- 1Department of Internal Medicine, Loma Linda University, Loma Linda, CA, United States
- 2Division of Hematology and Oncology, Department of Medicine, Loma Linda University, Loma Linda, CA, United States
- 3Division of Regenerative Medicine, Department of Medicine, Loma Linda University, Loma Linda, CA, United States
- 4Loma Linda University Cancer Center, Loma Linda, CA, United States
Understanding the factors which shape T-lymphocyte immunity is critical for the development and application of future immunotherapeutic strategies in treating hematological malignancies. The thymus, a specialized central lymphoid organ, plays important roles in generating a diverse T lymphocyte repertoire during the infantile and juvenile stages of humans. However, age-associated thymic involution and diseases or treatment associated injury result in a decline in its continuous role in the maintenance of T cell-mediated anti-tumor/virus immunity. Acute myeloid leukemia (AML) is an aggressive hematologic malignancy that mainly affects older adults, and the disease’s progression is known to consist of an impaired immune surveillance including a reduction in naïve T cell output, a restriction in T cell receptor repertoire, and an increase in frequencies of regulatory T cells. As one of the most successful immunotherapies thus far developed for malignancy, T-cell-based adoptive cell therapies could be essential for the development of a durable effective treatment to eliminate residue leukemic cells (blasts) and prevent AML relapse. Thus, a detailed cellular and molecular landscape of how the adult thymus functions within the context of the AML microenvironment will provide new insights into both the immune-related pathogenesis and the regeneration of a functional immune system against leukemia in AML patients. Herein, we review the available evidence supporting the potential correlation between thymic dysfunction and T-lymphocyte impairment with the ontogeny of AML (II-VI). We then discuss how the thymus could impact current and future therapeutic approaches in AML (VII). Finally, we review various strategies to rejuvenate thymic function to improve the precision and efficacy of cancer immunotherapy (VIII).
1 Introduction
Cancer immunotherapy has revolutionized the treatment landscape for different tumors and has demonstrated the feasibility of leveraging the host’s immune system to control and eliminate malignant cells (1). In hematological cancers, the discovery of the graft-vs-leukemia (GVL) effect after allogeneic hematopoietic stem cell transplantation (allo-HSCT) demonstrated that leukemia is susceptible to immune-mediated eradication (2). More recently in the last decade, the development of immune checkpoint inhibitors (ICIs) and genetically engineered chimeric antigen receptor (CAR)-T cell therapy has resulted in promising therapeutic responses in certain hematological malignancies (3–7).
T lymphocytes are major contributors to the success of these immunotherapies; however, optimal responses rely on the persistent availability of a diverse and functional T lymphocyte repertoire capable of recognizing tumor neo-antigens and eliciting cytotoxicity (8). The thymus constitutes of a lymphoid organ dedicated to generating new functional T lymphocytes and ‘educating’ them to recognize a wide diversity of tumor and pathogenic antigens while simultaneously avoiding self-reactivity (9). However, this process is significantly impaired in the adult thymus as a result of age and/or environmental insults, such as infections or cytoreductive therapies, and it may have serious consequences on the clinical efficacy of immune-based therapies (10).
Despite the success of allo-HSCT to treat younger patients, the treatment of acute myeloid leukemia (AML) in older patients and those with relapsed or refractory disease remains challenging in part due to poor immune T-cell responses (11). An emerging body of evidence has shown that AML patients have numerically and functionally defective T cells and NK cells at the time of diagnosis (12–14). The variable efficacy of T cell-based immunotherapeutic strategies underscores the compelling need to decipher the factors which shape the T-cell landscape in AML.
To date, major efforts have been made to understand the immune landscape within the context of the bone marrow microenvironment in AML (15, 16). However, the role of the thymic microenvironment in propagating AML progression is largely underrecognized, but a potentially important aspect of AML biology. In this review, we 1) provide an overview of our current knowledge of T cell development in the thymus (II-VI), 2) link the potential association between T-lymphocyte impairment caused by thymus dysfunction and the ontogeny of AML (VII), and 3) hypothesize how thymic regeneration may improve future immunotherapeutic strategies for the treatment of AML (VIII).
2 The role of thymus in T cell development
For centuries, the thymus was misconceived to be a vestigial organ or at most a ‘graveyard’ for old and dying lymphocytes. The observation that adult mice who underwent thymectomy had no defects in immune response and that the thymus shrank after infection and in adulthood initially suggested to many scientists that its function was inconsequential to the immune system. However an important paradigm shift occurred in the early 1960s, when Jacques Miller discovered that the removal of the thymus during neonatal development could cause marked lymphocyte deficiency (17). This was the first demonstration that the thymus played an essential role in immunological function and paved the way for fundamental discoveries in our understanding of T cell development, potentially transforming the way we practice modern medicine (Figure 1).
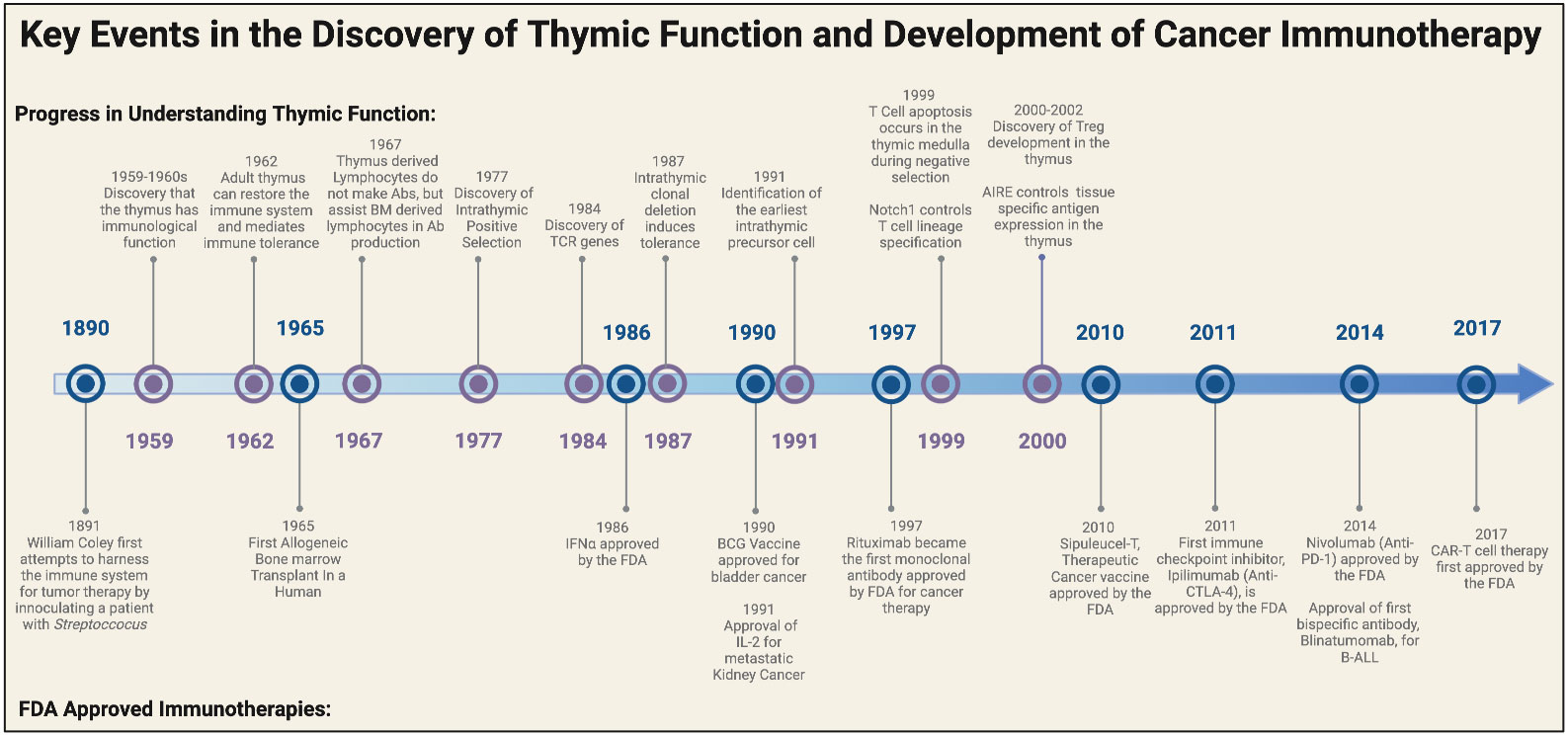
Figure 1 Historical timeline of major discoveries on the thymus and the development of FDA approved cancer immunotherapies. Major breakthroughs in understanding the functional role of the thymus began in the late 1950s to early 1960s. These landmark discoveries paved the way for breakthroughs in T cell biology and eventually ushered in a new era for cancer immunotherapy. Elements of this figure were generated using Biorender.com.
Since the discoveries made by Miller, it is now accepted that the thymus is the primary site of T cell development and is essential for maintaining homeostatic cellular immunity, central tolerance, and tumor immunity (9). The thymus coordinates the development of cell-mediated antitumor immunity through the generation of a diverse T lymphocyte repertoire capable of recognizing tumor neo-antigens (18–20). The acquisition of a tumor reactive T-cell population is orchestrated via cross talk between the bone marrow microenvironment and the stromal thymic microenvironment, which is formed by a meshwork of medullary and cortical thymic epithelial cells (mTECs/cTECs), macrophages, dendritic cells, fibroblasts and matrix molecules (Figure 2) (21).
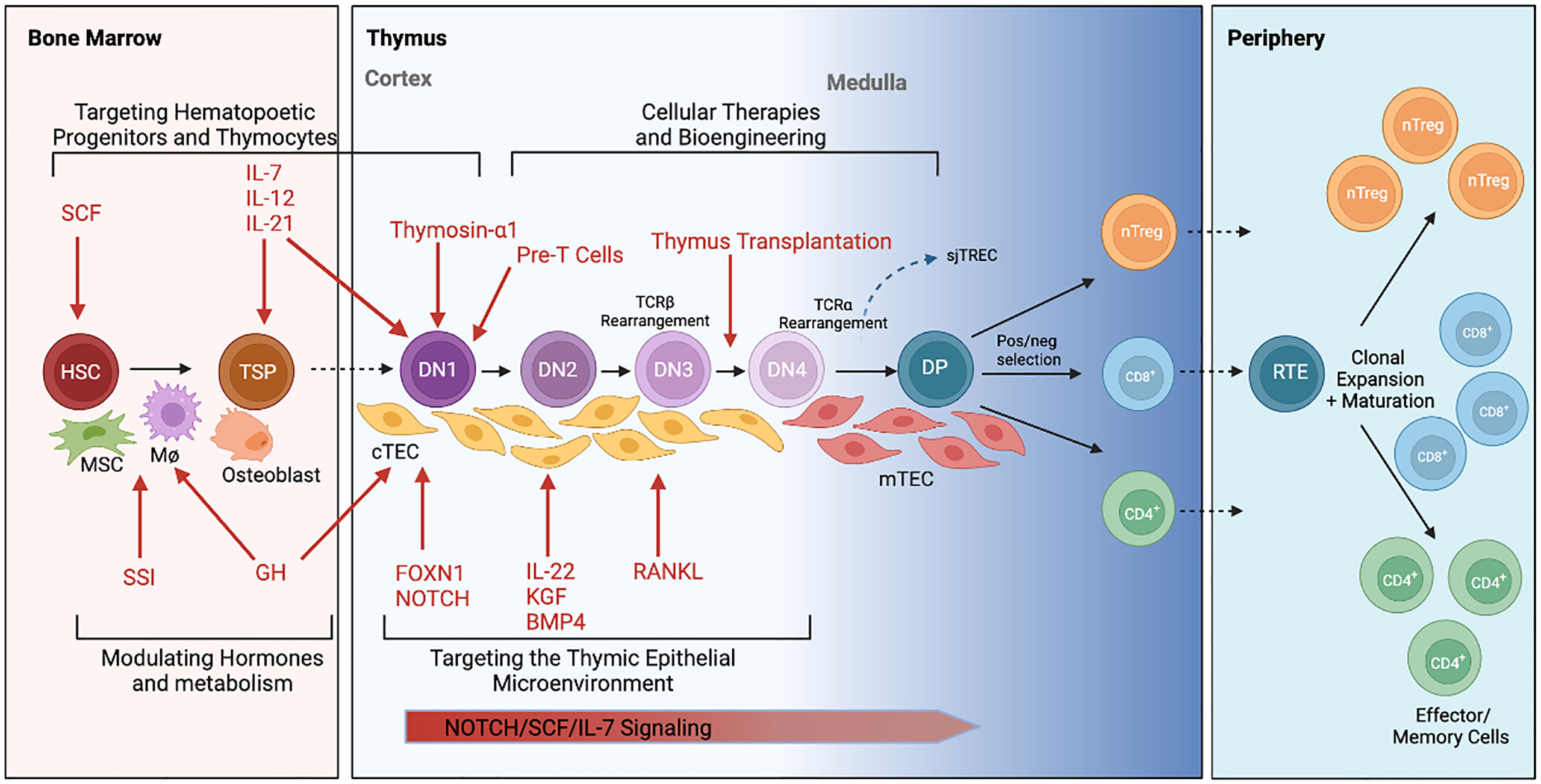
Figure 2 Schematic illustration of intrathymic T cell development and current approaches to thymus rejuvenation. T cell development begins with migration of hematopoietic stem cells (HSC) from the bone marrow to the thymus. These thymic seeding progenitors (TSPs) undergo lineage commitment as they transform from double negative (DN) to double positive (DP) to single positive T cells with the assistance from critical signals within the thymic epithelial microenvironment. T cells are eventually exported out of the thymus as CD4+, CD8+ or regulatory T cells (Tregs) where they function within the periphery. Various therapeutic approaches (in red) to rejuvenating thymic function are currently under investigation. This includes approaches to 1) target hematopoietic progenitors and thymocytes, 2) target the thymic epithelial microenvironment, 3) modulate hormones and metabolism, and 4) develop cellular therapies to replace thymic tissue. Elements of this figure were generated using Biorender.com.
T cell lineage identity is determined by a complex regulatory network of genetic interactions that are initially triggered by environmental signals and metabolic crosstalk (22, 23). Hematopoietic precursors from the bone marrow migrating to the thymus, termed thymocytes, receive a series of complex but critical signals (e.g. Notch ligands, Interleukin (IL)-7, and stem cell factor) from TECs to promote the survival and lineage commitment to CD4 or CD8 single positive T cells (Figure 2) (24, 25). The generation of unique T cell receptors (TCRs) able to recognize diverse antigenic peptides is a crucial step for T-cell development in the thymus and involves the somatic rearrangement of three complementary-determining regions (CDRs) of the TCR (26). During each step of T-cell receptor rearrangement, DNA fragments between rearranged gene segments are deleted as circular signal joint T cell receptor excision circles (sjTRECs), which are frequently evaluated using RT-PCR to quantify thymic activity (27).
Finally, the survival and differentiation of T cells is determined by positive and negative selection induced by interactions between TCR and major histocompatibility complex (MHC)/self-antigens. Strong TCR signals lead to clonal deletion of potentially dangerous, highly self-reactive CD4+ T cells, whereas weak signals lead to generation of conventional T cells (28). Prior to exiting the thymus, a proportion of CD4+ T cells with intermediate TCR signals differentiate into forkhead box P3 (FOXP3) expressing regulatory T cells (Tregs) (29, 30).
3 Age related thymic involution and AML
Age-associated alterations to the immune system result in progressive immunosenescence and is believed to potentially contribute to the increasing incidence of cancer in older patients (31, 32). Similarly, patients with advanced age constitute a large subset of patients with AML and are more often found to have unfavorable cytogenetics, multidrug resistance, and poor prognosis. This may suggest that there are differences in AML biology that occur with aging (33–35).
The physiological involution of the thymus after the first year of life is perhaps the most pronounced change to the aging immune system in humans (36). This is characterized by the profound perturbation of the thymic stromal microenvironment, including the loss of thymic epithelial cells and progressive replacement of healthy tissue with adipose tissue (37, 38). While the atrophied thymus is still capable of producing new T cells, age-related involution is well known to severely compromise naïve T cell output and perturb signal strength between thymocyte TCR and mTEC MHC-II, resulting in impaired thymic negative selection and enhanced thymic Treg generation (20). Thymic involution has additionally been shown to result in the expansion of oligoclonal peripheral memory T cells, thereby restricting TCR repertoire diversity and skewing type 1/type 2 cytokine production profiles (39, 40).
