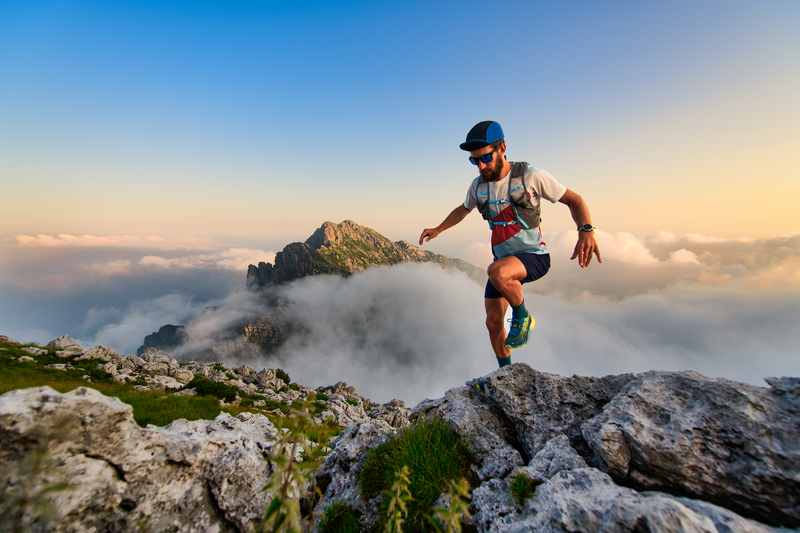
95% of researchers rate our articles as excellent or good
Learn more about the work of our research integrity team to safeguard the quality of each article we publish.
Find out more
ORIGINAL RESEARCH article
Front. Immunol. , 23 February 2023
Sec. Nutritional Immunology
Volume 14 - 2023 | https://doi.org/10.3389/fimmu.2023.1123052
This article is part of the Research Topic Innate Immunity and Cross-Talk with Microflora in the Regulation of Immune Recognition and Polarization During Immune-Related Diseases View all 9 articles
Introduction: Chronic inflammation caused by dietary obesity has been considered to induce lifestyle-related diseases and functional ingredients with anti-inflammatory effects are attracting attention. Although multiple studies on obesity had proved the anti-inflammatory effects of ingestion of lactic acid bacteria (LAB) and other functional ingredients on adipose tissue, the precise effects on the intestine, especially on the individual intestinal segments have not been made clear. In this study, we elucidated the mechanisms of Lactiplantibacillus plantarum (basonym: Lactobacillus plantarum) OLL2712 in suppressing obesity-induced inflammation using high fat diet (HFD)-fed mice obesity model.
Methods: We orally administered heat-treated LAB to HFD-fed mice model, and investigated the inflammatory changes in adipose tissue and intestinal immune cells. We also analyzed gut microbiota, and evaluated the inflammation and permeability of the duodenum, jejunum, ileum and colon; four intestinal segments differing in gut bacteria composition and immune response.
Results: After 3-week LAB administration, the gene expression levels of proinflammatory cytokines were downregulated in adipose tissue, colon, and Peyer’s patches (PP)-derived F4/80+ cells. The LAB treatment alleviated obesity-related gut microbiota imbalance. L. plantarum OLL2712 treatment helps maintain intestinal barrier function, especially in the ileum, possibly by preventing ZO-1 and Occludin downregulation.
Discussion: Our results suggest that the oral administration of the LAB strain regulated the gut microbiota, suppressed intestinal inflammation, and improved the gut barrier, which could inhibit the products of obesity-induced gut dysbiosis from translocating into the bloodstream and the adipose tissue, through which the LAB finally alleviated the inflammation caused by dietary obesity. Barrier improvement was observed, especially in the ileum, suggesting collaborative modulation of the intestinal immune responses by ingested LAB and microbiota.
According to the WHO Fact Sheet, worldwide obesity has nearly tripled since 1975, and the number of obese people is still rising due to the increased availability of high-calorie foods and lack of exercise, and it has become one of the most serious problems worldwide (1, 2). Multiple studies have shown that obesity can cause chronic inflammation (3–5). Persistent inflammatory conditions have been frequently reported to induce an exacerbation of lifestyle diseases, contributing to elevated risks of atherosclerosis, type 2 diabetes, and some cancers (6–8).
Gut microbiota, which represents the microorganisms in the gastrointestinal tracts of the animals, is mainly regulated by digested food. Gut microbiota is essential for the host metabolism, relating to the immune system and the barrier function (9, 10). Dysbiosis of gut microbiota is one of the key factors regulating obesity-associated disorders (11), as shown in the observation that germ-free mice do not show increased body fat mass or exacerbated insulin resistance when fed a high-fat (HFD) diet, and this phenomenon disappears after gut microbiota transplantation (12, 13). Multiple studies on gut microbiota in obese patients have suggested that obesity changes the gut microbiota, and excessive accumulation of adipose tissue is correlated with the composition of the gut microbiota. In addition, dietary obesity is known to reduce the diversity of the gut microbiota, followed by a disruption of the metabolic equilibrium, which is normally maintained by diverse components of the gut microbiota (14).
On the other hand, intestinal barrier dysfunction is also considered to be related to the aggravation of chronic inflammation caused by obesity. The gut is connected to the external environment for the absorption of nutrients. In the gastrointestinal tract, especially in the large intestine, there are large amounts of gut bacteria, as well as bacterial pathogens and other harmful substances. The intestinal barrier functions to protect the host from these harmful substances (15, 16). It has been reported that obesity increases intestinal permeability, which allows the leakage of inflammation inducible foreign substances such as lipopolysaccharide (LPS), which is one of cell component derived from Gram-negative bacteria. The leakage of Gram-negative bacteria and LPS into the bloodstream could induce the infiltration of proinflammatory macrophages in the adipose tissue and the liver tissue, inducing systemic inflammation (17). Furthermore, recent studies have suggested that dysbiosis results in intestinal inflammation in obesity (18).
Recently, functional ingredients with anti-inflammatory effects have received attention, from which lactic acid bacteria (LAB) are a diverse group of Gram-positive bacteria that produce lactic acid as the major end product of the carbohydrate fermentation, and are often considered as probiotics balancing the gut microbiota. As a LAB strain, Lactiplantibacillus plantarum OLL2712 has been selected owing to its capacity to accelerate the production of the anti-inflammatory cytokine interleukin (IL)-10 in murine marrow-derived dendritic cells (DCs) and peritoneal macrophages (19). Moreover, it has been reported that oral administration of L. plantarum OLL2712 alleviates chronic inflammation of adipose tissue in obese mouse models (20) and reduces fasting plasma glucose and serum proinflammatory cytokine concentrations in prediabetic individuals (21), suggesting that this functional LAB could be used as novel pharmaceuticals.
In this study, our main purpose was to focus on the intestine, especially on the different parts of the digestive tract. The anti-inflammatory functions of OLL2712 on the adipose tissue had been reported (19) but the pathways through which ingested OLL2712 exerted the anti-inflammatory effects on the adipose tissue remained unclear. In this regard, the effects on the intestine were unknown. We hypothesized that OLL2712 alleviated the adipocyte inflammation via the intestine by suppressing inflammation or enhancing the gut barrier, and we presumed that such functions were different among different parts. We investigated the mechanisms of the anti-inflammatory effects of the LAB strain, focusing on the gut microbiota and intestinal function using HFD-fed mouse model. We found a mechanism by which the oral administration of LAB regulated the gut microbiota, suppressed intestinal inflammation, and improved the gut barrier. This could inhibit bacterial harmful components, induced by obesity, from translocating into the bloodstream and adipose tissue, through which the LAB strain alleviated the inflammation caused by dietary obesity. Furthermore, the improvement of barrier function was observed, especially in the ileum of HFD-fed mice under the LAB treatment, suggesting collaborative modulation of the intestinal immune responses by the ingested LAB and microbiota.
L. plantarum OLL2712, which had been heat-treated by incubation at 75°C for 60 min and lyophilized, after being cultured in de Man, Rogosa, and Sharpe (MRS) broth (Becton Dickinson, USA), and stored at -20°C.
