- 1Instituto de Parasitología y Biomedicina López-Neyra, Consejo Superior de Investigaciones Científicas (IPBLN, CSIC), Granada, Spain
- 2Division of Virology, Department of Pathology, University of Cambridge, Cambridge, United Kingdom
The co-occurrence and the similarities between malaria and COVID-19 diseases raise the question of whether SARS-CoV-2 is capable of infecting red blood cells and, if so, whether these cells represent a competent niche for the virus. In this study, we first tested whether CD147 functions as an alternative receptor of SARS-CoV-2 to infect host cells. Our results show that transient expression of ACE2 but not CD147 in HEK293T allows SARS-CoV-2 pseudoviruses entry and infection. Secondly, using a SARS-CoV-2 wild type virus isolate we tested whether the new coronavirus could bind and enter erythrocytes. Here, we report that 10,94% of red blood cells had SARS-CoV-2 bound to the membrane or inside the cell. Finally, we hypothesized that the presence of the malaria parasite, Plasmodium falciparum, could make erythrocytes more vulnerable to SARS-CoV-2 infection due to red blood cell membrane remodelling. However, we found a low coinfection rate (9,13%), suggesting that P. falciparum would not facilitate the entry of SARS-CoV-2 virus into malaria-infected erythrocytes. Besides, the presence of SARS-CoV-2 in a P. falciparum blood culture did not affect the survival or growth rate of the malaria parasite. Our results are significant because they do not support the role of CD147 in SARS-CoV-2 infection, and indicate, that mature erythrocytes would not be an important reservoir for the virus in our body, although they can be transiently infected.
1 Introduction
The emergence of SARS-CoV-2 in late 2019 put the world on alert and research was quickly directed to characterize the mechanisms of entry and infection of this novel coronavirus. First studies published early in the pandemic confirmed that the angiotensin-converting enzyme 2 receptor (ACE2), a known receptor for SARS-CoV (1), was also used by SARS-CoV-2 (2, 3) along with the serine protease TMPRSS2 necessary for processing the viral spike (S) protein. However, further research proposed that different human receptors could facilitate viral invasion of different tissues. These included CD147 or basigin, and neuropillin-1 (4–6).
CD147 is a transmembrane glycoprotein of the immunoglobulin superfamily which is expressed in many cell types, including human erythrocytes. The non-glycosylated protein is 28 kDa but its molecular weight can increase to 42-66 kDa due to different levels of glycosylation. It has been described that CD147 presents two different forms of N-glycosylation, named LG (low glycosylation) and HG (high glycosylation), which are heterogeneously present on different cell types and tissues (7). In the context of COVID-19, it has been proposed that CD147 could act as an alternative route for SARS-CoV-2 entry in different cellular types where the expression of the ACE2 is low (e.g. pulmonary epithelial cells and lymphocytes) (4, 8). However, other studies have not found evidence supporting the role of CD147 as a functional SARS-CoV-2 receptor (9–11). Indeed, no change in susceptibility to SARS-CoV-2 infection was observed when CD147 was removed from the surface of lung epithelial cells (10).
Another line of evidence suggesting that red blood cells might be a competent niche for the novel coronavirus, is the report that human erythroid progenitors can be infected by SARS-CoV-2 via ACE2 expression during erythropoiesis (12). This could be related to the increase of erythroid progenitors in circulation, hypoxia, anaemia and coagulopathies observed in COVID-19 patients (12, 13).
Furthermore, in-silico studies have also proposed that the SARS-CoV-2 S and ORF3 proteins might have the ability to bind and to degrade haemoglobin (14). In addition, the band3 protein on the surface of red blood cells has also been postulated as a potential entry point for SARS-CoV-2 by biophysical modelling of proteins interaction (15); and it has been suggested that SARS-CoV-2 could form pores on the erythrocytes membrane and invade them via the membrane attack complex domain of the S protein (16). All this evidence suggest human erythrocytes could be a competent niche for SARS-CoV-2 via the receptor CD147 or by alternative mechanisms. To our knowledge this hypothesis has not been tested yet.
CD147 is also essential for red blood cells invasion by the malaria parasite Plasmodium falciparum (17) opening up a possible SARS-CoV-2/malaria co-infection scenario. The intra-erythrocytic cycle of P. falciparum takes around 48h to complete and involves several stages. It begins when the invasive form of the parasite, the merozoite, invades a new erythrocyte. In the first 12 hours post-invasion, the parasite develops into the ring form; and at 18 h, the trophozoite. Approximately 36h post invasion, the mitosis occurs and the parasite cell progresses to a multinucleated stage named schizonts which contains new merozoites that are released to the bloodstream to start a new cycle (18). We and others have already reported co-infection cases in malaria endemic countries (19, 20). Apart of this co-occurrence, some computational studies have found immunodominant epitopes shared by SARS-CoV-2 and P. falciparum proteins (21). P. falciparum is also known to remodel the membrane of their erythrocyte host (22, 23) and this could potentially enhance viral invasion by SARS-CoV-2. Experimental studies investigating this hypothesis are missing.
In this work, we tested whether mature erythrocytes could be bound or invaded by SARS-CoV-2, and therefore, serve as a niche or reservoir for the virus. We first carried out in vitro infection experiments with SARS-CoV-2 pseudoparticles in HEK 293T cells transiently expressing the CD147 or the ACE2 receptors. We found that transient expression of ACE2, but not CD147, allowed entry and infection by SARS-CoV-2 pseudoviruses. Although we did not find evidence that CD147 could act as a functional receptor for SARS-CoV-2, we decided to explore the hypothesis of an alternative mechanism of adhesion and/or entry to human erythrocytes using a SARS-CoV-2 wild type virus isolate. We reported adhesion and entry of SARS-CoV-2 to red blood cells, although at low frequency. We also tested if the presence of P. falciparum could make erythrocytes more vulnerable to SARS-CoV-2 infection. We found that P. falciparum did not facilitate the entry of SARS-CoV-2 into erythrocytes, and these cells were not frequently co-infected.
2 Methods
2.1 Cell culture
HEK 293T cells were cultured in Dulbecco’s modified Eagle’s medium (DMEM) with high glucose (Sigma D6429) supplemented with 5% heat-inactivated foetal bovine serum (iFBS) and 1x penicillin-streptomycin (P/S; Sigma P4333). VeroE6 cells were cultured in DMEM (Sigma D6429) supplemented with 10 mM HEPES, 1x MEM non-essential amino acid solution (Sigma M7145), 10% iFBS and 1x P/S.
2.2 Parasite culture
Plasmodium falciparum blood stage cultures (3D7) were maintained in RPMI 1640 (Gibco, ref. 51800-035) supplemented with 25 mM HEPES, 2.1 g/mL NaHCO3, 5% AlbuMAX II (Gibco), 0.37 mM hypoxhantine and 5% human erythrocytes. Cultures were incubated at 37°C under 1% CO2, 3% O2 and 96% N2 gas mixture. Parasite development was monitored by Giemsa staining, and cultures were maintained as previously described (24).