Reduced T cell-mediated tumor immune surveillance is a likely consequence of these age-related changes and may at least partially explain the clinical and biological differences of AML in older patients. A recent survey of pre-treated AML BM using multiplex immunohistochemical analysis demonstrated 2 major immunologic AML clusters differing significantly in age (median 54.8 vs 64.6 years). AML patients in the older cluster were observed to have higher TCR clonality as well as higher proportions of OX40+ and memory CD45RO+ T cells. Importantly, the higher TCR clonality in the older cluster correlated with higher age and worse prognosis (41). Thus, it’s possible that age-related thymic involution is one of the causative mechanisms leading to the decline in T cell-mediated tumor immune surveillance in the elderly who are developing AML (20, 36, 42–44).
Two primary mechanisms have been proposed to explain the observed aged-related decrease in thymopoiesis (45). Firstly, it has been proposed that diminished HSC output and increased rate of apoptosis with age reduces the number of thymus seeding progenitors (TSPs) entering the thymus from the BM. Aging TSPs have been shown to have reduced expansion and differentiation potential through increased Ink4a expression and diminished CD3 expression in DP and SP thymocytes (46–49). Secondly, aged induced defects in the thymic stromal niches also results in defects in thymopoiesis (50, 51). Alterations in thymic architecture are observed as early as puberty, when thymic atrophy is the greatest (52, 53). With increased age, the thymus undergoes notable reduction in TECs, blurred demarcation of thymic cortical and medullary compartments, downregulation of various TEC markers, as well as increased fibrosis and adipose deposition (54–56). Taken together, we hypothesize that these age-related physiological changes including impairments in both recruitment of TSPs and maintenance of thymic architecture might increase the risk of the development of AML in elderly patients.
4 Effect of thymic function on the tumor microenvironment
The dynamic interactions between AML cells and the tumor microenvironment (TME) create a specialized niche to support leukemogenesis and immune evasion (57). Compared to solid tumors, the leukemic TME inherently involves the bone marrow, which serves as a sanctuary for leukemic stem cells, extracellular matrix, stromal cells, immune cells and the soluble factors they secrete (58). Given its role in maintaining T cell repertoire and central tolerance, the thymus is another primary lymphoid organ that should be considered for its role in influencing the AML TME, especially with respect to shaping the T cell milieu. Age-related thymic involution has not been associated with any clinical consequences in healthy individuals, perhaps because residual thymic activity can still persist beyond the seventh decade of life and influence T cell responses, especially after immunological injury (10, 59).
4.1 Thymus influences regulatory T cell populations
Recently, CD4+ CD25+ FOXP3+ Tregs have gained attention for their immunosuppressive capabilities in the AML tumor microenvironment. Accumulating evidence has shown that proportions of Tregs are increased in the peripheral blood and bone marrow of AML patients and are associated with poorer prognosis (41, 60–63). In addition, current research suggests that Tregs promote leukemia cell survival by suppressing both expansion and effector functions of cytotoxic T lymphocytes, as well as directly promoting the stemness and survival of AML blasts through the IL10/PI3K/AKT signaling pathway (64–66).
Tregs are a heterogenous population and can be both naturally derived in the thymus (nTregs), which is believed to make up approximately 80% of Treg repertoire, or induced by conversion of conventional T cells existing in the periphery (iTregs). Whether Tregs within the tumor microenvironment are natural vs induced remains a subject of debate, as they are difficult to distinguish in vivo (67). However, several biomarkers such as Helios, NRP1, and FOXP3 methylation status have been reliably used to identify Tregs of thymic origin (68–70). In this regard, a previous study showed that expanded Tregs from AML patients treated with histamine dihydrochloride (HDC) and low dose IL-2 for relapse prevention predominantly resembled nTregs, based on Helios expression and TSDR methylation status in the FOXP3 gene locus (71). These findings corroborate a previous study showing that a majority of tumor-infiltrating Tregs are thymically derived and reactive to Aire-dependent self-antigens associated with the organ of cancer origin (72). Together, these studies have important clinical implications because thymic involution from aging or chemotherapy has been shown to enhance the generation of nTregs (73).
4.2 Thymus’ role in clonotype TCR diversity
As we have previously discussed, thymus involution has been known to result in limited TCR diversity. Several groups have similarly reported restricted TCR repertoire diversity and skewed oligoclonal αβ+ and γδ+ T- cell pools in AML (74–77). Notably, recent studies have shown restricted expression and oligoclonal expansion of certain Vβ subfamily T cells, such as PD-1+ Vβ T cells in patients with AML and CML (76, 77). The oligoclonal expansion of certain TCR γδ T cells from patients with AML have likewise been reported (78). Although γδ T cells are less studied and constitute a smaller percentage of the human T cell population, there is growing evidence to support its role in antitumor immunity (79). In fact, Vγ9Vδ2 T cells have been shown to specifically recognize and kill AML blasts in a TCR dependent manner (80). A study comparing TCR repertoires between pediatric and adult AML showed that the fraction of γδ T cells increases with age, which is the inverse of what is seen in healthy patients (74). Taken together, it is likely that certain leukemic-specific T cell clones in the TME may be preferentially expanded by AML-associated antigens in the periphery and/or are being restricted during development in the thymus. In support of the latter, a recent study found that effector functions of γδ T cell subsets within the TME was dictated by metabolic programing during early thymic development (81).
4.3 Thymic involution promotes inflammaging and the immunosuppressive TME
Age-related thymic atrophy has been correlated to the development of systemic inflammation with advanced age, termed inflammaging (45). Recent studies also suggest that mTEC dysfunction with age reduces the capacity to eliminate self-reactive thymocytes through impaired negative selection (82, 83). This process has been proposed to potentiate the increasing self-reactivity and auto-inflammation observed with aging (45). It is known that chronic inflammation can aid in tumor progression, metastasis and drug resistance in tumor cells (84), and pro-inflammatory mediators also promote the disease progression and relapse of AML (16, 85). Accordingly, it is thus possible that the disruption in T cell homeostasis derived from thymic atrophy might play a role in AML pathogenesis.
4.4 Thymus’ role in sustained CD8 T cell immunity during chronic antigen exposure
Previous studies have shown that CD8+ T cells are functionally impaired in AML including expressing aberrant phenotypes, having increased expression of exhaustion/senescence molecules (CD57, TIGIT, TIM-3 and PD-1), and forming defective immune synapses with blasts (11, 12, 86–90). While thymic function gradually decreases with age, continued thymopoiesis in residual thymus tissue has been shown to be essential for the generation of new CD8+ T cells to replenish attrition from exhausted T cells during chronic antigen exposure. This has been demonstrated in patients with chronic polyomavirus infection who depend on the reactivation of thymic function and de novo T cell generation to sustain antigen-specific CD8+ T cell immunity (91).
5 AML interferes with intrathymic T-cell development
AML has been shown to promote thymic atrophy, suggesting that AML may somehow damage the thymus and its production of functional T lymphocytes (92, 93). A dramatic reduction of sjTREC was found in peripheral T cells of AML patients when compared to age-matched healthy controls, raising the possibility that immunodeficiency in AML could be derived from the diminished thymic output of T cells (92). Furthermore, AML patients with high sjTREC counts have better disease prognosis, and the sjTREC counts were found to recover to normal values in those patients achieving complete remission (92, 94). Consistent with these clinical findings, another study observed that immune-competent mice challenged with AML blasts also developed premature thymic atrophy, which was characterized by reduced numbers of thymocyte subsets, most notably the DP population (93). Thymic atrophy resulted in significant loss of peripheral CD4+ and CD8+ T-cells with an increased frequency of CD4+Foxp3+ regulatory and activated/memory T cell subsets. The expression of monocyte chemoattractant protein 1 (MCP-1/CCL2) was found to be associated with thymic atrophy and the neutralization of CCL2 via an anti-CCL2 pAb enhanced antileukemic T-cell response and increased the survival of AML mice (93).
6 Can AML originate in the thymus?
AML is believed to originate from transformed myeloid restricted progenitors residing within the bone marrow (95). However, the existence of acute leukemia expressing both myeloid and lymphoid lineage-specific markers, known as mixed-lineage acute leukemia (MPAL), suggests that at least some blast populations may originate from leukemia stem cells possessing multilineage potential (96). By the same token, thymus-seeding progenitors (TSPs) have largely been regarded to have a restricted lineage towards T-lymphocytes. Yet several recent studies have shown that double negative (DN) TSPs may retain the potential to differentiate to alternative hematopoietic fates, including NK, B-cell, and myeloid lineages (97–101). Transformed DN2 murine T-cell progenitors overexpressing oncogenes Myc/Bcl2 have been shown to have high intrinsic potential to transdifferentiate into myeloid and biphenotypic leukemia. Remarkably, the resulting murine DN-2 derived leukemia was found to be genetically similar to a human AML cohort (102). Moreover, murine DN2 with a known human NUP98-HOXD13 (NHD13) fusion transcript have a predisposition to transform into a highly aggressive AML with similar gene expression profiles of several human AML subsets, including those with NPM1 mutations, MLL fusions and NUP98 fusions (103, 104). While rare, several case reports have described AML with involvement of the thymus/mediastinum without evidence of BM infiltration (105–108). Together, these studies pose the possibility that the thymus can be involved in the leukemogenesis of AML, and some subsets of AML might originate from thymic precursors.
7 Implication of thymic function on current therapies for AML
7.1 Cytoreductive therapies impair de novo T Cell recovery
The thymus is exquisitely sensitive to cytoreductive therapies that are traditionally used as the standard of care in AML, such as chemotherapies or radiation therapies. While all hematopoietic cell types are affected following chemotherapy, T cells appear to be most profoundly impacted, likely in part due to depletion of thymocytes and TECS within the thymic stromal compartment (109, 110). The recovery of the depleted T cell pool after cytoreductive therapy may take many years or be permanent (111, 112). Age-related thymic involution has also been linked to impaired immune reconstitution following chemotherapy, which may partially explain treatment failure in the elderly (113–115). Considering the impact of cytoreductive therapies on thymic function and de novo T cell output, adoptive T-cell based immunotherapies utilizing bioengineered allogenic or autologous T cells, such as CAR T cell therapy or tumor-infiltrating lymphocytes (TILs) may be a promising therapeutic approach, either as a supplement to first-line treatments or as a treatment for refractory cases (14, 116).
7.2 Chemotherapy- induced thymic atrophy creates a chemoprotective tumor reservoir
Relapsed and refractory disease presents a significant challenge and is primarily responsible for the poor prognosis in AML. The emergence of remnant populations of leukemic cells after exposure to chemotherapy, termed measurable residual disease (MRD), implies that AML cells may develop drug-resistance or mechanisms to evade exposure to therapy. Several premetastatic reservoirs, such as the bone marrow and the perivascular space of blood vessels, have been identified as potential reservoirs that permit primary tumor cells to hide following adjuvant chemotherapy (117–119). When damaged by chemotherapy, the atrophied and inflamed thymus has likewise been found to create a chemoprotective microenvironment for both solid and hematologic tumor cells (120–122). Notably, the release of the proinflammatory cytokines TIMP1 and IL-6 from TECs in response to cytotoxic chemotherapy has been shown to induce TEC senescence and promote lymphoma cell survival (121). Exposure to chemotherapy also induces thymic residing tumor cells to acquire an antiapoptotic chemo-resistant phenotype within the inflammatory thymic microenvironment (120). Finally, the presence of tumor cells within the thymus may also interfere with de novo T cell differentiation and lead to tumor-specific immune tolerance. This hypothesis is supported by several studies that have shown that infectious pathogens that invade the thymus lead to microbe-specific T cell tolerance (123–127). Together, these findings suggest that the atrophied thymic microenvironment may be chemoprotective to AML blasts, harbor MRD following adjuvant therapy, and result in tumor-specific T cell tolerance that leads to an eventual tumor relapse.
7.3 FLT3 ligand and CXCR4/CXCL12 regulates thymic precursors
FMS-like tyrosine kinase 3 (FLT3) is widely expressed by immature hematopoietic progenitors and over-expressed in a majority of AML blasts, often in the presence of activating mutations by tandem duplications (FLT3-ITD) and/or point mutations involving the tyrosine kinase domain (TKD) (128). The presence of FLT3-ITD is widely accepted to portend a poor prognosis in AML due to chemoresistance and the high rate of relapse. Recent data has suggested that FLT3 and its respective ligand (FLT3L) may be an important point of regulation for thymic function. FLT3L knockout mice were notably observed to have reduced immature thymocytes and Lin-SCA1+KIT+ (LSK) lymphoid-primed multipotent marrow progenitors (129, 130). More importantly, administration of FLT3 ligand was found to enhance the export and survival of LSK cells, early thymic progenitors after transplantation, and androgens via the downregulation of CXCR4 (131). Potentially, these findings have important clinical implications because the use of FLT3 inhibitors after HSCT may impair thymic recovery in AML.
7.4 Allogeneic hematopoietic stem cell transplantation
Allo-HSCT is currently the only curative treatment option for AML; though, its success both in regards to its ability to fight opportunistic pathogens and eliminate leukemia depends on optimal recovery of adaptive immunity after cytoreductive conditioning (132). Thymic-independent proliferation of donor-derived T-cells can initially restore peripheral T-cell numbers within the first year following Allo-HSCT. However, because these T-cells are derived from a limited number of donor precursors, their TCR repertoire is of limited diversity (133–136). This has important clinical implications, as lower TCR diversity has been associated with increased risk for disease relapse (137). Therefore, complete T-cell reconstitution with a diverse TCR repertoire may depend on the recovery of de novo T-cell production in the recipient thymus (132, 136, 138). A recent study has importantly demonstrated that some donor-derived CD8 T cells specific for hematopoietic cell-restricted antigen can escape deletion in the thymus and contribute to GVL effects in the periphery (139). Considering that thymic function is also highly sensitive to pre-transplant conditioning regimens, the above findings provide a rationale to developing strategies to boost thymic recovery to improve the outcome of Allo-HSCT (140).
7.5 Graft- versus- host disease
GVHD is a common but significant complication of Allo-HSCT and has additionally been shown to interfere with thymic-dependent T cell development by reducing TSP as well as altering thymic cellularity and architecture (138). This is in part mediated by a glucocorticoid-independent mechanism of DP thymocyte apoptosis, as well as the destruction of mTECs via donor alloreactive T cells by expression IFN-gamma, and the cognate proteins FasL and TNF-related apoptosis-inducing ligand (TRIAL) (138, 141–147). The capacity to affect thymic output after HSCT has been demonstrated by the observation that acute GVHD (aGvHD) is associated with a significant reduction in sjTREC counts (148–150). In chronic GvHD (cGvHD), research has demonstrated that sjTREC levels remain reduced even after recovery and long-term follow-up. However, this effect was transient in young patients <25 years old, highlighting the age-dependent regenerative capacity of the thymus (150).
7.6 Therapeutic cancer vaccines
Therapeutic cancer vaccination to induce remission or prevent relapse has been extensively explored in preclinical and clinical trials over the last decade (151). However, the efficacy of tumor vaccination strategies may be compromised by several tumor extrinsic factors secondary to thymic atrophy, including Treg driven immune suppression, T cell exhaustion, and reduced TCR repertoire. Though the role of thymic atrophy on anti-tumor vaccines has yet to be studied directly, age-related thymic involution has been cited as one cause for the diminished response to traditional vaccination. This has been suggested based on the observation that only an estimated 30-40% of elderly patients are able to mount an adequate immune response to the influenza vaccine (152).
7.7 T-cell directed Immunotherapy
The success of immunotherapy in AML is contingent on the presence of functional T cells capable of recognizing tumor specific antigens. Additionally, the degree of neoantigen heterogeneity, tumor mutational burden, and TCR repertoire diversity has been demonstrated to increase the likelihood of tumor antigen recognition and improve overall survival (153–155). Considering the role of the thymus in maintaining homeostatic T-cell immunity, it is likely that a decline in its function could compromise the efficacy of T-cell directed immunotherapies in AML.
The use of immune checkpoint inhibitors (ICIs) in AML, such as anti-CTLA-4 antibody ipilimumab, and the anti-PD-1 antibody nivolumab have demonstrated limited response compared to its impressive efficacy in solid tumors (156–158). Several strategies have been employed to predict response to ICI in solid tumors, including TCR repertoire profiling by high-throughput sequencing before and after ICI treatment (159). Previous studies in melanoma, gastrointestinal cancers, and relapsed/refractory classical Hodgkin lymphoma have overall shown that a broader TCR profile is associated with superior outcomes in patients receiving ICIs (160–164). Consistently, a recent paired analysis of single cell RNA and TCR repertoire profiling in relapsed/refractory AML demonstrated that TCR repertoires primarily from CD8+ cells expand in patients who responded to PD-1 treatment, but contract in those who were treatment resistant (165). Perhaps assessment of TCR repertoire and even the functional status of the thymus can be used to risk stratify and monitor patients receiving ICI therapy and thereby determine optimal personalized treatment strategies for AML patients.