C57BL/6 male mice were purchased from Charles River Laboratories (Japan, RRID : IMSR_CRL:027). Mice were fed sterilized (121°C, 20 min) water and maintained at an appropriate temperature (23 ± 2°C) and humidity (50 ± 5%) with a 12-hour light-dark cycle. All experiments were conducted with the approval of the Experimental Animal Ethics Committee of the Graduate School of Agriculture and Life Sciences of the University of Tokyo.
To create obese C57BL/6NCrl mice, mice were fed a HFD (60% kcal from fat; Oriental Yeast, Japan) from 8-week-old, and mice in the control group were fed a normal chow diet (AIN-93M; Oriental Yeast, Japan) (Figure S1A). 6 individuals were used for each group to investigate the proinflammatory changes induced by a 4-week HFD. The nutrient composition of HFD-60 and AIN-93M is shown in Tables S1, S2.
To investigate the effects of oral administration of L. plantarum OLL2712 in obese mice, C57BL/6NCrl mice were fed a HFD from 8-week-old for 4 weeks. In the last 3 weeks, L. plantarum OLL2712, suspended in the sterilized water to the concentration of 20 mg/mL, was orally administered every day, 4 mg to each mouse (Figure 1A). 8 – 12 individuals were used for each group.
Figure 1 Oral administration of L. plantarum OLL2712 alleviated the inflammation of adipocytes, colon and PP macrophages in mice but changed neither the body weight nor the adipose tissue weight. C57BL/6N male mice were fed a HFD (60% kcal from fat) for 4 weeks from 8 weeks of age. (A) In the last 3 weeks, mice were administered OLL2712 daily (4 mg dissolved in 200 μL water per dose), and mice administered water simultaneously were used as a control group (HFD). (B) Mice were weighed once per week. The weights of the epididymal adipose tissue (EAT) (C) and mesenteric adipose tissue (MAT) (D) were measured after 3 weeks of treatment with OLL2712 and compared with the control group. The SVF cells were isolated from the MAT. The mRNA expression of CCL2 (Ccl2) (E), IL-1β (Il1b) (F), TNF (Tnf) (G), and F4/80 (F4/80) (H) was measured by qPCR. The mRNA expression of CCL2 (Ccl2) (I), IL-1β (Il1b) (J), TNF (Tnf) (K), and F4/80 (F4/80) (L) in colon tissue was measured by qPCR. Macrophages were isolated from PPs in the mice. The mRNA expression of CCL2 (Ccl2) (M), IL-1β (Il1b) (N), TNF (Tnf) (O), and IL-6 (Il6) (P) was measured by qPCR. The results are representative of two independent experiments and are shown as the mean ± standard deviation (n = 8 - 12). *p<0.05; **p<0.01; ***p<0.001 (assessed using Student’s t-test). HFD, high-fat diet; LAB, lactic acid bacteria (L. plantarum OLL2712); MAT, mesenteric adipose tissue; SVF, stromal vascular fraction; PP, Peyer’s patch.
To investigate the effects of a short-term oral administration of L. plantarum OLL2712 in mice, L. plantarum OLL2712, suspended in the sterilized water to the concentration of 20 mg/mL, was orally administered every day, 4 mg to each C57BL/6NCrl mouse from 9-week-old (Figure S1B). 5 individuals were used for each group.
Epididymal adipose tissue (EAT) and mesenteric adipose tissue (MAT) were shredded into 2-3 mm pieces and dissociated with collagenase type II (1 mg/mL; Sigma-Aldrich, USA, Cat#C6885) at 37°C for 45-60 min until the adipose tissue was almost dissolved, and then the reaction was stopped with EDTA (10 mM) for 5 min. After being filtered with a 115 µm nylon mesh (Tokyo Screen, Japan), stromal vascular fraction (SVF) derived from adipose tissue was treated with red blood cell lysis buffer, made from ammonium chloride, potassium carbonate, and EDTA, for 5 min at room temperature. After centrifugation, the EAT SVF and MAT SVF were suspended in 10% FCS-RPMI.
Peyer’s patches (PPs) were treated with collagenase I (1 mg/mL; FUJIFILM Wako Pure Chemical, Japan, Cat#032-22364) and 10 μg/mL DNase I (Roche Diagnostics, Germany, Cat#10104159001) at 37°C for 60–90 min before being filtered with an 86 µm nylon mesh (Tokyo Screen, Japan). The PP cells were centrifuged twice and suspended in 10% FCS-RPMI. 10% FCS-RPMI was prepared using RPMI 1640 (Nissui Pharmaceutical, Japan, Cat#05918), containing 100 U/ml penicillin G potassium (Meiji Seika Pharma, Japan), 100 μg/ml streptomycin sulfate (Meiji Seika Pharma, Japan), 50 μM 2-mercaptoethanol (FUJIFILM Wako Pure Chemical, Japan, Cat#137-06862), 0.03% L-glutamine (FUJIFILM Wako Pure Chemical, Japan, Cat#074-00522), and 0.2% sodium hydrogen carbonate (FUJIFILM Wako Pure Chemical, Japan, Cat#191-01305), and 10% heat-inactivated fetal calf serum (Thermo Fisher Scientific, Germany, Cat#173012).
After F4/80 MicroBeads Ultrapure (Miltenyi Biotec, Germany, Cat#130-110-443) were added to PP whole cells, F4/80+ cells were isolated using a magnetic-activated cell sorting (MACS) system (Miltenyi Biotec, Germany). The obtained F4/80+ cells were used as macrophages.
The intestinal contents were removed, and the intestinal tract was washed with PBS (-), added to TRIzol (Invitrogen, USA, 15596026), and homogenized using TissueRuptor (QIAGEN, Germany) until the tissue was barely visible. The intestinal tissue was immediately frozen in liquid nitrogen and stored at -80°C.
The intestinal tissue samples were thawed at 4°C. Then, 0.2 mL of chloroform (FUJIFILM Wako Pure Chemical, Japan, Cat#038-02606) was added to 1 mL of the sample in TRIzol reagent, and the mixture was stirred manually and kept at room temperature for 3 minutes. After centrifugation, the upper layer was transferred to a new tube, and 0.5 mL of isopropanol (FUJIFILM Wako Pure Chemical, Japan, Cat#166-04836) was added. After being kept at room temperature for 10 min, the sample was centrifuged. The sample was washed with 1 mL of 75% ethanol and dried at room temperature until the precipitate turned translucent. The RNA solution derived from the intestinal tissue was dissolved in sterilized water, and any DNA was removed using an RNase-Free DNase (QIAGEN, Cat#79254).
Total RNA from cells was isolated using QIAshredder (QIAGEN, Germany, Cat#79656), 2-mercaptoethanol (FUJIFILM Wako Pure Chemical, Japan, Cat#137-06862), and an RNeasy mini kit (QIAGEN, Cat#74106) according to the provided protocol. Complementary DNA (cDNA) was synthesized using SuperScript VILO MasterMix (ThermoFisher Scientific, USA, Cat#11755-050) and the GeneAmp PCR System 9700 (Applied Biosystems).
Synthesized cDNA samples were added to a LightCycler 480 Multiwell Plate 96 (Roche Diagnostics), and quantitative PCR was performed with a QuantiTect SYBR Green PCR Kit (QIAGEN, Cat#204143) using a CFX Connect Real-Time PCR Detection System (Bio-Rad, USA). The relative expression levels of each gene were standardized against the gene expression levels of glyceraldehyde-3-phosphate dehydrogenase (GAPDH). The primer sequences for quantitative PCR (qPCR) are shown in Table S3.
Intestinal permeability in vivo, was measured using 4 kDa Fluorescein isothiocyanate-dextran (FITC-Dextran) (Sigma, Cat#46944). 4 hours after FITC-dextran was orally administered to mouse (12 mg per mouse), the serum was collected and diluted in a microplate reader (Greiner bio one, Austria), and the fluorescence was measured at 485(ex)/528(em) nm using a SpectraMax iD5 (Molecular Devices, Japan). The concentration of FITC-dextran was then calculated.
