- Tuberculosis Prevention and Control Key Laboratory/Beijing Key Laboratory of New Techniques of Tuberculosis Diagnosis and Treatment, Senior Department of Tuberculosis, The 8th Medical Center of PLA General Hospital, Beijing, China
Bacille Calmette-Guérin (BCG) is the only approved vaccine for tuberculosis (TB) prevention worldwide. BCG has an excellent protective effect on miliary tuberculosis and tuberculous meningitis in children or infants. Interestingly, a growing number of studies have shown that BCG vaccination can induce nonspecific and specific immunity to fight against other respiratory disease pathogens, including SARS-CoV-2. The continuous emergence of variants of SARS-CoV-2 makes the protective efficiency of COVID-19-specific vaccines an unprecedented challenge. Therefore, it has been hypothesized that BCG-induced trained immunity might protect against COVID-19 infection. This study comprehensively described BCG-induced nonspecific and specific immunity and the mechanism of trained immunity. In addition, this study also reviewed the research on BCG revaccination to prevent TB, the impact of BCG on other non-tuberculous diseases, and the clinical trials of BCG to prevent COVID-19 infection. These data will provide new evidence to confirm the hypotheses mentioned above.
1 Introduction
Tuberculosis (TB) is still the most common cause of death for single hereditary pathogens. According to the Global Tuberculosis Report 2022 released by the World Health Organization (WHO), there were an estimated 10.6 million new TB cases and an estimated 1.6 million deaths in 2021 (1). China remains one of the 30 high TB burden countries, with an estimated 842,000 new TB cases in 2020, ranking second and accounting for about 9% of new cases globally (2).
Bacille Calmette-Guérin (BCG), developed by Calmette and Guerin by attenuating the virulence of Mycobacterium bovis in 1908, is a century-old vaccine for TB prevention (3). BCG has an excellent protective effect on miliary tuberculosis and tuberculous meningitis in children or infants, but its protective efficacy in adults varies between 0% - 80% (4, 5). In 1995, the WHO did not recommend BCG revaccination because of the lack of sufficient scientific evidence on the protective efficiency induced by BCG revaccination, and the Chinese Health Commission also stopped the work related to BCG revaccination in adults in 1997 (6). Interestingly, a growing number of studies have demonstrated that BCG could induce a nonspecific memory immunity termed “trained immunity” in innate immune cells by activating higher frequencies of innate immune cells to secrete interleukin 1β (IL-1β), tumor necrosis factor α (TNF-α), and IL-6 cytokines (5, 7–12). Therefore, the hypothesis has been proposed that BCG-induced trained immunity can protect against infection with microorganisms other than Mycobacterium tuberculosis (M. tb) (13, 14).
The coronavirus disease 2019 (COVID-19) pandemic, caused by severe acute respiratory syndrome coronavirus 2 (SARS-CoV-2), has been raging worldwide for nearly three years. As of 13 January 2023, there were 661,545,258 confirmed cases of COVID-19 and 6,700,519 deaths worldwide (https://covid19.who.int/). More than ten COVID-19-specific vaccines have been approved for emergency use worldwide, such as Sinovac, Sinopharm BBIBP-CorV, Ad5-nCoV vaccine, ZF2001, Janssen’s Ad26.COV2.S, Pfizer-BioNTech’s BNT162b2, Moderna mRNA-1273, Gam-COVID-Vac (Sputnik V), EpiVacCorona vaccine, AstraZeneca’s ChAdOx1 nCoV-19 (AZD1222), CureVac, and BBV152 vaccines (10, 12, 15–26). However, with the emergence of new SARS-CoV-2 variants of concern (VOC), the protective efficiency of these vaccines is facing unprecedented challenges (16). Previous studies have suggested that BCG might be a potential candidate to compensate for the shortcomings of COVID-19-specific vaccines (4, 10–12, 27–29).
In this study, we summarized specific and nonspecific immune responses induced by the BCG vaccine, the effect of BCG revaccination on the incidence of TB and other diseases, and the effects of BCG vaccination against COVID-19 infection.
2 Immune responses induced by the BCG
BCG is the only approved vaccine for preventing TB, and the target population is infants and newborns. BCG can reduce the risk of developing the disease by 50% and has a better preventive effect on severe tuberculosis (30). In addition, it can effectively prevent tuberculous meningitis and disseminated tuberculosis in infants. BCG is a live vaccine made from Mycobacterium bovine tuberculosis bacilli. After vaccination, it can activate T lymphocytes to produce specific immune responses (31). Since 1921, BCG has been used to prevent TB in humans, and since 1974, the WHO has included it in the Expanded Program on Immunization (EPI) (31). In recent years, research has found that the BCG vaccine can stimulate our body to produce atopic immunity, mediate innate immunity, and provide non-specific immunity. Innate immunity is the initial barrier against infection by pathogenic microorganisms, which can produce nonspecific immune responses to different pathogens, such as chemotaxis, phagocytosis, and secreted cytokines. It has long been believed that innate immunity differs from adaptive immunity and does not have immune memory. However, more and more studies have shown that innate immune cells and their stem cells also have memory characteristics (32), indicating that the innate immune responses have been activated after receiving the first infection. Then it can produce a more robust immune responses after being infected again to provide a better protective effect in fighting against pathogens (32). After being activated by homologous or heterologous pathogens, the innate immune system can produce a more robust immune response, and this phenomenon is called “trained immunity” (33). It is worth noting that not all heterologous/homologous exposures lead to innate immune training, on the contrary, some exposures can induce the immunoparalysis of innate immune cells (34).
2.1 Specific immune responses induced by the BCG
Although BCG remains the world’s only vaccine against TB, the duration of its preventive effect on TB is limited. Its efficacy in children with tuberculous meningitis and miliary tuberculosis is consistent, but its effectiveness in adults with pulmonary TB is variable (35). Possible reasons are as follows: 1) Genetic variation in the BCG strains, such as Denmark/Copenhagen strain 1331, Russian/Moscow, and Japanese/Tokyo 172; 2) The immune response to the BCG vaccine is weakened in the elderly due to immune aging; 3) Non-tuberculosis mycobacteria (NTM) infection masked the true effect of the BCG vaccine against M.tb; 4) Continuous stimulation of BCG can only induce more short-lived effector memory T cells and effector T cells (36–38).
It has been observed that BCG could induce specific or adaptive immunity to fight against infection. The T helper (Th) lymphocyte response and CD4/CD8 T cells are contributed to the adaptive immune response induced by the BCG (39, 40) (Figure 1). The Th cells can secrete interferon γ (IFN-γ), and the BCG immunization after birth induces the production of IFN-γ by γδ T cells. It is necessary for protective immunity from tuberculosis because this cytokine increases antimycobacterial activity in macrophages (41). In addition, CD4 and CD8 T cells would be considered primary mediators for long-term immunity for M. tb infection. CD8 T cells could identify mycobacterium-infected macrophages, and these macrophages are attacked by enzymes secreted by CD8 T cells, which directly protect against M. tb (40). After immunization with BCG, B cell activation in serum increases, and IgG against M. tb increases significantly (42, 43). These antibodies can also form bacterial-antibody complexes that can induce increased M. tb processing and antigen presentation by antigen-presenting cells (APCs) to CD4T cells, leading to increased CD8 T cell activation and cytotoxic responses to M. tb (43, 44).
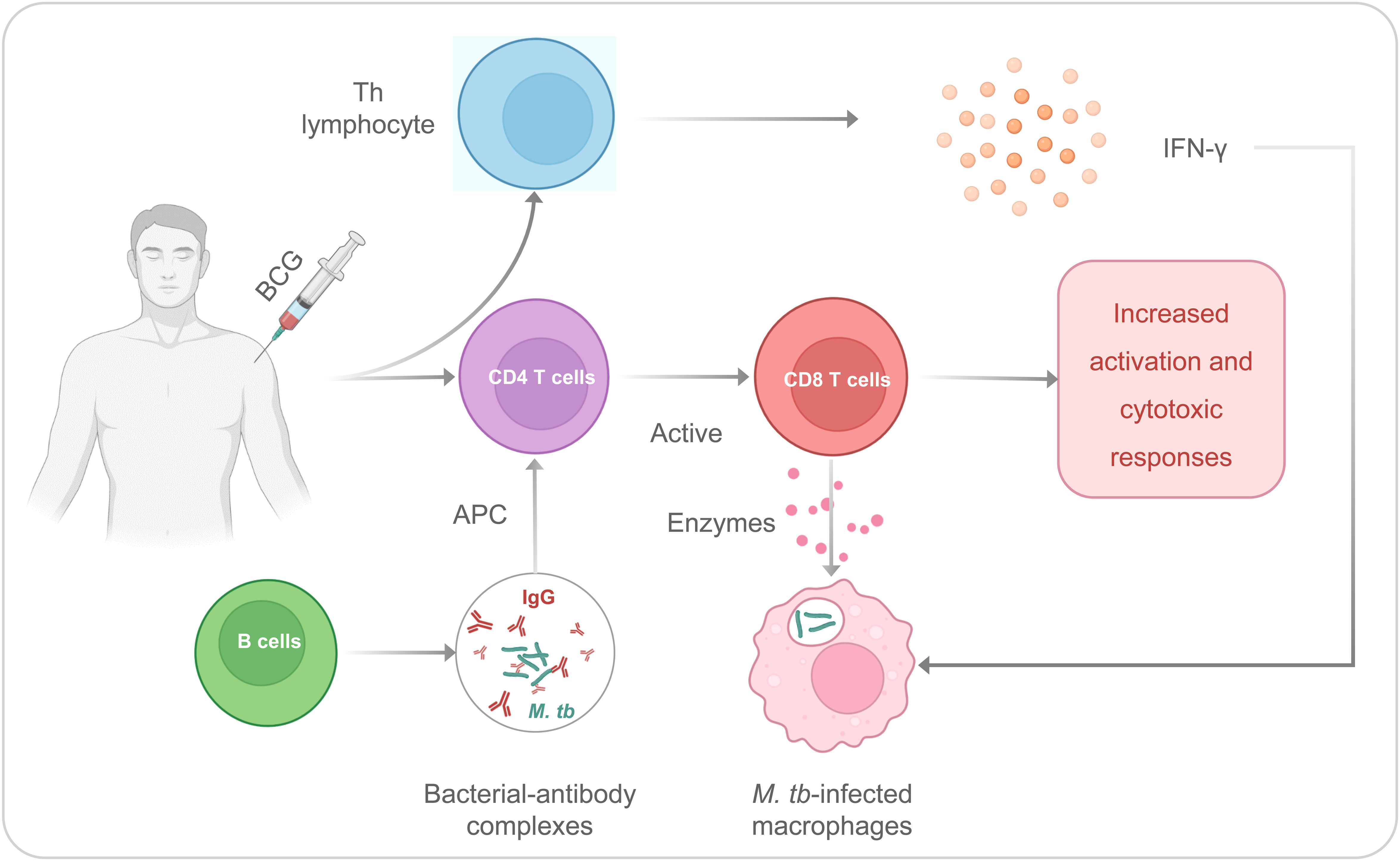
Figure 1 The Th and CD4/CD8 T cells are contributed to the adaptive immune responses induced by the BCG.