While the application of genetically engineered CAR-T-cell therapies have been successful for lymphoid malignancies, its use for the treatment of heterogeneous AML faces several unmet challenges (166). This includes the lack of AML-specific cell-surface antigen that minimizes off target toxicity and the suppression of T cell activity and proliferation by AML blasts (167). As previously discussed, the increased Treg frequency following thymic atrophy creates a hostile tumor microenvironment for CAR T cells who are already susceptible to exhaustion via chronic tumor antigen exposure. There is some research to suggest that mature peripheral T cells can re-enter the thymus, localize to the medulla, and alter the stromal microenvironment (168, 169), which was attributed to the onset of thymic involution (170). Remarkably, adoptively transferred syngeneic antigen-specific T cells in lymphopenic mice has been shown to enter the thymus and eliminate thymic dendritic cells (DCs) and mTECs presenting cognate antigen (171). These findings have important clinical implications, as CAR T cells may eradicate tumor antigen expressing APCs that mediate negative selection, thereby enhancing the patient’s endogenous antitumor repertoire. However, given that most AML antigens may also be expressed by normal HSPCs and healthy tissue, caution must be used, as this phenomenon may also augment the risk for autoreactivity via on-target, off-tumor toxicity (116).
8 Strategies to rejuvenate and boost thymic function
As discussed above, the thymus is important for the development of T cell mediated tumor immunity in AML, and boosting its function may be a promising route for improving the efficacy of future therapies. In this regard, several strategies are currently being investigated to rejuvenate thymic function, including approaches to target the TEC microenvironment, thymic/hematopoetic progenitors, thymic growth/metabolism, as well as approaches to generate or transplant new thymus tissue. While few have been successfully translated for clinical use, their use in combination with cancer immunotherapies may be a potential avenue for future research (113, 172). Here, we review promising approaches to improving thymic function within the AML microenvironment landscape (Table 1).
8.1 FOXN1
As previously discussed, the thymic stromal compartment is crucial for intrathymic T cell development. The expression of key transcription factor, Forkhead box N1 (FOXN1) is important for TEC differentiation, thymic organogenesis during embryonic development, antigen processing, thymocyte selection, and has been implicated in regulating age-related thymic involution (257–260). While downregulation of foxn1 gene with age results in functional decline in the TEC compartment, its overexpression has been shown to delay thymic degeneration (257, 261). A study by Bredenkamp and colleagues showed that increased FOXN1 expression is sufficient to drive regeneration of the aged thymus both in regards to its architecture, gene expression and functionality (192). More recently, Oh et al. demonstrated embryonic fibroblasts reprogrammed to overexpress FOXN1 could be engrafted to rejuvenate thymic function in mice (193). While there are currently no ongoing clinical trials, developing therapies which target FOXN1 expression may provide one strategy for regenerating an aged thymus further adversely affected by chemotherapeutic regimens used to treat AML.
8.2 Interleukin-7
Interleukin-7 has come into focus as an important non-redundant regulator of lymphopoiesis and mature T cell homeostasis through both thymic dependent and independent mechanisms (10, 262). In the thymus, IL-7 promotes the survival of DN thymocytes, TCR rearrangement, and lineage differentiation during positive selection (263). Furthermore, IL-7 expression appears to decline with age, correlate with thymic atrophy, and importantly appears to be down-regulated in the peripheral blood of AML patients (264–266). The use of exogenous IL-7 for immune reconstitution has been extensively investigated in several preclinical and clinical studies (262). Administration of IL-7 in mice after T-cell depleted allo-HSCT was found to significantly expand donor-derived thymocytes and peripheral T cells, but remarkably had no effect on alloreactive T cells and the development of graft-versus-host disease (206, 207). Several clinical trials have also evaluated the use of glycosylated recombinant human IL-7 (CYT107) in HIV-1 infected patients and found that IL-7 treatment was not only safe and well tolerated, but could also enhance thymopoeisis as demonstrated by an increased number of recent thymic emigrants (RTEs), increased TREC ratio, and increased TCR repertoire diversity (208–210). Notably, these findings were addressed in a phase 1 clinical trial in which CYT107 was used in recipients of T-cell depleted allo-HSCTs; unfortunately, changes in RTE or TREC levels were only appreciated in a small subset of patients (211).
8.3 Keratinocyte growth factor
Keratinocyte growth factor (KGF) is a potent mitogen expressed by thymic mesenchymal and stromal cells that mediates TEC proliferation and survival through activation of the PI3k-AKT-nuclear factor-kB and p53 pathways (176, 177). Several studies have demonstrated that treatment with exogenous KGR could avert GVHD-related injury, enhance thymopoiesis in HSCT recipients and protect TECs during irradiation-induced injury (176, 267, 268). Furthermore, the use of KGF could reverse age-related thymic involution and restore thymopoiesis in aged mice for up to 2 months after treatment (269). The use of recombinant KGF (palifermin) has since been approved by the US Food and Drug administration for the treatment of oral mucositis in patients receiving intensive chemotherapy (270). Currently, there are multiple clinical trials underway to further investigate the use of palifermin for T cell reconstitution (NCT01233921, NCT03042585, NT02356159 and NCT00593554).
8.4 Sex steroid inhibition
The observation that thymic function rapidly declines after puberty and that castration rejuvenates thymic function has suggested that sex hormones, particularly androgens, play a role in thymic involution (48, 271–273). Consistent with these findings, several studies have demonstrated that sex steroid inhibition (SSI) promotes thymopoeisis by increasing TEC expression of CCL25, Dll4, and Notch signaling pathways (223, 224). The regenerative impact of SSI has further been found to enhance immune function in immunocompromised patients or those undergoing auto-HSCT (274, 275). To date, several drugs have been developed to transiently inhibit sex steroids, such as luteinizing hormone-releasing hormone (LHRH) antagonists, for use in precocious puberty, prostate cancer, breast cancer, and endometriosis. More recently, two clinical trials (NCT01746849 and NCT01338987) are underway to evaluate the effects of SSI on immune reconstitution following allo-HSCT. A pilot study using LHRH agonist (goserelin) administration 3 weeks prior to HSCT has already demonstrated significant increases in naive CD4+ T cells, TRECS, and recovery of TCR repertoire diversity (225).
8.5 Growth hormone
The expression of growth hormone (GH) progressively declines after the third decade of life and has been linked to both hematopoietic and thymic function (194, 276, 277). Given its immunomodulatory effect in humans, several preclinical and clinical trials have investigated the use of exogenous GH to boost immune function and demonstrated that it can reverse thymic atrophy and improve TCR diversity (194–196). Notably, two prospective randomized trials reported that daily recombinant GH injections for 6-10 months could enhance thymic output and TREC levels in HIV-1 infected patients (195, 278). Another trial also remarkably showed that GH treatment reduced PD-1+ CD8 T cells, suggesting that this could also be used to reverse CD8 T cell exhaustion (197). While GH therapy has been approved for use in pediatric patients who develop post-radiation growth disorders after HSCT, GH therapy has yet to be implemented for immune reconstitution (279, 280). Before it may be used for thymic reconstitution, several concerns must be addressed. Firstly, it appears the effect on thymic output appear to only be transient, as discontinuation of GH results in recurrence of thymic atrophy (196). Secondly, GH is associated with many undesirable side effects, including increased risk for cardiovascular disease, stroke, and diabetes. Importantly, GH and growth hormone-releasing hormone (GHRH) have recently been implicated in AML proliferation (281, 282). These side effects reduce the enthusiasm for the use of GH or GHRH in treating AML.
8.6 Notch ligands
The expression of Notch ligands Delta-like-1 and 4 (DLL4 and DLL1) by cTECs is essential for supporting T-lineage commitment (283). In the absence of notch signaling, thymopoiesis is arrested early in T cell development during double negative thymocyte differentiation (284). Conversely, its constitutive activation in HSPC simultaneously results in inhibition of B cell development and promotion of T cell development toward double positive status in the thymus (285). Furthermore, a recent study by Tikhonova et al. investigating the transcriptional changes of the BM microenvironment in response to chemotherapy demonstrated that DLL4 and DLL1 is notably downregulated by the vascular endothelium in response to stress (286).
The potential for Notch signaling for T cell reconstitution has been explored in several studies. For instance, the treatment of lin- Sca-1+ C-kit- (LSK) hematopoietic progenitors with DLL1 ex vivo has been shown to accelerate thymus engraftment and T cell reconstitution after HSCT (204). More recently, it has been shown that activation of a BM-specific Notch/IL-21 signaling axis could lead to ex vivo expansion of T cell progenitors (205). It is important to note that Notch has also been cited as a tumor suppressor in myeloid malignancies and that reduced Notch signaling may play a role in skewing HSPC to premature myeloid lineages that could progress to leukemic myeloid production (287–289). Together, these findings provide a rationale to develop strategies to improve for T cell regeneration that utilize Notch and its downstream signaling pathways for use in treatment of AML.
8.7 Bone morphogenic protein-4
Bone morphogenic protein-4 (BMP4) is a member of the TGF-β superfamily, known for its role in regulating embryonic development (290). However, new research has highlighted its function in thymic tissue regeneration after injury (200, 201). A recent study by Wertheimer et al. showed that TECs increased expression of BMP4 after thymic injury, resulting in increased expression of Foxn1 and its downstream target, Notch ligand Dll4 (200). More remarkably, they found that inhibition of BMP4 resulted in impaired thymic regeneration, and its exogenous administration could rescue its ability to repair. While not yet in clinical trials, these studies highlight BMP4 as a novel target for improving immunologic recovery.
8.8 Cell- based therapies and bioengineering
Adoptive cell-based therapies and de novo thymus synthesis have also been investigated for the purpose of enhancing thymic function. Considering that T cell reconstitution is delayed after HSCT due to the limited availability of hematopoetic progenitors, several groups have investigated the potential of supplying donor precursor T cells to boost thymogenesis at the time of transplant (247–249). By expanding hematopoietic precursor cells ex vivo using Notch-1 stimulation, researchers have demonstrated significant increases in both thymic cellularity and peripheral T cell reconstitution (250–252). Alternatively, others have attempted to generate new thymus epithelial tissue through the isolation and expansion of thymic epithelial progenitor cells (TEPC) from fetal thymi (241, 242).
Here, we have extensively reviewed the regenerative factors that are currently being studied for repair of the dysregulated immune system, some of which are now being investigated in clinical trials (Table 1). We acknowledge the challenge in developing a successful and durable effective immunotherapy for heterogeneous AML in elderly patients, based on the rationale that their declined immune function leads to further disease progression (291). Thus, novel approaches will also be crucial. It has long been recognized from parabiotic mouse studies that there are circulating factors in young mice, which have not yet been identified but which could improve the metabolic and regenerative status of older mice (292). It will then be important to determine if the immune system in the aging mouse also improves with parabiosis. If so, parabiosis could be adapted to studies on the dysregulated immune system in a humanized AML mouse. A serial chronological study of the immune system utilizing the latest genomic techniques, such as single-cell RNA-seq and epigenetic approaches, could disclose key pathways involved in the deterioration of immune regulation with aging and AML. Such mechanistic studies and translational approaches could be applied to both the bone marrow niche and the thymic microenvironment. Searches for important molecular and genetic interactions could be sought as well.
9 Concluding remarks
A growing body of research has demonstrated that hematological malignancies are associated with profound dysregulated immune responses in the host, and this may relate to a worse prognosis and a suboptimal response to immunotherapy. Given the unique role of the thymus in shaping the T cell repertoire, it is conceivable that the crosstalk between the bone marrow and thymic microenvironments contributes to AML’s high rate of therapy resistance and disease relapse, and may have wide repercussions on the future of immunotherapy. As we have briefly reviewed, the thymus is a dynamic primary lymphoid organ impacted by many factors in AML patients, including age, availability of HSC progenitors, treatment with chemotherapeutics or HSCT, and the immunosuppressive tumor microenvironment (Figure 3). At the same time, the thymus influences the composition of the cellular milieu in TME. It is thus reasonable to postulate that perturbations to either the BM or the thymic microenvironment may affect the other and be at least partly responsible for the observed immunosenescence in patients with AML. The intense interest and scrutiny of T-cell based immunotherapies in hematological malignancies lends impetus to developing novel strategies to overcome T cell immune dysfunction in vivo (293). The clinical features of CAR-T cell exhaustion in vivo have been investigated by studying cell proliferation, cytotoxicity, and the median persistence of CAR-T cells in peripheral blood, which was found to be within a range of 20 to 617 days (294). Adequate nutrient levels are essential for restoring mitochondrial bioenergetic function, especially for the survival of functional immune cells and recovery from critical illness for cancer patients (295). Whether a rejuvenated thymus can provide such support to host exhausted CAR-T cells and restore their durable anti-leukemic function remains to be uncovered. Also, the success of T cell therapies relies on a diverse T-cell repertoire shaped by the thymus to be effective. Among the complex mechanisms underlying the multifactorial molecular and cellular interactions between immune senescence, cancer immunosurveillance, cancer immune-editing, and cancer initiation and promotion, thymic atrophy represents one piece of the puzzle (32, 296). Therefore, if immunotherapies are to eventually prove successful, one of future pre-clinical and observational studies should focus on improving our understanding of thymic involution with age, its dysfunction in hematological malignancies and novel strategies that can revitalize thymic function and immunosurveillance.
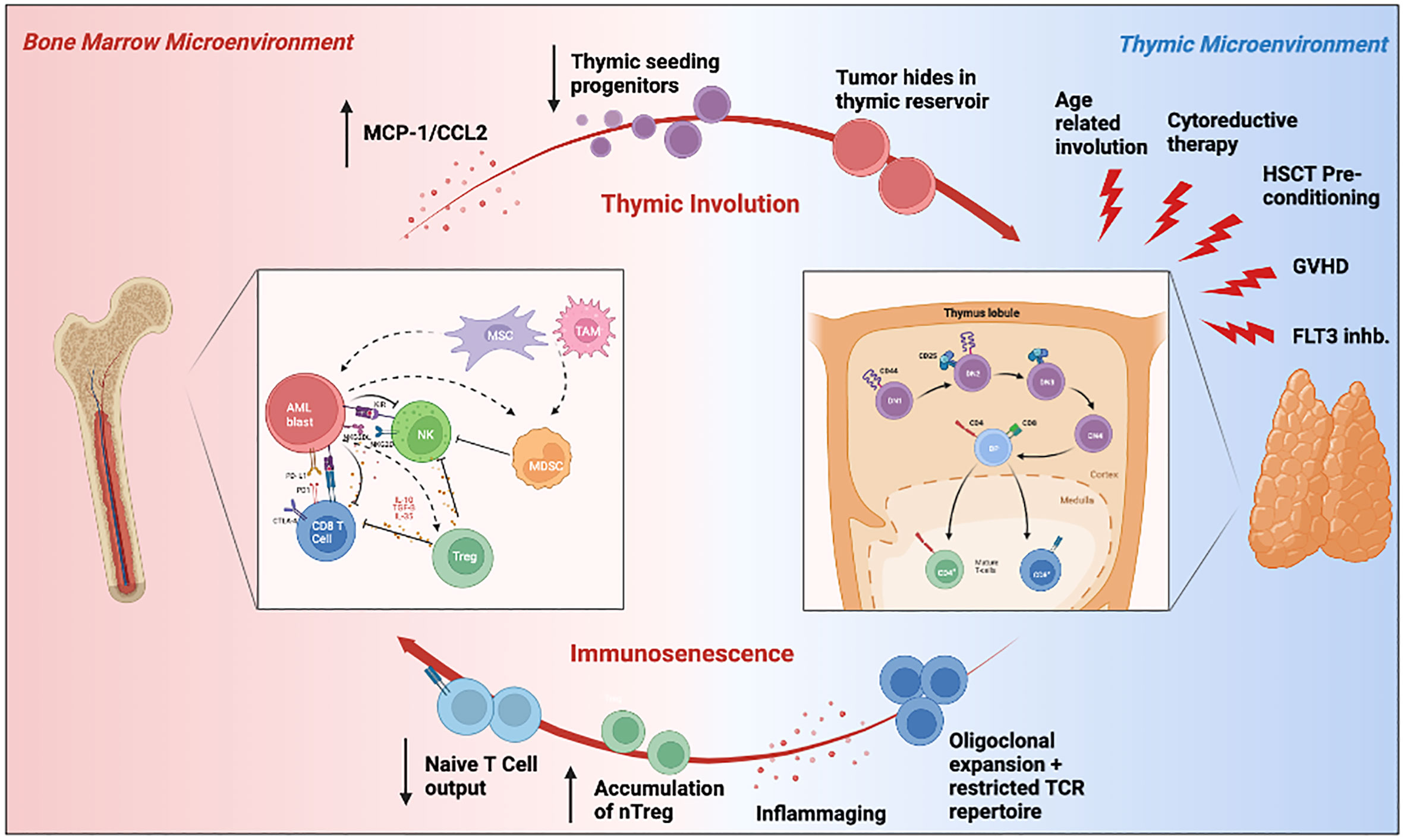
Figure 3 Schematic illustration summarizing the crosstalk between the bone marrow and thymic microenvironment, and its influence on the AML TME. Hematopoietic stem cells derived from the leukemic bone marrow microenvironment can travel to the thymus where they differentiate into T cells. The resulting T cells are then exported to the periphery, where they may then recirculate back into the bone marrow as tumor infiltrating lymphocytes. Environmental insults such as aging, cytoreductive therapy, HSCT pre-conditioning, and FLT3 inhibition may impair thymopoiesis and subsequently promote tumor immune evasion through immunosenscence. The immunosuppressive bone marrow microenvironment can also independently impair thymic function through MCP-1/CCl2 expression and reduction in thymic seeding progenitors. Elements of this figure were generated using Biorender.com.
