- 1State Key Laboratory of Trauma, Burns and Combines Injury, Department of Wound Infection and Drug, Daping Hospital, Army Medical University (Third Military Medical University), Chongqing, China
- 2School of Laboratory Medicine and Technology, Harbin Medical University, Daqing, China
Sepsis is one of the major causes of death in the hospital worldwide. The pathology of sepsis is tightly associated with dysregulation of innate immune responses. The contribution of macrophages, neutrophils, and dendritic cells to sepsis is well documented, whereas the role of natural killer (NK) cells, which are critical innate lymphoid lineage cells, remains unclear. In some studies, the activation of NK cells has been reported as a risk factor leading to severe organ damage or death. In sharp contrast, some other studies revealed that triggering NK cell activity contributes to alleviating sepsis. In all, although there are several reports on NK cells in sepsis, whether they exert detrimental or protective effects remains unclear. Here, we will review the available experimental and clinical studies about the opposing roles of NK cells in sepsis, and we will discuss the prospects for NK cell-based immunotherapeutic strategies for sepsis.
1 Introduction
Sepsis is a life-threatening multiple-organ dysfunction syndrome caused by localized or systemic infections, which is one of the major causes of death to patients in the hospital worldwide (1–3). It has been estimated that approximately 750,000 people suffer from sepsis every year in the United States and an estimated 20-30% patients die from it (4, 5). However, there is no specific, standardized treatment strategy for sepsis (6). Numerous studies have shown that dysregulation of innate immune responses is a major contributing factor to the incidence and development of sepsis (7, 8). For example, studies on monocytes, macrophages, neutrophils, and dendritic cells have provided insight into their roles in both the inflammatory and immunosuppressive phases of sepsis (9–14). Natural killer (NK) cells, which were discovered in the early 1970’s (15, 16), are a heterogeneous group of innate lymphocytes with the capacity to regulate both innate and adaptive immune responses. They are best known for their roles in fighting infections and tumors, mainly relying on their cytotoxicity and immune regulatory properties (17).
Recent studies have implicated NK cells in the pathological process of sepsis, suggesting that they might be employed as prognostic biomarkers or therapeutic targets (2, 18). However, seemingly contradictory conclusions about NK cells playing beneficial or harmful roles in sepsis have been obtained (19). Hence, we will review these reports to discuss whether NK cells are friends or foes in sepsis, and we will further discuss the prospects of NK cell-based immunotherapy for sepsis.
2 The immunological characteristics of sepsis
Sepsis has previously been used to describe severe disease caused by infection (20). However, this definition cannot accurately describe its complex pathological processes. Recently, a new definition has been published, stating that sepsis refers to a life-threatening, multiple-organ failure syndrome, caused by dysregulated responses to infection (21, 22). It is generally believed that immunological abnormalities are the pathological basis of sepsis (23), which is tightly associated with microvascular injury, abnormal coagulation, hemodynamic instability, multiple organ damage and other conditions (24). The immunological abnormities exhibit distinct disease stage-specific characteristics during sepsis: hyperinflammation at the initial stage and immunosuppression at the late stage (25). A diagram illustrating this process is shown in Figure 1.
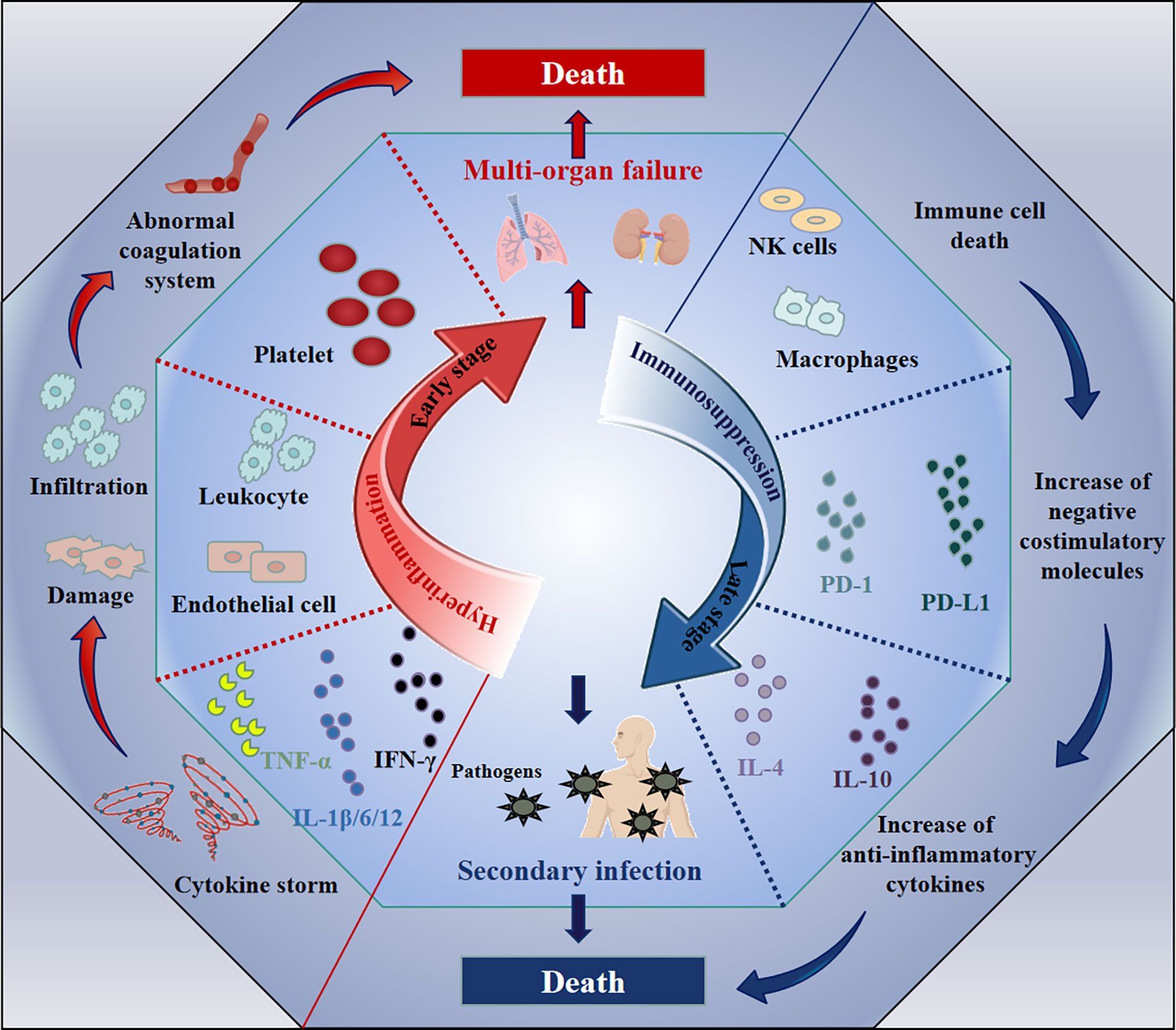
Figure 1 The immune changes during the pathological process of sepsis. The immunological abnormity exhibits two distinct stages accompanying with the sepsis development: hyperinflammation and immunosuppression. During the hyperinflammatory phase at early, the increase of pro-inflammatory cytokines (e.g., IL-1β/6/12, IFN-γ, and TNF-α) leads to cytokine storm, resulting in the vascular system damage (e.g., endothelial cell damage), the abnormal coagulation, finally multi-organ failure and death. Subsequently, the death of immune cells, the increase of negative costimulatory molecules (e.g., PD-1 and PD-L1) or anti-inflammatory cytokines (e.g., IL-4 and IL-10) induces immunosuppression, which leads to uncontrolled secondary infection and death.
