- Department of Orthopedic Surgery, Children’s Hospital, Zhejiang University School of Medicine, National Clinical Research Center for Child Health, Hangzhou, China
Spinal cord injury (SCI) and spinal cord tumor are devastating events causing structural and functional impairment of the spinal cord and resulting in high morbidity and mortality; these lead to a psychological burden and financial pressure on the patient. These spinal cord damages likely disrupt sensory, motor, and autonomic functions. Unfortunately, the optimal treatment of and spinal cord tumors is limited, and the molecular mechanisms underlying these disorders are unclear. The role of the inflammasome in neuroinflammation in diverse diseases is becoming increasingly important. The inflammasome is an intracellular multiprotein complex and participates in the activation of caspase-1 and the secretion of pro-inflammatory cytokines such as interleukin (IL)-1β and IL-18. The inflammasome in the spinal cord is involved in the stimulation of immune-inflammatory responses through the release of pro-inflammatory cytokines, thereby mediating further spinal cord damage. In this review, we highlight the role of inflammasomes in SCI and spinal cord tumors. Targeting inflammasomes is a promising therapeutic strategy for the treatment of SCI and spinal cord tumors.
1 Introduction
Spinal cord injury (SCI) is a devastating event that results in the structural and functional impairment of the spinal cord and may be caused by trauma to or infection or degeneration of the spinal cord (1). SCI incidence is approximately 13 per 100,000 people (2). It results in a varying extent of disability, scoping from partial or complete sensory or motor dysfunction to acute and chronic complications. These complications are accompanied by neuropathic pain, cardiovascular complexities, impaired pulmonary function, pressure ulcers, autonomic dysreflexia, or reduced mobility (3, 4), which have a considerable impact on patients and are an important cause of death after SCI (5). Current treatments for SCI include surgery, drug therapy, and cell therapy. However, these strategies can only improve symptoms and mitigate progression but cannot completely repair the injured spinal cord (6). Thus, SCI can impose a huge psychological and financial burden on individuals as well as society. Taken together, it is necessary to understand the mechanism of SCI and seek an emerging therapeutic blueprint.
The pathological process after SCI can be mainly divided into primary injury and secondary injury. Primary spinal cord injury is caused by the physical injury itself (7), followed by secondary injury caused by a series of biological events, including inflammation, ischemia, oxidative stress, axonal degeneration, astrocyte proliferation, necrosis, apoptosis, and glial scar formation (8). Although the central nervous system (CNS) possesses an innate regenerative capacity, the spinal cord has a poor regenerative ability, which is further complicated by both primary and secondary injuries during SCI. Moreover, the spinal cord regeneration of axons is usually decided by many factors, including the intrinsic growth potential of the CNS neurons, the inhibitory signals produced from CNS myelin damage, reactive astrogliosis, nerve growth factor, and neurotrophic factor (9).
Spinal cord tumors are a heterogeneous group of neoplasms that can be classified into primary and metastatic tumors. Primary spinal tumors account for only approximately 5%–12% of all primary CNS tumors (10) and can be classified into intradural, intramedullary, and extramedullary tumors based on their location. The spinal cord and spine are the common sites of tumor metastasis, and symptomatic metastatic epidural spinal cord compression can occur in 5% to 10% of patients with cancer (11). Owing to the anatomical site of the midline structure, the swelling caused by neuroinflammation is often not tolerated and may result in neurological deficits in both primary and metastatic tumors (12).
SCI and spinal cord tumors are not independent, as spinal tumor-associated compression causes 10% of new-onset SCI and 26% of non-traumatic SCI (13). Patients with spinal tumors might present with acute worsening of neurological function, which often necessitates prompt surgical decompression. Spinal tumors causing SCI are particularly challenging to treat; early diagnosis, multidisciplinary care, and appropriate rehabilitation are necessary to improve the outcomes and quality of life of these patients (14).
The biological process of an immune response is pivotal to the progression and recovery of SCI and the development of spinal cord tumors. Inflammasomes, as innate immune sensors, are multiprotein complexes that play an essential role in defense against pathogens and sterile inflammation (15). Inflammasomes have been demonstrated to be responsible for metabolic diseases, cardiovascular diseases, and tumors (16). However, the mechanism of immune response in SCI and spinal cord tumors remains unclear. This article reviews the mechanism, immune response, and application of inflammasomes in SCI and spinal cord tumors to provide novel insights for the treatment.
2 Inflammasome
The inflammasome is a multi-molecular complex consisting of three units: a sensor, an adaptor molecule called ASC (apoptosis-associated speck-like protein containing a caspase-activation and recruitment domain [CARD]), also known as PYCARD, and pro-caspase-1. Currently, the sensors that have been identified include NLR family pyrin domain containing (NLRP)1, NLRP3, and NOD-like receptor family CARD domain containing 4 (NLRC4)), absent in melanoma 2 (AIM2) or pyrin (17, 18). In line with the activation of caspases during the formation of the inflammasome, the inflammasome can be categorized into two types: classical and non-classical inflammasomes, depending on whether or not the inflammation is mediated by the caspase-1 activation (19). Inflammasome activation is usually triggered by host recognition of danger-related molecular patterns (DAMPs) or pathogen-related molecular patterns (PAMPs), which are ligands for the pattern recognition receptors (PRRs) (20). Before inflammasome activation, the PAMPs/DAMPs signals first “trigger” the innate immune cells, transcriptionally upregulating interleukin (IL)-1β and inflammasome sensor expression. When the triggered cells are stimulated by additional PAMPs/DAMPs, the inflammasome complex assembles and initiates a proteolytic cascade, resulting in the hydrolysis and release of IL-1β and IL-18. Programmed cell death in inflammatory forms, called pyroptosis, usually occurs.
In the CNS, DAMPs and PAMPs are mainly expressed by macrophages, astrocytes, and microglia (21). Recognition of DAMPs and PAMPs can induce the transcription and assembly of inflammasome proteins, such that the precursor of caspase-1 is transformed into active caspase-1 via autocatalysis. Activated caspase-1 regulates the maturation as well as the release of IL-1β, IL-18, and IL-33 (22). Functionally, IL-1β and IL-18 bind to the corresponding receptor to exert their biological effects (23, 24), whereas IL-33 can promote the T-helper 2 (Th2) to release IL-13 and IL-5 (25). In non-classical activation of inflammasomes, the release of IL-1β is mainly mediated by caspases-4 and 5 (26). Consequently, the secretion of these cytokines further induces the cleavage of gasdermin D (GSDMD) and drives the cell toward pyroptosis (27). Alterations of inflammasome-related pathways have been associated with the development and progression of common immune-mediated and neurodegenerative diseases (28), such as Alzheimer’s disease (29), multiple sclerosis (30), chronic brain injury (31), stroke (31), epilepsy (32), Parkinson disease (33), spinal cord diseases (34), and amyotrophic lateral sclerosis (35).
3 Inflammasomes are involved in inflammation and immune response after SCI
SCI can disrupt the homeostasis of the spinal cord microenvironment and result in a series of pathophysiological alterations. Inflammasomes play an essential role in this microenvironmental imbalance, including a shift in the components or their targets, regulation of immune cells, and the secretion of inflammatory factors, thereby impairing regeneration and functional recovery (Figure 1).