Author contributions
YX and CH conceived the study. CH prepared the figures, tables and wrote the manuscript. YX, CH, JX, DB, ME, and HC reviewed and edited the manuscript. All authors contributed to the article and approved the submitted version.
Funding
Loma Linda University GRASP 2020 (to Y.X.) and 3R01CA251848-04S1 (to H.C.).
Conflict of interest
The authors declare that the research was conducted in the absence of any commercial or financial relationships that could be construed as a potential conflict of interest.
Publisher’s note
All claims expressed in this article are solely those of the authors and do not necessarily represent those of their affiliated organizations, or those of the publisher, the editors and the reviewers. Any product that may be evaluated in this article, or claim that may be made by its manufacturer, is not guaranteed or endorsed by the publisher.
References
1. Wang JN, Gu T, Hu Y, Huang H. Novel cellular immunotherapies for hematological malignancies: recent updates from the 2021 ASH annual meeting. Exp Hematol Oncol (2022) 11(1):61. doi: 10.1186/s40164-022-00316-8
2. Kolb HJ. Graft-versus-leukemia effects of transplantation and donor lymphocytes. Blood (2008) 112(12):4371–83. doi: 10.1182/blood-2008-03-077974
3. Ribas A, Wolchok JD. Cancer immunotherapy using checkpoint blockade. Science (2018) 359(6382):1350–5. doi: 10.1126/science.aar4060
4. June CH, Sadelain M. Chimeric antigen receptor therapy. N Engl J Med (2018) 379(1):64–73. doi: 10.1056/NEJMra1706169
5. Ansell SM, Lesokhin AM, Borrello I, Halwani A, Scott EC, Gutierrez M, et al. PD-1 blockade with nivolumab in relapsed or refractory hodgkin’s lymphoma. N Engl J Med (2015) 372(4):311–9. doi: 10.1056/NEJMoa1411087
6. Maude SL, Frey N, Shaw PA, Aplenc R, Barrett DM, Bunin NJ, et al. Chimeric antigen receptor T cells for sustained remissions in leukemia. N Engl J Med (2014) 371(16):1507–17. doi: 10.1056/NEJMoa1407222
7. Schuster SJ, Svoboda J, Chong EA, Nasta SD, Mato AR, Anak Ö, et al. Chimeric antigen receptor T cells in refractory b-cell lymphomas. N Engl J Med (2017) 377(26):2545–54. doi: 10.1056/NEJMoa1708566
8. Waldman AD, Fritz JM, Lenardo MJ. A guide to cancer immunotherapy: From T cell basic science to clinical practice. Nat Rev Immunol (2020) 20(11):651–68. doi: 10.1038/s41577-020-0306-5
9. Miller JFAP. The function of the thymus and its impact on modern medicine. Science (2020) 369(6503):eaba2429. doi: 10.1126/science.aba2429
10. Velardi E, Tsai JJ, van den Brink MRM. T Cell regeneration after immunological injury. Nat Rev Immunol (2021) 21(5):277–91. doi: 10.1038/s41577-020-00457-z
11. Lamble AJ, Lind EF. Targeting the immune microenvironment in acute myeloid leukemia: A focus on T cell immunity. Front Oncol (2018) 8. doi: 10.3389/fonc.2018.00213
12. Le Dieu R, Taussig DC, Ramsay AG, Mitter R, Miraki-Moud F, Fatah R, et al. Peripheral blood T cells in acute myeloid leukemia (AML) patients at diagnosis have abnormal phenotype and genotype and form defective immune synapses with AML blasts. Blood (2009) 114(18):3909–16. doi: 10.1182/blood-2009-02-206946
13. Costello RT, Sivori S, Marcenaro E, Lafage-Pochitaloff M, Mozziconacci MJ, Reviron D, et al. Defective expression and function of natural killer cell-triggering receptors in patients with acute myeloid leukemia. Blood (2002) 99(10):3661–7. doi: 10.1182/blood.V99.10.3661
14. Cao H, Kim DH, Howard A, Moz H, Wasnik S, Baylink DJ, et al. Ex vivo isolation, expansion and bioengineering of CCR7+CD95-/or CD62L+CD45RA+ tumor infiltrating lymphocytes from acute myeloid leukemia patients’ bone marrow. Neoplasia (2021) 23(12):1252–60. doi: 10.1016/j.neo.2021.11.003
15. Tettamanti S, Pievani A, Biondi A, Dotti G, Serafini M. Catch me if you can: How AML and its niche escape immunotherapy. Leukemia (2022) 36(1):13–22. doi: 10.1038/s41375-021-01350-x
16. Hino C, Pham B, Park D, Yang C, Nguyen MHK, Kaur S, et al. Targeting the tumor microenvironment in acute myeloid leukemia: The future of immunotherapy and natural products. biomedicines. Biomedicines (2022) 10(6):1410. doi: 10.3390/biomedicines10061410
17. Miller JF. Immunological function of the thymus. Lancet (1961) 2(7205):748–9. doi: 10.1016/S0140-6736(61)90693-6
18. Scheper W, Kelderman S, Fanchi LF, Linnemann C, Bendle G, de Rooij MAJ, et al. Low and variable tumor reactivity of the intratumoral TCR repertoire in human cancers. Nat Med (2019) 25(1):89–94. doi: 10.1038/s41591-018-0266-5
19. Zhang H, Liu L, Zhang J, Chen J, Ye J, Shukla S, et al. Investigation of antigen-specific T-cell receptor clusters in human cancers. Clin Cancer Res Off J Am Assoc Cancer Res (2020) 26(6):1359–71. doi: 10.1158/1078-0432.CCR-19-3249
20. Wang W, Thomas R, Sizova O, Su DM. Thymic function associated with cancer development, relapse, and antitumor immunity – a mini-review. Front Immunol (2020) 11. doi: 10.3389/fimmu.2020.00773
21. Gameiro J, Nagib P, Verinaud L. The thymus microenvironment in regulating thymocyte differentiation. Cell Adhes Migr (2010) 4(3):382–90. doi: 10.4161/cam.4.3.11789
22. Kueh HY, Rothenberg EV. Regulatory gene network circuits underlying T cell development from multipotent progenitors. Wiley Interdiscip Rev Syst Biol Med (2012) 4(1):79–102. doi: 10.1002/wsbm.162
23. Shyer JA, Flavell RA, Bailis W. Metabolic signaling in T cells. Cell Res (2020) 30(8):649–59. doi: 10.1038/s41422-020-0379-5
24. Sambandam A, Maillard I, Zediak VP, Xu L, Gerstein RM, Aster JC, et al. Notch signaling controls the generation and differentiation of early T lineage progenitors. Nat Immunol (2005) 6(7):663–70. doi: 10.1038/ni1216
25. Boudil A, Matei IR, Shih HY, Bogdanoski G, Yuan JS, Chang SG, et al. IL-7 coordinates proliferation, differentiation and tcra recombination during thymocyte β-selection. Nat Immunol (2015) 16(4):397–405. doi: 10.1038/ni.3122
26. Davis MM, Bjorkman PJ. T-Cell antigen receptor genes and T-cell recognition. Nature (1988) 334(6181):395–402. doi: 10.1038/334395a0
27. Douek DC, McFarland RD, Keiser PH, Gage EA, Massey JM, Haynes BF, et al. Changes in thymic function with age and during the treatment of HIV infection. Nature (1998) 396(6712):690–5. doi: 10.1038/25374
28. Klein L, Kyewski B, Allen PM, Hogquist KA. Positive and negative selection of the T cell repertoire: what thymocytes see (and don’t see). Nat Rev Immunol (2014) 14(6):377–91. doi: 10.1038/nri3667
29. Thapa P, Farber DL. The role of the thymus in the immune response. Thorac Surg Clin (2019) 29(2):123–31. doi: 10.1016/j.thorsurg.2018.12.001
30. von Boehmer H. The thymus in immunity and in malignancy. Cancer Immunol Res (2014) 2(7):592–7. doi: 10.1158/2326-6066.CIR-14-0070
31. Aw D, Silva AB, Palmer DB. Immunosenescence: Emerging challenges for an ageing population. Immunology (2007) 120(4):435–46. doi: 10.1111/j.1365-2567.2007.02555.x
32. Palmer S, Albergante L, Blackburn CC, Newman TJ. Thymic involution and rising disease incidence with age. Proc Natl Acad Sci U.S.A. (2018) 115(8):1883–8. doi: 10.1073/pnas.1714478115
33. Zjablovskaja P, Florian MC. Acute myeloid leukemia: Aging and epigenetics. Cancers (2019) 12(1):E103. doi: 10.3390/cancers12010103
34. Büchner T, Berdel WE, Haferlach C, Haferlach T, Schnittger S, Müller-Tidow C, et al. Age-related risk profile and chemotherapy dose response in acute myeloid leukemia: A study by the German acute myeloid leukemia cooperative group. J Clin Oncol (2009) 27(1):61–9. doi: 10.1200/JCO.2007.15.4245
35. Appelbaum FR, Gundacker H, Head DR, Slovak ML, Willman CL, Godwin JE, et al. Age and acute myeloid leukemia. Blood (2006) 107(9):3481–5. doi: 10.1182/blood-2005-09-3724
36. Palmer DB. The effect of age on thymic function. Front Immunol (2013) 4. doi: 10.3389/fimmu.2013.00316
37. Flores KG, Li J, Sempowski GD, Haynes BF, Hale LP. Analysis of the human thymic perivascular space during aging. J Clin Invest (1999) 104(8):1031–9. doi: 10.1172/JCI7558
38. Yang H, Youm YH, Dixit VD. Inhibition of thymic adipogenesis by caloric restriction is coupled with reduction in age-related thymic involution. J Immunol (2009) 183(5):3040–52. doi: 10.4049/jimmunol.0900562
39. Hakim FT, Flomerfelt FA, Boyiadzis M, Gress RE. Aging, immunity and cancer. Curr Opin Immunol (2004) 16(2):151–6. doi: 10.1016/j.coi.2004.01.009
40. Foster AD, Sivarapatna A, Gress RE. The aging immune system and its relationship with cancer. Aging Health (2011) 7(5):707–18. doi: 10.2217/ahe.11.56
41. Brück O, Dufva O, Hohtari H, Blom S, Turkki R, Ilander M, et al. Immune profiles in acute myeloid leukemia bone marrow associate with patient age, T-cell receptor clonality, and survival. Blood Adv (2020) 4(2):274–86. doi: 10.1182/bloodadvances.2019000792
42. Hale JS, Boursalian TE, Turk GL, Fink PJ. Thymic output in aged mice. Proc Natl Acad Sci U.S.A. (2006) 103(22):8447–52. doi: 10.1073/pnas.0601040103
43. Rezzani R, Nardo L, Favero G, Peroni M, Rodella LF. Thymus and aging: morphological, radiological, and functional overview. Age Dordr Neth (2014) 36(1):313–51. doi: 10.1007/s11357-013-9564-5
44. Pawelec G. Does patient age influence anti-cancer immunity? Semin Immunopathol (2019) 41(1):125–31. doi: 10.1007/s00281-018-0697-6
45. Thomas R, Wang W, Su DM. Contributions of age-related thymic involution to immunosenescence and inflammaging. Immun Ageing (2020) 17(1):2. doi: 10.1186/s12979-020-0173-8
46. Sudo K, Ema H, Morita Y, Nakauchi H. Age-associated characteristics of murine hematopoietic stem cells. J Exp Med (2000) 192(9):1273–80. doi: 10.1084/jem.192.9.1273
47. Min H, Montecino-Rodriguez E, Dorshkind K. Reduction in the developmental potential of intrathymic T cell progenitors with age. J Immunol (2004) 173(1):245–50. doi: 10.4049/jimmunol.173.1.245
48. Heng TSP, Goldberg GL, Gray DHD, Sutherland JS, Chidgey AP, Boyd RL. Effects of castration on thymocyte development in two different models of thymic involution. J Immunol (2005) 175(5):2982–93. doi: 10.4049/jimmunol.175.5.2982
49. Berent-Maoz B, Montecino-Rodriguez E, Signer RAJ, Dorshkind K. Fibroblast growth factor-7 partially reverses murine thymocyte progenitor aging by repression of Ink4a. Blood (2012) 119(24):5715–21. doi: 10.1182/blood-2011-12-400002
50. Sun L, Brown R, Chen S, Zhuge Q, Su DM. Aging induced decline in T-lymphopoiesis is primarily dependent on status of progenitor niches in the bone marrow and thymus. Aging Health (2012) 4(9):606–19. doi: 10.18632/aging.100487
51. Su DM, Aw D, Palmer DB. Immunosenescence: a product of the environment? Curr Opin Immunol (2013) 25(4):498–503. doi: 10.1016/j.coi.2013.05.018
52. George AJ, Ritter MA. Thymic involution with ageing: Obsolescence or good housekeeping? Immunol Today (1996) 17(6):267–72. doi: 10.1016/0167-5699(96)80543-3
53. Drabkin MJ, Meyer JI, Kanth N, Lobel S, Fogel J, Grossman J, et al. Age-stratified patterns of thymic involution on multidetector CT. J Thorac Imaging (2018) 33(6):409–16. doi: 10.1097/RTI.0000000000000349
54. Dixit VD. Thymic fatness and approaches to enhance thymopoietic fitness in aging. Curr Opin Immunol (2010) 22(4):521–8. doi: 10.1016/j.coi.2010.06.010
55. Aw D, Silva AB, Maddick M, Von Zglinicki T, Palmer DB. Architectural changes in the thymus of aging mice. Aging Cell (2008) 7(2):158–67. doi: 10.1111/j.1474-9726.2007.00365.x
56. Gui J, Zhu X, Dohkan J, Cheng L, Barnes PF, Su DM. The aged thymus shows normal recruitment of lymphohematopoietic progenitors but has defects in thymic epithelial cells. Int Immunol (2007) 19(10):1201–11. doi: 10.1093/intimm/dxm095
57. Ben Khoud M, Ingegnere T, Quesnel B, Mitra S, Brinster C. Acute myeloid leukemia: Is it T time? Cancers (Basel) (2021) 13(10). doi: 10.3390/cancers13102385
58. Binnewies M, Roberts EW, Kersten K, Chan V, Fearon DF, Merad M, et al. Understanding the tumor immune microenvironment (TIME) for effective therapy. Nat Med (2018) 24(5):541–50. doi: 10.1038/s41591-018-0014-x
59. Jamieson BD, Douek DC, Killian S, Hultin LE, Scripture-Adams DD, Giorgi JV, et al. Generation of functional thymocytes in the human adult. Immunity (1999) 10(5):569–75. doi: 10.1016/S1074-7613(00)80056-4
60. Shenghui Z, Yixiang H, Jianbo W, Kang Y, Laixi B, Yan Z, et al. Elevated frequencies of CD4+CD25+CD127lo regulatory T cells is associated to poor prognosis in patients with acute myeloid leukemia. Int J Cancer (2011) 129(6):1373–81. doi: 10.1002/ijc.25791
61. Szczepanski MJ, Szajnik M, Czystowska M, Mandapathil M, Strauss L, Welsh A, et al. Increased frequency and suppression by regulatory T cells in patients with acute myelogenous leukemia. Clin Cancer Res (2009) 15(10):3325–32. doi: 10.1158/1078-0432.CCR-08-3010
62. Wang X, Zheng J, Liu J, Yao J, He Y, Li X, et al. Increased population of CD4(+)CD25(high), regulatory T cells with their higher apoptotic and proliferating status in peripheral blood of acute myeloid leukemia patients. Eur J Haematol (2005) 75(6):468–76. doi: 10.1111/j.1600-0609.2005.00537.x
63. Ustun C MJ, Munn DH, Weisdorf DJ, Blazar BR. Regulatory T cells in acute myelogenous leukemia: is it time for immunomodulation? Blood (2011) 118(19):5084–95. doi: 10.1182/blood-2011-07-365817
64. Zhou Q, Bucher C, Munger ME, Highfill SL, Tolar J, Munn DH, et al. Depletion of endogenous tumor-associated regulatory T cells improves the efficacy of adoptive cytotoxic T-cell immunotherapy in murine acute myeloid leukemia. Blood (2009) 114(18):3793–802. doi: 10.1182/blood-2009-03-208181
65. Wang R, Feng W, Wang H, Wang L, Yang X, Yang F, et al. Blocking migration of regulatory T cells to leukemic hematopoietic microenvironment delays disease progression in mouse leukemia model. Cancer Lett (2020) 469:151–61. doi: 10.1016/j.canlet.2019.10.032
66. Xu Y, Mou J, Wang Y, Zhou W, Rao Q, Xing H, et al. Regulatory T cells promote the stemness of leukemia stem cells through IL10 cytokine-related signaling pathway. Leukemia (2022) 36(2):403–15. doi: 10.1038/s41375-021-01375-2
67. Adeegbe D, Nishikawa H. Natural and induced T regulatory cells in cancer. Front Immunol (2013) 4. doi: 10.3389/fimmu.2013.00190
68. Shitara K, Nishikawa H. Regulatory T cells: A potential target in cancer immunotherapy. Ann N Y Acad Sci (2018) 1417(1):104–15. doi: 10.1111/nyas.13625
69. Thornton AM, Korty PE, Tran DQ, Wohlfert EA, Murray PE, Belkaid Y, et al. Expression of Helios, an ikaros transcription factor family member, differentiates thymic-derived from peripherally induced Foxp3+ T regulatory cells. J Immunol (2010) 184(7):3433–41. doi: 10.4049/jimmunol.0904028
70. Yadav M, Louvet C, Davini D, Gardner JM, Martinez-Llordella M, Bailey-Bucktrout S, et al. Neuropilin-1 distinguishes natural and inducible regulatory T cells among regulatory T cell subsets in vivo. J Exp Med (2012) 209(10):1713–22. doi: 10.1084/jem.20120822
71. Sander FE, Nilsson M, Rydström A, Aurelius J, Riise RE, Movitz C, et al. Role of regulatory T cells in acute myeloid leukemia patients undergoing relapse-preventive immunotherapy. Cancer Immunol Immunother (2017) 66(11):1473–84. doi: 10.1007/s00262-017-2040-9
72. Malchow S, Leventhal DS, Nishi S, Fischer BI, Shen L, Paner GP, et al. Aire-dependent thymic development of tumor-associated regulatory T cells. Science (2013) 339(6124):1219–24. doi: 10.1126/science.1233913
73. Oh J, Wang W, Thomas R, Su DM. Capacity of tTreg generation is not impaired in the atrophied thymus. PloS Biol (2017) 15(11):e2003352. doi: 10.1371/journal.pbio.2003352
74. Zhang J, Hu X, Wang J, Sahu AD, Cohen D, Song L, et al. Immune receptor repertoires in pediatric and adult acute myeloid leukemia. Genome Med (2019) 11:73. doi: 10.1186/s13073-019-0681-3
75. Feng Z, Fang Q, Kuang X, Liu X, Chen Y, Ma D, et al. Clonal expansion of bone marrow CD8+ T cells in acute myeloid leukemia patients at new diagnosis and after chemotherapy. Am J Cancer Res (2020) 10(11):3973–89.