After invading the body, pathogens will encounter the first line of defense composed of innate immune cells, activating PAMP (pathogen-associated molecular pattern)- or DAMP (damage-associated molecular pattern)-associated signaling pathways in these cells (26, 27). Once activated, these cells generate large amounts of inflammatory cytokines, such as IL-1β, IL-6, IL-12, TNF-α and IFN-γ (28, 29). These host responses are not limited to the infectious focus. The cytokines may trigger additional immune cells at distant sites to secrete inflammatory cytokines, and this cascading amplification reaction may finally result in systemically uncontrolled over-inflammation, which is termed a “cytokine storm” (30, 31). The massively increased cytokine levels may potentially enhance the elimination of pathogens by innate immune cells. However, they also lead to a series of pathological changes, such as endothelial cell damage, leukocyte infiltration, abnormal activation of the coagulation system and other abnormalities, resulting in multi-organ failure and even death (32–34). Consequently, the direct cause of death is not the invasive pathogens themselves, but the over-activated immune reactions. Therefore, the focus of clinical treatment at this inflammatory stage of sepsis is on ameliorating the uncontrolled inflammation (35).
The hyperinflammation at the early stage of sepsis will lead to immunosuppression during the late stage of sepsis: on the one hand, the cytokine storm directly induces cell death in various immune cells; on the other hand, the functions of some effector cells will be exhausted after their excessive activation (36, 37). Moreover, upregulation of some negative costimulatory molecules and anti-inflammatory cytokines has also been observed during this stage, and includes programmed cell death 1 (PD-1) (38), programmed cell death ligand 1 (PD-L1) (39), T-cell immunoglobulin and mucin domain-containing protein-3 (TIM-3) (40), T cell Ig and ITIM domain (TIGIT) (41), IL-4 (36), IL-10 (42, 43) and TGF-β (44, 45). These factors are mainly related to exhaustion of immune cells or inhibition of their effector functions (38, 46–48). As a result, the body presents with a continuously immunosuppressive state, nearly losing its capacity to clear pathogens (49). This will cause an extremely high risk for secondary infections, such as those mediated by opportunistic pathogens or iatrogenic infections caused by interventional therapy, which eventually leads to death of sepsis patients (50). For example, Huang et al. observed that the expression of TIM-3 on CD4 T cells in patients with sepsis-induced immunosuppression was significantly elevated, which impaired anti-infective responses and positively correlated with mortality (51). Hou et al. also found that, in a lipopolysaccharide (LPS)-induced murine sepsis model, TIM-3 expression on NK cells negatively regulated the production of IFN-γ, which caused death (40). Therefore, reestablishing immune functions is critical to reduce mortality risk of sepsis patients during the late immunosuppressive stage (52, 53).
3 NK cells play a role in antimicrobial responses
NK cells, a group of large granular lymphocytes derived from the bone marrow, are essential components of the innate immune response and can directly kill tumors and other target cells without prior activation (54–56). In humans, about 5-15% of lymphocytes are defined as NK cells in peripheral blood, and tissue-specific subpopulations are found in the spleen, liver, and lung (57–61). Generally, human NK cells can be divided into two subpopulations by the expression of CD56 and CD16 on the cell membrane (62, 63). About 90% of all NK cells in human peripheral blood are CD56dimCD16bright, whereas only 10% are CD56brightCD16-/dim (64). Distinct human NK cell subpopulations found in different tissues significantly differ in cytotoxicity and cytokine secreting capacity (65, 66). The two main subpopulations possess distinct functions: CD56dimCD16bright NK cells exhibit higher cytotoxicity and express increased levels of killer immunoglobulin-like receptors (KIR) or CD57 receptors; CD56brightCD16-/dim NK cells can secrete more cytokines and possess greater proliferative capacity (67, 68).
NK cells can be activated in several ways. Most importantly, the balance between signals from the inhibitory or activating receptors expressed on the cell surface plays a critical role in regulating their responses (69, 70). The activating receptors mainly include NCRs (NKp30, NKp44, and NKp46), KIR-2Ds, KIR-3Ds, NKG2D, CD226, 2B4, and NKG2C, whereas the inhibitory receptors mainly include NKG2A, TIGIT, KIR-2DL, and KIR-3DL (71). The biased expression of these receptors or their ligands calibrates the activation status of NK cells. For example, a clinical study reported that, in human immunodeficiency virus (HIV)-infected patients, a subpopulation of human NK cells that expresses NKG2C but not NKG2A has a stronger ability to secrete IFN-γ compared with other NK cells (72). Another typical way of NK activation is via their pathogen recognition receptors (PRRs), which bind with PAMPs on bacteria (73). For example, a previous study reported that high-mobility group box-1 (HMGB-1) up-regulated the levels of TLR-2/4, which belongs to the group of classical PRRs (74), on murine NK cells, leading to their activation in rotavirus-induced murine biliary atresia (75). Additionally, NK cells can also be activated by several cytokines, including type 1 interferon, IL-2, IL-12, IL-15, IL-18, IL-21, and IL-27 (76–80). For instance, IL-12 binding to IL-12Rβ1/2 stimulates NK cells through signal transducer and activator of transcription 4 (STAT4) phosphorylation, leading to abundant IFN-γ and TNF-α production (81).
