsu WH, Khan F, Dunterman M, Pang L, Wainwright DA, et al. Circadian regulator clock drives immunosuppression in glioblastoma. Cancer Immunol Res (2022) 10(6):770–84. doi: 10.1158/2326-6066.Cir-21-0559
62. Gabrusiewicz K, Rodriguez B, Wei J, Hashimoto Y, Healy LM, Maiti SN, et al. Glioblastoma-infiltrated innate immune cells resemble M0 macrophage phenotype. JCI Insight (2016) 1(2). doi: 10.1172/jci.insight.85841
63. Hu F, Dzaye O, Hahn A, Yu Y, Scavetta RJ, Dittmar G, et al. Glioma-derived versican promotes tumor expansion Via glioma-associated Microglial/Macrophages toll-like receptor 2 signaling. Neuro-oncology (2015) 17(2):200–10. doi: 10.1093/neuonc/nou324
64. Hu F, Ku MC, Markovic D, Dzaye O, Lehnardt S, Synowitz M, et al. Glioma-associated microglial Mmp9 expression is upregulated by Tlr2 signaling and sensitive to minocycline. Int J Cancer (2014) 135(11):2569–78. doi: 10.1002/ijc.28908
65. Xu C, Xiao M, Li X, Xin L, Song J, Zhan Q, et al. Origin, activation, and targeted therapy of glioma-associated macrophages. Front Immunol (2022) 13:974996. doi: 10.3389/fimmu.2022.974996
66. Platten M, Kretz A, Naumann U, Aulwurm S, Egashira K, Isenmann S, et al. Monocyte chemoattractant protein-1 increases microglial infiltration and aggressiveness of gliomas. Ann Neurol (2003) 54(3):388–92. doi: 10.1002/ana.10679
67. Okada M, Saio M, Kito Y, Ohe N, Yano H, Yoshimura S, et al. Tumor-associated Macrophage/Microglia infiltration in human gliomas is correlated with mcp-3, but not mcp-1. Int J Oncol (2009) 34(6):1621–7. doi: 10.3892/ijo_00000292
68. Wang SC, Hong JH, Hsueh C, Chiang CS. Tumor-secreted sdf-1 promotes glioma invasiveness and Tam tropism toward hypoxia in a murine astrocytoma model. Lab Invest (2012) 92(1):151–62. doi: 10.1038/labinvest.2011.128
69. Chen P, Zhao D, Li J, Liang X, Li J, Chang A, et al. Symbiotic macrophage-glioma cell interactions reveal synthetic lethality in pten-null glioma. Cancer Cell (2019) 35(6):868–84.e6. doi: 10.1016/j.ccell.2019.05.003
70. Coniglio SJ, Eugenin E, Dobrenis K, Stanley ER, West BL, Symons MH, et al. Microglial stimulation of glioblastoma invasion involves epidermal growth factor receptor (Egfr) and colony stimulating factor 1 receptor (Csf-1r) signaling. Mol Med (Cambridge Mass) (2012) 18(1):519–27. doi: 10.2119/molmed.2011.00217
71. Ku MC, Wolf SA, Respondek D, Matyash V, Pohlmann A, Waiczies S, et al. Gdnf mediates glioblastoma-induced microglia attraction but not astrogliosis. Acta neuropathologica (2013) 125(4):609–20. doi: 10.1007/s00401-013-1079-8
72. Aretz P, Maciaczyk D, Yusuf S, Sorg RV, Hänggi D, Liu H, et al. Crosstalk between B-catenin and Ccl2 drives migration of monocytes towards glioblastoma cells. Int J Mol Sci (2022) 23(9). doi: 10.3390/ijms23094562
73. Liu L, Zhou X, Cheng S, Ge Y, Chen B, Shi J, et al. Rna-binding protein Dhx9 promotes glioma growth and tumor-associated macrophages infiltration Via Tcf12. CNS Neurosci Ther (2022). doi: 10.1111/cns.14031
74. Mineo M, Ricklefs F, Rooj AK, Lyons SM, Ivanov P, Ansari KI, et al. The long non-coding rna Hif1a-As2 facilitates the maintenance of mesenchymal glioblastoma stem-like cells in hypoxic niches. Cell Rep (2016) 15(11):2500–9. doi: 10.1016/j.celrep.2016.05.018