Intestinal permeability ex vivo was investigated according to a previous report (22). The whole digestive tract from the stomach to the final part of colon was collected. After MAT was removed, specific intestinal sections were collected. A 4 cm segment under the stomach was selected as the duodenum, a segment from the 5th to the 10th centimetre below the stomach as the jejunum, a 5 cm intestinal section proximal to the cecum as the ileum, and a 5 cm segment below the cecum as the colon (Figure 2B). The collected intestinal tracts were washed by PBS (-) and the contents were gently removed without breaking the intestinal tissues. A 1 mg/mL solution of 4 kDa FITC-dextran was injected into the selected intestinal sections tied with surgical sutures, and each segment was moved to DMEM (Sigma-Aldrich, USA, Cat#11965092) and placed at 37°C (Figure S1C). The concentration of FITC-dextran transported from the lumen to the DMEM was measured every 30 minutes, and the cumulative concentration (Qt) of the DMEM, collected at each time point, was calculated using the following formula.
Figure 2 L. plantarum OLL2712 exhibited the ability to decrease intestinal permeability, especially in the distal intestine. (A) C57BL/6N male mice were treated with OLL2712 (4 mg dissolved in 200 μL water for each dose) for 7 days, and mice treated with water simultaneously were used as a control group (Ctrl) (n = 5). The concentration of FITC-dextran in serum was measured and calculated 4 hours after FITC-dextran was orally administered to mice to investigate epithelial permeability in vivo. (B) Specific intestinal sections were collected, and the permeability of each section was assessed. A 4 cm segment under the stomach was selected as the duodenum, a segment from the 5th to the 10th centimetre below the stomach as the jejunum, a 5 cm intestinal section proximal to the cecum as the ileum, and a 5 cm segment below the cecum as the colon. The apparent permeability of the duodenum (C), jejunum (D), ileum (E), and colon (F) in C57BL/6N male mice fed an HFD and treated with OLL2712 (HFD-LAB) were compared with those fed an HFD and treated with sterilized water (HFD) (n = 9 - 12). The relative expression of ZO-1 (ZO1) (G), Occludin (Ocln) (H), and MUC2 (I) in ileal tissues from ND or HFD-fed mice was measured by qPCR (n = 5 - 6). The relative expression of ZO-1 (ZO1) (J), Occludin (Ocln) (K), and MUC2 (L) in ileal tissues from mice fed HFD and treated with OLL2712 (HFD-LAB) were compared with those fed HFD and treated with sterilized water (HFD) (n = 10). The results are representative of two independent experiments and are shown as the mean ± standard deviation. *p<0.05; **p<0.01 (assessed using Student’s t-test). Ctrl, control; LAB, lactic acid bacteria (L. plantarum OLL2712); ND, normal diet; HFD, high-fat diet.
Qt = (Ct*Vr) + (Ct sum*Vs), where:
Qt = Cumulative concentration at time t
Ct = Concentration at time t (ng/mL)
Vr = Volume at the receiver side (mL)
Ctsum = Sum of all previous Ct
Vs = Volume sampled (mL)
Qt versus time (t) was plotted and the slope (δQt/δt) was calculated. And the apparent permeability (Papp) of each individual intestinal sac was calculated using the following formula:
Papp = (δQt/δt)/(A*Co), where:
A = Area of tissue (cm2)
Co = Initial concentration (ng/mL)
The cecal contents were collected in 1.5 mL tubes and stored at -80°C. The gut microbiota was analysed with next-generation sequencing and amplicon sequencing by TechnoSuruga Laboratory (Japan). DNA was extracted according to the method previously reported (23), using an automated DNA isolation system (GENE PREP STAR PI-480 KURABO, Japan). The details of the analysis were provided by TechnoSuruga Laboratory (Japan). The 341f/R806 primers and dual-index method was used to amplify the V3-V4 regions of Bacterial 16S rRNA (23–26). And then barcoded amplicons were paired-end sequenced on 2×284-bp cycle using the MiSeq system with MiSeq Reagent Kit version 3 (600 Cycle) chemistry. Paired-end sequencing reads were merged by fastq-join ver 1.3.1 with default setting (27).
FASTX-Toolkit ver 0.0.13 was being used to extract joined-reads, which had quality value score of ≥ 20 for more than 99% of the sequence. After the chimeric sequences were deleted with usearch61 (28, 29), nonchimeric reads were submitted for 16S rDNA-based taxonomic analysis using the Ribosomal Database Project ver 2.11 (RDP, RRID : SCR_006633). Finally, Metagenome@KIN Ver 2.2.1 analysis software (World Fusion, Japan) was used to perform the identification with confidence ≥ 0.8.
The results are given as the mean ± SD, and Student’s t-test was used for statistical analyses. A difference was considered significant at p<0.05.
It has been frequently reported that diet-induced obesity is related to chronic inflammation. A long period of intake of HFD, usually more than 12 weeks, could cause abnormal cytokine production, a disorder of lipid metabolism, and elevated blood glucose, followed by disruption of the regulatory mechanism of adipocytokine production (30). To investigate the inflammation in early obesity induced by a short period of HFD ingestion, C57BL/6N mice were fed HFD (60% kcal from fat) for 4 weeks, and mice fed a normal chow diet (AIN-93 M) were used as a control group (ND) (Figure S1A). The mice were weighed every 7 days, and it was found that the HFD group showed increasing body weights (Figure 3A), followed by increasing weight of their EAT and MAT (Figures 3B, C).
Figure 3 Four weeks of intake of a high-fat diet caused inflammation in the stromal vascular fraction (SVF) cells derived from adipose tissue of mice. C57BL/6N male mice were fed a HFD (60% kcal from fat) for 4 weeks from 8 weeks of age. Mice fed a standard chow diet (AIN-93 M) were used as a control group. (A) Mice were weighed once per week. The weights of the epididymal adipose tissue (EAT) (B) and mesenteric adipose tissue (MAT) (C) were measured after 4 weeks on a HFD and compared with the ND group. The stromal vascular fraction (SVF) cells were isolated from the MAT (D–G). The mRNA expression of CCL2 (Ccl2), IL-1β (Il1b), TNF (Tnf) and F4/80 (F4/80) was measured by qPCR. The results are representative of two independent experiments and are shown as the mean ± standard deviation (n = 6). *p<0.05; **p<0.01; ****p<0.0001 (assessed using Student’s t-test). ND, normal diet; HFD, high-fat diet; EAT, epididymal adipose tissue; MAT, mesenteric adipose tissue; SVF, stromal vascular fraction.
We isolated SVF, which contained immune cells, from the EAT and MAT, and the gene expression of proinflammatory cytokines in the EAT SVF and MAT SVF of mice was measured by qPCR (Figures S2A–C, 3D–F). And the gene expression of F4/80 (F4/80), as a marker of macrophages, was measured simultaneously (Figures S2D, 3G). Cytokine chemokine (C-C motif) ligand 2 (CCL2; Ccl2), as a macrophage-specific chemokine, increased with a 4-week HFD diet in EAT SVF and MAT SVF (Figures S2A, 3D). Nevertheless, other major proinflammatory cytokine such as IL-1β (Il1b) and TNF (Tnf), and macrophage marker F4/80 (F4/80) did not show a remarkable change (Figures S2B–D, 3E–G). These data suggested that a short-term of HFD feeding could induce increases in body weight and fat mass, followed by slight increase in inflammation in the adipose-derived SVF by inducing CCL2 (Ccl2).
