- 1Center for Vascularized Composite Allotransplantation, Chang Gung Memorial Hospital at Linkou, Taoyuan, Taiwan
- 2Department of Plastic and Reconstructive Surgery, Chang Gung Memorial Hospital at Linkou, Taoyuan, Taiwan
- 3School of Medicine, Chang Gung University, Taoyuan, Taiwan
Solid organ and composite tissue allotransplanation have been widely applied to treat end-stage organ failure and massive tissue defects, respectively. Currently there are a lot of research endeavors focusing on induction of transplantation tolerance, to relieve the burden derived from long-term immunosuppressant uptake. The mesenchymal stromal cells (MSCs) have been demonstrated with potent immunomodulatory capacities and applied as promising cellular therapeutics to promote allograft survival and induce tolerance. As a rich source of adult MSCs, adipose tissue provides additional advantages of easy accessibility and good safety profile. In recent years, the stromal vascular fraction (SVF) isolated from adipose tissues following enzymatic or mechanical processing without in vitro culture and expansion has demonstrated immunomodulatory and proangiogenic properties. Furthermore, the secretome of AD-MSCs has been utilized in transplantation field as a potential “cell-free” therapeutics. This article reviews recent studies that employ these adipose-derived therapeutics, including AD-MSCs, SVF, and secretome, in various aspects of organ and tissue allotransplantation. Most reports validate their efficacies in prolonging allograft survival. Specifically, the SVF and secretome have performed well for graft preservation and pretreatment, potentially through their proangiogenic and antioxidative capacities. In contrast, AD-MSCs were suitable for peri-transplantation immunosuppression. The proper combination of AD-MSCs, lymphodepletion and conventional immunosuppressants could consistently induce donor-specific tolerance to vascularized composite allotransplants (VCA). For each type of transplantation, optimizing the choice of therapeutics, timing, dose, and frequency of administration may be required. Future progress in the application of adipose-derived therapeutics to induce transplantation tolerance will be further benefited by continued research into their mechanisms of action and the development of standardized protocols for isolation methodologies, cell culture, and efficacy evaluation.
1 Introduction
Organ and tissue transplantation has emerged as a highly effective approach for patients with terminal illness or severe tissue defects; however, rejection caused by the immunological barrier between the donor and the recipient remains a significant problem. In most cases, this can be prevented or treated using combinations of immunosuppressants. However, long-term intake of these medications can potentially cause serious side effects such as organ failure due to drug toxicities and malignancies. A tremendous amount of research effort has focused on strategies to induce transplantation tolerance, which would enable transplant recipients to be free of the burden of immunosuppressants. To this end, therapy with cells exhibiting immunomodulatory properties, such as mesenchymal stromal cells (MSCs), has drawn considerable interest. Clinical studies have demonstrated the safety and feasibility of MSC therapy in transplantation (1). In kidney transplant patients, infused MSCs have allowed for a reduction in the dosage of the immunosuppressant tacrolimus (2, 3). In some living-donor kidney transplant patients, MSC therapy has been shown to facilitate the induction of transplantation tolerance (4, 5).
Although MSCs can be found in essentially all types of tissues, adipose tissue has been demonstrated to be a particularly good source of MSCs (6). The abundance of MSCs is approximately 500 times higher in adipose tissue than in bone marrow (BM) (7). Furthermore, adipose tissue is easily accessible due to its subcutaneous location and relatively rich volume, which are additional advantages of using it as a source of MSCs (8). In addition to adipose tissue-derived MSCs (AD-MSCs), stromal vascular fraction (SVF) and secretome have also been collected for use as therapeutics in various disease models, including transplantation. In this article, we present recent studies that have employed adipose-derived therapeutics in organ/tissue allotransplantation. The aim of this article is to stimulate interest in utilizing these therapeutic reagents in allotransplantation to promote allotransplant survival and explore their potential to achieve a consistent induction of tolerance.
2 Adipose tissue-derived therapeutics
In this article, we will discuss SVF, AD-MSCs, and secretome. Figure 1 illustrates the isolation of these three types of therapeutics from adipose tissue.
2.1 SVF
SVF is obtained by excluding mature adipocytes from adipose tissue through enzymatic digestion or mechanical disruption. It is characterized by multiple cell populations and generally includes 15–30% stromal cells (AD-MSCs), 10–20% endothelial cells (ECs) and endothelial progenitor cells (EPCs), 3–5% pericytes, and 25–45% hematopoietic cells (9, 10). Notably, SVF contains many regulatory-type leukocytes such as regulatory T cells (Tregs), regulatory B cells (Bregs), invariant natural killer (NK) T cells, and M2 macrophages (11–13). SVF has been shown to exhibit pro-angiogenic, anti-apoptotic, anti-inflammatory, and immunomodulatory capacities (14). Studies using a mouse hindlimb ischemia model have shown that SVF promotes wound healing (15) and vasculogenesis (16). SVF has also been demonstrated to ameliorate tubular injury in an acute kidney injury model by facilitating the proliferation of renal tubular epithelial cells (17). Andia et al. conducted a literature review and found that between 2010 and 2019, 73 clinical studies applied SVF in various contexts, such as arthritis, wound healing, erectile dysfunction, and cardiovascular and pulmonary diseases. The therapeutic efficacy of SVF has been demonstrated in many of these studies (18).
The therapeutic function of SVF can be derived from a specific cell population (such as AD-MSCs), or from crosstalk between SVF components. For example, reduced expression of costimulatory molecules and increased expression of transforming growth factor β (TGF-β) and interleukin-10 (IL-10) by M2 macrophages and AD-MSCs, respectively, enhanced the generation of Tregs, which then support the maintenance of M2 macrophages in SVF (19). The mutual paracrine interaction between AD-MSCs and EPCs, as well as the physical association between AD-MSCs and tubular structures formed by ECs, has been shown to contribute to the pro-angiogenic properties of SVF (20).
SVF has attracted considerable interest for clinical applications because it can be prepared at the point-of-care using commercially available devices which may be fully- or semi-automated, with established protocols (21, 22). SVF can be prepared within a few hours, making it a feasible option for emergencies (23). Other advantages include less manipulation and a lower risk of contamination (23). The therapeutic efficacy of SVF is greatly affected by the host condition due to the presence of multiple cell types and the lack of a culture or selection process between isolation and administration. For example, the reduction of vascular progenitor cells in adipose tissue with age leads to a decline in angiogenic potential and the capacity to establish a mature microcirculation of the isolated SVF (24, 25). Moreover, obesity results in increases in proinflammatory macrophages and CD8+ T cells, as well as a decrease in Tregs in adipose tissue, which subsequently affects SVF composition (26, 27). The preparation methods also influence the composition of SVF, with the percentage of AD-MSCs varying from 5% to 26% between mechanical and enzymatical preparation (28). The multiple-cell nature of SVF makes it potentially immunogenic, which is why autologous SVF has been preferred in most studies (29).
2.2 AD-MSCs
MSCs are heterogeneous progenitor cells with self-renewal and multilineage differentiation capacities (30). The criteria established by the International Society for Cell and Gene Therapy to define MSCs include plastic adherence; positive expression of CD73, CD90, and CD105; negative expression of human leukocyte antigen DR, hematopoietic and endothelial markers; and in vitro differentiation capacities into adipocyte, chondrocyte, and osteoblast lineages (31). By culturing SVF in an appropriate medium and onto culture dishes, AD-MSCs within the SVF can adhere, grow, and expand on the surface. Although AD-MSCs are less heterogeneous and better characterized than SVF, they require more time to culture and expand to reach substantial quantities. Cultured AD-MSCs are subject to risks of contamination, senescence (32, 33), and phenotypic changes, such as gradual disappearance of CD34 expression during in vitro culture (34, 35). The properties of AD-MSCs are also affected by cell culture parameters such as seeding density, growth media supplements such as fetal bovine serum, and environmental oxygen concentration (36). Therefore, the specific properties of MSCs can be enhanced by manipulating the culture conditions. For example, the addition of proinflammatory cytokines or reduction of environmental oxygen levels can enhance the immunomodulatory efficacy of AD-MSCs (37–39).
Numerous studies have demonstrated that AD-MSCs exhibit immunomodulatory functions. They are capable of suppressing the proliferation of effector T and B cells and impairing NK cell function and dendritic cell (DC) maturation, while enhancing the generation of Tregs, Bregs, and M2 macrophages (40–42). These actions are mainly mediated by soluble factors secreted by MSCs such as prostaglandin E2, TGF-β, IL-10, and indoleamine-2, 3-dioxygenase (IDO). Other secreted factors, including vascular endothelial growth factor (VEGF), hepatocyte growth factor (HGF), and angiopoietin-1, participate in angiogenesis and tissue repair. MSCs employ another mechanism to exert their function by transferring mitochondria to other cells. The transfer occurs through direct cell-cell contact, where healthy and functional mitochondria are transferred by MSCs to cells that need additional energy production. Do et al. reported that Tregs maintained robust FoxP3 expression and suppressive function even under proinflammatory conditions when they received mitochondria transferred from MSCs (43). Moreover, damaged cells can utilize the transferred mitochondria from MSCs to produce more energy and facilitate repair processes (44).
AD-MSCs are generally considered to have low immunogenicity due to the absence of costimulatory and major histocompatibility complex (MHC) class II molecules (45), and low level of MHC class I molecules (46). However, evidence has shown that MHC class I molecules play a role in evading NK cell-mediated cytotoxicity and thus the survival of MSCs in an allogeneic setting (47). Therefore, both autologous and allogeneic AD-MSCs can be used as therapeutic agents. These cells can be prepared in advance with careful characterization and preserved under appropriate conditions for later use. Furthermore, allogeneic AD-MSCs can be manufactured on a large scale, making them a readily available treatment option for various clinical conditions (40). To reduce donor-to-donor variations, pooled MSCs from several donors were used in a clinical trial for pediatric graft-versus-host disease, demonstrating superior therapeutic efficacy compared to MSCs from individual donors (48). Such practices may help to reduce the cost of MSC therapy and facilitate its clinical application.