76. Huang J, Tan J, Chen Y, Huang S, Xu L, Zhang Y, et al. A skewed distribution and increased PD-1+Vβ+CD4+/CD8+ T cells in patients with acute myeloid leukemia. J Leukoc Biol (2019) 106(3):725–32. doi: 10.1002/JLB.MA0119-021R
77. Li Y, Yang L, Chen S, Zhang Y, Wu X. The TCR vβ repertoire usage of T-cells from cord blood induced by chronic myelogenous leukemia associated antigen. Hematology (2005) 10(5):387–92. doi: 10.1080/10245330500226662
78. Jin Z, Luo Q, Lu S, Wang X, He Z, Lai J, et al. Oligoclonal expansion of TCR vδ T cells may be a potential immune biomarker for clinical outcome of acute myeloid leukemia. J Hematol Oncol (2016) 9(1):126. doi: 10.1186/s13045-016-0353-3
79. Silva-Santos B, Serre K, Norell H. γδ T cells in cancer. Nat Rev Immunol (2015) 15(11):683–91. doi: 10.1038/nri3904
80. Gertner-Dardenne J, Castellano R, Mamessier E, Garbit S, Kochbati E, Etienne A, et al. Human Vγ9Vδ2 T cells specifically recognize and kill acute myeloid leukemic blasts. J Immunol (2012) 188(9):4701–8. doi: 10.4049/jimmunol.1103710
81. Lopes N, McIntyre C, Martin S, Raverdeau M, Sumaria N, Kohlgruber AC, et al. Distinct metabolic programs established in the thymus control effector functions of γδ T cell subsets in tumor microenvironments. Nat Immunol (2021) 22(2):179–92. doi: 10.1038/s41590-020-00848-3
82. Coder BD, Wang H, Ruan L, Su DM. Thymic involution perturbs negative selection leading to autoreactive T cells that induce chronic inflammation. J Immunol (2015) 194(12):5825–37. doi: 10.4049/jimmunol.1500082
83. Fletcher AL, Seach N, Reiseger JJ, Lowen TE, Hammett MV, Scott HS, et al. Reduced thymic aire expression and abnormal NF-kappa B2 signaling in a model of systemic autoimmunity. J Immunol (2009) 182(5):2690–9. doi: 10.4049/jimmunol.0801752
84. Greten FR, Grivennikov SI. Inflammation and cancer: Triggers, mechanisms, and consequences. Immunity (2019) 51(1):27–41. doi: 10.1016/j.immuni.2019.06.025
85. Cao H, Tadros V, Hiramoto B, Leeper K, Hino C, Xiao J, et al. Targeting TKI-activated NFKB2-MIF/CXCLs-CXCR2 signaling pathways in FLT3 mutated acute myeloid leukemia reduced blast viability. Biomedicines (2022) 10(5):1038. doi: 10.3390/biomedicines10051038
86. Buggins AGS, Milojkovic D, Arno MJ, Lea NC, Mufti GJ, Thomas NSB, et al. Microenvironment produced by acute myeloid leukemia cells prevents T cell activation and proliferation by inhibition of NF-κB, c-myc, and pRb pathways. J Immunol (2001) 167(10):6021–30. doi: 10.4049/jimmunol.167.10.6021
87. Knaus HA, Berglund S, Hackl H, Blackford AL, Zeidner JF, Montiel-Esparza R, et al. Signatures of CD8+ T cell dysfunction in AML patients and their reversibility with response to chemotherapy. JCI Insight (2018) 3(21). doi: 10.1172/jci.insight.120974
88. Jia B, Zhao C, Rakszawski KL, Claxton DF, Ehmann WC, Rybka WB, et al. Eomes+T-betlow CD8+ T cells are functionally impaired and are associated with poor clinical outcome in patients with acute myeloid leukemia. Cancer Res (2019) 79(7):1635–45. doi: 10.1158/0008-5472.CAN-18-3107
89. Jia B, Wang L, Claxton DF, Ehmann WC, Rybka WB, Mineishi S, et al. Bone marrow CD8 T cells express high frequency of PD-1 and exhibit reduced anti-leukemia response in newly diagnosed AML patients. Blood Cancer J (2018) 8(3):34. doi: 10.1038/s41408-018-0069-4
90. Li C, Chen X, Yu X, Zhu Y, Ma C, Xia R, et al. Tim-3 is highly expressed in T cells in acute myeloid leukemia and associated with clinicopathological prognostic stratification. Int J Clin Exp Pathol (2014) 7(10):6880–8. doi: 10.1182/blood.V122.21.4931.4931
91. Vezys V, Masopust D, Kemball CC, Barber DL, O’Mara LA, Larsen CP, et al. Continuous recruitment of naive T cells contributes to heterogeneity of antiviral CD8 T cells during persistent infection. J Exp Med (2006) 203(10):2263–9. doi: 10.1084/jem.20060995
92. Li Y, Yin Q, Yang L, Chen S, Geng S, Wu X, et al. Reduced levels of recent thymic emigrants in acute myeloid leukemia patients. Cancer Immunol Immunother CII (2009) 58(7):1047–55. doi: 10.1007/s00262-008-0621-3
93. Driss V, Quesnel B, Brinster C. Monocyte chemoattractant protein 1 (MCP-1/CCL2) contributes to thymus atrophy in acute myeloid leukemia. Eur J Immunol (2015) 45(2):396–406. doi: 10.1002/eji.201444736
94. Petridou E, Klimentopoulou AE, Moustaki M, Kostrikis LG, Hatzakis A, Trichopoulos D. Recent thymic emigrants and prognosis in T- and b-cell childhood hematopoietic malignancies. Int J Cancer (2002) 101(1):74–7. doi: 10.1002/ijc.10568
95. Walter RB, Appelbaum FR, Estey EH, Bernstein ID. Acute myeloid leukemia stem cells and CD33-targeted immunotherapy. Blood (2012) 119(26):6198–208. doi: 10.1182/blood-2011-11-325050
96. Wolach O, Stone RM. How I treat mixed-phenotype acute leukemia. Blood (2015) 125(16):2477–85. doi: 10.1182/blood-2014-10-551465
97. Ceredig R, Bosco N, Rolink AG. The b lineage potential of thymus settling progenitors is critically dependent on mouse age. Eur J Immunol (2007) 37(3):830–7. doi: 10.1002/eji.200636728
98. Balciunaite G, Ceredig R, Rolink AG. The earliest subpopulation of mouse thymocytes contains potent T, significant macrophage, and natural killer cell but no b-lymphocyte potential. Blood (2005) 105(5):1930–6. doi: 10.1182/blood-2004-08-3087
99. Bell JJ, Bhandoola A. The earliest thymic progenitors for T cells possess myeloid lineage potential. Nature (2008) 452(7188):764–7. doi: 10.1038/nature06840
100. Luis TC, Luc S, Mizukami T, Boukarabila H, Thongjuea S, Woll PS, et al. Initial seeding of the embryonic thymus by immune-restricted lympho-myeloid progenitors. Nat Immunol (2016) 17(12):1424–35. doi: 10.1038/ni.3576
101. Wada H, Masuda K, Satoh R, Kakugawa K, Ikawa T, Katsura Y, et al. Adult T-cell progenitors retain myeloid potential. Nature (2008) 452(7188):768–72. doi: 10.1038/nature06839
102. Riemke P, Czeh M, Fischer J, Walter C, Ghani S, Zepper M, et al. Myeloid leukemia with transdifferentiation plasticity developing from T-cell progenitors. EMBO J (2016) 35(22):2399–416. doi: 10.15252/embj.201693927
103. Arai Y, Kyo T, Miwa H, Arai K, Kamada N, Kita K, et al. Heterogeneous fusion transcripts involving the NUP98 gene and HOXD13 gene activation in a case of acute myeloid leukemia with the t(2;11)(q31;p15) translocation. Leukemia (2000) 14(9):1621–9. doi: 10.1038/sj.leu.2401881
104. Kundu S, Park ES, Chung YJ, Walker RL, Zhu YJ, Negi V, et al. Thymic precursor cells generate acute myeloid leukemia in NUP98-PHF23/NUP98-HOXD13 double transgenic mice. Sci Rep (2019) 9(1):17213. doi: 10.1038/s41598-019-53610-7
105. Lee JY, Lee SM, Yoon HK, Kim KH, Choi MY, Lee WS. A case of synchronous multiple myeloma and chronic myeloid leukemia. Blood Res (2017) 52(3):219–21. doi: 10.5045/br.2017.52.3.219
106. Maschmeyer G BI, Jähne D, Arnold R, Schega O. Residual thymic tissue and lymph node involvement by acute myeloid leukaemia presenting as mediastinal, strongly 18 FDG-PET-positive masses. Eur J Haematol (2017) 99(3):286–8. doi: 10.1111/ejh.12906
107. Astall E, Yarranton H, Arno J, Marcus R. Granulocytic sarcoma preceding AML M0 and the diagnostic value of CD34. J Clin Pathol (1999) 52(9):705–7. doi: 10.1136/jcp.52.9.705
108. Chubachi A, Miura I, Takahashi N, Nimura T, Imai H, Miura AB. Acute myelogenous leukemia associated with a mediastinal tumor. Leuk Lymphoma (1993) 12(1–2):143–6. doi: 10.3109/10428199309059583
109. Goldberg GL, Dudakov JA, Reiseger JJ, Seach N, Ueno T, Vlahos K, et al. Sex steroid ablation enhances immune reconstitution following cytotoxic antineoplastic therapy in young mice. J Immunol (2010) 184(11):6014–24. doi: 10.4049/jimmunol.0802445
110. Mackall CL, Fleisher TA, Brown MR, Andrich MP, Chen CC, Feuerstein IM, et al. Age, thymopoiesis, and CD4+ T-lymphocyte regeneration after intensive chemotherapy. N Engl J Med (1995) 332(3):143–9. doi: 10.1056/NEJM199501193320303
111. Fagnoni FF, Lozza L, Zibera C, Zambelli A, Ponchio L, Gibelli N, et al. T-Cell dynamics after high-dose chemotherapy in adults: elucidation of the elusive CD8+ subset reveals multiple homeostatic T-cell compartments with distinct implications for immune competence. Immunology (2002) 106(1):27–37. doi: 10.1046/j.1365-2567.2002.01400.x
112. Mackall CL, Brown MR, Brown MR, Andrich MP, Chen CC, Feuerstein IM, et al. Distinctions between CD8+ and CD4+ T-cell regenerative pathways result in prolonged T-cell subset imbalance after intensive chemotherapy. Blood (1997) 89(10):3700–7. doi: 10.1182/blood.V89.10.3700
113. Holland AM, van den Brink MR. Rejuvenation of the aging T cell compartment. Curr Opin Immunol (2009) 21(4):454–9. doi: 10.1016/j.coi.2009.06.002
114. Lynch HE, Goldberg GL, Chidgey A, Van den Brink MRM, Boyd R, Sempowski GD. Thymic involution and immune reconstitution. Trends Immunol (2009) 30(7):366–73. doi: 10.1016/j.it.2009.04.003
115. Hakim FT, Memon SA, Cepeda R, Jones EC, Chow CK, Kasten-Sportes C, et al. Age-dependent incidence, time course, and consequences of thymic renewal in adults. J Clin Invest (2005) 115(4):930–9. doi: 10.1172/JCI200522492
116. Daver N, Alotaibi AS, Bücklein V, Subklewe M. T-Cell-based immunotherapy of acute myeloid leukemia: current concepts and future developments. Leukemia (2021) 35(7):1843–63. doi: 10.1038/s41375-021-01253-x
117. Ghajar CM, Peinado H, Mori H, Matei IR, Evason KJ, Brazier H, et al. The perivascular niche regulates breast tumour dormancy. Nat Cell Biol (2013) 15(7):807–17. doi: 10.1038/ncb2767
118. Shiozawa Y EM, Berry JE, Taichman RS. Bone marrow as a metastatic niche for disseminated tumor cells from solid tumors. BoneKEy Rep (2015) 4:689. doi: 10.1038/bonekey.2015.57
119. Marlow R, Honeth G, Lombardi S, Cariati M, Hessey S, Pipili A, et al. A novel model of dormancy for bone metastatic breast cancer cells. Cancer Res (2013) 73(23):6886–99. doi: 10.1158/0008-5472.CAN-13-0991
120. Sizova O, Kuriatnikov D, Liu Y, Su DM. Atrophied thymus, a tumor reservoir for harboring melanoma cells. Mol Cancer Res MCR (2018) 16(11):1652–64. doi: 10.1158/1541-7786.MCR-18-0308
121. Gilbert LA, Hemann MT. DNA Damage-mediated induction of a chemoresistant niche. Cell (2010) 143(3):355–66. doi: 10.1016/j.cell.2010.09.043
122. Bent EH, Gilbert LA, Hemann MT. A senescence secretory switch mediated by PI3K/AKT/mTOR activation controls chemoprotective endothelial secretory responses. Genes Dev (2016) 30(16):1811–21. doi: 10.1101/gad.284851.116
123. Nobrega C, Roque S, Nunes-Alves C, Coelho A, Medeiros I, Castro AG, et al. Dissemination of mycobacteria to the thymus renders newly generated T cells tolerant to the invading pathogen. J Immunol (2010) 184(1):351–8. doi: 10.4049/jimmunol.0902152
124. Nobrega C, Cardona PJ, Roque S, Pinto do OP, Appelberg R, Correia-Neves M. The thymus as a target for mycobacterial infections. Microbes Infect (2007) 9(14–15):1521–9. doi: 10.1016/j.micinf.2007.08.006
125. Korostoff JM, Nakada MT, Faas SJ, Blank KJ, Gaulton GN. Neonatal exposure to thymotropic gross murine leukemia virus induces virus-specific immunologic nonresponsiveness. J Exp Med (1990) 172(6):1765–75. doi: 10.1084/jem.172.6.1765
126. Jamieson BD, Ahmed R. T-Cell tolerance: exposure to virus in utero does not cause a permanent deletion of specific T cells. Proc Natl Acad Sci U.S.A. (1988) 85(7):2265–8. doi: 10.1073/pnas.85.7.2265
127. Milich DR, Jones JE, Hughes JL, Price J, Raney AK, McLachlan A. Is a function of the secreted hepatitis b e antigen to induce immunologic tolerance in utero? Proc Natl Acad Sci U.S.A. (1990) 87(17):6599–603. doi: 10.1073/pnas.87.17.6599
128. Thiede C, Steudel C, Mohr B, Schaich M, Schäkel U, Platzbecker U, et al. Analysis of FLT3-activating mutations in 979 patients with acute myelogenous leukemia: association with FAB subtypes and identification of subgroups with poor prognosis. Blood (2002) 99(12):4326–35. doi: 10.1182/blood.V99.12.4326
129. Sitnicka E, Buza-Vidas N, Ahlenius H, Cilio CM, Gekas C, Nygren JM, et al. Critical role of FLT3 ligand in IL-7 receptor–independent T lymphopoiesis and regulation of lymphoid-primed multipotent progenitors. Blood (2007) 110(8):2955–64. doi: 10.1182/blood-2006-10-054726
130. Jensen CT, Böiers C, Kharazi S, Lübking A, Rydén T, Sigvardsson M, et al. Permissive roles of hematopoietin and cytokine tyrosine kinase receptors in early T-cell development. Blood (2008) 111(4):2083–90. doi: 10.1182/blood-2007-08-108563
131. Williams KM, Moore AR, Lucas PJ, Wang J, Bare CV, Gress RE. FLT3 ligand regulates thymic precursor cells and hematopoietic stem cells through interactions with CXCR4 and the marrow niche. Exp Hematol (2017) 52:40–9. doi: 10.1016/j.exphem.2017.05.005
132. Gaballa A, Clave E, Uhlin M, Toubert A, Arruda LCM. Evaluating thymic function after human hematopoietic stem cell transplantation in the personalized medicine era. Front Immunol (2020) 11. doi: 10.3389/fimmu.2020.01341
133. Mackall CL, Bare CV, Granger LA, Sharrow SO, Titus JA, Gress RE. Thymic-independent T cell regeneration occurs via antigen-driven expansion of peripheral T cells resulting in a repertoire that is limited in diversity and prone to skewing. J Immunol (1996) 156(12):4609–16. doi: 10.4049/jimmunol.156.12.4609
134. Lin SJ, Peacock CD, Bahl K, Welsh RM. Programmed death-1 (PD-1) defines a transient and dysfunctional oligoclonal T cell population in acute homeostatic proliferation. J Exp Med (2007) 204(10):2321–33. doi: 10.1084/jem.20062150
135. Arruda LCM, Lima-Júnior JR, Clave E, Moraes DA, Douay C, Fournier I, et al. Homeostatic proliferation leads to telomere attrition and increased PD-1 expression after autologous hematopoietic SCT for systemic sclerosis. Bone marrow transplant. (2018) 53(10):1319–27. doi: 10.1038/s41409-018-0162-0
136. Roux E, Dumont-Girard F, Starobinski M, Siegrist CA, Helg C, Chapuis B, et al. Recovery of immune reactivity after T-cell-depleted bone marrow transplantation depends on thymic activity. Blood (2000) 96(6):2299–303. doi: 10.1182/blood.V96.6.2299
137. Yew PY, Alachkar H, Yamaguchi R, Kiyotani K, Fang H, Yap KL, et al. Quantitative characterization of T-cell repertoire in allogeneic hematopoietic stem cell transplant recipients. Bone Marrow Transplant (2015) 50(9):1227–34. doi: 10.1038/bmt.2015.133
138. Krenger W, Blazar BR, Holländer GA. Thymic T-cell development in allogeneic stem cell transplantation. Blood (2011) 117(25):6768–76. doi: 10.1182/blood-2011-02-334623
139. Ju JM, Jung MH, Nam G, Kim W, Oh S, Kim HD, et al. Escape from thymic deletion and anti-leukemic effects of T cells specific for hematopoietic cell-restricted antigen. Nat Commun (2018) 9(1):225. doi: 10.1038/s41467-017-02665-z
140. Velardi E, Clave E, Arruda LCM, Benini F, Locatelli F, Toubert A. The role of the thymus in allogeneic bone marrow transplantation and the recovery of the peripheral T-cell compartment. Semin Immunopathol (2021) 43(1):101–17. doi: 10.1007/s00281-020-00828-7
141. Beschorner WE, Hutchins GM, Elfenbein GJ, Santos GW. The thymus in patients with allogeneic bone marrow transplants. Am J Pathol (1978) 92(1):173–81.