2.2 Nonspecific immune responses induced by the BCG
BCG is a multifunctional vaccine with nonspecific or heterologous immunomodulatory effects, which can promote nonspecific immunomodulatory effects (45) (Figure 2). Our previous studies have indicated that mature myeloid cells, such as macrophages or monocytes, play an essential role in nonspecific immunity induced by the BCG vaccine against unrelated pathogens (4, 12, 27). Although it has previously been shown that BCG-induced immunity is compartmentalized, trained innate immunity induced at a bone-marrow level in their respective hematopoietic stem cells, recent studies have indicated that BCG vaccination can also induce trained innate immunity in tissue-resident macrophages such as alveolar macrophages and peritoneal macrophages (46). BCG primary immunization induces more open chromatin structure in macrophages or monocytes, increases transcriptional active histone modifications such as H3K4m3 (histone H3 trimethylation at lysine 4) and H3K27ac (histone H3 acetylation at lysine 27), and decreases transcriptional inhibitory histone modifications such as H3K9m3 (histone H3 trimethylation at lysine 9), which in turn activates the production of IL-1β, TNF-α, and IL-6 (9, 12, 33, 47). Interestingly, when infected with an unrelated pathogen such as SARS-CoV-2, these trained nonspecific immune cells can induce a quicker and more substantial increase of H3K27ac and H3K4m3 and a decrease of H3K9m3, resulting in a higher level of IL-1β, TNFα, and IL-6 (33, 48). It also has been reported that BCG-mediated nonspecific immunity involves toll-like receptors (TLR) on the cell membrane surface and nucleotide-binding oligomerization domain (NOD) receptors located in the cytoplasm of some nonspecific immune cells, such as macrophages, monocytes, natural killer (NK) cells, and dendritic cells (DCs) (49, 50). The activation of TLRs and NOD receptors initiates the inflammatory cascade mainly through the secretion of TNF-α, IL-1β, and IL-6 (48, 51, 52). These cytokines aggregate inflammatory cells and provide signals to innate immune cells (53).
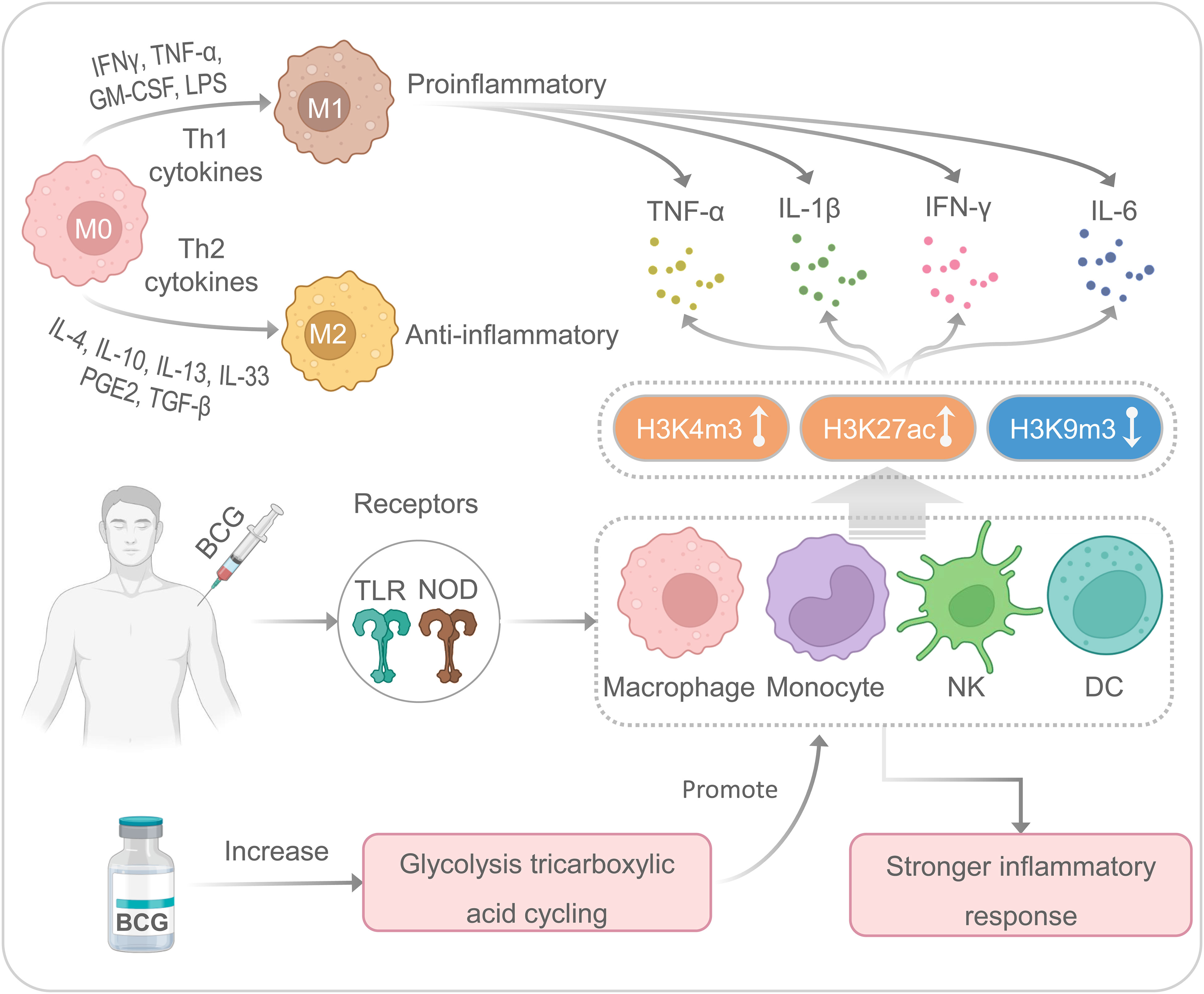
Figure 2 The nonspecific immune responses induced by the BCG. M, macrophages; NK, natural killer cells; DC, dendritic cells.
Moreover, enhanced glycolysis and glutamine-driven tricarboxylic acid cycling have been proven to be an essential metabolic pathway for trained immunity induced by BCG (54). There are two main types of macrophage polarization: classical polarization (M1) and alternative polarization (M2) (55). M1 macrophages are usually involved in eliminating pathogens and limiting tumor growth, while M2 macrophages are mainly involved in pathological processes such as anti-inflammatory response, tissue healing, fibrosis, and tumor survival (56). It has been suggested that lipopolysaccharide (LPS) and Th1 cytokines (IFN-γ, TNF-α, and GM-CSF) can stimulate M1-type polarization to produce pro-inflammatory cytokines (IL-1β, IL-6, IL-12, and IL-23), while Th2 cytokines, such as IL-4 and IL-13, can stimulate M2-type polarization to secrete anti-inflammatory cytokines (IL-10 and TGF-β) (57, 58). Interestingly, macrophages are highly plastic innate immune cells, and M1 and M2 macrophages can transform into each other based on different microenvironmental stimuli (59). In addition, M1 macrophages exert robust cytotoxic and antiproliferative effect activity by producing reactive oxygen species and nitric acid reactive substances (60, 61). However, recent studies have shown enhanced glycolysis and increased lactic acid production during M1 polarization (62). Several studies suggest that BCG can induce macrophages to secrete cytokines (such as IL-6, IL-12, and TNF-α) and a novel macrophage activated associated protein 1 (NMAAP1) (59, 63). Furthermore, BCG immunization can induce enhanced glucose consumption and lactate production via Akt/mTOR pathway (54).
3 Effect of BCG revaccination on the incidence of TB
Although the immune effects of BCG are not lifelong, BCG is still an important measure to control TB. The WHO recommends that BCG should be given as early as possible after the baby’s birth in countries with an endemic and high incidence of TB. It has been suggested that BCG revaccination could induce a more robust trained immunity defense against TB or other diseases (64) (Table 1). A previous study assessed the effectiveness and cost-effectiveness of BCG revaccination among adolescents, and the results showed that revaccination reduced the likelihood of TB transmission, with the risk of infection falling from 5.7% per year to 4.8% per year (65). In addition, in cost-benefit evaluations, if the cost of revaccination per person is set at $1-10, the effectiveness of the vaccine is between 10% and 80%, the term of protection is ten years, and the cost of treatment for the vaccinated population to restore healthy life (disability-adjusted life year, DALY) per year is $116 to $9237. The intervention was about twice as cost-effective as preventing transmission, with recovery costs of US $52 to US $4,540 per DALY at 80% effectiveness and 17% of cases averted with revaccination. According to the survey, BCG revaccination is cost-effective compared to international benchmarks (65).