During infection, activated NK cells perform their activity mainly in two ways: cytotoxicity and immune regulation. First, NK cells can directly lyse bacteria-infected cells with their cytotoxicity: on the one hand, they can induce target cell apoptosis depending on the binding of FAS-L to FAS death receptors (82); on the other hand, they directly kill targets by secreting cytotoxic proteins, such as perforin, granzyme and α-defensins (83–85). Specifically, some studies have reported that these cytotoxic proteins could disrupt the membrane of some bacteria, such as Mycobacterium, Salmonella typhimurium, Bacillus anthracis, Escherichia coli, and Staphylococcus aureus (86–89), thus causing their death. In addition to cytotoxicity, activated NK cells also secrete several cytokines to undertake the roles of immune regulation (90). IFN-γ, which is the major cytokine released by NK cells, was reported to play a critical role in fighting microbial infections (91). It modulates the activation of other immune cells, such as macrophages or dendritic cells, enabling them to perform comprehensive anti-bacterial responses (92, 93). Moreover, IL-32, previously named as NK cell transcript 4 (NK4), can be produced by NK cells when activated by IL-2 (94, 95). It also stimulates inflammatory responses by inducing monocytes or macrophages to secrete various cytokines, including TNF-α, IL-1β, IL-6 or IL-8 (96). Thus, IL-32 has been reported to exacerbate sepsis in the cecal-ligation and puncture (CLP) mouse model, via propagating vascular inflammation (97).
In addition to their positive regulatory roles, NK cells also possess the ability to limit antimicrobial responses. A recent study uncovered that NK cell-derived IFN-γ worsened macrophage phagocytosis of zymosan in mice and increased the susceptibility to secondary Candida infection during post-sepsis immunosuppression (98). However, whether this phenomenon exists in sepsis caused by other pathogens needs further study. Furthermore, activated NK cells also secrete IL-10, which is a well-known immunosuppressive cytokine (99–101). In fact, NK cells are the main source of IL-10 in systemic infection caused by some pathogens, such as Yersinia pestis, Listeria monocytogenes or Toxoplasma gondii (99). Interestingly, the NK cell-derived IL-10 appears to play dual roles in different types of infections. For example, in Listeria monocytogenes infection, the NK cell-derived IL-10 shows detrimental effects on host resistance against the invasive pathogen (102), whereas it can protect the host from murine cytomegalovirus infection or CLP-induced sepsis by reducing systemic inflammation (103, 104). The authors consider that the beneficial or detrimental roles of IL-10 might depend on whether the major cause of host death is pathogen overload or excessive inflammation during infection.
Summarily, the patterns of NK cell activation and their roles in antimicrobial responses are illustrated in Figure 2.
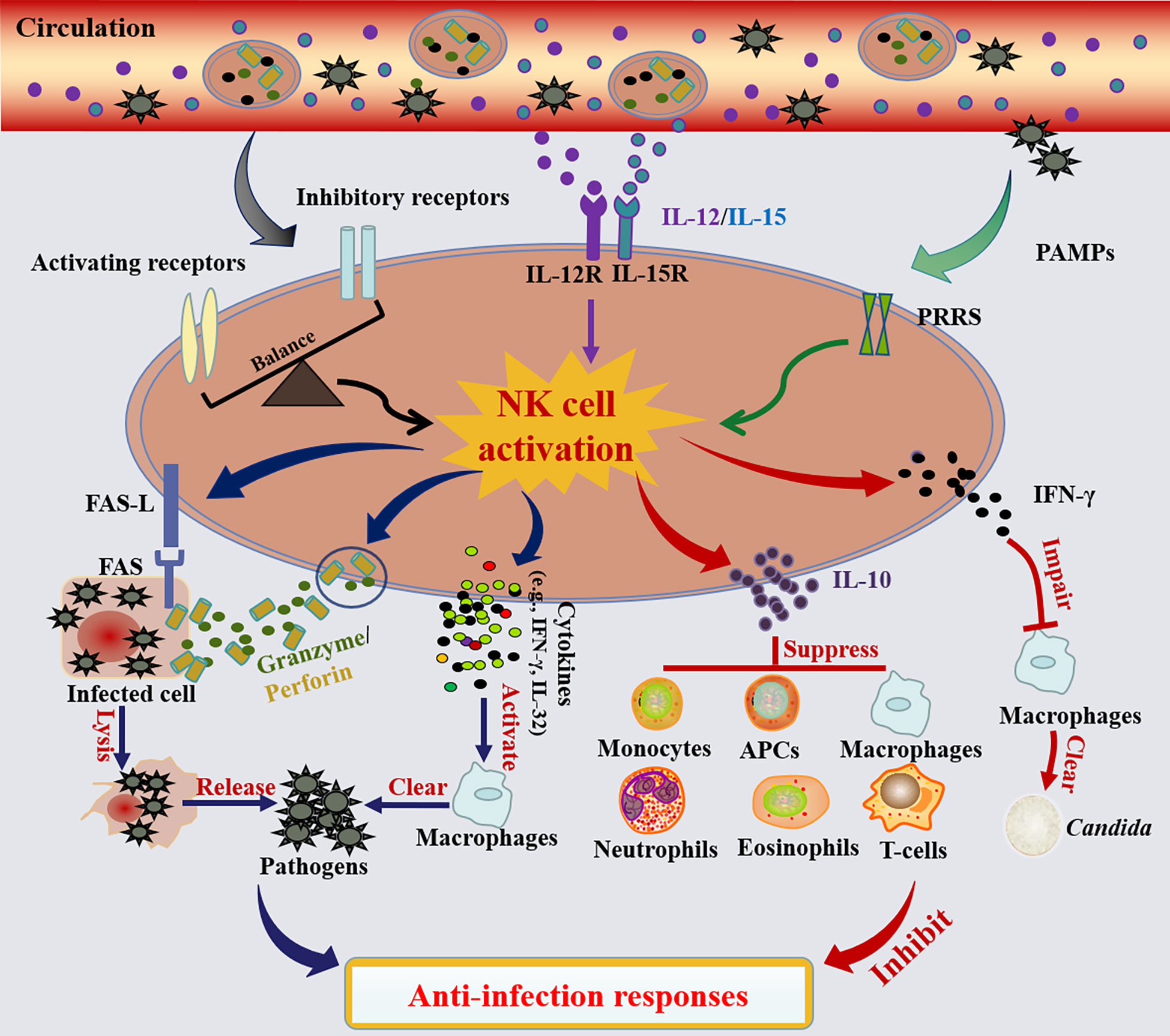
Figure 2 NK cell activation and their roles in the anti-infection responses. NK cells are mainly activated in three ways: 1) The activation of NK cells is governed by a balance between signals delivered through activated and inhibitory receptors. When the activating signal dominates, NK cells will be activated, and vice versa. 2) Activation of NK cells can also be achieved by stimulation with cytokines (e.g., IL-12 and IL-15). 3) NK cells are activated by pathogen-associated molecular patterns (PAMPs) through pattern recognition receptors (PRRs). Activated NK cells lysis infected cells and release pathogens via death receptor ligand/death receptor (e.g., FAS-L/FAS) and secreting cytotoxic proteins (e.g., perforin and granzyme). Meanwhile, activated NK cells promote the activation of macrophage-mediated microbial killing by the secretion of cytokines (e.g., IFN-γ, IL-32). In contrast, activated NK cells also possess the ability to limit the anti-infection responses. On one hand, NK cell-derived IFN-γ especially worsened macrophage phagocytosis of zymosan.; on the other hand, the activated NK cells also secrete IL-10, which can generally inhibit the anti-infection responses of monocytes, antigen-presenting cells (APCs), macrophages, neutrophils, eosinophils or T cells.
