- 1Affiliated Cancer Hospital & Institute and Key Laboratory for Cell Homeostasis and Cancer Research of Guangdong Higher Education Institutes, Guangzhou Medical University, Guangzhou, Guangdong, China
- 2Department of Infectious Diseases, Guangzhou Panyu Central Hospital, Guangzhou, Guangdong, China
- 3Department of Biotechnology and Laboratory Science in Medicine, National Yang Ming Chiao Tung University, Taipei, Taiwan
- 4Cancer Progression Research Center, National Yang Ming Chiao Tung University, Taipei, Taiwan
- 5Department of Biomedical Science, College of Medicine, and Program in Biomedical Sciences and Engineering, Inha University, Incheon, Republic of Korea
- 6Graduate Institute of Biomedical Sciences, China Medical University, Taichung, Taiwan
T cells play a crucial role in the regulation of immune response and are integral to the efficacy of cancer immunotherapy. Because immunotherapy has emerged as a promising treatment for cancer, increasing attention has been focused on the differentiation and function of T cells in immune response. In this review, we describe the research progress on T-cell exhaustion and stemness in the field of cancer immunotherapy and summarize advances in potential strategies to intervene and treat chronic infection and cancer by reversing T-cell exhaustion and maintaining and increasing T-cell stemness. Moreover, we discuss therapeutic strategies to overcome T-cell immunodeficiency in the tumor microenvironment and promote continuous breakthroughs in the anticancer activity of T cells.
1 Introduction
When the body is exposed to antigenic stimulation (such as that from an infection or tumor), the immune system learns to recognize antigens, generates an immune response, and forms immune memory. Immunotherapy can effectively remove pathogens and tumor cells from the body by activating or mobilizing patients’ own immune system and subsequently restarting and maintaining attacks on pathogens and cancer cells (1, 2). Currently, cancer immunotherapy is proven to prolong the survival of patients (3). However, cancer immunotherapy is beneficial only for specific populations. Thus, cancer immunotherapy is among the most widely researched topics in oncology in order to uncover methods to improve its efficacy. With the popularization of cancer immunotherapy (4), T cells in the tumor microenvironment (TME) have received increasing attention because of their powerful immune effects. T-cell therapy is a promising antitumor immunotherapy that can reduce tumor progression (5, 6). Adoptive T-cell transfer (ACT) immunotherapies currently used in cancer immunotherapy include chimeric antigen receptor (CAR) T-cell therapy (7, 8), tumor-infiltrating lymphocyte (TIL) therapy (9), and engineered T-cell receptor (TCR) T-cell therapy (10). Although T-cell therapy resulted in high response rates in nonsolid tumors and sustained clinical remission in clinical trials (11, 12), a significant reduction in antigen-specific T cells can impair the ability of T cells to kill infected or tumor cells, resulting in limited immune response (13).
T-cell exhaustion, a state of effector T-cell dysfunction, was originally identified by Moskophidis et al. (14). During chronic infection or cancer progression, with the continual stimulation of inflammatory factors or antigens, T cells gradually lose their effector function and memory T-cell characteristics, resulting in the body being unable to maintain a long-term durable and effective immune response (15). T-cell exhaustion is a major obstacle to developing effective cancer immunotherapy (16). Moreover, T-cell exhaustion in tumors is negatively related to the therapeutic efficacy of immunotherapy (17, 18). Immunotherapies based on T-cell exhaustion have been widely investigated and studied. Currently, immunotherapy used to inhibit effector T-cell exhaustion involves the blocking antibodies that target immune checkpoint molecules, including cytotoxic T-lymphocyte antigen 4 (CTLA4; ipilimumab and tremelimumab) and programmed cell death protein-1 (PD-1; pembrolizumab, nivolumab, and cemiplimab) and its ligand PD-L1 (atezolizumab, avelumab, and durvalumab). These monoclonal antibodies improve antigen-specific T-cell activity and persistence by blocking the critical negative regulators of its function; however, the emergence of immune-related adverse events limits their clinical benefits (19–21). Scientists are attempting to develop new strategies to target terminally exhausted T cells (TEX) and restore immune function (22).
Memory T lymphocytes (TM) play a key role in the immune response to cancer and infectious diseases, producing a rapid immune response upon repeated antigenic stimulation. On the basis of the cell phenotype, distribution location and function, TM can be divided into different subsets, namely stem cell-like memory T cells (TSCM), central memory T cells (TCM), effector memory T cells (TEM), tissue-resident effector memory T cells (TRM) and terminally differentiated effector memory T cells (TEMRA) (23–25). TSCM and TCM are mainly located in secondary lymphoid organs such as lymph nodes, TEM circulate throughout the body through the blood and lymph, TRM reside in various peripheral tissues, and TEMRA are present in blood and blood-rich tissues (e.g., the spleen, bone marrow, and lung) (25). TSCM play a crucial role in promoting antitumor and immune reconstitution because of their enhanced stem cell-like self-renewal capacity, multidifferentiation potential, and long-term effector functions, and TSCM have been applied in cancer therapy (26–28). TSCM can serve as a reservoir of effector T cells with potent therapeutic properties. Antigen-specific TSCM survived in tumor-bearing mice for a long time and differentiated into other T-cell subsets, which effectively removed targeted tumor cells and mediated a superior antitumor response (29). Given the role of TSCM in the antitumor response, TSCM can be used as host cells for CAR engineering to generate CAR-modified T cells carrying the TSCM-surface phenotype. Efficient derivation of CAR-TSCM could overcome cancer-specific T-cell deficiencies and improve the efficacy of adaptive cancer immunotherapy (30–32).
Currently, the limitations of T-cell therapy include low response rates, poor persistence, and proliferative exhaustion (33–35). Although T-cell exhaustion and TSCM were first identified in infectious diseases, their mechanism and functions in tumors are also vital for the development of antitumor immunotherapy. In clinical treatment, a clear understanding of the effect of T-cell exhaustion and stemness on tumors can help improve and promote T cell-based therapy, thus contributing to the rational design of new treatment strategies. Therefore, in this review, we discuss the mechanisms underlying T-cell exhaustion and stemness and the latest improvements in T-cell adoptive transfer therapies based on these mechanisms. This review provides new insights that can be beneficial for the further study of adoptive T-cell therapy and its related clinical trials, which may contribute to the design and implementation of new therapeutic strategies.
2 T-cell exhaustion
2.1 Concept of T-cell exhaustion
During chronic infection or cancer, antigens or inflammatory factors of pathogens or tumor cells stimulate T cells for a prolonged period, resulting in the gradual loss of the function of memory and effector T cells. This process is called T-cell exhaustion (15). When naïve T cells are activated by antigens, costimulation, or inflammation, they exponentially proliferate and differentiate into effector T-cell subsets within 2 weeks (36). After the alleviation of inflammation or the clearance of antigens, most effector T cells die, and a small proportion of activated T cells transform into memory T cells. Upon repeated stimulation, these memory T cells rapidly reactivate and perform effector T-cell functions (36). However, upon continual stimulation, effector T cells gradually lose their ability to produce an immune response and the memory phenotype, thus becoming functionally exhausted (36). Cytokine changes are a key component of the exhaustion phenotype. IL-2, interferon-γ (IFN-γ), and tumor necrosis factor-α (TNF-α) secretions gradually decrease during T-cell exhaustion. IL-2 is an essential cytokine for T-cell survival and the activation of effective cell-mediated immune responses to infection and tumor. Sustained IL-2 production is maintained in an antigen-rich environment, which enables T cells to harness a robust antigen response (15, 37). The definition of TEX remains controversial. The term was first used in the field of viral immunology where researchers observed exhaustion in CD8+ T cells during chronic lymphocytic choriomeningitis viral infection (14). This concept was later applied to the field of oncology. Exhaustion of T cells does not mean that T cells completely lose their function. Although TEX are observed to be completely devoid of effector function in many tumors (38), they still produce certain effector moieties, including inflammatory cytokines and granzymes (15). These inflammatory cytokines and granzymes can still play a role in killing tumor cells. T-cell exhaustion is an adaptation to chronic antigenic stimulation and prevents damage caused by an excessive immune response (39). Therefore, whether TEX are beneficial or harmful remains to be determined.
2.2 Biological characteristics of T-cell exhaustion
TEX are functionally distinct from effector and memory T cells. TEX exhibit persistently elevated expression levels of inhibitory receptors (IRs), such as PD-1, T-cell immunoglobulin and mucin domain 3 (TIM-3), lymphocyte activation gene 3 (LAG-3), CTLA4, and T-cell immunoreceptor with immunoglobulin and ITIM domains (TIGIT), and alterations in cytokines, epigenetic and transcriptional profiles, and metabolic patterns (15, 40, 41).
2.21 Elevated expression of IRs
Sustained high expression of multiple IRs is a hallmark of TEX. However, IRs can be expressed on effector T cells. Many IRs are consistently highly expressed on T cells, including PD-1, TIM-3, LAG-3, CTLA4, and TIGIT, which can negatively regulate T-cell function and properties (40). For example, a study including Korean patients with liver cancer reported that T cells with high PD-1 expression exhibited higher expression levels of exhaustion-related genes and produced low levels of type I IFN and TNF (42). Another study indicated that TIM-3 may cause CD8+ T-cell exhaustion, resulting in decreased production of T-cell killing factors, namely perforin and granzyme B (43). LAG-3 maintains an exhaustion-like pattern in CD8+ T cells, and LAG-3 deficiency can enhance CD8+ T-cell effector-like function (44). Another breast cancer study revealed that CTLA4 can negatively regulate CD8+ T-cell function (45). In colorectal cancer, TIGIT promotes CD8+ T-cell exhaustion, leading to poor prognosis (46). Under normal conditions, upon the clearance of antigens, IR expression can weaken T-cell activation and reduce autoimmune damage caused to the body. The expression level of IRs on T cells is low during normal conditions but high during chronic infection and cancer (47). Coexpression of multiple IRs has been observed in many animal models and human studies (48–51). To some extent, the degree of IR coexpression reflects the severity of T-cell exhaustion (52). IRs can regulate T-cell function through three mechanisms: (1) IRs sequester target receptors or ligands through ectodomain competition (53), (2) IRs decay signals from activated receptors (such as TCR and costimulatory receptors) by regulating intracellular mediators (54), and (3) IRs induce inhibitory gene expression (55). In conclusion, T-cell exhaustion is often accompanied by elevated IR expression.
2.2.2 Cytokines and T-cell exhaustion
Several inhibitory cytokines are present in the TME, including interleukin (IL)-10 and transforming growth factor-beta (TGF-β), both of which promote the generation of TEX (56). IL-10 is a STAT3-inducing cytokine that is often associated with attenuated T-cell activation (57). T-cell exhaustion can be prevented and reversed by blocking IL-10 (58, 59). IL-10 is produced by various immune cell types, such as dendritic cells, B cells, monocytes, CD8+ T cells, and nonregulatory CD4+ T cells (60). IL-10 can inhibit the antitumor activity of tumor cells and promotes T-cell exhaustion in breast cancer and B16 melanoma models (61). IL-10 may act directly on T cells through STAT-3, indirectly through the regulation of APC, or both (60). In a glioblastoma model, a study found that IL-10-driven T-cell exhaustion can be rescued by JAK/STAT inhibition (62). Another report found that the simultaneous blockade of the IL-10 and PD-1 pathways in mice synergistically reversed CD8+ T cell-exhaustion and enhanced viral control, which suggests that IL-10 plays a role in controlling CD8+ T-cell exhaustion (59). Another cytokine, TGF-β, attenuates or inhibits immune cell activation by activating downstream SMAD transcription (63). During acute infection, TGF-β acts as a negative regulator of effector function and upregulates the proapoptotic factor Bim by inhibiting the transcription factor T-box transcription factor 21 (T-bet) (64). TGF-β is often found to be highly expressed, and the TGF-β–SMAD2 signaling pathway is activated upon the formation of TEX during chronic antigenic stimulation (64, 65).
Other cytokines present in the chronic antigenic environment can inhibit T-cell exhaustion. IL-2 is a key cytokine necessary for T-cell survival and activation, enhancing infection and tumor immune responses. IL-2 is used in cancer therapy to enhance T-cell function (66). The combination of IL-2 with PD-1 pathway blockade can exert synergistic effects, and it may be an attractive strategy for tumor immunotherapy (67). However, IL-2 may exert opposite effects, resulting in the expansion of regulatory T cells (Tregs) (68). In HIV infection, although IL-2 increases the number of CD4+ T cells, it has a weak effect on CD8+ T cells and viral replication (68–71). Determining how IL-2 therapy can be used to suppress TEX while attenuating its effects on Treg expansion may help in modulating T-cell exhaustion in cancer. IL-21 is another γ chain cytokine similar to IL-2 that is mainly produced by follicular helper CD4+ T (Tfh) cells, thus directly promoting the expression of basic leucine zipper ATF-like transcription factor (BATF), which is involved in initiating or maintaining effector CD8+ T-cell function (72). In the absence of IL-21R signaling, CD8+ T cells fail to respond, suggesting that IL-21 plays a role in antagonizing effector T-cell exhaustion (73, 74). However, studies using IL-21 to treat T-cell exhaustion are scarce. The use of IL-21 to reverse T-cell exhaustion and enhance antitumor immunity may be a beneficial strategy. Other inflammatory cytokines, such as type I IFN, are associated with T-cell exhaustion. IFN-α/β are key proinflammatory cytokines that can directly induce antiviral activity and activate innate immunity (75). IFN-α/β signaling can antagonize the exhausted T-cell progenitor pool by affecting the transcription factor T-cell factor 1 (TCF1), thus inhibiting T-cell exhaustion (76).
2.2.3 Transcriptional regulation of TEX
The epigenetic characteristics of TEX markedly differ from those of effector and memory T cells. Many transcription factors, including interferon regulatory factor 4 (IRF4), BATF, nuclear factor of activated T cells (NFAT), nuclear receptor subfamily 4 group A (NR4A), thymocyte selection-associated high mobility group box (TOX), and TCF1, which constitute a transcription factor regulatory network, are involved in T-cell exhaustion (Figure 1).
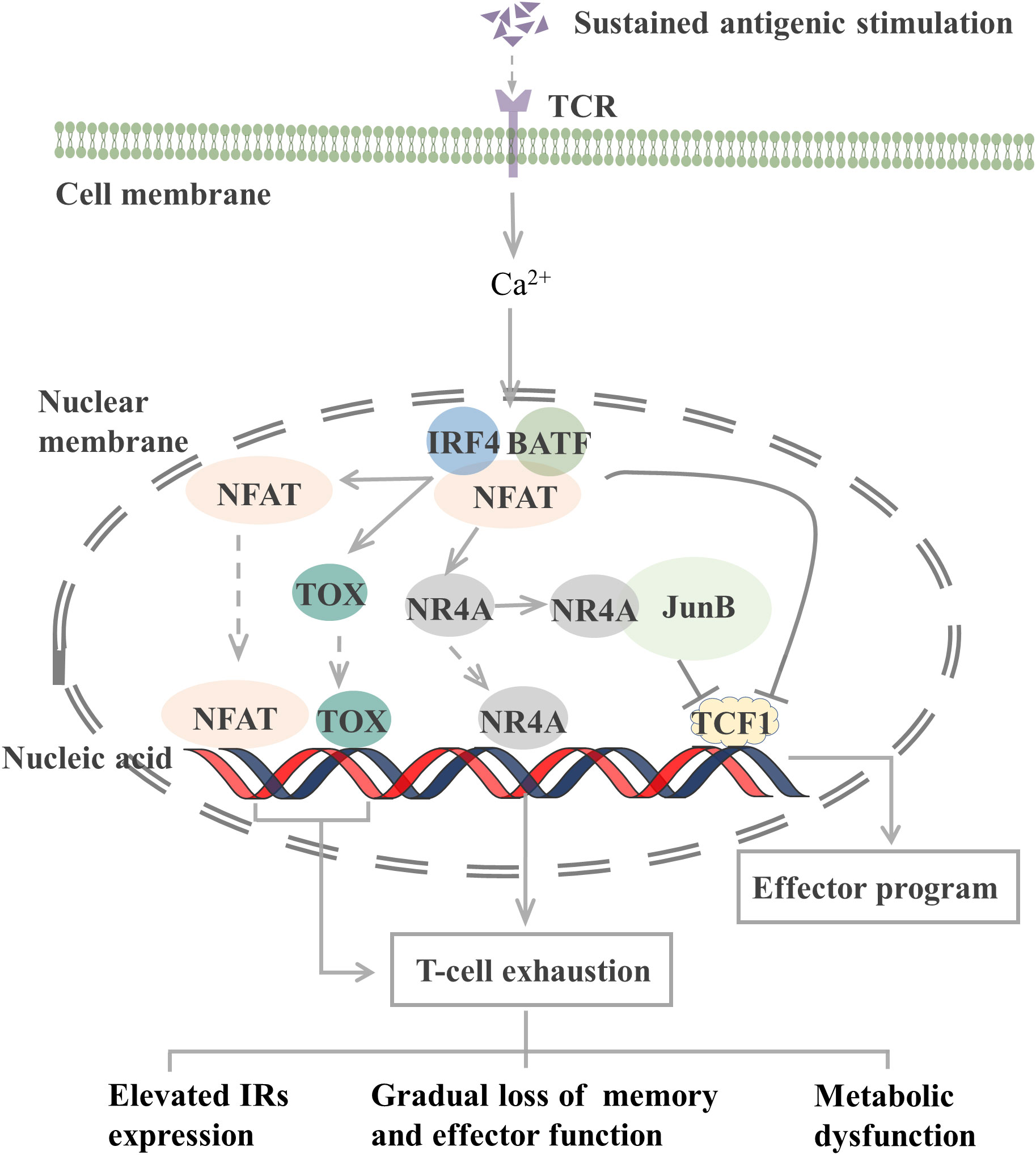
Figure 1 Transcription factors involved in the regulation of T cell exhaustion. The TCR signaling pathway activates the NFAT-BATF-IRF4 complex through calcineurin signaling, and the subsequent NFAT, TOX, and NR4A binding to the genome initiates T-cell exhaustion. When AP-1 is present, NR4A binds to it and represses TCF1 gene expression. In addition, the NFAT-BATF-IRF4 complex also inhibits TCF1 gene expression, which activates the effector function. T-cell exhaustion exhibits elevated IRs expression, metabolic dysfunction, and gradual loss of memory and effector function. TCR, T-cell receptor; NFAT, nuclear factor of activated T cells; BATF, basic leucine zipper ATF-like transcription factor; IRF4, Interferon regulatory factor 4; TOX, thymocyte selection-associated high mobility group box; NR4A, Nuclear receptor (NR) subfamily 4 group A; TCF1, T cell factor 1; IRs, inhibitory receptor. JunB, AP-1 transcription factor subunit.