142. Dertschnig S, Hauri-Hohl MM, Vollmer M, Holländer GA, Krenger W. Impaired thymic expression of tissue-restricted antigens licenses the de novo generation of autoreactive CD4+ T cells in acute GVHD. Blood (2015) 125(17):2720–3. doi: 10.1182/blood-2014-08-597245
143. Na IK, Lu SX, Yim NL, Goldberg GL, Tsai J, Rao U, et al. The cytolytic molecules fas ligand and TRAIL are required for murine thymic graft-versus-host disease. J Clin Invest (2010) 120(1):343–56. doi: 10.1172/JCI39395
144. Wu T, Young JS, Johnston H, Ni X, Deng R, Racine J, et al. Thymic damage, impaired negative selection, and development of chronic graft-versus-host disease caused by donor CD4+ and CD8+ T cells. J Immunol (2013) 191(1):488–99. doi: 10.4049/jimmunol.1300657
145. Hauri-Hohl MM, Keller MP, Gill J, Hafen K, Pachlatko E, Boulay T, et al. Donor T-cell alloreactivity against host thymic epithelium limits T-cell development after bone marrow transplantation. Blood (2007) 109(9):4080–8. doi: 10.1182/blood-2006-07-034157
146. Alawam AS, Cosway EJ, James KD, Lucas B, Bacon A, Parnell SM, et al. Failures in thymus medulla regeneration during immune recovery cause tolerance loss and prime recipients for auto-GVHD. J Exp Med (2022) 219(2):e20211239. doi: 10.1084/jem.20211239
147. Krenger W, Rossi S, Holländer GA. Apoptosis of thymocytes during acute graft-versus-host disease is independent of glucocorticoids. Transplantation (2000) 69(10):2190–3. doi: 10.1097/00007890-200005270-00040
148. Hazenberg MD, Otto SA, de Pauw ES, Roelofs H, Fibbe WE, Hamann D, et al. T-Cell receptor excision circle and T-cell dynamics after allogeneic stem cell transplantation are related to clinical events. Blood (2002) 99(9):3449–53. doi: 10.1182/blood.V99.9.3449
149. Storek J, Joseph A, Dawson MA, Douek DC, Storer B, Maloney DG. Factors influencing T-lymphopoiesis after allogeneic hematopoietic cell transplantation. Transplantation (2002) 73(7):1154–8. doi: 10.1097/00007890-200204150-00026
150. Clave E, Busson M, Douay C, Peffault de Latour R, Berrou J, Rabian C, et al. Acute graft-versus-host disease transiently impairs thymic output in young patients after allogeneic hematopoietic stem cell transplantation. Blood (2009) 113(25):6477–84. doi: 10.1182/blood-2008-09-176594
151. Saxena M, van der Burg SH, Melief CJM, Bhardwaj N. Therapeutic cancer vaccines. Nat Rev Cancer (2021) 21(6):360–78. doi: 10.1038/s41568-021-00346-0
152. Thompson WW, Shay DK, Weintraub E, Brammer L, Cox N, Anderson LJ, et al. Mortality associated with influenza and respiratory syncytial virus in the united states. JAMA (2003) 289(2):179–86. doi: 10.1001/jama.289.2.179
153. McGranahan N, Furness AJ, Rosenthal R, Ramskov S, Lyngaa R, Saini SK, et al. Clonal neoantigens elicit T cell immunoreactivity and sensitivity to immune checkpoint blockade. Science (2016) 351(6280):1463–9. doi: 10.1126/science.aaf1490
154. Schumacher TN, Kesmir C, van Buuren MM. Biomarkers in cancer immunotherapy. Cancer Cell (2015) 27(1):12–4. doi: 10.1016/j.ccell.2014.12.004
155. Valpione S, Mundra PA, Galvani E, Campana LG, Lorigan P, De Rosa F, et al. The T cell receptor repertoire of tumor infiltrating T cells is predictive and prognostic for cancer survival. Nat Commun (2021) 12(1):4098. doi: 10.1038/s41467-021-24343-x
156. Davids MS, Kim HT, Bachireddy P, Costello C, Liguori R, Savell A, et al. Ipilimumab for patients with relapse after allogeneic transplantation. N Engl J Med (2016) 375(2):143–53. doi: 10.1056/NEJMoa1601202
157. Daver N, Garcia-Manero G, Basu S, Boddu PC, Alfayez M, Cortes JE, et al. Efficacy, safety, and biomarkers of response to azacitidine and nivolumab in Relapsed/Refractory acute myeloid leukemia: A nonrandomized, open-label, phase II study. Cancer Discovery (2019) 9(3):370–83. doi: 10.1158/2159-8290.CD-18-0774
158. Stahl M, Goldberg AD. Immune checkpoint inhibitors in acute myeloid leukemia: Novel combinations and therapeutic targets. Curr Oncol Rep (2019) 21(4):37. doi: 10.1007/s11912-019-0781-7
159. Kidman J, Principe N, Watson M, Lassmann T, Holt RA, Nowak AK, et al. Characteristics of TCR repertoire associated with successful immune checkpoint therapy responses. Front Immunol (2020) 11. doi: 10.3389/fimmu.2020.587014
160. Postow MA, Manuel M, Wong P, Yuan J, Dong Z, Liu C, et al. Peripheral T cell receptor diversity is associated with clinical outcomes following ipilimumab treatment in metastatic melanoma. J Immunother Cancer (2015) 3:23. doi: 10.1186/s40425-015-0070-4
161. Arakawa A, Vollmer S, Tietze J, Galinski A, Heppt MV, Bürdek M, et al. Clonality of CD4+ blood T cells predicts longer survival with CTLA4 or PD-1 checkpoint inhibition in advanced melanoma. Front Immunol (2019) 10:1336. doi: 10.3389/fimmu.2019.01336
162. Hogan SA, Courtier A, Cheng PF, Jaberg-Bentele NF, Goldinger SM, Manuel M, et al. Peripheral blood TCR repertoire profiling may facilitate patient stratification for immunotherapy against melanoma. Cancer Immunol Res (2019) 7(1):77–85. doi: 10.1158/2326-6066.CIR-18-0136
163. Ji S, Chang L, Chang L, Zhao C, Jia R, Tan Z, et al. Peripheral blood T-cell receptor repertoire as a predictor of clinical outcomes in gastrointestinal cancer patients treated with PD-1 inhibitor. Clin Transl Oncol (2021) 23(8):1646–56. doi: 10.1007/s12094-021-02562-4
164. Cader FZ, Hu X, Goh WL, Wienand K, Ouyang J, Mandato E, et al. A peripheral immune signature of responsiveness to PD-1 blockade in patients with classical Hodgkin lymphoma. Nat Med (2020) 26(9):1468–79. doi: 10.1038/s41591-020-1006-1
165. Abbas HA, Hao D, Tomczak K, Barrodia P, Im JS, Reville PK, et al. Single cell T cell landscape and T cell receptor repertoire profiling of AML in context of PD-1 blockade therapy. Nat Commun (2021) 12(1):6071. doi: 10.1038/s41467-021-26282-z
166. Qu C, Zhang H, Cao H, Tang L, Mo H, Liu F, et al. Tumor buster - where will the CAR-T cell therapy 'missile' go? Mol Cancer (2022) 21(1):201. doi: 10.1186/s12943-022-01669-8
167. Mardiana S, Gill S. CAR T cells for acute myeloid leukemia: State of the art and future directions. Front Oncol (2020) 10:697. doi: 10.3389/fonc.2020.00697
168. Surh CD, Ernst B, Sprent J. Growth of epithelial cells in the thymic medulla is under the control of mature T cells. J Exp Med (1992) 176(2):611–6. doi: 10.1084/jem.176.2.611
169. Hale JS, Fink PJ. Back to the thymus: Peripheral T cells come home. Immunol Cell Biol (2009) 87(1):58–64. doi: 10.1038/icb.2008.87
170. Yin C, Pei XY, Shen H, Gao YN, Sun XY, Wang W, et al. Thymic homing of activated CD4(+) T cells induces degeneration of the thymic epithelium through excessive RANK signaling. Sci Rep (2017) 7(1):2421. doi: 10.1038/s41598-017-02653-9
171. Edelmann SL, Marconi P, Brocker T. Peripheral T cells re-enter the thymus and interfere with central tolerance induction. J Immunol (2011) 186(10):5612–9. doi: 10.4049/jimmunol.1004010
172. van den Brink MRM, Alpdogan O, Boyd RL. Strategies to enhance T-cell reconstitution in immunocompromised patients. Nat Rev Immunol (2004) 4(11):856–67. doi: 10.1038/nri1484
173. Dudakov JA, Hanash AM, Jenq RR, Young LF, Ghosh A, Singer NV, et al. Interleukin-22 drives endogenous thymic regeneration in mice. Science (2012) 336(6077):91–5. doi: 10.1126/science.1218004
174. Dudakov JA, Mertelsmann AM, O’Connor MH, Jenq RR, Velardi E, Young LF, et al. Loss of thymic innate lymphoid cells leads to impaired thymopoiesis in experimental graft-versus-host disease. Blood (2017) 130(7):933–42. doi: 10.1182/blood-2017-01-762658
175. Pan B, Zhang F, Lu Z, Li L, Shang L, Xia F, et al. Donor T-cell-derived interleukin-22 promotes thymus regeneration and alleviates chronic graft-versus-host disease in murine allogeneic hematopoietic cell transplant. Int Immunopharmacol (2019) 67:194–201. doi: 10.1016/j.intimp.2018.12.023
176. Rossi SW, Jeker LT, Ueno T, Kuse S, Keller MP, Zuklys S, et al. Keratinocyte growth factor (KGF) enhances postnatal T-cell development via enhancements in proliferation and function of thymic epithelial cells. Blood (2007) 109(9):3803–11. doi: 10.1182/blood-2006-10-049767
177. Erickson M, Morkowski S, Lehar S, Gillard G, Beers C, Dooley J, et al. Regulation of thymic epithelium by keratinocyte growth factor. Blood (2002) 100(9):3269–78. doi: 10.1182/blood-2002-04-1036
178. Rossi S, Blazar BR, Farrell CL, Danilenko DM, Lacey DL, Weinberg KI, et al. Keratinocyte growth factor preserves normal thymopoiesis and thymic microenvironment during experimental graft-versus-host disease. Blood (2002) 100(2):682–91. doi: 10.1182/blood.V100.2.682
179. Alpdogan Ö, Hubbard VM, Smith OM, Patel N, Lu S, Goldberg GL, et al. Keratinocyte growth factor (KGF) is required for postnatal thymic regeneration. Blood (2006) 107(6):2453. doi: 10.1182/blood-2005-07-2831
180. Min D, Taylor PA, Panoskaltsis-Mortari A, Chung B, Danilenko DM, Farrell C, et al. Protection from thymic epithelial cell injury by keratinocyte growth factor: a new approach to improve thymic and peripheral T-cell reconstitution after bone marrow transplantation. Blood (2002) 99(12):4592–600. doi: 10.1182/blood.V99.12.4592
181. Wang Y, Chen G, Qiao S, Ma X, Tang X, Sun A, et al. Keratinocyte growth factor enhanced immune reconstitution in murine allogeneic umbilical cord blood cell transplant. Leuk Lymphoma (2011) 52(8):1556–66. doi: 10.3109/10428194.2011.573037
182. Wils EJ, Aerts-Kaya FS, Rombouts EJC, van Mourik I, Rijken-Schelen A, Visser TP, et al. Keratinocyte growth factor and stem cell factor to improve thymopoiesis after autologous CD34+ cell transplantation in rhesus macaques. Biol Blood Marrow Transplant J Am Soc Blood Marrow Transplant (2012) 18(1):55–65. doi: 10.1016/j.bbmt.2011.09.010
183. Coles AJ, Azzopardi L, Kousin-Ezewu O, Mullay HK, Thompson SAJ, Jarvis L, et al. Keratinocyte growth factor impairs human thymic recovery from lymphopenia. JCI Insight (2019) 4(12). doi: 10.1172/jci.insight.125377
184. Patchen ML, Fischer R, Schmauder-Chock EA, Williams DE. Mast cell growth factor enhances multilineage hematopoietic recovery in vivo following radiation-induced aplasia. Exp Hematol (1994) 22(1):31–9.