4 NK cells act as risk factors in sepsis
Accumulating studies have shown that NK cells play a contributing role in the inflammatory responses caused by infection (105, 106). In this context, they are considered a risk factor for aggravating the septic process during the hyperinflammation stage (107). At the early stage of sepsis, NK cells will be activated through the ways discussed above, secreting abundant cytokines, such as IFN-γ, TNF-α or IL-32, which can trigger dramatic responses in macrophages or dendritic cells (54, 96). Mutually, the activated macrophages and dendritic cells secrete IL-2, IL-12 or IL-18 to subsequently further activate NK cells, forming a positive feedback loop (108, 109). This loop amplifies the pro-inflammatory responses, resulting in a cytokine storm and finally causing multiple organ failure (54). In addition, the cytotoxic proteins secreted from activated NK cells, including perforin and granzyme, are also reported to directly mediate tissue necrosis and damage (54) (Figure 3). Therefore, several studies have shown that antagonizing murine NK cells during sepsis significantly ameliorates multiorgan damage caused by inflammation and enhanced tolerance in mice. For example, in sepsis mouse models caused by CLP surgery, Streptococcus pneumoniae, Escherichia coli or Streptococcus pyogenes infection, NK cell clearance using anti-asialoGM1 and anti-NK1.1 antibodies can reduce systemic inflammation, stabilize acid-base balance in the circulation, improve organ damage, reverse physiological disorders and prolong overall survival (110–116). Moreover, in a murine polytrauma model, which is a major instigator of sepsis, murine NK cell depletion also attenuated inflammatory responses and improved the outcomes (117).
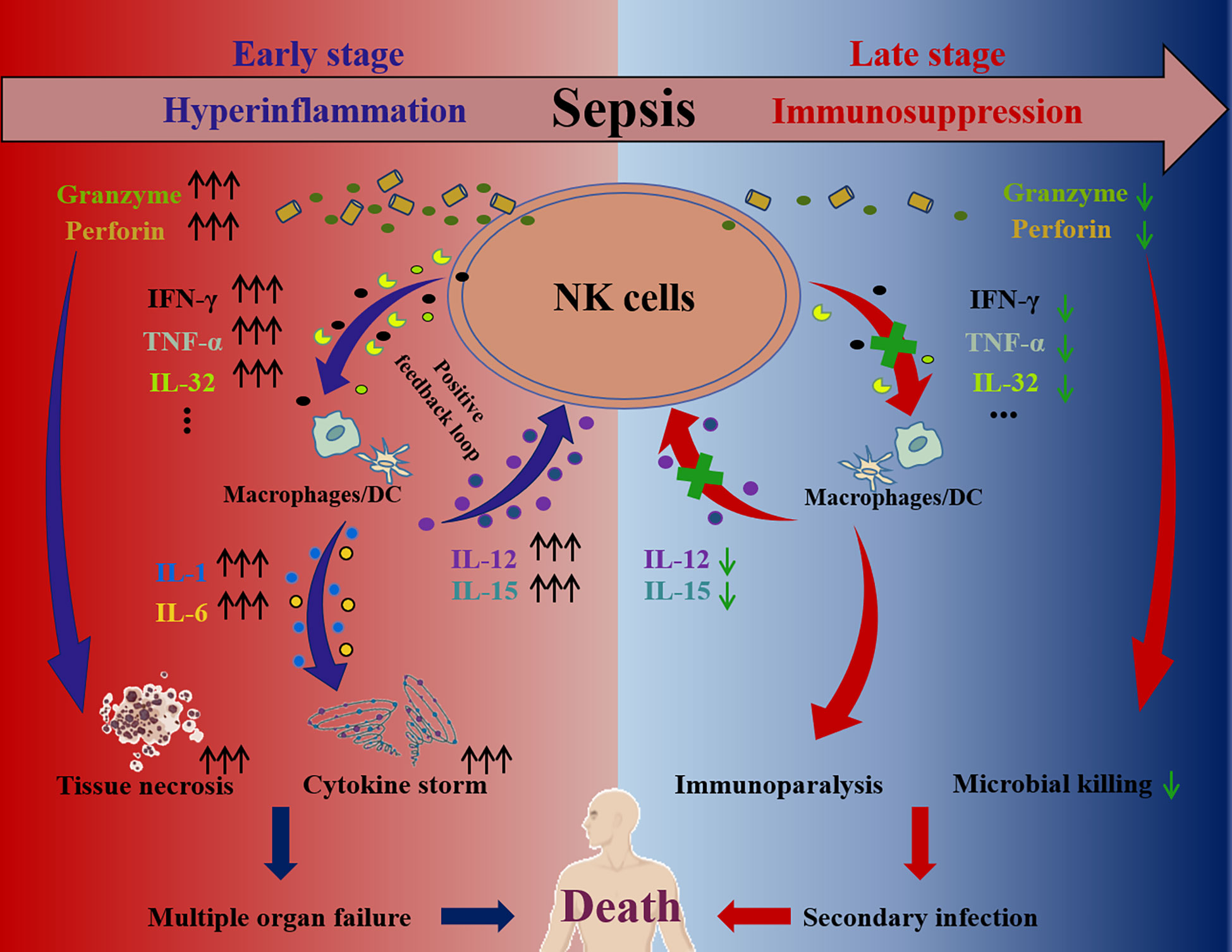
Figure 3 The pathological roles of NK cell at the hyperinflammation and immunosuppression stage of sepsis. During sepsis hyperinflammation, NK cells activation is dysregulated and NK cells secrete abundant cytokines, including IFN-γ, TNF-α, IL-32 and so on. These cytokines subsequently facilitate secretion of more cytokines (e.g., IL-12, IL-15, IL-1, IL-6, and so on) by dendritic cells and macrophages, establishing a positive feedback loop and amplifying cytokine storm. Furthermore, cytotoxic proteins (e.g., perforin, granzyme) secreted by NK cells are increased and cause tissue necrosis. As a result, the cytokine storm and tissue necrosis eventually lead to multiple organ failure and death. In contrast, the secretions of cytokines (e.g., IFN-γ, TNF-α, IL-32, and so on) and cytotoxic proteins (e.g., perforin, granzyme) of NK cells are impaired at the immunosuppression stage of sepsis, which contributes to the immunoparalysis, causing secondary infection and even death.