2.3 Human erythrocytes
Human whole blood of healthy donors was obtained from the Andalusian Public Health System Biobank. Blood was centrifuged at 2,000 rpm for 10 min, plasma and buffy coat were removed and the red blood cell pellet was washed three times in RPMI 1640 supplemented with 25 mM HEPES. Finally, the packed red blood cell pellet was resuspended with an equal volume of complete RPMI 1640 medium (cRPMI), this 50% haematocrit medium was stored at 4°C and used within three weeks for parasite culture and SARS-CoV-2 infection experiments.
2.4 Plasmids and lentiviral pseudovirus generation
pNL4-3.Luc.R-E- (NIH-AIDS reagent program, catalog n° 3418) is an HIV-1-luciferase reporter vector. It contains the luciferase gene flanked by the long-terminal regions (LTRs) for integration into the host genome, gag, pol, tat and rev genes. However, it is defective for nef, env and vpr genes, only allowing a single round of replication. To produce infectious pseudovirions, it needs to be co-transfected with a plasmid expressing an envelope protein: the vesicular stomatitis virus glycoprotein (VSV-G) envelope expressing plasmid (pMD2 VSV-G, Addgene, catalog n° 12259) as a positive control; or the pcDNA3.1 SARS-CoV-2 S prot-HA, a plasmid expressing the codon-optimized SARS-CoV-2 spike protein from isolate Wuhan-Hu-1 with the HA-tag. An empty pcDNA3.1(+) (Invitrogen) plasmid was used as a negative control.
Pseudovirus were produced by co-transfection of HEK 293T cells (plated in 6-well plates) with pNL4-3.Luc.R-E- (800 ng) and an envelope plasmid (800 ng): pMD2 VSV-G, pcDNA3.1 SARS-CoV-2 S prot-HA or pcDNA3.1(+) for no-envelope control. Transfection mixtures were made using 7.5 µL of TransIT®-LT1 Transfection Reagent (Mirus) and 250 µL of Opti-MEM I media (Gibco). Mock transfection was performed without plasmids as an additional control. Medium was changed at 24h and pseudovirus-containing supernatants were harvested at 48 h post-transfection, centrifuged at 500 x g for 5 min to remove cellular debris, aliquoted and used immediately or stored at −80°C for later use.
2.5 Reverse transfection of ACE2 or CD147 and TMPRSS2 into HEK293T cells
Transient expression of the human receptors ACE2 or CD147 and the protease TMPRSS2 was performed by reverse transfection the plasmids pcDNA3.1-ACE2, pcDNA3.1-CD147 and pcDNA3.1-TMPRSS2 into HEK 293T cells. 24 hours prior to transfection, cells were plated in a T75 flask to be 70-80% confluent the following day. Next day, cells were trypsinized, counted and resuspended at a density of 1.6 x 105 cells/mL. The transfection master mix was prepared with 5 µg of the plasmid of interest, 45 µL of TransIT®-LT1 Transfection Reagent (Mirus) and 1.5 mL of Opti-MEM media (Gibco), the mixture was incubated 15 min and then 11.04 mL of cell suspension was added to the mix. Cells were plated in quadruplicate in a 96-well plate (100 µL/well) and incubated for 24h.
2.6 Luciferase assay
96-well plates seeded with HEK 293T transfected with the receptors or mock transfected (control cells) were infected with 100 µl of pseudovirus-containing supernatants, plates were incubated for 48h and analysed for luciferase activity using the Luciferase Assay System (E1500, Promega), following the manufacturer’s protocol. Cells were rinsed once in 1x PBS and lysed with 40 μL of lysis reagent per well, 20 μL of cell lysate was transferred to a white 96-well plate and 50 µL of Luciferase Assay reagent was added to each well, luminescence was measured in a TECAN infinite 200 instrument with 7.5 sec of readout time.
2.7 Western blot
HEK 293T cells transfected with 2.5, 3.5 and 5 µg of pcDNA3.1-CD147 were lysed 24h and 48h post-transfection with 1x RIPA buffer (abcam) and 1x Complete EDTA free protease inhibitor cocktail (Roche). Cell lysates were quantified by DC protein Assay (Bio-Rad) following manufacturer’s instructions and then mixed with 4x Laemmli’s sample buffer (Bio-Rad) with 10% β-mercaptoethanol. Protein extracts were loaded and run on 10% SDS-PAGE gels and transferred onto a PVDF membrane (Bio-Rad). Membranes were blocked with 5% skimmed milk/TBST 1X (TBS1X-0.1% Tween 20) for 1 h and then incubated overnight at 4°C with primary antibodies; rabbit anti-CD147 (abcam ab108308; 1:1,000 dilution) and mouse anti-GAPDH (Affinity Biosciences, T0004; 1:10,000 dilution) in blocking solution. Following washing, membranes were incubated with the respective secondary antibodies for 1 h at room temperature; goat anti-rabbit IgG (IRDye® 800CW) (ab216773) and goat anti-mouse IgG (IRDye® 680RD) (ab216776) diluted 1:20,000. After washing, the fluorescence signal was visualized using an Odyssey CLx Imaging System (LI-COR Biosciences).
2.8 SARS-CoV-2 virus culture and infection assays
SARS-CoV-2/NL/2020 strain passage 3 was obtained from the European Virus Archive GLOBAL (EVA). Viral titre was determined as follows by plaque assay (protocol kindly shared by C. Suñé). Confluent monolayers of VeroE6 cells were grown on 6-well plates and incubated with 300 µL of a 10-fold serial dilution of the virus stock in DMEM supplemented with 0.2% BSA, 10 mM HEPES and 1x MEM, for 1 h at 37°C. After the adsorption hour, cells were overlaid with MEM 1x supplemented with 10% FSB, 2 mM Glutamine, 1x penicillin-streptomycin, plus 0.1% agarose and incubated for 72h at 37°C. Next, cells were fixed with 1% glutaraldehyde for 20 min. Medium was removed by aspiration and cells were washed with distilled water. Lysis plaques were stained with 0.1% crystal violet for 30 min at room temperature and washed with distilled water. Plaques were counted and quantified as plaque-forming units (PFU) per mL. To generate viral stocks, SARS-CoV-2 was propagated on VeroE6 cells in DMEM supplemented with 2% FSB, at MOI of 0.01. After 72h the virus-containing supernatant was collected, centrifuged for 10 min at 2.000 rpm, aliquoted and titrated as described above. Aliquots were stored at −80°C for later use.
Erythrocytes, stored in cRPMI medium at 50% haematocrit (see above), were quantified in a Neubauer chamber. 2 x 106 erythrocytes were incubated in protein low bind tubes with SARS-CoV-2 viral supernatant at a MOI 2, for 1h at 37°C with gentle agitation every 15 min to maintain the erythrocytes in suspension. After centrifugation at 1,500 rpm for 3 min, the supernatant was discarded and the erythrocytes were washed four times with 0.5 mL of phenol free RPMI to remove unbound virus. Erythrocytes were then resuspended at 5 x 104 cells/µL and 500,000 cells were seeded on microscope round cover glasses of 12 mm (VWR) previously treated with 50 uL Concanavalin A (Sigma, 5 mg/mL in water) for 20 min at 37°C in 24-well plates. Erythrocytes were allowed to settle for 20 min at 37°C and unbound cells were rinsed with two PBS washes. Seeded cells were fixed with 4% paraformaldehyde (PFA)/0.0075 glutaraldehyde (GA) in PBS for 20 min at 37°C and analysed by indirect immunofluorescence microscopy.