Furthermore, BCG revaccination may benefit individuals with latent tuberculosis infection (LTBI). An Indian study showed that after BCG revaccination, the response of Ag85A and BCG-specific CD4+ and CD8+ T cells were significantly enhanced. Furthermore, the re-inoculated BCG was immunogenic in both positive and negative subjects with interferon release tests, suggesting that the immunogenicity of BCG was not affected even in patients with LTBI (66). It was also shown that Ag85A-, BCG-and LTG-specific CD4+ and CD8+ T cell responses were more enhanced in individuals with positive interferon release tests than in individuals with negative interferon release tests (66). Therefore, BCG revaccination may benefit people with LTBI who are more likely to develop the disease. Another randomized controlled trial conducted in South Africa employed the QuantiFERON-TB Gold In-tube test to assess the protective effects of BCG revaccination, and the result showed an effectiveness of 45.4% for continuous QFT conversion by BCG reinoculation (67).
BCG revaccination is linked to a trained innate immune mechanism. For example, a clinical trial conducted in South African adults with latent tuberculosis infection (LTBI) showed that BCG revaccination promoted BCG-specific CD4+CD8+γδ T cells and enhanced the responses of BCG-reactive CD3+CD56+ NKT-like cells and CD3-CD56dimIFN-γ+ or CD3-CD56hi IFN-γ+ NK cells (68). Similarly, a phase 1b randomized study performed in South African adolescents observed the same outcome that BCG revaccination elicited robust, polyfunctional BCG-specific CD4+ T cells (69).
Although these studies suggest that BCG revaccination can protect against TB and LTBI, other studies have yielded different results. A randomized controlled trial assessed the effect of BCG revaccination on all-cause mortality in 46,889 individuals in Malawi, and the results showed that, with a 30-year follow-up, BCG revaccination did not have any beneficial effects on all-cause mortality (70). Therefore, whether BCG revaccination can bring benefits needs to be further studied and verified.
4 Effect of BCG vaccine on other diseases
4.1 Effect of BCG on respiratory diseases
The trained immunity of BCG vaccination showed a more robust and longer-lasting effect (9). These functions effectively prevent TB and protect against other infectious diseases, especially some respiratory diseases (9) (Table 2). Studies have demonstrated that BCG plays a protective role in fighting against respiratory syncytial virus (RSV) infection. RSV is the most common cause of lower respiratory diseases. It is the leading cause of hospitalization, morbidity, and mortality from respiratory infections, usually causing bronchiolitis and pneumonia in infants under six months, as well as rhinitis and colds in adults (71). RSV infections typically occur in 3% – 7% of the elderly, with respiratory symptoms more severe than influenza in this population (72, 73). Unfortunately, there is no licensed effective vaccine for RSV worldwide. Animal experiments have shown that a recombinant BCG vaccine (rBCG-N-hRSV) has endowed mice with protective immunity and enhanced their ability to challenge infection (74). On day 28 after BCG immunization, it was found that the mice in the BCG immunized group lost less body weight, revealed less neutrophil infiltration, and showed a lower viral load in bronchoalveolar lavage fluid than those in the control group (74). In addition, activated banks of T cells that release IFN-γ and IL-1 were found in spleen T cells, suggesting that this recombinant BCG vaccine could induce a mixed CD8 and CD4 T cell response to inhibit the viral spread and prevent lung damage (74, 75). Therefore, this recombinant BCG vaccine (rBCG-N-hRSV) is a promising candidate for preventing RSV infection.
The influenza A virus epidemic that occurred in 2009 brought a huge disease burden on global health. A randomized, placebo-controlled pilot study conducted in 2015 showed that the combination of BCG and influenza vaccine effectively prevented influenza virus infection (76). In brief, the individuals vaccinated with the BCG vaccine had a significantly stronger response to H1 antibodies against the influenza A (H1N1) vaccine strain and had a more rapid seroconversion trend than those in the placebo group (76). Furthermore, the pro-inflammatory leukocyte response is enhanced after BCG vaccination, and a non-specific effect of influenza vaccines has also been observed, which can modulate cytokine responses to unrelated pathogens, suggesting that BCG vaccination before influenza vaccination may improve influenza vaccination efficiency (76, 77). Another animal experiment showed that the BCG vaccine could significantly enhance the efferocytosis effect of alveolar phagocytes (AP) (77). AP is crucial in maintaining lung health, and its funerary effect prevents pathogenic acute lung injury caused by lung infections. The efferocytosis effect of AP was significantly enhanced in mice immunized with the BCG vaccine, which increased the uptake and digestion of AP by apoptotic cells and initiated the rapid clearance of alveolar apoptotic cells (77).
Recently, a clinical trial was conducted on elderly people to evaluate the protective efficacy of BCG against respiratory infections (78). The primary endpoints of the study were time to first infection and incidence of new infections (respiratory infections caused by viruses, community-acquired pneumonia, hospital-acquired pneumonia, intra-abdominal infections, urinary tract infections, bloodstream infections, and acute bacterial infections of skin and skin structures). The results showed that the time to first infection in the BCG group was significantly delayed compared to the placebo group (16 weeks after BCG vaccination and 11 weeks in the placebo group). The incidence of new infections also showed a noticeable reduction, with 42.3% in the placebo group and 25.0% in the BCG group. The risk of new infections was reduced by 45% in the BCG group compared to the placebo group. Especially in preventing respiratory infections caused by viruses, participants in the BCG group had a 79% lower risk of viral pneumonia infection (78).
4.2 Effect of BCG on other infectious diseases
In addition to respiratory infectious diseases, BCG may also have a specific protective effect on other contagious diseases. For example, it was reported that BCG has a positive therapeutic effect on skin and genital warts caused by the human papillomavirus (HPV). A comparative study performed in Egypt showed that 65% of children with common warts were effectively treated with BCG compared to the placebo group (79). In a similar clinical trial conducted in India, three doses of BCG vaccinations were given at four weeks intervals, and after treatment, the complete clearance of viral warts was 48.5% (80). At the same time, some animal experiments have confirmed that the BCG vaccine can also resist various DNA and RNA virus infections (81). One study showed that children who received BCG immunization at birth had lower mortality rates than children who delayed BCG vaccination, which can be attributed to the BCG vaccine’s prevention of neonatal sepsis, respiratory infections, and fever (82, 83).
4.3 Effect of BCG on noninfectious diseases
Besides infectious diseases, BCG has also shown a protective effect against noninfectious diseases, such as non-muscle-invasive bladder cancer (NMIBC), type 1 diabetes mellitus (T1DM), and atherosclerosis (Table 2). Since the 1970s, BCG immunotherapy has been the “gold standard” for treating NMIBC (84). A randomized, double-blind, randomized controlled clinical trial showed that the protection rate of BCG against leprosy was approximately 40% over 30 years (85). In addition, an observational study evaluated adverse events in patients with NMIBC receiving adjuvant treatment with BCG, mitomycin C (MMC), and chemohyperthermia (86). The results demonstrated no significant clinical differences among BCG, MMC, and chemohyperthermia in patient quality of life and side effects. In contrast, another study found a rapid and marked reduction in bladder volume in one patient after BCG instillation (87). However, a study compared the efficacy of chemotherapeutic drugs and BCG’s efficacy in treating bladder cancer in mice and found that cyclophosphamide significantly reduced tumor volume in mice compared with the control group, but the BCG vaccine did not enhance the anti-tumor effect of cyclophosphamide, and BCG treatment alone promoted tumor growth (88). These results indicate that although BCG has been used to treat NMIBC for decades, its therapeutic effect is still controversial, and a more in-depth understanding of its mechanism is needed (89).
Moreover, infection with M. tb is accompanied by increased circulating Treg cells (90). BCG can be used to treat autoimmune type 1 diabetes mellitus (T1DM) due to its ability to induce immunosuppressive T cells (Tregs) to restore the immune balance, and this effect can last for 2-3 years (91). In a Phase I clinical trial, patients with T1DM who received at least two doses of the BCG vaccine could return to normal glucose levels after three years, and similar treatment effects were observed even in patients with advanced diabetes for more than 20 years (92). Interestingly, the therapeutic effect of BCG can last for more than five years (92). In addition, one study found that postnatal BCG vaccination was protective in females with T1DM but not in those who did not receive BCG (93). The underlying mechanisms of BCG vaccination on T1DM may be: 1) BCG vaccination up-regulates Myc, activates nearly 24 Myc target genes under four metabolic pathways, and finally improves glucose metabolism in T1DM patients (94); 2) BCG vaccination induces an increase in the methylation levels of H3K4me3 (Histone 3 Lysine 4) and H3K36me3 (Histone 3 Lysine 36me2), which activates cytokines (TNF, IL-6, and TLR4) related to BCG therapy in T1DM patients (95). These findings suggest that BCG treatment of T1DM improves glycemic control by altering metabolism and persistence. However, a systematic review and meta-analysis revealed that although there was a trend toward improvement in glycated hemoglobin levels after BCG treatment, there was still a lack of strong evidence to support BCG’s efficacy in treating T1DM (96).
Besides, BCG can regulate the progression of atherosclerosis. For example, one study evaluated the effect of subcutaneous BCG vaccination on the development of atherosclerosis in ApoE −/− mice. It was found that BCG vaccination reduced plaque number and macrophage content but increased lipid content in a mouse model of atherosclerosis (97). Similarly, in another animal study, the treatment of BCG killed by extended freeze-drying significantly reduced the size of atherosclerotic lesions, upregulated IL-10 production, and downregulated the expression of pro-inflammatory cytokines (IL-6, IL-13, and TNF-α) in mice (98). Furthermore, BCG can reduce plasma total cholesterol levels in mice in the experimental group, reduce foam cell formation in peritoneal macrophages, delay the progression of atherosclerotic lesions at the root of the aortic, and decrease the severity of lesions (99).