IL-15 is an essential cytokine to maintain NK cell development and maturation, which can also strongly activate NK cells at high concentrations (118). It has been reported that excessive IL-15 stimulation leads to pathological inflammatory responses similar to sepsis, resulting in the death of mice due to massive NK cell proliferation and IFN-γ production (119). Furthermore, IL-15 knockout mice, characterized by NK cell loss, also showed tolerance to sepsis due to CLP surgery (120). When bacterial infection occurs, NK cells may rapidly migrate to the infection site and promote inflammation (121, 122). It has been reported that murine NK cells expressing CXCR3 can rapidly migrate to the abdominal cavity within 4-6 h following severe abdominal infection (123). These CXCR3-positive NK cells are similar to the human CD56bright subpopulation in their ability to secrete more proinflammatory cytokines and express more activation makers (124). Blocking CXCR3 or its ligand, CXCL10, can significantly reduce inflammation during sepsis in mice and increase their survival rate (125). In addition to the organ damage caused by massive inflammatory cytokine secretion, NK cell-mediated cytotoxicity is also detrimental in sepsis. For example, mice deficient in perforin or in granzymes A/M exhibit increased tolerance to sepsis caused by LPS (126).
Additionally, significant changes in the number, phenotypes, and functions of NK cells in sepsis patients have been observed in several clinical studies. David Andaluz-Ojeda et al. showed that NK cell levels were significantly increased in patients who died from sepsis and the cell counts at day 1 were independently associated with increased risk of death at 28 days (hazard ratio = 3.34, 95% CI = 1.29 to 8.64; P = 0.013). Analysis of survival curves provided evidence that human NK cell levels at day 1 (> 83 cells/mm³) were associated with early mortality (127). Palo et al. also found that sepsis patients with the highest NK cell numbers exhibit the lowest survival probability (128).
In all, during the hyperinflammation stage, the disturbance of inflammatory factors leads to abnormal NK cell activation, which can trigger a cytokine storm through a positive feedback loop, resulting in severe organ damage (92, 109). Thus, neutralizing or inhibiting NK cell-derived pro-inflammatory cytokines (e.g., IFN-γ) or cytotoxic proteins (e.g., perforin, granzyme) can alleviate systemic inflammatory responses and protect against organ damage. Furthermore, using anti-inflammatory cytokines, such as IL-10, to treat sepsis is also worth considering. We have summarized the evidence showing the detrimental roles of NK cells from both animal and human sepsis in Table 1. These findings implicate NK cells as risk factors during sepsis.
5 The protective roles of NK cells in sepsis
Conversely, some other studies have provided evidence for a protective role of NK cells in a variety of microbial infections. For instance, murine NK cells are essential in coordinating host responses against sepsis caused by Staphylococcus aureus infection (129, 130). This may be due to their interactions with the anti-inflammatory mechanisms of the host. Moreover, once the ability of NK cells to secrete IFN-γ is impaired, progressive immune disorders might be induced. There is evidence showing that neutralization of IL-10 with antibodies in mice improves the ability of NK cells to secrete IFN-γ, resulting in improved survival (131). Notably, in the Citrobacter rodentium infection model, murine NK cells not only directly lyse the bacteria but also recruit other intrinsic immune cells and activate their antibacterial functions by secreting cytokines (132). Similarly, during Pseudomonas aeruginosa infection, NK cells can recruit neutrophils to the lungs, alleviating infection and improving animal survival (133). In mice infected with pulmonary nontuberculous mycobacteria, the bacterial load and mortality rate are increased by NK cell clearance (134). Interestingly, it has also been reported that IL-15 treatment after CLP surgery can reduce immune cell apoptosis, improve immune disorders, and increase mouse survival (135, 136).