TCR-induced calcineurin signaling activates the transcription factors of the NFAT family and then interacts with other transcription factors, including activator protein-1 (AP-1), to control T-cell activation and effector cell differentiation. However, an elevated expression level of NFAT protein can drive CD8+ T-cell exhaustion (77). CD8+ T cells lacking NFAT fail to express exhaustion-related IRs. By preventing NFAT from interacting with AP-1, researchers determined that NFAT drives T-cell exhaustion by binding to sites in other genomic regions (77). Another study reported that the use of the calcineurin inhibitor FK506 to inhibit NFAT nuclear translocation and its transcriptional activity reduced the expression of IRs associated with T-cell exhaustion, including PD-1 and LAG-3, and increased the expression of TCF1, which is normally expressed on precursor exhausted T cells (TPEX, a population of T cells that maintain stemness) (78).
NFAT, together with BATF and IRF4, participates in a self-reinforcing transcriptional circuit, establishing a transcriptional network that promotes T-cell exhaustion during chronic infection and the transcription of Pdcd1 to produce PD-1 (79). BATF is a member of the AP-1 family of transcription factors, and when it dimerizes with JunB, it can inhibit the activity of other AP-1s and promote T-cell exhaustion. Silencing of BATF reversed the function of TEX in patients with HIV (55). IRF4 is a TCR signaling-sensitive transcription factor, and high IRF4 expression is a feature of TEX. IRF4 causes IR expression in TEX, impairs cytokine secretion, and inhibits anabolism. Low expression of IRF4 results in an overall increase in anabolism and a partial recovery of IFN-γ secretion (79). However, in a mouse tumor study, BATF and IRF4 synergistically shifted the phenotype and transcriptional profile of CAR-T cells from exhaustion to enhanced effector function, thus improving their antitumor response (80). In different microenvironments, transcription factors may exert different effects on T cells.
The NR4A family (orphan nuclear receptor family), including NR4A1, NR4A2, and NR4A3, and TOX are transcription factors downstream of NFAT that are involved in the development of T-cell exhaustion. When AP-1 protein is deficient, NFAT can activate TOX, TOX2, and NR4A families to induce the expression of IRs, such as PD-1, and inhibit the expression of effector molecules (81). Thus, the TOX and NR4A families are crucial transcription factors that limit the activity of effector T or CAR-T cells. CD8+ T cells express high levels of NR4As in tumors, and knockout of all three NR4As in CAR-T cells inhibited T-cell exhaustion, thus promoting tumor regression and prolonging survival in tumor-bearing mice (82). In the presence of AP-1, NR4A1 preferentially binds to AP-1 and inhibits its function to suppress effector gene expression. NR4A1 deletion inhibits T-cell exhaustion and enhances T-cell killing of tumors (83). Loss of TOX results in a reduction in the number of TCF1+ TIM-3+ progenitors that deplete CD8+ T cells. Knocking out TOX in mice reduced the expression of immune checkpoint molecules in immune cells and increased the expression of effector molecules (84). Taken together, both the NFAT-NR4A and NFAT-TOX pathways can cause T-cell exhaustion, which may be reversed by blocking these signaling.
2.2.4 Metabolic programming of TEX
The metabolic programming of TEX differs from that of effector or memory T cells. TEX often exhibit metabolic dysfunction, such as respiratory chain inhibition, glucose uptake inhibition, and mitochondrial energy dysregulation (85). The PI3K, AKT, and mTOR signaling pathways play vital roles in T-cell development, function, and stability. When the inhibitory receptor PD-1 is upregulated in T cells, it suppresses the PI3K, AKT, and mTOR signaling pathways, leading to an exhausted T-cell state (86, 87). These metabolic disorders are associated with the increased expression of some IRs, such as CTLA4 and PD-1, which may exert inhibitory effects by regulating CD28 signaling (87–89). When PD-1 is blocked, the mTOR pathway is activated in TEX and anabolic and glycolytic pathways are reactivated (90). In addition, tumor cell competition for glucose, oxygen, and other nutrients may promote T-cell exhaustion (40). Integrative omics analyses of liver cancer revealed the oncogenic reprogramming of hepatocellular carcinoma methionine recycling with elevated 5-methylthioadenosine and S-adenosylmethionine (SAM) expression to be closely linked to T-cell exhaustion (91). In conclusion, understanding the metabolic reprogramming of T-cell exhaustion can help in reversing the state of exhaustion and improving cancer immunotherapy.
2.3 “Stemness” in T-cell exhaustion
T-cell exhaustion is a gradual development process. The exhausted T cell pool is heterogeneous and consists of two phenotypically and functionally distinct subpopulations, including TPEX and TEX (Figure 2). Compared with TEX, TPEX are the class of stem cell-like subpopulations that maintain self-renewal, long-term proliferation, and differentiative capacity, which are beneficial for maintaining long-term antigen-specific T cell responses (92–94). TPEX exhibit characteristics of an early exhausted phenotype, such as high expression of IRs (such as PD-1, LAG-3), and an impaired ability to secrete cytokines, IFN-γ, and TNF. However, TPEX exhibit characteristics of stemness. TPEX can perform self-renewal capacity and express factors associated with memory T cells, such as the transcription factors, TCF1 and BLIMP1 (92, 95, 96). TPEX subsets have the potential to proliferate and differentiate into terminally exhausted effector T cells, which can continually replenish the terminally exhausted effector T cell pool to maintain T cell response function. It was reported that TPEX have a more diversified TCR repertoire, and TPEX differentiate into TEX upon stimulation by a large number of antigens (92, 93). Recently, researchers found that the transcription factor MYB regulated the lifespan and limited function of TPEX, and revealed that ICB treatment-induced cytotoxic T cell proliferation burst and increased effector function depended on TPEX and that increased TPEX cell frequency is associated with increased survival (97). In general, adoptively transferred TPEX mediates long-term inhibition of tumor growth and has become tumor therapeutic interventions (98, 99). In addition, TPEX show similar characteristics to CD4+ follicular helper T cells, CD8+ memory precursor cells, and hematopoietic stem cell progenitors (98). TPEX express the chemokine (CXC motif) receptor CXCR5 and the co-stimulatory molecule ICOS, which is beneficial for TPEX to interact with B cells in lymphoid tissues and maintain its effector functions. Furthermore, TPEX initiate a transcriptional program driven by TCF1/Bcl-6 to suppress T-cell exhaustion and maintain stemness characteristics, which suggests that this subset retains a long-lived memory potential. These regulations of TPEX may be essential for persistent antigen-specific T-cell responses (76, 100, 101). These findings indicated that it is possible to develop therapies against chronic viral infections and cancer by TPEX cells. As the central mediators of immune checkpoint blockade and TEX pool replenishment in chronic antigen stimulation, TPEX subsets can play key roles in the design of novel immunotherapeutic approaches. Thus, further understanding of TPEX may improve the prognosis of cancer therapy.
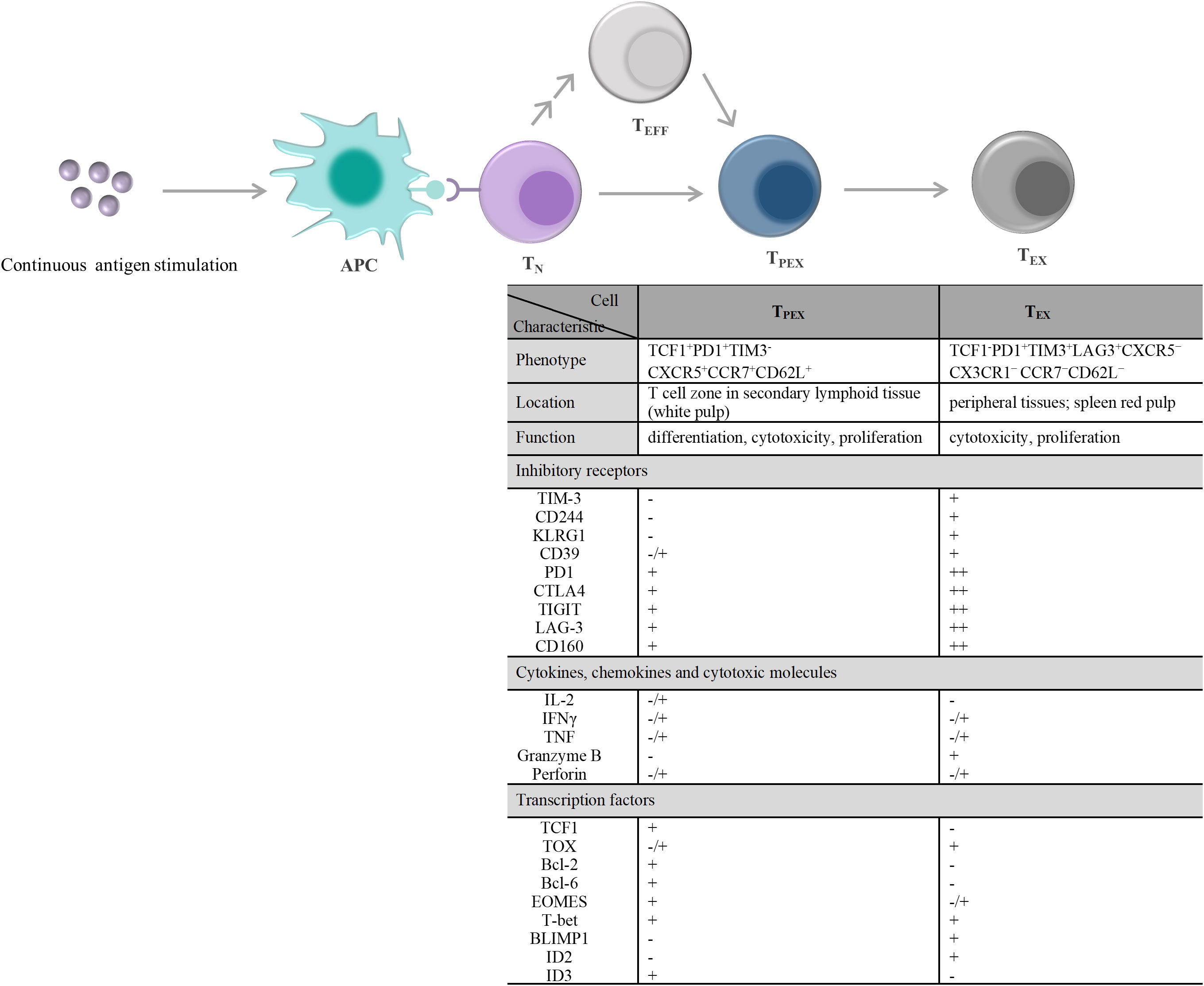
Figure 2 T cell exhaustion caused by continuous antigen stimulation and its alterations in the characteristic molecules. TN exposure to persistently high amounts of antigen stimulation, including chronic infections and tumors. On the one hand, TN differentiate into PD1+TCF1+ TPEX cells, and then TPEX continuously generate PD1+TCF1– TEX cells. On the other hand, TEFF generated by TN differentiation and/or produced by each stage of T cell differentiation also undergo the same T cell exhaustion. During exhaustion, there displayed a significant qualitative or quantitative difference about the inhibitory receptors expressed on the cell surface, the secretions of cytokines, chemokines, and cytotoxic molecules, and the transcription factors expression between TPEX and TEX. - denotes “lack of expression”, -/+ denotes “heterogeneous expression”, + and + + denote “level of expression”. APC, antigen-presenting cell; TN, naïve T cells; TEFF, effector T cells; TPEX, precursor exhausted T cells; TEX, exhausted T cells; CTLA4, cytotoxic T lymphocyte antigen 4; TIGIT, T-cell immunoreceptor with immunoglobulin and ITIM domains; TIM-3, T-cell immunoglobulin and mucin domain 3; CXCR5, C-X-C motif chemokine receptor 5; CCR7, C-C motif chemokine receptor 7; PD1,programmed cell death 1; LAG-3, lymphocyte activation gene 3 protein; IL-2, interleukin-2; IFN, interferon; TNF, tumor necrosis factor; TCF1, transcriptional regulator T cell factor 1; TOX, thymocyte selection-associated high mobility group box; Bcl, B-cell lymphoma; EOMES, eomesodermin homologue; T-bet, T-box transcription factor; BLIMP1, B lymphocyte-induced maturation protein 1; ID, inhibitor of DNA binding.
3 T-cell stemness
With the progression of T-cell exhaustion, the therapeutic efficacy of antitumor immunotherapy becomes limited, resulting in persistent infections and leading to inefficient cancer control (102). Some T cells exhibit stem cell-like characteristics that are believed to be the key to triggering a robust, long-lasting antitumor response (27, 28, 103). By using the graft-versus-host disease mouse model, Zhang et al. identified a population of T cells expressing CD44loCD62Lhi along with high expression of stem cell antigen-1, the antiapoptotic molecule B-cell lymphoma-2 (Bcl-2), and CD122 (IL-2 and IL-15 receptor β) in CD8+ T cells and named them TSCM (104). Human TSCM cells exhibited a CD45RA+CD45RO-CCR7+CD62L+CD27+CD28+CD127+CD95+ phenotype similar to that of TN (CD45RA+CD45RO-CCR7+CD62L+CD27+CD28+CD127+ CD95-), and expressed high levels of CD122, CXCR3, CXCR4 and leukocyte function-associated antigen-1. TSCM rapidly acquired effector functions upon TCR stimulation, which is relevant to the development of vaccines and T-cell therapy (28). Among all circulating T cells, TSCM are a relatively small subset, accounting for only 2% to 3% of total T cells (28). TSCM are characterized by their long lifespan and enhanced self-renewal and proliferation as well as their ability to replenish more differentiated memory and effector T-cell subsets (28, 104, 105). These characteristics make TSCM a particularly attractive candidate for T-cell adoptive cancer immunotherapy. In particular, the strong stemness profile of TSCM and their superior antitumor effect compared with those of other memory T lymphocytes make TSCM potentially more suitable for immunotherapy (106). Therefore, we will mainly focus on the role of TSCM in tumor immunity in this review.
3.1 Stem cell-like characteristics of TSCM
3.1.1 Long lifespan
TSCM can stably exist for a long time and survive longer than other memory subsets (105, 107). Genetically modified TSCM can survive for decades in the human body while retaining their function and differentiation potential (108, 109). Pedro et al. reported the presence of dynamic heterogeneity in the human TSCM pool. The TSCM population consists of two subsets. The first subset includes the long-lived subset with dynamic properties required to maintain CD4+ and CD8+ memory T cells that have a half-life of approximately 9 years and a high degree of self-renewal. Another subset has a very short average clone life (half-life = 5 months) in the TSCM pool. This high turnover rate causes difficulty in the formation of a stem cell-like population (110). The kinetic difference in the TSCM pool may be related to naïve T-cell (TN) differentiation, which is affected by the type of antigen stimulation. Approximately 50% of TSCM are differentiated from TN. Continual low-level exposure to novel environmental antigens and persistent antigens leads to continual TN differentiation (110, 111). TSCM are derived from thymic output (CD95- TN) and cell proliferation induced by antigen stimulation (28). Due to age-dependent thymic degeneration, the number of TN cells decreases, while the number of memory-differentiated T cells accumulates and the frequency of TSCM cells is in a state of dynamic flux throughout the human lifespan (105, 112, 113). Interestingly, one study found that at advanced age and after the emergence of chronic inflammation, the Wnt/β-catenin pathway is impaired, and CD4+ TSCM decrease (114). Therefore, future studies can determine how to characterize true TSCM subsets, analyze the functions of the subsets, and determine their targeted induction.