5 The effect of BCG on coronavirus infections
5.1 Amino acid sequence similarity between HSP65 protein of BCG strain and spike or nuclear protein of SARS-CoV-2
In the past 20 years, coronavirus (CoV) has triggered three major epidemic outbreaks worldwide, including severe acute respiratory syndrome (SARS), Middle East respiratory syndrome (MERS), and coronavirus disease 2019 (COVID-19) (100). On January 30, 2020, the WHO declared the severe acute respiratory syndrome coronavirus 2 (SARS-CoV-2) outbreak as a public health emergency of international concern. On February 11, 2020, the WHO officially named the pandemic as COVID-19 (101). SARS-CoV-2 is a new type of β coronavirus with the characteristics typical of the coronavirus family (102). Although SARS-CoV-2 infection does not cause as high a fatality rate as SARS-CoV or MERS-CoV, it is more transmissible (100). HSP 65, a major immunogenic component in BCG, is responsible for inducing efficient antigen-specific cell activation (103). A previous study comprehensively compared and analyzed the sequence similarity between the HSP65 protein of BCG strain and the spike and nuclear proteins of SARS-CoV-2, and the results show that the HSP65 protein sequence has a high similarity with the sequence of the spike and nuclear proteins of SARS-CoV-2 (104). This fundamental similarity suggests that BCG can induce cross-immune responses with SARS-CoV-2 antigens to reduce the susceptibility and severity of SARS-CoV-2 infection.
Additionally, studies have shown that BCG contains amino acid sequences similar to SARS-CoV-2 and has moderate to a high binding affinity for multiple common the human leukocyte antigen (HLA), suggesting that BCG vaccination can produce cross-reactive T cells against SARS-CoV-2 (105). HLA class I molecules typically bind peptides (mainly nine amino acids) in the length of 8-11 amino acids. Therefore, one study utilized two computer algorithms to analyze similar 9-amino acid sequences between SARS-CoV-2 and Mycobacterium bovis. The results showed that there were six distinct but closely related groups of peptides between SARS-CoV-2 and Mycobacterium bovis, and M. bovis contained many seven amino acid sequences that were identical to SARS-CoV-2 (106). Two computer algorithms analyzed the amino acid sequences of SARS-CoV-2 and M. bovis, and analysis using IEDB showed that six of the seven distinct but closely related peptides between SARS-CoV-2 and Mycobacterium bovis had moderate to a high binding affinity for multiple common HLA class I molecules. Analysis using NetMHCpan 4.1 showed that four groups of these similar peptides had weak to high binding affinities for common HLA class I molecules. HLA-binding affinity analysis in the computer showed that these very similar 9-mer peptides could be T-cell epitopes. BCG’s similar amino acid sequence to SARS-CoV-2 has the potential to induce cross-reactive T cells against SARS-CoV-2.
5.2 The potential protective role of BCG in fighting against COVID-19
5.2.1 COVID-19 Vaccine and its challenges
As of 29 November 2022, a total of 13,042,112,489 COVID-19 vaccine doses have been administered (https://covid19.who.int/). Currently, 199 COVID-19-specific vaccines are evaluated in animal models, and 175 COVID-19-specific vaccines are assessed in clinical trials (https://www.who.int/publications/m/item/draft-landscape-of-covid-19-candidate-vaccines). COVID-19 vaccines approved for emergency use can be grouped into four categories: inactivated vaccines, subunit vaccines, mRNA vaccines, and viral vector-based vaccines. A meta-analysis found that the overall effectiveness of inactivated vaccine, subunit vaccine, mRNA vaccine, and viral vector vaccine against SARS-CoV-2 was 73.11%, 89.33%, 94.29%, and 79.56%, respectively (107). Although these COVID-19 vaccines have shown excellent performance in protecting individuals from SARS-CoV-2 infection, there are concerns about their adverse effects in the population. A previous study has indicated that joint pain, muscle pain, and fever were common adverse events, about 50% of people experience these conditions after receiving the COVID-19 vaccine (107). Other common complications are headaches, chills, and fatigue (22, 108–112). In addition to these common adverse events, rare and severe adverse events have also been reported. For example, myocarditis or pericarditis and thromboembolism cases were reported in people who received viral vector and mRNA vaccines, and such adverse events might cause death (113–116).
In addition, acceptance is also one of the essential factors affecting the global distribution of COVID-19 vaccines. As early as 2021, Jeffrey V Lazarus and colleagues conducted a global survey of potential acceptance of a COVID-19 vaccine among 13,426 people in 19 countries (117). It was found that 71.5% of the participants were willing to receive COVID-19 vaccines, but up to 48.1% of the participants had concerns about the safety of COVID-19 vaccines (117). It is worrisome that COVID-19 vaccine acceptance is not only low among the general population but also among healthcare workers (HCWs). A systematic review and meta-analysis involving 23,739 African HCWs found that COVID-19 vaccine acceptance among African HCWs was only 56.59 (95%CI; 46.26-66.92; I2 = 99.6%, p = 0.000) (118).
The low COVID-19 vaccine acceptance reflects people’s hesitations and refusal to receive COVID-19 vaccines (119). As mentioned above, most of the new COVID-19 vaccines are still in preclinical studies or clinical trials, and the evidence of their effect and complications is unclear. According to a survey, one of the clearest reasons for refusing to be vaccinated is that a vaccine developed in a hurry is considered dangerous, and its efficacy and safety have not been confirmed (120). In addition, people with a medical background are generally expected to be more receptive to COVID-19 vaccines, but a European study suggests contrary results, showing no significant differences in COVID-19 vaccine acceptance between HCWs and non-HCWs (121). Unlike vaccine hesitance faced by COVID-19-specific vaccines, BCG has been used to prevent tuberculosis for more than 100 years, and its safety is widely recognized. Once BCG has been shown to be useful for COVID-19 prevention, it could be adopted by many more people.
5.2.2 The potential of BCG to prevent COVID-19
Based on BCG-induced trained immunity and the advantages of BCG mentioned above, a hypothesis has been proposed that BCG vaccination may reduce the morbidity and mortality of patients with COVID-19. Previous studies have shown that in countries without a universal BCG vaccination, such as Italy, the Netherlands, and the United States, populations were more likely to be infected by COVID-19 than in countries with universal and long-term BCG vaccination and countries that introduced BCG vaccine late to young children, such as Iran, have reported higher death rates from COVID-19 (122). Furthermore, it has been reported that BCG vaccination may reduce viremia after SARS-CoV-2 exposure, decrease the severity of COVID-19, and recover more quickly (123). Interestingly, BCG is a suitable vector for expressing the SARS-CoV-2 antigens. Furthermore, the trained immunity induced by the recombinant BCG vaccine and the SARS-CoV-2 specific immune response could cause a robust protective effect against COVID-19 (124). A study analyzed the association between BCG vaccination coverage and the incidence and mortality of COVID-19 (11). This study divided the countries into three groups according to their BCG coverage (coverage ≥90%, BCG has been recommended but coverage <90%, and BCG has never been introduced). The result showed that countries with higher BCG coverage showed a significantly lower incidence of COVID-19, and the mortality was much lower in countries with BCG coverage ≥90% (11). However, it is essential to note that these data are based on epidemiological and statistical analyses, and can be confounded by many factors, such as race, region, medical and economic level, weather changes, and different stages of COVID-19 (13). Therefore, a study that collected data in 171 countries and adjusted socioeconomic and weather changes showed a 30-fold reduction in COVID-19 mortality in countries with universal BCG vaccination compared to countries without universal BCG vaccination (125).
5.2.3 Landscape of clinical trials evaluating BCG vaccination against COVID-19
In recent years, a growing number of clinical trials have been conducted to evaluate the BCG vaccine’s effectiveness in preventing COVID-19 (Table 3). According to the data on ClinicalTrials.gov (https://clinicaltrials.gov/), 31 clinical trials have been conducted to investigate the immune effects of the BCG vaccine on COVID-19. We found that these clinical trials mainly focused on HCWs and older adults (≥60 years old) at high risk of COVID-19. Although some evidence has suggested that the BCG vaccine can beneficially influence the incidence and severity of COVID-19, the study of the long-term effects of BCG on COVID-19 are insufficient. A clinical trial, a 5-year cohort study, in older adults was conducted by Radbond University Medical Center to explore the long-term effects of BCG for infectious and inflammatory diseases, including COVID-19 (NCT05387655). Additionally, as mentioned before, the BCG vaccine has non-specific effects on the immune system, and WHO did not recommend BCG revaccination. Therefore, a clinical trial (NCT04347876) aimed to demonstrate how the previous BCG vaccination could protect people against infection with COVID-19, which mainly focused on altering the prognosis of COVID-19. Similarly, another clinical trial (NCT04369794) was developed to evaluate the impact of previous BCG vaccination and BCG revaccination on SARS-CoV-2 at different phases and disease phenotypes. These clinical trials focused on assessing the effect of BCG on reducing the incidence and severity of COVID-19. In contrast, an early Phase 1 clinical trial (NCT02403505) was conducted to evaluate COVID-19 Antigen Presentation Therapeutic Biological Product Mis for treating multiple gene mutation COVID-19 virus strains and activating human COVID-10 antigen presentation reaction. This clinical trial will recruit 20 participants with COVID-19, and the intervention was Ad26 COVID-19 Spike 1.0mL plus TICE®BCG Organism 50mg.
However, studies have also shown that BCG vaccination does not significantly protect against COVID-19. In a multicenter, randomized, double-blind, placebo-controlled phase III clinical trial in Poland in which investigators revaccinated HCWs with BCG, the results showed no significant difference in the incidence of COVID-19 between placebo and BCG groups (126). Results from another double-blind, randomized, controlled, phase 3 trial of HCWs in South Africa showed that vaccinating HCWs with BCG did not reduce the risk of COVID-19 and hospitalization for severe COVID-19 (127). In terms of the reasons contrary to other research findings, firstly, the success of BCG vaccination has not been verified. It can be seen that these two studies did not clarify whether TST-negative participants turned positive after receiving BCG, so this does not determine the protective efficacy of BCG. In addition, LTBI should receive significant concern. An animal study showed that Mycobacterium tuberculosis infection inhibited the trained immune processes in the bone marrow. Therefore, LTBI may have negatively influenced the non-specific role of BCG (128). However, these two studies did not address the confounding factor of LTBI.