Erythrocytes from a P. falciparum culture were quantified in a Neubauer chamber and the parasitemia was determined by Giemsa-stained blood smears. 2 x 106 erythrocytes from a P. falciparum culture at 5.5% parasitaemia were incubated in protein low bind tubes with SARS-CoV-2 at a MOI 2 for 1h at 37°C with gentle agitation every 15 min to maintain cells in suspension. After incubation, erythrocytes were washed, seeded, and fixed on round cover glasses, as described before. Indirect immunofluorescence was performed using an antibody against the SARS-CoV-2 N protein and DAPI staining for detection of the parasite DNA.
P. falciparum culture at 7% parasitaemia was incubated with SARS-CoV-2 at a MOI 2 for 1h at 37°C in a T-25 flask. A control culture was incubated with DMEM, 2% FSB. After incubation, erythrocytes were centrifuged at 1,500 rpm for 5 min, the red blood cell pellet was resuspended in 500 µL of cRPMI and transferred to a protein low-binding Eppendorf. After centrifugation, erythrocytes were resuspended in 50 µL of cRPMI and diluted with 50 µL of cRPMI medium, 5% haematocrit (to dilute the culture below 3.5% parasitemia), the cell suspension was transferred to a well in a 96-well plate and incubated for 24 and 48 hours at 37°C. Culture development was monitored by Giemsa-stained blood smears and quantified by microscopic examination.
2.9 Immunofluorescence microscopy
Coverslips with fixed erythrocytes were rinsed with PBS twice and permeabilized with 0.1% Triton® X-100 in PBS for 15 min, after three PBS washes, free aldehyde groups were quenched with 30mM glycine for 5 min followed by PBS washes. Then, cells were blocked with 3% BSA/1x PBS for 45 min. The primary antibody, mouse anti-SARS-CoV-2 N protein (abcam, ab281300), was diluted 1:1,000 in antibody solution (1% BSA/0.5% Tween® 20/1x PBS) and centrifuged at 13,300 rpm for 1 min to remove aggregates prior to incubation for 2h at room temperature on a horizontal shaker. After three washes with PBS for 10 min, the secondary antibody goat anti-mouse Alexa Fluor 594 (Invitrogen, A11020), was diluted 1:500 and incubated for 45 min. After three washes with PBS for 10 min, the coverslips were mounted on glass slides using VECTASHIELD® Vibrance™ mounting medium (Vector laboratories). Images were acquired using a fluorescence microscope (DMi8, Leica, Germany), with 100x objective and the LAS X software (Leica). Erythrocytes were visualized by phase contrast (gray). Stacks (0.19-μm z step) acquisition was performed to capture 3D images of the red blood cells. Maximum intensity projections of anti-N and DAPI channels were performed using ImageJ 1.52p software (National Institutes of Health). Quantification of SARS-CoV-2 viral particles bound to the erythrocytes after four washes was performed in maximum intensity projection images. The percentage of erythrocytes with viral particles was calculated counting 2,490 red blood cells and 3,418 red blood cells in the case of the P. falciparum cultures.
2.10 Biosafety statement
All work performed was approved by the local genetic manipulation (GM) safety committee. All work with live SARS-CoV-2 was performed in biosafety laboratory level 3 facilities at the IPBLN, Granada, Spain.
3 Results
3.1 Overexpression of the CD147 receptor in HEK 293T cells does not mediate the infection of SARS-CoV-2 pseudoparticles
Due to contradictory evidence of the function of CD147 as a truly SARS-CoV-2 receptor (9–11), we first investigated whether the transient overexpression of CD147 in HEK 293T cells could mediate the internalization of SARS-CoV-2 pseudovirions. In order to do this, luciferase reporter pseudovirions based in lentiviral vectors were constructed (25) where the cellular entry will be mediated by the expression of the SARS-CoV-2 spike (S) protein. In the same system, the vesicular stomatitis virus glycoprotein (VSV-G) was used as a positive control of infection due to its broad cell-type tropism. In addition, particles without the S protein were generated as a negative control. Infection was measured by a luciferase activity assay at 48 hours post-infection (h p.i.) in HEK 293T cells expressing the ACE2 or the CD147 receptors. The amount of luciferase activity reflects the expression of integrated proviruses (26). We found that only cells expressing the ACE2 receptor and the TMPRSS2 protease were permissive for the entry of SARS-CoV-2 pseudovirions (Figure 1; Supplementary Figure S1). As a positive control, assays were performed with the same amount of VSG-G pseudoviral supernatant, this showed higher luciferase activity in all the assays. In addition, pseudoparticles without an envelope protein were producing a similar level of luciferase activity as the mock supernatant without pseudoparticles.
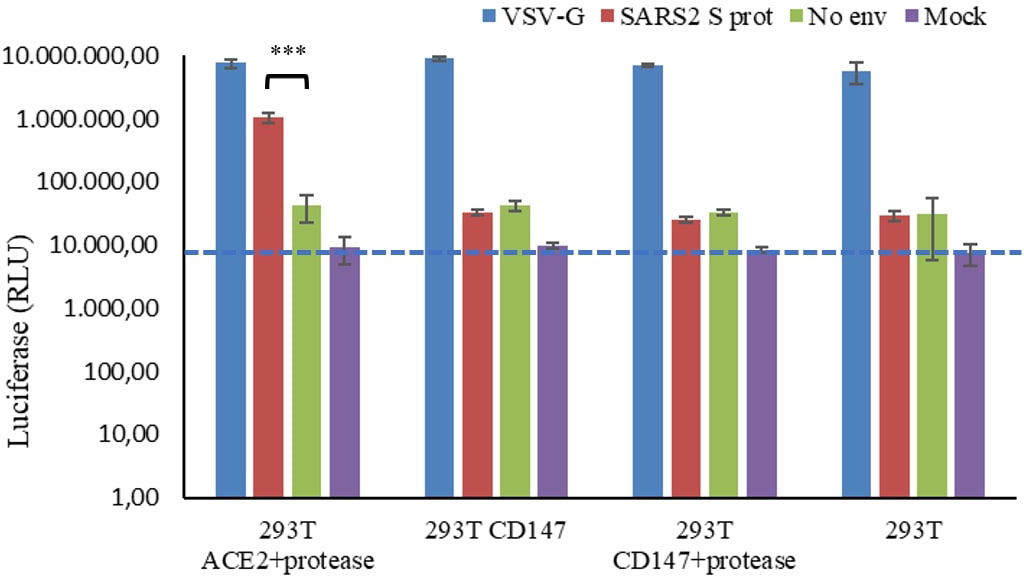
Figure 1 Luciferase activity of SARS-CoV-2 pseudovirus infection in HEK 293T cells expressing the ACE2 o the CD147 receptors. HEK 293T cells were transiently transfected with ACE2 and the protease TMPRSS2, or with CD147 alone or in combination with the TMPRSS2 protease. At 24 h post-transfection, cells were infected with SARS-CoV-2 (SARS2 S prot), VSV-G or naked (No Env) pseudovirions. Mock indicate fresh culture medium. After 48 h, cells were lysed and luciferase activity was measured. The average of three independent experiments conducted with quadruplicate samples are shown. Student’s t-test was performed, ***p<0.001. The blue line indicates background signal of mock infection (no virus). RLU: relative luminescence units in log scale.