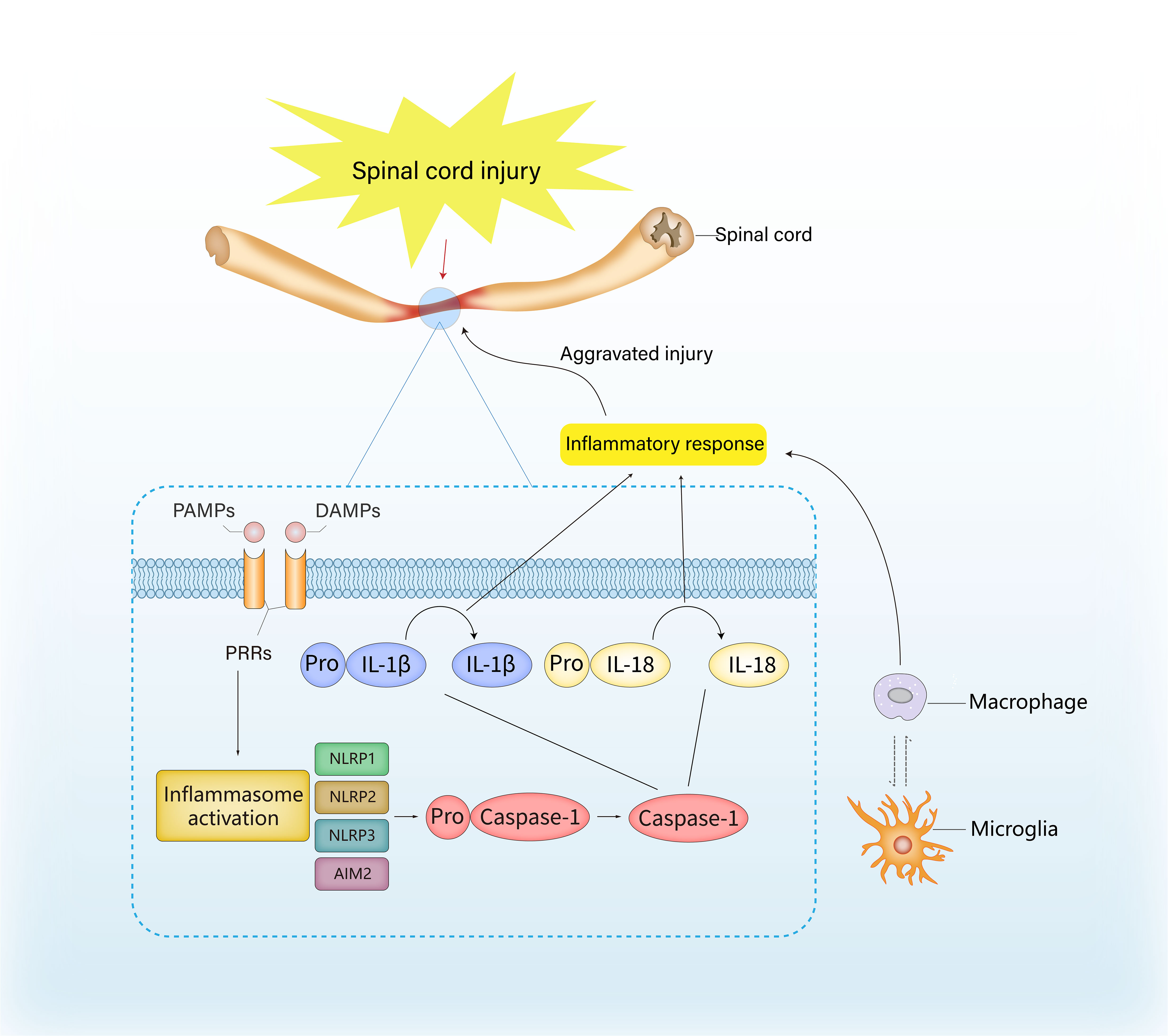
Figure 1 The role of inflammasome in inflammation and immune response after spinal cord injury. The activation of inflammasome is usually launched by host recognition of danger-related molecular patterns (DAMPs) or pathogen-related molecular pattern (PAMPs), which are considered as ligands for the pattern recognition receptors (PRRs). The recognition of DAMPs and PAMPs can induce the transcription and assembly of inflammasome genes, and the precursor of caspase-1 is transformed into active caspase-1 via autocatalysis, thereby resulting in the hydrolysis and release of IL-1β and IL-18, ultimately exacerbating the inflammatory response.
3.1 The role of inflammasomes
3.1.1 NLRP1 inflammasome
As the first inflammasome in the NLR family to be identified in detail, the NLRP1 inflammasome comprises ASC, NLRP1, an inhibitor of apoptosis protein called X-linked inhibitor of apoptosis protein (XIAP), caspase-1, and caspase-11 (36). Structurally, the human NLRP1 protein contains the following protein domains: a CARD, a function to find domain (FIIND), a nucleotide-binding domain (NBD), a leucine-rich repeat (LRR) domain, and an N-terminal PYD (37). NLRP1 inflammasomes have been found in microglia and motor neurons of the spinal cord (17, 38). Significantly enhanced NLRP1 and ASC immunoreactivity has been reported 6 h after moderate experimental cervical SCI, suggesting that SCI can mediate the upregulation of NLRP1 inflammasome (36). Moreover, co-immunoprecipitation of spinal cord lysates and preimmunized serum also revealed overexpression of ASC, NLRP1, and caspase-1 at 24 h after SCI. Interestingly, the cleavage of XIAP into small fragments produces N-terminal baculovirus inhibitor of apoptosis repeat (BIR)1 and BIR2 fragments, which reduce the threshold for caspase-1 activation, resulting in the secretion of IL-1β and IL-18, and aggravation of SCI (39). Additionally, XIAP also mediates innate immune signaling in a receptor interaction protein 2 (RIP2)-dependent manner (40). Vaccari et al. also reported that NLRP1, caspase-1, and ASC levels were elevated after SCI (41).
3.1.2 NLRP2 inflammasome
The NLRP2 inflammasome consists of NLRP2, ASC, and caspase-1, and was discovered in human astrocytes (42). The NLRP2 inflammasome in astrocytes can interact with the P2X7 receptor as well as pannexin-1, which is a transmembrane channel-forming glycoprotein (43). The P2X7 receptor allows the transmembrane fluxes of Ca2+ and causes cellular death and necrosis in neurodegenerative diseases (44). Pannexin-1 participates in intracellular Ca2+ overload during SCI by accelerating extracellular Ca2+ influx, thereby promoting apoptosis of spinal cord neurons (45).
3.1.3 NLRP3 inflammasome
NLRP3, an intracellular receptor, is found in neurons, microglia, and astrocytes (46). NLRP3 becomes activated in response to DAMPs and PAMPs and forms an inflammasome complex with ASC and caspase-1, which subsequently induces the activation and secretion of proinflammatory cytokines (47). The synthesis and activation of the NLRP3 inflammasome usually involve two procedures. First, the initiation of original signaling is triggered by the toll-like receptor/nuclear factor (NF)-κB pathway, increasing inflammasome transcription and promoting posttranslational modifications; this enables the regulation of the expression of NLRP3 inflammasome complexes and the precursors of IL-1β as well as IL-18 under inflammatory situation (48). Consistent with this phenomenon, Ni et al. found a high expression of toll-like receptor 4 and an increase in NF-κB DNA-binding activity at 72 h after SCI (49). The second signal exhibits far-ranging responses to various stimuli, involving the assembly and activation of inflammasome as well as the processing of IL (50). Further, asbestos, extracellular ATP, monosodium urate crystals, the bacterial pore-forming toxin nebramycin, and cholesterol crystals are all known as NLRP3 irritants (51). Huang et al. found that extracellular vesicles derived from epidural fat-mesenchymal stem cells improved neurological functional recovery after SCI, partly by inhibiting the activation of NLRP3 inflammasome (52). Hu et al. demonstrated that NLRP3-related inflammation in motor neurons was induced by microglial activation in the motor cortex, which impaired motor function recovery after SCI. Minocycline inhibited microglia activation, thus reducing NLRP3-related inflammation and promoting functional recovery after SCI (53). More recently, an increasing number of chemicals and molecules, such as trehalose (54), cannabinoid receptor-2 (55), zinc (56), melatonin (57), and dopamine (58), have been found to improve functional recovery after SCI by targeting NLRP3 inflammasome directly or indirectly.
3.1.4 AIM2 inflammasome
AIM2, a cytoplasmic double-stranded DNA (dsDNA) sensor, belongs to the hematopoietic interferon-induced nuclear 200 (HIN200) family; it contributes to the downstream signaling of ASC, which is responsive to the presence of bacterial as well as viral DNA (59). In the normal spinal cord, AIM2 is mainly observed in astrocytes, neurons, and oligodendrocytes (60). AIM2 is also found in activated microglia or macrophages and infiltrated leukocytes during SCI. Moreover, AIM2 can distinguish the DNA released from damaged cells, thereby triggering programmed cell death (61).
3.2 The role of immune cells: Microglia, macrophages, neutrophils, and astrocytes
In the CNS, microglia are the main resident macrophages, which play an important role in the process of secondary injury after SCI, especially by regulating the release of proinflammatory cytokines and chemokines (62). The number of activated microglia was shown to increase on the first day after SCI, which then continued to increase for 7 days until the number of cells stabilized between 2 and 4 weeks (63). In the early stages of SCI, activated microglia release trophic factors that promote axonal growth and regeneration at the lesion site and play a neuroprotective role by limiting lesion site enlargement (64). However, activated microglia can also induce peripheral circulating macrophages to infiltrate the injury site and express several pro-inflammatory cytokines such as IL-1α, IL-1β, and tumor necrosis factor-alpha (TNF-α) to mediate the inflammatory response (65). Both macrophages and microglia exert polarized capability with two major phenotypes, M1 and M2 (65). M1-like cells are classically activated and resemble activated microglia by exerting proinflammatory and destructive effects and producing several proinflammatory cytokines (65). Conversely, alternatively activated M2-like cells have strong protective effects that can promote tissue remodeling, wound healing, and angiogenesis. The microenvironment after SCI is detrimental to M2 macrophages, and the overexpression of TNF can inhibit the conversion of M1 to M2 (66). The ratio of M1 to M2 reflects the proportional balance in the spinal cord microenvironment; a disequilibrium in this proportion causes the release of proinflammatory cytokines, including IL-1β, IL-6, and TNF-α. The interplay between inflammasomes and microglia or macrophages has been described by several studies. Zendedel et al. found that ASC, the inflammasome adaptor protein, was predominantly expressed in microglia after SCI (46). Hu et al. demonstrated that microglial activation triggered NLRP3-related inflammation in the motor cortex after SCI (53). Liu et al. found that oxidation protein products, which served as biomarkers of oxidative stress-triggered inflammatory response after SCI, participated in NLRP3-mediated pyroptosis (67). AIM2 exerts regulatory effects in microglia, which is associated with the development of autoimmune encephalomyelitis in a mouse model (68), indicating that an interplay between AIM2 and microglia may also exist in SCI.