3.1.2 Enhanced proliferation
TSCM exhibit a substantial proliferative capacity, which increases the proliferation and survival of T cells after xenotransplantation compared with naïve and traditional memory subpopulations, enhances antitumor activity, and eventually triggers tumor regression and cure (27, 28, 104, 115). Stem cells replicate through asymmetric division to promote diversified differentiation, meaning that one daughter cell maintains the characteristics of the original parent cell and the other daughter cell further differentiates into other types of cells (116). T cells undergo asymmetric division similar to that of stem cells. The intensity of the TCR signal during cell division is a key factor for regulating T-cell asymmetric division (117). T cells generate two different cell types through asymmetric division: the distal daughter and proximal daughter. The distal daughter retains long-term developmental plasticity, self-renewal, and differentiation capacity, similar to long-lived memory cells. The proximal daughter responds to antigen exposure (118–120). Memory T-cell subsets share the same ability to divide asymmetrically (33, 121, 122). TSCM are a unique subpopulation of memory T cells. Therefore, TSCM might divide in a similar manner to maintain a stable number, but the exact mechanism remains unexplored.
3.1.3 Enhanced capacity for self-renewal and pluripotent differentiation
One early study found that TSCM are capable of self-renewal and differentiate into various memory T cells (115). Many studies support the linear lineage model of memory T-cell differentiation: TN→ TSCM →TCM→TEM→ effector T cells (TEFF) (33, 123, 124) (Figure 3; Table 1). It is acknowledged that there is heterogeneity in each stage of T cell differentiation, and the cells in the differentiation process also undergo proliferation and differentiation upon antigenic stimulation and exert their memory or effector functions. For example, the lineage model was proposed based on the progressive differentiation of the stem-like memory T cell subpopulation in circulation, in which TN first transform into memory T cells and then transition to effector T cells, thus the other T cell populations in peripheral tissues are not involved (25, 123). TSCM represent the least differentiated long-lived memory T-cell subset with pluripotency to differentiate into multiple memory T-cell subsets (104, 107). TSCM have the properties of traditional memory T cells, which can rapidly generate effector cytokines and enhance cellular immune effects upon secondary antigen stimulation, suggesting that TSCM are the key to maintaining long-term immune memory (28). Therefore, the translational application of TSCM in the development of new vaccines or adoptive T-cell therapy is promising. Most information on TSCM has been obtained by studying CD8+ T cells. However, Muranski and colleagues reported that Th17 cells, a CD4+ T-cell subset, are a long-lived memory T-cell population with an enhanced self-renewal capacity. Th17 cells generate different Th lineages and retain stem cell-like molecular properties similar to early memory CD8+ cells through the coordination of HIF1α/Notch/Bcl-2 and promote long-term immunity (131–133). Adoptive transfer therapy of tumor antigen-specific CD4+ T cells during cancer immunotherapy is promising.
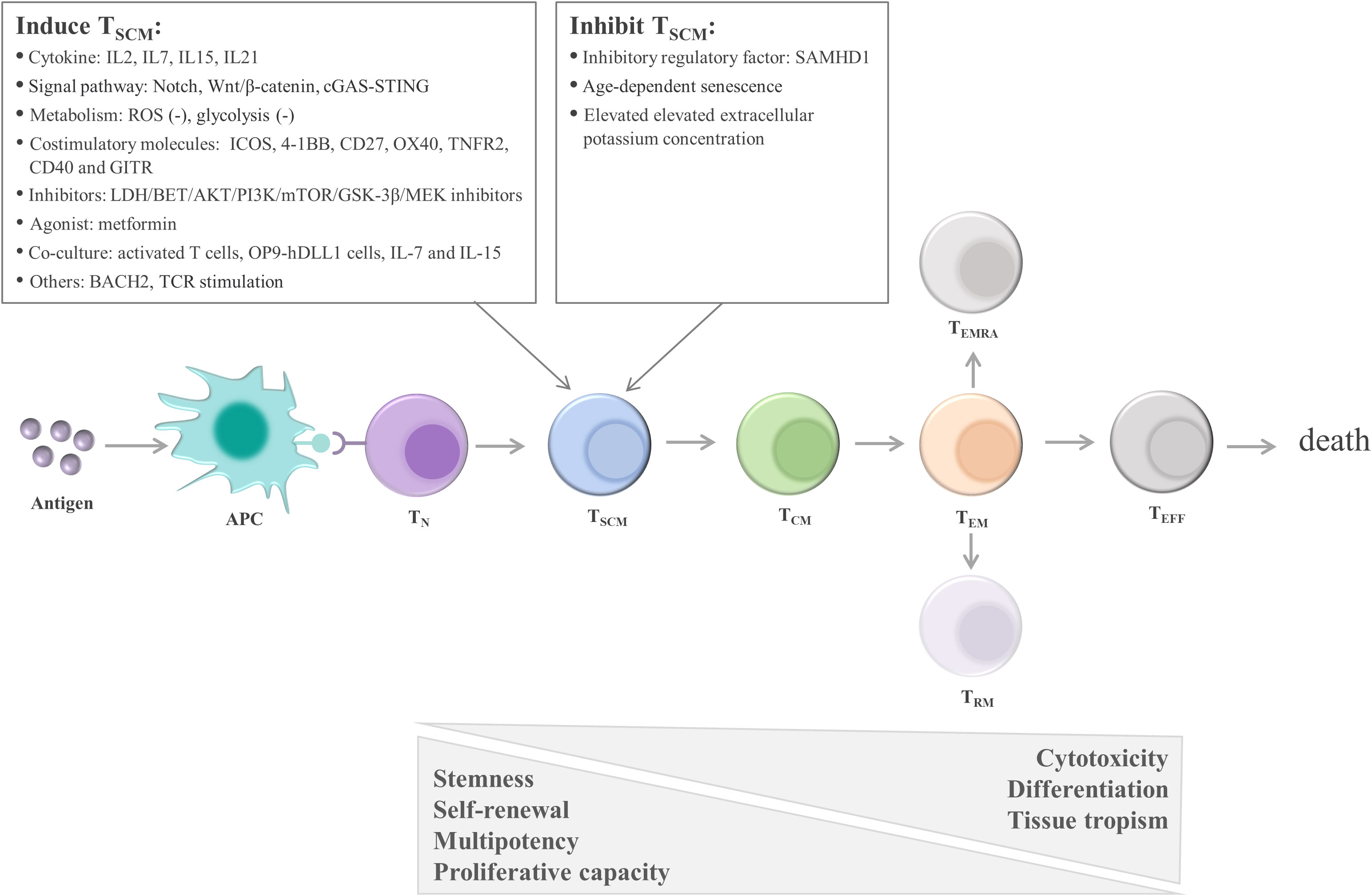
Figure 3 Linear lineage model and related regulatory factors in TSCM cell differentiation. After exposure to antigens, TN undergo proliferation, expansion, and differentiation. The differentiation process of TSCM follows the known linear lineage model, that is, TN→ TSCM → TCM → TEM → TEFF, eventually experiencing death. Furthermore, during T-cell exhaustion, TPEX continuously replenish the terminally differentiated effector TEX. TSCM are regulated by multiple factors, including cytokines, signaling pathways, metabolic factors, and costimulatory molecules. These factors regulate TSCM mainly by promoting the transition of TN to TSCM and inhibiting the differentiation of TSCM. APC, antigen-presenting cell; TN, naïve T cells; TSCM, stem cell-like memory T cells; TCM, central memory T cells; TEM, effector memory T cells; TEFF, effector T cells; TEX, exhausted T cells; TPEX, precursor exhausted T cells; SAMHD1, SAM and HD domain-containing protein 1; IL, interleukin; ROS, reactive oxygen species; ICOS, inducible T-cell costimulatory; 4-1BB, TNF receptor superfamily member 9; OX40, TNF receptor superfamily member 4; LDH, lactate dehydrogenase; BET, bromodomain and extra terminal domain; AKT, serine/threonine kinase; PI3K, phosphatidylinositol 3-kinase; mTOR, mechanistic target of rapamycin kinase; GSK-3β, glycogen synthase kinase-3β; MEK, mitogen-activated protein kinase kinase; OP9‐hDLL1 cells, OP9 cells expressing Notch ligand, Delta‐like 1; BACH2, the transcription repressor BTB domain and cnc homolog 2; TCR, T-cell receptor.
3.2 Role of TSCM in immune response and antitumor immunity
TSCM are a double-edged sword in immune response. CD4+ TSCM serve as a long-term viral reservoir for HIV type 1 (HIV-1) infection/latent infection, providing a stable host for HIV and functionally promoting long-term viral persistence in patients receiving highly active antiretroviral therapy (134). In adult T-cell leukemia (ATL), a T-cell malignant tumor caused by human T-cell leukemia type-1 (HTLV-1), the TSCM population is susceptible to HTLV-1 infection. TSCM are central to ATL initiation and maintenance and participate in ATL development by continually populating ATL clones (135). In addition, in autoimmune diseases, such as type 1 diabetes mellitus, systemic lupus erythematosus, immune thrombocytopenia, and rheumatoid arthritis, autoreactive TSCM exhibit a high clonal expansion capacity and produce proinflammatory cytokines, leading to chronic autoimmune destruction and disease progression (136–139). In conclusion, the TSCM pool might play a pathogenic role in T cell-mediated disease and contribute to disease maintenance and persistence. Thus, TSCM can be a target for immunotherapy, and eradication of antigen-specific TSCM can be the key to successful cures.
The long-term protective effect of TSCM against acute and chronic infection has been demonstrated. Vaccination with virus-specific vaccines induced the production of TSCM, which can provide long-lasting immune protection (109). In addition, CD8+ TSCM can control viral growth and enhance immune function, thus preventing the progression of chronic HIV-1 infection and improving clinical prognosis (140). In patients with chronic Chagas disease, CD8+ TSCM were involved in filling the T-cell pool that controlled infection, and the number of TSCM was negatively correlated with the severity of disease (141). The heterogeneous outcome resulting from these TSCM is critical for immunotherapy, suggesting that clinicians should evaluate the immune effects of this subset on patients during treatment, understand the pathogenesis of the disease, and administer individualized treatment by specifically promoting or inhibiting TSCM, which may otherwise lead to an exacerbated immunosuppressive phenotype.
TSCM exert strong and sustained antitumor effects (28, 142). Researchers have evaluated the effect of T-cell differentiation status (TSCM, TCM, and TEM) on tumor therapeutic efficacy. They found a significant negative correlation between T-cell differentiation status and antitumor efficacy in vivo. The lower the differentiation degree of T-cell subsets is, the more significant the antitumor effect is. The order of their therapeutic effect is TSCM > TCM > TEM (106). Furthermore, in the mesothelioma mouse model, human-derived mesothelin-specific TSCM exhibited enhanced antitumor activity (28). In the melanoma mouse model, TSCM caused a sustained decrease in tumor growth, which was characterized by a significant increase in the overall survival rate (142). In the local lymph nodes of patients with non-small-cell lung cancer, CD4+ TSCM were increased in number and function and produced IFN-γ, which may be related to sustained antigen stimulation or exposure to the TME. However, the mechanism by which such CD4+ TSCM exert antitumor effects remains unclear (109). In cervical cancer caused by human papillomavirus (HPV) infection, HPV16-specific CD8+ TSCM can induce long-term antitumor immunity and significantly inhibit tumor growth (143). The powerful immune reconstitution potential of TSCM indicates that TSCM can be useful in cancer treatment. Further exploration of TSCM and their regulation may contribute to the design and clinical development of adoptive T-cell therapy and provide new directions for further improvement of adoptive T-cell immunotherapy.
3.3 Factors that promote TSCM generation and enhance TSCM stemness
3.3.1 Cytokines
TN can be differentiated into TSCM in vitro. For example, stimulation with IL-2, IL-7, IL-15, and IL-21 promoted the enrichment and proliferation of tumor antigen-specific TSCM, which exhibited more distinct heterogeneous differentiation and self-renewal potential than did the original TSCM isolated in vivo (144–147). In addition, lactate dehydrogenase inhibitors synergize with IL-21, thus promoting CD8+ TSCM formation (148).
3.3.2 Signal pathways
TSCM phenotypes can be induced in vitro by suppressing genes related to T-cell differentiation, such as T-bet, BATF, and eomesodermin, and upregulating genes related to stemness, such as TCF1 and lymphoid enhancer-binding factor 1 (LEF1) (149). For example, the use of Notch signaling which induces the generation of antigen-specific TSCM with enhanced antitumor effects (150), pharmacological inhibition of PI3K/Akt/mTOR signaling (115, 151–154), restriction of reactive oxygen species metabolism with antioxidant N-acetylcysteine (155), and targeted inhibition of the glycolytic pathway (156). Moreover, pharmacological inhibition of bromodomain and extraterminal domain protein results in the downregulation of BATF, which is necessary to initiate effector CD8+ T-cell differentiation (157), and subsequently inhibits further differentiation of TSCM and improves T-cell persistence in the ACT immunotherapies (158). Moreover, weakening T-cell receptor signal intensity facilitates the formation of TSCM with enhanced antitumor effects (145, 159).
TWS119, an inhibitor of glycogen synthase kinase-3β (GSK-3β), induces the activation of the Wnt/β-catenin signaling pathway. Subsequently, TWS119 inhibits CD8+ T-cell proliferation and blocks TSCM cell differentiation by inducing the expression of TCF1 and LEF1, thus regulating the stemness of CD8+ T cells and promoting TSCM cell generation. GSK-3β inhibitors/Wnt signaling agonists can be used as adjuvants to improve the stemness characteristics of TSCM (29, 115, 160, 161). Inhibition of GSK-3β increases the cytotoxic capacity of CD8+ TSCM and may indirectly mediate antitumor effects by enhancing their proliferation and differentiation into terminally differentiated CD8+ T cells (162). Moreover, cGAS-STING-driven type I IFN signaling promotes the differentiation of TN into TSCM subset and improves the efficacy of CAR-T therapy in mice by increasing TCF1 expression (163). Pearce et al. reported that metformin treatment activated AMP-activated protein kinase, promoting the generation of memory T lymphocytes in infection and tumors and thus enhancing protective antitumor immunity (164). In addition, MEK inhibitors increased TSCM cell generation and antitumor effects by delaying T-cell division and enhancing mitochondrial biogenesis and fatty acid oxidation (165).
3.3.3 Noncytokine factors
The costimulatory molecules ICOS, 4-1BB, and other T cell costimulatory molecules CD27, OX40(CD134), TNFR2, CD40 and GITR in TNFRSF (143, 166–169) and the transcription repressor BTB domain and CNC homolog 2 (BACH2) (170, 171), as the key regulators of stem cell-like T-cell subsets, induce the generation of stem cell-like lineages. Furthermore, the duration and intensity of the antigenic peptide/MHC-TCR interaction can affect TSCM expansion and maintenance. CD8+ T cells transiently stimulated by CD3/CD28 antibodies have a more abundant TSCM subset and exhibit greater cytokine production capacity (145, 159, 172). An appropriate TCR signal-stimulation intensity can induce TSCM production to the greatest extent (173). Tumor antigen-specific TSCM can be transformed from TN. In addition, activated T cells can be transformed into TSCM-like cells. Coculture of activated T cells with OP9-hDLL1 cells, IL-7, and IL-15 efficiently generated TSCM with strong antitumor activity (174). The aforementioned findings demonstrate that TSCM can be induced in vitro or in vivo and used to promote cancer immunotherapy. Therefore, exploiting guidance signals to induce the formation and expansion of TSCM to supplement the effector T-cell pool represents a novel strategy to improve T cell-based tumor immunotherapy and enhance antitumor effects.
3.4 Factors associated with TSCM inhibition
SAM and HD domain-containing protein 1 (SAMHD1), a deoxyribonucleoside triphosphate triphosphohydrolase, is an active restriction factor in CD4+ TSCM. During HIV-1 infection, a proportion of CD4+ TSCM exhibit increased sensitivity to HIV-1 infection by expressing the HIV core receptors CCR5 and CXCR4. SAMHD1 exerts an inhibitory effect on CD4+ TSCM by inhibiting viral replication and transcription in CD4+ TSCM by hydrolyzing the active metabolite, nucleotide triphosphate (175). Moreover, the number of TSCM in peripheral circulation markedly declines with age (176). During aging, the aggravation of inflammation and chronic infection affects the distribution of CD4+ T-cell subsets. Moreover, persistent stimulation can lead to the differentiation of CD4+ TSCM into a proinflammatory state, with impaired signaling of genes related to the generation of TSCM, particularly the Wnt/β-catechin pathway, thereby disrupting the genetic signature and homeostasis of TSCM (114). Future studies can identify other factors that impair TSCM cell function and elucidate the regulatory mechanisms of TSCM to promote or restore the function of TSCM. Therefore, Various factors related to TSCM cell inhibition can be used as a target to improve the immune response and thus improve the efficacy of immunotherapy.
