- 1Transplant Immunology and Immunogenetics Lab, Advanced Centre for Treatment, Education and Research in Cancer (ACTREC), Tata Memorial Centre, Navi Mumbai, India
- 2Homi Bhabha National Institute, Mumbai, India
Acute Myeloid Leukemia (AML) is a complex disease with rapid progression and poor/unsatisfactory outcomes. In the past few years, the focus has been on developing newer therapies for AML; however, relapse remains a significant problem. Natural Killer cells have strong anti-tumor potential against AML. This NK-mediated cytotoxicity is often restricted by cellular defects caused by disease-associated mechanisms, which can lead to disease progression. A stark feature of AML is the low/no expression of the cognate HLA ligands for the activating KIR receptors, due to which these tumor cells evade NK-mediated lysis. Recently, different Natural Killer cell therapies have been implicated in treating AML, such as the adoptive NK cell transfer, Chimeric antigen receptor-modified NK (CAR-NK) cell therapy, antibodies, cytokine, and drug treatment. However, the data available is scarce, and the outcomes vary between different transplant settings and different types of leukemia. Moreover, remission achieved by some of these therapies is only for a short time. In this mini-review, we will discuss the role of NK cell defects in AML progression, particularly the expression of different cell surface markers, the available NK cell therapies, and the results from various preclinical and clinical trials.
1 Introduction
1.1 Acute myeloid leukemia
Acute Myeloid Leukemia (AML) is a type of blood cancer that affects the cells of the myeloid lineage (1). It is the most common type of blood cancer with poor survival due to the aggressiveness of the disease and high relapse rates (2, 3). To date, limited therapeutic options are available to treat AML (1, 3). Chemotherapy is the primary treatment given to AML patients. Depending on the risk of the disease, some patients may undergo Hematopoietic Stem Cell Transplantation (HSCT) (2, 3).
In the past few years, novel treatment options have been explored to improve the survival of patients suffering from AML. Targeted gene therapies, cytokine therapy, immunotherapy, etc., are some of the upcoming novel therapeutic options. Of these, immunotherapy is a promising strategy that explores the use of the patient’s immune system to kill leukemic cells (2–4).
1.2 NK cells and its receptors
Natural Killer cells play an essential role in innate immunity, and their anti-leukemic properties have been explored in the past few years (5). This anti-leukemic activity is driven by receptors on their surface, which transduces either activating or inhibitory signals. Most of these receptors belong to Killer Immunoglobulin-like receptors (KIRs), natural cytotoxicity receptors (NCRs) or NKG2 (Natural Killer Group 2) receptors. A balance between the activating and inhibitory receptors drives NK cell cytotoxicity. KIRs, NCRs and NKG2 can either activate NK cells by signaling through their immune receptor tyrosine-based activating motifs (ITAMs) or inhibit NK cells by signaling through their immune receptor tyrosine-based inhibitory motifs (ITIMs). These receptors need to recognize their cognate ligands to achieve their cytotoxic potential.
The KIR genes are present on chromosome 19q13.4. 16 KIR genes encode for the KIR receptors, namely KIR 2DL1, 2DL2, 2DL3, 2DL4, 2DL5, 2DS1, 2DS2, 2DS3, 2DS4, 2DS5, 3DL1, 3DL2, 3DL3, 3DS1 and two pseudogenes 2DP1, 3DP1 (5, 6). Inhibitory KIRs have a long cytoplasmic tail and hence are denoted as ‘L,’ whereas activating receptors have a short cytoplasmic tail and are denoted as ‘S.’Two KIR haplotypes, A and B, are determined by an individual’s KIR gene content. The A haplotype is inhibitory since it has more inhibitory KIR genes (KIR3DL1, KIR3DL2, KIR3DL3, KIR2DL1, KIR2DL3, KIR2DL4) and one activating KIR gene (KIR2DS4). On the other hand, the B haplotype is said to be an activating haplotype since it has more activating KIR genes (KIR2DS1, KIR2DS2, KIR2DS3, KIR2DS5, KIR3DS1) in addition to the inhibitory KIR genes (KIR3DL2, KIR3DL3, KIR2DL1, KIR2DL2, KIR2DL4, KIR2DL5A, KIR2DL5B) (7). The B haplotype is associated with lower relapse rates as it carries more activating KIR genes (8). KIR gene can further be divided into centromeric (Cen) and telomeric (Tel) regions to calculate the KIR B content score which can range from 0-2 B-content motifs for each of the centromeric and telomeric regions (i.e. AA, AB, or BB) (9). The haplogroup, which does not carry any of the B-content motifs is designated as CenAA/Tel-AA, whereas haplogroups that have scores ranging from 1 to 4, are designated as combinations of the following haplotypes CenAB/CenBB/TelAB/TelBB. The ligands for KIRs are the HLA Class I molecules (Bw4, C1, and C2). The inhibitory KIR2DL1 and activating KIR2DS1 recognize the C2 group alleles (possessing Lysine at position 80), the inhibitory KIR2DL2/3 and activating KIR2DS2/3 identify the C1 group alleles (having Asparagine at position 80), KIR3DL1 recognizes the HLA B alleles (with exceptions to B*13:01 and B*13:02) in the Bw4 group and KIR3DL2 identifies the HLA A*03/A*11 alleles (10). In the event of an inhibitory KIR receptor-ligand match, the NK cell activity is inhibited, leading to self-tolerance, whereas, in the case of an activating KIR receptor-ligand match, the NK cell activity is enhanced, leading to an increase in graft versus leukemia effect in the event of a transplant. Downregulation of the HLA ligands is an immune escape mechanism often used by tumor cells (11).
Among the NCRs, the activating NCRs are NKp30, NKp44, and NKp46, the ligands for which include various viral hemagglutinins (12–14), human CMV pp65 protein (15), and ligands such as B7-H6 and BAG6 which are expressed on the tumor cell surface. The NKP46 gene is very close to the Killer immunoglobulin-like receptor family on the human chromosome 19 (19q13.42) (16, 17). NKp44 and NKp30, on the other hand, are located in the MHC Class III region of Chromosome 6 (18, 19). NCRs such as NKp30 and NKp46, which were initially thought to be expressed only on resting NK cells (19– 20), has more recently been reported to be present on some T cells (Vd1 þ T cells for NKp30 and αβ T cells and δγ T cells for NKp44) and NK like cells (21, 22) where they perform similar functions as in NK cells. NKp44, on the other hand, is expressed only in activated NK cells. NCRs bind to their corresponding ligands, increasing cytotoxic activity and cytokine release by NK cells (23).
There are 7 members in the NKG2 family namely NKG2A, NKG2B, NKG2C, NKG2D, NKG2E, NKG2F and NKG2H. These transmembrane glycoproteins are encoded by genes in the NK complex present on chromosome 12. Of these 7 members, NKG2A, NKG2B (mRNA splice variant of NKG2A), NKG2C, NKG2E and NKG2H (mRNA splice variant of NKG2E) form heterodimers with CD94. NKG2F is expressed intracellularly (24).
NKG2A is an inhibitory receptor (25) CD56bright NK cell subsets show a higher expression of NKG2A than CD56dim NK cells (26). CD94/NKG2A recognizes its ligand, HLA-E, a non-classical HLA molecule and transmits inhibitory signals via ITIMs present in the cytoplasmic tails thus inhibiting NK cell activity (24–26). NKG2A is overexpressed on the NK cells of AML patients which is associated with poor remission (26, 27). NKG2C is an activating receptor that is expressed on NK cells during the later stages of maturation (25). It binds to its ligand HLA-E with lower affinity than NKG2A. NKG2C interacts noncovalently to DAP12 containing ITAMs. NKG2D is an activating receptor expressed as a homodimer on NK cells. It binds to its ligand MICA/B and ULBP (also known as NKG2D-L) (28). NKG2D interacts with 2 dimers of DAP10 which recruits phosphatidylinositol-3 kinase (PI3 kinase) thus activating further signaling and NKG2D associated cytotoxic response. Tumor cells tend to shed NKG2D thus facilitating tumor escape (24, 25). NKG2D is underexpressed on NK cells of AML patients (27). NKG2D releases signals to activate the immune system by binding to the NKG2D-L present on AML cells and lyses the leukemic cells. However, in an event of downregulation of NKG2D-L tumor cells evade surveillance facilitating tumor progression (28). This NKG2D-NKG2DL axis is involved in clearing tumor cells in the early phases of cancer development. There are various mechanisms by which tumor cells escape NK-mediated cytotoxicity (Figure 1). These could be due to numerical defects in functional NK cells, defective expression of KIR and NCR receptors on NK cells, and defective maturation of NK cells, among others. This tumor evasion, if left unchecked, leads to the progression of the disease.
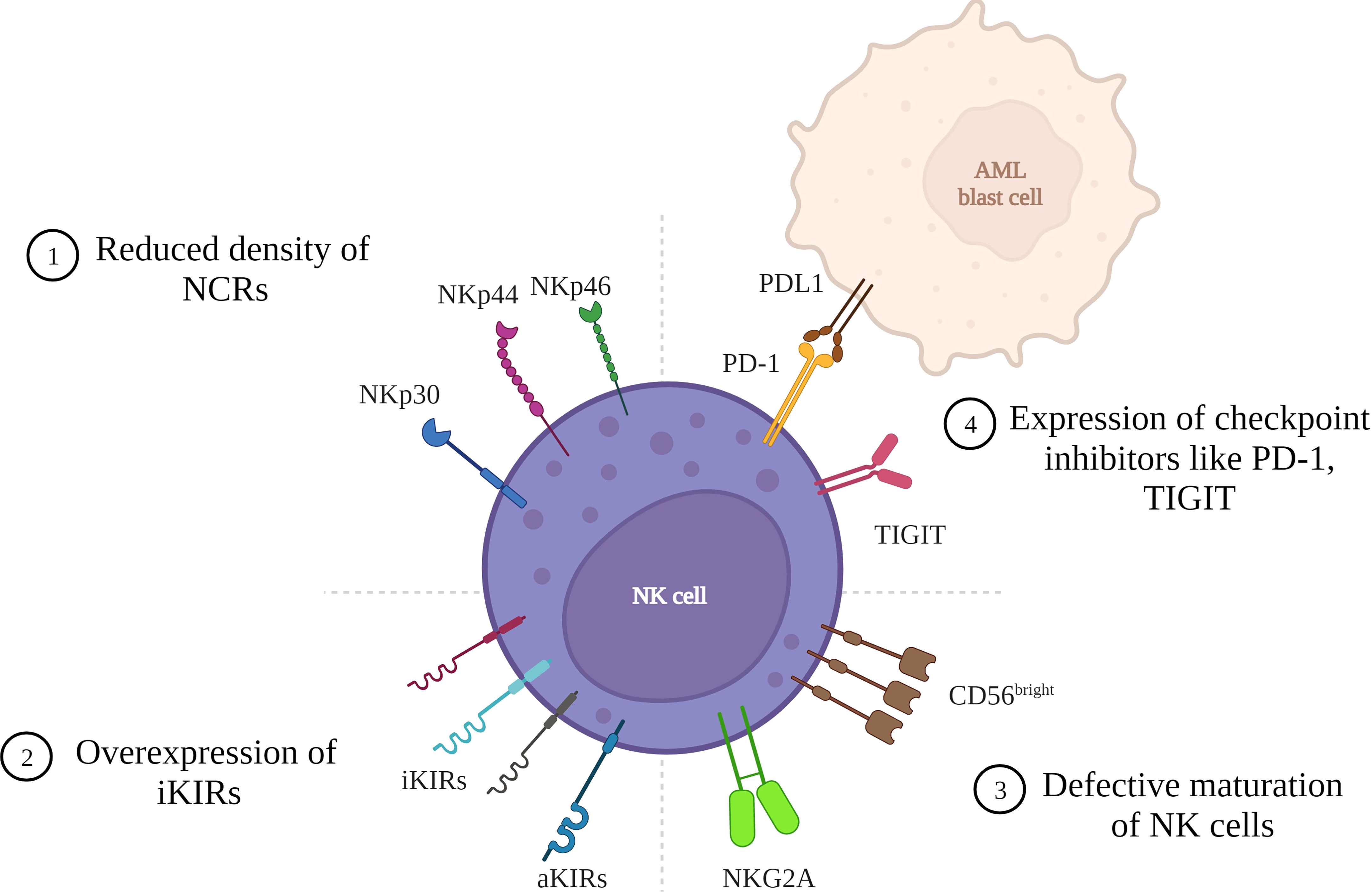
Figure 1 Mechanisms of tumor cell escape in AML due to NK cell defects. AML cells can evade tumor surveillance due to various defects in the NK cell that lead to reduced NK cell cytotoxicity: 1) Reduced density of natural cytotoxicity receptors (NCRs) on NK cell surface leading to a NCRdull phenotype with lower cytolytic activity. 2) Overexpression of inhibitory Killer immunoglobulin like receptors resulting in higher inhibition of cytotoxicity. 3) Defective maturation of NK cells with majority cells with hypomature profile expressing CD56bright/dim KIRs- CD57-. 4) Expression of checkpoint inhibitors like PD-1 and TIGIT resulting in cells with reduced proliferative potential and lower cytotoxic and cytokine-producing capabilities. (Figure created in BioRender).
2 NK cell defects
2.1 Numerical defects
One factor that correlates with AML disease progression is the anti-leukemic activity exhibited by the NK cells, which also depends on the number of functionally active NK cells present. The number of NK cells is lowest during active disease, i.e., at the time of diagnosis and in the event of disease relapse. In contrast, it increases when the patient is in remission (29, 30). Reconstitution of higher NK cells post HSCT has been associated with better 2 year OS, lower rates of CMV reactivation (31), lower 2 year relapse risk (32), lower non relapse mortality, higher progression free survival (33). Conversely, low NK cell counts post 60 days of HSCT was associated with higher relapse risk (34).
2.2 NK receptors expression in AML vs. healthy controls
2.2.1 Natural cytotoxicity receptors (NCR)
The activating NCRs are germline-encoded receptors that are immunoglobulin-like class I transmembrane molecules. They are essential in NK cell cytotoxicity against virus-infected and tumor cells. The brightness of immunofluorescence measures the density of NCRs. Most healthy donors express high densities of NCR exhibiting the NCRbright phenotype, whereas those that carry the NCRdull phenotype express a low density of NCRs (35, 36). There is a correlation between NCR expression and NK cell-mediated cytotoxicity and hence a correlation of NCR expression with leukemia response. NCRbright NK cells display vigorous cytolytic activity compared to the NCRdull NK cells that lack cytolytic activity (36). Various studies have shown that in most AML patients, the cell surface expression of NCRs is downregulated, thus exhibiting the NCRdull phenotype, affecting the NK cell function and cytokine production. Another mechanism by which leukemic blast cells escape immune surveillance is lowering the expression of NCR ligands on their cell surface, thus preventing the engagement of NCRs with their respective ligands required to activate NK cell-mediated target lysis (35–37).
Studies in AML patients suggest better outcomes and more prolonged remission in patients with NK cells having higher cytolytic activity (38–41). Fauriat et al. (34) studied NCR recovery in AML patients who achieved complete remission (CR). They compared the NCR expression on NK cells of these patients at diagnosis and after treatment. They concluded that there was a partial recovery of NKp30 and NKp46 in these patients suggesting a direct correlation between NCRs and leukemic cells.
2.2.2 KIR expression
In the past few years, researchers have sought to investigate the association of KIR and HLA genes with AML. These studies focus on assessing the KIR genes in AML patients and their association with post-transplant complications such as relapse, overall survival (OS), transplant related mortality (TRM) (5, 42). Daniele K.S. et al. (5) compared the KIR genes and haplotype frequency in patients suffering from various hematological malignancies with healthy individuals and observed that the frequency of the inhibitory genes - KIR2DL2 and KIR2DL5 and activating genes - KIR2DS1, KIR2DS2, and KIR2DS3 was more frequent in the controls than in the patients. Moreover, the frequency of KIR2DS3 was higher in AML patients than in ALL patients. The haplotype analysis suggested that haplotype A (more inhibitory) was more frequent in patients than in the controls. Victoria PV et al. (43) also reported a higher frequency of KIR2DL5A in the control group than in AML patients. Another study exploring the association of different AML risk groups with KIR and HLA genotypes observed that the Cen-AB/Tel-AB combination (B content =2), activating gene KIR2DS2 and the Bw4-80I and HLA-C2 allotype, was more frequent in AML patients than in the controls (42). Although these are a few individual studies, more extensive cohort studies are needed to assess the exact role of NK receptor expression profile in relation to AML disease progression.
2.3 Maturation of NK cells
There are two distinct Natural killer cell groups based on CD56 cell surface expression: 90% belong to the CD56dim CD16high group and are cytotoxic, and 10% belong to the CD56bright CD16dim/neg group and exhibit a more immunomodulatory role by releasing different cytokines (44). The maturation process of NK cells starts from CD34+ hematopoietic progenitor cells in the bone marrow, wherein cytokines like IL-15 and c-kit ligand, and flt-3 ligand help them reach their full potential from an NK cell progenitor (CD34dim/CD117-/NKG2A-) to immature CD56+NK cells (CD34-/CD117+/CD56+/-) precursor intermediate and finally a functional CD56bright NK cell (CD34-/CD117+/-/CD94+). They attain full functional maturity by expressing the NKG2A or KIRs (CD34-/CD117-/CD94+/CD16+/KIR+) (45). Another marker of importance in the identification of mature NK cells is CD57. Cells expressing CD57 have high cytotoxic potential with low proliferation capacity. NK cells are the first immune cells to reconstitute post-transplant and are the primary cells to keep leukemia in check immediately post-HSCT. The normal maturation process is essential in conferring NK cells the ability to recognize and eliminate cancer cells. Mundy-Bosse et al. (46) demonstrated that the immature NK phenotype is upregulated in murine AML models, whereas the intermediate phenotype is almost absent. Chretien et al. (47) in their study divided the AML patients into three groups based on their NK maturation profile: hypo-maturation group with CD56bright/dim KIRs- CD57-; intermediate group with CD56dim KIRs-/+ CD57-/+ and hyper-maturation group with CD56dim KIRs+ CD57+ profile. Their study reported that the patients with the hypo-maturation profile had inferior outcomes, such as three-year OS and RFS. This study highlighted that the maturation status of NK cells plays a vital role in predicting long-term outcomes. Post-transplant infections such as cytomegalovirus (CMV) have also been implicated in influencing the reconstitution of mature NK cells post HSCT resulting in lower rate of relapse. Cichocki et al. reported that mature NK cells (CD56dimCD57+NKG2C+) predominantly expand in post HSCT recipients after CMV reactivation leading to better DFS (48). Armin et al. (49) showed that recipients with CMV reactivation had a higher frequency of KIR+NKG2A- NK cells than in CMV seropositive recipients without reactivation and seronegative recipients. Hassan et al. (50) observed a rapid and sustained increase in NK cell numbers in patients with CMV reactivation, with an increase in the proportion of NKG2C expressing NK cells. Mundy-Bosse et al. (46) further showed that AML patients with defective NK cell maturation carrying lower levels of the transcription factors required for terminal NK differentiation (TBET and EOMES) was associated with elevated levels of miR-29b. These are important in controlling final NK cell differentiation. Moreover, lower T-bet expression leads to lower perforin in mature NK cells, which results in NK cells with reduced cytotoxic potential.