Many previous studies have reported that both obese patients and obese mice show an increase in Firmicutes and a decrease in Bacteroidetes in their gut microbiota (31, 32). We collected the contents of the cecum from mice fed a HFD for 4 weeks and analysed the gut microbiota composition using next-generation sequencing applications. The relative abundance of Firmicutes was found to increase in the HFD group compared to normal diet (ND) group (Figure 4A), which is a relevant marker of gut dysbiosis, and we detected a descending tendency in the relative abundance of Bacteroidetes (Figure 4B), although there was no change in the Firmicutes/Bacteroidetes ratio (Figure 4C). At the genus level, the mice in the HFD group showed an obvious distinction from the ND group (Figure 4D). The HFD group exhibited a decreasing tendency in the relative abundance of Lactobacillus (p = 0.06, Figure 4E) and the relative abundance of Lactococcus, Lachnospiracea incertae sedis, and Peptococcus which was almost absent in the cecum of the control group, was found to increase with a HFD feeding (Figures 4F, H, J). Although Pseudoflavonifractor did not change (Figure 4I), an increasing tendency induced by HFD was detected in the relative abundance of Clostridium cluster XIVa (p = 0.08, Figure 4G). These data indicated that a short-term HFD feeding had already resulted in a substantially different gut bacterial flora compared with the ND group, which could be involved in inflammation-associated diseases.
Figure 4 Four weeks of intake of a HFD caused significant changes in several genera derived from the cecum of mice. Male C57Bl/6N mice fed a HFD for 4 weeks were compared with mice fed a normal diet. The cecal contents of the mice were isolated, and the gut microbiota was investigated with next-generation sequencing applications. At the phylum level, the relative abundance of Firmicutes (A) and Bacteroidetes (B) and the ratio of the two (C) were calculated. At the genus level, the composition of the gut microbiota of each mouse was analysed and compared (D). The relative abundance of Lactobacillus (E), Lactococcus (F), Clostridium XIVa (G), Lachnospiraceae incertae sedis (H), Pseudoflavonifractor (I), and Peptococcus (J) in the HFD group were calculated and compared with those in the ND group. The results are representative of two independent experiments and are shown as the mean ± standard deviation (n = 6). *p<0.05; **p<0.01 (assessed using Student’s t-test). ND, normal diet; HFD, high-fat diet.
To explore the anti-inflammatory effects of L. plantarum OLL2712, we orally administered the heat-treated strain to mice on a HFD during the last 3 weeks (Figure 1A). We were unable to detect a significant difference in body weight or fat mass between mice treated with L. plantarum OLL2712 and those treated with sterilized water (Figures 1B–D). Nevertheless, in the EAT SVF, the gene expression of F4/80 (F4/80) decreased significantly in the LAB-treated mice (Figure S3D). Although not significant, the gene expression of CCL2 (Ccl2) showed a declining tendency with the LAB treatment (p = 0.05) (Figure S3A), while there was no change found in the gene expression of IL-1β (Il1b) and TNF (Tnf) (Figures S3B, C). Simultaneously, remarkable changes were found in the MAT SVF, as the gene expression of proinflammatory cytokines, CCL2 (Ccl2), IL-1β (Il1b) and TNF (Tnf), and macrophages marker, F4/80 (F4/80), decreased in the LAB group (Figures 1E–H).
Colonic macrophages play important roles in the induction of obesity-associated insulin resistance. Both macrophage-specific CCR2 knockout and intestinal epithelial cell-specific tamoxifen-inducible CCL2 knockout mice have been observed to be resistant to HFD, showing improved glucose and insulin tolerance (18). To investigate the colonic macrophage infiltration underlying dietary obesity, we evaluated the changes of CCL2 and F4/80 under HFD and LAB treatment. Unexpectedly, we did not find colonic inflammation in mice fed an HFD for 4 weeks (Figures S4A–D). Nevertheless, in macrophages derived from PP cells, the gene expression of CCL2 (Ccl2) and TNF (Tnf) was upregulated by dietary-induced obesity (Figures S4E, G), suggesting that intestinal inflammation was already elicited in the small intestinal compartment, although there was no detected change in the gene expression of IL-1β (Il1b) and IL-6 (Il6) in PP macrophages (Figures S4F, H).
On the other hand, a 3-week oral administration of L. plantarum OLL2712 elicited decreased expression of CCL2 (Ccl2), IL-1β (Il1b) and F4/80 (F4/80) (Figures 1I, J, L) in the colon, and TNF (Tnf) did not change in the gene expression levels (Figure 1K). Meanwhile, there was no change detected in the duodenum, jejunum, and ileum (data not shown). From these results, we supposed that the LAB strain had an anti-inflammatory effect on the large intestine of mice. Furthermore, with the oral administration of heat-treated OLL2712, the gene expression of the proinflammatory cytokines IL-1β (Il1b) and TNF (Tnf) were found to be downregulated in PP macrophages (Figures 1N, O), although CCL2 (Ccl2) and IL-6 (Il6) did not change (Figures 1M, P).
We analysed the gut microbiota in the cecum of mice treated daily with heat-treated L. plantarum OLL2712 for 3 weeks compared with the control mice treated with water. At the phylum level, Firmicutes and Bacteroidetes did not show a significant change under the OLL2712 treatment (Figures 5A–C). However, at the genus level, the gut bacterial flora displayed a remarkable difference between mice treated with LAB and the control group treated with water (Figure 5D). The relative abundance of Lactobacillus dramatically increased (Figure 5E). Furthermore, the relative abundance of Clostridium cluster XIVa, Lachnospiracea incertae sedis, and Pseudoflavonifractor, which had been increased by the HFD and are considered to be associated with host inflammation and diseases, showed a significant decrease under OLL2712 treatment (Figures 5G–I). And the relative abundance of Lactococcus showed a declining tendency (p = 0.05) (Figure 5F), while Peptococcus did not change with the LAB treatment (Figure 5J). These data suggested that the oral administration of OLL2712 could modulate the gut microbiota composition related to obesity.
Figure 5 Oral administration of L. plantarum OLL2712 caused changes in the gut microbiota composition of mice. C57BL/6N male mice fed an HFD and treated with OLL2712 were compared with those fed an HFD and treated with sterilized water. The cecal contents of the mice were isolated, and the gut microbiota was investigated with next-generation sequencing applications. At the phylum level, the relative abundance of Firmicutes (A) and Bacteroidetes (B) and the ratio of the two (C) were calculated. At the genus level, the composition of the gut microbiota of each mouse was analysed and compared (D). The relative abundance of Lactobacillus (E), Lactococcus (F), Clostridium XIVa (G), Lachnospiraceae incertae sedis (H), Pseudoflavonifractor (I), and Peptococcus (J) in mice treated with OLL2712 were calculated and compared with those treated with water. The results are representative of two independent experiments and are shown as the mean ± standard deviation (n = 6). *p<0.05; ***p<0.001; ****p<0.0001 (assessed using Student’s t-test). HFD, high-fat diet; LAB, lactic acid bacteria (L. plantarum OLL2712).
According to the experimental results obtained thus far, we confirmed the anti-inflammatory effects of heat-treated OLL2712 on adipose tissue, PP macrophages, and the colon. In addition, the LAB treatment caused a substantial change in the gut microbiome.
Obesity has been reported to increase intestinal permeability, after which the products of obesity-induced gut dysbiosis are allowed to translocate into the bloodstream, adipose tissue, or other organs, as one of the causes of chronic systemic inflammation (15, 33). Since intestinal inflammation and gut dysbiosis both affect the intestinal barrier (34–36), we next assessed the effects of the LAB strain on intestinal permeability. Seven days orally administration of heat-treated OLL2712 (Figure S1B) induced the lower levels of orally administered FITC-dextran in the serum compared to the control group, supporting our hypothesis (Figure 2A).
Since the proinflammatory cytokines in the colon decreased after 3 weeks of LAB treatment, while those in the jejunum did not, there was a possibility that different parts of the gut played different roles and might respond to the LAB strain in different ways. Assessment of the intestinal permeability in the previous in vivo experiment measured overall gastrointestinal absorption without any site specificity.