3.5 Other factors affecting T-cell stemness
As tumor cells die, K+ is released into the extracellular space, resulting in a substantial increase in potassium gradients in the TME. The elevated extracellular K+ concentration limits T-cell terminal effector differentiation while maintaining CD8+ T-cell stemness and enhancing antitumor function, suggesting that altered ion gradients in the TME are crucial for T-cell stemness and antitumor immunity (177, 178). Other immune and stromal cells also have been reported to affect T-cell stemness (179). The complex intercellular interactions in the immune system suggest the importance of these cells for the induction or maintenance of immune function in TSCM. However, current research in this field is scant, and future studies on this topic are warranted.
4 Targeting T-cell exhaustion and TSCM to enhance antitumor immunity
4.1 Reverse T-cell exhaustion and restore tumor-infiltrating T cells in the TME
4.1.1 Targeting immune checkpoint receptors
High expression of TIL inhibitory receptors, which may be affected by the TME, leads to the increased expression of immune checkpoint receptors and promotion of T-cell exhaustion. T cells with the exhausted phenotype cannot produce effector factors to successfully target tumor cells, resulting in the decreased secretion of IL-2 and IFN-γ (46). TEX characteristically express TIM-3, LAG-3, TIGIT, and PD-1 (46). Therefore, targeting these immune checkpoints may effectively alleviate T-cell exhaustion. Blocking the binding of PD-1 and its ligand PD-L1 and CTLA4 can significantly improve the killing function of T cells, and immune checkpoint inhibitor (ICB) monoclonal antibodies have been approved by the Food and Drug Administration for clinical use (180–182) (Figure 4).
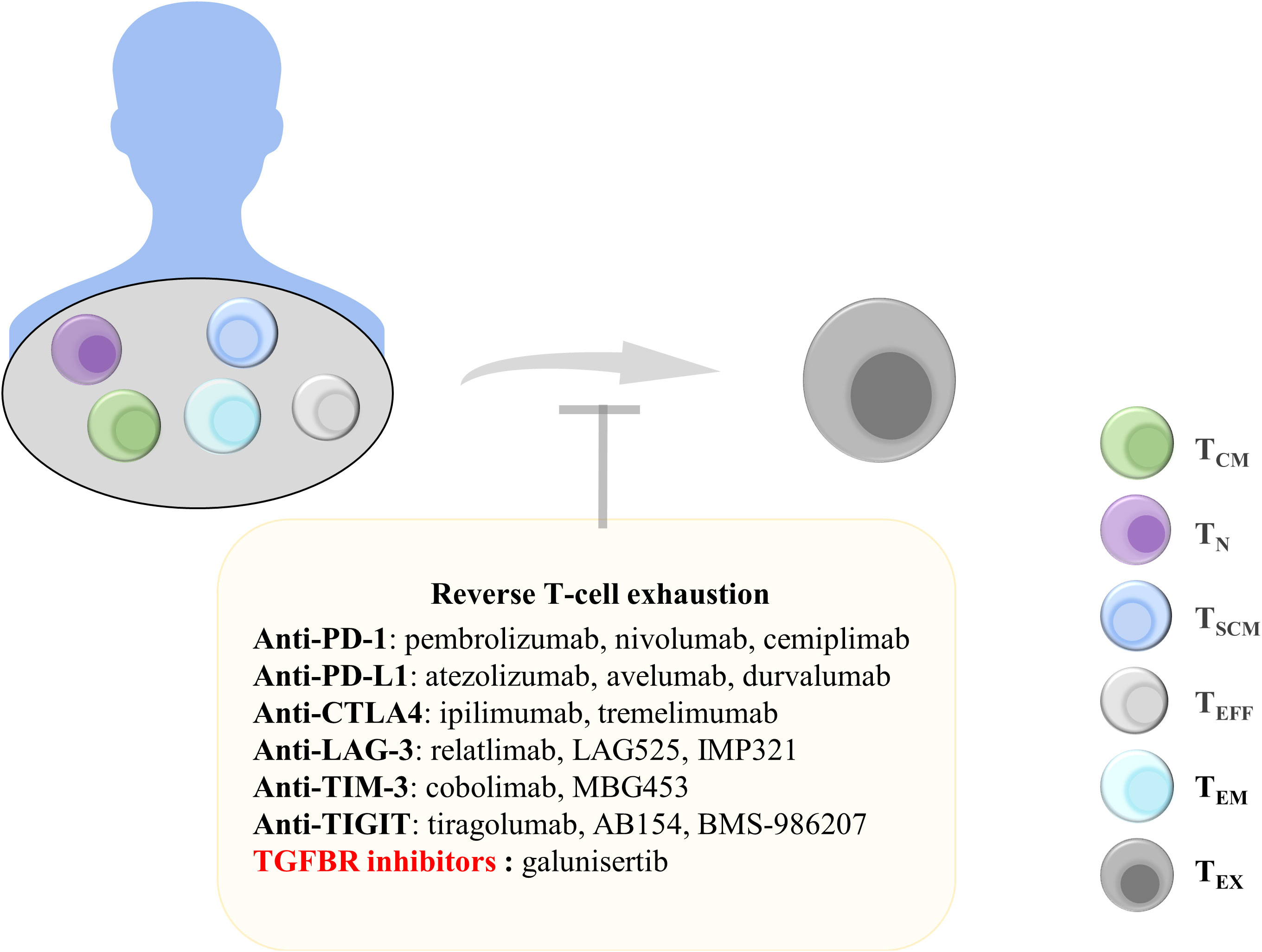
Figure 4 Treatments to reverse T-cell exhaustion. Reversing T-cell exhaustion by using anti-PD-1, anti-PD-L1, anti-CTLA4, Anti-LAG-3, Anti-TIM-3, Anti-TIGIT, and TGF-β inhibitors can prevent the generation of TEX. TCM, central memory T cells; TN, naïve T cells; TSCM, stem cell-like memory T cells; TEFF, effector T cells; TEM, effector memory T cells; TEx, exhausted T cells; Anti-PD-1, anti-programmed cell death protein 1; PD-L1, programmed death ligand 1; CTLA4, cytotoxic T lymphocyte antigen 4; LAG-3, lymphocyte Activation Gene 3; TIM-3, T-cell immunoglobulin and mucin domain 3; TIGIT, T-cell immunoreceptor with immunoglobulin and ITIM domains; TGFBR, transforming growth factor-beta receptor.
PD-1 is an inhibitory receptor expressed on TEX. Blocking PD-1 and PD-L1 binding can promote the effector function of CD8+ T cells. A study examining peripheral blood samples from patients with advanced non-small-cell lung cancer receiving PD-1-targeted therapy reported that most patients exhibited an increase in the number of PD-1+CD8+ T cells after treatment and that this increase was correlated with treatment benefits (183). Nivolumab and pembrolizumab, which are PD-1 monoclonal antibodies, have been approved for melanoma treatment (184, 185). In addition, atezolizumab has been approved as a PD-L1 antibody for the treatment of uroepithelial carcinoma, and it exhibited significant therapeutic effects when used clinically (186). Tumor regression was observed in mice with pancreatic tumors that received a combination of an anti-PD-1 antibody and an OX40 agonist after 225 days. The combination therapy reduced the proportion of Tregs and TEX in pancreatic tumors (187). In addition, the combined targeting of PD-1 and TIM-3 counteracted CD8+ T-cell exhaustion and restored T-cell function (188). Anti-PD-L1 antibodies can also block the binding of PD-L1 to PD-1, thereby inhibiting the exhaustion of T cells. Combination therapy with anti-PD-L1 and the tyrosine kinase inhibitor nilotinib can reduce T-cell exhaustion markers in leukemia and significantly improve the long-term survival rate of mice with leukemia (189). Anti-PD-1 and anti-PD-L1 therapies can reduce T-cell exhaustion and achieve superior treatment outcomes. Therefore, anti-PD-1 and anti-PD-L1 treatment strategies are still being explored to identify better therapeutic effects.
CTLA4 competes with CD28 to bind to B7 molecules; thus, T cells cannot receive costimulatory signals necessary for activation (190). CTLA4 is another valuable target for restoring T-cell exhaustion. The monoclonal antibody ipilimumab that targets CTLA4 is approved for the treatment of melanoma. The 5-year survival rate was significantly higher in patients with advanced melanoma receiving ipilimumab in combination with nivolumab than in patients receiving monotherapy (191). ICB use can result in satisfactory outcomes in patients with cancer. The discovery of additional inhibitory targets on TEX, including LAG-3 and TIGIT, can enable the development of targeted therapeutic strategies for tumors.
4.1.2 Inhibitors of soluble mediators
Some cytokines play a key role in T-cell exhaustion. For example, the use of IL-2 or blocking IL-10 and TGF-β can effectively block T-cell exhaustion. IL-2 can downregulate transcription factors related to T-cell exhaustion. Moreover, IL-2 combined with PD-1 treatment can significantly inhibit T-cell exhaustion and increase the expression of effector molecules (192). Alternatively, PD1-IL2v, a new immunocytokine that overcomes the need for IL-2 receptor α-chain (CD25) binding by docking cis into PD-1, differentiates stem cell-like CD8+ T cells into T-cell subsets by using a better effector function (193). IL-10 is an inhibitory cytokine that promotes T-cell exhaustion, and IL-10 reduction can improve antitumor immune responses (194). In patients with advanced melanoma, using the anti-PD-1 antibody while inhibiting IL-10 enhanced the cytotoxicity of CD8+ TIL cells (195). In addition, combining the anti-IL-10 monoclonal antibody with Toll-like receptor 9 ligand CpG significantly improved antitumor efficacy (196). IL-10 inhibition can be an effective tumor treatment strategy. In addition, TGF-β is involved in T-cell exhaustion. Blocking TGF-β is another target for T-cell therapy, which has been included in clinical trials (197). Compared with PD-1 therapy alone, treatment with a combination of TGF-β inhibitors and the PD-1 antibody more significantly increased the number of CD8+ T cells in patients with myeloma (198). In addition, galunisertib, a selective TGF-β receptor (TGFBR) inhibitor, can be used to prolong overall survival in patients with unresectable pancreatic cancer (199). Inhibition of these cellular mediators in the TME can effectively inhibit T-cell exhaustion, indicating their crucial role in T cell-based cancer immunotherapy.
4.2 Enhancement and maintenance of T-cell stemness
4.2.1 Enhancement of T-cell stemness-related gene expression
TSCM have stem cell-like properties and strong differentiation potential. TSCM and T cells both increased in patients with HIV after antiretroviral therapy (200). Stem cell-like T cells express CD62L, CCR7, TCF1, and LEF1 and can rapidly secrete IFN-γ, IL-2, and TNFα (201, 202). Increasing the expression of stemness-related genes can induce pluripotent stem cell-like T cells. Thus, the genetic reprogramming of differentiated T cells into less differentiated cells can generate a T cell-specific stemness phenotype (Figure 5).
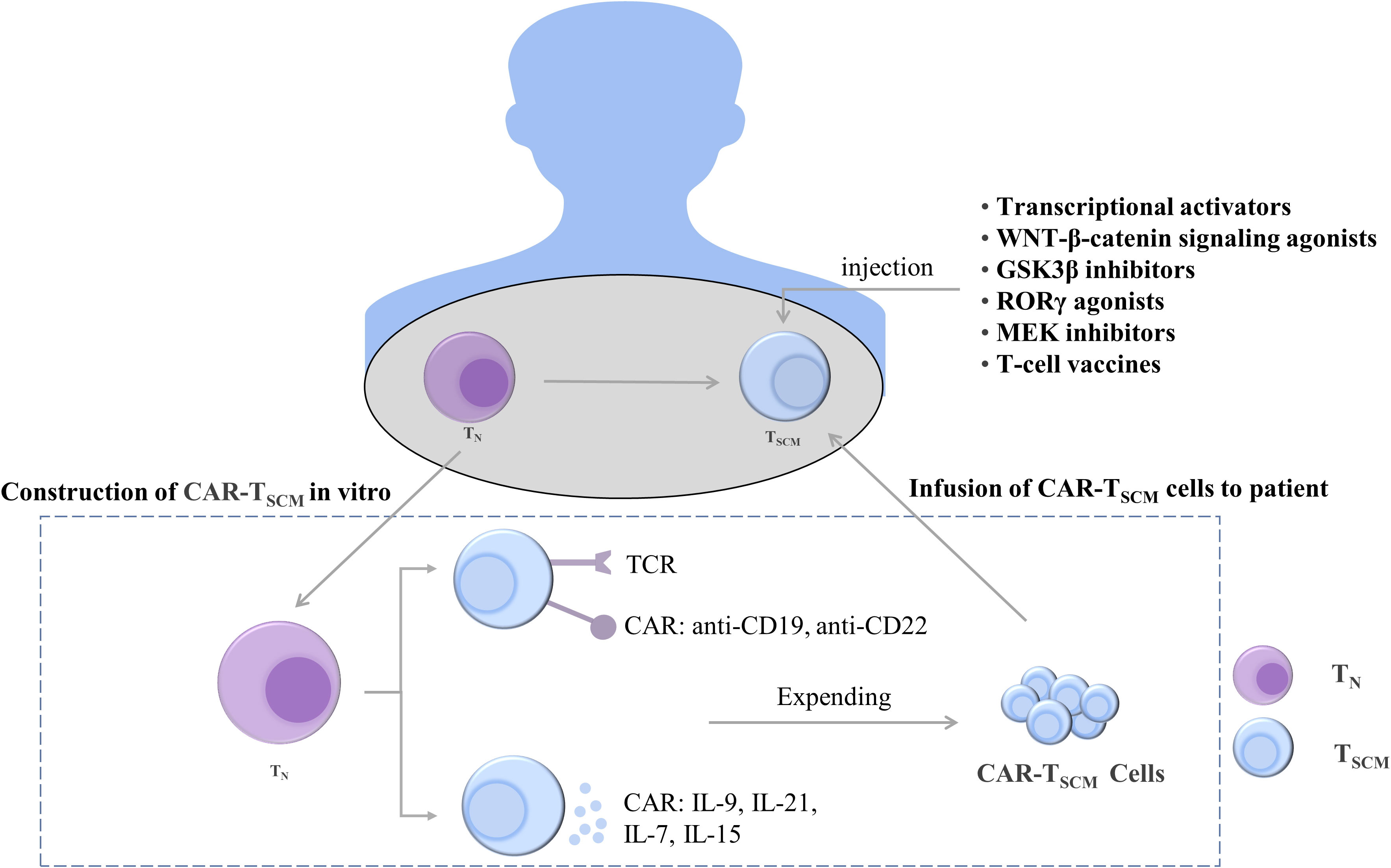
Figure 5 Treatments to maintain and induce T cell stemness. T cells can be constructed in vivo into CAR-TSCM cells expressing anti-CD19 and anti-CD22 and CAR-TSCM cells expressing cytokines IL-9, IL-21, IL-7, and IL-15. The stemness-enhancing CAR-TSCM cells can be infused back into patients for tumor treatment. In addition, enhanced T cell stemness can also be induced by using transcriptional activators, WNT-β-catenin signaling pathway agonists, GSK3β inhibitors, RORγ agonists, MEK inhibitors, and T cell vaccines. TN, naïve T cells; TSCM, stem cell-like memory T cells; IL, interleukin; TCR, T-cell receptor; CAR, chimeric antigen receptor; GSK-3β, glycogen synthase kinase-3β; RORγ, Retinoic acid receptor-related orphan receptor γ; MEK, mitogen-activated protein kinase.
T cells expressing TCF1 were identified as a group of cells with T-cell stemness; they possess the ability to undergo exponential expansion and self-renewal and differentiate into tumor-infiltrating lymphocytes. Intravenous injection of nanoparticle-based vaccines can generate more stem cell-like TCF1+ T cells, and the number of TCF1+ T cells in patients was positively correlated with the treatment outcome (99). Ablation of TCF1 suppressed the immunotherapy response (203). c-Myb is a transcriptional activator of TCF1, and overexpression of c-Myb in B16 tumor mice enhanced the multifunctionality of CD8+ T cells and production of TSCM as well as promoted the antitumor effect (204). The maintenance of T-cell stemness is dependent on WNT-β-catenin signaling, and WNT3A or GSK3β inhibitors promote stemness-related gene expression in TSCM (115, 205). When the expression of β-catenin is stabilized, T cells differentiate into effector T cells, inhibiting the stem cell-like characteristics of T cells and weakening the differentiation ability of T cells. The use of RORγ agonists increased the stemness of Th17 cells (206). In addition, selumetinib, an MEK1/2 inhibitor (MEKi), promoted the generation of TSCM by inhibiting the MAPK pathway, retarding cell cycle progression, and enhancing metabolic fitness. Moreover, MEKi treatment in tumor-bearing mice resulted in a strong antitumor effect (165). The enhancement of the stemness of T cells is helpful for treating tumors, and its therapeutic effect has been demonstrated. However, additional mechanisms through which the stemness of T cells is maintained remain to be identified.