2.4 Expression of checkpoint inhibitors
Immune checkpoint molecules are essential in maintaining the immune response by protecting self-cells from lysis. Immune checkpoints like PD-1, TIM-3, and TIGIT regulate NK cell activity (51). PD-1 is expressed in mature NK cells when stimulated by infected cells or tumor cells. NK cells expressing PD-1 have reduced proliferative potential and lower cytotoxic and cytokine-producing capabilities. Blocking the interaction of PD-1 with its ligand PDL-1 by using specific antibodies activates NK cytolytic activity. PDL-1 expression in AML patients has been reported with lower immune recognition (52). Goltz et al. (53) showed that reduced PDL-1 expression in AML cells correlated with better outcomes, such as a lower risk of relapse and prolonged OS. Another checkpoint TIGIT is expressed on NK cells. The ligand for TIGIT is CD112, CD113 and CD155 same as that for DNAM-1. Sanchez-Correa et al. (54) showed low expression of DNAM-1 in AML patients, which could favor the binding of TIGIT to its ligands, sending inhibitory signals and leading to tumor evasion. Blocking TIGIT promotes NK cell-mediated cytotoxicity, as shown in cancer mouse models by Zhang et al. (55).
The above studies highlight the role of different NK cell defects in AML disease susceptibility and progression (Figure 1). Many of these studies formed the basis on which various targets for cell-based therapies were devised for AML.
2.5 Unfavorable tumor microenvironment and epigenetic modifications enabling tumor evasion
The tumor microenvironment (TME) is diverse, made up of stromal cells, immune cells, extracellular matrix and secreted factors (56). Interaction of AML blasts with the TME can be responsible for AML disease development, relapse, progression and resistance to therapy. Mesenchymal stem cells (MSCs) modulate the development and differentiation of hematopoietic stem cells, in the case of AML, the bone marrow MSCs can contribute to tumor progression by providing anti-apoptotic signals to AML blasts (57, 58). Regulatory T cell (Tregs) subsets are responsible for maintaining the peripheral homeostasis and central tolerance by suppressing T- helper (Th) cell proliferation. A few studies have reported increased numbers of Tregs population in AML patients (59, 60), and is associated with unfavorable treatment outcomes (61). Depletion of these tumor associated Tregs results in better cytotoxic T (Tc) cell therapy (62). At the time of diagnosis NK cells in the AML patient are defective due to factors such as downregulation of activating receptors, upregulation of inhibitory receptors and lower cytotoxic and higher immature NK subsets (40, 47, 63). Paczulla et al. (64) showed that downregulation of the ligand for the NKG2D receptor (NKG2DL) via the PARP1 enables tumor evasion in AML blasts (64). Moreover, susceptibility of AML cells to NK cells is affected by the direct interactions between AML cells and mesenchymal stomal cells in the tumor microenvironment (65). Most common reasons for inhibition of NK activity in the TME are hypoxia, low glucose concentrations, cytokines, tumor cell derives factors such as IL6, IL10, TGF-Beta, prostaglandin E2 (PGE2). Myeloid derived suppressor cells (MDSCs) have an immunosuppressive role and expansion of MDSCs in AML patients leads to an immunosuppressive TME with a possible role in tumor progression (66–68). The expansion of MDSCs can take place through different pathways such as MUC1 oncogene expression through c-myc expression. Another group of cells in the TME is the Tumor associated macrophages (TAMs) which promote tumor progression. Al-Mataray et al. (2016) showed that AML promotes infiltration of TAMs (69). Further, AML blasts also secrete various immunosuppressive cytokine and chemokines such as IL-10, TGF B, IL-35 into the tumor microenvironment giving it an immune-inhibiting property (70–72).
Epigenetic modifications such as DNA methylation, histone modifications, Chromatin remodeling, control normal gene expression. Such epigenetic changes can also influence NK cell development, differentiation and functionality. DNA methylation involves transfer of a methyl group to 5’ position of cytosine molecule in CpG sites leading to normal gene expression. DNA methyltransferases (DNMTs) control these DNA methylation patterns. Nucleoside analogues of cytosine are integrated into the DNA and help in covalent bond formation with the DNMT, leading to DNA degradation and inhibition of DNA methylation (73, 74). Hypermethylation of the CpG islands can result in gene silencing. In case of tumor suppressor genes, hypermethylation promotes tumorigenesis (75, 76). Hypomethylating agents (HMAs) such as DNMT inhibitors and 5-aza-2’deoxycytidine (decitabine) have been used for at least a decade to treat high risk AML patients (77). Post translational modifications (PTMs) at the histone N-terminal tails, such as acetylation, methylation, phosphorylation, deamination etc. weakens the packed chromatin structure favoring the binding of transcription factors and normal genetic regulation. However, enzymes such as histone deacetylases (HDACs), demethylases, dephosphorylase reverse this function by removing the acetyl group from the histone tails leading to more compact packing of the DNA around the histones resulting in poor or no binding of transcriptional factors and hence affecting the gene transcription (78, 79). Such modifications of these epigenetic processes favor malignancy and tumor progression.
3 Therapeutic options for AML
3.1 Conventional therapies
Treatment for AML varies based on the stage of the disease and the type of mutation involved. Stem cell transplantation remains the most suitable treatment for AML patients to reduce the risk of relapse and mortality. However, the risk of relapse depends on several other factors, such as the blast clearance status in bone marrow, the presence of FLT3 mutations and cytogenetics, and molecular mutations, among others (80, 81).
Conventional treatment strategies for AML include chemotherapy, targeted therapy, and radiation treatment, with hematopoietic stem cell transplantation (HSCT) as the final treatment option. The most common chemotherapy regimens include standard protocol of 7 days of cytarabine and three days of anthracycline (82), fludarabine–Ara-C–granulocyte colony-stimulating factor–idarubicin (FLAG-IDA) for relapsed childhood AML (83), or similar kind of induction, followed by consolidation chemotherapy and hematopoietic stem cell transplantation in patients who are at a high risk of relapse. Although, these methods have resulted in complete remission in 60-80% of elderly patients the downside to this approach is induction-related mortality due to poor tolerance to these drugs (82, 84, 85).
HiDAC consolidation doses on days 1, 3, and 5 for younger patients not undergoing HSCT is a standard norm (86–88). Consolidation along with an added targeted drug results in more prolonged relapse-free survival (RFS) (89). Most AML patients achieve complete remission (CR) with intensive chemotherapy alone. However, many of those patients would relapse. In such a situation, hematopoietic stem cell transplantation (HSCT) becomes the treatment of choice.
3.2 Therapies targeting the tumor microenvironment and epigenetic modifications
In AML, the leukemic cells interact with the bone marrow microenvironment and secrete a cytokine called KIT ligand, which favors tumor progression by interfering with normal hematopoeisis (90–92). There have been studies to investigate the role of CXCR4/CXCL12 inhibitors, TGF-Beta neutralizing antibodies and use of monoclonal antibodies to block IL-6 (93–96). Hypoxia is another feature of the TME which favors the growth and proliferation of AML cells. Hypoxia downregulates the NK cell ligands like NKp30, NKp44, NKp46, NKG2D, perforin and granzymes. The NKG2D can be restored by administration of IL-2 (97). Hypoxia-activated prodrugs such as Evofosfamide and PR-104 have been shown to inhibit hypoxia associated resistance to therapy in AML (98–101). Glucose is a pre-requisite for generating ATP and NADPH in order for NK cells to function correctly, however, the TME is glucose deprived which impairs NK cell glycolytic activity. Some metabolic regulators like GLUTS have also been shown to regulate glucose influx (102). Recombinant cytokines such as Il2, Il-12, Il-18, IL-15, IL-21, IFN-gamma, GM-CSF have been shown to promote, mediate and enhance NK cell expansion and cytolytic potential (103–106). Although this therapeutic option of targeting different metabolites in the TME is fascinating, in AML the microenvironment is so complex that targeting a single metabolite to get the desired outcomes is a challenge.
Epigenetics is regulated by molecules such as DNA and histone methyltransferases (HMAs) and histone deacetylases (HDACs) (107). DNMT inhibitors dectabine and 5-aza-2’deoxycytidine (DAC) are known HMAs that have been approved for clinical treatment of AML and MDS. DAC is phosphorylated to decitabine triphosphate which then incorporates into the DNA and inhibits DNA synthesis causing a cytotoxic effect, whereas, 5-AZA gets integrated into the RNA structure and interferes with protein formation (108–110). There have been a few clinical trials investigating HMAs for treatment of AML. Issa et al. (111) have reported good efficacy and tolerance with lower doses of these drugs. In the first trials doses of 1500 to 2500 mg/m2 were used to stop DNA synthesis and cause cytotoxicity (112). Cashen et al. (113) in their Phase II clinical trial of decitabine treatment in elderly AML patients showed complete remission (CR) in 24% and overall response rate (ORR) in 25% with a median survival of 14 months. Lubbert et al. (114) treated their AML patients with decitabine + ATRA and observed ORR in 26%, with CR in 13.2% and PR in 12.8% with a median survival time of 5.5 months and 1 year OS of 28%. HDACs regulate cell apoptosis and proliferation by deacetylating lysine residues on proteins. HDAC inhibitors on the other hand inhibit this deacetylation process and help in controlling tumor progression. Some of the HDAC agents currently used are Valproic acid, Vorinostat and Entinostat among others. Vorinostat has a hydroxamic acid component which when binds to the zinc pocket of HDAC 1,2,3 and 6 results in reversible inhibition of HDACs and apoptosis of cancer cells (115).Valproic acid targets the AML1/ETO complex and induces apoptosis of AML cells (116). Entinostat helps in promoting loss of leukemia and longtime survival s proven in animal models (117, 118). Although by themselves HMA and HDAC inhibitors have shown moderate results combination therapies involving these molecules with other therapies may be promising.
3.3 Targeting the NKG2D-NKG2DL
Factors such as viral infections, oxidative damage, DNA damage increase the expression of metalloproteinases on tumor cells. Metalloproteinases have been suggested to be involved in the cleavage of NKG2D-L in AML (119–124). Raneros et al. (125) showed that azacitidine a DNA methyltransferase (DNMT) inhibitor reduces the release of soluble NKG2D-L in AML cells. Poly ADP ribose polymerase 1 (PARP1) inhibits the expression of NKG2D-L proteins in leukemic stem cells (LSCs) helping them evade surveillance (64). In case of DNA damage, the ATM/ATR pathway activates the PARP1 (126, 127), which in turn upregulates the expression of NKG2D-L on the surface of leukemic stem cells in AML (64). Nanbaksh et al. (128) reported that lower expression of c-Myc results in decreased expression of NKG2D-L in AML. Moreover, HDAC inhibitors and demethylating agents can also induce NKG2D-L in tumor cells making them susceptible to NK cells lysis (129–132). A few ongoing clinical trials are focused on targeting the NKG2D/NKG2DL axis in AML. CAR-NKG2D’s have been manufactured by introducing NKG2D into a CAR-T that will target the NKG2D-L in relapsed/refractory (r/r) AML patients (133–138). Baumeister SH et al. (134) confirmed the safety of such a CAR-T in a recent phase 1 clinical trial. These studies provide a new option for the treatment of AML through NK cells by targeting the NKG2D-NKG2D-L axis.
3.4 Hematopoietic stem cell transplantation
HSCT has been designated as the penultimate therapy for adult AML patients in CR1. It can reduce the risk of relapse by more than 60% compared to intensive chemotherapy alone by virtue of its potent graft-versus leukemia (GvL) effect (139). The advance of strategies, such as the use of post-transplant cyclophosphamide, has led to an increase in the number of suitable haplomatched donors for patients who do not find a fully matched HLA donor (140). However, even with changes in conditioning regimens (myeloablative to reduced intensity) and use of graft versus host disease (GvHD) prophylaxis such as cyclosporine, tacrolimus, mycophenolate mofetil, the success of HSCT is marred by post-transplant complications such as relapse, GvHD, and TRM. Hence, newer treatment options with fewer complications are sought. In recent years the role of KIR receptor-HLA ligand mismatch between the patient and donor favoring HSCT outcomes has gained much attention. Table 1 highlights some of the published data on patient-donor KIR genotype, KIR ligand match/mismatch and KIR receptor ligand match on transplant outcomes. Most of these studies show the role of KIR B genotype in reduced risk of leukemia relapse and improved disease-free survival, lower GvHD, lower risk of CMV reactivation (9, 141–143). On the other hand, Bultitude et al. (144) showed higher non relapse mortality (NRM) in AML patients undergoing T cell depleted HLA-matched, unrelated donor HSCT where the donor carried the centromeric KIR B haplotype. Bao et al. (145) reported a higher rate of relapse in patients transplanted with graft from KIR A/A donors, whereas Mansouri et al. (146) reported similar results when the patient had a KIR A/A haplotype. Some reports suggest no role of KIR mismatch on transplant outcomes (147–149), whereas higher TRM, reduced OS and DFS has been reported by Kroger et al. (150), DeSantis et al. (151), in the presence of KIR mismatch transplants. On the other hand, favorable outcomes such as lower aGvHD (152, 153), lower risk of relapse (154–157) and better OS and DFS (158) has been reported by other studies. These contradictory results across studies could be due to the difference in study designs such as heterogenous population, HLA match/mismatch, donor being related/unrelated to the patient and the conditioning regimen used. These studies suggested some insights into the role of KIR and NK cells as the basis for immunotherapies.
3.5 CAR-T therapies
Recently the focus has shifted to more and more adoptive cell therapies for treating leukemias. Chimeric Antigen receptor (CAR)-T cell therapy was the first cell therapy to receive FDA approval after showing success in patients with aggressive B cell malignancies. The idea that T cells could be used to target specific antigens was brought forward by Eshar et al. in 1989 (169). The use of CAR-T by engineering the patient’s self-cells to target CD19 which is an important target in ALL has been evaluated in various studies (170–172). CAR T cells secrete anti-tumor cytokines, perforin, and granzymes into the tumor microenvironment (TME) (173, 174). Generation of CAR-T involves high costs and takes a long time from generation to implementation as the patient’s self-cells are engineered. Various studies have evaluated the role of CAR-Ts targeted towards different AML antigens.
3.6 Monoclonal antibody targeted therapies
The cell surface expression of proteins differs on cancer cells compared to healthy cells. Cancer cells may over-express or under-express a surface protein and cause aberrant expression of proteins (175). CD33, CD45, CD123, and CD244, are some of the surface proteins ubiquitously expressed on AML blast cells (176). These molecules are promising targets for immunotherapy, and currently, there are many ongoing trials focused on developing monoclonal antibodies (mAbs) against these molecules to achieve better outcomes in AML patients. mAbs can be either naked antibodies that function through NK mediated ADCC, such as lintuzumab (anti-CD33) or mAbs conjugated to toxins (e.g., gemtuzumab ozogamicin – anti-CD33), or conjugated to radioactive particles (176).
3.7 NK cell-directed therapies for AML
Natural Killer (NK) cell immunotherapy is a novel immunotherapeutic treatment option. In AML patients, the NK cell function is suppressed, thus enabling the cancer cells’ to evade immune surveillance. NK cell immunotherapy prevents the suppression of NK cells so that they can carry out target lysis. Many clinical trials for various NK cell-based immunotherapies like adoptive NK cell transfer, chimeric antigen receptor (CAR) NK cells, cytokine-induced memory-like (CIML) NK cells, etc., are currently being carried out (2, 4).
NK cells lyse their target firstly by forming an immunological synapse with them, followed by the release of cytolytic granules and cytokines. The second mode of lysis is antibody-mediated cell cytotoxicity (ADCC), wherein NK cells recognize antigen-coated target cells by their FCgammaIIIA (CD16) receptor and trigger ADCC and cytokine production (177, 178). In 2012, Romee et al. (179) demonstrated memory like function of NK cells by showing that if NK cells are preactivated with IL12, IL15, and IL18 followed by 1-3 weeks’ rest, they were able to generate enhanced IFN-gamma production when exposed to cytokines or K562 leukemia cell line. These hypotheses led to the investigation of different cell sources that could be used to generate NK cells in adoptive cellular therapies. Some of the cell sources are cord blood, peripheral blood mononuclear cells (PBMC), cell lines such as NK-92, hematopoietic stem and progenitor cells, and induced pluripotent stem cells (179–188). There are advantages and disadvantages associated with every NK cell source used. Generation of NK cells from donor PBMCs is the safest and most favorable option as they carry various NK cell activating markers such as CD16, NKG2D, NKp44, and NKp46 which strongly recognize and kill non-self or tumor cells; however, the percentage of NK cells in peripheral blood is too low, and expansion of NK cells derived from PBMCs is costly and time-consuming (189–191). NK cells expanded from NK-92 cell lines, which are an immortalized NK lymphoma cell line, raises safety concerns and irradiation of products derived from these cell lines requires irradiation before infusion. NK-92 cell lines also lack various activating KIRs and Cd16 (FCRIII), which may limit their killing potential even through ADCC. The advantages of using NK-92 cell line-derived NK cells are the speed at which they multiply without the need for feeder cells and their ability to secrete higher amounts of perforin granzymes and cytolytic cytokines (192–195). Induced pluripotent stem cells are a good source for NK cells as they have a higher expansion rate; however, these, too, express lower CD16 levels, which could reduce their cytotoxic capacity, although this could be rectified by genetic engineering (196). Not only various sources of NK cells but also different NK cell-based immunotherapies such as adoptive transfer, CAR-NKs, bi-specific and tri-specific killer engagers (BiKEs and TriKEs) are recently being investigated in clinical trials to understand their role as a curative option for AML (Figure 2). Table 2 lists the various completed clinical trials of NK cells in AML.