6 Conclusions
The century-old BCG vaccine remains the only vaccine approved for TB prevention. BCG can induce trained immunity against the invasion of pathogens other than Mycobacterium tuberculosis, such as respiratory viruses. Based on this theory, it is speculated that BCG may potentially prevent COVID-19 morbidity and mortality. To verify this hypothesis, scientists worldwide have carried out a large number of epidemiological studies and statistical analyses, but the results of these studies are highly heterogeneous due to the interference of a variety of confounding factors. Therefore, more than 30 clinical trials have been conducted to evaluate the immune effect of BCG on COVID-19, and some clinical trials are exploring the long-term immune effects of BCG and the clinical benefits of COVID-19 antigen presentation therapeutic biological product mixed BCG on COVID-19. The protective effect of BCG on newborns and children is obvious, but the immune effect of BCG is not lifelong, and it is a question worth discussing how to produce immune effects on adults, especially adults without antibodies.
Author contributions
Conceptualization: JD and WG. Methodology: JD, YS, RW, ED, YC, WZ, and WG. Data Analysis: JD and YS. Software: YS and WG. Writing Original Manuscript: JD and YS. Review and revising manuscript: JD and WG. Funding Acquisition: JD and WG. All authors reviewed and approved the final manuscript.
Funding
This study was funded by the Special Research on Health and Epidemic Prevention (Grant No. 22FYFH02) and Beijing Municipal Science & Technology Commission (Grant No. 7212103 and L192065).
Conflict of interest
The authors declare that the research was conducted in the absence of any commercial or financial relationships that could be construed as a potential conflict of interest.
Publisher’s note
All claims expressed in this article are solely those of the authors and do not necessarily represent those of their affiliated organizations, or those of the publisher, the editors and the reviewers. Any product that may be evaluated in this article, or claim that may be made by its manufacturer, is not guaranteed or endorsed by the publisher.
References
1. WHO. Global tuberculosis report 2022. In: Geneva: World health organization. Geneva: World Health Organization (2022).
2. WHO. Global tuberculosis report 2021. In: Geneva pp: World health organization (2021). pp. 1–262.
3. Hussey G, Hawkridge T, Hanekom W. Childhood tuberculosis: old and new vaccines. Paediatr Respir Rev (2007) 8:148–54. doi: 10.1016/j.prrv.2007.04.009
4. Gong W, Mao Y, Li Y, Qi Y. BCG Vaccination: A potential tool against COVID-19 and COVID-19-like black swan incidents. Int Immunopharmacol (2022) 108:108870. doi: 10.1016/j.intimp.2022.108870
5. Gong W, An H, Wang J, Cheng P, Qi Y. The natural effect of BCG vaccination on COVID-19: The debate continues. Front Immunol (2022) 13:953228. doi: 10.3389/fimmu.2022.953228
7. Kumar NP, Padmapriyadarsini C, Rajamanickam A, Bhavani PK, Nancy A, Jeyadeepa B, et al. BCG Vaccination induces enhanced frequencies of dendritic cells and altered plasma levels of type I and type III interferons in elderly individuals. Int J Infect Dis IJID Off Publ Int Soc Infect Dis (2021) 110:98–104. doi: 10.1016/j.ijid.2021.07.041
8. Netea MG, Joosten LA, Latz E, Mills KH, Natoli G, Stunnenberg HG, et al. Trained immunity: A program of innate immune memory in health and disease. Science (2016) 352:aaf1098. doi: 10.1126/science.aaf1098
9. Kleinnijenhuis J, Quintin J, Preijers F, Joosten LA, Ifrim DC, Saeed S, et al. Bacille calmette-guerin induces NOD2-dependent nonspecific protection from reinfection via epigenetic reprogramming of monocytes. Proc Natl Acad Sci United States America (2012) 109:17537–42. doi: 10.1073/pnas.1202870109
10. Aspatwar A, Gong W, Wang S, Wu X, Parkkila S. Tuberculosis vaccine BCG: the magical effect of the old vaccine in the fight against the COVID-19 pandemic. Int Rev Immunol (2022) 41:283–96. doi: 10.1080/08830185.2021.1922685
11. Gong W, Wu X. Is the tuberculosis vaccine BCG an alternative weapon for developing countries to defeat COVID-19? Indian J tuberculosis (2021) 68:401–4. doi: 10.1016/j.ijtb.2020.10.012
12. Gong W, Aspatwar A, Wang S, Parkkila S, Wu X. COVID-19 pandemic: SARS-CoV-2 specific vaccines and challenges, protection via BCG trained immunity, and clinical trials. Expert Rev Vaccines (2021) 20:857–80. doi: 10.1080/14760584.2021.1938550
13. Wang J, Zhang Q, Wang H, Gong W. The potential roles of BCG vaccine in the prevention or treatment of COVID-19. Front Biosci (Landmark Ed) (2022) 27:157. doi: 10.31083/j.fbl2705157
14. Ujiie A, Watanabe M, Ushiba D. Non-specific resistance of the BCG vaccinated mice to the infection with salmonella enteritidis (I). Nihon Saikingaku Zasshi (1966) 21:675–82. doi: 10.3412/jsb.21.675
15. Doroftei B, Ciobica A, Ilie OD, Maftei R, Ilea C. Mini-review discussing the reliability and efficiency of COVID-19 vaccines. Diagnostics (Basel) (2021) 11:579. doi: 10.3390/diagnostics11040579
16. Jia Z, Gong W. Will mutations in the spike protein of SARS-CoV-2 lead to the failure of COVID-19 vaccines? J Korean Med Sci (2021) 36:e124. doi: 10.3346/jkms.2021.36.e124
17. Qin S, Cui M, Sun S, Zhou J, Du Z, Cui Y, et al. Genome characterization and potential risk assessment of the novel SARS-CoV-2 variant omicron (B.1.1.529). Zoonoses (2021) 1:18. doi: 10.15212/ZOONOSES-2021-0024
18. Garcia-Beltran WF, St Denis KJ, Hoelzemer A, Lam EC, Nitido AD, Sheehan ML, et al. mRNA-based COVID-19 vaccine boosters induce neutralizing immunity against SARS-CoV-2 omicron variant. Cell (2022) 185:457–66.e4. doi: 10.1016/j.cell.2021.12.033
19. Wang H, Zhang Y, Huang B, Deng W, Quan Y, Wang W, et al. Development of an inactivated vaccine candidate, BBIBP-CorV, with potent protection against SARS-CoV-2. Cell (2020) 182:713–21 e9. doi: 10.1016/j.cell.2020.06.008
20. Huang B, Dai L, Wang H, Hu Z, Yang X, Tan W, et al. Serum sample neutralisation of BBIBP-CorV and ZF2001 vaccines to SARS-CoV-2 501Y.V2. Lancet Microbe (2021) 2:e285. doi: 10.1016/S2666-5247(21)00082-3
21. Dolgin E. CureVac COVID vaccine let-down spotlights mRNA design challenges. Nature (2021) 594:483. doi: 10.1038/d41586-021-01661-0
22. Polack FP, Thomas SJ, Kitchin N, Absalon J, Gurtman A, Lockhart S, et al. Safety and efficacy of the BNT162b2 mRNA covid-19 vaccine. New Engl J Med (2020) 383:2603–15. doi: 10.1056/NEJMoa2034577
23. Wu S, Huang J, Zhang Z, Wu J, Zhang J, Hu H, et al. Safety, tolerability, and immunogenicity of an aerosolised adenovirus type-5 vector-based COVID-19 vaccine (Ad5-nCoV) in adults: preliminary report of an open-label and randomised phase 1 clinical trial. Lancet Infect Dis (2021) 21:1654–64. doi: 10.1016/S1473-3099(21)00396-0
24. Stephenson KE, Le Gars M, Sadoff J, de Groot AM, Heerwegh D, Truyers C, et al. Immunogenicity of the Ad26.COV2.S vaccine for COVID-19. JAMA (2021) 325:1535–44. doi: 10.1001/jama.2021.3645
25. Emary KRW, Golubchik T, Aley PK, Ariani CV, Angus B, Bibi S, et al. Efficacy of ChAdOx1 nCoV-19 (AZD1222) vaccine against SARS-CoV-2 variant of concern 202012/01 (B.1.1.7): an exploratory analysis of a randomised controlled trial. Lancet (2021) 397:1351–62. doi: 10.1016/S0140-6736(21)00628-0
26. Ella R, Reddy S, Blackwelder W, Potdar V, Yadav P, Sarangi V, et al. Efficacy, safety, and lot-to-lot immunogenicity of an inactivated SARS-CoV-2 vaccine (BBV152): interim results of a randomised, double-blind, controlled, phase 3 trial. Lancet (2021) 398:2173–84. doi: 10.1016/S0140-6736(21)02000-6
27. Gong W, Parkkila S, Wu X, Aspatwar A. SARS-CoV-2 variants and COVID-19 vaccines: Current challenges and future strategies. Int Rev Immunol (2022) 41:1–22. doi: 10.1080/08830185.2022.2079642
28. Czajka H, Zapolnik P, Krzych Ł, Kmiecik W, Stopyra L, Nowakowska A, et al. A multi-center, randomised, double-blind, placebo-controlled phase III clinical trial evaluating the impact of BCG re-vaccination on the incidence and severity of SARS-CoV-2 infections among symptomatic healthcare professionals during the COVID-19 pandemic in Poland–first results. Vaccines (2022) 10:314. doi: 10.3390/vaccines10020314
29. Singh S, Khera D, Chugh A, Khasbage S, Khera PS, Chugh VK. BCG Vaccination impact on mortality and recovery rates in COVID-19: A meta-analysis. Monaldi Arch Chest Dis (2021) 91. doi: 10.4081/monaldi.2021.1875
30. XM Z, CH X, XW P, F Z. Progress in researches on novel tuberculosis vaccine for different populations:a review. Chin J Publ Heal (2021) 11:1698–703. doi: 10.11847/zgggws1133162
31. Ren L, Di LY. New understanding and prospects for tuberculosis vaccines. Chin Med J Metallurgical Industry (2016) 33:143.