4.2.2 Enhancement of stemness characteristics of T cells by CAR-T therapy
Adoptive transfer of T-cell therapy is an emerging cancer treatment modality that involves genetically engineering a patient’s T cells to generate T cells that target cancer cells and are then infused back into the patient to exert an antitumor effect. Among them, CAR-T cell therapy is widely used in clinical practice, and it exerts a cytotoxic effect by binding to specific antigens on the surface of cancer cells (207). IL-9-secreting (T9) CAR-T cells have an enhanced T stem cell-like phenotype and can differentiate into effector T cells (208). CAR-T cells can secrete soluble molecules that activate T-cell stemness. For example, IL-21 maintains T-cell stemness and survival by inhibiting the formation of TEFF (148). The inoculation of CAR-T cells expressing IL-15 into mouse tumors promoted the upregulation and maintenance of TCF1 and increased the proportion of stem cell-like memory T cells. Furthermore, the inoculation of CAR-T cells coexpressing IL-15 and IL-21 in mice resulted in more robust T-cell expansion compared with IL-15 CAR-T treatment (209). In addition, IL-7 can stimulate the proliferation of TSCM. When CAR-T cells secreting IL-7 and chemokine (C–C motif) ligand 19 were intravenously injected into patients with advanced liver cancer, the tumors almost completely disappeared (210). These studies indicate that CAR-T cells, which secrete cytokines to enhance the stemness of T cells, exhibit significant antitumor activity in solid tumors.
More TSCM can be produced by enhancing the effect of CAR-T cells, which can increase the antitumor effect. Regnase-1 deficiency can reduce TCF1 CAR-T cell fatigue, indicating that CAR-T cells support the expansion and the formation of TSCM (211). Furthermore, the blockade of the BET bromodomain with JQ1 downregulated BATF expression to preserve the TSCM-enriched transcriptome signature, and CAR-T cells exhibited enhanced proliferative capacity (212). The addition of Cas-CLOVER, a high-fidelity nuclease, to CAR-T cells led to the production of stable TSCM exhibiting robust antitumor activity in both in vitro and in vivo models (213). Decitabine can be used to enhance the stemness of CAR-T cells. Compared with untreated CAR-T cells, CAR-T cells treated with low-dose decitabine exhibited higher expression of the T-cell stemness–related genes LEF1, TCF7, and Bcl6 and had better T-cell expansion ability (214). Construction of the allogeneic MUC1-C-specific CAR-T cells P-MUC1C-ALLO1, which were used to treat breast and ovarian cancer through xenotransplantation, exerted an effective curative effect in vivo (209). Wei et al. reported that the simultaneous activation of the costimulatory signals 4-1BB and DAP10 on NKG2D CAR-T cells resulted in the poor differentiation of T cells in vitro and in vivo, upregulation of Bcl-2 expression, and an increased proportion of TSCM subsets (215). CAR-T cell adoptive transfer therapy results in significant antitumor activity, but this effect is observed only in a small group of patients. By enhancing the stemness of T cells, CAR-T cells may produce stronger antitumor properties. These studies provide a strong scientific basis for the rapid development of TSCM in human clinical trials of adoptive immunotherapy.
4.2.3 Enhancement of the stemness characteristics of T cells by cancer vaccines
Tumor vaccines are currently under development. Cancer therapeutic vaccines use peptides to mimic cancer epitopes presented by major histocompatibility complex molecules in combination with potent adjuvants to induce TSCM. Cancer vaccines control and eliminate tumors by generating robust and durable antitumor responses while overcoming immune tolerance and immunosuppression. Currently, studies on T-cell vaccines are conducted enrolling only a small number of patients. Serial vaccination with the Melan-AMART-126-35 peptide, CpG-B, and complete Freund adjuvant stably expanded tumor-specific CD8+ TSCM cells after 6 months and supported long-term antitumor immunity (216). Therefore, tumor vaccines are used to enhance the anti-tumor effects of T cells by inducing the expression of TSCM. However, knowledge of cancer vaccines is inadequate. To substantially induce TSCM, the selection of antigen targets, the efficacy of adjuvants, and the optimization of drug delivery systems are crucial; these need to be explored in future studies. Cancer vaccines are a potential direction of immunotherapy.
5 Conclusion and perspectives
T cells play a crucial role in killing tumors in the TME. However, because of the immunodeficiency of T cells and the negative regulation of T cells by the TME, tumor progression cannot be optimally controlled currently. In this review, we discussed the characteristics of TEX and stem cell-like T cells to improve our understanding regarding them. T-cell exhaustion is a state of disability, which includes T-cell expression inhibitory immune checkpoints and cytokine secretion disorder. According to the characteristics of T-cell exhaustion, immune checkpoint inhibitors and soluble mediators can be used to reverse the exhaustion of T cells, thus enhancing the antitumor effect of T cells. TSCM have unique stemness characteristics, long-term self-renewal ability, and pluripotency, and maintaining the stemness of T cells has become the focus of current research. The induction and expansion of TSCM in vitro and infusing them into patients can produce a stable antitumor effect in vivo, which is the main goal of CAR-T therapy. We summarized the characteristics and mechanisms of action of TEX and stem cell-like T cells as well as discussed current therapies aimed at reversing T-cell exhaustion and supporting T-cell stemness to maintain durable T-cell responses. These methods have demonstrated effectiveness in the treatment of tumors. However, the specific mechanisms of action and related therapeutic regimens in TSCM and T-cell exhaustion need to be further explored to fully understand immune and molecular changes during the whole treatment process and to predict drug efficacy and possible recurrence. Efficient and persistent T-cell action can ensure the long-term prevention and treatment of cancer and infections.
Author contributions
XC, SL and PY wrote and edited the draft. W-LH and reviewed and edited the manuscript. W-HY, XY and J-HC wrote the manuscript and supervised the entire work. All authors agree to be responsible for the publication. All authors contributed to the article and approved the submitted version.
Funding
This work was supported by the Guangzhou key medical discipline construction project fund, the National Natural Science Foundation of China (82172789), the National Science and Technology Council, Taiwan (MOST 111-2320-B-039-061 and 111-2628-B-A49-017), an Innovative Research Grant from the National Health Research Institutes, Taiwan (NHRI- EX111-11010BI), a YingTsai Young Scholar Award (CMU108-YTY-04), the National Research Foundation of Korea grant funded by the Korea government Ministry of Science and ICT (MSIT; Grant Nos.2020R1C1C1005631 and 2021R1A4A1031856 to J-HC), a grant from the Yen Tjing Ling Medical Foundation (CI-111-15 to W-LH), a grant from the Ministry of Health and Welfare, Center of Excellence for Cancer Research (MOHW107-TDU-B-211-114019, 111W31208 to W-LH), and a grant from the Higher Education Sprout Project by the Ministry of Education (MOE) (110AC-D303 to W-LH).
Acknowledgments
We apologize for omitting any publications in the references due to the limited space.
Conflict of interest
The authors declare that the research was conducted in the absence of any commercial or financial relationships that could be construed as a potential conflict of interest.
Publisher’s note
All claims expressed in this article are solely those of the authors and do not necessarily represent those of their affiliated organizations, or those of the publisher, the editors and the reviewers. Any product that may be evaluated in this article, or claim that may be made by its manufacturer, is not guaranteed or endorsed by the publisher.
References
1. Bernadic M Jr., Duchon R, Aziri R, Mladosievicova B. New principles of cancer therapy give new hope for oncological patients. Bratisl Lek Listy (2019) 120(1):15–8. doi: 10.4149/bll_2019_002
2. Wen Y, Zhu Y, Zhang C, Yang X, Gao Y, Li M, et al. Chronic inflammation, cancer development and immunotherapy. Front Pharmacol (2022) 13:1040163. doi: 10.3389/fphar.2022.1040163
3. Rosenberg SA, Yang JC, Sherry RM, Kammula US, Hughes MS, Phan GQ, et al. Durable complete responses in heavily pretreated patients with metastatic melanoma using T-cell transfer immunotherapy. Clin Cancer Res (2011) 17(13):4550–7. doi: 10.1158/1078-0432.Ccr-11-0116
4. Khan M, Maker AV, Jain S. The evolution of cancer immunotherapy. Vaccines (Basel) (2021) 9(6):614. doi: 10.3390/vaccines9060614
5. Zhao L, Cao YJ. Engineered T cell therapy for cancer in the clinic. Front Immunol (2019) 10:2250. doi: 10.3389/fimmu.2019.02250
6. Perica K, Varela JC, Oelke M, Schneck J. Adoptive T cell immunotherapy for cancer. Rambam Maimonides Med J (2015) 6(1):e0004. doi: 10.5041/rmmj.10179
7. Qu C, Zhang H, Cao H, Tang L, Mo H, Liu F, et al. Tumor buster - where will the car-T cell therapy ‘Missile’ go? Mol Cancer (2022) 21(1):201. doi: 10.1186/s12943-022-01669-8
8. Stahl B. Chimeric antigen receptor T-cell therapy in cancer. Biology and Microbiology Graduate Students Plan B Research Projects (2022) 39. Available from: https://openprairie.sdstate.edu/biomicro_plan-b/39.
9. Ward JP. Tumor infiltrating lymphocytes (Til): From bench to bedside. In: Ghobadi A, DiPersio JF, editors. Gene and cellular immunotherapy for cancer. Cham: Springer International Publishing (2022) 223–48. doi: 10.1007/978-3-030-87849-8_13
10. Shafer P, Kelly LM, Hoyos V. Cancer therapy with tcr-engineered T cells: Current strategies, challenges, and prospects. Front Immunol (2022) 13:835762. doi: 10.3389/fimmu.2022.835762
11. Till BG, Jensen MC, Wang J, Qian X, Gopal AK, Maloney DG, et al. Cd20-specific adoptive immunotherapy for lymphoma using a chimeric antigen receptor with both Cd28 and 4-1bb domains: Pilot clinical trial results. Blood (2012) 119(17):3940–50. doi: 10.1182/blood-2011-10-387969
12. Turtle CJ, Hanafi LA, Berger C, Gooley TA, Cherian S, Hudecek M, et al. Cd19 car-T cells of defined Cd4+:Cd8+ composition in adult b cell all patients. J Clin Invest (2016) 126(6):2123–38. doi: 10.1172/jci85309
13. Lim WA, June CH. The principles of engineering immune cells to treat cancer. Cell (2017) 168(4):724–40. doi: 10.1016/j.cell.2017.01.016
14. Moskophidis D, Lechner F, Pircher H, Zinkernagel RM. Virus persistence in acutely infected immunocompetent mice by exhaustion of antiviral cytotoxic effector T cells. Nature (1993) 362(6422):758–61. doi: 10.1038/362758a0
16. Guo Y, Xie YQ, Gao M, Zhao Y, Franco F, Wenes M, et al. Metabolic reprogramming of terminally exhausted Cd8(+) T cells by il-10 enhances anti-tumor immunity. Nat Immunol (2021) 22(6):746–56. doi: 10.1038/s41590-021-00940-2
17. Miller BC, Sen DR, Al Abosy R, Bi K, Virkud YV, LaFleur MW, et al. Author correction: Subsets of exhausted Cd8(+) T cells differentially mediate tumor control and respond to checkpoint blockade. Nat Immunol (2019) 20(11):1556. doi: 10.1038/s41590-019-0528-5
18. Sade-Feldman M, Yizhak K, Bjorgaard SL, Ray JP, de Boer CG, Jenkins RW, et al. Defining T cell states associated with response to checkpoint immunotherapy in melanoma. Cell (2019) 176(1-2):404. doi: 10.1016/j.cell.2018.12.034
19. Wei SC, Duffy CR, Allison JP. Fundamental mechanisms of immune checkpoint blockade therapy. Cancer Discovery (2018) 8(9):1069–86. doi: 10.1158/2159-8290.Cd-18-0367
20. Kostine M, Finckh A, Bingham CO, Visser K, Leipe J, Schulze-Koops H, et al. Eular points to consider for the diagnosis and management of rheumatic immune-related adverse events due to cancer immunotherapy with checkpoint inhibitors. Ann Rheum Dis (2021) 80(1):36–48. doi: 10.1136/annrheumdis-2020-217139
21. Kraehenbuehl L, Weng CH, Eghbali S, Wolchok JD, Merghoub T. Enhancing immunotherapy in cancer by targeting emerging immunomodulatory pathways. Nat Rev Clin Oncol (2022) 19(1):37–50. doi: 10.1038/s41571-021-00552-7
22. Ford BR, Vignali PDA, Rittenhouse NL, Scharping NE, Peralta R, Lontos K, et al. Tumor microenvironmental signals reshape chromatin landscapes to limit the functional potential of exhausted T cells. Sci Immunol (2022) 7(74):eabj9123. doi: 10.1126/sciimmunol.abj9123
23. Mahnke YD, Brodie TM, Sallusto F, Roederer M, Lugli E. The who’s who of T-cell differentiation: Human memory T-cell subsets. Eur J Immunol (2013) 43(11):2797–809. doi: 10.1002/eji.201343751
24. Casey KA, Fraser KA, Schenkel JM, Moran A, Abt MC, Beura LK, et al. Antigen-independent differentiation and maintenance of effector-like resident memory T cells in tissues. J Immunol (2012) 188(10):4866–75. doi: 10.4049/jimmunol.1200402
25. Kumar BV, Connors TJ, Farber DL. Human T cell development, localization, and function throughout life. Immunity (2018) 48(2):202–13. doi: 10.1016/j.immuni.2018.01.007
26. Roberto A, Castagna L, Zanon V, Bramanti S, Crocchiolo R, McLaren JE, et al. Role of naive-derived T memory stem cells in T-cell reconstitution following allogeneic transplantation. Blood (2015) 125(18):2855–64. doi: 10.1182/blood-2014-11-608406
27. Gattinoni L, Speiser DE, Lichterfeld M, Bonini C. T Memory stem cells in health and disease. Nat Med (2017) 23(1):18–27. doi: 10.1038/nm.4241
28. Gattinoni L, Lugli E, Ji Y, Pos Z, Paulos CM, Quigley MF, et al. A human memory T cell subset with stem cell-like properties. Nat Med (2011) 17(10):1290–7. doi: 10.1038/nm.2446
29. Kaech SM, Cui W. Transcriptional control of effector and memory Cd8+ T cell differentiation. Nat Rev Immunol (2012) 12(11):749–61. doi: 10.1038/nri3307
30. Kranz E, Kuhlmann CJ, Chan J, Kim PY, Chen ISY, Kamata M. Efficient derivation of chimeric-antigen receptor-modified T(Scm) cells. Front Immunol (2022) 13:877682. doi: 10.3389/fimmu.2022.877682
31. Arcangeli S, Falcone L, Camisa B, De Girardi F, Biondi M, Giglio F, et al. Next-generation manufacturing protocols enriching T(Scm) car T cells can overcome disease-specific T cell defects in cancer patients. Front Immunol (2020) 11:1217. doi: 10.3389/fimmu.2020.01217