As SARS-CoV-2 pseudoparticles were not internalized in the CD147-transfected cells, we decided to test whether CD147 was properly expressed. The expression of CD147 in HEK 293T was confirmed by Western blot analysis (Figure 2). As observed in Figure 2A, when CD147 was transiently overexpressed, most of the protein was in the low glycosylated form (LG-CD147, ~32 kDa), and the amount increased with time. We also analysed the endogenous expression of CD147 in HEK 293T and we found that this cell line expressed low but detectable levels of LG-CD147 and high glycosylated CD147 (HG-CD147, ~42 kDa) (Figure 2B). Despite the expression of the two CD147 isoforms, we found not significant luciferase activity for SARS-CoV-2 pseudovirions when using non-transfected HEK 293T cells (Figure 1). In addition, the transient expression of ACE2 in HEK-293T was also analysed by immunofluorescence and flow cytometry, we found heterogeneous levels of expression in 10% of the transfected population (Supplementary Figure S1). Although the percentage of ACE2 expression was not high, it was enough to detect a 24.6-fold increase of SARS-CoV-2 pseudovirus entry in HEK 293T cells previously transfected with ACE2 and TMPRSS2 compared to the negative control (No env) in luciferase assays (Figure 1).
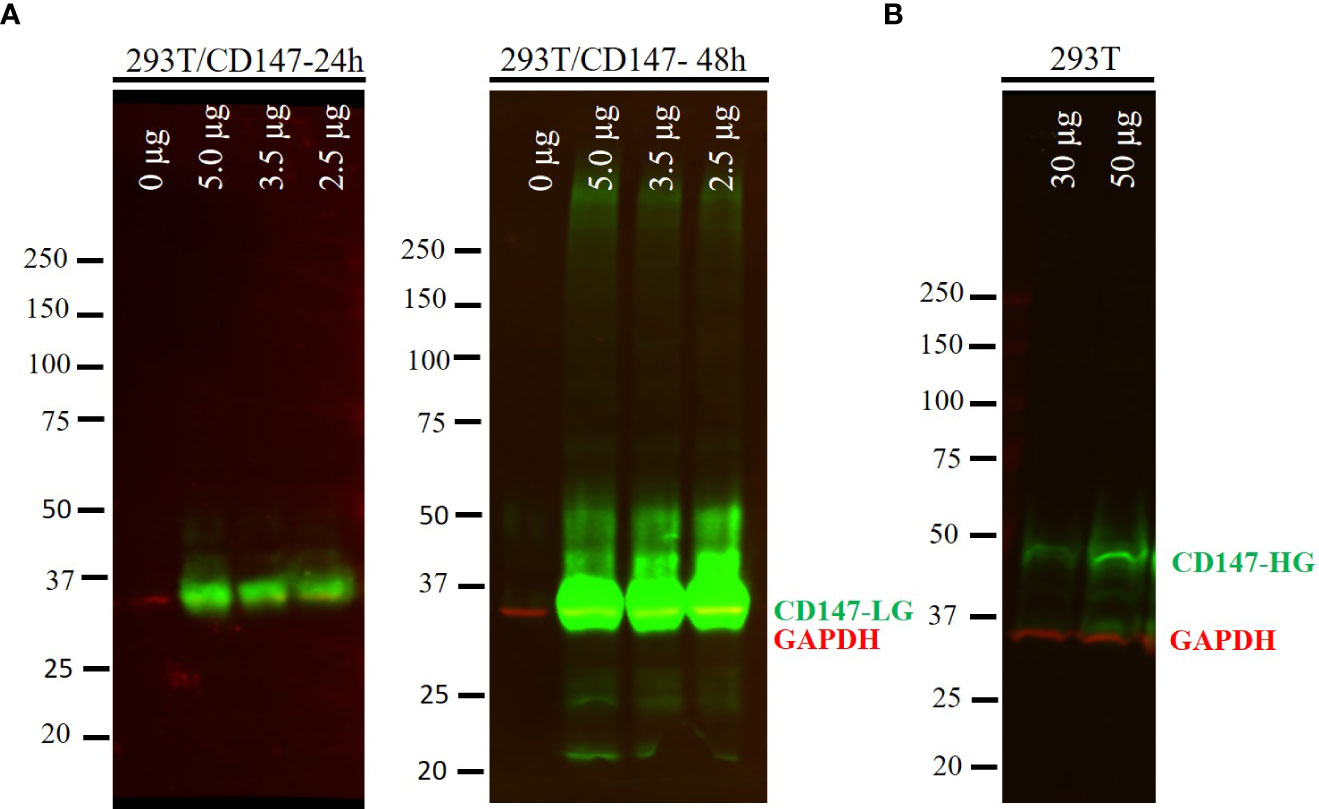
Figure 2 Protein expression of CD147 in HEK 293T cells. (A) HEK 293T cells transfected with different amounts of a plasmid expressing the CD147 protein (0, 2.5, 3.5 and 5.0 µg). Whole cell lysates were harvested at 24 and 48 h post-transfection and analysed by Western blotting. Proteins were loaded at 25 µg per line. (B) Endogenous expression of CD147 in HEK 293T cells was detected loading 30 and 50 µg of the whole cell extract. Low glycosylated CD147 (CD147-LG, ~32 kDa), high glycosylated CD147 (CD147-HG, ~42 kDa). GAPDH (34 kDa) was used as a loading control. Overlay of the simultaneous detection of CD147 (800 nm channel-green) and GAPDH (700 nm channel-red) fluorescent signals.
3.2 Low entry of SARS-CoV-2 to human erythrocytes in vitro
Since CD147 alone does not appear to be a functional receptor for SARS-CoV-2 infection, we explored whether SARS-CoV-2 was able to adhere and/or enter to human erythrocytes via an alternative mechanism. Infection assays were performed with the SARS-CoV-2/NL/2020 strain. Erythrocytes were incubated for 1 h with SARS-CoV-2 at a multiplicity of infection (MOI) of 2. After four washes with phenol red-free RPMI to remove unbound virus, erythrocytes were seeded on microscope coverslips, fixed and analysed by indirect immunofluorescence microscopy (IF) using an antibody against the SARS-CoV-2 nucleocapsid (N) protein. Adhesion and entry of SARS-CoV-2 to red blood cells could be observed (Figure 3) but to a lower rate in comparison with VeroE6 infected under the same conditions (Supplementary Figure S2). After four washes, 10.94% of the erythrocytes (standard deviation, SD 1.56%) had viral particles attached. However, virions were not accumulated inside the erythrocytes or the plasma membrane as observed in VeroE6 cells (Supplementary Figure S2).