32. Mulder WJM, Ochando J, Joosten LAB, Fayad ZA, Netea MG. Therapeutic targeting of trained immunity. Nat Rev Drug Discov (2019) 18:553–66. doi: 10.1038/s41573-019-0025-4
33. Netea MG, Domínguez-Andrés J, Barreiro LB, Chavakis T, Divangahi M, Fuchs E, et al. Defining trained immunity and its role in health and disease. Nat Rev Immunol (2020) 20:375–88. doi: 10.1038/s41577-020-0285-6
34. Roquilly A, Jacqueline C, Davieau M, Mollé A, Sadek A, Fourgeux C, et al. Alveolar macrophages are epigenetically altered after inflammation, leading to long-term lung immunoparalysis. Nat Immunol (2020) 21:636–48. doi: 10.1038/s41590-020-0673-x
35. Trunz BB, Fine P, Dye C. Effect of BCG vaccination on childhood tuberculous meningitis and miliary tuberculosis worldwide: a meta-analysis and assessment of cost-effectiveness. Lancet (2006) 367:1173–80. doi: 10.1016/S0140-6736(06)68507-3
36. Verma D, Chan ED, Ordway DJ. Non-tuberculous mycobacteria interference with BCG-current controversies and future directions. Vaccines (Basel) (2020) 8:688. doi: 10.3390/vaccines8040688
37. Kaveh DA, Garcia-Pelayo MC, Hogarth PJ. Persistent BCG bacilli perpetuate CD4 T effector memory and optimal protection against tuberculosis. Vaccine (2014) 32:6911–8. doi: 10.1016/j.vaccine.2014.10.041
38. Moliva JI, Turner J, Torrelles JB. Prospects in mycobacterium bovis bacille calmette et guérin (BCG) vaccine diversity and delivery: why does BCG fail to protect against tuberculosis? Vaccine (2015) 33:5035–41. doi: 10.1016/j.vaccine.2015.08.033
39. Gela A, Murphy M, Rodo M, Hadley K, Hanekom WA, Boom WH, et al. Effects of BCG vaccination on donor unrestricted T cells in two prospective cohort studies. EBioMedicine (2022) 76:103839. doi: 10.1016/j.ebiom.2022.103839
40. Sutiwisesak R, Hicks ND, Boyce S, Murphy KC, Papavinasasundaram K, Carpenter SM, et al. A natural polymorphism of mycobacterium tuberculosis in the esxH gene disrupts immunodomination by the TB10.4-specific CD8 T cell response. PloS Pathog (2020) 16:e1009000. doi: 10.1371/journal.ppat.1009000
41. Moreira-Teixeira L, Stimpson PJ, Stavropoulos E, Hadebe S, Chakravarty P, Ioannou M, et al. Type I IFN exacerbates disease in tuberculosis-susceptible mice by inducing neutrophil-mediated lung inflammation and NETosis. Nat Commun (2020) 11:5566. doi: 10.1038/s41467-020-19412-6
42. Chen T, Blanc C, Eder AZ, Prados-Rosales R, Souza AC, Kim RS, et al. Association of human antibodies to arabinomannan with enhanced mycobacterial opsonophagocytosis and intracellular growth reduction. J Infect Dis (2016) 214:300–10. doi: 10.1093/infdis/jiw141
43. Zhou KL, Li X, Zhang XL, Pan Q. Mycobacterial mannose-capped lipoarabinomannan: a modulator bridging innate and adaptive immunity. Emerg Microbes Infect (2019) 8:1168–77. doi: 10.1080/22221751.2019.1649097
44. Beveridge NE, Price DA, Casazza JP, Pathan AA, Sander CR, Asher TE, et al. Immunisation with BCG and recombinant MVA85A induces long-lasting, polyfunctional mycobacterium tuberculosis-specific CD4+ memory T lymphocyte populations. Eur J Immunol (2007) 37:3089–100. doi: 10.1002/eji.200737504
45. Soto JA, Gálvez NMS, Andrade CA, Ramírez MA, Riedel CA, Kalergis AM, et al. BCG Vaccination induces cross-protective immunity against pathogenic microorganisms. Trends Immunol (2022) 43:322–35. doi: 10.1016/j.it.2021.12.006
46. Jeyanathan M, Vaseghi-Shanjani M, Afkhami S, Grondin JA, Kang A, D'Agostino MR, et al. Parenteral BCG vaccine induces lung-resident memory macrophages and trained immunity via the gut-lung axis. Nat Immunol (2022) 23:1687–702. doi: 10.1038/s41590-022-01354-4
47. Arts RJW, Carvalho A, La Rocca C, Palma C, Rodrigues F, Silvestre R, et al. Immunometabolic pathways in BCG-induced trained immunity. Cell Rep (2016) 17:2562–71. doi: 10.1016/j.celrep.2016.11.011
48. Bickett TE, McLean J, Creissen E, Izzo L, Hagan C, Izzo AJ, et al. Characterizing the BCG induced macrophage and neutrophil mechanisms for defense against mycobacterium tuberculosis. Front Immunol (2020) 11:1202. doi: 10.3389/fimmu.2020.01202
49. Grassin-Delyle S, Abrial C, Salvator H, Brollo M, Naline E, Devillier P. The role of toll-like receptors in the production of cytokines by human lung macrophages. J Innate Immun (2020) 12:63–73. doi: 10.1159/000494463
50. Wannigama DL, Jacquet A. NOD2-dependent BCG-induced trained immunity: A way to regulate innate responses to SARS-CoV2? Int J Infect Dis IJID Off Publ Int Soc Infect Dis (2020) 101:52–5. doi: 10.1016/j.ijid.2020.09.1429
51. Ferluga J, Yasmin H, Al-Ahdal MN, Bhakta S, Kishore U. Natural and trained innate immunity against mycobacterium tuberculosis. Immunobiology (2020) 225:151951. doi: 10.1016/j.imbio.2020.151951
52. Joosten SA, van Meijgaarden KE, Arend SM, Prins C, Oftung F, Korsvold GE, et al. Mycobacterial growth inhibition is associated with trained innate immunity. J Clin Invest (2018) 128:1837–51. doi: 10.1172/JCI97508
53. Iqbal NT, Hussain R. Non-specific immunity of BCG vaccine: A perspective of BCG immunotherapy. Trials Vaccinol (2014) 3:143–9. doi: 10.1016/j.trivac.2014.08.002
54. Liu Y, Liang S, Ding R, Hou Y, Deng F, Ma X, et al. BCG-Induced trained immunity in macrophage: reprograming of glucose metabolism. Int Rev Immunol (2020) 39:83–96. doi: 10.1080/08830185.2020.1712379
55. Funes SC, Rios M, Escobar-Vera J, Kalergis AM. Implications of macrophage polarization in autoimmunity. Immunology (2018) 154:186–95. doi: 10.1111/imm.12910
56. Boutilier AJ, Elsawa SF. Macrophage polarization states in the tumor microenvironment. Int J Mol Sci (2021) 22:6995. doi: 10.3390/ijms22136995
57. Misson P, van den Brûle S, Barbarin V, Lison D, Huaux F. Markers of macrophage differentiation in experimental silicosis. J Leukoc Biol (2004) 76:926–32. doi: 10.1189/jlb.0104019
58. Shapouri-Moghaddam A, Mohammadian S, Vazini H, Taghadosi M, Esmaeili SA, Mardani F, et al. Macrophage plasticity, polarization, and function in health and disease. J Cell Physiol (2018) 233:6425–40. doi: 10.1002/jcp.26429
59. Dos Santos CC, Walburg KV, van Veen S, Wilson LG, Trufen CEM, Nascimento IP, et al. Recombinant BCG-LTAK63 vaccine candidate for tuberculosis induces an inflammatory profile in human macrophages. Vaccines (Basel) (2022) 10:831. doi: 10.3390/vaccines10060831
60. Mills CD, Kincaid K, Alt JM, Heilman MJ, Hill AM. M-1/M-2 macrophages and the Th1/Th2 paradigm. J Immunol (Baltimore Md 1950) (2000) 164:6166–73. doi: 10.4049/jimmunol.164.12.6166
61. Zhang X, Mosser DM. Macrophage activation by endogenous danger signals. J Pathol (2008) 214:161–78. doi: 10.1002/path.2284
62. Wang T, Liu HY, Lian G, Zhang SY, Wang X, Jiang CT. HIF1 alpha-induced glycolysis metabolism is essential to the activation of inflammatory macrophages. Mediators Inflammation (2017) 2017:10. doi: 10.1155/2017/9029327
63. Liu Q, Tian Y, Zhao X, Jing H, Xie Q, Li P, et al. NMAAP1 expressed in BCG-activated macrophage promotes M1 macrophage polarization. Molecules Cells (2015) 38:886–94. doi: 10.14348/molcells.2015.0125
64. Debisarun PA, Kilic G, de Bree LCJ, Pennings LJ, van Ingen J, Benn CS, et al. The impact of BCG dose and revaccination on trained immunity. Clin Immunol (2023) 246:109208. doi: 10.1016/j.clim.2022.109208
65. Dye C. Making wider use of the world's most widely used vaccine: Bacille calmette–guérin revaccination reconsidered. J R Soc Interface (2013) 10:20130365. doi: 10.1098/rsif.2013.0365
66. Rakshit S, Ahmed A, Adiga V, Sundararaj BK, Sahoo PN, Kenneth J, et al. BCG Revaccination boosts adaptive polyfunctional Th1/Th17 and innate effectors in IGRA+ and IGRA- Indian adults. JCI Insight (2019) 4:e130540. doi: 10.1172/jci.insight.130540
67. Nemes E, Geldenhuys H, Rozot V, Rutkowski KT, Ratangee F, Bilek N, et al. Prevention of m. tuberculosis infection with H4:IC31 vaccine or BCG revaccination. New Engl J Med (2018) 379:138–49. doi: 10.1056/NEJMoa1714021