32. Sabatino M, Hu J, Sommariva M, Gautam S, Fellowes V, Hocker JD, et al. Generation of clinical-grade Cd19-specific car-modified Cd8+ memory stem cells for the treatment of human b-cell malignancies. Blood (2016) 128(4):519–28. doi: 10.1182/blood-2015-11-683847
33. Gattinoni L, Klebanoff CA, Restifo NP. Paths to stemness: Building the ultimate antitumour T cell. Nat Rev Cancer (2012) 12(10):671–84. doi: 10.1038/nrc3322
34. Gattinoni L, Restifo NP. Moving T memory stem cells to the clinic. Blood (2013) 121(4):567–8. doi: 10.1182/blood-2012-11-468660
35. Minagawa A, Yoshikawa T, Yasukawa M, Hotta A, Kunitomo M, Iriguchi S, et al. Enhancing T cell receptor stability in rejuvenated ipsc-derived T cells improves their use in cancer immunotherapy. Cell Stem Cell (2018) 23(6):850–8.e4. doi: 10.1016/j.stem.2018.10.005
36. Masopust D, Schenkel JM. The integration of T cell migration, differentiation and function. Nat Rev Immunol (2013) 13(5):309–20. doi: 10.1038/nri3442
37. Balkhi MY, Ma Q, Ahmad S, Junghans RP. T Cell exhaustion and interleukin 2 downregulation. Cytokine (2015) 71(2):339–47. doi: 10.1016/j.cyto.2014.11.024
38. Philip M, Schietinger A. Heterogeneity and fate choice: T cell exhaustion in cancer and chronic infections. Curr Opin Immunol (2019) 58:98–103. doi: 10.1016/j.coi.2019.04.014
39. Blank CU, Haining WN, Held W, Hogan PG, Kallies A, Lugli E, et al. Defining ‘T cell exhaustion’. Nat Rev Immunol (2019) 19(11):665–74. doi: 10.1038/s41577-019-0221-9
40. McLane LM, Abdel-Hakeem MS, Wherry EJ. Cd8 T cell exhaustion during chronic viral infection and cancer. Annu Rev Immunol (2019) 37:457–95. doi: 10.1146/annurev-immunol-041015-055318
41. Schietinger A, Greenberg PD. Tolerance and exhaustion: Defining mechanisms of T cell dysfunction. Trends Immunol (2014) 35(2):51–60. doi: 10.1016/j.it.2013.10.001
42. Kim HD, Song GW, Park S, Jung MK, Kim MH, Kang HJ, et al. Association between expression level of Pd1 by tumor-infiltrating Cd8(+) T cells and features of hepatocellular carcinoma. Gastroenterology (2018) 155(6):1936–50.e17. doi: 10.1053/j.gastro.2018.08.030
43. Tao J, Han D, Gao S, Zhang W, Yu H, Liu P, et al. Cd8(+) T cells exhaustion induced by myeloid-derived suppressor cells in myelodysplastic syndromes patients might be through Tim3/Gal-9 pathway. J Cell Mol Med (2020) 24(1):1046–58. doi: 10.1111/jcmm.14825
44. Grebinoski S, Zhang Q, Cillo AR, Manne S, Xiao H, Brunazzi EA, et al. Autoreactive Cd8(+) T cells are restrained by an exhaustion-like program that is maintained by Lag3. Nat Immunol (2022) 23(6):868–77. doi: 10.1038/s41590-022-01210-5
45. Lu L, Bai Y, Wang Z. Elevated T cell activation score is associated with improved survival of breast cancer. Breast Cancer Res Treat (2017) 164(3):689–96. doi: 10.1007/s10549-017-4281-x
46. Liang R, Zhu X, Lan T, Ding D, Zheng Z, Chen T, et al. Tigit promotes Cd8(+)T cells exhaustion and predicts poor prognosis of colorectal cancer. Cancer Immunol Immunother (2021) 70(10):2781–93. doi: 10.1007/s00262-021-02886-8
47. Pauken KE, Wherry EJ. Overcoming T cell exhaustion in infection and cancer. Trends Immunol (2015) 36(4):265–76. doi: 10.1016/j.it.2015.02.008
48. Wherry EJ, Ha SJ, Kaech SM, Haining WN, Sarkar S, Kalia V, et al. Molecular signature of Cd8+ T cell exhaustion during chronic viral infection. Immunity (2007) 27(4):670–84. doi: 10.1016/j.immuni.2007.09.006
49. Velu V, Titanji K, Zhu B, Husain S, Pladevega A, Lai L, et al. Enhancing siv-specific immunity in vivo by pd-1 blockade. Nature (2009) 458(7235):206–10. doi: 10.1038/nature07662
50. Bengsch B, Seigel B, Ruhl M, Timm J, Kuntz M, Blum HE, et al. Coexpression of pd-1, 2b4, Cd160 and Klrg1 on exhausted hcv-specific Cd8+ T cells is linked to antigen recognition and T cell differentiation. PloS Pathog (2010) 6(6):e1000947. doi: 10.1371/journal.ppat.1000947
51. Bengsch B, Martin B, Thimme R. Restoration of hbv-specific Cd8+ T cell function by pd-1 blockade in inactive carrier patients is linked to T cell differentiation. J Hepatol (2014) 61(6):1212–9. doi: 10.1016/j.jhep.2014.07.005
52. Blackburn SD, Shin H, Haining WN, Zou T, Workman CJ, Polley A, et al. Coregulation of Cd8+ T cell exhaustion by multiple inhibitory receptors during chronic viral infection. Nat Immunol (2009) 10(1):29–37. doi: 10.1038/ni.1679
53. Pentcheva-Hoang T, Egen JG, Wojnoonski K, Allison JP. B7-1 and B7-2 selectively recruit ctla-4 and Cd28 to the immunological synapse. Immunity (2004) 21(3):401–13. doi: 10.1016/j.immuni.2004.06.017
54. Parry RV, Chemnitz JM, Frauwirth KA, Lanfranco AR, Braunstein I, Kobayashi SV, et al. Ctla-4 and pd-1 receptors inhibit T-cell activation by distinct mechanisms. Mol Cell Biol (2005) 25(21):9543–53. doi: 10.1128/mcb.25.21.9543-9553.2005
55. Quigley M, Pereyra F, Nilsson B, Porichis F, Fonseca C, Eichbaum Q, et al. Transcriptional analysis of hiv-specific Cd8+ T cells shows that pd-1 inhibits T cell function by upregulating batf. Nat Med (2010) 16(10):1147–51. doi: 10.1038/nm.2232
56. Pauken KE, Wherry EJ. Snapshot: T cell exhaustion. Cell (2015) 163(4):1038–.e1. doi: 10.1016/j.cell.2015.10.054
57. Wilson EB, Brooks DG. The role of il-10 in regulating immunity to persistent viral infections. Curr Top Microbiol Immunol (2011) 350:39–65. doi: 10.1007/82_2010_96
58. Said EA, Dupuy FP, Trautmann L, Zhang Y, Shi Y, El-Far M, et al. Programmed death-1-Induced interleukin-10 production by monocytes impairs Cd4+ T cell activation during hiv infection. Nat Med (2010) 16(4):452–9. doi: 10.1038/nm.2106
59. Brooks DG, Ha SJ, Elsaesser H, Sharpe AH, Freeman GJ, Oldstone MB. Il-10 and pd-L1 operate through distinct pathways to suppress T-cell activity during persistent viral infection. Proc Natl Acad Sci USA (2008) 105(51):20428–33. doi: 10.1073/pnas.0811139106
60. Couper KN, Blount DG, Riley EM. Il-10: The master regulator of immunity to infection. J Immunol (2008) 180(9):5771–7. doi: 10.4049/jimmunol.180.9.5771
61. Ruffell B, Chang-Strachan D, Chan V, Rosenbusch A, Ho CM, Pryer N, et al. Macrophage il-10 blocks Cd8+ T cell-dependent responses to chemotherapy by suppressing il-12 expression in intratumoral dendritic cells. Cancer Cell (2014) 26(5):623–37. doi: 10.1016/j.ccell.2014.09.006
62. Ravi VM, Neidert N, Will P, Joseph K, Maier JP, Kückelhaus J, et al. T-Cell dysfunction in the glioblastoma microenvironment is mediated by myeloid cells releasing interleukin-10. Nat Commun (2022) 13(1):925. doi: 10.1038/s41467-022-28523-1
63. Derynck R, Zhang YE. Smad-dependent and smad-independent pathways in tgf-beta family signalling. Nature (2003) 425(6958):577–84. doi: 10.1038/nature02006
64. Tinoco R, Alcalde V, Yang Y, Sauer K, Zuniga EI. Cell-intrinsic transforming growth factor-beta signaling mediates virus-specific Cd8+ T cell deletion and viral persistence in vivo. Immunity (2009) 31(1):145–57. doi: 10.1016/j.immuni.2009.06.015
65. Nelson DR, Gonzalez-Peralta RP, Qian K, Xu Y, Marousis CG, Davis GL, et al. Transforming growth factor-beta 1 in chronic hepatitis c. J Viral Hepat (1997) 4(1):29–35. doi: 10.1046/j.1365-2893.1997.00124.x
66. Gros A, Robbins PF, Yao X, Li YF, Turcotte S, Tran E, et al. Pd-1 identifies the patient-specific Cd8+ tumor-reactive repertoire infiltrating human tumors. J Clin Invest (2014) 124(5):2246–59. doi: 10.1172/jci73639
67. West EE, Jin HT, Rasheed AU, Penaloza-Macmaster P, Ha SJ, Tan WG, et al. Pd-L1 blockade synergizes with il-2 therapy in reinvigorating exhausted T cells. J Clin Invest (2013) 123(6):2604–15. doi: 10.1172/jci67008
68. Boyman O, Surh CD, Sprent J. Potential use of il-2/Anti-Il-2 antibody immune complexes for the treatment of cancer and autoimmune disease. Expert Opin Biol Ther (2006) 6(12):1323–31. doi: 10.1517/14712598.6.12.1323
69. Caggiari L, Zanussi S, Crepaldi C, Bortolin MT, Caffau C, D’Andrea M, et al. Different rates of Cd4+ and Cd8+ T-cell proliferation in interleukin-2-Treated human immunodeficiency virus-positive subjects. Cytometry (2001) 46(4):233–7. doi: 10.1002/cyto.1132
70. Marchetti G, Meroni L, Molteni C, Bandera A, Franzetti F, Galli M, et al. Interleukin-2 immunotherapy exerts a differential effect on Cd4 and Cd8 T cell dynamics. Aids (2004) 18(2):211–6. doi: 10.1097/00002030-200401230-00010
71. Abrams D, Lévy Y, Losso MH, Babiker A, Collins G, Cooper DA, et al. Interleukin-2 therapy in patients with hiv infection. N Engl J Med (2009) 361(16):1548–59. doi: 10.1056/NEJMoa0903175
72. Spolski R, Leonard WJ. Interleukin-21: Basic biology and implications for cancer and autoimmunity. Annu Rev Immunol (2008) 26:57–79. doi: 10.1146/annurev.immunol.26.021607.090316
73. Yi JS, Du M, Zajac AJ. A vital role for interleukin-21 in the control of a chronic viral infection. Science (2009) 324(5934):1572–6. doi: 10.1126/science.1175194
74. Fröhlich A, Kisielow J, Schmitz I, Freigang S, Shamshiev AT, Weber J, et al. Il-21r on T cells is critical for sustained functionality and control of chronic viral infection. Science (2009) 324(5934):1576–80. doi: 10.1126/science.1172815
75. McNab F, Mayer-Barber K, Sher A, Wack A, O’Garra A. Type I interferons in infectious disease. Nat Rev Immunol (2015) 15(2):87–103. doi: 10.1038/nri3787
76. Wu T, Ji Y, Moseman EA, Xu HC, Manglani M, Kirby M, et al. The Tcf1-Bcl6 axis counteracts type I interferon to repress exhaustion and maintain T cell stemness. Sci Immunol (2016) 1(6):eaai8593. doi: 10.1126/sciimmunol.aai8593
77. Martinez GJ, Pereira RM, Äijö T, Kim EY, Marangoni F, Pipkin ME, et al. The transcription factor nfat promotes exhaustion of activated Cd8+ T cells. Immunity (2015) 42(2):265–78. doi: 10.1016/j.immuni.2015.01.006
78. Philip M, Fairchild L, Sun L, Horste EL, Camara S, Shakiba M, et al. Chromatin states define tumour-specific T cell dysfunction and reprogramming. Nature (2017) 545(7655):452–6. doi: 10.1038/nature22367
79. Man K, Gabriel SS, Liao Y, Gloury R, Preston S, Henstridge DC, et al. Transcription factor Irf4 promotes Cd8(+) T cell exhaustion and limits the development of memory-like T cells during chronic infection. Immunity (2017) 47(6):1129–41.e5. doi: 10.1016/j.immuni.2017.11.021
80. Seo H, González-Avalos E, Zhang W, Ramchandani P, Yang C, Lio CJ, et al. Batf and Irf4 cooperate to counter exhaustion in tumor-infiltrating car T cells. Nat Immunol (2021) 22(8):983–95. doi: 10.1038/s41590-021-00964-8
81. Seo H, Chen J, González-Avalos E, Samaniego-Castruita D, Das A, Wang YH, et al. Tox and Tox2 transcription factors cooperate with Nr4a transcription factors to impose Cd8(+) T cell exhaustion. Proc Natl Acad Sci USA (2019) 116(39):19761. doi: 10.1073/pnas.1914896116
82. Chen J, López-Moyado IF, Seo H, Lio CJ, Hempleman LJ, Sekiya T, et al. Nr4a transcription factors limit car T cell function in solid tumours. Nature (2019) 567(7749):530–4. doi: 10.1038/s41586-019-0985-x
83. Liu X, Wang Y, Lu H, Li J, Yan X, Xiao M, et al. Genome-wide analysis identifies Nr4a1 as a key mediator of T cell dysfunction. Nature (2019) 567(7749):525–9. doi: 10.1038/s41586-019-0979-8
84. Alfei F, Kanev K, Hofmann M, Wu M, Ghoneim HE, Roelli P, et al. Tox reinforces the phenotype and longevity of exhausted T cells in chronic viral infection. Nature (2019) 571(7764):265–9. doi: 10.1038/s41586-019-1326-9
85. Bengsch B, Johnson AL, Kurachi M, Odorizzi PM, Pauken KE, Attanasio J, et al. Bioenergetic insufficiencies due to metabolic alterations regulated by the inhibitory receptor pd-1 are an early driver of Cd8(+) T cell exhaustion. Immunity (2016) 45(2):358–73. doi: 10.1016/j.immuni.2016.07.008
86. Patsoukis N, Brown J, Petkova V, Liu F, Li L, Boussiotis VA. Selective effects of pd-1 on akt and ras pathways regulate molecular components of the cell cycle and inhibit T cell proliferation. Sci Signal (2012) 5(230):ra46. doi: 10.1126/scisignal.2002796
87. Patsoukis N, Bardhan K, Chatterjee P, Sari D, Liu B, Bell LN, et al. Pd-1 alters T-cell metabolic reprogramming by inhibiting glycolysis and promoting lipolysis and fatty acid oxidation. Nat Commun (2015) 6:6692. doi: 10.1038/ncomms7692
88. Kamphorst AO, Wieland A, Nasti T, Yang S, Zhang R, Barber DL, et al. Rescue of exhausted Cd8 T cells by pd-1-Targeted therapies is Cd28-dependent. Science (2017) 355(6332):1423–7. doi: 10.1126/science.aaf0683
89. Hui E, Cheung J, Zhu J, Su X, Taylor MJ, Wallweber HA, et al. T Cell costimulatory receptor Cd28 is a primary target for pd-1-Mediated inhibition. Science (2017) 355(6332):1428–33. doi: 10.1126/science.aaf1292
90. Chang CH, Qiu J, O’Sullivan D, Buck MD, Noguchi T, Curtis JD, et al. Metabolic competition in the tumor microenvironment is a driver of cancer progression. Cell (2015) 162(6):1229–41. doi: 10.1016/j.cell.2015.08.016
91. Hung MH, Lee JS, Ma C, Diggs LP, Heinrich S, Chang CW, et al. Tumor methionine metabolism drives T-cell exhaustion in hepatocellular carcinoma. Nat Commun (2021) 12(1):1455. doi: 10.1038/s41467-021-21804-1
92. Kallies A, Zehn D, Utzschneider DT. Precursor exhausted T cells: Key to successful immunotherapy? Nat Rev Immunol (2020) 20(2):128–36. doi: 10.1038/s41577-019-0223-7
93. Miller BC, Sen DR, Al Abosy R, Bi K, Virkud YV, LaFleur MW, et al. Subsets of exhausted Cd8(+) T cells differentially mediate tumor control and respond to checkpoint blockade. Nat Immunol (2019) 20(3):326–36. doi: 10.1038/s41590-019-0312-6
94. Jansen CS, Prokhnevska N, Master VA, Sanda MG, Carlisle JW, Bilen MA, et al. An intra-tumoral niche maintains and differentiates stem-like Cd8 T cells. Nature (2019) 576(7787):465–70. doi: 10.1038/s41586-019-1836-5
95. Utzschneider DT, Charmoy M, Chennupati V, Pousse L, Ferreira DP, Calderon-Copete S, et al. T Cell factor 1-expressing memory-like Cd8(+) T cells sustain the immune response to chronic viral infections. Immunity (2016) 45(2):415–27. doi: 10.1016/j.immuni.2016.07.021
96. Utzschneider DT, Gabriel SS, Chisanga D, Gloury R, Gubser PM, Vasanthakumar A, et al. Early precursor T cells establish and propagate T cell exhaustion in chronic infection. Nat Immunol (2020) 21(10):1256–66. doi: 10.1038/s41590-020-0760-z
97. Tsui C, Kretschmer L, Rapelius S, Gabriel SS, Chisanga D, Knöpper K, et al. Myb orchestrates T cell exhaustion and response to checkpoint inhibition. Nature (2022) 609(7926):354–60. doi: 10.1038/s41586-022-05105-1