Neutrophils are one of the first immune cells entering the injured site after SCI (69). Infiltrating neutrophils cause damage to the blood-spinal barrier (BBB) and induce the release of many inflammatory factors, triggering a cascade of inflammatory effects (69). Various studies have shown that neuroprotective molecules can protect spinal cord tissue by inhibiting inflammasome activity, which is accompanied by reduced infiltration of neutrophils. OLT1177, a selective inhibitor of the NLRP3 inflammasome, reduced the infiltration of neutrophils and showed a protective role in SCI (70). Similar effects and mechanisms have also been observed for BAY 11-7082 or A438079 (71), topotecan (72), P2X4 receptors (73), asiatic acid (74), and polyphenols (75).
After SCI onset, astrocytes infiltrate the injured tissue, secrete several inflammatory factors, and promote fibrosis (76). Mi et al. found that silencing heat shock protein family A member 8 reduced SCI-caused damage by blocking astrocyte activation and lowing NLRP3 levels; knockdown of this protein protected astrocytes from oxygen and glucose deprivation/reoxygenation-induced injury via the blockade of NF-κB and NLRP3 inflammasome activation (77). ASC-dependent inflammasome formation, especially in resident cells of the spinal cord, including astrocytes, plays a pivotal role in the progression of secondary damage (78). Extracellular vesicles derived from the mesenchymal stem cells have the potential to regulate inflammasome activity after SCI. Moreover, extracellular vesicles stimulate neural progenitor cells and modulate astrocyte activity (79). Oligodendrocyte progenitor cells have significantly higher levels of inflammasome proteins than astrocytes, which may be associated with their high death rates after SCI (80).
3.3 The role of pro-inflammatory or anti-inflammatory cytokines
Cytokines, which can be classified as proinflammatory or anti-inflammatory mediators, are involved in neuroinflammation (81). Various cytokines such as IL-1, IL-6, TNF-α, and leukocyte inhibitory factors are associated with alterations in the microenvironment in SCI (82). When in low concentrations, several proinflammatory cytokines display protective effects by inducing the expression of neurotrophics (83); however, at higher concentrations, these cytokines also mediate the overexpression of neurotoxic genes, such as inducible nitric oxide synthase, proinflammatory proteases, and cyclooxygenase 2 (84). Moreover, IL-1 overexpression in the spinal cord facilitates vascular permeability and lymphocyte recruitment. Additionally, IL-6 promotes the infiltration and activation of macrophages and microglia (85). High levels of TNF-α are found in neurons, glial cells, and endothelial cells after SCI (86). TNF-α can enlist neutrophils to the lesions by inducing adhesion molecules such as intercellular adhesion molecule-1 and vascular cell adhesion protein-1 (87). Subsequently, the permeability of endothelial cells is altered, thereby leading to the disorder of the blood-spinal cord barrier (88). Moreover, TNF-α can induce the death of oligodendrocytes and cause demyelination (89). Generally, CNS cells maintain low IL-1β levels in the brain and spinal cord that are regulated by preassembled inflammasomes (90). Recently, it was shown that expression of NLRP3 inflammasome components increased in the spinal cord tissue of the mouse model of amyotrophic lateral sclerosis and induced superoxide dismutase 1-mediated microglial IL-1β (35).
4 The role of inflammasomes in spinal cord tumor
Aberrant activation of the inflammasomes and concurrent overexpression of their effector molecules have been observed in several malignancies (91). Inflammation is a hallmark of neurodegenerative diseases and central nervous tumors (92). SCI results in persistent inflammatory changes, which suggests that SCI may be a risk factor for central nervous tumors, especially spinal cord tumors. Therefore, in this review, we also summarized the research achievements regarding the role of inflammasomes in spinal cord tumors.
4.1 Tumorigenesis
A common feature of all cancers is their ability to continuously self-proliferate, which is primarily stimulated by inflammation-driven mechanisms (93). The release of proinflammatory cytokines, such as IL-1β and IL-18, may induce cell proliferation in a paracrine and autocrine manner during acute and chronic inflammation (94, 95). NLRP3 inflammasomes have also been reported to inhibit the function of natural killer cells in the control of carcinogenesis and metastasis (96). In addition, the NLRP3 inflammasome exerts a critical effect on tumorigenesis and may provide prognostic markers and promising therapeutic targets in patients with cancer (97). In the CNS, malignant glioma is the most common primary brain tumor with a poor prognosis. The NLRP3 inflammasome in glioma is also constitutively activated in glioblastoma multiforme cells (98). However, the underlying mechanism of the NLRP3 inflammasome in spinal cord tumorigenesis has not been fully elucidated. Further research is needed to understand the properties of the inflammasome and explore its therapeutic potential in spinal cord tumors. In addition to cytokine maturation, another consequence of inflammasome activation is the cleavage of GSDMD; the cleaved GSDMD forms membrane pores that lead to cytokine release and culminate in cell lysis (99). The exact relevance and function of pyroptosis executor GSDMD during tumorigenesis remain unclear. How these different signals are distributed in different tumor environments and ultimately integrated into different cell types requires further investigation (100).
4.2 Metastasis and angiogenesis
Tumor metastasis is an elusive process involving a series of successive events, from the spread of tumor cells from the primary lesion to the development of metastatic foci in distant organs (101). The environment of the distant metastatic target organs experiences reprogramming, primarily through the recruitment of immune cells, in favor of tumor growth. Notably, NLRP3 promotes epithelial–mesenchymal transition by enhancing transforming growth factor-β1 (TGF-β1) signaling and activating small mother against decapentaplegic (SMAD) (102, 103). Angiogenesis, a gradual process of the formation of new capillaries and blood vessels arising from pre-existing vascularity, is necessary for tumor progression (104). It is tightly modulated by multiple pro- and anti-angiogenic factors. Inflammasome complexes contribute to the regulation of angiogenesis in different tissues. IL-1β, produced by tumor cells, induces pro-angiogenic factors. IL-1β mediates the upregulation of hypoxia-inducing factor-1α to stimulate the overexpression of vascular endothelial growth factor (105). Notwithstanding these studies demonstrating the angiogenic function of IL-1β, further exploration is necessary to distinguish how different inflammasome complexes modulate the IIL-1β to regulate angiogenesis in tumors.
4.3 Immunosuppression
In response to the invading tumor cells, the immune system initiates a powerful anti-tumor reaction, and inflammatory cells flood the tumor microenvironment (TME) (106). However, cancer cells use several mechanisms to evade the immune system’s surveillance. The release of IL-1β as well as IL-18, is a universally acknowledged process for the immunosuppressive TME during the development of multiple tumors (107, 108). Bone marrow-derived suppressor cells (MDSCs) are crucial components of TME and demonstrate robust immunosuppressive activity (109). NLRP3 is crucial for accumulating MDSCs in tumors and inhibiting the anti-tumor effect of T cells. Moreover, NLRP1 inflammasome promotes the secretion of IL-18 in myeloma, thus resulting in accelerated progression (110).
In summary, the occurrence of tumor is the result of multiple factors, and long-term exposure to the inflammatory microenvironment will increases the risk of tumor development. As an important component of inflammatory response, inflammasome also involve in the occurrence and development of spinal cord tumors, as well as induce the formation of tumor blood vessels, thereby involving in metastasis. Furthermore, inflammasome may prompt the immunosuppression of TME during the development of spinal cord tumors. Although the role of inflammasome in tumors has received considerable attention, studies in spinal cord tumors are still rare. Therefore, further researches focus on the underlying mechanism of inflammasome in spinal cord tumor are quite necessary.
5 Inhibiting the inflammasome pathways
As mentioned above, the inflammasomes exert a pivotal effect on SCI. Although there are currently no approved inflammasome suppressor drugs for the treatment of SCI, many therapies are in development and show great promise (Table 1).