2.3 Secretome
As mentioned previously, MSCs exert various functions through secreting paracrine factors, collectively known as the “secretome” (49). Apart from cultured MSCs, the secretome can also be collected from the adipose tissue, and its therapeutic efficacy has been demonstrated (50, 51). The secretome mainly consists of soluble proteins such as growth factors and cytokines, lipid, free nucleic acids, and extracellular vesicles (EV) (52). The multitude of biologically active factors and the potential to serve as “cell-free” therapeutics, which may offer a better safety profile and lower immunogenicity, have drawn significant research interest in the secretome, especially EVs (53).
EVs refer to particles naturally released from cells and contain lipid bilayers but lack functional nuclei (54). Soluble factors secreted by AD-MSCs can be encapsulated as cargo and transported by EVs, which release the cargo through endocytosis, membrane fusion, or specific molecular recognition when they reach target cells (55). The cargo content varies depending on the cell origin and culture conditions. For instance, Shin et al. performed a proteomic analysis and identified 265, 253, and over 400 proteins in EVs from AD-MSCs, BM-derived MSCs (BM-MSCs), and fetal (placenta and Wharton’s jelly) MSCs, respectively. Of these proteins, 181 were common among EVs of all origins (56). Systemic literature searches have characterized 591 proteins, 604 miRNAs, and 84 mRNA species from AD-MSC-derived EVs, indicating the richness of the biological information carried by EVs (57). Additionally, the content and function of EVs can be regulated by manipulating the culture conditions of AD-MSCs. Treatment with proinflammatory interferon gamma (IFN-γ) and tumor necrosis factor α (TNF-α) can alter the miRNA profiles in EVs, which can shift macrophages from M1 to M2 phenotype (39). Hypoxia-conditioned MSCs secrete EVs with potentiated immunosuppressive capabilities (58). EVs derived from human AD-MSCs have been shown to promote the proliferation and migration of cultured ECs, neovascularization, re-epithelialization, and wound closure when applied in a murine skin injury model (59). Additional in vivo studies have demonstrated that EVs are capable of enhancing nerve regeneration and offering liver and cardiac protection (60–62). Purification of EVs from physiological fluids or conditioned cell culture medium can be achieved using various methods such as ultracentrifugation, size-exclusion chromatography, ultrafiltration, immunoaffinity capture, and polymer precipitation (63). However, acquiring EVs with high yield and consistent biological activities remains challenging. New technologies, such as microfluidics, are being developed to overcome this impediment (64, 65).
The conditioned medium (CM) is another form of AD-MSC secretome that has been demonstrated to have therapeutic efficacy in many reports (49). For instance, CM of human AD-MSC has been shown to promote mouse liver regeneration (66) and facilitate wound healing in a murine skin injury model (67). To partially purify the CM, fractionation techniques such as ultrafiltration can be used to concentrate the bioactive factors (65, 66).
3 Roles of adipose tissue-derived therapeutics in allotransplantation
The process of organ/tissue allotransplantation can be divided into several stages, as illustrated in Figure 2. Allotransplants are inevitably subjected to ischemia–reperfusion injury (IRI) caused by hypoxemia and hypoperfusion during graft procurement, as well as oxidative stress and inflammatory events after revascularization in the recipients, before performing their physiological functions in the recipients after transplantation (68). After the transplantation surgery, managing the immune response induced by allogenicity between the donor and the recipient, such as treating rejection and inducting tolerance, is crucial for the success of allotransplantation. In this context, we discuss the current progress in adipose-derived therapeutics that can facilitate successful allotransplantation at different stages.
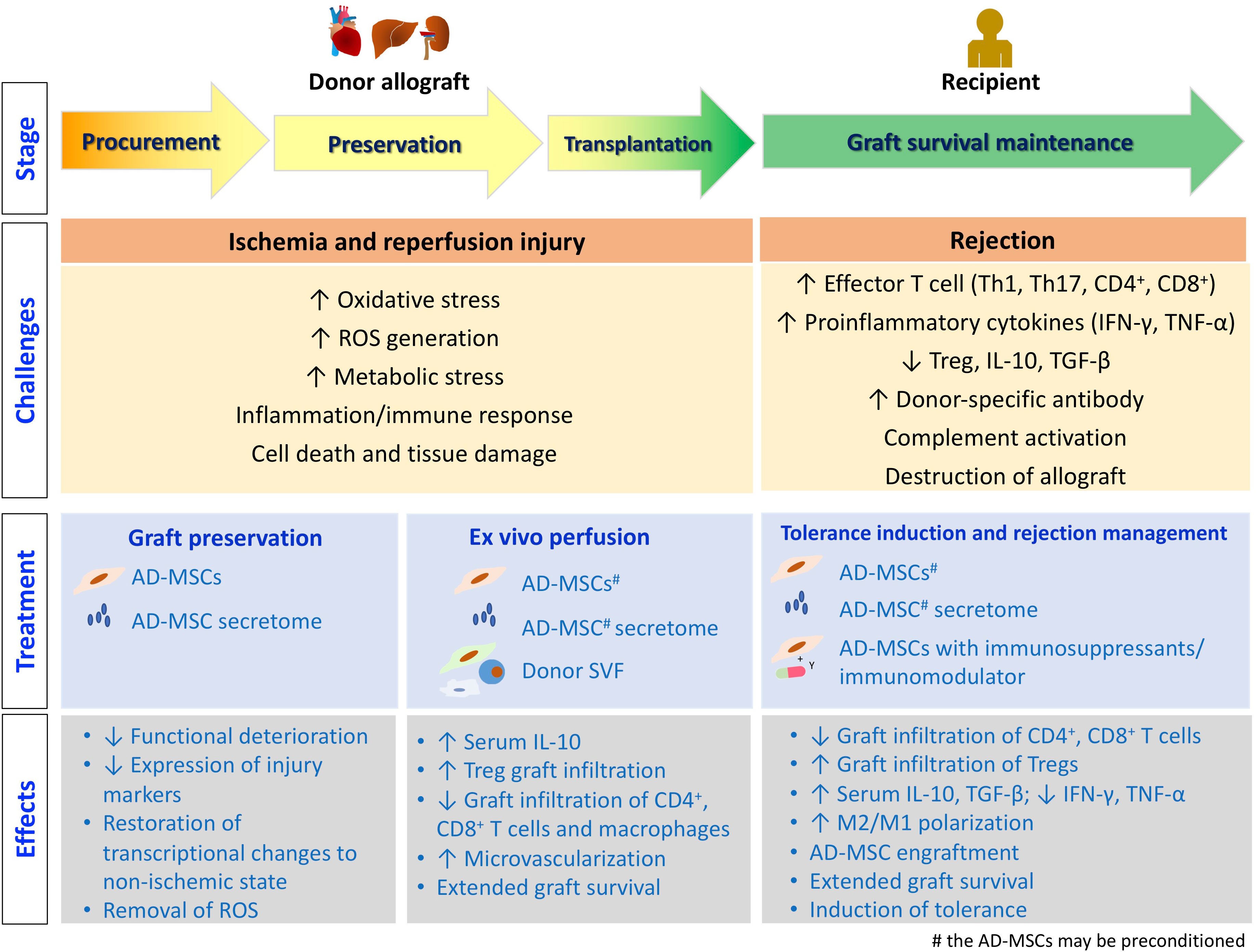
Figure 2 The stages of allotransplantation. The challenges encountered at each stage, along with the treatment strategies employing adipose tissue-derived therapeutics, are depicted. Additionally, the underlying molecular and cellular events are illustrated.
3.1 On the allotransplants
3.1.1 Allotransplant preservation
IRI leads to the release of damage-associated molecular patterns, and facilitates leukocyte migration into the graft, complement activation, and allograft destruction. The intensity and duration of IRI are correlated with graft dysfunction and rejection after transplantation. Therefore, effective management of IRI may promote allograft survival and suppress rejection (69).
After procuring the allografts from the donor, they are perfused and stored in various preservation solutions to decrease their cellular metabolism and oxygen consumption (70). The preservation mode has evolved from a statically cold solution to normothermical machine perfusion (NMP), which allows better penetration of the solution, delivery of oxygen and nutrients, removal of toxic metabolites, and maintenance of normal cellular metabolism (71). However, tissue deterioration still occurs during organ preservation and transportation, which may prevent the procured grafts from being used. The tissue repair capacity of MSCs has been explored in this context. Kasahara et al. fractionated the AD-MSC CM and reported that addition of >50 kD or 10-30 kD fractions to the preservation solution of isolated islets during 24 hours of cold preservation can increase ATP production and restore the insulin-producing capabilities of the islets in vitro (72). When streptozotocin-induced diabetic mice were transplanted with islets that were previously preserved in the presence of these two AD-MSC secretome fractions, the recipients exhibited better glycemic control (73). Ellis et al. reported that functional deterioration of murine heart allografts was mitigated when AD-MSC secretome was added to the cold preservation solution (65). Wang et al. showed that miR-199-3p in the heart allograft was depleted during cold preservation, and the level can be recovered when the AD-MSC-derived EVs in the preservation solution transferred miR-199-3p to the graft (74). Ablation of superoxide dismutase 3 and/or catalase expression in AD-MSCs with siRNA attenuated the protective effects of AD-MSC secretome, suggesting that the removal of reactive oxygen species (ROS) generated during preservation is critical. Gene expression profiling studies showed that AD-MSC secretome partially restored the transcriptional changes induced by cold preservation, including mitochondrial dysfunction, apoptosis, and oxidative stress, to the non-ischemic (normal) state (75).
When kidney grafts underwent 2-3 hours of oxygenated hypotheric machine perfusion (HMP) followed by 7 hours of NMP, the addition of AD-MSCs during NMP suppressed the expression of injury markers such as neutrophil gelatinase-associated lipocalin and N-acetyl-β-d-glucosaminidase, while enhancing the expression of cytokines IL-8 and IL-6, which have proangiogenic and immunomodulatory effects (76). Subsequently, the authors tested another preservation protocol of 14 hours of oxygenated HMP followed by 4 hours of NMP for the kidney grafts and performed transplantation. Although approximately 5% of AD-MSCs were retained in the graft 2 weeks after transplantation, they had no effects on perfusion dynamics and did not improve graft function or survival (77). The authors suggested to optimize the dose and timing of AD-MSC administration, but the varied duration of HMP (2-3 hours versus 14 hours) and NMP (7 hours versus 4 hours) may have different effects on the graft and should be considered.