68. Suliman S, Geldenhuys H, Johnson JL, Hughes JE, Smit E, Murphy M, et al. Bacillus calmette-guérin (BCG) revaccination of adults with latent mycobacterium tuberculosis infection induces long-lived BCG-reactive NK cell responses. J Immunol (Baltimore Md 1950) (2016) 197:1100–10. doi: 10.4049/jimmunol.1501996
69. Bekker LG, Dintwe O, Fiore-Gartland A, Middelkoop K, Hutter J, Williams A, et al. A phase 1b randomized study of the safety and immunological responses to vaccination with H4:IC31, H56:IC31, and BCG revaccination in mycobacterium tuberculosis-uninfected adolescents in cape town, south Africa. EClinicalMedicine (2020) 21:100313. doi: 10.1016/j.eclinm.2020.100313
70. Glynn JR, Dube A, Fielding K, Crampin AC, Karonga Prevention Trial G, Kanjala C, et al. The effect of BCG revaccination on all-cause mortality beyond infancy: 30-year follow-up of a population-based, double-blind, randomised placebo-controlled trial in Malawi. Lancet Infect Dis (2021) 21:1590–7. doi: 10.1016/S1473-3099(20)30994-4
71. Sadoff J, De Paepe E, DeVincenzo J, Gymnopoulou E, Menten J, Murray B, et al. Prevention of respiratory syncytial virus infection in healthy adults by a single immunization of Ad26.RSV.preF in a human challenge study. J Infect Dis (2022) 226:396–406. doi: 10.1093/infdis/jiab003
72. Pastula ST, Hackett J, Coalson J, Jiang X, Villafana T, Ambrose C, et al. Hospitalizations for respiratory syncytial virus among adults in the united states, 1997-2012. Open Forum Infect Dis (2017) 4:ofw270. doi: 10.1093/ofid/ofw270
73. Ackerson B, Tseng HF, Sy LS, Solano Z, Slezak J, Luo Y, et al. Severe morbidity and mortality associated with respiratory syncytial virus versus influenza infection in hospitalized older adults. Clin Infect Dis an Off Publ Infect Dis Soc America (2019) 69:197–203. doi: 10.1093/cid/ciy991
74. Cespedes PF, Rey-Jurado E, Espinoza JA, Rivera CA, Canedo-Marroquin G, Bueno SM, et al. A single, low dose of a cGMP recombinant BCG vaccine elicits protective T cell immunity against the human respiratory syncytial virus infection and prevents lung pathology in mice. Vaccine (2017) 35:757–66. doi: 10.1016/j.vaccine.2016.12.048
75. Rey-Jurado E, Soto J, Galvez N, Kalergis AM. A safe and efficient BCG vectored vaccine to prevent the disease caused by the human respiratory syncytial virus. Hum Vaccin Immunother (2017) 13:2092–7. doi: 10.1080/21645515.2017.1334026
76. Leentjens J, Kox M, Stokman R, Gerretsen J, Diavatopoulos DA, van Crevel R, et al. BCG Vaccination enhances the immunogenicity of subsequent influenza vaccination in healthy volunteers: A randomized, placebo-controlled pilot study. J Infect Dis (2015) 212:1930–8. doi: 10.1093/infdis/jiv332
77. Mukherjee S, Subramaniam R, Chen H, Smith A, Keshava S, Shams H. Boosting efferocytosis in alveolar space using BCG vaccine to protect host against influenza pneumonia. PloS One (2017) 12:e0180143. doi: 10.1371/journal.pone.0180143
78. Giamarellos-Bourboulis EJ, Tsilika M, Moorlag S, Antonakos N, Kotsaki A, Domínguez-Andrés J, et al. Activate: Randomized clinical trial of BCG vaccination against infection in the elderly. Cell (2020) 183:315–23.e9. doi: 10.1016/j.cell.2020.08.051
79. Salem A, Nofal A, Hosny D. Treatment of common and plane warts in children with topical viable bacillus calmette-guerin. Pediatr Dermatol (2013) 30:60–3. doi: 10.1111/j.1525-1470.2012.01848.x
80. Podder I, Bhattacharya S, Mishra V, Sarkar TK, Chandra S, Sil A, et al. Immunotherapy in viral warts with intradermal bacillus calmette-guerin vaccine versus intradermal tuberculin purified protein derivative: A double-blind, randomized controlled trial comparing effectiveness and safety in a tertiary care center in Eastern India. Indian J Dermatol Venereol Leprol (2017) 83:411. doi: 10.4103/0378-6323.193623
81. Moorlag SJCFM, Arts RJW, van Crevel R, Netea MG. Non-specific effects of BCG vaccine on viral infections. Clin Microbiol Infect (2019) 25:1473–8. doi: 10.1016/j.cmi.2019.04.020
82. Aaby P, Roth A, Ravn H, Napirna BM, Rodrigues A, Lisse IM, et al. Randomized trial of BCG vaccination at birth to low-Birth-Weight children: Beneficial nonspecific effects in the neonatal period? J Infect Dis (2011) 204:245–52. doi: 10.1093/infdis/jir240
83. Biering-Sørensen S, Aaby P, Lund N, Monteiro I, Jensen KJ, Eriksen HB, et al. Early BCG-Denmark and neonatal mortality among infants weighing <2500 g: A randomized controlled trial. Clin Infect Dis (2017) 65:1183–90. doi: 10.1093/cid/cix525
84. Bajic P, Wolfe AJ, Gupta GN. Old instillations and new implications for bladder cancer: the urinary microbiome and intravesical BCG. BJU Int (2019) 124:7–8. doi: 10.1111/bju.14683
85. Glynn JR, Fielding K, Mzembe T, Sichali L, Banda L, McLean E, et al. BCG Re-vaccination in Malawi: 30-year follow-up of a large, randomised, double-blind, placebo-controlled trial. Lancet Global Health (2021) 9:e1451–e9. doi: 10.1016/S2214-109X(21)00309-0
86. González-Padilla DA, González-Díaz A, Guerrero-Ramos F, Rodríguez-Serrano A, García-Jarabo E, Corona-laPuerta M, et al. Quality of life and adverse events in patients with nonmuscle invasive bladder cancer receiving adjuvant treatment with BCG, MMC, or chemohyperthermia. Urologic Oncol (2021) 39:76.e9–.e14. doi: 10.1016/j.urolonc.2020.07.00
87. Yang C, Lv Z, Li Y. Reduction of bladder volume after BCG immunotherapy. Urologia Internationalis (2021) 105:155–8. doi: 10.1159/000510167
88. Soloway MS. Effectiveness of long-term chemotherapy and/or BCG on murine bladder cancer. Natl Cancer Institute monograph (1978) 49:327–32.
89. Lim CJ, Nguyen PHD, Wasser M, Kumar P, Lee YH, Nasir NJM, et al. Immunological hallmarks for clinical response to BCG in bladder cancer. Front Immunol (2020) 11:615091. doi: 10.3389/fimmu.2020.615091
90. Cardona P, Cardona PJ. Regulatory T cells in mycobacterium tuberculosis infection. Front Immunol (2019) 10:2139. doi: 10.3389/fimmu.2019.02139
91. Keefe RC, Takahashi H, Tran L, Nelson K, Ng N, Kühtreiber WM, et al. BCG Therapy is associated with long-term, durable induction of treg signature genes by epigenetic modulation. Sci Rep (2021) 11:14933. doi: 10.1038/s41598-021-94529-2
92. Kuhtreiber WM, Faustman DL. BCG Therapy for type 1 diabetes: Restoration of balanced immunity and metabolism. Trends Endocrinol Metab (2019) 30:80–92. doi: 10.1016/j.tem.2018.11.006
93. Klein BY. Newborn BCG vaccination complemented by boosting correlates better with reduced juvenile diabetes in females, than vaccination alone. Vaccine (2020) 38:6427–34. doi: 10.1016/j.vaccine.2020.07.066
94. Kühtreiber WM, Takahashi H, Keefe RC, Song Y, Tran L, Luck TG, et al. BCG Vaccinations upregulate myc, a central switch for improved glucose metabolism in diabetes. iScience (2020) 23:101085. doi: 10.1016/j.isci.2020.101085
95. Dias HF, Kühtreiber WM, Nelson KJ, Ng NC, Zheng H, Faustman DL. Epigenetic changes related to glucose metabolism in type 1 diabetes after BCG vaccinations: A vital role for KDM2B. Vaccine (2022) 40:1540–54. doi: 10.1016/j.vaccine.2021.04.011
96. Chang YC, Lin CJ, Hsiao YH, Chang YH, Liu SJ, Hsu HY. Therapeutic effects of BCG vaccination on type 1 diabetes mellitus: A systematic review and meta-analysis of randomized controlled trials. J Diabetes Res (2020) 2020:8954125. doi: 10.1155/2020/8954125
97. Bekkering S, Singh K, Lu H, Limawan AP, Nold-Petry CA, Wallace MJ, et al. Neonatal subcutaneous BCG vaccination decreases atherosclerotic plaque number and plaque macrophage content in ApoE(-/-) mice. Biology (2022) 11:151. doi: 10.3390/biology11101511
98. Ovchinnikova OA, Berge N, Kang C, Urien C, Ketelhuth DF, Pottier J, et al. Mycobacterium bovis BCG killed by extended freeze-drying induces an immunoregulatory profile and protects against atherosclerosis. J Intern Med (2014) 275:49–58. doi: 10.1111/joim.12127
99. van Dam AD, Bekkering S, Crasborn M, van Beek L, van den Berg SM, Vrieling F, et al. BCG Lowers plasma cholesterol levels and delays atherosclerotic lesion progression in mice. Atherosclerosis (2016) 251:6–14. doi: 10.1016/j.atherosclerosis.2016.05.031