75. Guo X, Xue H, Shao Q, Wang J, Guo X, Chen X, et al. Hypoxia promotes glioma-associated macrophage infiltration Via periostin and subsequent M2 polarization by upregulating tgf-beta and m-csfr. Oncotarget (2016) 7(49):80521–42. doi: 10.18632/oncotarget.11825
76. Liao Y, Luo Z, Lin Y, Chen H, Chen T, Xu L, et al. Prmt3 drives glioblastoma progression by enhancing Hif1a and glycolytic metabolism. Cell Death Dis (2022) 13(11):943. doi: 10.1038/s41419-022-05389-1
77. De Boeck A, Ahn BY, D’Mello C, Lun X, Menon SV, Alshehri MM, et al. Glioma-derived il-33 orchestrates an inflammatory brain tumor microenvironment that accelerates glioma progression. Nat Commun (2020) 11(1):4997. doi: 10.1038/s41467-020-18569-4
78. Bettinger I, Thanos S, Paulus W. Microglia promote glioma migration. Acta neuropathologica (2002) 103(4):351–5. doi: 10.1007/s00401-001-0472-x
79. Markovic DS, Glass R, Synowitz M, Rooijen N, Kettenmann H. Microglia stimulate the invasiveness of glioma cells by increasing the activity of metalloprotease-2. J Neuropathol Exp Neurol (2005) 64(9):754–62. doi: 10.1097/01.jnen.0000178445.33972.a9
80. Markovic DS, Vinnakota K, Chirasani S, Synowitz M, Raguet H, Stock K, et al. Gliomas induce and exploit microglial Mt1-mmp expression for tumor expansion. Proc Natl Acad Sci U.S.A. (2009) 106(30):12530–5. doi: 10.1073/pnas.0804273106
81. Zhou W, Yu X, Sun S, Zhang X, Yang W, Zhang J, et al. Increased expression of mmp-2 and mmp-9 indicates poor prognosis in glioma recurrence. BioMed Pharmacother (2019) 118:109369. doi: 10.1016/j.biopha.2019.109369
82. Singh MK, Bhattacharya D, Chaudhuri S, Acharya S, Kumar P, Santra P, et al. T11ts inhibits glioma angiogenesis by modulation of mmps, timps, with related integrin Av and tgf-B1 expressions. Tumour Biol (2014) 35(3):2231–46. doi: 10.1007/s13277-013-1296-8
83. Yu-Ju Wu C, Chen CH, Lin CY, Feng LY, Lin YC, Wei KC, et al. Ccl5 of glioma-associated Microglia/Macrophages regulates glioma migration and invasion Via calcium-dependent matrix metalloproteinase 2. Neuro-oncology (2020) 22(2):253–66. doi: 10.1093/neuonc/noz189
84. Pandey M, Awasthi S, Baranwal S. Il-6: An endogenous activator of mmp-9 in preterm birth. J Reprod Immunol (2020) 141:103147. doi: 10.1016/j.jri.2020.103147
85. Zhang J, Sarkar S, Cua R, Zhou Y, Hader W, Yong VW. A dialog between glioma and microglia that promotes tumor invasiveness through the Ccl2/Ccr2/Interleukin-6 axis. Carcinogenesis (2012) 33(2):312–9. doi: 10.1093/carcin/bgr289
86. Gu QH, Jia XY, Hu SY, Wang SX, Zou WZ, Cui Z, et al. The clinical and immunologic features of patients with combined anti-gbm disease and castleman disease. Am J Kidney Dis (2018) 71(6):904–8. doi: 10.1053/j.ajkd.2018.01.029
87. Greenall SA, McKenzie M, Seminova E, Dolezal O, Pearce L, Bentley J, et al. Most clinical anti-egfr antibodies do not neutralize both wtegfr and egfrviii activation in glioma. Neuro-oncology (2019) 21(8):1016–27. doi: 10.1093/neuonc/noz073
88. Brandenburg S, Müller A, Turkowski K, Radev YT, Rot S, Schmidt C, et al. Resident microglia rather than peripheral macrophages promote vascularization in brain tumors and are source of alternative pro-angiogenic factors. Acta neuropathologica (2016) 131(3):365–78. doi: 10.1007/s00401-015-1529-6