3.1.2 Allotransplant pretreatment (ex vivo perfusion)
AD-MSCs and their secretome have been applied directly to allotransplants to mitigate injuries during procurement and storage before reperfusion is established in recipients. For example, when AD-MSCs were infused into the hindlimb allograft through the artery after procurement, allograft survival after transplantation was significantly prolonged from 15.5 days to 26 days (78). Hypoxia-preconditioned AD-MSCs had more pronounced effects and further extended graft survival to 32 days. Recipients of AD-MSC-infused allograft exhibited higher serum levels of IL-10 and increased infiltration of Tregs into the grafts than those without MSC infusion (78). Kato et al. found that kidney graft survival was significantly prolonged when AD-MSCs were administered intra-arterially to the kidneys before procurement. After transplantation, the AD-MSC-treated kidney grafts showed approximately a 50% reduction in CD4+ and CD8+ T cell infiltration, in addition to 3-fold induction of the anti-inflammatory factor TNF-stimulated gene 6. The infused AD-MSCs were identified in the graft glomeruli from postoperative day (POD) 1 to 3 and disappeared on POD 5 (79). However, the intra-arterial infusion of AD-MSCs into the kidney graft after warm ischemia and 16 hours of static cold storage did not mitigate the deterioration of the ischemia-induced glomerular infiltration rate or tissue fibrosis-related gene expression (80).
The mode of ex vivo infusion of AD-MSCs affects the outcome of allotransplantation. AD-MSC infusion into the lumen and subserosal of the small intestine allograft before reperfusion helped reduce the occurrence of acute rejection and significantly prolonged recipient survival, along with elevation of blood Tregs and TGF-β levels (81). However, intra-arterial infusion of AD-MSCs had no effect on the rejection of small intestine allografts (82). In a skin graft model, subcutaneous injection of AD-MSC-derived EVs into a graft that experienced 6 hours of ischemia before reperfusion reduced the inflammatory response and apoptosis. The area of blood perfusion and microvascular density measured 5 days after surgery were significantly improved with AD-MSC-EV administration. The beneficial effects on graft survival and neovascularization were significantly enhanced when the flap was treated with EVs derived from the H2O2-pretreated AD-MSCs (83).
Although ex vivo perfusion appears promising, caution should be exercised when administering high cell doses. Munk et al. reported that intra-arterial infusion of 107 AD-MSCs was well tolerated during porcine renal transplantation, whereas the infusion of 108 AD-MSCs caused serious adverse effects, including reduced renal perfusion and serious inflammatory reactions (84).
In addition to AD-MSCs, donor SVF was administered intra-arterially before reperfusion in a rat donation after circulatory death (DCD) kidney allotransplantation model. The recipients showed alleviated IRI and attenuated acute rejection. Enhanced microvascularization, elevated IDO expression and increased Treg infiltration were identified in the grafts. In contrast, reduced infiltration of CD8+ T cells and macrophages was observed (23, 85). Chen et al. compared the allografts infused with AD-MSCs and SVF prior to transplantation and reported that the SVF group had a significantly longer survival time than the AD-MSC group (35 versus 25.7 days), along with higher IL-10 and TGF-β levels and lower TNF-α levels in serum. Moreover, the SVF-infused graft had a higher density of CD31+ cells and vessels, suggesting that the pro-angiogenic effect of SVF is advantageous for graft survival (86).
In summary, current data have demonstrated that applying adipose-derived therapeutics to allografts can potentially promote graft function and survival after transplantation. Adding AD-MSC secretome to a cold preservation solution has been shown to improve graft function after transplantation (65, 72, 73). However, the benefits may be limited if the grafts undergo prolonged cold preservation before the application of AD-MSCs in NMP (77, 80). For ex vivo perfusion, SVF appears to be more effective than AD-MSCs, potentially due to its pro-angiogenic capacity (86). The choice of therapeutics, dose, timing, and application mode may need to be optimized based on the type of allotransplantation.
3.2 On the recipients
3.2.1 Peri-transplantation immunosuppression/tolerance induction
The well-documented immunomodulatory capacities of MSCs have led to their use in many applications during the peri-transplantation period to mitigate the alloimmune responses elicited by the interaction between donor antigens and the recipient immune system after allotransplantation. Adipose-derived therapeutics have gradually gained recognition in this field. For example, among the preclinical studies summarized in Table 1, approximately one-third were published after 2020, including three with AD-MSC-derived secretome (91, 97, 115). As each study applied different variables, we group them into three subsections (3.2.1.1 to 3.2.1.3) for discussion.
3.2.1.1 Single treatment of AD-MSC or secretome
AD-MSCs alone have been shown to slightly prolong the survival of transplanted kidney, skin, intestine, and hindlimb allografts (97, 100, 103, 104, 106, 108). The associated mechanisms included AD-MSC engraftment; increased circulatory levels of IL-10, TGF-β, and Tregs; suppressed T helper 1 (Th1) and T helper 17 (Th17) cells; decreased circulatory levels of TNF-α, IFN-γ, and IL-6; and reduced graft infiltration of lymphocytes. Some studies have reported benefits of improving graft function with AD-MSC administration (88, 98, 101). Most studies employed systemic administration through the intravenous or intraperitoneal route. However, direct infusion of AD-MSCs through the portal vein after transplantation of a liver graft procured from a DCD donor and in cold static storage for 4 hours rescued the liver from primary graft nonfunction and prolonged graft survival from less than 24 hours (no AD-MSC infusion) to more than 7 days (100). Intraportal administration of AD-MSCs also extended graft survival from 13 days to 26 days in a small-for-size liver transplantation model (99). Advantage of the proximity of AD-MSCs with the allograft was corroborated when AD-MSCs were transplanted together with the islet grafts and led to a significant extension of transplanted islet survival from 1.2 days to 13.6 days. However, no benefits were observed when the AD-MSCs were implanted on the contralateral side to the islet graft (95).
Lee et al. compared the effects of AD-MSCs with those of concentrated AD-MSC CM and found that concentrated CM was less effective than AD-MSCs in prolonging graft survival when administered after surgery but transiently induced VEGF expression, which implies a proangiogenic effect (103). Ramirez-Bajo et al. reported that AD-MSC-derived EVs did not provide benefits in prolonging graft survival in a heterotopic kidney transplantation model (97).
Notably, Yip et al. adopted a combinatorial approach in a heart transplantation model. They infused AD-MSCs into the donor 3 hours after induced brain death. Following transplantation, AD-MSCs were administered to the recipients on POD 1 and 3. The recipients showed fewer circulatory proinflammatory Ly6G+ cells and more Tregs. A lower degree of DNA damage and less infiltration of CD4+ T cells, DCs, and macrophages were observed in the heart allograft. The effects were less pronounced when cells were administered to either the donor or the recipient alone (88).
3.2.1.2 Treatment with preconditioned AD-MSCs
The immunomodulatory effects of MSCs can be enhanced by preconditioning before their in vivo application (119, 120). Two approaches to preconditioning are typically used. First, MSCs can be cultured under modified conditions, such as exposure to reagents like IFN-γ and TNF-α, or hypoxic environments. Second, specific protein expression level can be adjusted by gene transfection of MSCs (37). Because Toll-like receptor 3 (TLR3) activation has been shown to enhance the anti-inflammatory capacity of MSCs, Bao et al. preincubated AD-MSCs with the TLR3 agonist poly(I:C) before infusion into heart transplant recipients. The poly(I:C)-pretreated AD-MSCs expressed and secreted higher levels of fibrinogen-like protein 2 (Fgl2), a Treg-secreted factor. Transplant survival was 10.2 and 12.3 days when the recipients were infused with AD-MSCs and poly(I:C)-pretreated AD-MSCs, respectively. The extended survival was associated with reduced lymphocyte infiltration into the graft and an increased percentage of splenic Tregs (94). Fgl2 has been shown to potentiate M2 macrophage polarization. AD-MSCs overexpressing soluble Fgl2 can migrate to the transplant site and induce a higher intragraft M2/M1 ratio, resulting in significantly prolonged graft survival (89). When overexpressing another Treg effector, IL-35, the administered AD-MSCs resulted in lower splenic Th17 levels and Th1/Th2 ratio, in addition to prolonging the graft survival from 6.2 days to 17.5 days, which was extended further to 24 days when the administration frequency was increased to 5 doses (90, 91). EVs from IL-35-transfected cells also prolonged the graft survival, which was only partially blocked by the IL-35 neutralizing antibody, suggesting the involvement of other factors in EVs. The capacity of AD-MSCs to suppress T cell proliferation was potentiated by overexpressing another costimulatory molecule, OX40Ig. When the OX40Ig-transfected AD-MSCs were administered to the recipients, graft survival was extended, along with reduced tissue damage in the transplanted kidney (96). AD-MSCs transfected with secondary lymphoid organ (SLO)-targeting CCR7 migrated to the SLO following in vivo administration to skin flap recipients. Transplant survival was prolonged in association with lower Th1/Th2 and Th17/Treg ratios within the spleen and lymph nodes (107).
3.2.1.3 Effects of supplementary immunomodulators and immunosuppressants
The immunomodulatory effects of wild-type AD-MSCs can be potentiated by co-administration of immunomodulatory melatonin (87) or immunosuppressants (121). Obermajer et al. reported that AD-MSCs only prolonged graft survival when co-administered with the immunosuppressant mycophenolate mofetil (MMF) in a murine heart transplantation model. The authors found that the administered AD-MSCs induced Th17 generation, and the presence of MMF induced the conversion of Th17 to Tregs (92). These effects were abolished when AD-MSCs underwent heat inactivation, suggesting the requirement of intact cells (93). Interactions between MSCs and common immunosuppressants have been demonstrated in many studies. For example, cyclosporin A (CsA) has been shown to promote the immunomodulatory function and survival of MSCs (122). Conversely, MSCs potentiate the suppression of Th17 and Th1 by immunosuppressants (123). In a lung transplantation model, supplementary FK506 to AD-MSCs significantly ameliorated the severity of rejection and increased the production of hepatocyte growth factor (HGF) (101). Several studies have demonstrated the successful induction of donor-specific tolerance (111–113, 116, 117) or long-term graft survival (>120 days) (109, 110, 118) in vascularized composite allotransplantation (VCA) models, including transplantation of hindlimb and hindlimb osteomyocutaneous (HLOMC) grafts, with AD-MSC infusion supplemented with short-term (10–28 days) application of immunosuppressants and lymphodepletion reagents. The associated molecular/cellular changes included reduction in blood CD8+ cells and graft C4d deposits, and increased peripheral/spleen/graft Tregs (114). Serum levels of proinflammatory cytokines (TNF-α and IFN-γ) were decreased, whereas those of anti-inflammatory TGF-β and IL-10 were elevated in AD-MSC-infused VCA recipients (107, 109). Chen et al. reported that AD-MSC-derived exosomes extended hindlimb allograft survival in association with significantly higher levels of donor chimerism, Treg and type I regulatory T cells (115). Compared to the study by Plock et al., in which similar immunosuppression protocol was applied, the hindlimb allograft survival time was 92 and 58 days when the recipients were infused with intact AD-MSCs and AD-MSC-exosomes, respectively (112, 115). Although not a direct comparison, it appears that intact AD-MSCs perform better than AD-MSC-derived secretome in modulating the recipient immune response.