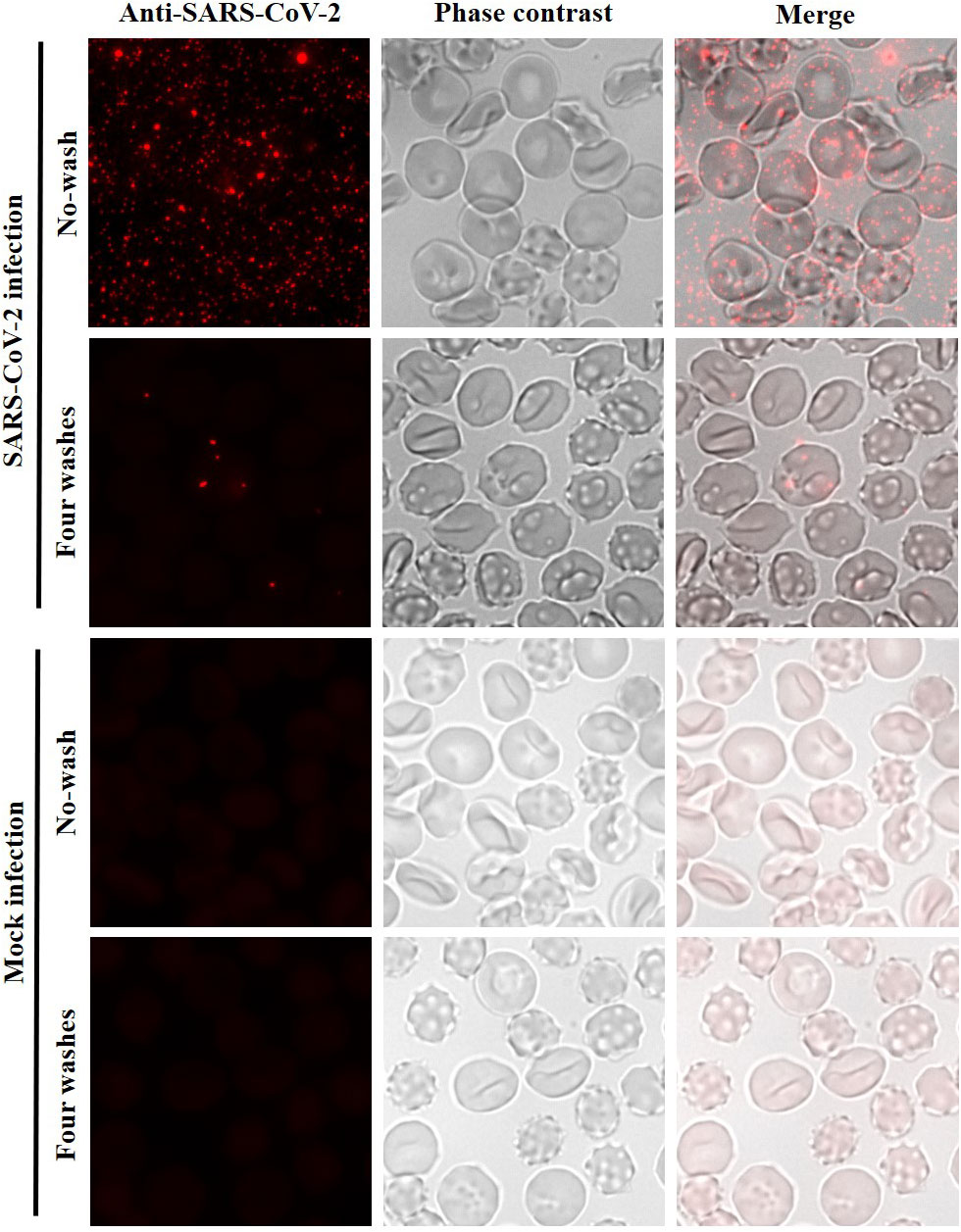
Figure 3 Low entry of SARS-CoV-2 to human erythrocytes. Immunofluorescence analysis using an anti-SARS-CoV-2 nucleocapsid antibody for detection of SARS-CoV-2 in infection assays with human erythrocytes. Erythrocytes were incubated with SARS-CoV-2 at MOI of 2 or with infection medium without virus (mock infection). No washed and four washed samples were analysed. Erythrocytes were visualized by phase contrast (gray). Maximum intensity projections of three-dimensional stacks are shown for the red channel. The percentage of erythrocytes with viral particles (red dots) attached after four washes was calculated counting several fields with a total of 2,490 red blood cells (10.94%; SD 1.56%).
3.3 P. falciparum does not promote the entry of SARS-CoV-2 to erythrocytes
As P. falciparum remodels the erythrocyte membrane (22, 23), this could render malaria-infected red blood cells (iRBCs) more vulnerable to the adhesion and entry of SARS-CoV-2, potentially, promoting co-infection. To test this hypothesis, a P. falciparum culture at 5.5% parasitaemia was incubated for 1 h with SARS-CoV-2 at MOI:2. After incubation, erythrocytes were washed with phenol red-free RPMI, seeded on coverslips, fixed and analysed by IF using a SARS-CoV-2 N antibody for SARS-CoV-2 detection, and DAPI staining for detection of the parasite DNA. As observed in Figure 4, after four washes, few viral particles were detected (1 to 5 particles per cell) in a low percentage of the parasitized red blood cells (9.13%; SD 1.82%). In addition, the potential co-infection of SARS-CoV-2 and P. falciparum in red blood cells was mainly observed during the ring stage of the parasite. These results suggest that the presence of P. falciparum inside erythrocytes does not facilitate or increase the SARS-CoV-2 entry to these cells.