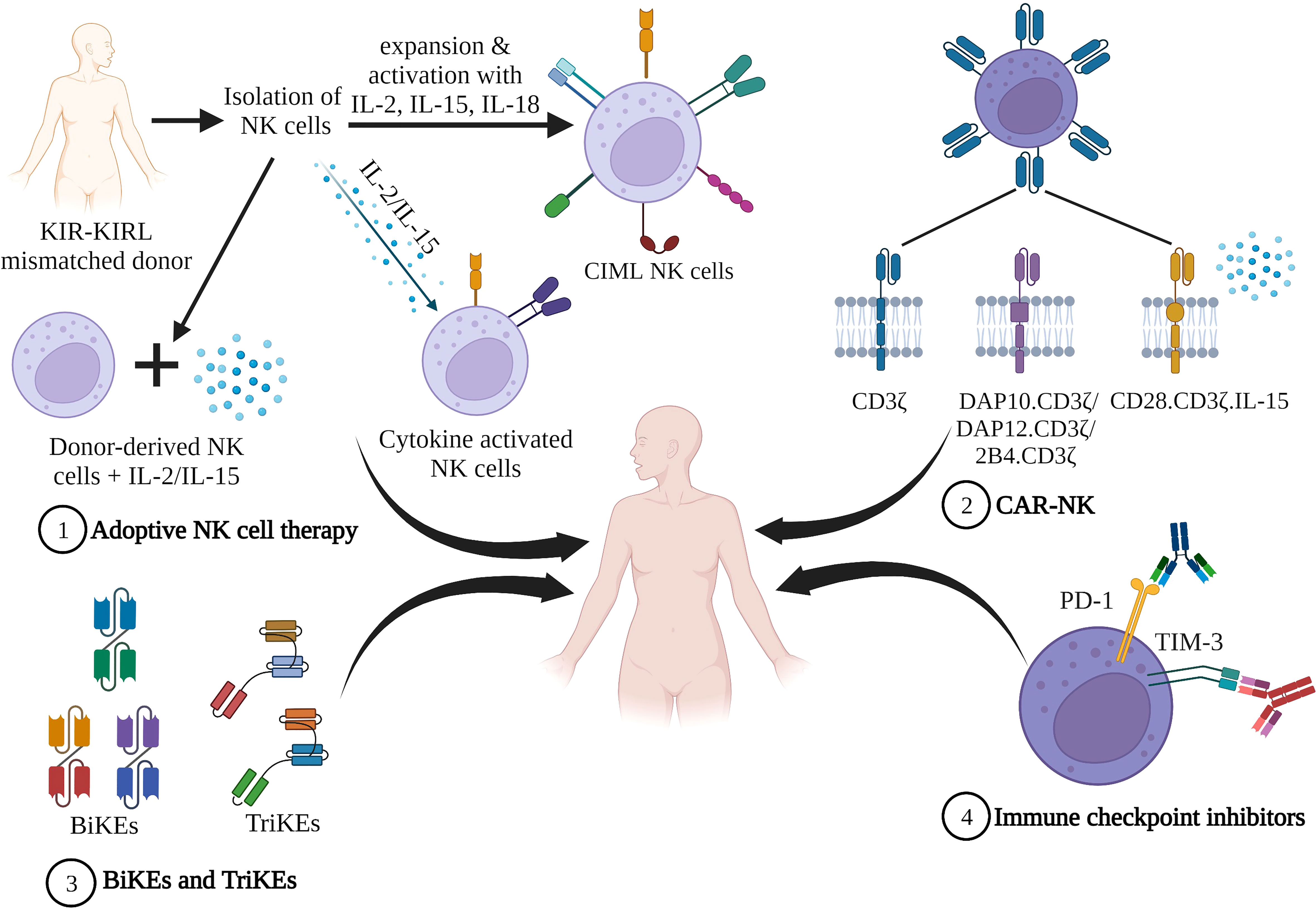
Figure 2 NK cell based immunotherapies. 1) Adoptive NK cell therapy. Infusion of cytokine stimulated ex vivo expanded donor derived NK cells 2) Infusion of CAR-NK construct that comprises an extracellular receptor recognizing a specific antigen, a hinge region, and a transmembrane domain and intracellular co-stimulatory domains. 3) NK cell engagers consisting of bi-specific killer engagers (BiKEs) and tri-specific killer engagers (TriKEs), comprised of a single variable antibody portion (VH and VL) that links to either one (BiKE) or two (TriKE) variable portions on other antibodies. 4) Immune checkpoint proteins when bound to their ligands suppress NK cell activity facilitating tumor evasion. Blocking these checkpoint proteins with inhibitors prevents NK suppression (Figure created in BioRender).
3.7.1 Adoptive NK cell therapy
Adoptive NK cell therapy is the transfer of NK cells to a patient. The NK cells are isolated from a healthy donor (allogenic) or patient (autologous) using PB, bone marrow, or umbilical cord as the source. These NK cells are then either expanded or activated ex vivo using cytokines such as IL-2, IL-12, IL-15, IL-18, and IL-21 (197, 198). NK cells isolated from haploidentical donors show alloreactivity against leukemic cells due to KIR-KIR Ligand (KIR-KIRL) mismatch leading to the GvL effect (197). Currently, many ongoing clinical trials are investigating the efficacy of NK cell therapy in combination with various cytokines. Rubnitz et al. demonstrated the feasibility of donor-recipient inhibitory KIR-HLA mismatched NK cell transfer and the administration of IL-2 after an immunosuppressive regimen in 10 AML patients. Transient engraftment was observed in all patients, all patients were in remission at 2 years and the 2 year event free survival (EFS) was 100% (199).
Inpyo Choi et al. carried out a clinical trial to determine optimal dose of donor NK cell infusion (DNKI) to be administered in patients suffering from various hematological malignancies post HSCT (NCT00823524) (200). On receiving high doses of DNKI, no acute toxicity was observed in the patients, and a significant reduction was observed in post-transplant leukemia progression. Dolstra et al. (182) showed that an increased dose of NK cells generated from umbilical cord blood hematopoietic stem and progenitor cells (HSPC) was well tolerated in AML patients in CR and did not result in toxicity or GVHD.
Many studies involve the use of IL-2 along with NK cell infusion as IL-2 aids in stimulation of the infused NK cells (197). IL-2 is also responsible for the increased expression of the activating receptor NKG2D (190). Miller et al. (201) conducted a study to check the safety and efficacy of haploidentical donor NK cell infusion in AML patients who received either low-intensity or high-intensity immunosuppressive regimens. These patients were given a subcutaneous injection of IL-2 after NK cell infusions. Successful in vivo expansion of donor NK cells was observed in these patients with 60% patients achieving CR. At low doses, IL-2 can stimulate NK cells as well as the Treg of the host, which can affect the NK cell function, whereas high doses can cause toxicity. As an alternative treatment, the use of IL-15 has been considered (202). In a first-in-human trial, recombinant human IL-15 (rhIL-15) was administered intravenously (NCT01385423) or subcutaneously (NCT02395822) to R/R AML patients after haploidentical NK cell transfer. Compared to previous studies using IL-2, it was observed that in vivo NK cell expansion was better when rhIL-15 was used. However, cytokine release syndrome (CRS) was seen in patients who received rhIL-15 subcutaneously (203). In the phase I/II trial, high doses of membrane-bound IL-21 (mb-IL21) ex vivo expanded donor-derived NK cells (NCT01904136) resulted in lower 2-year relapse rate with better disease-free survival (DFS) in patients suffering from various myeloid malignancies (204).
Recently, the use of memory NK cells for the treatment of leukemia has been gaining importance. It has been observed that NK cells activated in response to interleukins like IL-12, IL-15, and IL-18 exhibit memory cell-like characteristics. These cytokine-induced memory-like NK cells (CIML NK), also called memory-like NK cells (ML NK cells), display better effector function (103, 205). Many clinical trials are being carried out to explore CIML NK as an effective immunotherapy against AML (206). Shapiro et al. (207) reported that CIML NK cells expanded in vivo within 30 days of infusion in patients who have relapsed after haploidentical HSCT (NCT040247761). The leukocyte and granulocyte chimerism were high post-CIML NK infusion. Bednarski et al. (208) showed that the adoptive transfer of ML NK cells has no significant toxicity. These ML NK cells display potent cytotoxicity against leukemic cells and demonstrate long-term in vivo persistence.
3.7.2 Monoclonal antibody therapy
Recently, a few clinical trials have been designed to explore the efficiency of killing by NK cells through ADCC using monoclonal antibodies (Table 3).
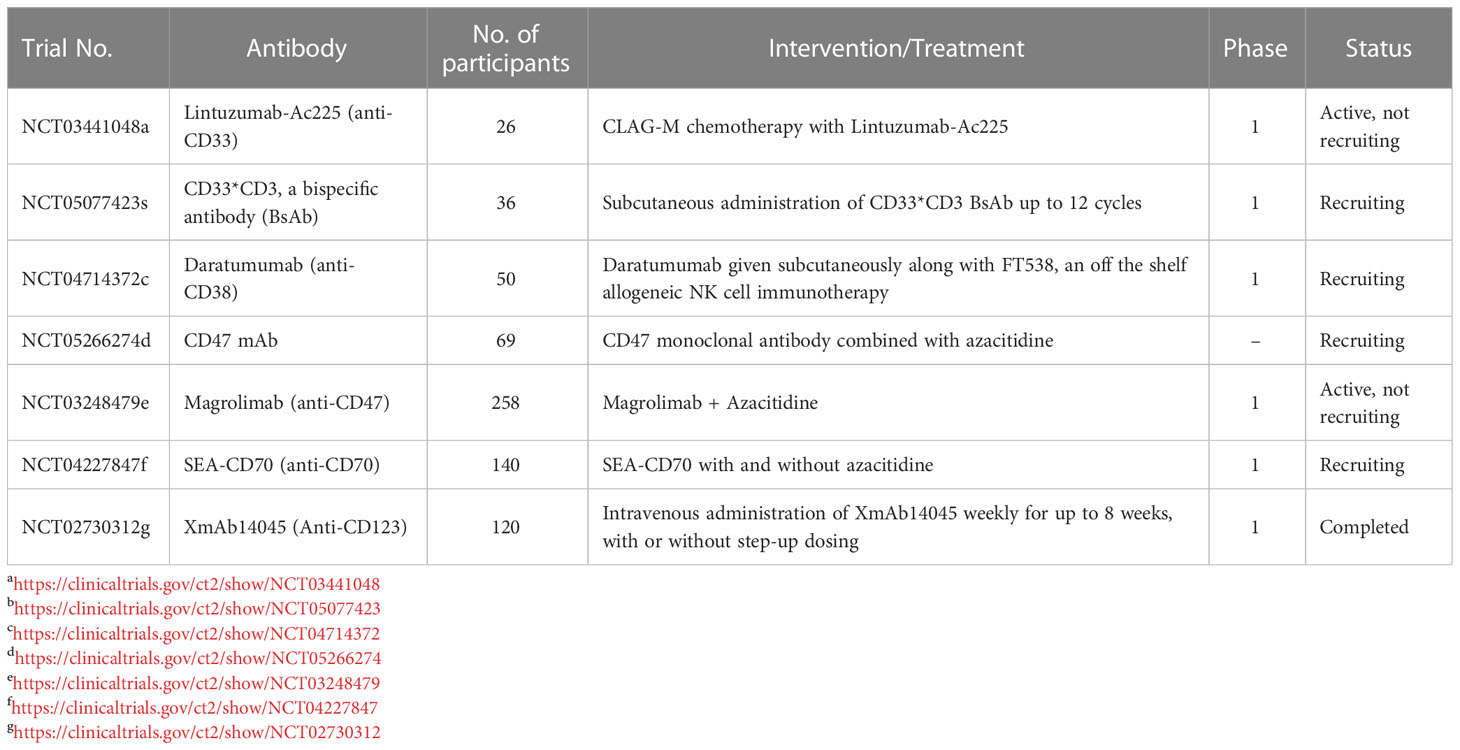
Table 3 Various ongoing clinical trials using monoclonal antibodies against different surface antigens.
3.7.3 CARs
There are four generations of CARs that are currently being investigated: first generation-CARs comprising of the basic structure and one signaling region, second generation-CARs with an extra co-stimulatory domain, third generation –CARs with multiple co-stimulatory domains, and fourth generation-CARs with multiple co-stimulatory domains and cytokine signals (209–214). A CAR construct comprises a synthetic extracellular receptor that will recognize the specific antigen, a hinge region, and a transmembrane domain and may carry one (first generation) (209) or multiple (2nd, 3rd generation) (210–213) intracellular co-stimulatory domains. CARs are fusion proteins expressed on immune cells so that these cells recognize specific targets. Initially, the CARs used for CAR-T therapy comprising CD3 ζ and the T co-stimulatory molecule were used for generating CAR-NK cells and evaluating their efficacy against tumors (215). Although there have been promising results, CAR-T therapy has been associated with side effects such as cytokine release syndrome (216–218), whereby an increased production of IL6, IFN-gamma, GM-CSF, and TNF-alpha result in severe neurological conditions, myalgia, hypoxia, hypotension, and vascular leakage (219). On-target/Off-tumor is another complication associated with CAR-T therapy (217, 220–222). The manufacturing process for CAR-T cells takes about 2-3 weeks post-patient cell accrual. Due to these limitations, researchers have started evaluating other immune cells for adoptive cell therapies. NK cells bind only to their cognate human leukocyte antigen (HLA) ligands on leukemic cells hence taking care of the toxicities that could occur due to nonspecific recognition of healthy cells. This power of distinguishing between self and non-self cells is conferred by the various activating and inhibitory receptors present on the NK cell surface (223, 224). NK cells also do not cause GvHD. These properties of NK cells have made them the cells of choice for adoptive therapies as they overcome most of the problems associated with CAR-T cell therapy.
Although, transfusion of unmodified NK cells has shown some efficacy in AML patients, such NK cells have a short lifespan leading to lower success rates, hence, the focus has shifted to CAR-NK cells, and their efficacy is being tested in various clinical trials. The success received from earlier studies then led to the generation of 2nd and 3rd generation CAR-NKs which utilizes the unique properties of NK cell stimulatory receptors such as DAP12, DAP10, and 2B4 (225–227). DAP10 and DAP12 are intracellular signaling domains and activated molecules such as NKp44, activating KIRs, and NKG2C (226, 228). DAP12 has ITAMs like KIRs, which, once phosphorylated, initiate the release of proinflammatory cytokines such as TNF-alpha and IFN-gamma (226). DAP10, on the other hand, does not carry an ITAM motif but induces potent NK cytotoxicity by signaling through the NKG2D-DAP10 axis (225). Both DAP10, along with the CD3 ζ CAR NK constructs and 2B4 with anti-CD5 CAR NK constructs, have shown promising results in B-ALL and T-ALL, respectively (228, 229). This was then expanded to include multi-specific target strategies (4th generation), such as NKG2D CAR-NK cells which target tumors expressing ligands for NKG2D. The first success of CAR-NK cells in AML was reported by Tang et al. in 2018 (230). In their first-in-man clinical trial (NCT02944162), they tested the safety and efficacy of third generation CD33 directed CAR NK construct with CD28 and 4-1BB co-stimulatory domains using NK cells derived from NK-92 cell lines in 3 relapsed/refractory AML patients. The dosage used was 5x 109 cells. None of the participants had adverse effects; however, there was no durable remission. The main limitation of this study was the short lifespan of the irradiated NK92 cell lines, with the number of CAR-NKs depleting to below measurable levels in 1-week post-infusion. Since there were no unfavorable effects, it was established that functional CAR-NK92 cells could be produced at a much lower cost than the CAR-T cells; however, low numbers post-infusion would still remain a problem. In preclinical studies, Christodoulou et al., 2021 (231) generated CARs with 2B4.ζ or 4-1BB.ζ signaling domains. These CAR NKs were tested for their cytotoxic activity in vitro and xenograft mouse models. It was observed that these CAR NKs show higher anti-AML cytotoxic activity in vitro; this activity could be enhanced by transient expression of secretory IL-15; however, only the 2B4.ζ Chimeric Antigen Receptor (CAR)-NK cells exhibited transient anti-AML activity in mice models. Further, in the in vivo 2B4.ζ/sIL-15 CAR-NK cells experiments, one set showed potent anti-AML activity, whereas the other set showed lethal toxicity. Morgan et al., 2021 (232) used alpha-retroviral vectors to modify NK92 cells and designed a third-generation CAR NK to target CD123, which is strongly expressed on the surface of AML cells. The vector also carried a transgene cassette to allow constitutive expression of human IL15, which ensures enhanced NK persistence in vivo. Salman et al. (233) developed a third-generation CAR construct (CD28-4-1BB- CD3 ζ) with CD4 as a target and tested them against AML in a cell line-derived xenograft mice model. Their study showed specific elimination of primary CD4 positive AML blasts and suppression of disease progression. Quite recently, Mezger’s group from Germany transduced NK-92 cells with CD276 CAR. Simultaneously using CRISPR technology, they introduced gene knock-out of the NK inhibitory checkpoints: CBLB, NKG2A, TIGIT) to enhance NK cytotoxicity. They tested the cytotoxic capacity of these cells against various leukemic cell lines. When they compared the cytotoxic potential between the two groups, there was a significantly higher cytotoxic potential CRISPR-Cas9 knock-out compared to the parental NK-92 cells. It was also observed that the triple knock-out CD276-CAR-NK-92 cells, as well as single CBLB or TIGIT knock-out NK-92 cells, showed significantly superior cytotoxicity against U-937 or U-937 CD19/tag AML cell lines. These results conclude that knock-out cells could be used as promising off-the-shelf therapeutic options for treating AML (234). Another target for NK CAR is NKG2D which interacts with various stress ligands expressed on abnormal cells. In vivo experiments with such NKG2D-directed NK, CARS have shown promising anti-leukemic response (235) and have now moved on to phase I clinical trials in R/R AML patients (NCT04623944). Such off-the-shelf therapeutics can reduce the cost and time required for NK cell therapies. Garrison et al. (236) used a data-driven bioinformatics approach to devise logic-gated CD33-OR-FLT3-NOT-EMCN CAR NK cells. The novel idea behind this approach is that such constructs will specifically target AML blasts and leukemia-initiating cells as they carry both CD33 and FLT3 markers; however, normal hematopoietic stem cells will be unharmed as they carry the endomucin (EMCN) marker. This hypothesis was proven successful in co-cultures. Over the past few years, researchers have constantly been working on developing safer and more effective CAR-NK therapy for AML by using different targets, different sources of NK cells, and different types of CAR constructs. Table 4 shows the clinical trials currently in recruiting stage, where different CAR-NK cells are being tested against AML.