3.2.1.4 Application of AD-MSCs for induction of clinical tolerance
In contrast to preclinical studies, the clinical application of AD-MSCs in organ/tissue allotransplantation is significantly lower, and available reports are from a single group. Vanikar et al. designed a protocol to infuse AD-MSCs, hematopoietic stem cells (HSC), peripheral blood stem cells (PBSCs), and donor-specific transfusion through portal circulation prior to living-donor kidney transplantation (LDRT) in recipients who were preconditioned with cyclophosphamide, total lymphoid irradiation, and rabbit anti-thymocyte globulin (rATG). They compared two groups of patients whose treatment differed only in the presence of AD-MSCs and showed that the group with AD-MSCs had better graft survival, lower serum creatine level, and fewer rejection episodes. In a group of 95 patients, 3 were weaned off immunosuppressants over the course of 7 years (4, 124). In a large-scale trial with 916 patients treated with the same protocol, pre-transplant infusion of stem cells resulted in a reduction of the triple-immunosuppressant regimen to two drugs in 71% (433/606) of patients or one drug in 3.9% (24/606) of patients (125). In a smaller group of patients who received AD-MSC/HSC/PBSC co-infusion 14 days prior to LDRT, followed by treatment with bortezomib, methylprednisolone, rATG, and rituximab between transplantation and POD 14, the authors reported that 5 of 10 patients did not require any conventional immunosuppressants and can be maintained with a low dose of prednisone (5mg/day) during the 6-year follow-up period (126). This protocol circumvented the use of conventional calcineurin inhibitors and promoted patient/graft survival by reducing the susceptibility to infection (126). A large-scale trial with different populations is necessary to further evaluate the efficacy of this protocol.
3.2.2 Treatment of rejection
Clinically, rescuing the allotransplants from destruction when rejection occurs is a critical issue in transplantation. Although AD-MSCs have not been extensively explored for this purpose and no published reports are available, at least two clinical trials are currently underway to examine the efficacy of AD-MSCs in treating the rejection of lung and kidney transplants (NCT04714801 and NCT05456243, respectively). In contrast, completed clinical studies using BM-MSCs on chronic lung allograft rejection (127) or chronic antibody-mediated rejection of kidney transplants (128, 129) have demonstrated the safety, feasibility, and efficacy of MSC therapy for rejection. Additionally, umbilical cord MSCs have been shown to alleviate liver transplant damage during rejection (130). Given the promising results of BM-MSCs and umbilical cord MSCs for treating rejection, AD-MSCs have great potential to serve as an effective therapeutic option to rescue allotransplant rejection. However, additional investigation and validation are warranted.
4 Discussion
The adipose-derived therapeutics described in this article, namely SVFs, AD-MSCs, and secretome, have distinct advantages and disadvantages, as summarized in Table 2. Although SVFs are easy to isolate, their composition can vary depending on the host’s condition and the preparation methods. The efficacy of SVFs in allograft pretreatment has been demonstrated (85, 86). Secretome also performed well in allograft preservation and pretreatment (65, 72–75, 83). However, currently available data indicate that AD-MSCs are more effective than their secretome in managing the alloimmune response post-transplantation (97, 112, 115). Several studies have demonstrated the presence of AD-MSCs in the graft after systemic administration (100, 101, 103, 118), suggesting that the cellular responses elicited by AD-MSCs when encountering donor cells are beneficial for maintaining allograft survival in recipients. The timing and frequency of administration are also critical parameters. Plock et al. found that the recipient groups that were infused with AD-MSCs on POD 1 and 4 developed tolerance and rejected the graft by POD 60, respectively (112). As the recipients were treated with preconditioning reagents and immunosuppressants, the AD-MSCs may have encountered different states of the recipient immune system when they were infused at different timing. Furthermore, these reagents may influence MSC survival and competency. Notably, the group that received multiple cell administrations on POD 4, 8, and 15 developed transplantation tolerance, associated with a higher level of donor chimerism and sustained elevation of Tregs. Therefore, the interactions among the AD-MSCs, donor antigens, and recipient cells led to the cellular and molecular events that are crucial for determining the allograft outcome.
Based on the available literature, it is clear that administration of adipose-derived therapeutics alone is insufficient to induce transplantation tolerance and wean off immunosuppression usage for the recipients. However, transplantation tolerance has been induced in VCA models through the use of AD-MSCs along with preconditioning and short-term immunosuppression (131). A key characteristic of VCA is the inclusion of vascularized donor BM (VBM), which allows for the instant engraftment of donor bone marrow cells (BMCs) in a natural stromal-supporting microenvironment within recipients (132, 133). In allotransplantation, donor BMCs help establish transient or sustained chimerism, which may evoke central or peripheral clonal deletion and facilitate the induction of transplantation tolerance (134). Davis et al. successfully induced tolerance in a murine skin graft model by combining donor BMCs and AD-MSCs with CD4 and CD8 antibodies, as well as the antineoplastic reagent busulfan (105). The recipients showed sustained chimerism in the spleen and BM, and clonal deletion was evidient. Therefore, it is worth exploring a similar strategy that involves a combination of AD-MSCs, VBM/BMCs, lymphodepleting preconditioning, and short-term immunosuppression to induce tolerance in other types of allotransplants.
The effects of each therapeutic varied among studies. For example, donor-derived AD-MSCs performed worse than the recipient-derived cells in terms of kidney graft survival, but better in terms of skin graft survival (97, 102). Similarly, EVs were shown to prolong skin graft survival, but did not affect kidney graft survival (97, 103). Some of the observed variation may be due to inconsistent properties derived from variations in AD-MSC culture conditions or secretome collection. Furthermore, the efficacy of AD-MSCs and EVs may be improved by modifying the cell culture conditions. For instance, the preconditioning strategies mentioned earlier and three-dimensional (3D) spheroid cultures are worth further exploration. Previous studies reported that the 3D spheroids contain aggregates of cells and an intact extracellular matrix, closely simulating a natural environment compared to two-dimensional cultures. MSCs cultured in 3D have been shown with enhanced anti-inflammatory capacity (135, 136). EVs derived from 3D MSC cultures were also demonstrated to have better therapeutic efficacy than those derived from 2D cultures (137).
Overall, the use of SVFs, AD-MSCs, and EVs shows promise as an alternative to the lifelong intake of traditional immunosuppressive drugs. However, optimization of parameters such as administration timing, dose, and frequency is essential to maximize their benefits for each type of transplantation. Therefore, appropriate therapeutic agents should be selected for different purposes. For example, a combined strategy of applying SVF or secretome to pretreat allografts before transplantation to mitigate IRI, followed by utilizing the immunomodulatory function of AD-MSCs in the recipients, may be a feasible approach to improving transplantation survival. Future progress in the application of adipose-derived therapeutics in transplantation settings will be facilitated by continuing research into their mechanisms of action and the development of standardized protocols for isolation methodologies, AD-MSC culture, and efficacy evaluation.
5 Conclusion
Herein, we present recent studies that utilized the adipose-derived therapeutics including SVFs, AD-MSCs, and secretome to improve graft survival and induce transplantation tolerance in organ and tissue transplantation. These studies demonstrated their efficacy at different stages of allotransplantation. Although most of the evidence come from preclinical models, further standardization of techniques will help to allow a wider application of adipose-derived therapeutics for the induction of transplantation tolerance in clinical practice.
Author contributions
H-YC conceived the study. H-YC and MRA wrote the manuscript. C-HL and F-CW reviewed and revised the manuscript. All authors contributed to the article and approved the submitted version.
Funding
This work was supported by the grants from Chang Gung Medical Foundation (CMRPG3M0161-2).
Conflict of interest
The authors declare that the research was conducted in the absence of any commercial or financial relationships that could be construed as a potential conflict of interest.
The handling editor GB declared a past co-authorship with the authors H-YC, MRA, C-HL and F-CW.
Publisher’s note
All claims expressed in this article are solely those of the authors and do not necessarily represent those of their affiliated organizations, or those of the publisher, the editors and the reviewers. Any product that may be evaluated in this article, or claim that may be made by its manufacturer, is not guaranteed or endorsed by the publisher.
References
1. Garakani R, Saidi RF. Recent progress in cell therapy in solid organ transplantation. Int J Organ Transplant Med (2017) 8(3):125–31.