To further investigate site specificity of the protective effects of LAB treatment on the gut barrier, we collected duodenum, jejunum, ileum, and colon from the LAB treated mice and assessed the permeability ex vivo (Figures 2B, S1C). After 3 weeks of LAB treatment, the permeability of the ileum derived from HFD mice showed a significant reduction (Figure 2E), while the permeability of other segments had barely changed compared to that of HFD mice treated with water (Figures 2C, D, F). To gain mechanistic insight, we investigated the relative expression of barrier-related genes in each intestinal section. In the ileum, the expression of Occludin (Ocln), as one of the proteins forming tight junctions, decreased with a 4-week HFD feeding (Figure 2H). In addition, the gene expression of a secreted mucin with a physical barrier function, MUC2 (Muc2), also decreased with obesity (Figure 2I). And there was no significant change detected in the gene expression of ZO-1 (ZO1) (Figure 2G). The gene expression of ZO-1 (ZO1) and Occludin (Ocln) increased with the LAB treatment (Figures 2J, K), although no significant change was found in MUC2 (Muc2) (Figure 2L). These data suggested that LAB treatment blocked intestinal barrier disruption in the ileum of HFD-fed mice.
This study clarified the mechanism by which oral administration of L. plantarum OLL2712 suppressed obesity-induced inflammation. Ingested OLL2712 might directly regulate the gut microbiota in the large intestine and reduce harmful substances, which are derived from obesity-induced gut dysbiosis and leak into the blood, eventually relieving adipocyte inflammation. Simultaneously, the LAB strain enhanced the intestinal barrier, especially in the ileum, suggesting collaborative modulation of intestinal immune responses by ingested LAB and microbiota. As a result of the enhancement of the gut barrier, the leakage of harmful substances into the bloodstream was reduced, which resulted in anti-inflammatory changes in the adipose tissue.
Obesity, which is usually caused by unhealthy eating habits, can induce chronic inflammation, leading to high risks of metabolic and immunological diseases (37, 38). The suppression and prevention of obesity-induced chronic inflammation by functional components have been frequently investigated (39–41). As functional ingredients, multiple LAB strains have been proven to be anti-inflammatory probiotics (42, 43), among which L. plantarum OLL2712 was focused on due to its good ability to highly induce the anti-inflammatory cytokine IL-10 (19). In recent studies, L. plantarum OLL2712 has been shown to hamper obesity-induced inflammation in vivo, reducing proinflammatory cytokines in murine adipose tissue (20) and human serum (21). In this study, we confirmed the anti-inflammatory effects of the LAB strain in the early period of obesity, focused on the regulatory effects of OLL2712 on the intestinal environment, and investigated the pathway by which this LAB strain exerted anti-inflammatory effects.
First, we fed mice a HFD for 4 weeks to examine the inflammatory responses triggered by early obesity. We found an increasing body weight and an enlarging fat mass in mice, with proinflammatory cytokines increasing in the adipose tissue-derived SVF, such as adipocyte immune cells. With the daily administration of the LAB strain for 3 weeks in the early period of obesity, although there was no alteration found in body weight or fat mass, the expression of macrophage-specific chemokine CCL2 (Ccl2) and proinflammatory cytokine IL-1β (Il1b) decreased, suggesting that the LAB could alleviate the macrophage infiltration and inflammation of adipose tissue caused by obesity.
We treated mice with the heat-treated L. plantarum OLL2712, because in previous studies, it had been found that the strain demonstrated strong anti-inflammatory effects on bone marrow-derived dendritic cells and peritoneal macrophages after being heat-treated to 75°C (19). We considered that the anti-inflammatory effects of the strain were stabilized by this heat treatment.
We believe that orally administered OLL2712 first reached the intestine and did not exert its effect directly on adipose tissue. It is well known that gut bacterial bias and disruption of the intestinal barrier contribute to chronic inflammation in obesity (33, 44–47). Furthermore, it has been suggested that intestinal inflammation precedes the inflammation in the adipose tissue (18). Therefore, we focused on gut microbiota and intestinal inflammation, examining the pathways of L. plantarum OLL2712 before it suppressed adipocyte inflammation.
We collected cecal contents and investigated the microbiota alterations caused by early obesity and the LAB treatment. Lactobacillus showed a significant decrease with HFD and an increase with the LAB treatment. We consider there was a possibility that the large increase in Lactobacillus was partly due to the administration of OLL2712 and simultaneously it was also possibly induced by the change of other strains belonging to Lactobacillus genus. Simultaneously, we detected a significant difference in gut microbiota between the short-term HFD group and the ND group, with an increasing trend in Lactococcus, Clostridium cluster XIVa, Lachnospiracea incertae sedis, and Pseudoflavonifractor. We found those genera changed oppositely in response to treatment with OLL2712. The genera we focused on have been reported to be involved in inflammation-associated diseases. An upregulation of bile acids production was detected in the intestines and feces of obese rodents, being related to the host inflammation, and was reported to be correlated to an increase in abundance of Lactococcus (48). It is known that diet-induced obesity induces the overproduction of Clostridium cluster XIVa (49), increasing the levels of deoxycholic acid, a gut bacterial metabolite that can cause DNA damage and is involved in the enhancement of obesity-associated hepatocellular carcinoma development in mice (50, 51). Lachnospiracea incertae sedis showed an enrichment in faecal samples of NAFLD (nonalcoholic fatty liver disease) patients (52), and Pseudoflavonifractor was reported to increase in the faeces of patients with ulcerative colitis (53). We cannot give a definite answer about whether OLL2712 was used as a food source by other bacteria or not. Nevertheless, there are multiple studies discussing that components derived from heat-sterilized products of LAB might feed intestinal bacteria and change the gut microbiota (54–56), which might be due to the proliferation of the gut bacteria that could easily utilize the active components of the LAB strain.
Furthermore, we examined intestinal inflammation by investigating the intestinal tissue in relation to their permeability as a hallmark of gut barrier enhancement (22). Considering that different parts of the gastrointestinal tract differ not only in their immune response but also in their number and composition of intestinal bacteria, we evaluated the inflammation and barrier function of the duodenum, jejunum, ileum, and colon to determine the effects of OLL2712 on each part of the intestine. We found that anti-inflammatory effects and intestinal barrier-enhancing effects of OLL2712 were exerted differently in the individual intestinal segments. The expression of CCL2 (Ccl2) and IL-1β (Il1b) was found to decrease in colon tissue but not in the small intestine after the LAB treatment. The apparent permeability of the ileum significantly decreased in response to the LAB treatment. Meanwhile, the gene expression of Occludin (Ocln) and MUC2 (Muc2) in ileum tissue declined in the HFD mice, while Occludin (Ocln) increased under the LAB treatment.
Occludin is well known as one of the proteins expressed in the intestine, forming tight junctions together with ZO-1 and claudins, which protect the body from harmful substances and pathogenic bacteria (57). MUC2 is a secreted mucin with a physical barrier function in the intestinal tract. Furthermore, we detected a significant decrease in serum FITC-dextran levels after 7-day treatment with LAB, which suggested that the administration of the LAB strain decreased the overall intestinal permeability (58). Therefore, it was suggested that OLL2712 could enhance the barrier function and alleviate adipocyte inflammation in obese mice by protecting them from harmful substances derived from the intestinal tract. Moreover, we found that such effects of L. plantarum OLL2712 on intestinal permeability were most noticeable in the ileum.
It was interesting that with the administration of L. plantarum OLL2712, the large intestine showed no change in barrier function, but the colonic inflammation was alleviated. Since there was a high possibility that the ingested LAB strain might not directly induce an immune response in the large intestine, our results suggested that the colonic inflammation might be alleviated by OLL2712 through regulating gut microbiota. On the other hand, there was no inflammatory change found in the small intestine tissue except for the PPs, but the barrier function was improved by the LAB strain in the distal part of the small intestine. The large intestine and small intestine are anatomically and functionally distinct (59). Most functions of the large intestine rely on gut bacteria (60), which include fermenting dietary fiber, producing SCFAs, and modulating the immune response (61, 62). On the other hand, the small intestine harbors lower numbers of commensal bacteria, such as segment filamentous bacteria, which mostly participate in the immune response by reacting directly to ingested food (63, 64). The duodenum is connected directly with the stomach, participating in food digestion (65), while the jejunum is believed to be involved in the immune response, as well as nutrient absorption (66). Compared to the jejunum, the ileum is closer to the large intestine, both physically and functionally. Meanwhile, unlike the large intestine, which cannot respond to orally ingested ingredients directly, in the ileum, multiple PPs are highly developed (67), and an immune response could be directly triggered by ingested food. Thus, the ileum, which is located in the final part of the small intestine, is the gut tract both easily influenced by gut microbiota and directly affected by the immunomodulatory effects of ingested components (68).