Even with all the advantages of using NK CAR therapies, this technology carries a few disadvantages. The first drawback is the low numbers and short lifespan of NK cells, approximately 1-4 weeks. However, incorporating cytokines such as IL2 and IL15 during the construction of CAR-NKs can improve the expansion and persistence of NK cells post-infusion (237). Newer ex-vivo expansion strategies have been used to generate more functional NK cells that could be used in CAR-NK therapy. These expansion methods involve culturing NK cells with cytokines such as IL15 and IL2, which aid in promoting NK expansion post-infusion and maintaining homeostasis. Large-scale expansion protocols use feeder cells such as Jurkat cell lines and the Epstein Barr virus-transformed lymphoblastic cell lines (EBV-LCL). However, it is yet to be seen which expansion methods can result in GMP-grade NK cells with high expansion potential and sustained numbers post-infusion, along with enhanced cytotoxic activity (238).
Another drawback is that most tumor cells have antigen loss or downregulation of these antigens, evading immune surveillance by CAR-NKs. Researchers have come up with various strategies to combat this issue. One strategy is to target two antigens so that the tumor cell will be detected. This can be done by generating a construct that can encode two different CARs, each with specificity for one antigen or a single CAR with two different recognition domains (239). Further, NK CARs are difficult to engineer due to their higher chances of apoptosis and low gene expression. Gene manipulation in NK cells is currently performed using electroporation which results in rapid transient expression, or by using viral vectors. However, these methods are still under development and need to be optimized for the widespread use of CAR-NK therapies in specific cancers (240, 241). Yet another limitation to CAR-NK-based therapies is that these can detect antigens only when they are expressed on the surface of the cells. Intracellular antigens are presented by MHC molecules and detected by TCRs. Taking advantage of this fact, Dr. Walchli and Dr. Inderberg edited the NK cell to display anti-cancer TCRs on their cell surface. They successfully created a functional NK-CAR expressing a TCR, which could kill cancer cells (242). Although these modified products hold a promising future as therapeutics, they are still in the validation phase, and their potential is yet to be proven.
3.7.4 BiKEs and TriKEs
Although CAR-NKs have been showing promising results in preclinical and early clinical studies, these are expensive, time-consuming, and difficult to expand on a large scale. Hence, newer molecules known as NK cell engagers have recently gained interest as NK-based therapeutics. These molecules consist of bi-specific killer engagers (BiKEs) and tri-specific killer engagers (TriKEs), which are small molecules comprised of a single variable antibody portion (VH and VL) that links to either one (BiKE) or two (TriKE) variable portions on other antibodies. In the case of BiKEs, one of the variable antibody domains recognizes CD16 and the other a targeted tumor antigen (242), whereas, TriKEs, in addition to these two domains, have an interleukin 15 (IL15) element that connects the two different antibody domains. This IL15 moiety enhances proliferation, persistence, cytokine production, and NK cytolytic potential, as proven in in-vitro assays with AML blast cells (243).
Additionally, these molecules may also have a tetra-specific design which can bind to multiple antigens on tumor cells or cross-link with cytokine units to favor NK expansion (243–247).
CD16A on NK cells is a receptor for Fc fragment of IgG antibodies. BiKEs engage CD16 activating receptors present on the NK cells by one of their variable fragments and a target antigen on the tumor cell by the other variable fragment. CD16A carries an ITAM which is phosphorylated upon binding of the CD16A to the antigen bound IgG. This phosphorylation results in inducing a kinase dependent signaling cascade leading to release of cytokines and cytolysis. Such molecules take care of the major difficulties faced with CAR molecules, such as there is no need to engineer these molecules and no requirement for gene transfer, which makes the production and expansion of these molecules much cheaper. Moreover, introduction of IL15 in TriKEs has been shown to improve in-vivo NK cell expansion and tumor control in mice models, taking care of the limitation of low NK cell numbers in the patient (243).
For AML, bi-specific reagents involve a construct of CD33, whereas tri-specific reagents involve a construct of CD33 and CD123 on AML (248–250). Wiernek et al. (251) created a BiKE against AML. This construct comprised a domain-specific for CD16 on NK cells and CD33 on AML cells. This was known as the 1633 BiKE. They, along with Gleason et al. (252), tested the potential of this construct in vitro. The results indicated that such a BiKE could overcome the inhibitory KIR signaling and stimulate NK cells to kill AML blasts, and stimulation of NK cells with such BiKEs in vitro restore the inhibited NK cell function in MDS patients. Valera et al. in 2016 (243) built the first TriKE by improvising the already existing BiKE 1633. They added IL15 to the BiKE and called it 161533, wherein the TriKE facilitates the formation of a synapse between the NK cell (CD16) and the tumor cell (CD33), binding to the CD16 on the NK cells to trigger ADCC and the IL15 will promote NK cell activation and expansion. Wiernek et al. (251) improvised on these TriKEs by co-administering ADAM17 inhibitors, which could help sustain CD16 expression on NK cells. Arvindham et al. (247) recently created a TriKE targeting CLEC12A on AML cells with anti-CD16 single-domain antibody and IL-15. Their results showed a potent NK cell-mediated response against primary patient-derived AML blasts. Another TriKE molecule developed against R/R AML is the GTB-3550 molecule currently in Phase 1/2 trial (NCT03214666). The interim data is promising, with a significant reduction in leukemic blasts in AML patients without adverse side effects such as cytokine release syndrome (253, 254).
There are issues faced with BiKEs and TriKEs, too, as these molecules are still in the early development phase. The use of IL15 in generating TriKEs may also pose a risk for stimulation of T cells resulting in cytokine release syndrome. Another issue similar to CAR-NKs is that TriKEs can kill healthy cells, too, as some of the tumor target antigens can be present on healthy cells too. Further, the effectiveness of such therapies is based on the binding of CD16 of the NK cell with the Fc portion of the antibody. However, mechanisms such as clipping of CD16 by matrix metalloproteins such as ADAM-17 can lead to diminished ADCC. Wernick et al. (251) provided a solution to this problem in their in vitro studies which showed that introducing ADAM-17 inhibitors with such therapies enhances cytolysis by BiKE against myeloid target cells. Another solution could be to target receptors other than CD16 on NK cells, such as NKG2D and 2B4, which have been proven to show similar activation as that compared to CD16 activation alone (255). It is too early to comment on the long-term survival, expansion, cytolytic activity, and safety of using these molecules for NK-mediated immunotherapies, and in-human trial data is warranted.
3.7.5 Immune Checkpoint inhibitor blockade therapy
Newer strategies for NK immunotherapy target immune checkpoints, inhibition of which leads to tumor evasion. In relation to AML, the NK cell receptors such as KIRs and molecules such as TIM-3, CD200R, and others are currently being investigated for their potential in NK-mediated checkpoint inhibitor therapy. Two IgG4 monoclonal antibodies, IPH2101 and IPH2102 (lirilumab), that target KIR2DL1/2/3 NK inhibitory receptors are presently being investigated for single drug/combination therapy (256, 257). Romagne et al. (258), in their in vivo experiments, showed preclinical efficacy of blocking IPH2101 in AML cells. This efficacy was further proven in clinical trials by Vey et al. (257), where comparatively better clinical efficacy was evident in AML patients [NCT01256073, NCT01222286]. In a Phase II efficacy study, administration of Lirilumab did not show a significant difference when compared to placebo [NCT01687387] (259); however, when used in a combination therapy along with azacytidine, it was well tolerated in high-risk R/R AML patients (260).
TIM-3 is a co-inhibitory receptor that recognizes galectin-9 as a ligand. Binding TIM-3 to its ligand induces immune tolerance due to NK cell exhaustion, making it a negative regulator of NK cell immunity. Blocking TIM-3 has been shown to reverse NK cell dysfunction in various diseased conditions (261–265). Recently, an ongoing randomized phase I clinical trial investigating the efficacy of MBG453, an anti-TIM3 antibody alone or in combination with decitabine/spartalizumab/MBG453+decitabine+spartalizumab is underway [NCT03066648]. Another inhibitory receptor expressed on NK cells is CD200R which binds to its ligand CD200 expressed on various tumor tissues. Atfy et al. showed that overexpression of CD200 led to suppressed NK cell anti-tumor activity in AML patients resulting in an increased risk of relapse (266). Coles et al. (267) showed that blocking CD200 restored the NK activity to normal levels. A few other clinical trials investigating blockage of checkpoint inhibitors in AML, such as NCT03248479 [Magrolimab alone or in combination with azaticidine] and NCT03922477 [Magrolimab plus atezolizumab], are still in recruiting phase, and the results are yet to be seen.
4 Future directions
Over the years our understanding of NK biology and its role in designing anti-leukemic therapies has drastically improved. It is a known fact that leukemic patients most often carry NK cells that are dysfunctional/defective/low in numbers. Adoptive NK cell infusions have proven useful in restoring these functions albeit transiently. Recently, the focus has shifted from CAR-T and antibody therapies to NK cell-based immunotherapy due to the higher manufacturing cost and lower safety of T cell-directed therapies. CAR-NKs also exhibit fewer neurological toxicities and cytokine storm than CAR-T cell therapies.
Researchers are investigating cytokine-stimulated NK cell transfer, TriKEs, and BiKEs that boost NK functions and lead to longer proliferation of NK cells post infusion and hence longer periods of remission. Further, antibodies targeting immune checkpoints such as TIGIT, PD-1, and TIM-3 have theoretical potential, as blocking these checkpoints with specific antibodies helps in reversing the diminished NK cytolytic activity in AML patients. A few clinical trials are exploring the potential of combination therapies focusing on the infusion of the NK cell product and conventional treatments. NK cell therapies have proven their dominance over other cellular therapies due to their non MHC restricted recognition of tumor cells, potential to cure wider population, cost-effective manufacturing off the shelf that can be easily expanded in vivo and personalized for every patient. However, most of these studies are still in the preclinical and clinical stages, and as such, more extensive studies with reproducible results on methods of expansion, cryopreservation, infusion protocols, and safety are required to validate these therapies and make them a reality.
Author contributions
The authors confirm contribution to the paper as follows: Concept: MS and SD’S. Draft manuscript preparation: SD’S and AP. Approving and Finalizing manuscript: SD’S and MS. All authors contributed to the article and approved the submitted version.
Conflict of interest
The authors declare that the research was conducted in the absence of any commercial or financial relationships that could be construed as a potential conflict of interest.
Publisher’s note
All claims expressed in this article are solely those of the authors and do not necessarily represent those of their affiliated organizations, or those of the publisher, the editors and the reviewers. Any product that may be evaluated in this article, or claim that may be made by its manufacturer, is not guaranteed or endorsed by the publisher.
Glossary
References
1. Raneros AB, López-Larrea C, Suárez-Álvarez B. Acute myeloid leukemia and NK cells: two warriors confront each other. OncoImmunology (2019) 8:2. doi: 10.1080/2162402X.2018.1539617
2. Kaweme NM, Zhou F. Optimizing NK cell-based immunotherapy in myeloid Leukemia:Abrogating an immunosuppressive microenvironment. Front Immunol (2021) 12:683381. doi: 10.3389/fimmu.2021.683381
3. Hussein BA, Hallner A, Wennström L, Brune M, Martner A, Hellstrand K, et al. Impact of NK cell activating receptor gene variants on receptor expression and outcome of immunotherapy in acute myeloid leukemia. Front Immunol (2021) 12:796072. doi: 10.3389/fimmu.2021.796072
4. Xu J, Niu T. Natural killer cell-based immunotherapy for acute myeloid leukemia. J Hematol Oncol (2020) 13:167. doi: 10.1186/s13045-020-00996-x
5. Sugioka DK, Gonçalves CE, Bicalho MD. KIR repertory in patients with hematopoietic diseases and healthy family members. BMC hematology (2016) 16:25. doi: 10.1186/s12878-016-0064-6
6. Dekojová T, Houdová L, Fatka J, Pitule P, Ostašov P, Caputo VS, et al. Dynamic changes of inhibitory killer-Immunoglobulin-Like receptors on NK cells after allogeneic hematopoietic stem cell transplantation: an initial study. J Clin Med (2020) 9(11):3502. doi: 10.3390/jcm9113502
7. D’Silva SZ, Singh M. Killer immunoglobulin like receptor and hematopoetic stem cell transplantation: a brief review. J Blood Lymph (2018) 8:218. doi: 10.4172/2165-7831.1000218
8. Impola U, Turpeinen H, Alakulppi N, Linjama T, Volin L, Niittyvuopio R, et al. Donor haplotype b of NK KIR receptor reduces the relapse risk in HLA-identical sibling hematopoietic stem cell transplantation of AML patients. Front Immunol (2014) 5:405. doi: 10.3389/fimmu.2014.00405
9. Cooley S, Weisdorf DJ, Guethlein LA, Klein JP, Wang T, Le CT, et al. Donor selection for natural killer cell receptor genes leads to superior survival after unrelated transplantation for acute myelogenous leukemia. Blood. (2010) 116(14):2411–9. doi: 10.1182/blood-2010-05-283051
10. Velardi A. Role of KIRs and KIR ligands in hematopoietic transplantation. Curr Opin Immunol (2008) 20(5):581–7. doi: 10.1016/j.coi.2008.07.004
11. Garrido F, Cabrera T, Lopez-Nevot MA, Ruiz-Cabello F. HLA class I antigens in human tumors. In: F G, Woude V, Klein G, editors. Advances in cancer research, vol. 67. USA: Academic Press (1995). p. 155–95. doi: 10.1016/S0065-230X(08)60713-7
12. Zorn U, Dallmann I, Grosse J, Kirchner H, Poliwoda H, Atzpodien J. Induction of cytokines and cytotoxicity against tumor cells by Newcastle disease virus. Cancer Biother (1994) 9:225–35. doi: 10.1089/cbr.1994.9.225
13. Alsheikhly A, Orvell C, Harfast B, Andersson T, Perlmann P, Norrby E. Sendai-Virusinduced cell-mediated cytotoxicity in vitro. the role of viral glycoproteins in cell-mediated cytotoxicity. Scand J Immunol (1983) 17:129–38. doi: 10.1111/j.1365-3083.1983.tb00775.x
14. Alsheikhly AR, Orvell C, Andersson T, Perlmann P. The role of serologically defined epitopes on mumps virus HN-glycoprotein in the induction of virus-dependent cellmediated cytotoxicity. Anal monoclonal antibodies Scand J Immunol (1985) 22:529–38. doi: 10.1111/j.1365-3083.1985.tb01912.x
15. Arnon TI, Achdout H, Levi O, Markel G, Saleh N, Katz G, et al. Inhibition of the NKp30 activating receptor by pp65 of human cytomegalovirus. Nat Immunol (2005) 6:515–23. doi: 10.1038/ni1190