5.1 NLRP3 inflammasome inhibition
Methylene blue alleviates neuroinflammation post-SCI by inhibiting the activation of the NLRP3 inflammasome in microglia (111). Polydatin, a glycoside of resveratrol, can reduce the activation of the NLRP3 inflammasome and then relieve microglial inflammation, which has a neuroprotective effect on SCI (112). Wogonoside has antioxidant, anti-inflammatory, anti-allergic, and anti-tumor properties (113). Echinoside accelerates the recovery of motor function in rats after SCI by inhibiting the NLRP3 inflammasome (114). Oral glycyrrhizin inhibits NLRP3 inflammasome activation and promotes microglial M2 polarization after a traumatic SC (115). Paeonol may alleviate SCI by regulating the NLRP3 inflammasome and pyroptosis, which is a feasible clinical treatment for SCI (116). Ulinastatin significantly improves neurological function after SCI by regulating the AMP-activated protein kinase/NLRP3 inflammasome signaling pathway (117). MCC950, a small-molecule inhibitor of NLRP3, directly interacts with the Walker B motif within the nucleotide-binding NACHT domain in NLRP3, thereby impeding ATP hydrolysis and inhibiting the synthesis of NLRP3 inflammasome (118, 119). Furthermore, 3,4-methylenedioxy-β-nitrostyrene can block NLRP3-mediated ASC spot formation and oligomerization, but not NLRP3 agonist-induced potassium efflux (120). OLT1177, an orally active β-sulfonyl butyryl molecule, suppresses NLRP3 inflammasome activation. Notably, nanomolar concentrations of OLT1177 can inhibit the secretion of IL-1β and IL-18 following classical and atypical activation of NLRP3 inflammasome in vitro (121). Bigford et al. demonstrated that NLRP3 inflammasome was activated in adipose tissue and pancreas in a chronic SCI mouse model (127). Jiang et al. found that topoisomerase 1 inhibition prevented NLRP3 inflammasome activation and pyroptosis to improve recovery after SCI (128). Antioxidants improved peripheral neuropathy in a tumor-bearing mouse model by regulating spinal cord oxidative stress and inflammation (129). Compared with NLRP3, other inflammasomes have been less studied. Vaccari et al. showed that NLRP1 inflammasome proteins presented in the cerebrospinal fluid of patients with SCI and traumatic brain injury (41). Yutaka et al. found an increased expression level of NLRP2 inflammasome in the dorsal root ganglion, which was associated with inflammatory pain hypersensitivity (130).
5.2 Cytokine inhibition
Production of proinflammatory cytokines such as IL-1β is a pivotal step in the development and progression of various neurological diseases. TNF-α-stimulated gene 6 has been demonstrated to be a promising immunomodulatory target in neurodegenerative diseases (131). In terms of SCI, IL-1β has been proposed as a therapeutic target. In 2020, Tang et al. found that binding of a secretory leukocyte protease inhibitor to the promoter region of TNF-α and IL-8 inhibited the NF-κB signaling pathway, which exerts anti-inflammatory and anti-bacterial effects and promotes recovery after SCI (132). TNF is another pivotal cytokine released after SCI onset, as increased TNF expression has been demonstrated throughout the acute and chronic stages of SCI in the resident cells of spinal tissue. TNF inhibitors, such as adalimumab, infliximab, and etanercept, promoted functional recovery after SCI (122). Yuan et al. found that curcumin inhibited a novel cytokine signaling pathway (TGF-β-SOX9) and improved recovery after SCI (123).
5.3 Caspase-1 inhibition
Previous studies have demonstrated that the absence of caspase-1 can alleviate neuroinflammation and neuronal damage during SCI, which implicates the potential significance of caspase-1 inhibitors as a therapeutic target. Pralnacasan (VX-740) and its analog VX-765 are peptide-like caspase-1 inhibitors that act by covalent modification of the catalytic site of caspase-1, thereby suppressing the activation of caspase-1 and the subsequent cleavage of the precursors of IL-1β and IL-18 (124, 125). Chen et al. demonstrated that VX-765 reduced neuroinflammation in an SCI mouse model by inhibiting caspase-1/IL-1β/IL-18 (126).
6 Perspectives
Aberrantly-activated inflammasomes are involved in SCI and the development of spinal cord tumors; this process depends on several factors, such as the expression patterns and effector molecules of inflammasomes and the profile and composition of the spinal cord microenvironment. However, the current research on inflammasomes in SCI and spinal cord tumors is still scarce, with many unresolved questions, including (1) how is the inflammasome activated in SCI and spinal cord tumors?, (2) what are the effects of the other signaling molecules on the inflammasome and what is the significance of their interaction in the development of SCI and spinal cord tumors?, (3) what is the effect of inflammasome activation in different cell types post-SCI and on the progression of spinal cord tumors?, and (4) what are the effects of each inflammasome pathway on host immunity and immunotherapy? The role of inflammasome-related immune response is less studied, which may be partly due to the difficulty in creating an accurate animal model. With the development of current biotechniques, new models like organoids may be applied to investigate the detailed mechanism in SCI and spinal cord tumors. In this review, we highlight the role of inflammasomes and their effector molecules in SCI and spinal cord tumors. Targeting inflammasomes and effector molecules is expected to bring new hope for treating SCI and spinal cord tumors, which needs to be systematically and comprehensively studied.
Author contributions
JSC search the literature and wrote the draft. WLW conceived and supervised this work. YGS drawn the figure. YGS and XBS critically revised the manuscript. All authors approved the final version.
Conflict of interest
The authors declare that the research was conducted in the absence of any commercial or financial relationships that could be construed as a potential conflict of interest.
Publisher’s note
All claims expressed in this article are solely those of the authors and do not necessarily represent those of their affiliated organizations, or those of the publisher, the editors and the reviewers. Any product that may be evaluated in this article, or claim that may be made by its manufacturer, is not guaranteed or endorsed by the publisher.
Glossary
References
1. Shende P, Subedi M. Pathophysiology, mechanisms and applications of mesenchymal stem cells for the treatment of spinal cord injury. BioMed Pharmacother (2017) 91:693–706. doi: 10.1016/j.biopha.2017.04.126
2. Feigin VL, Nichols E, Alam T, Bannick MS, Beghi E, Blake N, et alGlobal, regional, and national burden of neurological disorders, 1990-2016: A systematic analysis for the global burden of disease study 2016. Lancet Neurol (2019) 18(5):459–80. doi: 10.1016/s1474-4422(18)30499-x
3. McKinley WO, Jackson AB, Cardenas DD, DeVivo MJ. Long-term medical complications after traumatic spinal cord injury: A regional model systems analysis. Arch Phys Med Rehabil (1999) 80(11):1402–10. doi: 10.1016/s0003-9993(99)90251-4
4. Chen Y, Devivo MJ, Jackson AB. Pressure ulcer prevalence in people with spinal cord injury: Age-Period-Duration effects. Arch Phys Med Rehabil (2005) 86(6):1208–13. doi: 10.1016/j.apmr.2004.12.023