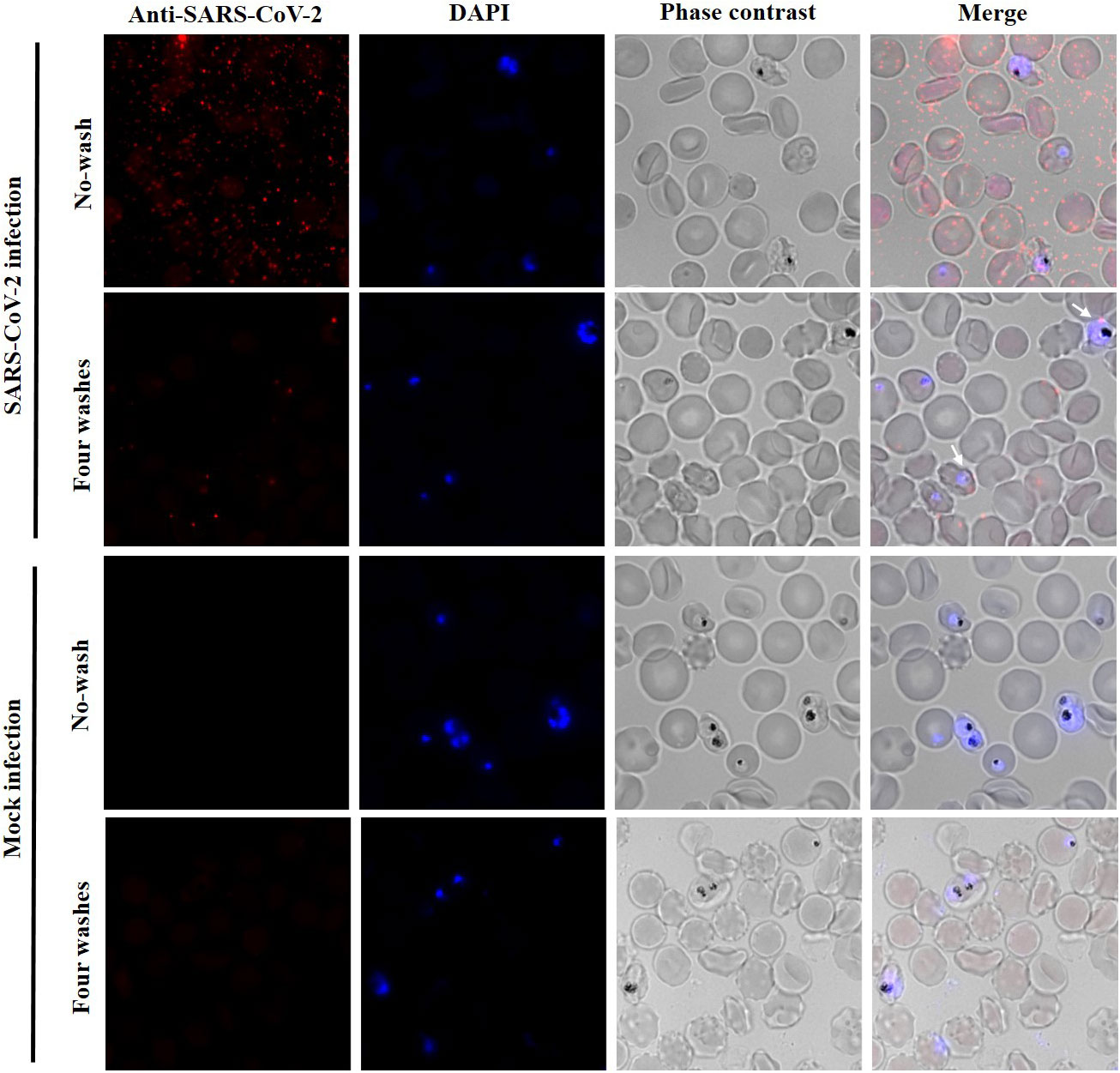
Figure 4 Low adhesion or entry of SARS-CoV-2 to P. falciparum infected erythrocytes. Immunofluorescence analysis with an anti-SARS-CoV-2 nucleocapsid antibody. P. falciparum culture at 5.5% parasitaemia was incubated with SARS-CoV-2 at MOI of 2 or with infection medium without virus (mock infection). No washed and four washed samples were analysed. P. falciparum DNA was stained with DAPI. Erythrocytes were visualized by phase contrast (gray). Maximum intensity projections of three-dimensional stacks are shown for red and DAPI channels. The percentage of P. falciparum infected erythrocytes with viral particles (red dots) attached after four washes was calculated counting several fields with a total of 3,418 red blood cells (9.13%; SD 1.82%).
3.4 SARS-CoV-2 does not impact red blood cell development of P. falciparum
We also wanted to test whether the presence of SARS-CoV-2 in malaria-infected erythrocytes could affect the parasite infection process, especially invasion and growth. To test this, we incubated a P. falciparum culture at high parasitaemia with SARS-CoV-2 for 1 h at MOI:2. After incubation, culture cells were washed once with RPMI and further incubated for 24 and 48 h at 37°C in a 96-well plate. After incubation, culture development was monitored by Giemsa-stained blood smears that were quantified by microscopic examination. We found no significant effect in P. falciparum parasitaemia and cell stages between SARS-CoV-2 pre-incubated cultures and control cultures incubated with infection medium without the virus (Supplementary Table S1).
4 Discussion
The CD147 receptor has been proposed as an alternative receptor for SARS-CoV-2 in cellular types where the ACE2 expression is low (4). However, recent studies have not found enough evidence supporting this idea (9–11). In agreement with this, in this study we report that transient expression of CD147 in HEK 293T cells does not result in SARS-CoV-2 pseudoviruses entry.
We thought that the contradictory results regarding CD147-mediated entry could be due to heterogeneous glycosylation of the expressed protein in different cell lines or tissues analysed (7). In our study, we have confirmed that HEK 293T cells express, although to a lower extent, detectable endogenous levels of two isoforms of CD147: the high glycosylated HG-CD147 form (~42 kDa) and a low glycosylated LG-CD147 form (~32 kDa). It has been reported that different cell types express HG/LG CD147 to different ratios (27). However, we found that neither the endogenous expression nor the overexpression of LG-CD147 in HEK 293T cells was enough to mediate SARS-CoV-2 pseudovirus entry (Figure 1). Indeed, it has been confirmed that HEK 293T are not permissive cells for SARS-CoV-2 infection (28).
Some studies have reported that only fully glycosylated CD147 can be found on plasma membrane and be biologically functional, while other studies have reported that both isoforms can be detected on the plasma membrane (27). It is important to indicate that the overexpression of two highly glycosylated isoforms of recombinant CD147 did not show direct interaction with the S protein (10).
Apart from CD147, alternative mechanisms for SARS-CoV-2 entry to human erythrocytes have been proposed based on computational analysis of epitopes shared by SARS-CoV-2 and P. falciparum proteins (14, 16, 21).
Here, we experimentally tested the hypothesis of a possible scenario of infection of human erythrocytes with SARS-CoV-2. Our immunofluorescence analysis showed that SARS-CoV-2 could adhere and enter to erythrocytes but less efficiently compared to a SARS-CoV-2 permissive cell line such as VeroE6. Viral particles did not accumulate inside red blood cells and only 1-5 viral particles were observed in a low percentage of cells (10.9% of erythrocytes) (Figure 3).
Due to erythrocyte membrane remodelling after P. falciparum infection (23), we also investigated whether malaria-infected red blood cells could be more permissive to viral infection. This could lead to a co-infection scenario by the two pathogens in human red blood cells. However, the incubation of a P. falciparum culture with SARS-CoV-2 showed similar results that incubation with uninfected erythrocytes, and only 9.1% of the parasitized erythrocytes showed viral particles attached (Figure 4), indicating that the interaction between the two pathogens is not frequent.