A protective role of NK cells in sepsis has also been documented in several clinical studies. Some researchers reported a significant increase in the number of human peripheral blood NK cells, their expression of active biomarkers, and their ability to secrete granzyme A/B, IFN-γ or IL-12P40 (117, 137–139), which were considered to provide a survival benefit for septic patients. Bourboulis et al. showed that sepsis patients with increased levels of NK cells (>20% of all lymphocytes) survived longer than those patients with lower levels of NK cells (< or =20% of all lymphocytes) (140). Boomer et al. reported that NK cells in peripheral blood of sepsis patients were significantly reduced within 24 h, which may predispose some patients to nosocomial infections and poor outcomes (141). Consistently, Holub et al. found that human NK cells were decreased within the first 48 h of sepsis, especially in patients with Gram-negative bacterial infection, resulting in increased risk of septic complications (142). Moreover, single-cell RNA-sequencing (scRNA-seq) analysis revealed that various cytotoxic genes of NK cells were downregulated in patients with late sepsis (n=4), which might be associated with the re-occurrence of severe infections (143).
Under the conditions described in this section, replenishing subjects with functional NK cells may hinder the immunosuppressive stage of sepsis. Furthermore, blocking inhibitory receptors, activating NK cells by cytokines (e.g., IL-15, IL-2) or neutralizing suppressive cytokines (e.g., IL-4, IL-10) may also be beneficial. In summary, the evidence supporting the protective roles of NK cells in both animal and clinical studies are shown in Table 2.
Taken together, the roles of NK cells in sepsis remain controversial. Furthermore, animal and clinical studies have revealed dual roles of NK cell activity on sepsis progression. The impact on disease mainly depends on the pathological stage and the initial infection focus. Although the functional changes of NK cells and their influence on pathological progresses have been explored in previous studies, they mainly focused on the early stages after sepsis. During the sepsis process lasting several months from occurrence to recovery, the impact of continuous changes in NK cell numbers and characteristics remains unclear.
6 NK cells in COVID-19 infection
In late 2019, coronavirus disease 2019 (COVID-19) emerged and rapidly spread throughout the world (144, 145). As of December 2022, the COVID-19 pandemic has resulted in approximately 641,915,931 confirmed cases, including 6,622,760 deaths worldwide (https://covid19.who.int/ ). A meta-analysis revealed that the overall pooled sepsis prevalence estimates among 218,184 COVID-19 patients, irrespective of ICU or non-ICU admission, were 51.6% (95% CI, 47.6-55.5, I2 = 100%) (146). Sepsis was one of the major causes of death for COVID-19 patients. During acute COVID-19 infection, the number of the CD56bright and CD56dim human NK cells dropped dramatically in the circulation (147, 148). However, this drop was likely related to the homing of human NK cells from the circulation to the lung because NK cells were increased in bronchoalveolar lavage (BAL) (149, 150). Moreover, a clinical trial discovered that a high frequency of NK cells was significantly associated with asymptomatic COVID-19 infection (151). In addition to lower circulating counts, NK cell dysfunction was also observed. NK cell hyperactivation driven by IL-6, IL-15 and IL-18 has been considered as one of the features of COVID-19 (152–154). Furthermore, Maucourant et al. used high-dimensional flow cytometry to reveal that NK cells in COVID-19 patients were at a higher activation state containing high levels of cytotoxic proteins, such as perforin (155). However, prolonged hyperactivation usually leads to impaired NK cell function. Yao et al. reported that genes involved in NK cell cytotoxicity were suppressed in severely ill COVID-19 patients (156). Moreover, some studies also reported that NK cell activity was impaired via over expression of the inhibitory receptor NKG2A in COVID-19 patients (157, 158).
Due to their lower circulating counts and dysfunction, NK cell adoptive transfer or reconstitution could be a possible treatment for COVID-19 patients. In fact, some innovate clinical trials using human NK cells to treat COVID-19 patients are active (ClinicalTrials.gov# NCT04280224, NCT04578210). Additionally, a clinical trial to determine the safety and efficacy of NK cells derived from human placental hematopoietic stem cells in patients with moderate COVID-19 is also ongoing (ClinicalTrials.gov# NCT04365101). Finally, an NKG2D chimeric antigen receptor (CAR)-NK cell-based trial may provide a safe and effective cell therapy for COVID-19 (ClinicalTrials.gov# NCT04324996). These studies are summarized in Table 3.
7 The prospects of NK cell-based immunotherapy for sepsis
Recently, NK cells have gained great attention in the field of immunotherapy, especially in cancer treatment. The anti-tumor activities of infused NK cells have been demonstrated widely in mouse models of glioblastoma, ovarian cancer, and metastatic colorectal cancer (165–167). For example, Veluchamy et al. showed that adoptive transfer of NK cells into mice with metastatic colorectal cancer inhibited tumor growth in vivo and prolonged survival time (168). There has an explosion of NK cell-based cancer immunotherapies in clinical trials on acute myeloid leukemia (AML), non-Hodgkin lymphoma (NHL), neuroblastoma, multiple myeloma (MM) and other cancers (159–164). In addition, a few clinical trials using NK cells to treat patients with ovarian carcinomas, hematological cancer, B cell lymphoma, and glioblastoma are ongoing (ClinicalTrials.gov# NCT03539406, NCT03841110, NCT04023071, NCT03383978). We have summarized these completed and ongoing clinical trials in Table 3. Recently, a variety of NK cell-based immunotherapies were developed to treat viral infections such as COVID-19 (as discussed above) and HIV (ClinicalTrials.gov# NCT03899480, NCT03346499). Although these treatments have not yet achieved the same degree of success as clinical T cell-based therapies, the abundant pre-clinical or clinical studies with NK cell-based immunotherapies have led to increasing enthusiasm in exploring their potential to treat other diseases, including sepsis.