5. Meyers AR, Andresen EM, Hagglund KJ. A model of outcomes research: Spinal cord injury. Arch Phys Med Rehabil (2000) 81(12 Suppl 2):S81–90. doi: 10.1053/apmr.2000.20629
6. Shao A, Tu S, Lu J, Zhang J. Crosstalk between stem cell and spinal cord injury: Pathophysiology and treatment strategies. Stem Cell Res Ther (2019) 10(1):238. doi: 10.1186/s13287-019-1357-z
7. Haisma JA, van der Woude LH, Stam HJ, Bergen MP, Sluis TA, Post MW, et al. Complications following spinal cord injury: Occurrence and risk factors in a longitudinal study during and after inpatient rehabilitation. J Rehabil Med (2007) 39(5):393–8. doi: 10.2340/16501977-0067
8. Noreau L, Proulx P, Gagnon L, Drolet M, Laramée MT. Secondary impairments after spinal cord injury: A population-based study. Am J Phys Med Rehabil (2000) 79(6):526–35. doi: 10.1097/00002060-200011000-00009
9. Liu J, Han D, Wang Z, Xue M, Zhu L, Yan H, et al. Clinical analysis of the treatment of spinal cord injury with umbilical cord mesenchymal stem cells. Cytotherapy (2013) 15(2):185–91. doi: 10.1016/j.jcyt.2012.09.005
10. Furlan JC, Wilson JR, Massicotte EM, Sahgal A, Fehlings MG. Recent advances and new discoveries in the pipeline of the treatment of primary spinal tumors and spinal metastases: A scoping review of registered clinical studies from 2000 to 2020. Neuro Oncol (2022) 24(1):1–13. doi: 10.1093/neuonc/noab214
11. Quraishi NA, Arealis G, Salem KM, Purushothamdas S, Edwards KL, Boszczyk BM. The surgical management of metastatic spinal tumors based on an epidural spinal cord compression (Escc) scale. Spine J (2015) 15(8):1738–43. doi: 10.1016/j.spinee.2015.03.040
12. Mount CW, Majzner RG, Sundaresh S, Arnold EP, Kadapakkam M, Haile S, et al. Potent antitumor efficacy of anti-Gd2 car T cells in H3-K27m(+) diffuse midline gliomas. Nat Med (2018) 24(5):572–9. doi: 10.1038/s41591-018-0006-x
13. Ge L, Arul K, Mesfin A. Spinal cord injury from spinal tumors: Prevalence, management, and outcomes. World Neurosurg (2019) 122:e1551–e6. doi: 10.1016/j.wneu.2018.11.099
14. Lawton AJ, Lee KA, Cheville AL, Ferrone ML, Rades D, Balboni TA, et al. Assessment and management of patients with metastatic spinal cord compression: A multidisciplinary review. J Clin Oncol (2019) 37(1):61–71. doi: 10.1200/jco.2018.78.1211
15. Kelley N, Jeltema D, Duan Y, He Y. The Nlrp3 inflammasome: An overview of mechanisms of activation and regulation. Int J Mol Sci (2019) 20(13). doi: 10.3390/ijms20133328
16. Guo H, Callaway JB, Ting JP. Inflammasomes: Mechanism of action, role in disease, and therapeutics. Nat Med (2015) 21(7):677–87. doi: 10.1038/nm.3893
17. Fleshner M, Frank M, Maier SF. Danger signals and inflammasomes: Stress-evoked sterile inflammation in mood disorders. Neuropsychopharmacology (2017) 42(1):36–45. doi: 10.1038/npp.2016.125
18. Ketelut-Carneiro N, Fitzgerald KA. Inflammasomes. Curr Biol (2020) 30(12):R689–r94. doi: 10.1016/j.cub.2020.04.065
19. Szabo G, Csak T. Inflammasomes in liver diseases. J Hepatol (2012) 57(3):642–54. doi: 10.1016/j.jhep.2012.03.035
20. Martinon F, Tschopp J. Inflammatory caspases and inflammasomes: Master switches of inflammation. Cell Death differentiation (2007) 14(1):10–22. doi: 10.1038/sj.cdd.4402038
21. Bryant C, Fitzgerald KA. Molecular mechanisms involved in inflammasome activation. Trends Cell Biol (2009) 19(9):455–64. doi: 10.1016/j.tcb.2009.06.002
22. Mamik MK, Power C. Inflammasomes in neurological diseases: Emerging pathogenic and therapeutic concepts. Brain (2017) 140(9):2273–85. doi: 10.1093/brain/awx133
23. Iannitti RG, Napolioni V, Oikonomou V, De Luca A, Galosi C, Pariano M, et al. Il-1 receptor antagonist ameliorates inflammasome-dependent inflammation in murine and human cystic fibrosis. Nat Commun (2016) 7:10791. doi: 10.1038/ncomms10791
24. Wawrocki S, Druszczynska M, Kowalewicz-Kulbat M, Rudnicka W. Interleukin 18 (Il-18) as a target for immune intervention. Acta Biochim Pol (2016) 63(1):59–63. doi: 10.18388/abp.2015_1153
25. Kotsiou OS, Gourgoulianis KI, Zarogiannis SG. Il-33/St2 axis in organ fibrosis. Front Immunol (2018) 9:2432. doi: 10.3389/fimmu.2018.02432
26. Viganò E, Diamond CE, Spreafico R, Balachander A, Sobota RM, Mortellaro A. Human caspase-4 and caspase-5 regulate the one-step non-canonical inflammasome activation in monocytes. Nat Commun (2015) 6:8761. doi: 10.1038/ncomms9761
27. Shi J, Gao W, Shao F. Pyroptosis: Gasdermin-mediated programmed necrotic cell death. Trends Biochem Sci (2017) 42(4):245–54. doi: 10.1016/j.tibs.2016.10.004
28. Lünemann JD, Malhotra S, Shinohara ML, Montalban X, Comabella M. Targeting inflammasomes to treat neurological diseases. Ann Neurol (2021) 90(2):177–88. doi: 10.1002/ana.26158
29. Shippy DC, Wilhelm C, Viharkumar PA, Raife TJ, Ulland TK. B-hydroxybutyrate inhibits inflammasome activation to attenuate alzheimer's disease pathology. J Neuroinflamm (2020) 17(1):280. doi: 10.1186/s12974-020-01948-5
30. McKenzie BA, Mamik MK, Saito LB, Boghozian R, Monaco MC, Major EO, et al. Caspase-1 inhibition prevents glial inflammasome activation and pyroptosis in models of multiple sclerosis. Proc Natl Acad Sci United States America (2018) 115(26):E6065–e74. doi: 10.1073/pnas.1722041115
31. Kim H, Seo JS, Lee SY, Ha KT, Choi BT, Shin YI, et al. Aim2 inflammasome contributes to brain injury and chronic post-stroke cognitive impairment in mice. Brain Behav Immun (2020) 87:765–76. doi: 10.1016/j.bbi.2020.03.011
32. Zhang H, Yu S, Xia L, Peng X, Wang S, Yao B. Nlrp3 inflammasome activation enhances adk expression to accelerate epilepsy in mice. Neurochem Res (2022) 47(3):713–22. doi: 10.1007/s11064-021-03479-8
33. Panicker N, Kam TI, Wang H, Neifert S, Chou SC, Kumar M, et al. Neuronal Nlrp3 is a parkin substrate that drives neurodegeneration in parkinson's disease. Neuron (2022) 110(15):2422–37.e9. doi: 10.1016/j.neuron.2022.05.009
34. Xu S, Wang J, Zhong J, Shao M, Jiang J, Song J, et al. Cd73 alleviates gsdmd-mediated microglia pyroptosis in spinal cord injury through Pi3k/Akt/Foxo1 signaling. Clin Transl Med (2021) 11(1):e269. doi: 10.1002/ctm2.269
35. Deora V, Lee JD, Albornoz EA, McAlary L, Jagaraj CJ, Robertson AAB, et al. The microglial Nlrp3 inflammasome is activated by amyotrophic lateral sclerosis proteins. Glia (2020) 68(2):407–21. doi: 10.1002/glia.23728
36. de Rivero Vaccari JP, Lotocki G, Marcillo AE, Dietrich WD, Keane RW. A molecular platform in neurons regulates inflammation after spinal cord injury. J Neurosci (2008) 28(13):3404–14. doi: 10.1523/jneurosci.0157-08.2008
37. Martinon F, Burns K, Tschopp J. The inflammasome: A molecular platform triggering activation of inflammatory caspases and processing of proil-beta. Mol Cell (2002) 10(2):417–26. doi: 10.1016/s1097-2765(02)00599-3
38. de Rivero Vaccari JP, Dietrich WD, Keane RW. Therapeutics targeting the inflammasome after central nervous system injury. Transl Res (2016) 167(1):35–45. doi: 10.1016/j.trsl.2015.05.003
39. Deveraux QL, Leo E, Stennicke HR, Welsh K, Salvesen GS, Reed JC. Cleavage of human inhibitor of apoptosis protein xiap results in fragments with distinct specificities for caspases. EMBO J (1999) 18(19):5242–51. doi: 10.1093/emboj/18.19.5242