89. Bayerl SH, Niesner R, Cseresnyes Z, Radbruch H, Pohlan J, Brandenburg S, et al. Time lapse in vivo microscopy reveals distinct dynamics of microglia-tumor environment interactions-a new role for the tumor perivascular space as highway for trafficking microglia. Glia (2016) 64(7):1210–26. doi: 10.1002/glia.22994
90. Zhang G, Tao X, Ji B, Gong J. Hypoxia-driven M2-polarized macrophages facilitate cancer aggressiveness and temozolomide resistance in glioblastoma. Oxid Med Cell Longev (2022) 2022:1614336. doi: 10.1155/2022/1614336
91. Felsenstein M, Blank A, Bungert AD, Mueller A, Ghori A, Kremenetskaia I, et al. Ccr2 of tumor microenvironmental cells is a relevant modulator of glioma biology. Cancers (Basel) (2020) 12(7). doi: 10.3390/cancers12071882
92. Mercurio L, Ajmone-Cat MA, Cecchetti S, Ricci A, Bozzuto G, Molinari A, et al. Targeting Cxcr4 by a selective peptide antagonist modulates tumor microenvironment and microglia reactivity in a human glioblastoma model. J Exp Clin Cancer research: CR (2016) 35:55. doi: 10.1186/s13046-016-0326-y
93. Zhu C, Kros JM, Cheng C, Mustafa D. The contribution of tumor-associated macrophages in glioma neo-angiogenesis and implications for anti-angiogenic strategies. Neuro-oncology (2017) 19(11):1435–46. doi: 10.1093/neuonc/nox081
94. Piao Y, Liang J, Holmes L, Zurita AJ, Henry V, Heymach JV, et al. Glioblastoma resistance to anti-vegf therapy is associated with myeloid cell infiltration, stem cell accumulation, and a mesenchymal phenotype. Neuro-oncology (2012) 14(11):1379–92. doi: 10.1093/neuonc/nos158
95. Menna G, Mattogno PP, Donzelli CM, Lisi L, Olivi A, Della Pepa GM. Glioma-associated microglia characterization in the glioblastoma microenvironment through a ‘Seed-and soil’ approach: A systematic review. Brain Sci (2022) 12(6). doi: 10.3390/brainsci12060718
96. Dumas AA, Pomella N, Rosser G, Guglielmi L, Vinel C, Millner TO, et al. Microglia promote glioblastoma Via mtor-mediated immunosuppression of the tumour microenvironment. EMBO J (2020) 39(15):e103790. doi: 10.15252/embj.2019103790
97. Mukherjee S, Fried A, Hussaini R, White R, Baidoo J, Yalamanchi S, et al. Phytosomal curcumin causes natural killer cell-dependent repolarization of glioblastoma (Gbm) tumor-associated Microglia/Macrophages and elimination of gbm and gbm stem cells. J Exp Clin Cancer research: CR (2018) 37(1):168. doi: 10.1186/s13046-018-0792-5
98. Zhang L, Liu W, Alizadeh D, Zhao D, Farrukh O, Lin J, et al. S100b attenuates microglia activation in gliomas: Possible role of Stat3 pathway. Glia (2011) 59(3):486–98. doi: 10.1002/glia.21118
99. Pattwell SS, Arora S, Cimino PJ, Ozawa T, Szulzewsky F, Hoellerbauer P, et al. A kinase-deficient Ntrk2 splice variant predominates in glioma and amplifies several oncogenic signaling pathways. Nat Commun (2020) 11(1):2977. doi: 10.1038/s41467-020-16786-5
100. Gao X, Xia X, Li F, Zhang M, Zhou H, Wu X, et al. Circular rna-encoded oncogenic e-cadherin variant promotes glioblastoma tumorigenicity through activation of egfr-Stat3 signalling. Nat Cell Biol (2021) 23(3):278–91. doi: 10.1038/s41556-021-00639-4