Therefore, we hypothesized that the administered L. plantarum OLL2712 might decrease intestinal permeability by cooperating with the gut microbiota via modulating the intestinal production of SCFAs. SCFAs, such as acetate and butyrate produced by the balanced bacteria, could inhibit the pathways of hyodeoxycholic acid (HDCA) or NF-κB to alleviate the intestinal inflammation and enhance the gut barrier (69). On the other hand, OLL2712 might also enhance the barrier by inducing the production of IgA, which may protect the intestinal epithelial cells from LPS and pathogenic bacteria and alleviated the intestinal inflammation (70). Nevertheless, we consider there was still a possibility that OLL2712 function directly on the intestinal epithelial cells via Toll-like receptors (especially TLR2) or Nod-like receptors, well known as the pathways through which the intestinal epithelial cells recognized the bacteria (71–73). Further studies especially in vitro experiments to co-culture SCFAs or the intestinal contents and OLL2712, are needed to confirm the hypothesis.
The results of this research suggested that OLL2712 reached the small intestine, alleviating inflammation and cooperating with the gut bacteria to enhance barrier function, especially in the ileum. This prevented the leakage of harmful substances, thereby suppressing adipocyte inflammation (Figure 6). If intestinal substances that cooperate with OLL2712 and participate in anti-inflammatory effects can be identified, we could elucidate the mechanisms of the health function of LAB to alleviate metabolic diseases and chronic inflammation.
Figure 6 The pathways by which the lactic acid bacteria (LAB) strain exerted its anti-inflammatory effects. Ingested OLL2712 might directly regulate the gut microbiota in the large intestine and reduce harmful substances, which are derived from obesity-induced gut dysbiosis and leak into the blood, eventually relieving adipocyte inflammation. Simultaneously, the LAB strain enhanced the intestinal barrier, especially in the ileum, suggesting collaborative modulation of intestinal immune responses by ingested lactic acid bacteria and microbiota. The enhancement of the gut barrier reduced the leakage of harmful substances into the bloodstream, which resulted in anti-inflammatory changes in the adipose tissue.
The data supporting this study are available from the corresponding author upon request.
The animal study was reviewed and approved by the Experimental Animal Ethics Committee of the Graduate School of Agriculture and Life Sciences of the University of Tokyo.
YW and SH conceived this study. YW designed the research studies. YW, TTa, YZ and RW performed the experiments. YW analyzed the data and wrote the manuscript. TTo and TS prepared materials and reviewed the manuscript. TTa, YZ, RW, HN-A, TM, MT and SH reviewed the manuscript. All authors contributed to the article and approved the submitted version.
This research was partly supported by Grant-in-Aid for Scientific Research (B) (Grant numbers 18H02152 and 22H02283) from the Japan Society for the Promotion of Science (JSPS) and a grant from the Food Science Institute Foundation (Ryoushoku-kenkyukai).
The authors thank Arisa Yoshida and Hibine Mizobuchi for their technical assistance.
TTo and TS are employees of Meiji Holdings Co., Ltd. SH received a grant from Meiji Holdings Co., Ltd.
The remaining authors declare that the research was conducted in the absence of any commercial or financial relationships that could be construed as a potential conflict of interest.
All claims expressed in this article are solely those of the authors and do not necessarily represent those of their affiliated organizations, or those of the publisher, the editors and the reviewers. Any product that may be evaluated in this article, or claim that may be made by its manufacturer, is not guaranteed or endorsed by the publisher.
The Supplementary Material for this article can be found online at: https://www.frontiersin.org/articles/10.3389/fimmu.2023.1123052/full#supplementary-material
1. Caballero B. Humans against obesity: Who will win? Adv Nutr (2019) 10(suppl_1):S4–9. doi: 10.1093/advances/nmy055
2. Apovian CM. Obesity: definition, comorbidities, causes, and burden. Am J Manag Care (2016) 22(7 Suppl):s176–85.
3. de Heredia FP, Gómez-Martínez S, Marcos A. Obesity, inflammation and the immune system. Proc Nutr Soc (2012) 71(2):332–8. doi: 10.1017/S0029665112000092
4. Deng T, Lyon CJ, Bergin S, Caligiuri MA, Hsueh WA. Obesity, inflammation, and cancer. Annu Rev Pathol (2016) 11:421–49. doi: 10.1146/annurev-pathol-012615-044359
5. Saltiel AR, Olefsky JM. Inflammatory mechanisms linking obesity and metabolic disease. J Clin Invest (2017) 127(1):1–4. doi: 10.1172/JCI92035
6. Hotamisligil GS. Inflammation and metabolic disorders. Nature (2006) 444(7121):860–7. doi: 10.1038/nature05485
7. Gregor MF, Hotamisligil GS. Inflammatory mechanisms in obesity. Annu Rev Immunol (2011) 29:415–45. doi: 10.1146/annurev-immunol-031210-101322
8. Kolb R, Sutterwala FS, Zhang W. Obesity and cancer: Inflammation bridges the two. Curr Opin Pharmacol (2016) 29:77–89. doi: 10.1016/j.coph.2016.07.005
9. Round JL, Mazmanian SK. The gut microbiota shapes intestinal immune responses during health and disease. Nat Rev Immunol (2009) 9(5):313–23. doi: 10.1038/nri2515
10. Obata Y, Furusawa Y, Hase K. Epigenetic modifications of the immune system in health and disease. Immunol Cell Biol (2015) 93(3):226–32. doi: 10.1038/icb.2014.114
11. Winer DA, Luck H, Tsai S, Winer S. The intestinal immune system in obesity and insulin resistance. Cell Metab (2016) 23(3):413–26. doi: 10.1016/j.cmet.2016.01.003
12. Bäckhed F, Ding H, Wang T, Hooper LV, Koh GY, Nagy A, et al. The gut microbiota as an environmental factor that regulates fat storage. Proc Natl Acad Sci U S A (2004) 101(44):15718–23. doi: 10.1073/pnas.0407076101
13. Bäckhed F, Manchester JK, Semenkovich CF, Gordon JI. Mechanisms underlying the resistance to diet-induced obesity in germ-free mice. Proc Natl Acad Sci U S A (2007) 104(3):979–84. doi: 10.1073/pnas.0605374104
14. Le Chatelier E, Nielsen T, Qin J, Prifti E, Hildebrand F, Falony G, et al. Richness of human gut microbiome correlates with metabolic markers. Nature (2013) 500(7464):541–6. doi: 10.1038/nature12506
15. Camilleri M, Madsen K, Spiller R, Greenwood-Van Meerveld B, Verne GN. Intestinal barrier function in health and gastrointestinal disease. Neurogastroenterol Motil (2012) 24(6):503–12. doi: 10.1111/j.1365-2982.2012.01921.x
16. Tsukita S, Tanaka H, Tamura A. The claudins: From tight junctions to biological systems. Trends Biochem Sci (2019) 44(2):141–52. doi: 10.1016/j.tibs.2018.09.008
17. Sato J, Kanazawa A, Ikeda F, Yoshihara T, Goto H, Abe H, et al. Gut dysbiosis and detection of “live gut bacteria” in blood of Japanese patients with type 2 diabetes. Diabetes Care (2014) 37(8):2343–50. doi: 10.2337/dc13-2817