98. Im SJ, Hashimoto M, Gerner MY, Lee J, Kissick HT, Burger MC, et al. Defining Cd8+ T cells that provide the proliferative burst after pd-1 therapy. Nature (2016) 537(7620):417–21. doi: 10.1038/nature19330
99. Baharom F, Ramirez-Valdez RA, Tobin KKS, Yamane H, Dutertre CA, Khalilnezhad A, et al. Intravenous nanoparticle vaccination generates stem-like Tcf1(+) neoantigen-specific Cd8(+) T cells. Nat Immunol (2021) 22(1):41–52. doi: 10.1038/s41590-020-00810-3
100. Leong YA, Chen Y, Ong HS, Wu D, Man K, Deleage C, et al. Cxcr5+ follicular cytotoxic T cells control viral infection in b cell follicles. Nat Immunol (2016) 17(10):1187–96. doi: 10.1038/ni.3543
101. He R, Hou S, Liu C, Zhang A, Bai Q, Han M, et al. Follicular Cxcr5- expressing Cd8(+) T cells curtail chronic viral infection. Nature (2016) 537(7620):412–28. doi: 10.1038/nature19317
102. Kurachi M. Cd8+ T cell exhaustion. Semin Immunopathol (2019) 41(3):327–37. doi: 10.1007/s00281-019-00744-5
103. Morrot A. Human stem memory T cells (T(Scm)) as critical players in the long-term persistence of immune responses. Ann Transl Med (2017) 5(5):120. doi: 10.21037/atm.2017.02.28
104. Zhang Y, Joe G, Hexner E, Zhu J, Emerson SG. Host-reactive Cd8+ memory stem cells in graft-Versus-Host disease. Nat Med (2005) 11(12):1299–305. doi: 10.1038/nm1326
105. Ahmed R, Roger L, Costa Del Amo P, Miners KL, Jones RE, Boelen L, et al. Human stem cell-like memory T cells are maintained in a state of dynamic flux. Cell Rep (2016) 17(11):2811–8. doi: 10.1016/j.celrep.2016.11.037
106. Klebanoff CA, Gattinoni L, Palmer DC, Muranski P, Ji Y, Hinrichs CS, et al. Determinants of successful Cd8+ T-cell adoptive immunotherapy for Large established tumors in mice. Clin Cancer Res (2011) 17(16):5343–52. doi: 10.1158/1078-0432.Ccr-11-0503
107. Lugli E, Dominguez MH, Gattinoni L, Chattopadhyay PK, Bolton DL, Song K, et al. Superior T memory stem cell persistence supports long-lived T cell memory. J Clin Invest (2013) 123(2):594–9. doi: 10.1172/jci66327
108. Biasco L, Scala S, Basso Ricci L, Dionisio F, Baricordi C, Calabria A, et al. In vivo tracking of T cells in humans unveils decade-long survival and activity of genetically modified T memory stem cells. Sci Transl Med (2015) 7(273):273ra13. doi: 10.1126/scitranslmed.3010314
109. Fuertes Marraco SA, Soneson C, Cagnon L, Gannon PO, Allard M, Abed Maillard S, et al. Long-lasting stem cell-like memory Cd8+ T cells with a naïve-like profile upon yellow fever vaccination. Sci Transl Med (2015) 7(282):282ra48. doi: 10.1126/scitranslmed.aaa3700
110. Costa Del Amo P, Lahoz-Beneytez J, Boelen L, Ahmed R, Miners KL, Zhang Y, et al. Human tscm cell dynamics in vivo are compatible with long-lived immunological memory and stemness. PloS Biol (2018) 16(6):e2005523. doi: 10.1371/journal.pbio.2005523
111. Gossel G, Hogan T, Cownden D, Seddon B, Yates AJ. Memory Cd4 T cell subsets are kinetically heterogeneous and replenished from naive T cells at high levels. Elife (2017) 6:e23013. doi: 10.7554/eLife.23013
112. Di Benedetto S, Derhovanessian E, Steinhagen-Thiessen E, Goldeck D, Müller L, Pawelec G. Impact of age, sex and cmv-infection on peripheral T cell phenotypes: Results from the Berlin base-ii study. Biogerontology (2015) 16(5):631–43. doi: 10.1007/s10522-015-9563-2
113. Kilpatrick RD, Rickabaugh T, Hultin LE, Hultin P, Hausner MA, Detels R, et al. Homeostasis of the naive Cd4+ T cell compartment during aging. J Immunol (2008) 180(3):1499–507. doi: 10.4049/jimmunol.180.3.1499
114. Kared H, Tan SW, Lau MC, Chevrier M, Tan C, How W, et al. Immunological history governs human stem cell memory Cd4 heterogeneity Via the wnt signaling pathway. Nat Commun (2020) 11(1):821. doi: 10.1038/s41467-020-14442-6
115. Gattinoni L, Zhong XS, Palmer DC, Ji Y, Hinrichs CS, Yu Z, et al. Wnt signaling arrests effector T cell differentiation and generates Cd8+ memory stem cells. Nat Med (2009) 15(7):808–13. doi: 10.1038/nm.1982
116. Knoblich JA. Mechanisms of asymmetric stem cell division. Cell (2008) 132(4):583–97. doi: 10.1016/j.cell.2008.02.007
117. Jung HR, Song KH, Chang JT, Doh J. Geometrically controlled asymmetric division of Cd4+ T cells studied by immunological synapse arrays. PloS One (2014) 9(3):e91926. doi: 10.1371/journal.pone.0091926
118. Chang JT, Palanivel VR, Kinjyo I, Schambach F, Intlekofer AM, Banerjee A, et al. Asymmetric T lymphocyte division in the initiation of adaptive immune responses. Science (2007) 315(5819):1687–91. doi: 10.1126/science.1139393
119. van der Windt GJ, Everts B, Chang CH, Curtis JD, Freitas TC, Amiel E, et al. Mitochondrial respiratory capacity is a critical regulator of Cd8+ T cell memory development. Immunity (2012) 36(1):68–78. doi: 10.1016/j.immuni.2011.12.007
120. Pollizzi KN, Sun IH, Patel CH, Lo YC, Oh MH, Waickman AT, et al. Asymmetric inheritance of Mtorc1 kinase activity during division dictates Cd8(+) T cell differentiation. Nat Immunol (2016) 17(6):704–11. doi: 10.1038/ni.3438
121. Ciocca ML, Barnett BE, Burkhardt JK, Chang JT, Reiner SL. Cutting edge: Asymmetric memory T cell division in response to rechallenge. J Immunol (2012) 188(9):4145–8. doi: 10.4049/jimmunol.1200176
122. van der Windt GJ, O’Sullivan D, Everts B, Huang SC, Buck MD, Curtis JD, et al. Cd8 memory T cells have a bioenergetic advantage that underlies their rapid recall ability. Proc Natl Acad Sci USA (2013) 110(35):14336–41. doi: 10.1073/pnas.1221740110
123. Restifo NP, Gattinoni L. Lineage relationship of effector and memory T cells. Curr Opin Immunol (2013) 25(5):556–63. doi: 10.1016/j.coi.2013.09.003
124. Cieri N, Oliveira G, Greco R, Forcato M, Taccioli C, Cianciotti B, et al. Generation of human memory stem T cells after haploidentical T-replete hematopoietic stem cell transplantation. Blood (2015) 125(18):2865–74. doi: 10.1182/blood-2014-11-608539
125. Yu X, Zhang L, Chaudhry A, Rapaport AS, Ouyang W. Unravelling the heterogeneity and dynamic relationships of tumor-infiltrating T cells by single-cell rna sequencing analysis. J Leukocyte Biol (2020) 107(6):917–32. doi: 10.1002/JLB.6MR0320-234R
126. Caccamo N, Joosten SA, Ottenhoff THM, Dieli F. Atypical human Effector/Memory Cd4(+) T cells with a naive-like phenotype. Front Immunol (2018) 9:2832. doi: 10.3389/fimmu.2018.02832
127. Nadazdin O, Boskovic S, Murakami T, O’Connor DH, Wiseman RW, Karl JA, et al. Phenotype, distribution and alloreactive properties of memory T cells from cynomolgus monkeys. Am J Transplant (2010) 10(6):1375–84. doi: 10.1111/j.1600-6143.2010.03119.x
128. Mami-Chouaib F, Blanc C, Corgnac S, Hans S, Malenica I, Granier C, et al. Resident memory T cells, critical components in tumor immunology. J Immunother Cancer (2018) 6(1):87. doi: 10.1186/s40425-018-0399-6
129. Bannard O, Kraman M, Fearon D. Pathways of memory Cd8+ T-cell development. Eur J Immunol (2009) 39(8):2083–7. doi: 10.1002/eji.200939555
130. Thome JJ, Yudanin N, Ohmura Y, Kubota M, Grinshpun B, Sathaliyawala T, et al. Spatial map of human T cell compartmentalization and maintenance over decades of life. Cell (2014) 159(4):814–28. doi: 10.1016/j.cell.2014.10.026
131. Kryczek I, Zhao E, Liu Y, Wang Y, Vatan L, Szeliga W, et al. Human Th17 cells are long-lived effector memory cells. Sci Transl Med (2011) 3(104):104ra0. doi: 10.1126/scitranslmed.3002949
132. Wei S, Zhao E, Kryczek I, Zou W. Th17 cells have stem cell-like features and promote long-term immunity. Oncoimmunology (2012) 1(4):516–9. doi: 10.4161/onci.19440
133. Muranski P, Borman ZA, Kerkar SP, Klebanoff CA, Ji Y, Sanchez-Perez L, et al. Th17 cells are long lived and retain a stem cell-like molecular signature. Immunity (2011) 35(6):972–85. doi: 10.1016/j.immuni.2011.09.019
134. Buzon MJ, Sun H, Li C, Shaw A, Seiss K, Ouyang Z, et al. Hiv-1 persistence in Cd4+ T cells with stem cell-like properties. Nat Med (2014) 20(2):139–42. doi: 10.1038/nm.3445
135. Nagai Y, Kawahara M, Hishizawa M, Shimazu Y, Sugino N, Fujii S, et al. T Memory stem cells are the hierarchical apex of adult T-cell leukemia. Blood (2015) 125(23):3527–35. doi: 10.1182/blood-2014-10-607465
136. Cianciotti BC, Ruggiero E, Campochiaro C, Oliveira G, Magnani ZI, Baldini M, et al. Cd4+ memory stem T cells recognizing citrullinated epitopes are expanded in patients with rheumatoid arthritis and sensitive to tumor necrosis factor blockade. Arthritis Rheumatol (2020) 72(4):565–75. doi: 10.1002/art.41157
137. Cao J, Zhang C, Han X, Cheng H, Chen W, Qi K, et al. Emerging role of stem cell memory-like T cell in immune thrombocytopenia. Scand J Immunol (2019) 89(3):e12739. doi: 10.1111/sji.12739
138. Vignali D, Cantarelli E, Bordignon C, Canu A, Citro A, Annoni A, et al. Detection and characterization of Cd8(+) autoreactive memory stem T cells in patients with type 1 diabetes. Diabetes (2018) 67(5):936–45. doi: 10.2337/db17-1390
139. Lee YJ, Park JA, Kwon H, Choi YS, Jung KC, Park SH, et al. Role of stem cell-like memory T cells in systemic lupus erythematosus. Arthritis Rheumatol (2018) 70(9):1459–69. doi: 10.1002/art.40524
140. Ribeiro SP, Milush JM, Cunha-Neto E, Kallas EG, Kalil J, Somsouk M, et al. The Cd8+ memory stem T cell (T(Scm)) subset is associated with improved prognosis in chronic hiv-1 infection. J Virol (2014) 88(23):13836–44. doi: 10.1128/jvi.01948-14
141. Mateus J, Lasso P, Pavia P, Rosas F, Roa N, Valencia-Hernández CA, et al. Low frequency of circulating Cd8+ T stem cell memory cells in chronic chagasic patients with severe forms of the disease. PloS Negl Trop Dis (2015) 9(1):e3432. doi: 10.1371/journal.pntd.0003432
142. Flynn JK, Gorry PR. Stem memory T cells (Tscm)-their role in cancer and hiv immunotherapies. Clin Transl Immunol (2014) 3(7):e20. doi: 10.1038/cti.2014.16
143. Zhang Y, Wang N, Ding M, Yang Y, Wang Z, Huang L, et al. Cd40 accelerates the antigen-specific stem-like memory Cd8(+) T cells formation and human papilloma virus (Hpv)-positive tumor eradication. Front Immunol (2020) 11:1012. doi: 10.3389/fimmu.2020.01012
144. Hurton LV, Singh H, Najjar AM, Switzer KC, Mi T, Maiti S, et al. Tethered il-15 augments antitumor activity and promotes a stem-cell memory subset in tumor-specific T cells. Proc Natl Acad Sci USA (2016) 113(48):E7788–e97. doi: 10.1073/pnas.1610544113
145. Zanon V, Pilipow K, Scamardella E, De Paoli F, De Simone G, Price DA, et al. Curtailed T-cell activation curbs effector differentiation and generates Cd8(+) T cells with a naturally-occurring memory stem cell phenotype. Eur J Immunol (2017) 47(9):1468–76. doi: 10.1002/eji.201646732
146. Cieri N, Camisa B, Cocchiarella F, Forcato M, Oliveira G, Provasi E, et al. Il-7 and il-15 instruct the generation of human memory stem T cells from naive precursors. Blood (2013) 121(4):573–84. doi: 10.1182/blood-2012-05-431718
147. Jeza VT, Li X, Chen J, Liang Z, Aggrey AO, Wu X. Il-21 augments rapamycin in expansion of alpha fetoprotein antigen specific stem-Cell-Like memory T cells in vitro. Pan Afr Med J (2017) 27:163. doi: 10.11604/pamj.2017.27.163.11072
148. Hermans D, Gautam S, García-Cañaveras JC, Gromer D, Mitra S, Spolski R, et al. Lactate dehydrogenase inhibition synergizes with il-21 to promote Cd8(+) T cell stemness and antitumor immunity. Proc Natl Acad Sci USA (2020) 117(11):6047–55. doi: 10.1073/pnas.1920413117
149. Lugli E, Galletti G, Boi SK, Youngblood BA. Stem, effector, and hybrid states of memory Cd8(+) T cells. Trends Immunol (2020) 41(1):17–28. doi: 10.1016/j.it.2019.11.004
150. Kondo T, Morita R, Okuzono Y, Nakatsukasa H, Sekiya T, Chikuma S, et al. Notch-mediated conversion of activated T cells into stem cell memory-like T cells for adoptive immunotherapy. Nat Commun (2017) 8:15338. doi: 10.1038/ncomms15338
151. Scholz G, Jandus C, Zhang L, Grandclément C, Lopez-Mejia IC, Soneson C, et al. Modulation of mtor signalling triggers the formation of stem cell-like memory T cells. EBioMedicine (2016) 4:50–61. doi: 10.1016/j.ebiom.2016.01.019
152. Zheng W, O’Hear CE, Alli R, Basham JH, Abdelsamed HA, Palmer LE, et al. Pi3k orchestration of the in vivo persistence of chimeric antigen receptor-modified T cells. Leukemia (2018) 32(5):1157–67. doi: 10.1038/s41375-017-0008-6
153. Mousset CM, Hobo W, Ji Y, Fredrix H, De Giorgi V, Allison RD, et al. Ex vivo akt-inhibition facilitates generation of polyfunctional stem cell memory-like Cd8(+) T cells for adoptive immunotherapy. Oncoimmunology (2018) 7(10):e1488565. doi: 10.1080/2162402x.2018.1488565
154. Crompton JG, Sukumar M, Roychoudhuri R, Clever D, Gros A, Eil RL, et al. Akt inhibition enhances expansion of potent tumor-specific lymphocytes with memory cell characteristics. Cancer Res (2015) 75(2):296–305. doi: 10.1158/0008-5472.Can-14-2277
155. Pilipow K, Scamardella E, Puccio S, Gautam S, De Paoli F, Mazza EM, et al. Antioxidant metabolism regulates Cd8+ T memory stem cell formation and antitumor immunity. JCI Insight (2018) 3(18):e122299. doi: 10.1172/jci.insight.122299
156. Sukumar M, Liu J, Ji Y, Subramanian M, Crompton JG, Yu Z, et al. Inhibiting glycolytic metabolism enhances Cd8+ T cell memory and antitumor function. J Clin Invest (2013) 123(10):4479–88. doi: 10.1172/jci69589
157. Godec J, Cowley GS, Barnitz RA, Alkan O, Root DE, Sharpe AH, et al. Inducible rnai in vivo reveals that the transcription factor batf is required to initiate but not maintain Cd8+ T-cell effector differentiation. Proc Natl Acad Sci USA (2015) 112(2):512–7. doi: 10.1073/pnas.1413291112
158. Kagoya Y, Nakatsugawa M, Yamashita Y, Ochi T, Guo T, Anczurowski M, et al. Bet bromodomain inhibition enhances T cell persistence and function in adoptive immunotherapy models. J Clin Invest (2016) 126(9):3479–94. doi: 10.1172/jci86437
159. Wu S, Zhu W, Peng Y, Wang L, Hong Y, Huang L, et al. The antitumor effects of vaccine-activated Cd8(+) T cells associate with weak tcr signaling and induction of stem-like memory T cells. Cancer Immunol Res (2017) 5(10):908–19. doi: 10.1158/2326-6066.Cir-17-0016
160. Jeannet G, Boudousquié C, Gardiol N, Kang J, Huelsken J, Held W. Essential role of the wnt pathway effector tcf-1 for the establishment of functional Cd8 T cell memory. Proc Natl Acad Sci USA (2010) 107(21):9777–82. doi: 10.1073/pnas.0914127107