101. Feng X, Wu J, Xu X, Liu H, Liu J, Li J, et al. [a preliminary study about the interaction between basic fibroblast growth factor and signal transducer and activator of transcription 3 in glioma apoptosis]. Zhonghua wai ke za zhi [Chinese J surgery] (2014) 52(12):939–44. doi: 10.3760/cma.j.issn.0529-5815.2014.12.014
102. Zhang L, Alizadeh D, Van Handel M, Kortylewski M, Yu H, Badie B. Stat3 inhibition activates tumor macrophages and abrogates glioma growth in mice. Glia (2009) 57(13):1458–67. doi: 10.1002/glia.20863
103. Gao Z, Xu J, Fan Y, Zhang Z, Wang H, Qian M, et al. Arpc1b promotes mesenchymal phenotype maintenance and radiotherapy resistance by blocking Trim21-mediated degradation of Ifi16 and hur in glioma stem cells. J Exp Clin Cancer research: CR (2022) 41(1):323. doi: 10.1186/s13046-022-02526-8
104. Mizoguchi M, Betensky RA, Batchelor TT, Bernay DC, Louis DN, Nutt CL. Activation of Stat3, mapk, and akt in malignant astrocytic gliomas: Correlation with egfr status, tumor grade, and survival. J Neuropathol Exp Neurol (2006) 65(12):1181–8. doi: 10.1097/01.jnen.0000248549.14962.b2
105. Wang H, Wang H, Zhang W, Huang HJ, Liao WS, Fuller GN. Analysis of the activation status of akt, nfkappab, and Stat3 in human diffuse gliomas. Lab Invest (2004) 84(8):941–51. doi: 10.1038/labinvest.3700123
106. Lo HW, Cao X, Zhu H, Ali-Osman F. Constitutively activated Stat3 frequently coexpresses with epidermal growth factor receptor in high-grade gliomas and targeting Stat3 sensitizes them to iressa and alkylators. Clin Cancer Res (2008) 14(19):6042–54. doi: 10.1158/1078-0432.Ccr-07-4923
107. Ignatova TN, Kukekov VG, Laywell ED, Suslov ON, Vrionis FD, Steindler DA. Human cortical glial tumors contain neural stem-like cells expressing astroglial and neuronal markers in vitro. Glia (2002) 39(3):193–206. doi: 10.1002/glia.10094
108. Galli R, Binda E, Orfanelli U, Cipelletti B, Gritti A, De Vitis S, et al. Isolation and characterization of tumorigenic, stem-like neural precursors from human glioblastoma. Cancer Res (2004) 64(19):7011–21. doi: 10.1158/0008-5472.Can-04-1364
109. Yuan X, Curtin J, Xiong Y, Liu G, Waschsmann-Hogiu S, Farkas DL, et al. Isolation of cancer stem cells from adult glioblastoma multiforme. Oncogene (2004) 23(58):9392–400. doi: 10.1038/sj.onc.1208311
110. Hemmati HD, Nakano I, Lazareff JA, Masterman-Smith M, Geschwind DH, Bronner-Fraser M, et al. Cancerous stem cells can arise from pediatric brain tumors. Proc Natl Acad Sci U.S.A. (2003) 100(25):15178–83. doi: 10.1073/pnas.2036535100
111. Sanai N, Alvarez-Buylla A, Berger MS. Neural stem cells and the origin of gliomas. N Engl J Med (2005) 353(8):811–22. doi: 10.1056/NEJMra043666
112. Singh SK, Hawkins C, Clarke ID, Squire JA, Bayani J, Hide T, et al. Identification of human brain tumour initiating cells. Nature (2004) 432(7015):396–401. doi: 10.1038/nature03128
113. Chen J, Li Y, Yu TS, McKay RM, Burns DK, Kernie SG, et al. A restricted cell population propagates glioblastoma growth after chemotherapy. Nature (2012) 488(7412):522–6. doi: 10.1038/nature11287
114. Lathia JD, Gallagher J, Myers JT, Li M, Vasanji A, McLendon RE, et al. Direct in vivo evidence for tumor propagation by glioblastoma cancer stem cells. PloS One (2011) 6(9):e24807. doi: 10.1371/journal.pone.0024807