100. Harrison AG, Lin T, Wang P. Mechanisms of SARS-CoV-2 transmission and pathogenesis. Trends Immunol (2020) 41:1100–15. doi: 10.1016/j.it.2020.10.004
101. Sun P, Lu X, Xu C, Sun W, Pan B. Understanding of COVID-19 based on current evidence. J Med virology. (2020) 92:548–51. doi: 10.1002/jmv.25722
102. Zhou P, Yang XL, Wang XG, Hu B, Zhang L, Zhang W, et al. A pneumonia outbreak associated with a new coronavirus of probable bat origin. Nature (2020) 579:270–3. doi: 10.1038/s41586-020-2012-7
103. Ottenhoff TH, Ab BK, Van Embden JD, Thole JE, Kiessling R. The recombinant 65-kD heat shock protein of mycobacterium bovis bacillus calmette-Guerin/M. tuberculosis is a target molecule for CD4+ cytotoxic T lymphocytes that lyse human monocytes. J Exp Med (1988) 168:1947–52. doi: 10.1084/jem.168.5.1947
104. Finotti P. Sequence similarity of HSP65 of mycobacterium bovis BCG with SARS-CoV-2 spike and nuclear proteins: may it predict an antigen-dependent immune protection of BCG against COVID-19? Cell Stress Chaperones (2021) 27:37–43. doi: 10.1007/s12192-021-01244-y
105. Eggenhuizen PJ, Ng BH, Chang J, Fell AL, Cheong RMY, Wong WY, et al. BCG Vaccine derived peptides induce SARS-CoV-2 T cell cross-reactivity. Front Immunol (2021) 12:692729. doi: 10.3389/fimmu.2021.692729
106. Tomita Y, Sato R, Ikeda T, Sakagami T. BCG Vaccine may generate cross-reactive T cells against SARS-CoV-2: In silico analyses and a hypothesis. Vaccine (2020) 38:6352–6. doi: 10.1016/j.vaccine.2020.08.045
107. Cai C, Peng Y, Shen E, Huang Q, Chen Y, Liu P, et al. A comprehensive analysis of the efficacy and safety of COVID-19 vaccines. Mol Ther (2021) 29:2794–805. doi: 10.1016/j.ymthe.2021.08.001
108. Baden LR, El Sahly HM, Essink B, Kotloff K, Frey S, Novak R, et al. Efficacy and safety of the mRNA-1273 SARS-CoV-2 vaccine. N Engl J Med (2021) 384:403–16. doi: 10.1056/NEJMoa2035389
109. Voysey M, Clemens SAC, Madhi SA, Weckx LY, Folegatti PM, Aley PK, et al. Safety and efficacy of the ChAdOx1 nCoV-19 vaccine (AZD1222) against SARS-CoV-2: an interim analysis of four randomised controlled trials in Brazil, south Africa, and the UK. Lancet (London England) (2021) 397:99–111. doi: 10.1016/S0140-6736(20)32661-1
110. Keech C, Albert G, Cho I, Robertson A, Reed P, Neal S, et al. Phase 1-2 trial of a SARS-CoV-2 recombinant spike protein nanoparticle vaccine. New Engl J Med (2020) 383:2320–32. doi: 10.1056/NEJMoa2026920
111. Xia S, Zhang Y, Wang Y, Wang H, Yang Y, Gao GF, et al. Safety and immunogenicity of an inactivated SARS-CoV-2 vaccine, BBIBP-CorV: a randomised, double-blind, placebo-controlled, phase 1/2 trial. Lancet Infect Dis (2021) 21:39–51. doi: 10.1016/S1473-3099(20)30831-8
112. Zhu FC, Li YH, Guan XH, Hou LH, Wang WJ, Li JX, et al. Safety, tolerability, and immunogenicity of a recombinant adenovirus type-5 vectored COVID-19 vaccine: a dose-escalation, open-label, non-randomised, first-in-human trial. Lancet (2020) 395:1845–54. doi: 10.1016/S0140-6736(20)31208-3
113. Sadoff J, Gray G, Vandebosch A, Cardenas V, Shukarev G, Grinsztejn B, et al. Safety and efficacy of single-dose Ad26.COV2.S vaccine against covid-19. New Engl J Med (2021) 384:2187–201. doi: 10.1056/NEJMoa2101544
114. Hviid A, Hansen JV, Thiesson EM, Wohlfahrt J. Association of AZD1222 and BNT162b2 COVID-19 vaccination with thromboembolic and thrombocytopenic events in frontline personnel : A retrospective cohort study. Ann Internal Med (2022) 175:541–6. doi: 10.7326/M21-2452
115. Pottegård A, Lund LC, Karlstad Ø, Dahl J, Andersen M, Hallas J, et al. Arterial events, venous thromboembolism, thrombocytopenia, and bleeding after vaccination with Oxford-AstraZeneca ChAdOx1-s in Denmark and Norway: population based cohort study. Bmj (2021) 373:n1114. doi: 10.1136/bmj.n1114
116. Zhu F, Jin P, Zhu T, Wang W, Ye H, Pan H, et al. Safety and immunogenicity of a recombinant adenovirus type-5-vectored COVID-19 vaccine with a homologous prime-boost regimen in healthy participants aged 6 years and above: a randomised, double-blind, placebo-controlled, phase 2b trial. Clin Infect Dis (2022) 75:e783–e791. doi: 10.1093/cid/ciab845
117. Lazarus JV, Ratzan SC, Palayew A, Gostin LO, Larson HJ, Rabin K, et al. A global survey of potential acceptance of a COVID-19 vaccine. Nat Med (2021) 27:225–8. doi: 10.1038/s41591-020-1124-9
118. Figa Z, Temesgen T, Zemeskel AG, Ganta M, Alemu A, Abebe M, et al. Acceptance of COVID-19 vaccine among healthcare workers in Africa, systematic review and meta-analysis. Public Health Pract (Oxford England) (2022) 4:100343. doi: 10.1016/j.puhip.2022.100343
119. Dror AA, Eisenbach N, Taiber S, Morozov NG, Mizrachi M, Zigron A, et al. Vaccine hesitancy: the next challenge in the fight against COVID-19. Eur J Epidemiol (2020) 35:775–9. doi: 10.1007/s10654-020-00671-y
120. Troiano G, Nardi A. Vaccine hesitancy in the era of COVID-19. Public Health (2021) 194:245–51. doi: 10.1016/j.puhe.2021.02.025
121. Larson H, Karafilakis E, Antoniadou E, Baka A, Kramarz P. Vaccine hesitancy among healthcare workers and their patients in Europe. Vaccine (2015) 34:5013–20. doi: 10.1016/j.vaccine.2016.08.029
122. Miller A, Reandelar MJ, Fasciglione K, Roumenova V, Otazu GH. Correlation between universal BCG vaccination policy and reduced morbidity and mortality for COVID-19: an epidemiological study. medRxiv (2020), 20042937. doi: 10.1101/2020.03.24.20042937
123. Curtis N, Sparrow A, Ghebreyesus TA, Netea MG. Considering BCG vaccination to reduce the impact of COVID-19. Lancet (London England) (2020) 395:1545–6. doi: 10.1016/S0140-6736(20)31025-4
124. Gupta PK. New disease old vaccine: Is recombinant BCG vaccine an answer for COVID-19? Cell Immunol (2020) 356:104187–. doi: 10.1016/j.cellimm.2020.104187
125. Ebina-Shibuya R, Horita N, Namkoong H, Kaneko T. Current national policies for infant universal bacille calmette-guérin vaccination were associated with lower mortality from coronavirus disease 2019. Clin Exp Vaccine Res (2020) 9:179–82. doi: 10.7774/cevr.2020.9.2.179
126. Czajka H, Zapolnik P, Krzych Ł, Kmiecik W, Stopyra L, Nowakowska A, et al. A multi-center, randomised, double-blind, placebo-controlled phase III clinical trial evaluating the impact of BCG re-vaccination on the incidence and severity of SARS-CoV-2 infections among symptomatic healthcare professionals during the COVID-19 pandemic in Poland-first results. Vaccines (Basel) (2022) 10:314. doi: 10.3390/vaccines10020314
127. Upton CM, van Wijk RC, Mockeliunas L, Simonsson USH, McHarry K, van den Hoogen G, et al. Safety and efficacy of BCG re-vaccination in relation to COVID-19 morbidity in healthcare workers: A double-blind, randomised, controlled, phase 3 trial. EClinicalMedicine (2022) 48:101414. doi: 10.1016/j.eclinm.2022.101414
Keywords: Bacille Calmette-Guerin (BCG), COVID-19, specific and non-specific immunity, tuberculosis (TB), trained immunity
Citation: Du J, Su Y, Wang R, Dong E, Cao Y, Zhao W and Gong W (2023) Research progress on specific and non-specific immune effects of BCG and the possibility of BCG protection against COVID-19. Front. Immunol. 14:1118378. doi: 10.3389/fimmu.2023.1118378
Received: 07 December 2022; Accepted: 20 January 2023;
Published: 31 January 2023.
Edited by:
Jochen Mattner, University of Erlangen Nuremberg, GermanyReviewed by:
Anil Pooran, University of Cape Town, South AfricaMangalakumari Jeyanathan, McMaster University, Canada
Copyright © 2023 Du, Su, Wang, Dong, Cao, Zhao and Gong. This is an open-access article distributed under the terms of the Creative Commons Attribution License (CC BY). The use, distribution or reproduction in other forums is permitted, provided the original author(s) and the copyright owner(s) are credited and that the original publication in this journal is cited, in accordance with accepted academic practice. No use, distribution or reproduction is permitted which does not comply with these terms.
*Correspondence: Wenping Gong, Z3dwODkxMDE1QHdodS5lZHUuY24=; Jingli Du, ZHVqaW5nbGkyMDA2QHNvaHUuY29t
†These authors have contributed equally to this work