40. Krieg A, Correa RG, Garrison JB, Le Negrate G, Welsh K, Huang Z, et al. Xiap mediates nod signaling Via interaction with Rip2. Proc Natl Acad Sci United States America (2009) 106(34):14524–9. doi: 10.1073/pnas.0907131106
41. de Rivero Vaccari JP, Brand F 3rd, Adamczak S, Lee SW, Perez-Barcena J, Wang MY, et al. Exosome-mediated inflammasome signaling after central nervous system injury. J Neurochem (2016) 136 Suppl 1(0 1):39–48. doi: 10.1111/jnc.13036
42. Minkiewicz J, de Rivero Vaccari JP, Keane RW. Human astrocytes express a novel Nlrp2 inflammasome. Glia (2013) 61(7):1113–21. doi: 10.1002/glia.22499
43. de Rivero Vaccari JP, Dietrich WD, Keane RW. Activation and regulation of cellular inflammasomes: Gaps in our knowledge for central nervous system injury. J Cereb Blood Flow Metab (2014) 34(3):369–75. doi: 10.1038/jcbfm.2013.227
44. Illes P. P2x7 receptors amplify cns damage in neurodegenerative diseases. Int J Mol Sci (2020) 21(17). doi: 10.3390/ijms21175996
45. Huang Y, Lin J, Chen X, Lin J. Pannexin-1 contributes to the apoptosis of spinal neurocytes in spinal cord injury. Front Physiol (2021) 12:656647. doi: 10.3389/fphys.2021.656647
46. Zendedel A, Mönnink F, Hassanzadeh G, Zaminy A, Ansar MM, Habib P, et al. Estrogen attenuates local inflammasome expression and activation after spinal cord injury. Mol Neurobiol (2018) 55(2):1364–75. doi: 10.1007/s12035-017-0400-2
47. Wallisch JS, Simon DW, Bayır H, Bell MJ, Kochanek PM, Clark RSB. Cerebrospinal fluid Nlrp3 is increased after severe traumatic brain injury in infants and children. Neurocrit Care (2017) 27(1):44–50. doi: 10.1007/s12028-017-0378-7
48. Fann DY, Lim YA, Cheng YL, Lok KZ, Chunduri P, Baik SH, et al. Evidence that nf-Kb and mapk signaling promotes nlrp inflammasome activation in neurons following ischemic stroke. Mol Neurobiol (2018) 55(2):1082–96. doi: 10.1007/s12035-017-0394-9
49. Ni H, Jin W, Zhu T, Wang J, Yuan B, Jiang J, et al. Curcumin modulates Tlr4/Nf-Kb inflammatory signaling pathway following traumatic spinal cord injury in rats. J Spinal Cord Med (2015) 38(2):199–206. doi: 10.1179/2045772313y.0000000179
50. Zhou K, Shi L, Wang Y, Chen S, Zhang J. Recent advances of the Nlrp3 inflammasome in central nervous system disorders. J Immunol Res (2016) 2016:9238290. doi: 10.1155/2016/9238290
51. Sagulenko V, Thygesen SJ, Sester DP, Idris A, Cridland JA, Vajjhala PR, et al. Aim2 and Nlrp3 inflammasomes activate both apoptotic and pyroptotic death pathways Via asc. Cell Death differentiation (2013) 20(9):1149–60. doi: 10.1038/cdd.2013.37
52. Huang JH, Fu CH, Xu Y, Yin XM, Cao Y, Lin FY. Extracellular vesicles derived from epidural fat-mesenchymal stem cells attenuate Nlrp3 inflammasome activation and improve functional recovery after spinal cord injury. Neurochem Res (2020) 45(4):760–71. doi: 10.1007/s11064-019-02950-x
53. Hu X, Zhang Y, Wang L, Ding J, Li M, Li H, et al. Microglial activation in the motor cortex mediated Nlrp3-related neuroinflammation and neuronal damage following spinal cord injury. Front Cell Neurosci (2022) 16:956079. doi: 10.3389/fncel.2022.956079
54. Li Y, Lei Z, Ritzel RM, He J, Li H, Choi HMC, et al. Impairment of autophagy after spinal cord injury potentiates neuroinflammation and motor function deficit in mice. Theranostics (2022) 12(12):5364–88. doi: 10.7150/thno.72713
55. Jiang F, Xia M, Zhang Y, Chang J, Cao J, Zhang Z, et al. Cannabinoid receptor-2 attenuates neuroinflammation by promoting autophagy-mediated degradation of the Nlrp3 inflammasome post spinal cord injury. Front Immunol (2022) 13:993168. doi: 10.3389/fimmu.2022.993168
56. Xu C, Zhou Z, Zhao H, Lin S, Zhang P, Tian H, et al. Zinc promotes spinal cord injury recovery by blocking the activation of Nlrp3 inflammasome through Sirt3-mediated autophagy. Neurochem Res (2023) 48(2):435–46. doi: 10.1007/s11064-022-03762-2
57. Wang H, Wang H, Huang H, Qu Z, Ma D, Dang X, et al. Melatonin attenuates spinal cord injury in mice by activating the Nrf2/Are signaling pathway to inhibit the Nlrp3 inflammasome. Cells (2022) 11(18). doi: 10.3390/cells11182809
58. Jiang W, He F, Ding G, Wu J. Dopamine inhibits pyroptosis and attenuates secondary damage after spinal cord injury in female mice. Neurosci Lett (2023) 792:136935. doi: 10.1016/j.neulet.2022.136935
59. Sharma M, de Alba E. Structure, activation and regulation of Nlrp3 and Aim2 inflammasomes. Int J Mol Sci (2021) 22(2). doi: 10.3390/ijms22020872
60. Wang SN, Guo XY, Tang J, Ding SQ, Shen L, Wang R, et al. Expression and localization of absent in melanoma 2 in the injured spinal cord. Neural Regener Res (2019) 14(3):542–52. doi: 10.4103/1673-5374.245481
61. Adamczak SE, de Rivero Vaccari JP, Dale G, Brand FJ 3rd, Nonner D, Bullock MR, et al. Pyroptotic neuronal cell death mediated by the Aim2 inflammasome. J Cereb Blood Flow Metab (2014) 34(4):621–9. doi: 10.1038/jcbfm.2013.236
62. David S, Greenhalgh AD, Kroner A. Macrophage and microglial plasticity in the injured spinal cord. Neuroscience (2015) 307:311–8. doi: 10.1016/j.neuroscience.2015.08.064
63. Popovich PG, Wei P, Stokes BT. Cellular inflammatory response after spinal cord injury in sprague-dawley and Lewis rats. J Comp Neurol (1997) 377(3):443–64. doi: 10.1002/(sici)1096-9861(19970120)377:3<443::aid-cne10>3.0.co;2-s
64. Hines DJ, Hines RM, Mulligan SJ, Macvicar BA. Microglia processes block the spread of damage in the brain and require functional chloride channels. Glia (2009) 57(15):1610–8. doi: 10.1002/glia.20874
65. David S, Kroner A. Repertoire of microglial and macrophage responses after spinal cord injury. Nat Rev Neurosci (2011) 12(7):388–99. doi: 10.1038/nrn3053
66. Gensel JC, Zhang B. Macrophage activation and its role in repair and pathology after spinal cord injury. Brain Res (2015) 1619:1–11. doi: 10.1016/j.brainres.2014.12.045
67. Liu Z, Yao X, Jiang W, Li W, Zhu S, Liao C, et al. Advanced oxidation protein products induce microglia-mediated neuroinflammation Via mapks-Nf-Kb signaling pathway and pyroptosis after secondary spinal cord injury. J Neuroinflamm (2020) 17(1):90. doi: 10.1186/s12974-020-01751-2
68. Ma C, Li S, Hu Y, Ma Y, Wu Y, Wu C, et al. Aim2 controls microglial inflammation to prevent experimental autoimmune encephalomyelitis. J Exp Med (2021) 218(5). doi: 10.1084/jem.20201796
69. Zivkovic S, Ayazi M, Hammel G, Ren Y. For better or for worse: A look into neutrophils in traumatic spinal cord injury. Front Cell Neurosci (2021) 15:648076. doi: 10.3389/fncel.2021.648076
70. Amo-Aparicio J, Garcia-Garcia J, Puigdomenech M, Francos-Quijorna I, Skouras DB, Dinarello CA, et al. Inhibition of the Nlrp3 inflammasome by Olt1177 induces functional protection and myelin preservation after spinal cord injury. Exp Neurol (2022) 347:113889. doi: 10.1016/j.expneurol.2021.113889
71. Jiang W, Li M, He F, Zhou S, Zhu L. Targeting the Nlrp3 inflammasome to attenuate spinal cord injury in mice. J Neuroinflamm (2017) 14(1):207. doi: 10.1186/s12974-017-0980-9
72. Jiang W, He F, Ding G, Wu J. Topotecan reduces neuron death after spinal cord injury by suppressing caspase-1-Dependent pyroptosis. Mol Neurobiol (2022) 59(10):6033–48. doi: 10.1007/s12035-022-02960-x
73. de Rivero Vaccari JP, Bastien D, Yurcisin G, Pineau I, Dietrich WD, De Koninck Y, et al. P2x4 receptors influence inflammasome activation after spinal cord injury. J Neurosci (2012) 32(9):3058–66. doi: 10.1523/jneurosci.4930-11.2012