115. Fries G, Perneczky A, Kempski O. Glioblastoma-associated circulating monocytes and the release of epidermal growth factor. J Neurosurg (1996) 85(4):642–7. doi: 10.3171/jns.1996.85.4.0642
116. Gjorgjevski M, Hannen R, Carl B, Li Y, Landmann E, Buchholz M, et al. Molecular profiling of the tumor microenvironment in glioblastoma patients: Correlation of Microglia/Macrophage polarization state with metalloprotease expression profiles and survival. Bioscience Rep (2019) 39(6). doi: 10.1042/bsr20182361
117. Martin S, Dicou E, Vincent JP, Mazella J. Neurotensin and the neurotensin receptor-3 in microglial cells. J Neurosci Res (2005) 81(3):322–6. doi: 10.1002/jnr.20477
118. Yi L, Xiao H, Xu M, Ye X, Hu J, Li F, et al. Glioma-initiating cells: A predominant role in Microglia/Macrophages tropism to glioma. J Neuroimmunol (2011) 232(1-2):75–82. doi: 10.1016/j.jneuroim.2010.10.011
119. Li D, Zhang Q, Li L, Chen K, Yang J, Dixit D, et al. B2-microglobulin maintains glioblastoma stem cells and induces M2-like polarization of tumor-associated macrophages. Cancer Res (2022) 82(18):3321–34. doi: 10.1158/0008-5472.Can-22-0507
120. Ye XZ, Xu SL, Xin YH, Yu SC, Ping YF, Chen L, et al. Tumor-associated Microglia/Macrophages enhance the invasion of glioma stem-like cells Via tgf-B1 signaling pathway. J Immunol (2012) 189(1):444–53. doi: 10.4049/jimmunol.1103248
121. Li J, Kaneda MM, Ma J, Li M, Shepard RM, Patel K, et al. Pi3kγ inhibition suppresses Microglia/Tam accumulation in glioblastoma microenvironment to promote exceptional temozolomide response. Proc Natl Acad Sci U.S.A. (2021) 118(16). doi: 10.1073/pnas.2009290118
122. Wang H, Lathia JD, Wu Q, Wang J, Li Z, Heddleston JM, et al. Targeting interleukin 6 signaling suppresses glioma stem cell survival and tumor growth. Stem Cells (Dayton Ohio) (2009) 27(10):2393–404. doi: 10.1002/stem.188
123. Sarkar S, Döring A, Zemp FJ, Silva C, Lun X, Wang X, et al. Therapeutic activation of macrophages and microglia to suppress brain tumor-initiating cells. Nat Neurosci (2014) 17(1):46–55. doi: 10.1038/nn.3597
124. Tu S, Lin X, Qiu J, Zhou J, Wang H, Hu S, et al. Crosstalk between tumor-associated Microglia/Macrophages and Cd8-positive T cells plays a key role in glioblastoma. Front Immunol (2021) 12:650105. doi: 10.3389/fimmu.2021.650105
125. Liau LM, Ashkan K, Tran DD, Campian JL, Trusheim JE, Cobbs CS, et al. First results on survival from a Large phase 3 clinical trial of an autologous dendritic cell vaccine in newly diagnosed glioblastoma. J Trans Med (2018) 16(1):142. doi: 10.1186/s12967-018-1507-6
126. Choe JH, Watchmaker PB, Simic MS, Gilbert RD, Li AW, Krasnow NA, et al. Synnotch-car T cells overcome challenges of specificity, heterogeneity, and persistence in treating glioblastoma. Sci Trans Med (2021) 13(591). doi: 10.1126/scitranslmed.abe7378
127. Cohen AL, Anker CJ, Johnson B, Burt LM, Shrieve DC, Salzman K, et al. Repeat radiation with bevacizumab and minocycline in bevacizumab-refractory high grade gliomas: A prospective phase 1 trial. J neuro-oncol (2020) 148(3):577–85. doi: 10.1007/s11060-020-03551-3