2. Pan GH, Chen Z, Xu L, Zhu JH, Xiang P, Ma JJ, et al. Low-dose tacrolimus combined with donor-derived mesenchymal stem cells after renal transplantation: a prospective, non-randomized study. Oncotarget (2016) 7(11):12089–101. doi: 10.18632/oncotarget.7725
3. Tan J, Wu W, Xu X, Liao L, Zheng F, Messinger S, et al. Induction therapy with autologous mesenchymal stem cells in living-related kidney transplants: a randomized controlled trial. JAMA (2012) 307(11):1169–77. doi: 10.1001/jama.2012.316
4. Vanikar AV, Trivedi HL, Kumar A, Gopal SC, Patel HV, Gumber MR, et al. Co-Infusion of donor adipose tissue-derived mesenchymal and hematopoietic stem cells helps safe minimization of immunosuppression in renal transplantation - single center experience. Renal failure. (2014) 36(9):1376–84. doi: 10.3109/0886022X.2014.950931
5. Casiraghi F, Perico N, Gotti E, Todeschini M, Mister M, Cortinovis M, et al. Kidney transplant tolerance associated with remote autologous mesenchymal stromal cell administration. Stem Cells Trans Med (2020) 9(4):427–32. doi: 10.1002/sctm.19-0185
6. López-Iglesias P, Blázquez-Martínez A, Fernández-Delgado J, Regadera J, Nistal M, Miguel MPD. Short and long term fate of human AMSC subcutaneously injected in mice. World J Stem Cells (2011) 3(6):53–62. doi: 10.4252/wjsc.v3.i6.53
7. Murphy MB, Moncivais K, Caplan AI. Mesenchymal stem cells: environmentally responsive therapeutics for regenerative medicine. Exp Mol Med (2013) 45(11):e54. doi: 10.1038/emm.2013.94
8. Bateman ME, Strong AL, Gimble JM, Bunnell BA. Concise review: using fat to fight disease: a systematic review of nonhomologous adipose-derived Stromal/Stem cell therapies. Stem Cells (Dayton Ohio). (2018) 36(9):1311–28. doi: 10.1002/stem.2847
9. Bourin P, Bunnell BA, Casteilla L, Dominici M, Katz AJ, March KL, et al. Stromal cells from the adipose tissue-derived stromal vascular fraction and culture expanded adipose tissue-derived stromal/stem cells: a joint statement of the international federation for adipose therapeutics and science (IFATS) and the international society for cellular therapy (ISCT). Cytotherapy (2013) 15(6):641–8. doi: 10.1016/j.jcyt.2013.02.006
10. Guo J, Nguyen A, Banyard DA, Fadavi D, Toranto JD, Wirth GA, et al. Stromal vascular fraction: a regenerative reality? part 2: mechanisms of regenerative action. J plastic reconstructive aesthetic Surg (2016) 69(2):180–8. doi: 10.1016/j.bjps.2015.10.014
11. Onodera T, Fukuhara A, Jang MH, Shin J, Aoi K, Kikuta J, et al. Adipose tissue macrophages induce PPARγ-high FOXP3(+) regulatory T cells. Sci Rep (2015) 5:16801. doi: 10.1038/srep16801
12. Lynch L, Michelet X, Zhang S, Brennan PJ, Moseman A, Lester C, et al. Regulatory iNKT cells lack expression of the transcription factor PLZF and control the homeostasis of t(reg) cells and macrophages in adipose tissue. Nat Immunol (2015) 16(1):85–95. doi: 10.1038/ni.3047
13. Nishimura S, Manabe I, Takaki S, Nagasaki M, Otsu M, Yamashita H, et al. Adipose natural regulatory b cells negatively control adipose tissue inflammation. Cell Metab (2013) 18(5):759–66. doi: 10.1016/j.cmet.2013.09.017
14. Stivers KB, Beare JE, Chilton PM, Williams SK, Kaufman CL, Hoying JB. Adipose-derived cellular therapies in solid organ and vascularized-composite allotransplantation. Curr Opin Organ transplantation. (2017) 22(5):490–8. doi: 10.1097/MOT.0000000000000452
15. Chae DS, Han S, Son M, Kim SW. Stromal vascular fraction shows robust wound healing through high chemotactic and epithelialization property. Cytotherapy (2017) 19(4):543–54. doi: 10.1016/j.jcyt.2017.01.006
16. Jin E, Chae DS, Son M, Kim SW. Angiogenic characteristics of human stromal vascular fraction in ischemic hindlimb. Int J Cardiol (2017) 234:38–47. doi: 10.1016/j.ijcard.2017.02.080
17. Zhou L, Song Q, Shen J, Xu L, Xu Z, Wu R, et al. Comparison of human adipose stromal vascular fraction and adipose-derived mesenchymal stem cells for the attenuation of acute renal ischemia/reperfusion injury. Sci Rep (2017) 7:44058. doi: 10.1038/srep44058
18. Andia I, Maffulli N, Burgos-Alonso N. Stromal vascular fraction technologies and clinical applications. Expert Opin Biol Ther (2019) 19(12):1289–305. doi: 10.1080/14712598.2019.1671970
19. Riordan NH, Ichim TE, Min WP, Wang H, Solano F, Lara F, et al. Non-expanded adipose stromal vascular fraction cell therapy for multiple sclerosis. J Trans Med (2009) 7:29. doi: 10.1186/1479-5876-7-29
20. Traktuev DO, Merfeld-Clauss S, Li J, Kolonin M, Arap W, Pasqualini R, et al. A population of multipotent CD34-positive adipose stromal cells share pericyte and mesenchymal surface markers, reside in a periendothelial location, and stabilize endothelial networks. Circ Res (2008) 102(1):77–85. doi: 10.1161/CIRCRESAHA.107.159475
21. Domenis R, Lazzaro L, Calabrese S, Mangoni D, Gallelli A, Bourkoula E, et al. Adipose tissue derived stem cells: in vitro and in vivo analysis of a standard and three commercially available cell-assisted lipotransfer techniques. Stem Cell Res Ther (2015) 6(1):2. doi: 10.1186/scrt536
22. Trivisonno A, Alexander RW, Baldari S, Cohen SR, Di Rocco G, Gentile P, et al. Intraoperative strategies for minimal manipulation of autologous adipose tissue for cell- and tissue-based therapies: concise review. Stem Cells Trans Med (2019) 8(12):1265–71. doi: 10.1002/sctm.19-0166
23. Wang X, Zhou C, Liu J, Mao L, Yang T, Hong X, et al. Administration of adipose stromal vascular fraction attenuates acute rejection in donation after circulatory death rat renal transplantation. Int J Urol (2022) 29(3):266–75. doi: 10.1111/iju.14757
24. Madonna R, Renna FV, Cellini C, Cotellese R, Picardi N, Francomano F, et al. Age-dependent impairment of number and angiogenic potential of adipose tissue-derived progenitor cells. Eur J Clin Invest. (2011) 41(2):126–33. doi: 10.1111/j.1365-2362.2010.02384.x
25. Aird AL, Nevitt CD, Christian K, Williams SK, Hoying JB, LeBlanc AJ. Adipose-derived stromal vascular fraction cells isolated from old animals exhibit reduced capacity to support the formation of microvascular networks. Exp Gerontol. (2015) 63:18–26. doi: 10.1016/j.exger.2015.01.044
26. Lee BC, Lee J. Cellular and molecular players in adipose tissue inflammation in the development of obesity-induced insulin resistance. Biochim Biophys Acta (2014) 1842(3):446–62. doi: 10.1016/j.bbadis.2013.05.017
27. Dykstra JA, Facile T, Patrick RJ, Francis KR, Milanovich S, Weimer JM, et al. Concise review: fat and furious: harnessing the full potential of adipose-derived stromal vascular fraction. Stem Cells Trans Med (2017) 6(4):1096–108. doi: 10.1002/sctm.16-0337
28. Raposio E, Simonacci F, Perrotta RE. Adipose-derived stem cells: comparison between two methods of isolation for clinical applications. Ann Med Surg (Lond). (2017) 20:87–91. doi: 10.1016/j.amsu.2017.07.018
29. Bora P, Majumdar AS. Adipose tissue-derived stromal vascular fraction in regenerative medicine: a brief review on biology and translation. Stem Cell Res Ther (2017) 8(1):145. doi: 10.1186/s13287-017-0598-y
30. Wu Y, Hoogduijn MJ, Baan CC, Korevaar SS, de Kuiper R, Yan L, et al. Adipose tissue-derived mesenchymal stem cells have a heterogenic cytokine secretion profile. Stem Cells Int (2017) 2017:4960831. doi: 10.1155/2017/4960831
31. Viswanathan S, Shi Y, Galipeau J, Krampera M, Leblanc K, Martin I, et al. Mesenchymal stem versus stromal cells: international society for cell & gene therapy (ISCT®) mesenchymal stromal cell committee position statement on nomenclature. Cytotherapy (2019) 21(10):1019–24. doi: 10.1016/j.jcyt.2019.08.002
32. Fang J, Yan Y, Teng X, Wen X, Li N, Peng S, et al. Melatonin prevents senescence of canine adipose-derived mesenchymal stem cells through activating NRF2 and inhibiting ER stress. Aging (2018) 10(10):2954–72. doi: 10.18632/aging.101602
33. Legzdina D, Romanauska A, Nikulshin S, Kozlovska T, Berzins U. Characterization of senescence of culture-expanded human adipose-derived mesenchymal stem cells. Int J Stem Cells (2016) 9(1):124–36. doi: 10.15283/ijsc.2016.9.1.124
34. Mitchell JB, McIntosh K, Zvonic S, Garrett S, Floyd ZE, Kloster A, et al. Immunophenotype of human adipose-derived cells: temporal changes in stromal-associated and stem cell-associated markers. Stem Cells (Dayton Ohio). (2006) 24(2):376–85. doi: 10.1634/stemcells.2005-0234