18. Kawano Y, Nakae J, Watanabe N, Kikuchi T, Tateya S, Tamori Y, et al. Colonic pro-inflammatory macrophages cause insulin resistance in an intestinal Ccl2/Ccr2-dependent manner. Cell Metab (2016) 24(2):295–310. doi: 10.1016/j.cmet.2016.07.009
19. Toshimitsu T, Mochizuki J, Ikegami S, Itou H. Identification of a lactobacillus plantarum strain that ameliorates chronic inflammation and metabolic disorders in obese and type 2 diabetic mice. J Dairy Sci (2016) 99(2):933–46. doi: 10.3168/jds.2015-9916
20. Toshimitsu T, Ozaki S, Mochizuki J, Furuichi K, Asami Y. Effects of lactobacillus plantarum strain OLL2712 culture conditions on the anti-inflammatory activities for murine immune cells and obese and type 2 diabetic mice. Appl Environ Microbiol (2017) 83(7):e03001-16. doi: 10.1128/AEM.03001-16
21. Toshimitsu T, Gotou A, Furuichi K, Hachimura S, Asami Y. Effects of 12-wk lactobacillus plantarum OLL2712 treatment on glucose metabolism and chronic inflammation in prediabetic individuals: A single-arm pilot study. Nutrition (2019) 58:175–80. doi: 10.1016/j.nut.2018.07.116
22. Mateer SW, Cardona J, Marks E, Goggin BJ, Hua S, Keely S. Ex vivo intestinal sacs to assess mucosal permeability in models of gastrointestinal disease. J Vis Exp (2016) (108):e53250. doi: 10.3791/53250
23. Takahashi S, Tomita J, Nishioka K, Hisada T, Nishijima M. Development of a prokaryotic universal primer for simultaneous analysis of bacteria and archaea using next-generation sequencing. PloS One (2014) 9(8):e105592. doi: 10.1371/journal.pone.0105592
24. Hisada T, Endoh K, Kuriki K. Inter- and intra-individual variations in seasonal and daily stabilities of the human gut microbiota in Japanese. Arch Microbiol (2015) 197(7):919–34. doi: 10.1007/s00203-015-1125-0
25. Muyzer G, de Waal EC, Uitterlinden AG. Profiling of complex microbial populations by denaturing gradient gel electrophoresis analysis of polymerase chain reaction-amplified genes coding for 16S rRNA. Appl Environ Microbiol (1993) 59(3):695–700. doi: 10.1128/aem.59.3.695-700.1993
26. Caporaso JG, Lauber CL, Walters WA, Berg-Lyons D, Lozupone CA, Turnbaugh PJ, et al. Global patterns of 16S rRNA diversity at a depth of millions of sequences per sample. Proc Natl Acad Sci U S A (2011) 108(Suppl 1):4516–22. doi: 10.1073/pnas.1000080107
27. Aronesty E. Comparison of sequencing utility programs. Open Bioinforma J (2013) 7(1):1–8. doi: 10.2174/1875036201307010001
28. Caporaso JG, Kuczynski J, Stombaugh J, Bittinger K, Bushman FD, Costello EK, et al. QIIME allows analysis of high-throughput community sequencing data. Nat Methods (2010) 7(5):335–6. doi: 10.1038/nmeth.f.303
29. Edgar RC, Haas BJ, Clemente JC, Quince C, Knight R. UCHIME improves sensitivity and speed of chimera detection. Bioinformatics (2011) 27(16):2194–200. doi: 10.1093/bioinformatics/btr381
30. Kawai T, Autieri MV, Scalia R. Adipose tissue inflammation and metabolic dysfunction in obesity. Am J Physiol Cell Physiol (2021) 320(3):C375–91. doi: 10.1152/ajpcell.00379.2020
31. Ley RE, Turnbaugh PJ, Klein S, Gordon JI. Microbial ecology: Human gut microbes associated with obesity. Nature (2006) 444(7122):1022–3. doi: 10.1038/4441022a
32. Ley RE, Bäckhed F, Turnbaugh P, Lozupone CA, Knight RD, Gordon JI. Obesity alters gut microbial ecology. Proc Natl Acad Sci U S A (2005) 102(31):11070–5. doi: 10.1073/pnas.0504978102
33. Portincasa P, Bonfrate L, Khalil M, De Angelis M, Calabrese FM, D’Amato M, et al. Intestinal barrier and permeability in health, obesity and NAFLD. Biomedicines (2021) 10(1):83. doi: 10.3390/biomedicines10010083
34. Tappenden KA. Inflammation and intestinal function: Where does it start and what does it mean? JPEN J Parenter Enteral Nutr (2008) 32(6):648–50. doi: 10.1177/0148607108325177
35. Gomes AC, Hoffmann C, Mota JF. The human gut microbiota: Metabolism and perspective in obesity. Gut Microbes (2018) 9(4):308–25. doi: 10.1080/19490976.2018.1465157
36. Ahmad Kendong SM, Raja Ali RA, Nawawi KNM, Ahmad HF, Mokhtar NM. Gut dysbiosis and intestinal barrier dysfunction: Potential explanation for early-onset colorectal cancer. Front Cell Infect Microbiol (2021) 11:744606. doi: 10.3389/fcimb.2021.744606
37. Iyengar NM, Gucalp A, Dannenberg AJ, Hudis CA. Obesity and cancer mechanisms: Tumor microenvironment and inflammation. J Clin Oncol Off J Am Soc Clin Oncol (2016) 34(35):4270–6. doi: 10.1200/JCO.2016.67.4283
38. Stolarczyk E. Adipose tissue inflammation in obesity: a metabolic or immune response? Curr Opin Pharmacol (2017) 37:35–40. doi: 10.1016/j.coph.2017.08.006
39. Alkhatib A, Tsang C, Tiss A, Bahorun T, Arefanian H, Barake R, et al. Functional foods and lifestyle approaches for diabetes prevention and management. Nutrients (2017) 9(12):1310. doi: 10.3390/nu9121310
40. Kunnumakkara AB, Bordoloi D, Padmavathi G, Monisha J, Roy NK, Prasad S, et al. Curcumin, the golden nutraceutical: Multitargeting for multiple chronic diseases. Br J Pharmacol (2017) 174(11):1325–48. doi: 10.1111/bph.13621
41. Ma L, Sun Z, Zeng Y, Luo M, Yang J. Molecular mechanism and health role of functional ingredients in blueberry for chronic disease in human beings. Int J Mol Sci (2018) 19(9):2785. doi: 10.3390/ijms19092785
42. Torres S, Fabersani E, Marquez A, Gauffin-Cano P. Adipose tissue inflammation and metabolic syndrome. The proactive role of probiotics (2019) 58(1):27–43. doi: 10.1007/s00394-018-1790-2
43. Million M, Angelakis E, Paul M, Armougom F, Leibovici L, Raoult D. Comparative meta-analysis of the effect of lactobacillus species on weight gain in humans and animals. Microb Pathog (2012) 53(2):100–8. doi: 10.1016/j.micpath.2012.05.007
44. Saad MJA, Santos A, Prada PO. Linking gut microbiota and inflammation to obesity and insulin resistance. Physiol (Bethesda) (2016) 31(4):283–93. doi: 10.1152/physiol.00041.2015
45. Holmes E, Kinross J, Gibson GR, Burcelin R, Jia W, Pettersson S, et al. Therapeutic modulation of microbiota-host metabolic interactions. Sci Transl Med (2012) 4(137):137rv6. doi: 10.1126/scitranslmed.3004244
46. Thevaranjan N, Puchta A, Schulz C, Naidoo A, Szamosi JC, Verschoor CP, et al. Age-associated microbial dysbiosis promotes intestinal permeability, systemic inflammation, and macrophage dysfunction. Cell Host Microbe (2017) 21(4):455–66. doi: 10.1016/j.chom.2017.03.002
47. Bischoff SC, Barbara G, Buurman W, Ockhuizen T, Schulzke J-D, Serino M, et al. Intestinal permeability–a new target for disease prevention and therapy. BMC Gastroenterol (2014) 14:189. doi: 10.1186/s12876-014-0189-7
48. Lin H, An Y, Tang H, Wang Y. Alterations of bile acids and gut microbiota in obesity induced by high fat diet in rat model. J Agric Food Chem (2019) 67(13):3624–32. doi: 10.1021/acs.jafc.9b00249
49. Yoshimoto S, Loo TM, Atarashi K, Kanda H, Sato S, Oyadomari S, et al. Obesity-induced gut microbial metabolite promotes liver cancer through senescence secretome. Nature (2013) 499(7456):97–101. doi: 10.1038/nature12347