A variety of tissue sources for deriving NK cells for immunotherapy have been developed, including autologous and allogeneic NK cells (169). Autologous NK cell infusion using the patient′s own blood as a source was the first focus in adoptive NK cell therapy, which is associated with low risk of graft-versus-host disease (169). However, this approach usually leads to exhausted NK cell functions (170). Furthermore, patients must receive an extensive preparative treatment regimen before infusion, which may cause serious negative side effects (171). For allogeneic NK cells, the requirement for a healthy donor as source of NK cells and expanding them to clinically relevant doses is the most critical step (172). Therefore, umbilical cord blood (UCB) (173) and induced pluripotent stem cells (iPSCs) have been considered as optimal sources (174). UCB NK cells are younger and more proliferative (175), can be manufactured at multiple doses (176), and possess high cytotoxicity to lyse target cells (177). However, UCB NK cells are relatively unstable due to common delays in blood collection and heterogeneity of leukocytes from different donors (169). Stem cells represent a potentially unlimited source of NK cells for adoptive immunotherapy, and iPSCs provide a universal cell source (174). NK cells derived from iPSCs can be genetically modified and expanded to a homogenous population on a large scale (178). Furthermore, NK cells derived from iPSCs display increased cytotoxicity and greater antitumor activity than UCB NK cells in models of leukemia (179). However, more efficient strategies to generate NK cells from iPSCs are still needed.
As discussed above, NK cells significantly impact the pathological progression of sepsis. We postulate that NK cell-based immunotherapies may be developed as an excellent therapeutic option for sepsis, for the following reasons: 1. The adoptive transfer of NK cells has been proven safe due to their short lifespan and the low risk of triggering graft-versus-host reactions (180, 181); 2. NK cells can kill targets without sensitization; therefore, developing NK cells as “off-the-shelf” products has recently attracted great attention in the field (182), which can overcome the challenging problem of the narrow time window available for sepsis treatment; 3. The pathological process of sepsis is characterized by distinct stages of hyperinflammation and immunosuppression, and NK cells also have dual roles in immune regulation. Therefore, we may envisage an “off-the-shelf” NK cell product developed from editable iPSC-NK cells, which can sense its immune microenvironment to program opposing activities: in a hyperinflammatory environment, these NK cells may be programmed to mainly exert anti-inflammatory properties, whereas in an immunosuppressive environment, they are programmed to promote immune activation. Although few studies on NK cell-based immunotherapies for sepsis have been performed, inspired by explorations on cancer and viral infection and with the expanded knowledge on mechanisms of NK cell responses in sepsis, we can make the bold prediction that the future of NK cell-based immunotherapy for sepsis is bright.
In conclusion, developing NK cell-targeted immunotherapeutic strategies for sepsis highly depends on the disease state. A dynamic and more comprehensive understanding of the pathological process of sepsis will be critically important. Therefore, we consider using high-throughput sequencing technologies to dynamically monitor NK cell alterations during the early, middle, and late stages of sepsis essential for an accurate and deep understanding of NK cells in sepsis. Hopefully, with the growing understanding about NK cells in sepsis, safer and more efficient immunotherapies for sepsis can be developed.
Author contributions
The work presented was performed in collaboration by all authors. FW and YC designed and wrote the manuscript. DH revised the manuscript. LG improved the language. HL devised the concept and revised the paper. All authors contributed to the article and approved the submitted version.
Funding
This study was supported by grants from Military Biosafety Program (19SWAQ18, to HL), National Defense Science and Technology Basic Enhancement Program (2021-JCJQ-JJ-1081, to HL), the Natural Science Foundation of Chongqing (cstc2020jcyj-msxmX0510, to FW), 2019 Army Medical Center Talent Innovation Program (2019CXJSC016, to FW) and the Doctor’s Through Train Project of Chongqing (CSTB2022BSXM-JCX0024, to FW).
Acknowledgments
We thank Li Wei, Ma Xiaoyuan, Gao Rui, Zhu Junyu and Luo Li from the Department of Wound Infection and Drug of Daping Hospital (Army Medical University, Chongqing, China) for their valuable advice.
Conflict of interest
The authors declare that the research was conducted in the absence of any commercial or financial relationships that could be construed as a potential conflict of interest.
The reviewer YD declared a shared affiliation with the authors to the handling editor at the time of review.
Publisher’s note
All claims expressed in this article are solely those of the authors and do not necessarily represent those of their affiliated organizations, or those of the publisher, the editors and the reviewers. Any product that may be evaluated in this article, or claim that may be made by its manufacturer, is not guaranteed or endorsed by the publisher.
References
1. Gül F, Arslantaş MK, Cinel İ, Kumar A. Changing definitions of sepsis. Turk J Anaesthesiol Reanim (2017) 45(3):129–38. doi: 10.5152/tjar.2017.93753
2. Kumar V. Natural killer cells in sepsis: Underprivileged innate immune cells. Eur J Cell Biol (2019) 98(2-4):81–93. doi: 10.1016/j.ejcb.2018.12.003
3. Ackerman MH, Ahrens T, Kelly J, Pontillo A. Sepsis. Crit Care Nurs Clinics North America (2021) 33(4):407–18. doi: 10.1016/j.cnc.2021.08.003
4. Fleischmann C, Scherag A, Adhikari NK, Hartog CS, Tsaganos T, Schlattmann P, et al. Assessment of global incidence and mortality of hospital-treated sepsis. current estimates and limitations. Am J Respir Crit Care Med (2016) 193(3):259–72. doi: 10.1164/rccm.201504-0781OC
5. Kahn JM, Le T, Angus DC, Cox CE, Hough CL, White DB, et al. The epidemiology of chronic critical illness in the united states*. Crit Care Med (2015) 43(2):282–7. doi: 10.1097/ccm.0000000000000710
6. Landersdorfer CB, Nation RL. Key challenges in providing effective antibiotic therapy for critically ill patients with bacterial sepsis and septic shock. Clin Pharmacol Ther (2021) 109(4):892–904. doi: 10.1002/cpt.2203
7. Patricio P, Paiva JA, Borrego LM. Immune response in bacterial and candida sepsis. Eur J Microbiol Immunol (2019) 9(4):105–13. doi: 10.1556/1886.2019.00011
8. Chen H, Zhang X, Su H, Zeng J, Chan H, Li Q, et al. Immune dysregulation and RNA N6-methyladenosine modification in sepsis. Wiley Interdiscip Rev RNA (2022):e1764. doi: 10.1002/wrna.1764
9. Qiu P, Liu Y, Zhang J. Review: The role and mechanisms of macrophage autophagy in sepsis. Inflammation (2019) 42(1):6–19. doi: 10.1007/s10753-018-0890-8
10. Cheng Y, Marion TN, Cao X, Wang W, Cao Y. Park 7: A novel therapeutic target for macrophages in sepsis-induced immunosuppression. Front Immunol (2018) 9:2632. doi: 10.3389/fimmu.2018.02632
11. Shen XF, Cao K, Jiang JP, Guan WX, Du JF. Neutrophil dysregulation during sepsis: An overview and update. J Cell Mol Med (2017) 21(9):1687–97. doi: 10.1111/jcmm.13112
12. Shen X, Cao K, Zhao Y, Du J. Targeting neutrophils in sepsis: From mechanism to translation. Front Pharmacol (2021) 12:644270. doi: 10.3389/fphar.2021.644270
13. Wu DD, Li T, Ji XY. Dendritic cells in sepsis: Pathological alterations and therapeutic implications. J Immunol Res (2017) 2017:3591248. doi: 10.1155/2017/3591248
14. Kumar V. Dendritic cells in sepsis: Potential immunoregulatory cells with therapeutic potential. Mol Immunol (2018) 101:615–26. doi: 10.1016/j.molimm.2018.07.007
15. Kiessling R, Klein E, Pross H, Wigzell H. "Natural" killer cells in the mouse. ii. cytotoxic cells with specificity for mouse moloney leukemia cells. characteristics of the killer cell. Eur J Immunol (1975) 5(2):117–21. doi: 10.1002/eji.1830050209
16. Pross HF, Jondal M. Cytotoxic lymphocytes from normal donors. a functional marker of human non-T lymphocytes. Clin Exp Immunol (1975) 21(2):226–35.