35. Jeske R, Yuan X, Fu Q, Bunnell BA, Logan TM, Li Y. In Vitro Culture expansion shifts the immune phenotype of human adipose-derived mesenchymal stem cells. Front Immunol (2021) 12:621744. doi: 10.3389/fimmu.2021.621744
36. Phetfong J, Tawonsawatruk T, Seenprachawong K, Srisarin A, Isarankura-Na-Ayudhya C, Supokawej A. Re-using blood products as an alternative supplement in the optimisation of clinical-grade adipose-derived mesenchymal stem cell culture. Bone Joint Res (2017) 6(7):414–22. doi: 10.1302/2046-3758.67.BJR-2016-0342.R1
37. Cheng HY, Anggelia MR, Lin CH, Lin CF. Preconditioned mesenchymal stromal cells to improve allotransplantation outcome. Cells (2021) 10(9):2325. doi: 10.3390/cells10092325
38. Crop MJ, Baan CC, Korevaar SS, Ijzermans JN, Pescatori M, Stubbs AP, et al. Inflammatory conditions affect gene expression and function of human adipose tissue-derived mesenchymal stem cells. Clin Exp Immunol (2010) 162(3):474–86. doi: 10.1111/j.1365-2249.2010.04256.x
39. Domenis R, Cifù A, Quaglia S, Pistis C, Moretti M, Vicario A, et al. Pro inflammatory stimuli enhance the immunosuppressive functions of adipose mesenchymal stem cells-derived exosomes. Sci Rep (2018) 8(1):13325. doi: 10.1038/s41598-018-31707-9
40. Patrikoski M, Mannerström B, Miettinen S. Perspectives for clinical translation of adipose Stromal/Stem cells. Stem Cells Int (2019) 2019:5858247. doi: 10.1155/2019/5858247
41. Franquesa M, Mensah FK, Huizinga R, Strini T, Boon L, Lombardo E, et al. Human adipose tissue-derived mesenchymal stem cells abrogate plasmablast formation and induce regulatory b cells independently of T helper cells. Stem Cells (Dayton Ohio). (2015) 33(3):880–91. doi: 10.1002/stem.1881
42. Gao F, Chiu SM, Motan DA, Zhang Z, Chen L, Ji HL, et al. Mesenchymal stem cells and immunomodulation: current status and future prospects. Cell Death disease. (2016) 7(1):e2062. doi: 10.1038/cddis.2015.327
43. Do JS, Zwick D, Kenyon JD, Zhong F, Askew D, Huang AY, et al. Mesenchymal stromal cell mitochondrial transfer to human induced T-regulatory cells mediates FOXP3 stability. Sci Rep (2021) 11(1):10676. doi: 10.1038/s41598-021-90115-8
44. Mohammadalipour A, Dumbali SP, Wenzel PL. Mitochondrial transfer and regulators of mesenchymal stromal cell function and therapeutic efficacy. Front Cell Dev Biol (2020) 8(603292). doi: 10.3389/fcell.2020.603292
45. Tse WT, Pendleton JD, Beyer WM, Egalka MC, Guinan EC. Suppression of allogeneic T-cell proliferation by human marrow stromal cells: implications in transplantation. Transplantation (2003) 75(3):389–97. doi: 10.1097/01.TP.0000045055.63901.A9
46. Le Blanc K, Tammik C, Rosendahl K, Zetterberg E, Ringdén O. HLA expression and immunologic properties of differentiated and undifferentiated mesenchymal stem cells. Exp hematol. (2003) 31(10):890–6. doi: 10.1016/S0301-472X(03)00110-3
47. Oh JY, Kim H, Lee HJ, Lee K, Barreda H, Kim HJ, et al. MHC class I enables MSCs to evade NK-Cell-Mediated cytotoxicity and exert immunosuppressive activity. Stem Cells (Dayton Ohio). (2022) 40(9):870–82. doi: 10.1093/stmcls/sxac043
48. Kuçi Z, Bönig H, Kreyenberg H, Bunos M, Jauch A, Janssen JW, et al. Mesenchymal stromal cells from pooled mononuclear cells of multiple bone marrow donors as rescue therapy in pediatric severe steroid-refractory graft-versus-host disease: a multicenter survey. Haematologica (2016) 101(8):985–94. doi: 10.3324/haematol.2015.140368
49. PK L, Kandoi S, Misra R, Vijayalakshmi S, Rajagopal K, Verma RS. The mesenchymal stem cell secretome: a new paradigm towards cell-free therapeutic mode in regenerative medicine. Cytokine Growth Factor Rev (2019) 46:1–9. doi: 10.1016/j.cytogfr.2019.04.002
50. Bellei B, Papaccio F, Filoni A, Caputo S, Lopez G, Migliano E, et al. Extracellular fraction of adipose tissue as an innovative regenerative approach for vitiligo treatment. Exp Dermatol (2019) 28(6):695–703. doi: 10.1111/exd.13954
51. Bellei B, Migliano E, Tedesco M, Caputo S, Papaccio F, Lopez G, et al. Adipose tissue-derived extracellular fraction characterization: biological and clinical considerations in regenerative medicine. Stem Cell Res Ther (2018) 9(1):207. doi: 10.1186/s13287-018-0956-4
52. Vizoso FJ, Eiro N, Cid S, Schneider J, Perez-Fernandez R. Mesenchymal stem cell secretome: toward cell-free therapeutic strategies in regenerative medicine. Int J Mol Sci (2017) 18(9):1852. doi: 10.3390/ijms18091852
53. Gowen A, Shahjin F, Chand S, Odegaard KE, Yelamanchili SV. Mesenchymal stem cell-derived extracellular vesicles: challenges in clinical applications. Front Cell Dev Biol (2020) 8:149. doi: 10.3389/fcell.2020.00149
54. Théry C, Witwer KW, Aikawa E, Alcaraz MJ, Anderson JD, Andriantsitohaina R, et al. Minimal information for studies of extracellular vesicles 2018 (MISEV2018): a position statement of the international society for extracellular vesicles and update of the MISEV2014 guidelines. J Extracell Vesicles. (2018) 7(1):1535750. doi: 10.1080/20013078.2018.1535750
55. Bray ER, Oropallo AR, Grande DA, Kirsner RS, Badiavas EV. Extracellular vesicles as therapeutic tools for the treatment of chronic wounds. Pharmaceutics (2021) 13(10):1543. doi: 10.3390/pharmaceutics13101543
56. Shin S, Lee J, Kwon Y, Park KS, Jeong JH, Choi SJ, et al. Comparative proteomic analysis of the mesenchymal stem cells secretome from adipose, bone marrow, placenta and wharton's jelly. Int J Mol Sci (2021) 22(2):845. doi: 10.3390/ijms22020845
57. Alonso-Alonso ML, García-Posadas L, Diebold Y. Extracellular vesicles from human adipose-derived mesenchymal stem cells: a review of common cargos. Stem Cell Rev Rep (2022) 18(3):854–901. doi: 10.1007/s12015-021-10155-5
58. Gómez-Ferrer M, Villanueva-Badenas E, Sánchez-Sánchez R, Sánchez-López CM, Baquero MC, Sepúlveda P, et al. HIF-1α and pro-inflammatory signaling improves the immunomodulatory activity of MSC-derived extracellular vesicles. Int J Mol Sci (2021) 22(7):3416. doi: 10.3390/ijms22073416
59. Ren S, Chen J, Duscher D, Liu Y, Guo G, Kang Y, et al. Microvesicles from human adipose stem cells promote wound healing by optimizing cellular functions via AKT and ERK signaling pathways. Stem Cell Res Ther (2019) 10(1):47. doi: 10.1186/s13287-019-1152-x
60. Lee M, Ban JJ, Yang S, Im W, Kim M. The exosome of adipose-derived stem cells reduces β-amyloid pathology and apoptosis of neuronal cells derived from the transgenic mouse model of alzheimer's disease. Brain Res (2018) 1691:87–93. doi: 10.1016/j.brainres.2018.03.034
61. Lou G, Song X, Yang F, Wu S, Wang J, Chen Z, et al. Exosomes derived from miR-122-modified adipose tissue-derived MSCs increase chemosensitivity of hepatocellular carcinoma. J Hematol Oncol (2015) 8:122. doi: 10.1186/s13045-015-0220-7
62. Luo Q, Guo D, Liu G, Chen G, Hang M, Jin M. Exosomes from MiR-126-Overexpressing adscs are therapeutic in relieving acute myocardial ischaemic injury. Cell Physiol Biochem (2017) 44(6):2105–16. doi: 10.1159/000485949
63. Zhao R, Zhao T, He Z, Cai R, Pang W. Composition, isolation, identification and function of adipose tissue-derived exosomes. Adipocyte (2021) 10(1):587–604. doi: 10.1080/21623945.2021.1983242
64. Gandham S, Su X, Wood J, Nocera AL, Alli SC, Milane L, et al. Technologies and standardization in research on extracellular vesicles. Trends Biotechnol (2020) 38(10):1066–98. doi: 10.1016/j.tibtech.2020.05.012
65. Ellis BW, Traktuev DO, Merfeld-Clauss S, Can UI, Wang M, Bergeron R, et al. Adipose stem cell secretome markedly improves rodent heart and human induced pluripotent stem cell-derived cardiomyocyte recovery from cardioplegic transport solution exposure. Stem Cells (Dayton Ohio). (2021) 39(2):170–82. doi: 10.1002/stem.3296
66. Lee SC, Jeong HJ, Lee SK, Kim SJ. Hypoxic conditioned medium from human adipose-derived stem cells promotes mouse liver regeneration through JAK/STAT3 signaling. Stem Cells Trans Med (2016) 5(6):816–25. doi: 10.5966/sctm.2015-0191
67. Silveira BM, Ribeiro TO, Freitas RS, Carreira ACO, Gonçalves MS, Sogayar M, et al. Secretome from human adipose-derived mesenchymal stem cells promotes blood vessel formation and pericyte coverage in experimental skin repair. PloS One (2022) 17(12):e0277863. doi: 10.1371/journal.pone.0277863