Our results suggest that mature erythrocytes in the bloodstream are not a critical niche for SARS-CoV-2 that could contribute to the spreading or hiding of the virus. This agrees with previous studies that report low frequency detection and quantity of viral RNA in the blood from COVID-19 patients (29, 30). In addition, PCR-positive plasma was unable to be cultured in cells. This indicates no presence of infective virions in blood from COVID-19 patients or donors (30, 31).
A possible limitation of this study is that experiments with red blood cells were carried out using 2D in vitro cultures, with blood obtained from healthy donors and not with COVID-19 patients. This could be important since damage and clumps of red blood cells in blood vessels have been reported in COVID-19 patient biopsies (32, 33). In addition, complement activation products and S protein binding have been detected in the erythrocyte membrane COVID-19 patients (34). Other possible limitations could be the MOI used and the viral incubation time. Conditions used here were on the high range for the detection of adherence and entry of SARS-CoV-2 to VERO E6, according to previous studies, but there is the possibility that these parameters might not be optimal for erythrocytes.
Our in vitro study complements previous field studies reporting non-negligible but low frequency of SARS-CoV-2 and P. falciparum co-infection in malaria endemic countries in Africa (19, 20). In these studies, the low co-occurrence of COVID-19 and malaria was in part attributed to cross-immunity due to common immunodominant epitopes shared between P. falciparum and SARS-CoV-2 (21, 35–37). Possible mechanisms that would explain the low rate of viral entry to the erythrocytes could be the involvement of secondary SARS-CoV-2 receptors such as neurophilin-1 (5, 6) or the band3 protein, as it has been previously postulated (15).
In this contribution, we designed a novel pseudovirus system that allowed us to show the no involvement of the CD147 receptor in SARS-CoV-2 infection. Secondly, we settled a novel culture approach infecting human red blood cells with a wild type SARS-CoV-2 that allowed us to test a possible co-infection scenario between this novel coronavirus and the human malaria parasite P. falciparum. To our knowledge, this is the first study that has experimentally tested the hypotheses of SARS-CoV-2 erythrocyte invasion and co-infection with the malaria parasite. Our results contribute to a better understanding of the SARS-CoV-2 potential cellular niches, and suggest that the damage to the red blood cells, blood vessels, and other coagulopathies observed in severe COVID-19 patients (32, 38) would not be due to viral infection of red blood cells.
Data availability statement
The original contributions presented in the study are included in the article/Supplementary Material. Further inquiries can be directed to the corresponding authors.
Author contributions
EG-D and NI conceived and designed the original study. DL-F performed the experiments and wrote the manuscript. EG-D and NI supervised the project, edited and reviewed the manuscript. All authors contributed to the article and approved the submitted version.
Funding
This project received funding from the Spanish National Research Council through the grant 202020E159 within the PTI Salud Global initiative and Spanish Ministry of Science and Innovation (grant: PID2019-111109RB-I00).
Acknowledgments
We thank the Andalusian Public Health System Biobank for providing blood samples; Dr Urtzi Garaigorta (CBN-CSIC, Madrid-Spain) for providing VeroE6 cells; Dr Lisa Ranford-Cartwright (Institute of Biodiversity, Animal Health and Comparative Medicine, University of Glasgow) for providing the P. falciparum (3D7) strain; Dr Carles Suñe (IPBLN, Granada-Spain) for kindly share the plaque assay protocol. The European Virus Archive GLOBAL (EVA) for providing us the SARS-CoV-2 isolate. Dr Laura Montosa at the IPBLN microscopy facility for technical support, Dr. María Pérez Sánchez-Cañete at the IPBLN BSL-3 facility and Flow Cytometry service, and Dr Jenny Campos-Salinas (IPBLN, Granada-Spain) for technical advice.
Conflict of interest
The authors declare that the research was conducted in the absence of any commercial or financial relationships that could be construed as a potential conflict of interest.
Publisher’s note
All claims expressed in this article are solely those of the authors and do not necessarily represent those of their affiliated organizations, or those of the publisher, the editors and the reviewers. Any product that may be evaluated in this article, or claim that may be made by its manufacturer, is not guaranteed or endorsed by the publisher.
Supplementary material
The Supplementary Material for this article can be found online at: https://www.frontiersin.org/articles/10.3389/fimmu.2023.1120298/full#supplementary-material
References
1. Li W, Moore MJ, Vasllieva N, Sui J, Wong SK, Berne MA, et al. Angiotensin-converting enzyme 2 is a functional receptor for the SARS coronavirus(2003) (Accessed 2022 Dec 8).
2. Zhou P, Yang X-L, Wang X-G, Hu B, Zhang L, Zhang W, et al. A pneumonia outbreak associated with a new coronavirus of probable bat origin(2012) (Accessed 2022 Dec 8).
3. Hoffmann M, Kleine-Weber H, Schroeder S, Krüger N, Herrler T, Erichsen S, et al. SARS-CoV-2 cell entry depends on ACE2 and TMPRSS2 and is blocked by a clinically proven protease inhibitor(2020) (Accessed 2022 Dec 8).
4. Wang K, Chen W, Zhang Z, Deng Y, Lian JQ, Du P, et al. CD147-spike protein is a novel route for SARS-CoV-2 infection to host cells(2020) (Accessed 2022 Dec 8).
5. Cantuti-Castelvetri L, Ojha R, Pedro LD, Djannatian M, Franz J, Kuivanen S, et al. Neuropilin-1 facilitates SARS-CoV-2 cell entry and infectivity(2020) (Accessed 2022 Aug 9).
6. Daly JL, Simonetti B, Klein K, Chen KE, Williamson MK, Antó;;n-Plá;garo C, et al. Neuropilin-1 is a host factor for SARS-CoV-2 infection. Sci (80-). (2020) 370(6518):861–5.
7. Hu X, Su J, Zhou Y, Xie X, Peng C, Yuan Z, et al. Repressing CD147 is a novel therapeutic strategy for malignant melanoma(2017) (Accessed 2022 Dec 9).
8. Qi F, Qian S, Zhang S, Zhang Z. Single cell RNA sequencing of 13 human tissues identify cell types and receptors of human coronaviruses(2020) (Accessed 2022 Dec 8).
9. Ahmetaj-Shala B, Peacock Bsc TP, Baillon Bsc L, Swann OC, Gashaw H, Barclay WS, et al. Resistance of endothelial cells to SARS-CoV-2 infection in vitro(2020) (Accessed 2022 Aug 9).
10. Shilts J, Crozier TWM, Greenwood EJD, Lehner PJ, Wright GJ. No evidence for basigin/CD147 as a direct SARS-CoV-2 spike binding receptor(2021) (Accessed 2022 Dec 8).
11. Ragotte RJ, Pulido D, Donnellan FR, Hill ML, Gorini G, Davies H, et al. Human basigin (CD147) does not directly interact with SARS-CoV-2 spike glycoprotein(2021) (Accessed 2023 Feb 24).
12. Huerga Encabo H, Grey W, Garcia-Albornoz M, Wood H, Ulferts R, Aramburu IV, et al. Human erythroid progenitors are directly infected by SARS-CoV-2: Implications for emerging erythropoiesis in severe COVID-19 patients(2021) (Accessed 2022 Aug 10).