185. Chung B, Min D, Joo LW, Krampf MR, Huang J, Yang Y, et al. Combined effects of interleukin-7 and stem cell factor administration on lymphopoiesis after murine bone marrow transplantation. Biol Blood Marrow Transplant J Am Soc Blood Marrow Transplant (2011) 17(1):48–60. doi: 10.1016/j.bbmt.2010.07.027
186. Wils EJ, Rombouts EJ, van Mourik I, Spits H, Legrand N, Braakman E, et al. Stem cell factor consistently improves thymopoiesis after experimental transplantation of murine or human hematopoietic stem cells in immunodeficient mice. J Immunol (2011) 187(6):2974–81. doi: 10.4049/jimmunol.1004209
187. Ding JH, Wang LL, Chen Z, Wang J, Yu ZP, Zhao G, et al. The role of Tα1 on the infective patients after hematopoietic stem cell transplantation. Int J Hematol (2013) 97(2):280–3. doi: 10.1007/s12185-012-1208-5
188. Sirohi B, Powles R, Morgan G, Treleaven J, Kulkarni S, Horton C, et al. Use of physiological doses of human growth hormone in haematological patients receiving intensive chemotherapy promotes haematopoietic recovery: a double-blind randomized, placebo-controlled study. Bone Marrow Transplant (2007) 39(2):115–20. doi: 10.1038/sj.bmt.1705545
189. Lopes N, Vachon H, Marie J, Irla M. Administration of RANKL boosts thymic regeneration upon bone marrow transplantation. EMBO Mol Med (2017) 9(6):835–51. doi: 10.15252/emmm.201607176
190. Montero-Herradón S, García-Ceca J, Zapata AG. Altered maturation of medullary TEC in EphB-deficient thymi is recovered by RANK signaling stimulation. Front Immunol (2018) 9. doi: 10.3389/fimmu.2018.01020
191. Akiyama T, Shimo Y, Yanai H, Qin J, Ohshima D, Maruyama Y, et al. The tumor necrosis factor family receptors RANK and CD40 cooperatively establish the thymic medullary microenvironment and self-tolerance. Immunity (2008) 29(3):423–37. doi: 10.1016/j.immuni.2008.06.015
192. Bredenkamp N, Ulyanchenko S, O’Neill KE, Manley NR, Vaidya HJ, Blackburn CC. An organized and functional thymus generated from FOXN1-reprogrammed fibroblasts. Nat Cell Biol (2014) 16(9):902–8. doi: 10.1038/ncb3023
193. Oh J, Wang W, Thomas R, Su DM. Thymic rejuvenation via FOXN1-reprogrammed embryonic fibroblasts (FREFs) to counteract age-related inflammation. JCI Insight (2020) 5(18):e140313. doi: 10.1172/jci.insight.140313
194. Chen BJ, Cui X, Sempowski GD, Chao NJ. Growth hormone accelerates immune recovery following allogeneic T-cell-depleted bone marrow transplantation in mice. Exp Hematol (2003) 31(10):953–8. doi: 10.1016/S0301-472X(03)00196-6
195. Napolitano LA, Schmidt D, Gotway MB, Ameli N, Filbert EL, Ng MM, et al. Growth hormone enhances thymic function in HIV-1–infected adults. J Clin Invest (2008) 118(3):1085–98. doi: 10.1172/JCI32830
196. Napolitano LA, Lo JC, Gotway MB, Mulligan K, Barbour JD, Schmidt D, et al. Increased thymic mass and circulating naive CD4 T cells in HIV-1-infected adults treated with growth hormone. AIDS Lond Engl (2002) 16(8):1103–11. doi: 10.1097/00002030-200205240-00003
197. Fahy GM, Brooke RT, Watson JP, Good Z, Vasanawala SS, Maecker H, et al. Reversal of epigenetic aging and immunosenescent trends in humans. Aging Cell (2019) 18(6):e13028. doi: 10.1111/acel.13028
198. Carlo-Stella C, Di Nicola M, Milani R, Longoni P, Milanesi M, Bifulco C, et al. Age- and irradiation-associated loss of bone marrow hematopoietic function in mice is reversed by recombinant human growth hormone. Exp Hematol (2004) 32(2):171–8. doi: 10.1016/j.exphem.2003.11.007
199. Dixit VD, Yang H, Sun Y, Weeraratna AT, Youm YH, Smith RG, et al. Ghrelin promotes thymopoiesis during aging. J Clin Invest (2007) 117(10):2778–90. doi: 10.1172/JCI30248
200. Wertheimer T, Velardi E, Tsai J, Cooper K, Xiao S, Kloss CC, et al. Production of BMP4 by endothelial cells is crucial for endogenous thymic regeneration. Sci Immunol (2018) 3(19):eaal2736. doi: 10.1126/sciimmunol.aal2736
201. Swann JB, Krauth B, Happe C, Boehm T. Cooperative interaction of BMP signalling and Foxn1 gene dosage determines the size of the functionally active thymic epithelial compartment. Sci Rep (2017) 7(1):8492. doi: 10.1038/s41598-017-09213-1
202. Tsai PT, Lee RA, Wu H. BMP4 acts upstream of FGF in modulating thymic stroma and regulating thymopoiesis. Blood (2003) 102(12):3947–53. doi: 10.1182/blood-2003-05-1657
203. Bleul CC, Boehm T. BMP signaling is required for normal thymus development. J Immunol (2005) 175(8):5213–21. doi: 10.4049/jimmunol.175.8.5213
204. Dallas MH, Varnum-Finney B, Martin PJ, Bernstein ID. Enhanced T-cell reconstitution by hematopoietic progenitors expanded ex vivo using the notch ligand Delta1. Blood (2007) 109(8):3579–87. doi: 10.1182/blood-2006-08-039842
205. Sottoriva K, Paik NY, White Z, Bandara T, Shao L, Sano T, et al. A Notch/IL-21 signaling axis primes bone marrow T cell progenitor expansion. JCI Insight (2022) 7(9). doi: 10.1172/jci.insight.157015
206. Alpdogan O, Muriglan SJ, Eng JM, Willis LM, Greenberg AS, Kappel BJ, et al. IL-7 enhances peripheral T cell reconstitution after allogeneic hematopoietic stem cell transplantation. J Clin Invest (2003) 112(7):1095–107. doi: 10.1172/JCI200317865
207. Alpdogan O, Schmaltz C, Muriglan SJ, Kappel BJ, Perales MA, Rotolo JA, et al. Administration of interleukin-7 after allogeneic bone marrow transplantation improves immune reconstitution without aggravating graft-versus-host disease. Blood (2001) 98(7):2256–65. doi: 10.1182/blood.V98.7.2256
208. Lévy Y, Sereti I, Tambussi G, Routy JP, Lelièvre JD, Delfraissy JF, et al. Effects of recombinant human interleukin 7 on T-cell recovery and thymic output in HIV-infected patients receiving antiretroviral therapy: results of a phase I/IIa randomized, placebo-controlled, multicenter study. Clin Infect Dis Off Publ Infect Dis Soc Am (2012) 55(2):291–300. doi: 10.1093/cid/cis383
209. Sportès C, Hakim FT, Memon SA, Zhang H, Chua KS, Brown MR, et al. Administration of rhIL-7 in humans increases in vivo TCR repertoire diversity by preferential expansion of naive T cell subsets. J Exp Med (2008) 205(7):1701–14. doi: 10.1084/jem.20071681
210. Rosenberg SA, Sportès C, Ahmadzadeh M, Fry TJ, Ngo LT, Schwarz SL, et al. IL-7 administration to humans leads to expansion of CD8+ and CD4+ cells but a relative decrease of CD4+ T-regulatory cells. J Immunother (2006) 29(3):313–9. doi: 10.1097/01.cji.0000210386.55951.c2
211. Perales MA, Goldberg JD, Yuan J, Koehne G, Lechner L, Papadopoulos EB, et al. Recombinant human interleukin-7 (CYT107) promotes T-cell recovery after allogeneic stem cell transplantation. Blood (2012) 120(24):4882–91. doi: 10.1182/blood-2012-06-437236
212. Abdul-Hai A, Or R, Slavin S, Friedman G, Weiss L, Matsa D, et al. Stimulation of immune reconstitution by interleukin-7 after syngeneic bone marrow transplantation in mice. Exp Hematol (1996) 24(12):1416–22.
213. Trédan O, Ménétrier-Caux C, Ray-Coquard I, Garin G, Cropet C, Verronèse E, et al. ELYPSE-7: a randomized placebo-controlled phase IIa trial with CYT107 exploring the restoration of CD4+ lymphocyte count in lymphopenic metastatic breast cancer patients. Ann Oncol Off J Eur Soc Med Oncol (2015) 26(7):1353–62. doi: 10.1093/annonc/mdv173
214. Sheikh V, Porter BO, DerSimonian R, Kovacs SB, Thompson WL, Perez-Diez A, et al. Administration of interleukin-7 increases CD4 T cells in idiopathic CD4 lymphocytopenia. Blood (2016) 127(8):977–88. doi: 10.1182/blood-2015-05-645077
215. Mackall CL, Fry TJ, Bare C, Morgan P, Galbraith A, Gress RE. IL-7 increases both thymic-dependent and thymic-independent T-cell regeneration after bone marrow transplantation. Blood (2001) 97(5):1491–7. doi: 10.1182/blood.V97.5.1491
216. Fry TJ, Moniuszko M, Creekmore S, Donohue SJ, Douek DC, Giardina S, et al. IL-7 therapy dramatically alters peripheral T-cell homeostasis in normal and SIV-infected nonhuman primates. Blood (2003) 101(6):2294–9. doi: 10.1182/blood-2002-07-2297
217. Li L, Hsu HC, Stockard CR, Yang P, Zhou J, Wu Q, et al. IL-12 inhibits thymic involution by enhancing IL-7- and IL-2-Induced thymocyte proliferation. J Immunol (2004) 172(5):2909–16. doi: 10.4049/jimmunol.172.5.2909
218. Chen T, Burke KA, Zhan Y, Wang X, Shibata D, Zhao Y. IL-12 facilitates both the recovery of endogenous hematopoiesis and the engraftment of stem cells after ionizing radiation. Exp Hematol (2007) 35(2):203–13. doi: 10.1016/j.exphem.2006.10.002
219. Al-Chami E, Tormo A, Pasquin S, Kanjarawi R, Ziouani S, Rafei M. Interleukin-21 administration to aged mice rejuvenates their peripheral T-cell pool by triggering de novo thymopoiesis. Aging Cell (2016) 15(2):349–60. doi: 10.1111/acel.12440
220. Tormo A, Khodayarian F, Cui Y, Al-Chami E, Kanjarawi R, Noé B, et al. Interleukin-21 promotes thymopoiesis recovery following hematopoietic stem cell transplantation. J Hematol Oncol (2017) 10(1):120. doi: 10.1186/s13045-017-0490-3
221. Rafei M, Dumont-Lagacé M, Rouette A, Perreault C. Interleukin-21 accelerates thymic recovery from glucocorticoïd-induced atrophy. PloS One (2013) 8(9):e72801. doi: 10.1371/journal.pone.0072801
222. Perruccio K, Bonifazi P, Topini F, Tosti A, Bozza S, Aloisi T, et al. Thymosin alpha1 to harness immunity to pathogens after haploidentical hematopoietic transplantation. Ann N Y Acad Sci (2010) 1194:153–61. doi: 10.1111/j.1749-6632.2010.05486.x
223. Velardi E, Tsai JJ, Holland AM, Wertheimer T, Yu VWC, Zakrzewski JL, et al. Sex steroid blockade enhances thymopoiesis by modulating notch signaling. J Exp Med (2014) 211(12):2341–9. doi: 10.1084/jem.20131289
224. Williams KM, Lucas PJ, Bare CV, Wang J, Chu YW, Tayler E, et al. CCL25 increases thymopoiesis after androgen withdrawal. Blood (2008) 112(8):3255–63. doi: 10.1182/blood-2008-04-153627
225. Sutherland JS, Spyroglou L, Muirhead JL, Heng TS, Prieto-Hinojosa A, Prince HM, et al. Enhanced immune system regeneration in humans following allogeneic or autologous hemopoietic stem cell transplantation by temporary sex steroid blockade. Clin Cancer Res Off J Am Assoc Cancer Res (2008) 14(4):1138–49. doi: 10.1158/1078-0432.CCR-07-1784
226. Fletcher AL, Lowen TE, Sakkal S, Reiseger JJ, Hammett MV, Seach N, et al. Ablation and regeneration of tolerance-inducing medullary thymic epithelial cells after cyclosporine, cyclophosphamide, and dexamethasone treatment. J Immunol (2009) 183(2):823–31. doi: 10.4049/jimmunol.0900225
227. Khong DM, Dudakov JA, Hammett MV, Jurblum MI, Khong SML, Goldberg GL, et al. Enhanced hematopoietic stem cell function mediates immune regeneration following sex steroid blockade. Stem Cell Rep (2015) 4(3):445–58. doi: 10.1016/j.stemcr.2015.01.018
228. Dudakov JA, Goldberg GL, Reiseger JJ, Vlahos K, Chidgey AP, Boyd RL. Sex steroid ablation enhances hematopoietic recovery following cytotoxic antineoplastic therapy in aged mice. J Immunol (2009) 183(11):7084–94. doi: 10.4049/jimmunol.0900196
229. Dudakov JA, Goldberg GL, Reiseger JJ, Chidgey AP, Boyd RL. Withdrawal of sex steroids reverses age- and chemotherapy-related defects in bone marrow lymphopoiesis. J Immunol (2009) 182(10):6247–60. doi: 10.4049/jimmunol.0802446
230. Goldberg GL, King CG, Nejat RA, Suh DY, Smith OM, Bretz JC, et al. Luteinizing hormone-releasing hormone enhances T cell recovery following allogeneic bone marrow transplantation. J Immunol (2009) 182(9):5846–54. doi: 10.4049/jimmunol.0801458
231. Sutherland JS, Goldberg GL, Hammett MV, Uldrich AP, Berzins SP, Heng TS, et al. Activation of thymic regeneration in mice and humans following androgen blockade. J Immunol (2005) 175(4):2741–53. doi: 10.4049/jimmunol.175.4.2741
232. Lai KP, Lai JJ, Chang P, Altuwaijri S, Hsu JW, Chuang KH, et al. Targeting thymic epithelia AR enhances T-cell reconstitution and bone marrow transplant grafting efficacy. Mol Endocrinol (2013) 27(1):25–37. doi: 10.1210/me.2012-1244
233. Heng TSP, Reiseger JJ, Fletcher AL, Leggatt GR, White OJ, Vlahos K, et al. Impact of sex steroid ablation on viral, tumour and vaccine responses in aged mice. PloS One (2012) 7(8):e42677. doi: 10.1371/journal.pone.0042677
234. Goldberg GL, Alpdogan O, Muriglan SJ, Hammett MV, Milton MK, Eng JM, et al. Enhanced immune reconstitution by sex steroid ablation following allogeneic hemopoietic stem cell transplantation. J Immunol (2007) 178(11):7473–84. doi: 10.4049/jimmunol.178.11.7473
235. Velardi E, Tsai JJ, Radtke S, Cooper K, Argyropoulos KV, Jae-Hung S, et al. Suppression of luteinizing hormone enhances HSC recovery after hematopoietic injury. Nat Med (2018) 24(2):239–46. doi: 10.1038/nm.4470
236. Obukhova LA, Skulachev VP, Kolosova NG. Mitochondria-targeted antioxidant SkQ1 inhibits age-dependent involution of the thymus in normal and senescence-prone rats. Aging (2009) 1(4):389–401. doi: 10.18632/aging.100043
237. Tajima A, Pradhan I, Trucco M, Fan Y. Restoration of thymus function with bioengineered thymus organoids. Curr Stem Cell Rep (2016) 2(2):128–39. doi: 10.1007/s40778-016-0040-x
238. Fan Y, Tajima A, Goh SK, Geng X, Gualtierotti G, Grupillo M, et al. Bioengineering thymus organoids to restore thymic function and induce donor-specific immune tolerance to allografts. Mol Ther J Am Soc Gene Ther (2015) 23(7):1262–77. doi: 10.1038/mt.2015.77
239. Bortolomai I, Sandri M, Draghici E, Fontana E, Campodoni E, Marcovecchio GE, et al. Gene modification and three-dimensional scaffolds as novel tools to allow the use of postnatal thymic epithelial cells for thymus regeneration approaches. Stem Cells Transl Med (2019) 8(10):1107–22. doi: 10.1002/sctm.18-0218
240. Chung B, Montel-Hagen A, Ge S, Blumberg G, Kim K, Klein S, et al. Engineering the human thymic microenvironment to support thymopoiesis in vivo. Stem Cells (2014) 32(9):2386–96. doi: 10.1002/stem.1731
241. Kim MJ, Miller CM, Shadrach JL, Wagers AJ, Serwold T. Young, proliferative thymic epithelial cells engraft and function in aging thymuses. J Immunol (2015) 194(10):4784–95. doi: 10.4049/jimmunol.1403158