68. Bejaoui M, Pantazi E, Folch-Puy E, Baptista PM, García-Gil A, Adam R, et al. Emerging concepts in liver graft preservation. World J gastroenterol. (2015) 21(2):396–407. doi: 10.3748/wjg.v21.i2.396
69. He J, Khan UZ, Qing L, Wu P, Tang J. Improving the ischemia-reperfusion injury in vascularized composite allotransplantation: clinical experience and experimental implications. Front Immunol (2022) 13:998952. doi: 10.3389/fimmu.2022.998952
70. McAnulty JF. Hypothermic organ preservation by static storage methods: current status and a view to the future. Cryobiology (2010) 60(3 Suppl):S13–9. doi: 10.1016/j.cryobiol.2009.06.004
71. Oliva J. Therapeutic properties of mesenchymal stem cell on organ ischemia-reperfusion injury. Int J Mol Sci (2019) 20(21):5511. doi: 10.3390/ijms20215511
72. Kasahara N, Teratani T, Doi J, Iijima Y, Maeda M, Uemoto S, et al. Use of mesenchymal stem cell-conditioned medium to activate islets in preservation solution. Cell Med (2013) 5(2-3):75–81. doi: 10.3727/215517913X666477
73. Teratani T, Kasahara N, Fujimoto Y, Sakuma Y, Miki A, Goto M, et al. Mesenchymal stem cells secretions enhanced ATP generation on isolated islets during transplantation. Islets (2022) 14(1):69–81. doi: 10.1080/19382014.2021.2022423
74. Wang M, Yan L, Li Q, Yang Y, Turrentine M, March K, et al. Mesenchymal stem cell secretions improve donor heart function following ex vivo cold storage. J Thorac Cardiovasc surgery. (2022) 163(4):e277–e92. doi: 10.1016/j.jtcvs.2020.08.095
75. Scott SR, March KL, Wang IW, Singh K, Liu J, Turrentine M, et al. Bone marrow- or adipose-mesenchymal stromal cell secretome preserves myocardial transcriptome profile and ameliorates cardiac damage following ex vivo cold storage. J Mol Cell Cardiol (2022) 164:1–12. doi: 10.1016/j.yjmcc.2021.11.002
76. Pool MBF, Vos J, Eijken M, van Pel M, Reinders MEJ, Ploeg RJ, et al. Treating ischemically damaged porcine kidneys with human bone marrow- and adipose tissue-derived mesenchymal stromal cells during ex vivo normothermic machine perfusion. Stem Cells Dev (2020) 29(20):1320–30. doi: 10.1089/scd.2020.0024
77. Lohmann S, Pool MBF, Rozenberg KM, Keller AK, Moers C, Møldrup U, et al. Mesenchymal stromal cell treatment of donor kidneys during ex vivo normothermic machine perfusion: a porcine renal autotransplantation study. Am J Transplant (2021) 21(7):2348–59. doi: 10.1111/ajt.16473
78. Wang Y, Wang S, Gu C, Xiong Y, Shen H, Liu F, et al. Ex-vivo treatment of allografts using adipose-derived stem cells induced prolonged rejection-free survival in an allogenic hind-limb transplantation model. Ann Trans Med (2020) 8(14):867. doi: 10.21037/atm.2019.12.141
79. Kato T, Okumi M, Tanemura M, Yazawa K, Kakuta Y, Yamanaka K, et al. Adipose tissue-derived stem cells suppress acute cellular rejection by TSG-6 and CD44 interaction in rat kidney transplantation. Transplantation (2014) 98(3):277–84. doi: 10.1097/TP.0000000000000230
80. Lohmann S, Eijken M, Møldrup U, Møller BK, Hunter J, Moers C, et al. Ex vivo administration of mesenchymal stromal cells in kidney grafts against ischemia-reperfusion injury-effective delivery without kidney function improvement posttransplant. Transplantation (2021) 105(3):517–28. doi: 10.1097/TP.0000000000003429
81. Navarro-Zorraquino M, Pastor C, Stringa P, Soria J, Hernández F, López-Santamaría M, et al. Implant of mesenchymal cells decreases acute cellular rejection in small bowel transplantation. Cir Cir. (2020) 88(5):554–61. doi: 10.24875/CIRU.20000130
82. Andres AM, Stringa P, Talayero P, Santamaria M, García-Arranz M, García Gómez-Heras S, et al. Graft infusion of adipose-derived mesenchymal stromal cells to prevent rejection in experimental intestinal transplantation: a feasibility study. Clin transplantation. (2021) 35(4):e14226. doi: 10.1111/ctr.14226
83. Bai Y, Han YD, Yan XL, Ren J, Zeng Q, Li XD, et al. Adipose mesenchymal stem cell-derived exosomes stimulated by hydrogen peroxide enhanced skin flap recovery in ischemia-reperfusion injury. Biochem Biophys Res Commun (2018) 500(2):310–7. doi: 10.1016/j.bbrc.2018.04.065
84. Munk A, Duvald CS, Pedersen M, Lohmann S, Keller AK, Møller BK, et al. Dosing limitation for intra-renal arterial infusion of mesenchymal stromal cells. Int J Mol Sci (2022) 23(15):8268. doi: 10.3390/ijms23158268
85. Wang X, Zhou C, Liu J, Yang T, Mao L, Hong X, et al. Administration of donor-derived nonexpanded adipose stromal vascular fraction attenuates ischemia-reperfusion injury in donation after cardiac death rat renal transplantation. Transplant Proc (2021) 53(6):2070–81. doi: 10.1016/j.transproceed.2021.05.012
86. Chen J, Wang Y, Hu H, Xiong Y, Wang S, Yang J. Adipose-derived cellular therapies prolong graft survival in an allogenic hind limb transplantation model. Stem Cell Res Ther (2021) 12(1):94. doi: 10.1186/s13287-021-02162-7
87. Qin YF, Kong DJ, Qin H, Zhu YL, Li GM, Sun CL, et al. Melatonin synergizes with mesenchymal stromal cells attenuates chronic allograft vasculopathy. Front Immunol (2021) 12:672849. doi: 10.3389/fimmu.2021.672849
88. Yip HK, Lee MS, Sun CK, Chen KH, Chai HT, Sung PH, et al. Therapeutic effects of adipose-derived mesenchymal stem cells against brain death-induced remote organ damage and post-heart transplant acute rejection. Oncotarget (2017) 8(65):108692–711. doi: 10.18632/oncotarget.21433
89. Gao C, Wang X, Lu J, Li Z, Jia H, Chen M, et al. Mesenchymal stem cells transfected with sFgl2 inhibit the acute rejection of heart transplantation in mice by regulating macrophage activation. Stem Cell Res Ther (2020) 11(1):241. doi: 10.1186/s13287-020-01752-1
90. Wang W, Zhao N, Li B, Gao H, Yan Y, Guo H. Inhibition of cardiac allograft rejection in mice using interleukin-35-modified mesenchymal stem cells. Scandinavian J Immunol (2019) 89(4):e12750. doi: 10.1111/sji.12750
91. Guo H, Li B, Li N, Liu X, Gao H, Sun X, et al. Exosomes: potential executors of IL-35 gene-modified adipose-derived mesenchymal stem cells in inhibiting acute rejection after heart transplantation. Scandinavian J Immunol (2022) 96(2):e13171. doi: 10.1111/sji.13171
92. Obermajer N, Popp FC, Soeder Y, Haarer J, Geissler EK, Schlitt HJ, et al. Conversion of Th17 into IL-17A(neg) regulatory T cells: a novel mechanism in prolonged allograft survival promoted by mesenchymal stem cell-supported minimized immunosuppressive therapy. J Immunol (Baltimore Md: 1950). (2014) 193(10):4988–99. doi: 10.4049/jimmunol.1401776
93. Weiss ARR, Lee O, Eggenhofer E, Geissler E, Korevaar SS, Soeder Y, et al. Differential effects of heat-inactivated, secretome-deficient MSC and metabolically active MSC in sepsis and allogenic heart transplantation. Stem Cells (Dayton Ohio). (2020) 38(6):797–807. doi: 10.1002/stem.3165
94. Bao Z, Li J, Zhang P, Pan Q, Liu B, Zhu J, et al. Toll-like receptor 3 activator preconditioning enhances modulatory function of Adipose−Derived mesenchymal stem cells in a fully MHC-mismatched murine model of heterotopic heart transplantation. Ann transplantation. (2020) 25:e921287. doi: 10.12659/AOT.921287
95. Ohmura Y, Tanemura M, Kawaguchi N, Machida T, Tanida T, Deguchi T, et al. Combined transplantation of pancreatic islets and adipose tissue-derived stem cells enhances the survival and insulin function of islet grafts in diabetic mice. Transplantation (2010) 90(12):1366–73. doi: 10.1097/TP.0b013e3181ffba31
96. Liu T, Zhang Y, Shen Z, Zou X, Chen X, Chen L, et al. Immunomodulatory effects of OX40Ig gene-modified adipose tissue-derived mesenchymal stem cells on rat kidney transplantation. Int J Mol Med (2017) 39(1):144–52. doi: 10.3892/ijmm.2016.2808
97. Ramirez-Bajo MJ, Rovira J, Lazo-Rodriguez M, Banon-Maneus E, Tubita V, Moya-Rull D, et al. Impact of mesenchymal stromal cells and their extracellular vesicles in a rat model of kidney rejection. Front Cell Dev Biol (2020) 8:10. doi: 10.3389/fcell.2020.00010
98. Wan CD, Cheng R, Wang HB, Liu T. Immunomodulatory effects of mesenchymal stem cells derived from adipose tissues in a rat orthotopic liver transplantation model. Hepatobiliary Pancreat Dis Int (2008) 7(1):29–33.