16. Ponassi M, Cantoni C, Biassoni R, Conte R, Spallarossa A, Pesce A, et al. Structure of the human NK cell triggering receptor NKp46 ectodomain. Biochem Biophys Res Commun (2003) 309:317–23. doi: 10.1016/j.bbrc.2003.08.007
17. Foster CE, Colonna M, Sun PD. Crystal structure of the human NK cell activating receptor NKp46 reveals structural relationship to other leukocyte receptor complex immunoreceptors. J Biol Chem (2003) 278:46081–6. doi: 10.1074/jbc.M308491200
18. Cantoni C, Bottino C, Vitale M, Pessino A, Augugliaro R, Malaspina A, et al. NKp44, a triggering receptor involved in tumor cell lysis by activated human natural killer cells, is a novel member of the immunoglobulin superfamily. J Exp Med (1999) 189:787–96. doi: 10.1084/jem.189.5.787
19. Pende D, Parolini S, Pessino A, Sivori S, Augugliaro R, Morelli L, et al. Identification and molecular characterization of NKp30, a novel triggering receptor involved in natural cytotoxicity mediated by human natural killer cells. J Exp Med (1999) 190:1505–16. doi: 10.1084/jem.190.10.1505
20. Moretta A, Bottino C, Mingari MC, Biassoni R, Moretta L. What is a natural killer cell? nat. Immunol. (2002) 3:6–8. doi: 10.1038/ni0102-6
21. Meresse B, Curran SA, Ciszewski C, Orbelyan G, Setty M, Bhagat G, et al. Reprogramming of CTLs into natural killer-like cells in celiac disease. J.Exp.Med. (2006) 203:1343–55. doi: 10.1084/jem.20060028
22. von Lilienfeld-Toal M, Nattermann J, Feldmann G, Sievers E, Frank S, Strehl J, et al. Activated gammadelta T cells express the natural cytotoxicity receptor natural killer p 44 and show cytotoxic activity against myeloma cells. Clin Exp Immunol (2006) 144:528–33. doi: 10.1111/j.1365-2249.2006.03078.x
23. Moretta A, Biassoni R, Bottino C, Mingari MC, Moretta L. Natural cytotoxicity receptors that trigger human NK-cell-mediated cytolysis. Immunol Today (2000) 21:228–34. doi: 10.1016/s0167-5699(00)01596-6
24. Posch P, Hurley CK. (2011). Histocompatibility. HLA and other systems. Blood and Bone Marrow Pathology. 641–76. doi: 10.1016/B978-0-7020-3147-2.00039-0
25. Sivori S, Vacca P, Del Zotto G, Munari E, Mingari MC, Moretta L. Human NK cells: surface receptors, inhibitory checkpoints, and translational applications. Cell Mol Immunol (2019) 16(5):430–41. doi: 10.1038/s41423-019-0206-4
26. Fisher JG, Doyle ADP, Graham LV, Khakoo SI, Blunt MD. Disruption of the NKG2A: HLA-e immune checkpoint axis to enhance NK cell activation against cancer. Vaccines. (2022) 10:1993. doi: 10.3390/vaccines10121993
27. Sandoval-Borrego D, Moreno-Lafont MC, Vazquez-Sanchez EA, Gutierrez-Hoya A, López-Santiago R, Montiel-Cervantes LA, et al. Overexpression of CD158 and NKG2A inhibitory receptors and underexpression of NKG2D and NKp46 activating receptors on NK cells in acute myeloid leukemia. Arch Med Res (2016) 47(1):55–64. doi: 10.1016/j.arcmed.2016.02.001
28. Wu Z, Zhang H, Wu M, Peng G, He Y, Wan N, et al. Targeting the NKG2D/NKG2D-l axis in acute myeloid leukemia. BioMed Pharmacother (2021) 137:111299. doi: 10.1016/j.biopha.2021.111299
29. Pizzolo G, Trentin L, Vinante F, Agostini C, Zambello R, Masciarelli M, et al. Natural killer cell function and lymphoid subpopulations in acute non-lymphoblastic leukaemia in complete remission. Br J Cancer (1988) 58:368–72. doi: 10.1038/bjc.1988.221
30. Rey J, Fauriat C, Kochbati E, Orlanducci F, Charbonnier A, D’Incan E, et al. Kinetics of cytotoxic lymphocytes reconstitution after induction chemotherapy in elderly AML patients reveals progressive recovery of normal phenotypic and functional features in NK cells. Front Immunol (2017) 8:64. doi: 10.3389/fimmu.2017.00064
31. Minculescu L, Marquart HV, Friis LS, Petersen SL, Schiødt I, Ryder LP, et al. Early natural killer cell reconstitution predicts overall survival in T cell-replete allogeneic hematopoietic stem cell transplantation. Biol Blood Marrow Transplant (2016) 22(12):2187–93. doi: 10.1016/j.bbmt.2016.09.006
32. Lang P, Pfeiffer M, Teltschik HM, Schlegel P, Feuchtinger T, Ebinger M, et al. Natural killer cell activity influences outcome after T cell depleted stem cell transplantation from matched unrelated and haploidentical donors. Best Pract Res Clin Haematol Sep (2011) 24(3):403–11. doi: 10.1016/j.beha.2011.04.009
33. McCurdy SR, Radojcic V, Tsai H-L, Vulic A, Thompson E, Ivcevic S, et al. Signatures of GVHD and relapse after posttransplant cyclophosphamide revealed by immune profiling and machine learning. Blood (2022) 139(4):608–23. doi: 10.1182/blood.2021013054
34. Dunbar EM, Buzzeo MP, Levine JB, Schold JD, Meier-Kriesche HU, Reddy V. The relationship between circulating natural killer cells after reduced intensity conditioning hematopoietic stem cell transplantation and relapse-free survival and graft-versus-host disease. Haematologica (2008) 93(12):1852–8. doi: 10.3324/haematol.13033
35. Fauriat C, Just-Landi S, Mallet F, Arnoulet C, Sainty D, Olive D, et al. Deficient expression of NCR in NK cells from acute myeloid leukemia: evolution during leukemia treatment and impact of leukemia cells in NCRdull phenotype induction. Blood. (2007) 109(1):323–30. doi: 10.1182/blood-2005-08-027979
36. Costello RT, Sivori S, Marcenaro E, Lafage-Pochitaloff M, Mozziconacci MJ, Reviron D, et al. Defective expression and function of natural killer cell-triggering receptors in patients with acute myeloid leukemia. Blood (2002) 99(10):3661–7. doi: 10.1182/blood.v99.10.3661
37. Lion E, Willemen Y, Berneman ZN, Van Tendeloo VF, Smits EL. Natural killer cell immune escape in acute myeloid leukemia. Leukemia (2012) 26(9):2019–26. doi: 10.1038/leu.2012.87
38. Lowdell MW, Ray N, Craston R, Corbett T, Deane M, Prentice HG. The in vitro detection of anti-leukaemia-specific cytotoxicity after autologous bone marrow transplantation for acute leukaemia. Bone Marrow Transplant (1997) 19:891–7. doi: 10.1038/sj.bmt.1700756
39. Lowdell MW, Craston R, Samuel D, Wood ME, O’Neill E, Saha V, et al. Evidence that continued remission in patients treated for acute leukaemia is dependent upon autologous natural killer cells. Br J Haematol (2002) 117:821–7. doi: 10.1046/j.1365-2141.2002.03495.x
40. Khaznadar Z, Boissel N, Agaugué S, Henry G, Cheok M, Vignon M, et al. Defective NK cells in acute myeloid leukemia patients at diagnosis are associated with blast transcriptional signatures of immune evasion. J Immunol (2015) 195:2580–90. doi: 10.4049/jimmunol.1500262
41. Alcasid M, Ma L, Gotlib JR, Arber DA, Ohgami RS. The clinicopathologic significance of lymphocyte subsets in acute myeloid leukemia. Int J Lab Hematol (2017) 39:129–36. doi: 10.1111/ijlh.12594
42. Shen M, Linn YC, Ren EC. KIR-HLA profiling shows presence of higher frequencies of strong inhibitory KIR-ligands among prognostically poor risk AML patients. Immunogenetics. (2016) 68(2):133–44. doi: 10.1007/s00251-015-0888-4
43. Varbanova VP, Mihailova S, Naumova E, Mihaylova AP. Certain killer immunoglobulin-like receptor (KIR)/KIR HLA class I ligand genotypes influence natural killer antitumor activity in myelogenous leukemia but not in acute lymphoblastic leukemia: a case control leukemia association study. Turkish J haematology: Off J Turkish Soc Haematology (2019) 36(4):238–46. doi: 10.4274/tjh.galenos.2019.2019.0079
44. Ljunggren HG, Malmberg KJ. Prospects for the use of NK cells in immunotherapy of human cancer. Nat Rev Immunol (2007) 7(5):329–39. doi: 10.1038/nri2073
45. Freud AG, Yokohama A, Becknell B, Lee MT, Mao HC, Ferketich AK, et al. Evidence for discrete stages of human natural killer cell differentiation in vivo. J Exp Med (2006) 203:1033–43. doi: 10.1084/jem.20052507
46. Mundy-Bosse BL, Scoville SD, Chen L, McConnell K, Mao HC, Ahmed EH, et al. MicroRNA-29b mediates altered innate immune development in acute leukemia. J Clin Invest (2016) 126(12):4404–16. doi: 10.1172/JCI85413
47. Chretien AS, Fauriat C, Orlanducci F, Galseran C, Rey J, Bouvier, et al. Natural killer defective maturation is associated with adverse clinical outcome in patients with acute myeloid leukemia. Front Immunol (2017) 8:573. doi: 10.3389/fimmu.2017.00573
48. Cichocki F, Taras E, Chiuppesi F, Wagner JE, Blazar BR, Brunstein C, et al. Adaptive NK cell reconstitution is associated with better clinical outcomes. JCI Insight (2019) 24;4(2):e125553. doi: 10.1172/jci.insight.125553
49. Rashidi A, Luo X, Cooley S, Anasetti C, Waller EK, Brunstein CG, et al. The association of CMV with NK-cell reconstitution depends on graft source: results from BMT CTN-0201 samples. Blood Adv (2019) 3(16):2465–9. doi: 10.1182/bloodadvances.2019000298
50. Hassan N, Eldershaw S, Stephens C, Kinsella F, Craddock C, Malladi R, et al. CMV reactivation initiates long-term expansion and differentiation of the NK cell repertoire. Front Immunol (2022) 1;13:935949. doi: 10.3389/fimmu.2022.935949
51. Beldi-Ferchiou A, Caillat-Zucman S. Control of NK cell activation by immune checkpoint molecules. Int J Mol Sci (2017) 18(10):E2129. doi: 10.3390/ijms18102129
52. Berthon C, Driss V, Liu J, Kuranda K, Leleu X, Jouy N, et al. In acute myeloid leukemia, B7-H1 (PD-L1) protection of blasts from cytotoxic T cells is induced by TLR ligands and interferon-gamma and can be reversed using MEK inhibitors. Cancer Immunol Immunother (2010) 59(12):1839–49. doi: 10.1007/s00262-010-0909-y
53. Goltz D, Gevensleben H, Grünen S, Dietrich J, Kristiansen G, Landsberg J, et al. PD-L1 (CD274) promoter methylation predicts survival in patients with acute myeloid leukemia. Leukemia. (2017) 31(3):738–43. doi: 10.1038/leu.2016.328
54. Sanchez-Correa B, Gayoso I, Bergua JM, Casado JG, Morgado S, Solana R, et al. Decreased expression of DNAM-1 on NK cells from acute myeloid leukemia patients. Immunol Cell Biol (2012) 90(1):109–15. doi: 10.1038/icb.2011.15
55. Zhang Q, Bi J, Zheng X, Chen Y, Wang H, Wu W, et al. Blockade of the checkpoint receptor TIGIT prevents NK cell exhaustion and elicits potent anti-tumor immunity. Nat Immunol (2018) 19:723–32. doi: 10.1038/s41590-018-0132-0
56. Casey SC, Amedei A, Aquilano K, Azmi AS, Benencia F, Bhakta D, et al. Cancer prevention and therapy through the modulation of the tumor microenvironment. Semin Cancer Biol (2015) 35 Suppl(Suppl):S199–223. doi: 10.1016/j.semcancer.2015.02.007
57. Garrido SM, Appelbaum FR, Willman CL, Banker DE. Acute myeloid leukemia cells are protected from spontaneous and drug-induced apoptosis by direct contact with a human bone marrow stromal cell line (HS-5). Exp Hematol (2001) 29:448–57. doi: 10.1016/s0301-472x(01)00612-9
58. Chen P, Jin Q, Fu Q, You P, Jiang X, Yuan Q, et al. Induction of multidrug resistance of acute myeloid leukemia cells by cocultured stromal cells via upregulation of the PI3K/Akt signaling pathway. Oncol Res Featur Preclin Clin Cancer Ther (2016) 24:215–23. doi: 10.3727/096504016X14634208143021
59. Szczepanski MJ, Szajnik M, Czystowska M, Mandapathil M, Strauss L, Welsh A, et al. Increased frequency and suppression by regulatory T cells in patients with acute myelogenous leukemia. Clin Cancer Res (2009) 15:3325–32. doi: 10.1158/1078-0432.CCR-08-3010
60. Yang W, Xu Y. Clinical significance of treg cell frequency in acute myeloid leukemia. Int J Hematol (2013) 98:558–62. doi: 10.1007/s12185-013-1436-3
61. Shenghui Z, Yixiang H, Jianbo W, Kang Y, Laixi B, Yan Z, et al. Elevated frequencies of CD4+CD25+CD127lo regulatory T cells is associated to poor prognosis in patients with acute myeloid leukemia. Int J Cancer (2011) 129:1373–81. doi: 10.1002/ijc.25791
62. Zhou Q, Bucher C, Munger ME, Highfill SL, Tolar J, Munn DH, et al. Depletion of endogenous tumor-associated regulatory T cells improves the efficacy of adoptive cytotoxic T-cell immunotherapy in murine acute myeloid leukemia. Blood (2009) 114:3793–802. doi: 10.1182/blood-2009-03-208181
63. Cianga VA, Campos Catafal L, Cianga P, Pavel Tanasa M, Cherry M, Collet P, et al. Natural killer cell subpopulations and inhibitory receptor dynamics in myelodysplastic syndromes and acute myeloid leukemia. Front Immunol (2021) 12:665541. doi: 10.3389/fimmu.2021.665541
64. Paczulla AM, Rothfelder K, Raffel S, Konantz M, Steinbacher J, Wang H, et al. Absence of NKG2D ligands defines leukaemia stem cells and mediates their immune evasion. Nature (2019) 572:254–9. doi: 10.1038/s41586-019-1410-1
65. Vasold J, Wagner M, Drolle H, Deniffel C, Kütt A, Oostendorp R, et al. The bone marrow microenvironment is a critical player in the NK cell response against acute myeloid leukaemia in vitro. Leuk Res (2015) 39(2):257–62. doi: 10.1016/j.leukres.2014.12.001
66. Sun H, Li Y, Zhang Z, Ju Y, Li L, Zhang B, et al. Increase in myeloid-derived suppressor cells (MDSCs) associated with minimal residual disease (MRD) detection in adult acute myeloid leukemia. Int J Hematol (2015) 102:579–86. doi: 10.1007/s12185-015-1865-2
67. Pyzer AR, Stroopinsky D, Rajabi H, Washington A, Tagde A, Coll M, et al. MUC1-mediated induction of myeloid-derived suppressor cells in patients with acute myeloid leukemia. Blood (2017) 129:1791–801. doi: 10.1182/blood-2016-07-730614
68. Jitschin R, Saul D, Braun M, Tohumeken S, Völkl S, Kischel R, et al. CD33/CD3-bispecific T-cell engaging (BiTE®) antibody construct targets monocytic AML myeloid-derived suppressor cells. J Immunother Cancer (2018) 6:116. doi: 10.1186/s40425-018-0432-9
69. Al-Matary YS, Botezatu L, Opalka B, Hones JM, Lams RF, Thivakaran A, et al. Acute myeloid leukemia cells polarize macrophages towards a leukemia supporting state in a growth factor independence 1 dependent manner. Haematologica (2016) 101:1216–27. doi: 10.3324/haematol.2016.143180
70. Chen W, Jin W, Hardegen N, Lei KJ, Li L, Marinos N, et al. Conversion of peripheral CD4+CD25- naive T cells to CD4+CD25+ regulatory T cells by TGF-beta induction of transcription factor Foxp3. J Exp Med (2003) 198:1875–86. doi: 10.1084/jem.20030152
71. Walker MR, Kasprowicz DJ, Gersuk VH, Bènard A, Van Landeghen M, Buckner JH, et al. Induction of FoxP3 and acquisition of T regulatory activity by stimulated human CD4+CD25– T cells. J Clin Investig (2003) 112:1437–43. doi: 10.1172/JCI19441
72. Cools N, Van Tendeloo VFI, Smits ELJM, Lenjou M, Nijs G, Van Bockstaele DR, et al. Immunosuppression induced by immature dendritic cells is mediated by TGF-Beta/IL-10 double-positive CD4+ regulatory T cells. J Cell Mol Med (2008) 12:690–700. doi: 10.1111/j.1582-4934.2007.00084.x
73. Erdmann A, Halby L, Fahy J, Arimondo PB. Targeting DNA methylation with small molecules: what’s next? J Med Chem (2015) 58:2569–83. doi: 10.1021/jm500843d
74. Stresemann C, Lyko F. Modes of action of the DNA methyltransferase inhibitors azacytidine and decitabine. Int J Cancer (2008) 123:8–13. doi: 10.1002/ijc.23607
75. Lyko F. The DNA methyltransferase family: a versatile toolkit for epigenetic regulation. Nat Rev Genet (2018) 19:81–92. doi: 10.1038/nrg.2017.80
76. Chen Z, Chen Z, Chen Z, Zhang Y, Zhang Y, Zhang Y, et al. Role of mammalian DNA methyltransferases in development. Annu Rev Biochem (2020) 89:135–58. doi: 10.1146/annurev-biochem-103019-102815
77. Xu QY, Yu L. Epigenetic therapies in acute myeloid leukemia: the role of hypomethylating agents, histone deacetylase inhibitors and the combination of hypomethylating agents with histone deacetylase inhibitors. Chin Med J (Engl) (2020) 133(6):699–715. doi: 10.1097/CM9.0000000000000685
78. Rothbart SB, Strahl BD. Interpreting the language of histone and DNA modifcations. Biochim Biophys Acta Gene Regul Mech (2014) 1839:627–43. doi: 10.1016/j.bbagrm.2014.03.001
79. Zhao Z, Shilatifard A. Epigenetic modifcations of histones in cancer. Genome Biol (2019) 20:1–16. doi: 10.1186/s13059-019-1870-5