13. Shahbaz S, Xu L, Osman M, Sligl W, Shields J, Joyce M, et al. Erythroid precursors and progenitors suppress adaptive immunity and get invaded by SARS-CoV-2. Stem Cell Rep (2021) 16(5):1165–81.
14. Wenzhong L, Hualan L. COVID-19: Attacks the 1-beta chain of hemoglobin to disrupt respiratory function and escape immunity. ChemRxiv Cambridge: Cambridge Open Engage (2022). doi: 10.26434/chemrxiv-2021-dtpv3-v11
15. Cosic I, Cosic D, Loncarevic I. RRM prediction of erythrocyte Band3 protein as alternative receptor for SARS-CoV-2 virus. Applied Sciences (2020) 10(11):4053. doi: 10.3390/app10114053
16. Wenzhong L, Hualan Li. COVID-19: Invades erythrocytes through plasmodium falciparum antigen and complement-like system(2021) (Accessed 2022 Dec 9).
17. Crosnier C, Bustamante LY, Bartholdson SJ, Bei AK, Theron M, Uchikawa M, et al. Basigin is a receptor essential for erythrocyte invasion by plasmodium falciparum(2011) (Accessed 2022 Dec 8).
18. Arnot DE. Cell cycle regulation in plasmodium malaria vaccine view project antigenic variation in plasmodium: Review view project(2014) (Accessed 2023 Jan 18).
19. Wilairatana P, Masangkay FR, Kotepui KU, Milanez GDJ, Kotepui M. Prevalence and characteristics of malaria among covid-19 individuals: A systematic review, meta-analysis, and analysis of case reports. PloS Negl Trop Dis (2021) 15(10):1–18. doi: 10.1371/journal.pntd.0009766
20. Ló;;pez-Farfá;n D, Yerbanga RS, Parres-Mercader M, Torres-Puente M, Gó;;mez-Navarro I, Sanou DMS, et al. Prevalence of SARS-CoV-2 and co-infection with malaria during the first wave of the pandemic (the Burkina Faso case)(2022) (Accessed 2023 Jan 18).
21. Iesa MAM, Osman MEM, Hassan MA, Dirar AIA, Abuzeid N, Mancuso JJ, et al. SARS-CoV-2 and plasmodium falciparum common immunodominant regions may explain low COVID-19 incidence in the malaria-endemic belt(2020) (Accessed 2022 Jul 7).
22. Neveu G, Lavazec C. Erythrocyte membrane makeover by plasmodium falciparum gametocytes(2019) (Accessed 2022 Dec 9).
25. Echavarría-Consuegra L, Cook GM, Busnadiego I, Lefèvre C, Keep S, Brown K, et al. Manipulation of the unfolded protein response: A pharmacological strategy against coronavirus infection(2021) (Accessed 2022 Dec 9).
26. Connor RI, Chen BK, Choe S, Landau NR. Vpr is required for efficient replication of human immunodeficiency virus type-1 in mononuclear phagocytes. Virology. (1995) 206(2):935–44.
27. Bai Y, Huang W, Ma LT, Jiang JL, Chen ZN. Importance of n-glycosylation on CD147 for its biological functions(2014) (Accessed 2022 Sep 1).
28. Modrof J, Kerschbaum A, Farcet MR, Niemeyer D, Corman VM, Kreil TR. SARS-CoV-2 and the safety margins of cell-based biological medicinal products. Biologicals. (2020) 68:122–4.
29. Moustafa A, Khalel RS, Aziz RK. Traces of SARS-CoV-2 RNA in peripheral blood cells of patients with COVID-19(2021) (Accessed 2022 Dec 9).
30. Matthews PC, Andersson MI, Arancibia-Carcamo CV, Auckland K, Baillie JK, Barnes E, et al. SARS-CoV-2 RNA detected in blood products from patients with COVID-19 is not associated with infectious virus(2020) (Accessed 2022 Dec 9).
31. Cappy P, Candotti D, Sauvage V, Lucas Q, Boizeau L, Gomez J, et al. No evidence of SARS-CoV-2 transfusion transmission despite RNA detection in blood donors showing symptoms after donation(2020) (Accessed 2022 Dec 9).
32. Reva I, Yamamoto T, Rasskazova M, Lemeshko T, Usov V, Krasnikov Y, et al. Erythrocytes as a target of sars cov-2 in pathogenesis of covid-19(2020) (Accessed 2022 Dec 9).
33. Scheim DE. A deadly embrace: Hemagglutination mediated by SARS-CoV-2 spike protein at its 22 n-glycosylation sites, red blood cell surface sialoglycoproteins, and antibody(2022) (Accessed 2022 Dec 9).
34. Metthew Lam L, Murphy SJ, Kuri-Cervantes L, Weisman AR, G Ittner CA, Reilly JP, et al. Erythrocytes reveal complement activation in patients with COVID-19 (Accessed 2022 Sep 2).
35. Osei SA, Biney RP, Anning AS, Nortey LN, Ghartey-Kwansah G. Low incidence of COVID-19 case severity and mortality in africa; could malaria co-infection provide the missing link? BMC Infect Dis [Internet] (2022) 22(1):1–11. doi: 10.1186/s12879-022-07064-4
36. Achan J, Serwanga A, Wanzira H, Kyagulanyi T, Nuwa A, Magumba G, et al. Current malaria infection, previous malaria exposure, and clinical profiles and outcomes of COVID-19 in a setting of high malaria transmission: An exploratory cohort study in Uganda. Lancet Microbe [Internet]. (2022) 3(1):e62–71. doi: 10.1016/S2666-5247(21)00240-8
37. Raham TF. Influence of malaria endemicity and tuberculosis prevalence on COVID-19 mortality. Public Health [Internet]. (2021) 194:33–5. doi: 10.1016/j.puhe.2021.02.018
Keywords: COVID-19, malaria, novel coronavirus, ACE2, CD147, red blood cells
Citation: López-Farfán D, Irigoyen N and Gómez-Díaz E (2023) Exploring SARS-CoV-2 and Plasmodium falciparum coinfection in human erythrocytes. Front. Immunol. 14:1120298. doi: 10.3389/fimmu.2023.1120298
Received: 09 December 2022; Accepted: 27 February 2023;
Published: 13 March 2023.
Edited by:
Stephanie Yanow, University of Alberta, CanadaReviewed by:
Rafael Freitas-Silva, Medical University of Vienna, AustriaMichael Francis Good, Griffith University, Australia
Copyright © 2023 López-Farfán, Irigoyen and Gómez-Díaz. This is an open-access article distributed under the terms of the Creative Commons Attribution License (CC BY). The use, distribution or reproduction in other forums is permitted, provided the original author(s) and the copyright owner(s) are credited and that the original publication in this journal is cited, in accordance with accepted academic practice. No use, distribution or reproduction is permitted which does not comply with these terms.
*Correspondence: Diana López-Farfán, ZGlhbmEubG9wZXpAaXBiLmNzaWMuZXM=
†These authors have contributed equally to this work and share last authorship