161. Boudousquié C, Danilo M, Pousse L, Jeevan-Raj B, Angelov GS, Chennupati V, et al. Differences in the transduction of canonical wnt signals demarcate effector and memory Cd8 T cells with distinct recall proliferation capacity. J Immunol (2014) 193(6):2784–91. doi: 10.4049/jimmunol.1400465
162. Zhang JY, Zhao YL, Lv YP, Cheng P, Chen W, Duan M, et al. Modulation of Cd8(+) memory stem T cell activity and glycogen synthase kinase 3β inhibition enhances anti-tumoral immunity in gastric cancer. Oncoimmunology (2018) 7(4):e1412900. doi: 10.1080/2162402x.2017.1412900
163. Li W, Lu L, Lu J, Wang X, Yang C, Jin J, et al. Cgas-Sting-Mediated DNA sensing maintains Cd8(+) T cell stemness and promotes antitumor T cell therapy. Sci Transl Med (2020) 12(549):eaay9013. doi: 10.1126/scitranslmed.aay9013
164. Pearce EL, Walsh MC, Cejas PJ, Harms GM, Shen H, Wang LS, et al. Enhancing Cd8 T-cell memory by modulating fatty acid metabolism. Nature (2009) 460(7251):103–7. doi: 10.1038/nature08097
165. Verma V, Jafarzadeh N, Boi S, Kundu S, Jiang Z, Fan Y, et al. Mek inhibition reprograms Cd8(+) T lymphocytes into memory stem cells with potent antitumor effects. Nat Immunol (2021) 22(1):53–66. doi: 10.1038/s41590-020-00818-9
166. Guedan S, Posey AD Jr., Shaw C, Wing A, Da T, Patel PR, et al. Enhancing car T cell persistence through icos and 4-1bb costimulation. JCI Insight (2018) 3(1):e96976. doi: 10.1172/jci.insight.96976
167. Van Braeckel-Budimir N, Dolina JS, Wei J, Wang X, Chen SH, Santiago P, et al. Combinatorial immunotherapy induces tumor-infiltrating Cd8(+) T cells with distinct functional, migratory, and stem-like properties. J Immunother Cancer (2021) 9(12):e003614. doi: 10.1136/jitc-2021-003614
168. Riether C, Schürch CM, Bührer ED, Hinterbrandner M, Huguenin AL, Hoepner S, et al. Cd70/Cd27 signaling promotes blast stemness and is a viable therapeutic target in acute myeloid leukemia. J Exp Med (2017) 214(2):359–80. doi: 10.1084/jem.20152008
169. Wang Y, Qiu F, Xu Y, Hou X, Zhang Z, Huang L, et al. Stem cell-like memory T cells: The generation and application. J Leukoc Biol (2021) 110(6):1209–23. doi: 10.1002/jlb.5mr0321-145r
170. Yao C, Lou G, Sun HW, Zhu Z, Sun Y, Chen Z, et al. Bach2 enforces the transcriptional and epigenetic programs of stem-like Cd8(+) T cells. Nat Immunol (2021) 22(3):370–80. doi: 10.1038/s41590-021-00868-7
171. Roychoudhuri R, Clever D, Li P, Wakabayashi Y, Quinn KM, Klebanoff CA, et al. Bach2 regulates Cd8(+) T cell differentiation by controlling access of ap-1 factors to enhancers. Nat Immunol (2016) 17(7):851–60. doi: 10.1038/ni.3441
172. Kagoya Y, Nakatsugawa M, Ochi T, Cen Y, Guo T, Anczurowski M, et al. Transient stimulation expands superior antitumor T cells for adoptive therapy. JCI Insight (2017) 2(2):e89580. doi: 10.1172/jci.insight.89580
173. Kavazović I, Han H, Balzaretti G, Slinger E, Lemmermann NAW, Ten Brinke A, et al. Eomes broadens the scope of Cd8 T-cell memory by inhibiting apoptosis in cells of low affinity. PloS Biol (2020) 18(3):e3000648. doi: 10.1371/journal.pbio.3000648
174. Kondo T, Imura Y, Chikuma S, Hibino S, Omata-Mise S, Ando M, et al. Generation and application of human induced-stem cell memory T cells for adoptive immunotherapy. Cancer Sci (2018) 109(7):2130–40. doi: 10.1111/cas.13648
175. Tabler CO, Lucera MB, Haqqani AA, McDonald DJ, Migueles SA, Connors M, et al. Cd4+ memory stem cells are infected by hiv-1 in a manner regulated in part by Samhd1 expression. J Virol (2014) 88(9):4976–86. doi: 10.1128/jvi.00324-14
176. Heikkilä N, Hetemäki I, Sormunen S, Isoniemi H, Kekäläinen E, Saramäki J, et al. Peripheral differentiation patterns of human T cells. Eur J Immunol (2022) 52(6):882–94. doi: 10.1002/eji.202149465
177. Vodnala SK, Eil R, Kishton RJ, Sukumar M, Yamamoto TN, Ha NH, et al. T Cell stemness and dysfunction in tumors are triggered by a common mechanism. Science (2019) 363(6434):eaau0135. doi: 10.1126/science.aau0135
178. Eil R, Vodnala SK, Clever D, Klebanoff CA, Sukumar M, Pan JH, et al. Ionic immune suppression within the tumour microenvironment limits T cell effector function. Nature (2016) 537(7621):539–43. doi: 10.1038/nature19364
179. Wherry EJ, Kurachi M. Molecular and cellular insights into T cell exhaustion. Nat Rev Immunol (2015) 15(8):486–99. doi: 10.1038/nri3862
180. Wei SC, Anang NAS, Sharma R, Andrews MC, Reuben A, Levine JH, et al. Combination anti-Ctla-4 plus anti-Pd-1 checkpoint blockade utilizes cellular mechanisms partially distinct from monotherapies. Proc Natl Acad Sci USA (2019) 116(45):22699–709. doi: 10.1073/pnas.1821218116
181. de Klerk LK, Patel AK, Derks S, Pectasides E, Augustin J, Uduman M, et al. Phase ii study of pembrolizumab in refractory esophageal cancer with correlates of response and survival. J Immunother Cancer (2021) 9(9):e002472. doi: 10.1136/jitc-2021-002472
182. Dammeijer F, van Gulijk M, Mulder EE, Lukkes M, Klaase L, van den Bosch T, et al. The pd-1/Pd-L1-Checkpoint restrains T cell immunity in tumor-draining lymph nodes. Cancer Cell (2020) 38(5):685–700.e8. doi: 10.1016/j.ccell.2020.09.001
183. Kamphorst AO, Pillai RN, Yang S, Nasti TH, Akondy RS, Wieland A, et al. Proliferation of pd-1+ Cd8 T cells in peripheral blood after pd-1-Targeted therapy in lung cancer patients. Proc Natl Acad Sci USA (2017) 114(19):4993–8. doi: 10.1073/pnas.1705327114
184. Navani V, Graves MC, Marchett GC, Mandaliya H, Bowden NA, van der Westhuizen A. Overall survival in metastatic melanoma correlates with pembrolizumab exposure and T cell exhaustion markers. Pharmacol Res Perspect (2021) 9(4):e00808. doi: 10.1002/prp2.808
185. Koppolu V, Rekha Vasigala VK. Checkpoint immunotherapy by nivolumab for treatment of metastatic melanoma. J Cancer Res Ther (2018) 14(6):1167–75. doi: 10.4103/jcrt.JCRT_1290_16
186. Powles T, Kockx M, Rodriguez-Vida A, Duran I, Crabb SJ, van der Heijden MS, et al. Clinical efficacy and biomarker analysis of neoadjuvant atezolizumab in operable urothelial carcinoma in the abacus trial. Nat Med (2019) 25(11):1706–14. doi: 10.1038/s41591-019-0628-7
187. Ma Y, Li J, Wang H, Chiu Y, Kingsley CV, Fry D, et al. Combination of pd-1 inhibitor and Ox40 agonist induces tumor rejection and immune memory in mouse models of pancreatic cancer. Gastroenterology (2020) 159(1):306–19.e12. doi: 10.1053/j.gastro.2020.03.018
188. Sakuishi K, Apetoh L, Sullivan JM, Blazar BR, Kuchroo VK, Anderson AC. Targeting Tim-3 and pd-1 pathways to reverse T cell exhaustion and restore anti-tumor immunity. J Exp Med (2010) 207(10):2187–94. doi: 10.1084/jem.20100643
189. Tracy SI, Venkatesh H, Hekim C, Heltemes-Harris LM, Knutson TP, Bachanova V, et al. Combining nilotinib and pd-L1 blockade reverses Cd4+ T-cell dysfunction and prevents relapse in acute b-cell leukemia. Blood (2022) 140(4):335–48. doi: 10.1182/blood.2021015341
190. Vaddepally RK, Kharel P, Pandey R, Garje R, Chandra AB. Review of indications of fda-approved immune checkpoint inhibitors per nccn guidelines with the level of evidence. Cancers (Basel) (2020) 12(3):738. doi: 10.3390/cancers12030738
191. Larkin J, Chiarion-Sileni V, Gonzalez R, Grob JJ, Rutkowski P, Lao CD, et al. Five-year survival with combined nivolumab and ipilimumab in advanced melanoma. N Engl J Med (2019) 381(16):1535–46. doi: 10.1056/NEJMoa1910836
192. Hashimoto M, Araki K, Cardenas MA, Li P, Jadhav RR, Kissick HT, et al. Pd-1 combination therapy with il-2 modifies Cd8(+) T cell exhaustion program. Nature (2022) 610(7930):173–81. doi: 10.1038/s41586-022-05257-0
193. Codarri Deak L, Nicolini V, Hashimoto M, Karagianni M, Schwalie PC, Lauener L, et al. Pd-1-Cis il-2r agonism yields better effectors from stem-like Cd8(+) T cells. Nature (2022) 610(7930):161–72. doi: 10.1038/s41586-022-05192-0
194. Sawant DV, Yano H, Chikina M, Zhang Q, Liao M, Liu C, et al. Adaptive plasticity of il-10(+) and il-35(+) T(Reg) cells cooperatively promotes tumor T cell exhaustion. Nat Immunol (2019) 20(6):724–35. doi: 10.1038/s41590-019-0346-9
195. Sun Z, Fourcade J, Pagliano O, Chauvin JM, Sander C, Kirkwood JM, et al. Il10 and pd-1 cooperate to limit the activity of tumor-specific Cd8+ T cells. Cancer Res (2015) 75(8):1635–44. doi: 10.1158/0008-5472.Can-14-3016
196. Adams SF, Grimm AJ, Chiang CL, Mookerjee A, Flies D, Jean S, et al. Rapid tumor vaccine using toll-like receptor-activated ovarian cancer ascites monocytes. J Immunother Cancer (2020) 8(2):e000875. doi: 10.1136/jitc-2020-000875
197. Yang ZZ, Grote DM, Xiu B, Ziesmer SC, Price-Troska TL, Hodge LS, et al. Tgf-B upregulates Cd70 expression and induces exhaustion of effector memory T cells in b-cell non-hodgkin’s lymphoma. Leukemia (2014) 28(9):1872–84. doi: 10.1038/leu.2014.84
198. Kwon M, Kim CG, Lee H, Cho H, Kim Y, Lee EC, et al. Pd-1 blockade reinvigorates bone marrow Cd8(+) T cells from patients with multiple myeloma in the presence of tgfβ inhibitors. Clin Cancer Res (2020) 26(7):1644–55. doi: 10.1158/1078-0432.Ccr-19-0267
199. Melisi D, Garcia-Carbonero R, Macarulla T, Pezet D, Deplanque G, Fuchs M, et al. Galunisertib plus gemcitabine vs. gemcitabine for first-line treatment of patients with unresectable pancreatic cancer. Br J Cancer (2018) 119(10):1208–14. doi: 10.1038/s41416-018-0246-z
200. Ponnan SM, Vidyavijayan KK, Thiruvengadam K, Hilda JN, Mathayan M, Murugavel KG, et al. Role of circulating T follicular helper cells and stem-like memory Cd4(+) T cells in the pathogenesis of hiv-2 infection and disease progression. Front Immunol (2021) 12:666388. doi: 10.3389/fimmu.2021.666388
201. Yi L, Yang L. Stem-like T cells and niches: Implications in human health and disease. Front Immunol (2022) 13:907172. doi: 10.3389/fimmu.2022.907172
202. Connolly KA, Kuchroo M, Venkat A, Khatun A, Wang J, William I, et al. A reservoir of stem-like Cd8(+) T cells in the tumor-draining lymph node preserves the ongoing antitumor immune response. Sci Immunol (2021) 6(64):eabg7836. doi: 10.1126/sciimmunol.abg7836
203. Mo F, Yu Z, Li P, Oh J, Spolski R, Zhao L, et al. An engineered il-2 partial agonist promotes Cd8(+) T cell stemness. Nature (2021) 597(7877):544–8. doi: 10.1038/s41586-021-03861-0
204. Gautam S, Fioravanti J, Zhu W, Le Gall JB, Brohawn P, Lacey NE, et al. The transcription factor c-myb regulates Cd8(+) T cell stemness and antitumor immunity. Nat Immunol (2019) 20(3):337–49. doi: 10.1038/s41590-018-0311-z
205. Huang D, Wang Y, Thompson JW, Yin T, Alexander PB, Qin D, et al. Cancer-Cell-Derived gaba promotes B-Catenin-Mediated tumour growth and immunosuppression. Nat Cell Biol (2022) 24(2):230–41. doi: 10.1038/s41556-021-00820-9
206. Hu X, Majchrzak K, Liu X, Wyatt MM, Spooner CJ, Moisan J, et al. In vitro priming of adoptively transferred T cells with a rorγ agonist confers durable memory and stemness in vivo. Cancer Res (2018) 78(14):3888–98. doi: 10.1158/0008-5472.Can-17-3973
207. Pietrobon V, Todd LA, Goswami A, Stefanson O, Yang Z, Marincola F. Improving car T-cell persistence. Int J Mol Sci (2021) 22(19):10828. doi: 10.3390/ijms221910828
208. Liu L, Bi E, Ma X, Xiong W, Qian J, Ye L, et al. Enhanced car-T activity against established tumors by polarizing human T cells to secrete interleukin-9. Nat Commun (2020) 11(1):5902. doi: 10.1038/s41467-020-19672-2
209. Zhang Y, Kozlowska A, Fritz J, Zhao YY, La Torre CP, Cranert S, et al. P-Muc1c-Allo1: A fully allogeneic stem cell memory T cell (Tscm) car-T therapy with broad potential in solid tumor. J Immunother Cancer (2021) 9:A132–A. doi: 10.1136/jitc-2021-SITC2021.123
210. Pang N, Shi J, Qin L, Chen A, Tang Y, Yang H, et al. Il-7 and Ccl19-secreting car-T cell therapy for tumors with positive glypican-3 or mesothelin. J Hematol Oncol (2021) 14(1):118. doi: 10.1186/s13045-021-01128-9
211. Zheng W, Wei J, Zebley CC, Jones LL, Dhungana Y, Wang YD, et al. Regnase-1 suppresses tcf-1+ precursor exhausted T-cell formation to limit car-T-Cell responses against all. Blood (2021) 138(2):122–35. doi: 10.1182/blood.2020009309
212. Kong W, Dimitri A, Wang W, Jung IY, Ott CJ, Fasolino M, et al. Bet bromodomain protein inhibition reverses chimeric antigen receptor extinction and reinvigorates exhausted T cells in chronic lymphocytic leukemia. J Clin Invest (2021) 131(16):e145459. doi: 10.1172/jci145459
213. Madison BB, Patil D, Richter M, Li X, Tong M, Cranert S, et al. Cas-clover is a novel high-fidelity nuclease for safe and robust generation of T(Scm)-enriched allogeneic car-T cells. Mol Ther Nucleic Acids (2022) 29:979–95. doi: 10.1016/j.omtn.2022.06.003
214. Wang Y, Tong C, Dai H, Wu Z, Han X, Guo Y, et al. Low-dose decitabine priming endows car T cells with enhanced and persistent antitumour potential Via epigenetic reprogramming. Nat Commun (2021) 12(1):409. doi: 10.1038/s41467-020-20696-x
215. Wei C, Xia K, Xie Y, Ye S, Ding Y, Liu Z, et al. Combination of 4-1bb and Dap10 promotes proliferation and persistence of Nkg2d(Bbz) car-T cells. Front Oncol (2022) 12:893124. doi: 10.3389/fonc.2022.893124
Keywords: exhaustion, stemness, TSCM, chronic antigenic stimulation, cancer immunotherapy
Citation: Chi X, Luo S, Ye P, Hwang W-L, Cha J-H, Yan X and Yang W-H (2023) T-cell exhaustion and stemness in antitumor immunity: Characteristics, mechanisms, and implications. Front. Immunol. 14:1104771. doi: 10.3389/fimmu.2023.1104771
Received: 22 November 2022; Accepted: 07 February 2023;
Published: 20 February 2023.
Edited by:
Shahram Salek-Ardakani, Pfizer (United States), United StatesReviewed by:
Ramon Arens, Leiden University Medical Center (LUMC), NetherlandsDallas Flies, NextCure, Inc., United States
Copyright © 2023 Chi, Luo, Ye, Hwang, Cha, Yan and Yang. This is an open-access article distributed under the terms of the Creative Commons Attribution License (CC BY). The use, distribution or reproduction in other forums is permitted, provided the original author(s) and the copyright owner(s) are credited and that the original publication in this journal is cited, in accordance with accepted academic practice. No use, distribution or reproduction is permitted which does not comply with these terms.
*Correspondence: Wen-Hao Yang, d2h5MDMzMUBnbWFpbC5jb20=; Xiuwen Yan, c3VyZTgzQGd6aG11LmVkdS5jbg==; Jong-Ho Cha, Y2hhMzg0M0BnbWFpbC5jb20=
†These authors have contributed equally to this work