242. Lai L, Cui C, Jin J, Hao Z, Zheng Q, Ying M, et al. Mouse embryonic stem cell-derived thymic epithelial cell progenitors enhance T-cell reconstitution after allogeneic bone marrow transplantation. Blood (2011) 118(12):3410–8. doi: 10.1182/blood-2011-03-340794
243. Gill J, Malin M, Holländer GA, Boyd R. Generation of a complete thymic microenvironment by MTS24+ thymic epithelial cells. Nat Immunol (2002) 3(7):635–42. doi: 10.1038/ni812
244. Bennett AR, Farley A, Blair NF, Gordon J, Sharp L, Blackburn CC. Identification and characterization of thymic epithelial progenitor cells. Immunity (2002) 16(6):803–14. doi: 10.1016/S1074-7613(02)00321-7
245. Depreter MGL, Blair NF, Gaskell TL, Nowell CS, Davern K, Pagliocca A, et al. Identification of plet-1 as a specific marker of early thymic epithelial progenitor cells. Proc Natl Acad Sci (2008) 105(3):961–6. doi: 10.1073/pnas.0711170105
246. Rossi SW, Chidgey AP, Parnell SM, Jenkinson WE, Scott HS, Boyd RL, et al. Redefining epithelial progenitor potential in the developing thymus. Eur J Immunol (2007) 37(9):2411–8. doi: 10.1002/eji.200737275
247. Zakrzewski JL, Suh D, Markley JC, Smith OM, King C, Goldberg GL, et al. Tumor immunotherapy across MHC barriers using allogeneic T-cell precursors. Nat Biotechnol (2008) 26(4):453–61. doi: 10.1038/nbt1395
248. Zakrzewski JL, Kochman AA, Lu SX, Terwey TH, Kim TD, Hubbard VM, et al. Adoptive transfer of T-cell precursors enhances T-cell reconstitution after allogeneic hematopoietic stem cell transplantation. Nat Med (2006) 12(9):1039–47. doi: 10.1038/nm1463
249. Arber C, BitMansour A, Sparer TE, Higgins JP, Mocarski ES, Weissman IL, et al. Common lymphoid progenitors rapidly engraft and protect against lethal murine cytomegalovirus infection after hematopoietic stem cell transplantation. Blood (2003) 102(2):421–8. doi: 10.1182/blood-2002-12-3834
250. Seet CS, He C, Bethune MT, Li S, Chick B, Gschweng EH, et al. Generation of mature T cells from human hematopoietic stem and progenitor cells in artificial thymic organoids. Nat Methods (2017) 14(5):521–30. doi: 10.1038/nmeth.4237
251. Schmitt TM, Zúñiga-Pflücker JC. Induction of T cell development from hematopoietic progenitor cells by delta-like-1 in vitro. Immunity (2002) 17(6):749–56. doi: 10.1016/S1074-7613(02)00474-0
252. Shukla S, Langley MA, Singh J, Edgar JM, Mohtashami M, Zúñiga-Pflücker JC, et al. Progenitor T-cell differentiation from hematopoietic stem cells using delta-like-4 and VCAM-1. Nat Methods (2017) 14(5):531–8. doi: 10.1038/nmeth.4258
253. Holland AM, Zakrzewski JL, Goldberg GL, Ghosh A, van den Brink MRM. Adoptive precursor cell therapy to enhance immune reconstitution after hematopoietic stem cell transplantation in mouse and man. Semin Immunopathol (2008) 30(4):479–87. doi: 10.1007/s00281-008-0138-z
254. Awong G, Singh J, Mohtashami M, Malm M, La Motte-Mohs RN, Benveniste PM, et al. Human proT-cells generated in vitro facilitate hematopoietic stem cell-derived T-lymphopoiesis in vivo and restore thymic architecture. Blood (2013) 122(26):4210–9. doi: 10.1182/blood-2012-12-472803
255. Smith MJ, Reichenbach DK, Parker SL, Riddle MJ, Mitchell J, Osum KC, et al. T Cell progenitor therapy–facilitated thymopoiesis depends upon thymic input and continued thymic microenvironment interaction. JCI Insight (2017) 2(10). doi: 10.1172/jci.insight.92056
256. Reimann C, Dal Cortivo L, Hacein-Bey-Abina S, Fischer A, André-Schmutz I, Cavazzana-Calvo M. Advances in adoptive immunotherapy to accelerate T-cellular immune reconstitution after HLA-incompatible hematopoietic stem cell transplantation. Immunotherapy (2010) 2(4):481–96. doi: 10.2217/imt.10.36
257. Zook EC, Krishack PA, Zhang S, Zeleznik-Le NJ, Firulli AB, Witte PL, et al. Overexpression of Foxn1 attenuates age-associated thymic involution and prevents the expansion of peripheral CD4 memory T cells. Blood (2011) 118(22):5723–31. doi: 10.1182/blood-2011-03-342097
258. Ortman CL, Dittmar KA, Witte PL, Le PT. Molecular characterization of the mouse involuted thymus: aberrations in expression of transcription regulators in thymocyte and epithelial compartments. Int Immunol (2002) 14(7):813–22. doi: 10.1093/intimm/dxf042
259. Rode I, Martins VC, Küblbeck G, Maltry N, Tessmer C, Rodewald HR. Foxn1 protein expression in the developing, aging, and regenerating thymus. J Immunol (2015) 195(12):5678–87. doi: 10.4049/jimmunol.1502010
260. Žuklys S, Handel A, Zhanybekova S, Govani F, Keller M, Maio S, et al. Foxn1 regulates key target genes essential for T cell development in postnatal thymic epithelial cells. Nat Immunol (2016) 17(10):1206–15. doi: 10.1038/ni.3537
261. Chen L, Xiao S, Manley NR. Foxn1 is required to maintain the postnatal thymic microenvironment in a dosage-sensitive manner. Blood (2009) 113(3):567–74. doi: 10.1182/blood-2008-05-156265
262. Mackall CL, Fry TJ, Gress RE. Harnessing the biology of IL-7 for therapeutic application. Nat Rev Immunol (2011) 11(5):330–42. doi: 10.1038/nri2970
263. Hong C, Luckey MA, Park JH. Intrathymic IL-7: The where, when, and why of IL-7 signaling during T cell development. Semin Immunol (2012) 24(3):151–8. doi: 10.1016/j.smim.2012.02.002
264. Li ZH, Liu Y, Gao SY. Correlation between IL-7 genomic protein methylation level and acute myeloid leukemia. Eur Rev Med Pharmacol Sci (2019) 23(3):1196–202. doi: 10.26355/eurrev_201902_17012
265. Wendelbo Ø, Glenjen N, Bruserud Ø. Interleukin-7 (IL-7) in patients receiving intensive chemotherapy for acute myelogenous leukemia: studies of systemic IL-7 levels and IL-7 responsiveness of circulating T lymphocytes. J Interferon Cytokine Res Off J Int Soc Interferon Cytokine Res (2002) 22(10):1057–65. doi: 10.1089/107999002760624297
266. Andrew D, Aspinall R. Age-associated thymic atrophy is linked to a decline in IL-7 production. Exp Gerontol (2002) 37(2–3):455–63. doi: 10.1016/S0531-5565(01)00213-3
267. Kelly RM, Highfill SL, Panoskaltsis-Mortari A, Taylor PA, Boyd RL, Holländer GA, et al. Keratinocyte growth factor and androgen blockade work in concert to protect against conditioning regimen-induced thymic epithelial damage and enhance T-cell reconstitution after murine bone marrow transplantation. Blood (2008) 111(12):5734–44. doi: 10.1182/blood-2008-01-136531
268. Kelly RM, Goren EM, Taylor PA, Mueller SN, Stefanski HE, Osborn MJ, et al. Short-term inhibition of p53 combined with keratinocyte growth factor improves thymic epithelial cell recovery and enhances T-cell reconstitution after murine bone marrow transplantation. Blood (2010) 115(5):1088–97. doi: 10.1182/blood-2009-05-223198
269. Min D, Panoskaltsis-Mortari A, Kuro-O M, Holländer GA, Blazar BR, Weinberg KI. Sustained thymopoiesis and improvement in functional immunity induced by exogenous KGF administration in murine models of aging. Blood (2007) 109(6):2529–37. doi: 10.1182/blood-2006-08-043794
270. Spielberger R, Stiff P, Bensinger W, Gentile T, Weisdorf D, Kewalramani T, et al. Palifermin for oral mucositis after intensive therapy for hematologic cancers. N Engl J Med (2004) 351(25):2590–8. doi: 10.1056/NEJMoa040125
271. Öner H, Ozan E. Effects of gonadal hormones on thymus gland after bilateral ovariectomy and orchidectomy in rats. Arch Androl (2002) 48(2):115–26. doi: 10.1080/014850102317267427
272. Windmill KF, Meade BJ, Lee VW. Effect of prepubertal gonadectomy and sex steroid treatment on the growth and lymphocyte populations of the rat thymus. Reprod Fertil Dev (1993) 5(1):73–81. doi: 10.1071/RD9930073
273. Utsuyama M, Hirokawa K. Hypertrophy of the thymus and restoration of immune functions in mice and rats by gonadectomy. Mech Ageing Dev (1989) 47(3):175–85. doi: 10.1016/0047-6374(89)90030-4
274. Velardi E, Dudakov JA, van den Brink MRM. Sex steroid ablation: an immunoregenerative strategy for immunocompromised patients. Bone Marrow Transplant (2015) 50:S77–81. doi: 10.1038/bmt.2015.101
275. Goldberg GL, Sutherland JS, Hammet MV, Milton MK, Heng TSP, Chidgey AP, et al. Sex steroid ablation enhances lymphoid recovery following autologous hematopoietic stem cell transplantation. Transplantation (2005) 80(11):1604–13. doi: 10.1097/01.tp.0000183962.64777.da
276. Hattori N. Expression, regulation and biological actions of growth hormone (GH) and ghrelin in the immune system. Growth Horm IGF Res (2009) 19(3):187–97. doi: 10.1016/j.ghir.2008.12.001
277. Taub DD, Murphy WJ, Longo DL. Rejuvenation of the aging thymus: growth hormone-mediated and ghrelin-mediated signaling pathways. Curr Opin Pharmacol (2010) 10(4):408–24. doi: 10.1016/j.coph.2010.04.015
278. Hansen BR, Kolte L, Haugaard SB, Dirksen C, Jensen FK, Ryder LP, et al. Improved thymic index, density and output in HIV-infected patients following low-dose growth hormone therapy: a placebo controlled study. AIDS Lond Engl (2009) 23(16):2123–31. doi: 10.1097/QAD.0b013e3283303307
279. Bakker B, Oostdijk W, Geskus RB, Stokvis-Brantsma WH, Vossen JM, Wit JM. Growth hormone (GH) secretion and response to GH therapy after total body irradiation and haematopoietic stem cell transplantation during childhood. Clin Endocrinol (2007) 67(4):589–97. doi: 10.1111/j.1365-2265.2007.02930.x
280. Isfan F, Kanold J, Merlin E, Contet A, Sirvent N, Rochette E, et al. Growth hormone treatment impact on growth rate and final height of patients who received HSCT with TBI or/and cranial irradiation in childhood: a report from the French leukaemia long-term follow-up study (LEA). Bone Marrow Transplant (2012) 47(5):684–93. doi: 10.1038/bmt.2011.139
281. Jimenez JJ, DelCanto GM, Popovics P, Perez A, Vila Granda A, Vidaurre I, et al. A new approach to the treatment of acute myeloid leukaemia targeting the receptor for growth hormone-releasing hormone. Br J Haematol (2018) 181(4):476–85. doi: 10.1111/bjh.15207
282. Estrov Z, Meir R, Barak Y, Zaizov R, Zadik Z. Human growth hormone and insulin-like growth factor-1 enhance the proliferation of human leukemic blasts. J Clin Oncol Off J Am Soc Clin Oncol (1991) 9(3):394–9. doi: 10.1200/JCO.1991.9.3.394
283. Maillard I, Fang T, Pear WS. Regulation of lymphoid development, differentiation, and function by the notch pathway. Annu Rev Immunol (2005) 23:945–74. doi: 10.1146/annurev.immunol.23.021704.115747
284. Radtke F, Wilson A, Stark G, Bauer M, van Meerwijk J, MacDonald HR, et al. Deficient T cell fate specification in mice with an induced inactivation of Notch1. Immunity (1999) 10(5):547–58. doi: 10.1016/S1074-7613(00)80054-0
285. Pear WS, Aster JC, Scott ML, Hasserjian RP, Soffer B, Sklar J, et al. Exclusive development of T cell neoplasms in mice transplanted with bone marrow expressing activated notch alleles. J Exp Med (1996) 183(5):2283–91. doi: 10.1084/jem.183.5.2283
286. Tikhonova AN, Dolgalev I, Hu H, Sivaraj KK, Hoxha E, Cuesta-Domínguez Á, et al. The bone marrow microenvironment at single-cell resolution. Nature (2019) 569(7755):222–8. doi: 10.1038/s41586-019-1104-8
287. Lobry C, Oh P, Mansour MR, Look AT, Aifantis I. Notch signaling: switching an oncogene to a tumor suppressor. Blood (2014) 123(16):2451–9. doi: 10.1182/blood-2013-08-355818
288. Lobry C, Ntziachristos P, Ndiaye-Lobry D, Oh P, Cimmino L, Zhu N, et al. Notch pathway activation targets AML-initiating cell homeostasis and differentiation. J Exp Med (2013) 210(2):301–19. doi: 10.1084/jem.20121484
289. Kang YA, Pietras EM, Passegué E. Deregulated notch and wnt signaling activates early-stage myeloid regeneration pathways in leukemia. J Exp Med (2019) 217(3):e20190787. doi: 10.1084/jem.20190787
290. Alarmo EL, Huhtala H, Korhonen T, Pylkkänen L, Holli K, Kuukasjärvi T, et al. Bone morphogenetic protein 4 expression in multiple normal and tumor tissues reveals its importance beyond development. Mod Pathol (2013) 26(1):10–21. doi: 10.1038/modpathol.2012.128
291. Quinn KM, Palchaudhuri R, Palmer CS, La Gruta NL. The clock is ticking: the impact of ageing on T cell metabolism. Clin Transl Immunol (2019) 8(11):e01091. doi: 10.1002/cti2.1091
292. Wang H, Gavil NV, Koewler N, Masopust D, Jameson SC. Parabiosis in mice to study tissue residency of immune cells. Curr Protoc (2022) 2(5):e446. doi: 10.1002/cpz1.446
293. Huang Y, Si X, Shao M, Teng X, Xiao G, Huang H. Rewiring mitochondrial metabolism to counteract exhaustion of CAR-T cells. J Hematol Oncol (2022) 15(1):38. doi: 10.1186/s13045-022-01255-x
294. Maude SL, Laetsch TW, Buechner J, Rives S, Boyer M, Bittencourt H, et al. Tisagenlecleucel in children and young adults with b-cell lymphoblastic leukemia. N Engl J Med (2018) 378(5):439–48. doi: 10.1056/NEJMoa1709866
295. Wesselink E, Koekkoek WAC, Grefte S, Witkamp RF, van Zanten ARH. Feeding mitochondria: Potential role of nutritional components to improve critical illness convalescence. Clin Nutr (2019) 38(3):982–95. doi: 10.1016/j.clnu.2018.08.032
Keywords: AML-acute myeloid leukemia, thymus, immunotherapy, T lymphocytes, aging, immunosenescence, hematopoietic (stem) cell transplant (HCST), tumor microenvironment
Citation: Hino C, Xu Y, Xiao J, Baylink DJ, Reeves ME and Cao H (2023) The potential role of the thymus in immunotherapies for acute myeloid leukemia. Front. Immunol. 14:1102517. doi: 10.3389/fimmu.2023.1102517
Received: 19 November 2022; Accepted: 20 January 2023;
Published: 06 February 2023.
Edited by:
Jenny L. Persson, Umeå University, SwedenReviewed by:
Robert J. Canter, University of California, Davis, United StatesMinoru Kanaya, Aiiku Hospital, Japan
Copyright © 2023 Hino, Xu, Xiao, Baylink, Reeves and Cao. This is an open-access article distributed under the terms of the Creative Commons Attribution License (CC BY). The use, distribution or reproduction in other forums is permitted, provided the original author(s) and the copyright owner(s) are credited and that the original publication in this journal is cited, in accordance with accepted academic practice. No use, distribution or reproduction is permitted which does not comply with these terms.
*Correspondence: Yi Xu, ZHl4dUBsbHUuZWR1