128. Butowski N, Colman H, De Groot JF, Omuro AM, Nayak L, Wen PY, et al. Orally administered colony stimulating factor 1 receptor inhibitor Plx3397 in recurrent glioblastoma: An ivy foundation early phase clinical trials consortium phase ii study. Neuro-oncology (2016) 18(4):557–64. doi: 10.1093/neuonc/nov245
129. Pradel LP, Ooi CH, Romagnoli S, Cannarile MA, Sade H, Rüttinger D, et al. Macrophage susceptibility to emactuzumab (Rg7155) treatment. Mol Cancer Ther (2016) 15(12):3077–86. doi: 10.1158/1535-7163.Mct-16-0157
130. Chiocca EA, Gelb AB, Chen CC, Rao G, Reardon DA, Wen PY, et al. Combined immunotherapy with controlled interleukin-12 gene therapy and immune checkpoint blockade in recurrent glioblastoma: An open-label, multi-institutional phase I trial. Neuro-oncology (2022) 24(6):951–63. doi: 10.1093/neuonc/noab271
131. Ursu R, Carpentier A, Metellus P, Lubrano V, Laigle-Donadey F, Capelle L, et al. Intracerebral injection of cpg oligonucleotide for patients with De novo glioblastoma-a phase ii multicentric, randomised study. Eur J Cancer (Oxford England: 1990) (2017) 73:30–7. doi: 10.1016/j.ejca.2016.12.003
132. Thomas RP, Nagpal S, Iv M, Soltys SG, Bertrand S, Pelpola JS, et al. Macrophage exclusion after radiation therapy (Mert): A first in human phase I/Ii trial using a Cxcr4 inhibitor in glioblastoma. Clin Cancer Res (2019) 25(23):6948–57. doi: 10.1158/1078-0432.Ccr-19-1421
133. Lee EQ, Duda DG, Muzikansky A, Gerstner ER, Kuhn JG, Reardon DA, et al. Phase I and biomarker study of plerixafor and bevacizumab in recurrent high-grade glioma. Clin Cancer Res (2018) 24(19):4643–9. doi: 10.1158/1078-0432.Ccr-18-1025
134. Groot J, Ott M, Wei J, Kassab C, Fang D, Najem H, et al. A first-in-Human phase I trial of the oral p-Stat3 inhibitor Wp1066 in patients with recurrent malignant glioma. CNS Oncol (2022) 11(2):Cns87. doi: 10.2217/cns-2022-0005
135. Rao R, Han R, Ogurek S, Xue C, Wu LM, Zhang L, et al. Glioblastoma genetic drivers dictate the function of tumor-associated Macrophages/Microglia and responses to Csf1r inhibition. Neuro-oncology (2022) 24(4):584–97. doi: 10.1093/neuonc/noab228
136. Elmore MR, Najafi AR, Koike MA, Dagher NN, Spangenberg EE, Rice RA, et al. Colony-stimulating factor 1 receptor signaling is necessary for microglia viability, unmasking a microglia progenitor cell in the adult brain. Neuron (2014) 82(2):380–97. doi: 10.1016/j.neuron.2014.02.040
137. Crotty EE, Smith SMC, Brasel K, Pakiam F, Girard EJ, Connor YD, et al. Medulloblastoma recurrence and metastatic spread are independent of colony-stimulating factor 1 receptor signaling and macrophage survival. J neuro-oncol (2021) 153(2):225–37. doi: 10.1007/s11060-021-03767-x
138. Chiu TL, Peng CW, Wang MJ. Enhanced anti-glioblastoma activity of microglia by Aav2-mediated il-12 through trail and phagocytosis in vitro. Oncol Rep (2011) 25(5):1373–80. doi: 10.3892/or.2011.1213
139. Jia H, Xie X, Wang L, Wang L, Che F. Ifn-Γ induces pd-L1 through P38/Jnk/Erk signaling pathways and counteracts the tumor promoting effect mediated by pd-L1 in glioblastoma. Comput Intell Neurosci (2022) 2022:5492602. doi: 10.1155/2022/5492602
140. Ueda M, Usami K, Yamao Y, Yamawaki R, Umaba C, Liang N, et al. Correlation between brain functional connectivity and neurocognitive function in patients with left frontal glioma. Sci Rep (2022) 12(1):18302. doi: 10.1038/s41598-022-22493-6