74. Jiang W, Li M, He F, Yao W, Bian Z, Wang X, et al. Protective effects of Asiatic acid against spinal cord injury-induced acute lung injury in rats. Inflammation (2016) 39(6):1853–61. doi: 10.1007/s10753-016-0414-3
75. Iqubal A, Ahmed M, Iqubal MK, Pottoo FH, Haque SE. Polyphenols as potential therapeutics for pain and inflammation in spinal cord injury. Curr Mol Pharmacol (2021) 14(5):714–30. doi: 10.2174/1874467213666201223111743
76. Li X, Li M, Tian L, Chen J, Liu R, Ning B. Reactive astrogliosis: Implications in spinal cord injury progression and therapy. Oxid Med Cell Longev (2020) 2020:9494352. doi: 10.1155/2020/9494352
77. Mi J, Yang Y, Yao H, Huan Z, Xu C, Ren Z, et al. Inhibition of heat shock protein family a member 8 attenuates spinal cord ischemia-reperfusion injury Via astrocyte nf-Kb/Nlrp3 inflammasome pathway : Hspa8 inhibition protects spinal ischemia-reperfusion injury. J Neuroinflamm (2021) 18(1):170. doi: 10.1186/s12974-021-02220-0
78. Shiraishi Y, Kimura A, Kimura H, Ohmori T, Takahashi M, Takeshita K. Deletion of inflammasome adaptor protein asc enhances functional recovery after spinal cord injury in mice. J Orthop Sci (2021) 26(3):487–93. doi: 10.1016/j.jos.2020.04.006
79. Noori L, Arabzadeh S, Mohamadi Y, Mojaverrostami S, Mokhtari T, Akbari M, et al. Intrathecal administration of the extracellular vesicles derived from human wharton's jelly stem cells inhibit inflammation and attenuate the activity of inflammasome complexes after spinal cord injury in rats. Neurosci Res (2021) 170:87–98. doi: 10.1016/j.neures.2020.07.011
80. Yanagisawa S, Katoh H, Imai T, Nomura S, Watanabe M. The relationship between inflammasomes and the endoplasmic reticulum stress response in the injured spinal cord. Neurosci Lett (2019) 705:54–9. doi: 10.1016/j.neulet.2019.04.033
81. Turner MD, Nedjai B, Hurst T, Pennington DJ. Cytokines and chemokines: At the crossroads of cell signalling and inflammatory disease. Biochim Biophys Acta (2014) 1843(11):2563–82. doi: 10.1016/j.bbamcr.2014.05.014
82. Mortazavi MM, Verma K, Harmon OA, Griessenauer CJ, Adeeb N, Theodore N, et al. The microanatomy of spinal cord injury: A review. Clin Anat (2015) 28(1):27–36. doi: 10.1002/ca.22432
83. Ellison JA, Velier JJ, Spera P, Jonak ZL, Wang X, Barone FC, et al. Osteopontin and its integrin receptor Alpha(V)Beta3 are upregulated during formation of the glial scar after focal stroke. Stroke (1998) 29(8):1698–706. doi: 10.1161/01.str.29.8.1698
84. Liu NK, Xu XM. Neuroprotection and its molecular mechanism following spinal cord injury. Neural Regener Res (2012) 7(26):2051–62. doi: 10.3969/j.issn.1673-5374.2012.26.007
85. Guerrero AR, Uchida K, Nakajima H, Watanabe S, Nakamura M, Johnson WE, et al. Blockade of interleukin-6 signaling inhibits the classic pathway and promotes an alternative pathway of macrophage activation after spinal cord injury in mice. J Neuroinflamm (2012) 9:40. doi: 10.1186/1742-2094-9-40
86. Yan P, Li Q, Kim GM, Xu J, Hsu CY, Xu XM. Cellular localization of tumor necrosis factor-alpha following acute spinal cord injury in adult rats. J Neurotrauma (2001) 18(5):563–8. doi: 10.1089/089771501300227369
87. Garcia E, Aguilar-Cevallos J, Silva-Garcia R, Ibarra A. Cytokine and growth factor activation in vivo and in vitro after spinal cord injury. Mediators Inflammation (2016) 2016:9476020. doi: 10.1155/2016/9476020
88. Paintlia MK, Paintlia AS, Singh AK, Singh I. Synergistic activity of interleukin-17 and tumor necrosis factor-A enhances oxidative stress-mediated oligodendrocyte apoptosis. J Neurochem (2011) 116(4):508–21. doi: 10.1111/j.1471-4159.2010.07136.x
89. Wang L, Wei FX, Cen JS, Ping SN, Li ZQ, Chen NN, et al. Early administration of tumor necrosis factor-alpha antagonist promotes survival of transplanted neural stem cells and axon myelination after spinal cord injury in rats. Brain Res (2014) 1575:87–100. doi: 10.1016/j.brainres.2014.05.038
90. Hu X, Chen H, Xu H, Wu Y, Wu C, Jia C, et al. Role of pyroptosis in traumatic brain and spinal cord injuries. Int J Biol Sci (2020) 16(12):2042–50. doi: 10.7150/ijbs.45467
91. Karki R, Man SM, Kanneganti TD. Inflammasomes and cancer. Cancer Immunol Res (2017) 5(2):94–9. doi: 10.1158/2326-6066.Cir-16-0269
92. Sevenich L. Brain-resident microglia and blood-borne macrophages orchestrate central nervous system inflammation in neurodegenerative disorders and brain cancer. Front Immunol (2018) 9:697. doi: 10.3389/fimmu.2018.00697
93. Greten FR, Grivennikov SI. Inflammation and cancer: Triggers, mechanisms, and consequences. Immunity (2019) 51(1):27–41. doi: 10.1016/j.immuni.2019.06.025
94. Deswaerte V, Nguyen P, West A, Browning AF, Yu L, Ruwanpura SM, et al. Inflammasome adaptor asc suppresses apoptosis of gastric cancer cells by an Il18-mediated inflammation-independent mechanism. Cancer Res (2018) 78(5):1293–307. doi: 10.1158/0008-5472.Can-17-1887
95. Perez-Yepez EA, Ayala-Sumuano JT, Lezama R, Meza I. A novel B-catenin signaling pathway activated by il-1β leads to the onset of epithelial-mesenchymal transition in breast cancer cells. Cancer Lett (2014) 354(1):164–71. doi: 10.1016/j.canlet.2014.08.015
96. Chow MT, Sceneay J, Paget C, Wong CS, Duret H, Tschopp J, et al. Nlrp3 suppresses nk cell-mediated responses to carcinogen-induced tumors and metastases. Cancer Res (2012) 72(22):5721–32. doi: 10.1158/0008-5472.Can-12-0509
97. Chen LC, Wang LJ, Tsang NM, Ojcius DM, Chen CC, Ouyang CN, et al. Tumour inflammasome-derived il-1β recruits neutrophils and improves local recurrence-free survival in ebv-induced nasopharyngeal carcinoma. EMBO Mol Med (2012) 4(12):1276–93. doi: 10.1002/emmm.201201569
98. Tarassishin L, Casper D, Lee SC. Aberrant expression of interleukin-1β and inflammasome activation in human malignant gliomas. PloS One (2014) 9(7):e103432. doi: 10.1371/journal.pone.0103432
99. Kesavardhana S, Kanneganti TD. Mechanisms governing inflammasome activation, assembly and pyroptosis induction. Int Immunol (2017) 29(5):201–10. doi: 10.1093/intimm/dxx018
100. Gao J, Qiu X, Xi G, Liu H, Zhang F, Lv T, et al. Downregulation of gsdmd attenuates tumor proliferation Via the intrinsic mitochondrial apoptotic pathway and inhibition of Egfr/Akt signaling and predicts a good prognosis in Non−Small cell lung cancer. Oncol Rep (2018) 40(4):1971–84. doi: 10.3892/or.2018.6634
101. Valastyan S, Weinberg RA. Tumor metastasis: Molecular insights and evolving paradigms. Cell (2011) 147(2):275–92. doi: 10.1016/j.cell.2011.09.024
102. Wang W, Wang X, Chun J, Vilaysane A, Clark S, French G, et al. Inflammasome-independent Nlrp3 augments tgf-B signaling in kidney epithelium. J Immunol (2013) 190(3):1239–49. doi: 10.4049/jimmunol.1201959
103. Wang H, Wang Y, Du Q, Lu P, Fan H, Lu J, et al. Inflammasome-independent Nlrp3 is required for epithelial-mesenchymal transition in colon cancer cells. Exp Cell Res (2016) 342(2):184–92. doi: 10.1016/j.yexcr.2016.03.009
104. McMahon G. Vegf receptor signaling in tumor angiogenesis. oncologist (2000) 5 Suppl 1:3–10. doi: 10.1634/theoncologist.5-suppl_1-3
105. Jung YJ, Isaacs JS, Lee S, Trepel J, Neckers L. Il-1beta-Mediated up-regulation of hif-1alpha Via an Nfkappab/Cox-2 pathway identifies hif-1 as a critical link between inflammation and oncogenesis. FASEB J (2003) 17(14):2115–7. doi: 10.1096/fj.03-0329fje