99. Gao W, Zhang L, Zhang Y, Sun C, Chen X, Wang Y. Adipose-derived mesenchymal stem cells promote liver regeneration and suppress rejection in small-for-size liver allograft. Transplant Immunol (2017) 45:1–7. doi: 10.1016/j.trim.2017.07.005
100. Sasajima H, Miyagi S, Yamada S, Kakizaki Y, Kamei T, Unno M, et al. Cytoprotective effects of mesenchymal stem cells during liver transplantation from donors after cardiac death in swine. Transplant Proc (2020) 52(6):1891–900. doi: 10.1016/j.transproceed.2020.01.165
101. Watanabe H, Tsuchiya T, Shimoyama K, Shimizu A, Akita S, Yukawa H, et al. Adipose-derived mesenchymal stem cells attenuate rejection in a rat lung transplantation model. J Surg Res (2018) 227:17–27. doi: 10.1016/j.jss.2018.01.016
102. Larocca RA, Moraes-Vieira PM, Bassi EJ, Semedo P, de Almeida DC, da Silva MB, et al. Adipose tissue-derived mesenchymal stem cells increase skin allograft survival and inhibit Th-17 immune response. PloS One (2013) 8(10):e76396. doi: 10.1371/journal.pone.0076396
103. Lee SM, Lee SC, Kim SJ. Contribution of human adipose tissue-derived stem cells and the secretome to the skin allograft survival in mice. J Surg Res (2014) 188(1):280–9. doi: 10.1016/j.jss.2013.10.063
104. Hu JL, Kim BJ, Yu NH, Kwon ST. Impact of injection frequency of adipose-derived stem cells on allogeneic skin graft survival outcomes in mice. Cell transplantation. (2021) 30:1–12. doi: 10.1177/09636897211041966
105. Davis TA, Anam K, Lazdun Y, Gimble JM, Elster EA. Adipose-derived stromal cells promote allograft tolerance induction. Stem Cells Trans Med (2014) 3(12):1444–50. doi: 10.5966/sctm.2014-0131
106. Zhang Y, Meng Q, Zhang Y, Chen X, Wang Y. Adipose-derived mesenchymal stem cells suppress of acute rejection in small bowel transplantation. Saudi J Gastroenterol (2017) 23(6):323–9. doi: 10.4103/sjg.SJG_122_17
107. Ma T, Luan S, Tao R, Lu D, Guo L, Liu J, et al. Targeted migration of human adipose-derived stem cells to secondary lymphoid organs enhances their immunomodulatory effect and prolongs the survival of allografted vascularized composites. Stem Cells (Dayton Ohio). (2019) 37(12):1581–94. doi: 10.1002/stem.3078
108. Jeong SH, Ji YH, Yoon ES. Immunosuppressive activity of adipose tissue-derived mesenchymal stem cells in a rat model of hind limb allotransplantation. Transplant Proc (2014) 46(5):1606–14. doi: 10.1016/j.transproceed.2013.12.069
109. Kuo YR, Chen CC, Goto S, Lee IT, Huang CW, Tsai CC, et al. Modulation of immune response and T-cell regulation by donor adipose-derived stem cells in a rodent hind-limb allotransplant model. Plast reconstructive surgery. (2011) 128(6):661e–72e. doi: 10.1097/PRS.0b013e318230c60b
110. Kuo YR, Chen CC, Goto S, Huang YT, Tsai CC, Yang MY. Proteomic analysis in serum of rat hind-limb allograft tolerance induced by immunosuppressive therapy with adipose-derived stem cells. Plast reconstructive surgery. (2014) 134(6):1213–23. doi: 10.1097/PRS.0000000000000725
111. Plock JA, Schnider JT, Zhang W, Schweizer R, Tsuji W, Kostereva N, et al. Adipose- and bone marrow-derived mesenchymal stem cells prolong graft survival in vascularized composite allotransplantation. Transplantation (2015) 99(9):1765–73. doi: 10.1097/TP.0000000000000731
112. Plock JA, Schnider JT, Schweizer R, Zhang W, Tsuji W, Waldner M, et al. The influence of timing and frequency of adipose-derived mesenchymal stem cell therapy on immunomodulation outcomes after vascularized composite allotransplantation. Transplantation (2017) 101(1):e1–e11. doi: 10.1097/TP.0000000000001498
113. Schweizer R, Taddeo A, Waldner M, Klein HJ, Fuchs N, Kamat P, et al. Adipose-derived stromal cell therapy combined with a short course nonmyeloablative conditioning promotes long-term graft tolerance in vascularized composite allotransplantation. Am J Transplant (2020) 20(5):1272–84. doi: 10.1111/ajt.15726
114. Chen CC, Chen RF, Shao JS, Li YT, Wang YC, Brandacher G, et al. Adipose-derived stromal cells modulating composite allotransplant survival is correlated with b cell regulation in a rodent hind-limb allotransplantation model. Stem Cell Res Ther (2020) 11(1):478. doi: 10.1186/s13287-020-01961-8
115. Chen Z, Xue S, Zhang S, Cheng K, Ye Q. Exosomes from donor-derived adipose mesenchymal stem cells prolong the survival of vascularized composite allografts. J Cell Physiol (2021) 236(8):5895–905. doi: 10.1002/jcp.30274
116. Cheng HY, Ghetu N, Huang WC, Wang YL, Wallace CG, Wen CJ, et al. Syngeneic adipose-derived stem cells with short-term immunosuppression induce vascularized composite allotransplantation tolerance in rats. Cytotherapy (2014) 16(3):369–80. doi: 10.1016/j.jcyt.2013.06.020
117. Ramirez AE, Cheng HY, Lao WW, Wang YL, Wen CJ, Wallace CG, et al. A novel rat full-thickness hemi-abdominal wall/hindlimb osteomyocutaneous combined flap: influence of allograft mass and vascularized bone marrow content on vascularized composite allograft survival. Transplant Int (2014) 27(9):977–86. doi: 10.1111/tri.12364
118. Kuo YR, Chen CC, Chen YC, Chien CM. Recipient adipose-derived stem cells enhance recipient cell engraftment and prolong allotransplant survival in a miniature swine hind-limb model. Cell transplantation. (2017) 26(8):1418–27. doi: 10.1177/0963689717724534
119. Cabezas J, Rojas D, Wong Y, Telleria F, Manriquez J, Mançanares ACF, et al. In vitro Preconditioning of equine adipose mesenchymal stem cells with prostaglandin E(2), substance p and their combination changes the cellular protein secretomics and improves their immunomodulatory competence without compromising stemness. Veterinary Immunol immunopathol. (2020) 228:110100. doi: 10.1016/j.vetimm.2020.110100
120. An JH, Li Q, Bhang DH, Song WJ, Youn HY. TNF-α and INF-γ primed canine stem cell-derived extracellular vesicles alleviate experimental murine colitis. Sci Rep (2020) 10(1):2115. doi: 10.1038/s41598-020-58909-4
121. Hoogduijn MJ, Crop MJ, Korevaar SS, Peeters AM, Eijken M, Maat LP, et al. Susceptibility of human mesenchymal stem cells to tacrolimus, mycophenolic acid, and rapamycin. Transplantation (2008) 86(9):1283–91. doi: 10.1097/TP.0b013e31818aa536
122. Hajkova M, Jaburek F, Porubska B, Bohacova P, Holan V, Krulova M. Cyclosporine a promotes the therapeutic effect of mesenchymal stem cells on transplantation reaction. Clin Sci (London England: 1979). (2019) 133(21):2143–57. doi: 10.1042/CS20190294
123. Hajkova M, Hermankova B, Javorkova E, Bohacova P, Zajicova A, Holan V, et al. Mesenchymal stem cells attenuate the adverse effects of immunosuppressive drugs on distinct T cell subopulations. Stem Cell Rev Rep (2017) 13(1):104–15. doi: 10.1007/s12015-016-9703-3
124. Vanikar AV, Trivedi HL, Gopal SC, Kumar A, Dave SD. Pre-transplant co-infusion of donor-adipose tissue derived mesenchymal stem cells and hematopoietic stem cells may help in achieving tolerance in living donor renal transplantation. Renal failure. (2014) 36(3):457–60. doi: 10.3109/0886022X.2013.868295
125. Vanikar AV, Trivedi HL. Stem cell transplantation in living donor renal transplantation for minimization of immunosuppression. Transplantation (2012) 94(8):845–50. doi: 10.1097/TP.0b013e3182664000
126. Vanikar AV, Trivedi HL, Thakkar UG. Six years' experience of tolerance induction in renal transplantation using stem cell therapy. Clin Immunol (Orlando Fla). (2018) 187:10–4. doi: 10.1016/j.clim.2017.07.024
127. Chambers DC, Enever D, Lawrence S, Sturm MJ, Herrmann R, Yerkovich S, et al. Mesenchymal stromal cell therapy for chronic lung allograft dysfunction: results of a first-in-Man study. Stem Cells Trans Med (2017) 6(4):1152–7. doi: 10.1002/sctm.16-0372
128. Ban TH, Lee S, Kim HD, Ko EJ, Kim BM, Kim KW, et al. Clinical trial of allogeneic mesenchymal stem cell therapy for chronic active antibody-mediated rejection in kidney transplant recipients unresponsive to rituximab and intravenous immunoglobulin. Stem Cells Int (2021) 2021:6672644. doi: 10.1155/2021/6672644
129. Wei Y, Chen X, Zhang H, Su Q, Peng Y, Fu Q, et al. Efficacy and safety of bone marrow-derived mesenchymal stem cells for chronic antibody-mediated rejection after kidney transplantation- a single-arm, two-Dosing-Regimen, phase I/II study. Front Immunol (2021) 12:662441. doi: 10.3389/fimmu.2021.662441
130. Shi M, Liu Z, Wang Y, Xu R, Sun Y, Zhang M, et al. A pilot study of mesenchymal stem cell therapy for acute liver allograft rejection. Stem Cells Trans Med (2017) 6(12):2053–61. doi: 10.1002/sctm.17-0134
131. Anggelia MR, Cheng HY, Lai PC, Hsieh YH, Lin CH, Lin CH. Cell therapy in vascularized composite allotransplantation. Biomed J (2022) 45(3):454–64. doi: 10.1016/j.bj.2022.01.005
132. Lin CH, Anggelia MR, Cheng HY, Wang AYL, Chuang WY, Lin CH, et al. The intragraft vascularized bone marrow component plays a critical role in tolerance induction after reconstructive transplantation. Cell Mol Immunol (2021) 18(2):363–73. doi: 10.1038/s41423-019-0325-y
133. Gordon CR, Tai CY, Suzuki H, Strande LF, Ramsamooj R, Matthews MS, et al. Review of vascularized bone marrow transplantation: current status and future clinical applications. Microsurgery (2007) 27(4):348–53. doi: 10.1002/micr.20367
134. Podestà MA, Sykes M. Chimerism-based tolerance to kidney allografts in humans: novel insights and future perspectives. Front Immunol (2021) 12:791725. doi: 10.3389/fimmu.2021.791725
135. Bartosh TJ, Ylöstalo JH, Mohammadipoor A, Bazhanov N, Coble K, Claypool K, et al. Aggregation of human mesenchymal stromal cells (MSCs) into 3D spheroids enhances their antiinflammatory properties. Proc Natl Acad Sci United States America. (2010) 107(31):13724–9. doi: 10.1073/pnas.1008117107
136. Bogers SH, Barrett JG. Three-dimensional culture of equine bone marrow-derived mesenchymal stem cells enhances anti-inflammatory properties in a donor-dependent manner. Stem Cells Dev (2022). doi: 10.1089/scd.2022.0074
Keywords: transplantation, tolerance, stromal vascular fraction (SVF), secretome, AD-MSCs
Citation: Cheng H-Y, Anggelia MR, Lin C-H and Wei F-C (2023) Toward transplantation tolerance with adipose tissue-derived therapeutics. Front. Immunol. 14:1111813. doi: 10.3389/fimmu.2023.1111813
Received: 30 November 2022; Accepted: 07 April 2023;
Published: 28 April 2023.
Edited by:
Gerald Brandacher, Johns Hopkins University, United StatesReviewed by:
Teresa Lopes Ramos, Stanford University, United StatesLesley Ann Smyth, University of East London, United Kingdom
Carlo Tremolada, Manchester Metropolitan University, United Kingdom
Copyright © 2023 Cheng, Anggelia, Lin and Wei. This is an open-access article distributed under the terms of the Creative Commons Attribution License (CC BY). The use, distribution or reproduction in other forums is permitted, provided the original author(s) and the copyright owner(s) are credited and that the original publication in this journal is cited, in accordance with accepted academic practice. No use, distribution or reproduction is permitted which does not comply with these terms.
*Correspondence: Hui-Yun Cheng, aHljaGVuZzIxQGdtYWlsLmNvbQ==
†These authors have contributed equally to this work and share first authorship