80. Grimwade D, Walker H, Harrison G, Oliver F, Chatters S, Harrison C, et al. The predictive value of hierarchical cytogenetic classification in older adults with AML: analysis of 1065 patients entered into the MRC AML11 trial. Blood. (2001) 98:1312–20. doi: 10.1182/blood.v98.5.1312
81. Wheatley K, Burnett AK, Goldstone AH, Gray RG, Hann IM, Harrison CJ, et al. A simple, robust, validated and highly predictive index for the determination of risk-directed therapy in acute myeloid leukaemia derived from the MRC AML 10 trial. united kingdom medical research council’s adult and childhood leukaemia working parties. Brit J Haematol (1999) 107:69–79. doi: 10.1046/j.1365-2141.1999.01684.x
82. Chen KTJ, Gilabert-Oriol R, Bally MB, Leung AWY. Recent treatment advances and the role of nanotechnology, combination products, and immunotherapy in changing the therapeutic landscape of acute myeloid leukemia. Pharm Res (2019) 36:125. doi: 10.1007/s11095-019-2654-z
83. Nakayama H, Tomizawa D, Tanaka S, Iwamoto S, Shimada A, Saito AM, et al. Fludarabine, cytarabine, granulocyte colony-stimulating factor and idarubicin for relapsed childhood acute myeloid leukemia. Pediatr Int (2017) 59(10):1046–52. doi: 10.1111/ped.13378
84. Tamamyan G, Kadia T, Ravandi F, Borthakur G, Cortes J, Jabbour E, et al. Frontline treatment of acute myeloid leukemia in adults. Crit Rev Oncol Hematol (2017) 110:20–34. doi: 10.1016/j.critrevonc.2016.12.004
85. Knipp S, Hildebrand B, Kündgen A, Giagounidis A, Kobbe G, Haas R, et al. Intensive chemotherapy is not recommended for patients aged >60 years who have myelodysplastic syndromes or acute myeloid leukemia with high-risk karyotypes. Cancer. (2007) 110(2):345–52. doi: 10.1002/cncr.22779
86. Mayer RJ, Davis RB, Schiffer CA, Berg DT, Powell BL, Schulman P, et al. Intensive postremission chemotherapy in adults with acute myeloid leukemia. Cancer Leukemia Group B N Engl J Med (1994) 331(14):896–903. doi: 10.1056/NEJM199410063311402
87. Löwenberg B. Sense and nonsense of high-dose cytarabine for acute myeloid leukemia. Blood. (2013) 121(1):26–8. doi: 10.1182/blood-2012-07-444851
88. Schlenk RF. Post-remission therapy for acute myeloid leukemia. Haematologica. (2014) 99(11):1663–70. doi: 10.3324/haematol.2014.114611
89. Thomas X, De Botton S, Chevret S, Caillot D, Raffoux E, Lemasle E, et al. Clofarabine-based consolidation improves relapse-free survival of younger adults with non-favorable acute myeloid leukemia (AML) in first remission: results of the randomized ALFA-0702/Clara study (NCT 00932412). Blood (2015) 126(23):218. doi: 10.1182/blood.V126.23.218.218
90. Ladikou EE, Sivaloganathan H, Pepper A, Chevassut T. Acute myeloid leukaemia in its niche: the bone marrow microenvironment in acute myeloid leukaemia. Curr Oncol Rep (2020) 22(3):27. doi: 10.1007/s11912-020-0885-0
91. Mosteo L, Storer J, Batta K, Searle EJ, Duarte D, Wiseman DH. The dynamic interface between the bone marrow vascular niche and hematopoietic stem cells in myeloid malignancy. Front Cell Dev Biol (2021) 9:635189. doi: 10.3389/fcell.2021.635189
92. Colmone A, Amorim M, Pontier AL, Wang S, Jablonski E, Sipkins DA. Leukemic cells create bone marrow niches that disrupt the behavior of normal hematopoietic progenitor cells. Science (2008) 322(5909):1861–5. doi: 10.1126/science.1164390
93. Sugiyama T, Kohara H, Noda M, Nagasawa T. Maintenance of the hematopoietic stem cell pool by CXCL12-CXCR4 chemokine signaling in bone marrow stromal cell niches. Immunity (2006) 25(6):977–88. doi: 10.1016/j.immuni.2006.10.016
94. Cancilla D, Rettig MP, DiPersio JF. Targeting CXCR4 in AML and ALL. Front Oncol (2020) 10:1672. doi: 10.3389/fonc.2020.01672
95. Tabe Y, Shi YX, Zeng Z, Jin L, Shikami M, Hatanaka Y, et al. Tgf-bNeutralizing antibody 1d11 enhances cytarabine-induced apoptosis in AML cells in the bone marrow microenvironment. PloS One (2013) 8(6):e62785. doi: 10.1371/journal.pone.0062785
96. Zhang TY, Dutta R, Benard B, Zhao F, Yin R, Majeti R. IL-6 blockade reverses bone marrow failure induced by human acute myeloid leukemia. Sci Transl Med (2020) 12(538):eaax5104. doi: 10.1126/scitranslmed.aax5104
97. Melaiu O, Lucarini V, Cifaldi L, Fruci D. Influence of the tumor microenvironment on NK cell function in solid tumors. Front Immunol (2020) 10:3038. doi: 10.3389/fimmu.2019.03038
98. Irigoyen M, Garcıa-Ruiz JC, Berra E. The hypoxia signalling pathway in í haematological malignancies. Oncotarget (2017) 8(22):36832–44. doi: 10.18632/oncotarget.15981
99. Konopleva M, Thall PF, Yi CA, Borthakur G, Coveler A, Bueso-Ramos C, et al. Phase I/II study of the hypoxia-activated prodrug PR104 in refractory/ relapsed acute myeloid leukemia and acute lymphoblastic leukemia. Haematologica (2015) 100(7):927–34. doi: 10.3324/haematol.2014.118455
100. Coltella N, Valsecchi R, Ponente M, Ponzoni M, Bernardi R. Synergistic leukemia eradication by combined treatment with retinoic acid and HIF inhibition by EZN-2208 (Peg-SN38) in preclinical models of PML-rara and PLZF-Rara–driven leukemia. Clin Cancer Res (2015) 21(16):3685–94. doi: 10.1158/1078-0432.CCR-14-3022
101. Deynoux M, Sunter N, Heí rault O, Mazurier F. Hypoxia and HypoxiaInducible factors in leukemias. Front Oncol (2016) 6:41. doi: 10.3389/fonc.2016.00041
102. El Hassouni B, Granchi C, Valleí s-Martıí A, Supadmanaba IGP, Bononi G, Tuccinardi T, et al. The dichotomous role of the glycolytic metabolism pathway in cancer metastasis: interplay with the complex tumor microenvironment and novel therapeutic strategies. Semin Cancer Biol (2020) 60:238–48. doi: 10.1016/j.semcancer.2019.08.025
103. Romee R, Rosario M, Berrien-Elliott MM, Wagner JA, Jewell BA, Schappe T, et al. Cytokine-induced memory-like natural killer cells exhibit enhanced responses against myeloid leukemia. Sci Transl Med (2016) 8(357):357ra123–357ra123. doi: 10.1126/scitranslmed.aaf2341
104. Berraondo P, Sanmamed MF, Ochoa MC, Etxeberria I, Aznar MA, PeírezGracia JL, et al. Cytokines in clinical cancer immunotherapy. Br J Cancer (2019) 120(1):6–15. doi: 10.1038/s41416-018-0328-y
105. Daher M, Basar R, Gokdemir E, Baran N, Uprety N, Nunez Cortes AK, et al. Targeting a cytokine checkpoint enhances the fitness of armored cord blood CAR-NK cells. Blood (2021) 137(5):624–36. doi: 10.1182/blood.2020007748
106. Freund-Brown J, Chirino L, Kambayashi T. Strategies to enhance NK cell function for the treatment of tumors and infections. Crit Rev Immunol (2018) 38(2):105–30. doi: 10.1615/CritRevImmunol.2018025248
107. Sun Y, Chen BR, Deshpande A. Epigenetic regulators in the development, maintenance, and therapeutic targeting of acute myeloid leukemia. Front Oncol (2018) 8:41. doi: 10.3389/fonc.2018.00041
108. Christman JK. 5-azacytidine and 5-aza-20 -deoxycytidine as inhibitors of DNA methylation: mechanistic studies and their implications for cancer therapy. Oncogene (2002) 21:5483–95. doi: 10.1038/sj.onc.1205699
109. Oki Y, Issa JPJ. (2009). Epigenetic Mechanisms in AML – A Target for Therapy. In: Nagarajan L. (eds) Acute Myelogenous Leukemia. Cancer Treatment and Research, vol 145. New York, NY: Springer. doi: 10.1007/978-0-387-69259-3_2
110. Qin TC, Jelinek J, Si JL, Shu JM, Issa J-PJ. Mechanisms of resistance to 5-aza-20 -deoxycytidine in human cancer cell lines. Blood (2009) 113:659–67. doi: 10.1182/blood-2008-02-140038
111. Issa J-PJ, Garcia-Manero G, Giles FJ, Mannari R, Thomas D, Faderl S, et al. Phase 1 study of low-dose prolonged exposure schedules of the hypomethylating agent 5-aza-20 -deoxycytidine (decitabine) in hematopoietic malignancies. Blood (2004) 103:1635–40. doi: 10.1182/blood-2003-03-0687
112. Daskalakis M, Blagitko-Dorfs N, Hackanson B. Decitabine. Small Molecules in Oncology (2009), 131–157. doi: 10.1007/978-3-642-01222-8_10
113. Cashen AF, Schiller GJ, O’Donnell MR, DiPersio JF. Multicenter, phase II study of decitabine for the first-line treatment of older patients with acute myeloid leukemia. J Clin Oncol (2009) 28:556–61. doi: 10.1200/JCO.2009.23.9178
114. Lübbert M, Rüter BH, Claus R, Schmoor C, Schmid M, Germing U, et al. A multicenter phase II trial of decitabine as first-line treatment for older patients with acute myeloid leukemia judged unfit for induction chemotherapy. Haematologica (2012) 97:393–401. doi: 10.3324/haematol.2011.048231
115. Mitsiades CS, Mitsiades NS, McMullan CJ, Poulaki V, Shringarpure R, Hideshima T, et al. Transcriptional signature of histone deacetylase inhibition in multiple myeloma: biological and clinical implications. Proc Natl Acad Sci (2004) 101:540–5. doi: 10.1073/pnas.2536759100
116. Liu SJ, Klisovic RB, Vukosavljevic T, Yu JH, Paschka P, Huynh L, et al. Targeting AML1/ETO-histone deacetylase repressor complex: a novel mechanism for valproic acid-mediated gene expression and cellular differentiation in AML1/ETO-positive acute myeloid leukemia cells. J Pharmacol Exp Ther (2007) 321:953–60. doi: 10.1124/jpet.106.118406
117. Saito A, Yamashita T, Mariko Y, Nosaka Y, Tsuchiya K, Ando T, et al. A synthetic inhibitor of histone deacetylase, MS-27-275, with marked in vivo antitumor activity against human tumors. Proc Natl Acad Sci (1999) 96:4592–7. doi: 10.1073/pnas.96.8.4592
118. Ramsey JM, Kettyle LM, Sharpe DJ, Mulgrew NM, Dickson GJ, Bijl JJ, et al. Entinostat prevents leukemia maintenance in a collaborating oncogene-dependent model of cytogenetically normal acute myeloid leukemia. Stem Cells (2013) 31:1434–45. doi: 10.1002/stem.1398
119. Liu G, Atteridge CL, Wang X, Lundgren AD, Wu JD. Cutting edge: the membrane type matrix metalloproteinase MMP14 mediates constitutive shedding of MHC class I chain-related molecule a independent of a disintegrin and metalloproteinases, J. Immunol. (2010) 184:3346–50. doi: 10.4049/jimmunol.0903789
120. Waldhauer I, Goehlsdorf D, Gieseke F, Weinschenk T, Wittenbrink M, Ludwig A, et al. Tumor-associated MICA is shed by ADAM proteases. Cancer Res (2008) 68:6368–76. doi: 10.1158/0008-5472.CAN-07-6768
121. Waldhauer I, Steinle A. Proteolytic release of soluble UL16-binding protein 2 from tumor cells. Cancer Res (2006) 66:2520–6. doi: 10.1158/0008-5472.CAN-05-2520
122. Zingoni F, Cecere E, Vulpis C, Fionda R, Molfetta A, Soriani MT, et al. Genotoxic stress induces senescence-associated ADAM10-dependent release of NKG2D MIC ligands in multiple myeloma cells, J. Immunol. (2015) 195:736–48. doi: 10.4049/jimmunol.1402643
123. Zingoni A, Vulpis E, Loconte L, Santoni A. NKG2D ligand shedding in response to stress: role of ADAM10, front. Immunol. (2020) 11:447. doi: 10.3389/fimmu.2020.00447
124. Salih HR, Rammensee HG, Steinle A. Cutting edge: down-regulation of MICA on human tumors by proteolytic shedding, J. Immunol. (2002) 169:4098–102. doi: 10.4049/jimmunol.169.8.4098
125. Raneros AB, Minguela A, Rodriguez RM, Colado E, Bernal T, Anguita E, et al. Increasing TIMP3 expression by hypomethylating agents diminishes soluble MICA, MICB and ULBP2 shedding in acute myeloid leukemia, facilitating NK cell-mediated immune recognition. Oncotarget (2017) 8:31959–76. doi: 10.18632/oncotarget.16657
126. Cerboni A, Zingoni A, Cippitelli M, Piccoli M, Frati L, Santoni A. Antigenactivated human T lymphocytes express cell-surface NKG2D ligands via an ATM/ ATR-dependent mechanism and become susceptible to autologous NK-cell lysis. Blood (2007) 110:606–15. doi: 10.1182/blood-2006-10-052720
127. Gasser S, Orsulic S, Brown EJ, Raulet DH. The DNA damage pathway regulates innate immune system ligands of the NKG2D receptor. Nature (2005) 436:1186–90. doi: 10.1038/nature03884
128. Nanbakhsh A, Pochon C, Mallavialle A, Amsellem S, Bourhis JH, Chouaib S. C-myc regulates expression of NKG2D ligands ULBP1/2/3 in AML and modulates their susceptibility to NK-mediated lysis. Blood (2014) 123:3585–95. doi: 10.1182/blood-2013-11-536219
129. Ullrich E, Koch J, Cerwenka A, Steinle A. New prospects on the NKG2D/ NKG2DL system for oncology. Oncoimmunology (2013) 2:e26097. doi: 10.4161/onci.26097
130. Diermayr S, Himmelreich H, Durovic B, Mathys-Schneeberger A, Siegler U, Langenkamp U, et al. NKG2D ligand expression in AML increases in response to HDAC inhibitor valproic acid and contributes to allorecognition by NK-cell lines with single KIRHLA class I specificities. Blood (2008) 111:1428–36. doi: 10.1182/blood-2007-07-101311
131. Langenkamp U, Siegler U, Jorger S, Diermayr S, Gratwohl A, Kalberer CP, et al. Human acute myeloid leukemia CD34+CD38- stem cells are susceptible to allorecognition and lysis by single KIR-expressing natural killer cells. Haematologica (2009) 94:1590–4. doi: 10.3324/haematol.2009.005967
132. Skov S, Pedersen MT, Andresen L, Straten PT, Woetmann A, Ødum N. Cancer cells become susceptible to natural killer cell killing after exposure to histone deacetylase inhibitors due to glycogen synthase kinase-3-dependent expression of MHC class I-related chain a and b. Cancer Res (2005) 65:11136–45. doi: 10.1158/0008-5472.CAN-05-0599
133. Driouk L, Gicobi J, Kamihara Y, Rutherford K, Dranoff G, Ritz J, et al. Chimeric antigen receptor t cells targeting NKG2D-ligands show robust efficacy against acute myeloid leukemia and T-cell acute lymphoblastic leukemia. Blood (2019) 134:1930. doi: 10.1182/blood-2019-130113
134. Baumeister SH, Murad J, Werner L, Daley H, Trebeden-Negre H, Gicobi JK, et al. Phase i trial of autologous CAR T cells targeting NKG2D ligands in patients with AML/MDS and multiple myeloma, cancer immunol. Res. (2019) 7:100–12. doi: 10.1158/2326-6066.CIR-18-0307
135. Nikiforow S, Werner L, Murad J, Jacobs M, Johnston L, Patches S, et al. Safety data from a first-in-human phase 1 trial of NKG2D chimeric antigen receptor-T cells in AML/MDS and multiple myeloma. Blood (2016) 128:4052–2. doi: 10.1182/blood.v128.22.4052.4052
136. Sallman DA, Brayer J, Sagatys EM, Lonez C, Breman E, Agaugu´e S, et al. NKG2D-based chimeric antigen receptor therapy induced remission in a relapsed/refractory acute myeloid leukemia patient. Haematologica (2018) 103:424–6. doi: 10.3324/haematol.2017.186742
137. Sallman DA, Brayer JB, Poire X, Havelange V, Awada A, Lewalle P, et al. Results from the completed dose-escalation of the hematological arm of the phase I think study evaluating multiple infusions of NKG2D-based CAR T-cells as standalone therapy in relapse/ refractory acute myeloid leukemia and myelodysplastic syndrome patient. Blood (2019) 134:3826. doi: 10.1182/blood-2019-128020
138. Al-Homsi AS, Purev E, Lewalle P, Abdul-Hay M, Pollyea DA, Salaroli A, et al. Interim results from the phase I deplethink trial evaluating the infusion of a NKG2D CAR T-cell therapy post a non-myeloablative conditioning in relapse or refractory acute myeloid leukemia and myelodysplastic syndrome patients. Blood (2019) 134:3844. doi: 10.1182/blood-2019-128267
139. Cornelissen JJ, Breems D, van Putten WL, Gratwohl AA, Passweg JR, Pabst T, et al. Comparative analysis of the value of allogeneic hematopoietic stem-cell transplantation in acute myeloid leukemia with monosomal karyotype versus other cytogenetic risk categories. J Clin Oncol (2012) 30(17):2140–6. doi: 10.1200/JCO.2011.39.6499
140. Luznik L, O'Donnell PV, Symons HJ, Chen AR, Leffell MS, Zahurak M, et al. HLA-haploidentical bone marrow transplantation for hematologic malignancies using nonmyeloablative conditioning and high-dose, posttransplantation cyclophosphamide. Biol Blood Marrow Transplant (2008) 14(6):641–50. doi: 10.1016/j.bbmt.2008.03.005
141. Weisdorf D, Cooley S, Wang T, Trachtenberg E, Vierra-Green C, Spellman S, et al. KIR b donors improve the outcome for AML patients given reduced intensity conditioning and unrelated donor transplantation. Blood Adv (2020) 4(4):740–54. doi: 10.1182/bloodadvances.2021005395
142. Gautam M, Ghimire G, Fu LC. Donor with cen b motifs and high KIR b gene motifs contents has a better HSCT outcome in pediatric patients. South Asian Res J Med Sci (2021) 3(3):25–32.