50. Ridlon JM, Kang D-J, Hylemon PB. Bile salt biotransformations by human intestinal bacteria. J Lipid Res (2006) 47(2):241–59. doi: 10.1194/jlr.R500013-JLR200
51. Payne CM, Weber C, Crowley-Skillicorn C, Dvorak K, Bernstein H, Bernstein C, et al. Deoxycholate induces mitochondrial oxidative stress and activates NF-kappaB through multiple mechanisms in HCT-116 colon epithelial cells. Carcinogenesis (2007) 28(1):215–22. doi: 10.1093/carcin/bgl139
52. Shen F, Zheng R-D, Sun X-Q, Ding W-J, Wang X-Y, Fan J-G. Gut microbiota dysbiosis in patients with non-alcoholic fatty liver disease. Hepatob Pancreat Dis Int (2017) 16(4):375–81. doi: 10.1016/S1499-3872(17)60019-5
53. Masoodi I, Alshanqeeti AS, Ahmad S, Alyamani EJ, Al-Lehibi AA, Qutub AN, et al. Microbial dysbiosis in inflammatory bowel diseases: Results of a metagenomic study in Saudi Arabia. Minerva Gastroenterol Dietol. (2019) 65(3):177–86. doi: 10.23736/S1121-421X.19.02576-5
54. Nam Y, Yoon S, Baek J, Kim J-H, Park M, Hwang K, et al. Heat-killed lactiplantibacillus plantarum LRCC5314 mitigates the effects of stress-related type 2 diabetes in mice via gut microbiome modulation. J Microbiol Biotechnol (2022) 32(3):324–32. doi: 10.4014/jmb.2111.11008
55. Warda AK, Rea K, Fitzgerald P, Hueston C, Gonzalez-Tortuero E, Dinan TG, et al. Heat-killed lactobacilli alter both microbiota composition and behaviour. Behav Brain Res (2019) 362:213–23. doi: 10.1016/j.bbr.2018.12.047
56. Ryu S, Kyoung H, Park K, Oh S, Song M, Kim Y. Postbiotic heat-killed lactobacilli modulates on body weight associated with gut microbiota in a pig model. AMB Express (2022) 12(1):83. doi: 10.1186/s13568-022-01424-8
57. Feldman GJ, Mullin JM, Ryan MP. Occludin: Structure, function and regulation. Adv Drug Delivery Rev (2005) 57(6):883–917. doi: 10.1016/j.addr.2005.01.009
58. Li BR, Wu J, Li HS, Jiang ZH, Zhou XM, Xu CH, et al. In vitro and In vivo approaches to determine intestinal epithelial cell permeability. J Vis Exp (2018) 140:57032. doi: 10.3791/57032
59. Mowat AM, Agace WW. Regional specialization within the intestinal immune system. Nat Rev Immunol (2014) 14(10):667–85. doi: 10.1038/nri3738
60. Vaga S, Lee S, Ji B, Andreasson A, Talley NJ, Agréus L, et al. Compositional and functional differences of the mucosal microbiota along the intestine of healthy individuals. Sci Rep (2020) 10(1):14977. doi: 10.1038/s41598-020-71939-2
61. Martin-Gallausiaux C, Marinelli L, Blottière HM, Larraufie P, Lapaque N. SCFA: Mechanisms and functional importance in the gut. Proc Nutr Soc (2021) 80(1):37–49. doi: 10.1017/S0029665120006916
62. Morrison DJ, Preston T. Formation of short chain fatty acids by the gut microbiota and their impact on human metabolism. Gut Microbes (2016) 7(3):189–200. doi: 10.1080/19490976.2015.1134082
63. Jonsson H. Segmented filamentous bacteria in human ileostomy samples after high-fiber intake. FEMS Microbiol Lett (2013) 342(1):24–9. doi: 10.1111/1574-6968.12103
64. Oemcke LA, Anderson RC, Altermann E, Roy NC, McNabb WC. The role of segmented filamentous bacteria in immune barrier maturation of the small intestine at weaning. Front Nutr (2021) 8:759137. doi: 10.3389/fnut.2021.759137
65. Schulze K. Imaging and modelling of digestion in the stomach and the duodenum. Neurogastroenterol Motil (2006) 18(3):172–83. doi: 10.1111/j.1365-2982.2006.00759.x
66. Watanabe-Asaka T, Hayashi M, Maejima D, Kawai Y, Ohhashi T. From digestion and absorption to innate immunity and health care: Water and food intake may contribute to IL-22 in ILC3-dependent mucosal immunity in the jejunum. J Physiol Sci (2021) 71(1):31. doi: 10.1186/s12576-021-00817-x
67. Griebel PJ, Hein WR. Expanding the role of Peyer’s patches in B-cell ontogeny. Immunol Today (1996) 17(1):30–9. doi: 10.1016/0167-5699(96)80566-4
68. Meyer-Myklestad MH, Medhus AW, Stiksrud B, Lorvik KB, Seljeflot I, Hansen SH, et al. Probiotics to HIV-infected immunological nonresponders: Altered mucosal immunity and microbial diversity restricted to ileum. J Acquir Immune Defic Syndr (2022) 89(1):77–86. doi: 10.1097/QAI.0000000000002817
69. Salinas E, Reyes-Pavón D, Cortes-Perez NG, Torres-Maravilla E, Bitzer-Quintero OK, Langella P, et al. Bioactive compounds in food as a current therapeutic approach to maintain a healthy intestinal epithelium. Microorganisms (2021) 9(8):1634. doi: 10.3390/microorganisms9081634
70. Xiong N, Hu S. Regulation of intestinal IgA responses. Cell Mol Life Sci (2015) 72(14):2645–55. doi: 10.1007/s00018-015-1892-4
71. Podolsky DK, Gerken G, Eyking A, Cario E. Colitis-associated variant of TLR2 causes impaired mucosal repair because of TFF3 deficiency. Gastroenterology (2009) 137(1):209–20. doi: 10.1053/j.gastro.2009.03.007
72. Cario E, Gerken G, Podolsky DK. Toll-like receptor 2 enhances ZO-1-associated intestinal epithelial barrier integrity via protein kinase c. Gastroenterology (2004) 127(1):224–38. doi: 10.1053/j.gastro.2004.04.015
Keywords: obesity, proinflammatory cytokines, macrophages, gut microbiota, intestinal permeability, lactic acid bacteria
Citation: Wang Y, Takano T, Zhou Y, Wang R, Toshimitsu T, Sashihara T, Tanokura M, Miyakawa T, Nakajima-Adachi H and Hachimura S (2023) Orally administered Lactiplantibacillus plantarum OLL2712 decreased intestinal permeability, especially in the ileum: Ingested lactic acid bacteria alleviated obesity-induced inflammation by collaborating with gut microbiota. Front. Immunol. 14:1123052. doi: 10.3389/fimmu.2023.1123052
Received: 13 December 2022; Accepted: 31 January 2023;
Published: 23 February 2023.
Edited by:
Francisco José Pérez-Cano, University of Barcelona, SpainReviewed by:
Julio Plaza-Diaz, Children’s Hospital of Eastern Ontario (CHEO), CanadaCopyright © 2023 Wang, Takano, Zhou, Wang, Toshimitsu, Sashihara, Tanokura, Miyakawa, Nakajima-Adachi and Hachimura. This is an open-access article distributed under the terms of the Creative Commons Attribution License (CC BY). The use, distribution or reproduction in other forums is permitted, provided the original author(s) and the copyright owner(s) are credited and that the original publication in this journal is cited, in accordance with accepted academic practice. No use, distribution or reproduction is permitted which does not comply with these terms.
*Correspondence: Satoshi Hachimura, YWhhY2hpQG1haWwuZWNjLnUtdG9reW8uYWMuanA=
Disclaimer: All claims expressed in this article are solely those of the authors and do not necessarily represent those of their affiliated organizations, or those of the publisher, the editors and the reviewers. Any product that may be evaluated in this article or claim that may be made by its manufacturer is not guaranteed or endorsed by the publisher.
Research integrity at Frontiers
Learn more about the work of our research integrity team to safeguard the quality of each article we publish.