106. Gajewski TF, Schreiber H, Fu YX. Innate and adaptive immune cells in the tumor microenvironment. Nat Immunol (2013) 14(10):1014–22. doi: 10.1038/ni.2703
107. Carbotti G, Barisione G, Orengo AM, Brizzolara A, Airoldi I, Bagnoli M, et al. The il-18 antagonist il-18-Binding protein is produced in the human ovarian cancer microenvironment. Clin Cancer Res (2013) 19(17):4611–20. doi: 10.1158/1078-0432.Ccr-13-0568
108. Herremans KM, Szymkiewicz DD, Riner AN, Bohan RP, Tushoski GW, Davidson AM, et al. The interleukin-1 axis and the tumor immune microenvironment in pancreatic ductal adenocarcinoma. Neoplasia (2022) 28:100789. doi: 10.1016/j.neo.2022.100789
109. Marvel D, Gabrilovich DI. Myeloid-derived suppressor cells in the tumor microenvironment: Expect the unexpected. J Clin Invest (2015) 125(9):3356–64. doi: 10.1172/jci80005
110. Nakamura K, Kassem S, Cleynen A, Chrétien ML, Guillerey C, Putz EM, et al. Dysregulated il-18 is a key driver of immunosuppression and a possible therapeutic target in the multiple myeloma microenvironment. Cancer Cell (2018) 33(4):634–48.e5. doi: 10.1016/j.ccell.2018.02.007
111. Lin ZH, Wang SY, Chen LL, Zhuang JY, Ke QF, Xiao DR, et al. Methylene blue mitigates acute neuroinflammation after spinal cord injury through inhibiting Nlrp3 inflammasome activation in microglia. Front Cell Neurosci (2017) 11:391. doi: 10.3389/fncel.2017.00391
112. Lv R, Du L, Liu X, Zhou F, Zhang Z, Zhang L. Polydatin alleviates traumatic spinal cord injury by reducing microglial inflammation Via regulation of inos and Nlrp3 inflammasome pathway. Int Immunopharmacol (2019) 70:28–36. doi: 10.1016/j.intimp.2019.02.006
113. Zhu Y, Zhu H, Wang Z, Gao F, Wang J, Zhang W. Wogonoside alleviates inflammation induced by traumatic spinal cord injury by suppressing nf-Kb and Nlrp3 inflammasome activation. Exp Ther Med (2017) 14(4):3304–8. doi: 10.3892/etm.2017.4904
114. Gao S, Xu T, Guo H, Deng Q, Xun C, Liang W, et al. Ameliorative effects of echinacoside against spinal cord injury Via inhibiting Nlrp3 inflammasome signaling pathway. Life Sci (2019) 237:116978. doi: 10.1016/j.lfs.2019.116978
115. Su XQ, Wang XY, Gong FT, Feng M, Bai JJ, Zhang RR, et al. Oral treatment with glycyrrhizin inhibits Nlrp3 inflammasome activation and promotes microglial M2 polarization after traumatic spinal cord injury. Brain Res Bull (2020) 158:1–8. doi: 10.1016/j.brainresbull.2020.02.009
116. Zhao H, Wang X, Liu S, Zhang Q. Paeonol regulates Nlrp3 inflammasomes and pyroptosis to alleviate spinal cord injury of rat. BMC Neurosci (2022) 23(1):16. doi: 10.1186/s12868-022-00698-9
117. Guo R, Gao S, Feng Y, Mao C, Sheng W. Ulinastatin attenuates spinal cord injury by targeting Ampk/Nlrp3 signaling pathway. J Chem Neuroanat (2022) 125:102145. doi: 10.1016/j.jchemneu.2022.102145
118. Coll RC, Hill JR, Day CJ, Zamoshnikova A, Boucher D, Massey NL, et al. Mcc950 directly targets the Nlrp3 atp-hydrolysis motif for inflammasome inhibition. Nat Chem Biol (2019) 15(6):556–9. doi: 10.1038/s41589-019-0277-7
119. Coll RC, Robertson AA, Chae JJ, Higgins SC, Muñoz-Planillo R, Inserra MC, et al. A small-molecule inhibitor of the Nlrp3 inflammasome for the treatment of inflammatory diseases. Nat Med (2015) 21(3):248–55. doi: 10.1038/nm.3806
120. He Y, Varadarajan S, Muñoz-Planillo R, Burberry A, Nakamura Y, Núñez G. 3,4-Methylenedioxy-B-Nitrostyrene inhibits Nlrp3 inflammasome activation by blocking assembly of the inflammasome. J Biol Chem (2014) 289(2):1142–50. doi: 10.1074/jbc.M113.515080
121. Marchetti C, Swartzwelter B, Gamboni F, Neff CP, Richter K, Azam T, et al. Olt1177, a B-sulfonyl nitrile compound, safe in humans, inhibits the Nlrp3 inflammasome and reverses the metabolic cost of inflammation. Proc Natl Acad Sci United States America (2018) 115(7):E1530–e9. doi: 10.1073/pnas.1716095115
122. Lund MC, Clausen BH, Brambilla R, Lambertsen KL. The role of tumor necrosis factor following spinal cord injury: A systematic review. Cell Mol Neurobiol (2022). doi: 10.1007/s10571-022-01229-0
123. Yuan J, Botchway BOA, Zhang Y, Tan X, Wang X, Liu X. Curcumin can improve spinal cord injury by inhibiting tgf-B-Sox9 signaling pathway. Cell Mol Neurobiol (2019) 39(5):569–75. doi: 10.1007/s10571-019-00671-x
124. Cornelis S, Kersse K, Festjens N, Lamkanfi M, Vandenabeele P. Inflammatory caspases: Targets for novel therapies. Curr Pharm design (2007) 13(4):367–85. doi: 10.2174/138161207780163006
125. Linton SD. Caspase inhibitors: A pharmaceutical industry perspective. Curr Top Med Chem (2005) 5(16):1697–717. doi: 10.2174/156802605775009720
126. Chen J, Chen YQ, Shi YJ, Ding SQ, Shen L, Wang R, et al. Vx-765 reduces neuroinflammation after spinal cord injury in mice. Neural Regener Res (2021) 16(9):1836–47. doi: 10.4103/1673-5374.306096
127. Bigford GE, Bracchi-Ricard VC, Keane RW, Nash MS, Bethea JR. Neuroendocrine and cardiac metabolic dysfunction and Nlrp3 inflammasome activation in adipose tissue and pancreas following chronic spinal cord injury in the mouse. ASN Neuro (2013) 5(4):243–55. doi: 10.1042/an20130021
128. Jiang W, He F, Ding G, Wu J. Topoisomerase 1 inhibition modulates pyroptosis to improve recovery after spinal cord injury. FASEB J (2022) 36(6):e22294. doi: 10.1096/fj.202100713RR
129. Agnes JP, Santos VWD, das Neves RN, Gonçalves RM, Delgobo M, Girardi CS, et al. Antioxidants improve oxaliplatin-induced peripheral neuropathy in tumor-bearing mice model: Role of spinal cord oxidative stress and inflammation. J Pain (2021) 22(8):996–1013. doi: 10.1016/j.jpain.2021.03.142
130. Matsuoka Y, Yamashita A, Matsuda M, Kawai K, Sawa T, Amaya F. Nlrp2 inflammasome in dorsal root ganglion as a novel molecular platform that produces inflammatory pain hypersensitivity. Pain (2019) 160(9):2149–60. doi: 10.1097/j.pain.0000000000001611
131. La Russa D, Di Santo C, Lizasoain I, Moraga A, Bagetta G, Amantea D. Tumor necrosis factor (Tnf)-A-Stimulated gene 6 (Tsg-6): A promising immunomodulatory target in acute neurodegenerative diseases. Int J Mol Sci (2023) 24(2). doi: 10.3390/ijms24021162
Keywords: spinal cord injury, spinal cord tumor, inflammasome, caspase-1, immune response
Citation: Chen J, Shen Y, Shao X and Wu W (2023) An emerging role of inflammasomes in spinal cord injury and spinal cord tumor. Front. Immunol. 14:1119591. doi: 10.3389/fimmu.2023.1119591
Received: 09 December 2022; Accepted: 27 February 2023;
Published: 09 March 2023.
Edited by:
Anwen Shao, Zhejiang University, ChinaReviewed by:
Chen Linying, First Affiliated Hospital of Fujian Medical University, ChinaYuchun Zuo, Xiangya Hospital, Central South University, China
Copyright © 2023 Chen, Shen, Shao and Wu. This is an open-access article distributed under the terms of the Creative Commons Attribution License (CC BY). The use, distribution or reproduction in other forums is permitted, provided the original author(s) and the copyright owner(s) are credited and that the original publication in this journal is cited, in accordance with accepted academic practice. No use, distribution or reproduction is permitted which does not comply with these terms.
*Correspondence: Weiliang Wu, Y3l0b2tpbmVAemp1LmVkdS5jbg==