143. Nakamura R, Gendzekhadze K, Palmer J, Tsai NC, Mokhtari S, Forman SJ, et al. Influence of donor KIR genotypes on reduced relapse risk in acute myelogenous leukemia after hematopoietic stem cell transplantation in patients with CMV reactivation. Leuk Res (2019) 87:106230. doi: 10.1016/j.leukres.2019.106230
144. Bultitude WP, Schellekens J, Szydlo RM, Anthias C, Cooley SA, Miller JS, et al. Presence of donor-encoded centromeric KIR b content increases the risk of infectious mortality in recipients of myeloablative, T-cell deplete, HLA-matched HCT to treat AML. Bone Marrow Transplant (2020) 55(10):1975–84. doi: 10.1038/s41409-020-0858-9
145. Bao X, Hou L, Sun A, Chen M, Chen Z, He J. An allelic typing method for 2DS4 variant used in study of haplotypes of killer cell immunoglobulin-like receptor gene. Int J Lab Hematol (2010) 32(6 Pt 2):625–32. doi: 10.1111/j.1751-553X.2010.01234.x
146. Mansouri M, Villard J, Ramzi M, Alavianmehr A, Farjadian S. Impact of donor KIRs and recipient KIR/HLA class I combinations on GVHD in patients with acute leukemia after HLA-matched sibling HSCT. Hum Immunol (2020) 81(6):285–92. doi: 10.1016/j.humimm.2020.03.004
147. Weisdorf D, Cooley S, Devine S, Fehniger TA, DiPersio J, Anasetti C, et al. T Celldepleted partial matched unrelated donor transplant for advanced myeloid malignancy: KIR ligand mismatch and outcome. Biol Blood Marrow Transplant (2012) 18:937–43. doi: 10.1016/j.bbmt.2011.11.024
148. Sivula J, Volin L, Porkka K, Vettenranta K, Itälä M, Partanen J, et al. Killer-cell immunoglobulin-like receptor ligand compatibility in the outcome of Finnish unrelated donor hematopoietic stem cell transplantation. Transpl Immunol (2007) 18:62–6. doi: 10.1016/j.trim.2007.03.006
149. Farag SS, Bacigalupo A, Eapen M, Hurley C, Dupont B, Caligiuri MA, et al. The effect of KIR ligand incompatibility on the outcome of unrelated donor transplantation: a report from the center for international blood and marrow transplant research, the European blood and marrow transplant registry, and the Dutch registry. Biol Blood Marrow Transplant (2006) 12:876–84. doi: 10.1016/j.bbmt.2006.05.007
150. Kröger N, Binder T, Zabelina T, Wolschke C, Schieder H, Renges H, et al. Low number of donor activating killer immunoglobulin- like receptors (KIR) genes but not KIR-ligand mismatch prevents relapse and improves disease-free survival in leukemia patients after in vivo T-cell depleted unrelated stem cell transplantation. Transplantation (2006) 82:1024–30. doi: 10.1097/01.tp.0000235859.24513.43
151. de Santis D, Bishara A, Witt CS, Nagler A, Brautbar C, Slavin S, et al. Natural killer cell HLA-c epitopes and killer cell immunoglobulin-like receptors both influence outcome of mismatched unrelated donor bone marrow transplants. Tissue Antigens (2005) 65:519–28. doi: 10.1111/j.1399-0039.2005.00396.x
152. Zhang Y, Ye C, Zhu H, Zhuang Y, Chen S, Weng Y, et al. Association of iKIR-mismatch model and donor aKIRs with better outcome in haploidentical hematopoietic stem cell transplantation for acute myeloid leukemia. Front Immunol (2023) 24;13:1091188. doi: 10.3389/fimmu.2022.1091188
153. Davies SM, Iannone R, Alonzo TA, Wang YC, Gerbing R, Soni S, et al. A phase 2 trial of KIR-mismatched unrelated donor transplantation using in vivo T cell depletion with antithymocyte globulin in acute myelogenous leukemia: children's oncology group AAML05P1 study. Biol Blood Marrow Transplant (2020) 26(4):712–7. doi: 10.1016/j.bbmt.2019.12.723
154. Wu GQ, Zhao YM, Lai XY, Luo Y, Tan YM, Shi JM, et al. The beneficial impact of missing KIR ligands and absence of donor KIR2DS3 gene on outcome following unrelated hematopoietic SCT for myeloid leukemia in the Chinese population. Bone Marrow Transplant (2010) 45:1514–21. doi: 10.1038/bmt.2010.3
155. Duan LN, Han HX, Liu J, Yan HM, Zhu L, Xue M, et al. Impact of incompatible killer cell immunoglobulin-like receptor and its ligand on the outcome of haploidentical bone marrow transplantation. Zhongguo Shi Yan Xue Ye Xue Za Zhi (2007) 15:809–15.
156. Yokoyama H, Kanda J, Kawahara Y, Uchida N, Tanaka M, Takahashi S, et al. Reduced leukemia relapse through cytomegalovirus reactivation in killer cell immunoglobulin-like receptor-ligand-mismatched cord blood transplantation. Bone Marrow Transplant (2021) 56(6):1352–63. doi: 10.1038/s41409-020-01203-8
157. Krieger E, Qayyum R, Toor A. Inhibitory KIR-ligand interactions and relapse protection following HLA matched allogeneic HCT for AML. Blood (2020) 136(Supplement 1):47. doi: 10.1182/blood-2020-134614
158. Giebel S, Locatelli F, Lamparelli T, Velardi A, Davies S, Frumento G, et al. Survival advantage with KIR ligand incompatibility in hematopoietic stem cell transplantation from unrelated donors. Blood (2003) 102:814–9. doi: 10.1182/blood-2003-01-0091
159. Dubreuil L, Maniangou B, Chevallier P, Quéméner A, Legrand N, Béné MC, et al. Centromeric KIR AA individuals harbor particular KIR alleles conferring beneficial NK cell features with implications in haplo-identical hematopoietic stem cell transplantation. Cancers (Basel) (2020) 12(12):3595. doi: 10.3390/cancers12123595
160. Wanquet A, Bramanti S, Harbi S, Fürst S, Legrand F, Faucher C, et al. Killer cell immunoglobulin-like receptor-ligand mismatch in donor versus recipient direction provides better graft-versus-tumor effect in patients with hematologic malignancies undergoing allogeneic T cell replete haploidentical transplantation followed by post-tansplant cyclophosphamide. Biol Blood Marrow Transplant (2018) 24:549–54. doi: 10.1016/j.bbmt.2017.11.042
161. Shimoni A, Labopin M, Lorentino F, Van Lint MT, Koc Y, Gülbas Z, et al. Killer cell immunoglobulin-like receptor ligand mismatching and outcome after haploidentical transplantation with post-transplant cyclophosphamide. Leukemia. (2019) 33(1):230–9. doi: 10.1038/s41375-018-0170-5
162. An K, Li B, Luo C, Wang J, Luo C, Chen J. The impact of donor full-length KIR2DS4 in the development of acute and chronic GVHD after unrelated allogeneic HSCT. Pediatr Transplant (2020) 24(6):e13728. doi: 10.1111/petr.13728
163. Tordai A, Bors A, Kiss KP, Balassa K, Andrikovics H, Batai A, et al. Donor KIR2DS1 reduces the risk of transplant related mortality in HLA-C2 positive young recipients with hematological malignancies treated by myeloablative conditioning. PloS One (2019) 14(6):e0218945. doi: 10.1371/journal.pone.0218945
164. Wang X, Liu XF, Shang QN, Yu XX, Fan ZY, Cao XH, et al. Donor activating killer cell immunoglobulin-like receptors genes correlated with Epstein-Barr virus reactivation after haploidentical haematopoietic stem cell transplantation. Br J Haematol (2022) 196(4):1007–17. doi: 10.1111/bjh.17950
165. Ido K, Koh H, Hirose A, Okamura H, Koh S, Nanno S, et al. Donor KIR2DS1-mediated decreased relapse and improved survival depending on remission status at HLA-haploidentical transplantation with post-transplantation cyclophosphamide. Biol Blood Marrow Transplant (2020) 26(4):723–33. doi: 10.1016/j.bbmt.2019.12.765
166. Nikoloudis A, Wagner H, Machherndl-Spandl S, Buxhofer-Ausch V, Strassl I, Stiefel O, et al. Relapse protection following early cytomegalovirus reactivation after hematopoietic stem cell transplantation is limited to HLA-c killer cell immunoglobulin-like receptor ligand homozygous recipients. Transplant Cell Ther (2021) 27(8):686.e1–9. doi: 10.1016/j.jtct.2021.04.028
167. Hsu KC, Chida S, Geraghty DE, Dupont B. The killer cell immunoglobulinlikereceptor (KIR) genomic region: gene-order, haplotypes and allelic polymorphism. Immunol Rev (2002) 190:40–52. doi: 10.1034/j.1600-065x.2002.19004.x
168. Wang H, He Y, Zhai WJ, Wang M, Zhou Z, Zhao YX, et al. The impact of recipient HLA-cw and donor killer immunoglobulin-like receptor genotyping on the outcome of patients receiving HLA-matched sibling donor hematopoietic stem cell transplantation for myeloid malignancies. Swiss Med Wkly (2013) 143:w13717. doi: 10.4414/smw.2013.13717
169. Gross G, Waks T, Eshhar Z. Expression of immunoglobulin-t-cell receptor chimeric molecules as functional receptors with antibody-type specificity. Proc Natl Acad Sci U S A (1989) 86(24):10024–8. doi: 10.1073/pnas.86.24.10024
170. Grupp SA, Kalos M, Barrett D, Aplenc R, Porter DL, Rheingold SR, et al. Chimeric antigen receptor-modified T cells for acute lymphoid leukemia. N Engl J Med (2013) 368(16):1509–18. doi: 10.1056/NEJMoa1215134
171. Kochenderfer JN, Wilson WH, Janik JE, Dudley ME, Stetler-Stevenson M, Feldman SA, et al. Eradication of b-lineage cells and regression of lymphoma in a patient treated with autologous T cells genetically engineered to recognize CD19. Blood. (2010) 116(20):4099–102. doi: 10.1182/blood-2010-04-281931
172. Porter DL, Levine BL, Kalos M, Bagg A, June CH. Chimeric antigen receptor-modified T cells in chronic lymphoid leukemia. N Engl J Med (2011) 365(8):725–33. doi: 10.1056/NEJMoa1103849
173. Zhang E, Xu H. A new insight in chimeric antigen receptor-engineered T cells for cancer immunotherapy. J Hematol Oncol (2017) 10(1):1. doi: 10.1186/s13045-016-0379-6
174. Marofi F, Motavalli R, Safonov VA, Thangavelu L, Yumashev AV, Alexander M, et al. CAR T cells in solid tumors: challenges and opportunities. Stem Cell Res Ther (2021) 12:1–16. doi: 10.1186/s13287-020-02128-1
175. Schürch CM. Therapeutic antibodies for myeloid neoplasms–current developments and future directions. Front Oncol (2018) 8:152. doi: 10.3389/fonc.2018.00152
176. Isidori A, Cerchione C, Daver N, DiNardo C, Garcia-Manero G, Konopleva M, et al. Immunotherapy in acute myeloid leukemia: where we stand. Front Oncol (2021) 11:656218. doi: 10.3389/fonc.2021.656218
177. Prager I, Watzl C. Mechanisms of natural killer cell-mediated cellular cytotoxicity. J Leukoc Biol (2019) 105(6):1319–29. doi: 10.1002/JLB.MR0718-269R
178. Wang W, Erbe AK, Hank JA, Morris ZS, Sondel PM. NK cell-mediated antibody-dependent cellular cytotoxicity in cancer immunotherapy. Front Immunol (2015) 6:368. doi: 10.3389/fimmu.2015.00368
179. Romee R, Schneider SE, Leong JW, Chase JM, Keppel CR, Sullivan RP, et al. Cytokine activation induces human memory-like NK cells. Blood. (2012) 120(24):4751–60. doi: 10.1182/blood-2012-04-419283
180. Shapiro RM, Birch GC, Hu G, Vergara Cadavid J, Nikiforow S, Baginska J, et al. Expansion, persistence, and efficacy of donor memory-like NK cells infused for posttransplant relapse. J Clin Invest (2022) 132(11):e154334. doi: 10.1172/JCI154334
181. Spanholtz J, Tordoir M, Eissens D, Preijers F, van der Meer A, Joosten I, et al. High log-scale expansion of functional human natural killer cells from umbilical cord blood CD34-positive cells for adoptive cancer immunotherapy. PloS One (2010) 5(2):e9221. doi: 10.1371/journal.pone.0009221
182. Dolstra H, Roeven MWH, Spanholtz J, Hangalapura BN, Tordoir M, Maas F, et al. Successful transfer of umbilical cord blood CD34+ hematopoietic stem and progenitor-derived NK cells in older acute myeloid leukemia patients. Clin Cancer Res (2017) 23(15):4107–18. doi: 10.1158/1078-0432.CCR-16-2981
183. Liu E, Tong Y, Dotti G, Shaim H, Savoldo B, Mukherjee M, et al. Cord blood NK cells engineered to express IL-15 and a CD19-targeted CAR show long-term persistence and potent antitumor activity. Leukemia. (2018) 32(2):520–31. doi: 10.1038/leu.2017.226
184. Berrien-Elliott MM, Cashen AF, Cubitt CC, Neal CC, Wong P, Wagner JA, et al. Multidimensional analyses of donor memory-like NK cells reveal new associations with response after adoptive immunotherapy for leukemia. Cancer Discovery (2020) 10(12):1854–71. doi: 10.1158/2159-8290.CD-20-0312
185. Liu E, Marin D, Banerjee P, Macapinlac HA, Thompson P, Basar R, et al. Use of CAR-transduced natural killer cells in CD19-positive lymphoid tumors. N Engl J Med (2020) 382(6):545–53. doi: 10.1056/NEJMoa1910607
186. Hoogstad-van Evert JS, Cany J, van den Brand D, Oudenampsen M, Brock R, Torensma R, et al. Umbilical cord blood CD34+ progenitor-derived NK cells efficiently kill ovarian cancer spheroids and intraperitoneal tumors in NOD/SCID/IL2Rgnull mice. Oncoimmunology. (2017) 6(8):e1320630. doi: 10.1080/2162402X.2017.1320630
187. Knorr DA, Ni Z, Hermanson D, Hexum MK, Bendzick L, Cooper LJ, et al. Clinical-scale derivation of natural killer cells from human pluripotent stem cells for cancer therapy. Stem Cells Transl Med (2013) 2(4):274–83. doi: 10.5966/sctm.2012-0084
188. Li Y, Hermanson DL, Moriarity BS, Kaufman DS. Human iPSC-derived natural killer cells engineered with chimeric antigen receptors enhance anti-tumor activity. Cell Stem Cell (2018) 23(2):181–92.e5. doi: 10.1016/j.stem.2018.06.002
189. Shoae-Hassani A, Behfar M, Mortazavi-Tabatabaei SA, Ai J, Mohseni R, Hamidieh AA. Natural killer cells from the subcutaneous adipose tissue underexpress the NKp30 and NKp44 in obese persons and are less active against major histocompatibility complex class I non-expressing neoplastic cells. Front Immunol (2017) 8:1486. doi: 10.3389/fimmu.2017.01486
190. Kim S, Poursine-Laurent J, Truscott SM, Lybarger L, Song YJ, Yang L, et al. Licensing of natural killer cells by host major histocompatibility complex class I molecules. Nature. (2005) 436(7051):709–13. doi: 10.1038/nature03847
191. Martín-Antonio B, Suñe G, Perez-Amill L, Castella M, Urbano-Ispizua A. Natural killer cells: angels and devils for immunotherapy. Int J Mol Sci (2017) 18(9):1868. doi: 10.3390/ijms18091868
192. Klingemann H, Boissel L, Toneguzzo F. Natural killer cells for immunotherapy - advantages of the NK-92 cell line over blood NK cells. Front Immunol (2016) 7:91. doi: 10.3389/fimmu.2016.00091
193. Zhang C, Oberoi P, Oelsner S, Waldmann A, Lindner A, Tonn T, et al. Chimeric antigen receptor-engineered NK-92 cells: an off-the-Shelf cellular therapeutic for targeted elimination of cancer cells and induction of protective antitumor immunity. Front Immunol (2017) 8:533. doi: 10.3389/fimmu.2017.00533
194. Hermanson DL, Kaufman DS. Utilizing chimeric antigen receptors to direct natural killer cell activity. Front Immunol (2015) 6:195. doi: 10.3389/fimmu.2015.00195
195. Matosevic S. Viral and nonviral engineering of natural killer cells as emerging adoptive cancer immunotherapies. J Immunol Res (2018), 4054815. doi: 10.1155/2018/4054815
196. Zhu H, Blum RH, Bjordahl R, Gaidarova S, Rogers P, Lee TT, et al. Pluripotent stem cell-derived NK cells with high-affinity noncleavable CD16a mediate improved antitumor activity. Blood (2020) 135(6):399–410. doi: 10.1182/blood.2019000621
197. Allison M, Mathews J, Gilliland T, Mathew SO. Natural killer cell-mediated immunotherapy for leukemia. Cancers (Basel) (2022) 14(3):843. doi: 10.3390/cancers14030843
198. Lupo KB, Matosevic S. Natural killer cells as allogeneic effectors in adoptive cancer immunotherapy. Cancers (Basel) (2019) 11(6):769. doi: 10.3390/cancers11060769
199. Rubnitz JE, Inaba H, Ribeiro RC, Pounds S, Rooney B, Bell T, et al. NKAML: a pilot study to determine the safety and feasibility of haploidentical natural killer cell transplantation in childhood acute myeloid leukemia. J Clin Oncol (2010) 28(6):955–9. doi: 10.1200/JCO.2009.24.4590
200. Choi I, Yoon SR, Park SY, Kim H, Jung SJ, Jang YJ, et al. Donor-derived natural killer cells infused after human leukocyte antigen-haploidentical hematopoietic cell transplantation: a dose-escalation study. Biol Blood Marrow Transplant (2014) 20(5):696–704. doi: 10.1016/j.bbmt.2014.01.031
201. Miller JS, Soignier Y, Panoskaltsis-Mortari A, McNearney SA, Yun GH, Fautsch SK, et al. Successful adoptive transfer and in vivo expansion of human haploidentical NK cells in patients with cancer. Blood. (2005) 105(8):3051–7. doi: 10.1182/blood-2004-07-2974
202. Handgretinger R, Lang P, André MC. Exploitation of natural killer cells for the treatment of acute leukemia. Blood. (2016) 127(26):3341–9. doi: 10.1182/blood-2015-12-629055
203. Cooley S, He F, Bachanova V, Vercellotti GM, DeFor TE, Curtsinger JM, et al. First-in-human trial of rhIL-15 and haploidentical natural killer cell therapy for advanced acute myeloid leukemia. Blood Adv (2019) 3(13):1970–80. doi: 10.1182/bloodadvances.2018028332
204. Ciurea SO, Schafer JR, Bassett R, Denman CJ, Cao K, Willis D, et al. Phase 1 clinical trial using mbIL21 ex vivo-expanded donor-derived NK cells after haploidentical transplantation. Blood. (2017) 130(16):1857–68. doi: 10.1182/blood-2017-05-785659