141. Ginzkey C, Eicker SO, Marget M, Krause J, Brecht S, Westphal M, et al. Increase in tumor size following intratumoral injection of immunostimulatory cpg-containing oligonucleotides in a rat glioma model. Cancer immunol immunother: CII (2010) 59(4):541–51. doi: 10.1007/s00262-009-0771-y
142. Zhang L, Nesvick CL, Day CA, Choi J, Lu VM, Peterson T, et al. Stat3 is a biologically relevant therapeutic target in H3k27m-mutant diffuse midline glioma. Neuro-oncology (2022) 24(10):1700–11. doi: 10.1093/neuonc/noac093
143. Zhou Y, Guo Y, Chen L, Zhang X, Wu W, Yang Z, et al. Co-Delivery of phagocytosis checkpoint and sting agonist by a Trojan horse nanocapsule for orthotopic glioma immunotherapy. Theranostics (2022) 12(12):5488–503. doi: 10.7150/thno.73104
144. Waziri A, Killory B, Ogden AT 3rd, Canoll P, Anderson RC, Kent SC, et al. Preferential in situ Cd4+Cd56+ T cell activation and expansion within human glioblastoma. J Immunol (2008) 180(11):7673–80. doi: 10.4049/jimmunol.180.11.7673
145. Hu J, Dong F, He Y, Xia X, Cheng F, Chen S, et al. Lrig2 promotes glioblastoma progression by modulating innate antitumor immunity through macrophage infiltration and polarization. J immunother Cancer (2022) 10(9). doi: 10.1136/jitc-2021-004452
146. Xu B, Tian L, Chen J, Wang J, Ma R, Dong W, et al. An oncolytic virus expressing a full-length antibody enhances antitumor innate immune response to glioblastoma. Nat Commun (2021) 12(1):5908. doi: 10.1038/s41467-021-26003-6
Keywords: glioma, glioblastoma, glioma-associated macrophage and microglia, tumor microenvironment, immunotherapy
Citation: Lin C, Wang N and Xu C (2023) Glioma-associated microglia/macrophages (GAMs) in glioblastoma: Immune function in the tumor microenvironment and implications for immunotherapy. Front. Immunol. 14:1123853. doi: 10.3389/fimmu.2023.1123853
Received: 14 December 2022; Accepted: 28 February 2023;
Published: 09 March 2023.
Edited by:
Xiangsheng Zhang, Affiliated Beijing Friendship Hospital, Capital Medical University, ChinaReviewed by:
Jun Yan, Guangxi Medical University Cancer Hospital, ChinaCopyright © 2023 Lin, Wang and Xu. This is an open-access article distributed under the terms of the Creative Commons Attribution License (CC BY). The use, distribution or reproduction in other forums is permitted, provided the original author(s) and the copyright owner(s) are credited and that the original publication in this journal is cited, in accordance with accepted academic practice. No use, distribution or reproduction is permitted which does not comply with these terms.
*Correspondence: Chengyan Xu, NjUwOTAzMUB6anUuZWR1LmNu
Disclaimer: All claims expressed in this article are solely those of the authors and do not necessarily represent those of their affiliated organizations, or those of the publisher, the editors and the reviewers. Any product that may be evaluated in this article or claim that may be made by its manufacturer is not guaranteed or endorsed by the publisher.
Research integrity at Frontiers
Learn more about the work of our research integrity team to safeguard the quality of each article we publish.