205. Cooper MA, Elliott JM, Keyel PA, Yang L, Carrero JA, Yokoyama WM. Cytokine-induced memory-like natural killer cells. Proc Natl Acad Sci U.S.A. (2009) 106(6):1915–9. doi: 10.1073/pnas.0813192106
206. Parihar R. Memory NK cells to forget relapsed AML. Blood (2022) 139(11):1607–8. doi: 10.1182/blood.2021014906
207. Shapiro RM, Nikiforow S, Rambaldi B, Vergara J, Daley H, Kim HT, et al. Cytokine-induced memory-like NK cells exhibit massive expansion and long-term persistence after infusion post-haploidentical stem cell transplantation: a report of the first three cases in a phase I trial. Blood (2020) 136(1):8–9. doi: 10.1182/blood-2020-133933
208. Bednarski JJ, Zimmerman C, Berrien-Elliott MM, Foltz JA, Becker-Hapak M, Neal CC, et al. Donor memory-like NK cells persist and induce remissions in pediatric patients with relapsed AML after transplant. Blood. (2022) 139(11):1670–83. doi: 10.1182/blood.2021013972
209. Jensen MC, Popplewell L, Cooper LJ, DiGiusto D, Kalos M, Ostberg JR, et al. Antitransgene rejection responses contribute to attenuated persistence of adoptively transferred CD20/CD19-specific chimeric antigen receptor redirected T cells in humans. Biol Blood Marrow Transplant (2010) 16(9):1245–56. doi: 10.1016/j.bbmt.2010.03.014
210. Maher J, Brentjens RJ, Gunset G, Rivière I, Sadelain M. Human T-lymphocyte cytotoxicity and proliferation directed by a single chimeric TCRzeta /CD28 receptor. Nat Biotechnol (2002) 20(1):70–5. doi: 10.1038/nbt0102-70
211. Imai C, Mihara K, Andreansky M, Nicholson IC, Pui CH, Geiger TL, et al. Chimeric receptors with 4-1BB signaling capacity provoke potent cytotoxicity against acute lymphoblastic leukemia. Leukemia. (2004) 18(4):676–84. doi: 10.1038/sj.leu.2403302
212. Carpenito C, Milone MC, Hassan R, Simonet JC, Lakhal M, Suhoski MM, et al. Control of large, established tumor xenografts with genetically retargeted human T cells containing CD28 and CD137 domains. Proc Natl Acad Sci U.S.A. (2009) 106(9):3360–5. doi: 10.1073/pnas.0813101106
213. Hombach AA, Abken H. Costimulation by chimeric antigen receptors revisited the T cell antitumor response benefits from combined CD28-OX40 signalling. Int J Cancer (2011) 129(12):2935–44. doi: 10.1002/ijc.25960
214. Weinkove R, George P, Dasyam N, McLellan AD. Selecting costimulatory domains for chimeric antigen receptors: functional and clinical considerations. Clin Transl Immunol (2019) 8(5):e1049. doi: 10.1002/cti2.1049
215. Imai K, Matsuyama S, Miyake S, Suga K, Nakachi K. Natural cytotoxic activity of peripheral-blood lymphocytes and cancer incidence: an 11-year follow-up study of a general population. Lancet (2000) 356(9244):1795–9. doi: 10.1016/S0140-6736(00)03231-1
216. Morgan RA, Yang JC, Kitano M, Dudley ME, Laurencot CM, Rosenberg SA. Case report of a serious adverse event following the administration of T cells transduced with a chimeric antigen receptor recognizing ERBB2. Mol Ther (2010) 18(4):843–51. doi: 10.1038/mt.2010.24
217. Brudno JN, Kochenderfer JN. Toxicities of chimeric antigen receptor T cells: recognition and management. Blood (2016) 127(26):3321–30. doi: 10.1182/blood-2016-04-703751
218. Shimabukuro-Vornhagen A, Gödel P, Subklewe M, Stemmler HJ, Schlößer HA, Schlaak M, et al. Cytokine release syndrome. J Immunother Cancer (2018) 6(1):56. doi: 10.1186/s40425-018-0343-9
219. Brentjens RJ, Rivière I, Park JH, Davila ML, Wang X, Stefanski J, et al. Safety and persistence of adoptively transferred autologous CD19-targeted T cells in patients with relapsed or chemotherapy refractory b-cell leukemias. Blood (2011) 118(18):4817–28. doi: 10.1182/blood-2011-04-348540
220. Liu X, Zhang N, Shi H. Driving better and safer HER2-specific CARs for cancer therapy. Oncotarget (2017) 8(37):62730. doi: 10.18632/oncotarget.17528
221. Fried S, Avigdor A, Bielorai B, Meir A, Besser MJ, Schachter J, et al. Early and late hematologic toxicity following CD19 CAR-T cells. Bone Marrow Transplant (2019) 54(10):1643–50. doi: 10.1038/s41409-019-0487-3
222. Laszlo GS, Harrington KH, Gudgeon CJ, Beddoe ME, Fitzgibbon MP, Ries RE, et al. Expression and functional characterization of CD33 transcript variants in human acute myeloid leukemia. Oncotarget. (2016) 7(28):43281–94. doi: 10.18632/oncotarget.9674
223. Malmberg KJ, Carlsten M, Björklund A, Sohlberg E, Bryceson YT, Ljunggren HG. Natural killer cell-mediated immunosurveillance of human cancer. Semin Immunol (2017) 31:20–9. doi: 10.1016/j.smim.2017.08.002
224. Lanier LL. Up on the tightrope: natural killer cell activation and inhibition. Nat Immunol (2008) 9(5):495–502. doi: 10.1038/ni1581
225. Billadeau DD, Upshaw JL, Schoon RA, Dick CJ, Leibson PJ. NKG2D-DAP10 triggers human NK cell-mediated killing via a syk-independent regulatory pathway. Nat Immunol (2003) 4(6):557–64. doi: 10.1038/ni929
226. Lanier LL, Corliss BC, Wu J, Leong C, Phillips JH. Immunoreceptor DAP12 bearing a tyrosine-based activation motif is involved in activating NK cells. Nature. (1998) 391(6668):703–7. doi: 10.1038/35642
227. Nakajima H, Colonna M. 2B4: an NK cell activating receptor with unique specificity and signal transduction mechanism. Hum Immunol (2000) 61(1):39–43. doi: 10.1016/s0198-8859(99)00170-6
228. Brentjens R, Yeh R, Bernal Y, Riviere I, Sadelain M. Treatment of chronic lymphocytic leukemia with genetically targeted autologous T cells: case report of an unforeseen adverse event in a phase I clinical trial. Mol Ther (2010) 18(4):666–8. doi: 10.1038/mt.2010.31
229. Xu Y, Liu Q, Zhong M, Wang Z, Chen Z, Zhang Y, et al. 2B4 costimulatory domain enhancing cytotoxic ability of anti-CD5 chimeric antigen receptor engineered natural killer cells against T cell malignancies. J Hematol Oncol (2019) 12(1):49. doi: 10.1186/s13045-019-0732-7
230. Tang X, Yang L, Li Z, Nalin AP, Dai H, Xu T, et al. First-in-man clinical trial of CAR NK-92 cells: safety test of CD33-CAR NK-92 cells in patients with relapsed and refractory acute myeloid leukemia. Am J Cancer Res (2018) 8(6):1083–9.
231. Christodoulou I, Ho WJ, Marple A, Ravich JW, Tam A, Rahnama R, et al. Engineering CAR-NK cells to secrete IL-15 sustains their anti-AML functionality but is associated with systemic toxicities. J Immunother Cancer (2021) 9(12):e003894. doi: 10.1136/jitc-2021-003894
232. Morgan MA, Kloos A, Lenz D, Kattre N, Nowak J, Bentele M, et al. Improved activity against acute myeloid leukemia with chimeric antigen receptor (CAR)-NK-92 cells designed to target CD123. Viruses. (2021) 13(7):1365. doi: 10.3390/v13071365
233. Salman H, Pinz KG, Wada M, Shuai X, Yan LE, Petrov JC, et al. Preclinical targeting of human acute myeloid leukemia using CD4-specific chimeric antigen receptor (CAR) T cells and NK cells. J Cancer (2019) 10(18):4408–19. doi: 10.7150/jca.28952
234. Ureña-Bailén G, Dobrowolski JM, Hou Y, Dirlam A, Roig-Merino A, Schleicher S, et al. Preclinical evaluation of CRISPR-edited CAR-NK-92 cells for off-the-Shelf treatment of AML and b-ALL. Int J Mol Sci (2022) 23(21):12828. doi: 10.3390/ijms232112828
235. Bachier C, Borthakur G, Hosing C, Blum W, Rotta M, Ojeras P, et al. A phase 1 study of NKX101, an allogeneic CAR natural killer (NK) cell therapy, in subjects with relapsed/refractory (R/R) acute myeloid leukemia (AML) or higher-risk myelodysplastic syndrome (MDS). Blood (2020) 136(Supplement 1):42–3. doi: 10.1182/blood-2020-134625
236. Garrison BS, Deng H, Yucel G, Frankel NW, Guzman MA, Gordley R, et al. Precise targeting of AML with first-inClass OR / NOT logic-gated gene circuits in CAR-NK cells. In: Presented at the 24th ASGCT annual meeting, vol. 29. (2021). Available at: https://www.cell.com/action/showPdf?pii=S15250016%2821%2900206-9.
237. Shimasaki N, Jain A, Campana D. NK cells for cancer immunotherapy. Nat Rev Drug Discovery (2020) 19(3):200–18. doi: 10.1038/s41573-019-0052-1
238. Cronk RJ, Zurko J, Shah NN. Bispecific chimeric antigen receptor T cell therapy for b cell malignancies and multiple myeloma. Cancers (Basel) (2020) 12(9):2523. doi: 10.3390/cancers12092523
239. Schmidt P, Raftery MJ, Pecher G. Engineering NK cells for CAR therapy-recent advances in gene transfer methodology. Front Immunol (2021) 11:611163. doi: 10.3389/fimmu.2020.611163
240. Allan DSJ, Chakraborty M, Waller GC, Hochman MJ, Poolcharoen A, Reger RN, et al. Systematic improvements in lentiviral transduction of primary human natural killer cells undergoing ex vivo expansion. Mol Ther Methods Clin Dev (2021) 20:559–71. doi: 10.1016/j.omtm.2021.01.008
241. Mensali N, Dillard P, Hebeisen M, Lorenz S, Theodossiou T, Myhre MR, et al. NK cells specifically TCR-dressed to kill cancer cells. EBioMedicine (2019) 40:106–17. doi: 10.1016/j.ebiom.2019.01.031
242. Davis ZB, Vallera DA, Miller JS, Felices M. Natural killer cells unleashed: checkpoint receptor blockade and BiKE/TriKE utilization in NK-mediated anti-tumor immunotherapy. Semin Immunol (2017) 31:64–75. doi: 10.1016/j.smim.2017.07.011
243. Vallera DA, Felices M, McElmurry R, McCullar V, Zhou X, Schmohl JU, et al. IL15 trispecific killer engagers (TriKE) make natural killer cells specific to CD33+ targets while also inducing persistence, In vivo expansion, and enhanced function. Clin Cancer Res (2016) 22(14):3440–50. doi: 10.1158/1078-0432.CCR-15-2710
244. Reusch U, Burkhardt C, Fucek I, Le Gall F, Le Gall M, Hoffmann K, et al. A novel tetravalent bispecific TandAb (CD30/CD16A) efficiently recruits NK cells for the lysis of CD30+ tumor cells. MAbs (2014) 6(3):728–39. doi: 10.4161/mabs.28591
245. Schmohl JU, Felices M, Todhunter D, Taras E, Miller JS, Vallera DA. Tetraspecific scFv construct provides NK cell mediated ADCC and self-sustaining stimuli via insertion of IL-15 as a cross-linker. Oncotarget (2016) 7(45):73830–44. doi: 10.18632/oncotarget.12073
246. Schmohl JU, Felices M, Oh F, Lenvik AJ, Lebeau AM, Panyam J, et al. Engineering of anti-CD133 trispecific molecule capable of inducing NK expansion and driving antibody-dependent cell-mediated cytotoxicity. Cancer Res Treat (2017) 49(4):1140–52. doi: 10.4143/crt.2016.491
247. Arvindam US, van Hauten PMM, Schirm D, Schaap N, Hobo W, Blazar BR, et al. A trispecific killer engager molecule against CLEC12A effectively induces NK-cell mediated killing of AML cells. Leukemia. (2021) 35(6):1586–96. doi: 10.1038/s41375-020-01065-5
248. Silla LM, Chen J, Zhong RK, Whiteside TL, Ball ED. Potentiation of lysis of leukaemia cells by a bispecific antibody to CD33 and CD16 (Fc gamma RIII) expressed by human natural killer (NK) cells. Br J Haematol (1995) 89(4):712–8. doi: 10.1111/j.1365-2141.1995.tb08406.x
249. Singer H, Kellner C, Lanig H, Aigner M, Stockmeyer B, Oduncu F, et al. Effective elimination of acute myeloid leukemic cells by recombinant bispecific antibody derivatives directed against CD33 and CD16. J Immunother (2010) 33(6):599–608. doi: 10.1097/CJI.0b013e3181dda225
250. Kugler M, Stein C, Kellner C, Mentz K, Saul D, Schwenkert M, et al. A recombinant trispecific single-chain fv derivative directed against CD123 and CD33 mediates effective elimination of acute myeloid leukaemia cells by dual targeting. Br J haematology (2010) 150(5):574–86. doi: 10.1111/j
251. Wiernik A, Foley B, Zhang B, Verneris MR, Warlick E, Gleason MK, et al. Targeting natural killer cells to acute myeloid leukemia In vitro with a CD16 × 33 bispecific killer cell engager and ADAM17 inhibition. Clin Cancer research: an Off J Am Assoc Cancer Res (2013) 19(14):3844–55. doi: 10.1158/1078-0432.CCR-13-0505
252. Gleason MK, Ross JA, Warlick ED, Lund TC, Verneris MR, Wiernik A, et al. CD16xCD33 bispecific killer cell engager (BiKE) activates NK cells against primary MDS and MDSC CD33C targets. Blood (2014) 123:3016–26. doi: 10.1182/blood2013-10-533398
253. Miller JS. NK cell therapeutics: off-the-shelf strategies to increase activity and specificity. In: The innate killer summit 2021. Available at: https://www.gtbiopharma.com/news-media/pressreleases/detail/216/gt-biopharma-announces-gtb-3550-trikemonotherapy.
254. Felices M, Warlick E, Juckett M, Weisdorf D, Vallera D, Miller S, et al. GTB-3550 tri-specific killer engager TriKE™ drives NK cells expansion and cytotoxicity in acute myeloid leukemia (AML) and myelodysplastic syndromes (MDS) patients. J Immunother Cancer (2021) 9:A444.
255. Bryceson YT, Ljunggren HG, Long EO. Minimal requirement for induction of natural cytotoxicity and intersection of activation signals by inhibitory receptors. Blood. (2009) 114(13):2657–66. doi: 10.1182/blood-2009-01-201632
256. Vey N, Bourhis J-H, Dombret H, Bordessoule D, Prébet T, Charbonnier A, et al. A phase I study of the anti-natural killer inhibitory receptor (KIR) monoclonal antibody (1-7F9, IPH2101) in elderly patients with acute myeloid leukemia (AML): clinical and immunological effects of a single dose followed by repeated dosing. Blood. (2009) 114:632. doi: 10.1182/blood.V114.22.632.632
257. Vey N, Bourhis JH, Boissel N, Bordessoule D, Prebet T, Charbonnier A, et al. A phase 1 trial of the anti-inhibitory KIR mAbIPH2101 for AML in complete remission. Blood. (2012) 120:4317–23. doi: 10.1182/blood-2012-06-437558
258. Romagne F, Andre P, Spee P, Zahn S, Anfossi N, Gauthier L, et al. Preclinical characterization of 1-7F9, a novel human anti-KIR receptor therapeutic antibody that augments natural killer-mediated killing of tumor cells. Blood. (2009) 114:2667–77. doi: 10.1182/blood-2009-02-206532
259. Vey N, Dumas P-Y, Recher C, Gastaud L, Lioure B, Bulabois C-E, et al. Randomized phase 2 trial of lirilumab (anti-KIR monoclonal antibody, mAb) as maintenance treatment in elderly patients (pts) with acute myeloid leukemia (AML): results of the effikir trial. Blood. (2017) 130(Suppl. 1):889. doi: 10.1182/blood.V130.Suppl_1.889.889
260. Daver N, Garcia-Manero G, Basu S, Cortes JE, Ravandi F, Jabbour EJ, et al. Phase IB/II study of lirilumab in combination with azacytidine (AZA) in patients (pts) with relapsed acute myeloid leukemia (AML). Blood (2016) 128:1641. doi: 10.1182/blood.V128.22.1641.1641
261. Xu L, Huang Y, Tan L, Yu W, Chen D, Lu C, et al. Increased TIM-3 expression in peripheral NK cells predicts a poorer prognosis and TIM-3 blockade improves NK cell-mediated cytotoxicity in human lung adenocarcinoma. Int Immunopharmacol (2015) 29:635–41. doi: 10.1016/j.intimp.2015.09.017
262. Ngiow SF, von Scheidt B, Akiba H, Yagita H, Teng MW, Smyth MJ. Anti-TIM3 antibody promotes T cell IFN-gamma-mediated antitumor immunity and suppresses established tumors. Cancer Res (2011) 71:3540– 51. doi: 10.1158/0008-5472.CAN-11-0096
263. Wu W, Shi Y, Li S, Zhang Y, Liu Y, Wu Y, et al. Blockade of TIM-3 signaling restores the virus-specific CD8(+) T-cell response in patients with chronic hepatitis b. Eur J Immunol (2012) 42:1180–91. doi: 10.1002/eji.201141852
264. Gallois A, Silva I, Osman I, Bhardwaj N. Reversal of natural killer cell exhaustion by TIM-3 blockade. Oncoimmunology (2014) 3:e946365. doi: 10.4161/21624011.2014.946365
265. da Silva IP, Gallois A, Jimenez-Baranda S, Khan S, Anderson AC, Kuchroo VK, et al. Reversal of NK-cell exhaustion in advanced melanoma by TIM-3 blockade. Cancer Immunol Res (2014) 2:410–22. doi: 10.1158/2326-6066.CIR-13-0171
266. Atfy M, Ebian H, Mostafa S, Atteia HH. CD200 suppresses the natural killer cells and decreased its activity in acute myeloid leukemia patients. J Leukemia (2015) 3:190. doi: 10.4172/2329-6917.1000190
Keywords: natural killer cells, immunotherapy, AML, CAR-NK, BiKEs, TriKEs
Citation: D’Silva SZ, Singh M and Pinto AS (2023) NK cell defects: implication in acute myeloid leukemia. Front. Immunol. 14:1112059. doi: 10.3389/fimmu.2023.1112059
Received: 30 November 2022; Accepted: 25 April 2023;
Published: 09 May 2023.
Edited by:
Shanmuganathan Chandrakasan, Emory University, United StatesReviewed by:
Jeffrey J. Bednarski, Washington University in St. Louis, United StatesHila Shaim, University of Texas Medical Branch at Galveston, United States
Copyright © 2023 D’Silva, Singh and Pinto. This is an open-access article distributed under the terms of the Creative Commons Attribution License (CC BY). The use, distribution or reproduction in other forums is permitted, provided the original author(s) and the copyright owner(s) are credited and that the original publication in this journal is cited, in accordance with accepted academic practice. No use, distribution or reproduction is permitted which does not comply with these terms.
*Correspondence: Selma Z. D’Silva, c2VsbWFkc2lsdmFAZ21haWwuY29t; c2RzaWx2YUBhY3RyZWMuZ292Lmlu