17. Kucuksezer UC, Aktas Cetin E, Esen F, Tahrali I, Akdeniz N, Gelmez MY, et al. The role of natural killer cells in autoimmune diseases. Front Immunol (2021) 12:622306. doi: 10.3389/fimmu.2021.622306
18. Souza-Fonseca-Guimaraes F, Cavaillon JM, Adib-Conquy M. Bench-to-Bedside review: Natural killer cells in sepsis - guilty or not guilty? Crit Care (2013) 17(4):235. doi: 10.1186/cc12700
19. Bohannon J, Guo Y, Sherwood ER. The role of natural killer cells in the pathogenesis of sepsis: The ongoing enigma. Crit Care (2012) 16(6):185. doi: 10.1186/cc11881
20. Faix JD. Biomarkers of sepsis. Crit Rev Clin Lab Sci (2013) 50(1):23–36. doi: 10.3109/10408363.2013.764490
21. Singer M, Deutschman CS, Seymour CW, Shankar-Hari M, Annane D, Bauer M, et al. The third international consensus definitions for sepsis and septic shock (Sepsis-3). JAMA (2016) 315(8):801–10. doi: 10.1001/jama.2016.0287
22. Cecconi M, Evans L, Levy M, Rhodes A. Sepsis and septic shock. Lancet (London England) (2018) 392(10141):75–87. doi: 10.1016/s0140-6736(18)30696-2
23. Huang M, Cai S, Su J. The pathogenesis of sepsis and potential therapeutic targets. . Int J Mol Sci (2019) 20(21):5376. doi: 10.3390/ijms20215376
24. Jacobi J. The pathophysiology of sepsis - 2021 update: Part 2, organ dysfunction and assessment. Am J health-system Pharm AJHP Off J Am Soc Health-System Pharmacists (2022) 79(6):424–36. doi: 10.1093/ajhp/zxab393
25. Mithal LB, Arshad M, Swigart LR, Khanolkar A, Ahmed A, Coates BM. Mechanisms and modulation of sepsis-induced immune dysfunction in children. Pediatr Res (2022) 91(2):447–53. doi: 10.1038/s41390-021-01879-8
26. Moriyama K, Nishida O. Targeting cytokines, pathogen-associated molecular patterns, and damage-associated molecular patterns in sepsis Via blood purification. Int J Mol Sci (2021) 22(16):8882. doi: 10.3390/ijms22168882
27. Takeuchi O, Akira S. Pattern recognition receptors and inflammation. Cell (2010) 140(6):805–20. doi: 10.1016/j.cell.2010.01.022
28. Chousterman BG, Swirski FK, Weber GF. Cytokine storm and sepsis disease pathogenesis. Semin immunopathology (2017) 39(5):517–28. doi: 10.1007/s00281-017-0639-8
29. Nakamori Y, Park EJ, Shimaoka M. Immune deregulation in sepsis and septic shock: Reversing immune paralysis by targeting pd-1/Pd-L1 pathway. Front Immunol (2020) 11:624279. doi: 10.3389/fimmu.2020.624279
30. Giamarellos-Bourboulis EJ, Raftogiannis M. The immune response to severe bacterial infections: Consequences for therapy. Expert Rev anti-infective Ther (2012) 10(3):369–80. doi: 10.1586/eri.12.2
31. Kumar V. Toll-like receptors in sepsis-associated cytokine storm and their endogenous negative regulators as future immunomodulatory targets. Int Immunopharmacol (2020) 89(Pt B):107087. doi: 10.1016/j.intimp.2020.107087
32. Van den Berghe G, Téblick A, Langouche L, Gunst J. The hypothalamus-Pituitary-Adrenal axis in sepsis- and hyperinflammation-induced critical illness: Gaps in current knowledge and future translational research directions. EBioMedicine (2022) 84:104284. doi: 10.1016/j.ebiom.2022.104284
33. Vincent JL, Mongkolpun W. Non-antibiotic therapies for sepsis: An update. Expert Rev anti-infective Ther (2019) 17(3):169–75. doi: 10.1080/14787210.2019.1581606
34. Shimabukuro-Vornhagen A, Gödel P, Subklewe M, Stemmler HJ, Schlößer HA, Schlaak M, et al. Cytokine release syndrome. J immunotherapy Cancer (2018) 6(1):56. doi: 10.1186/s40425-018-0343-9
35. Peters van Ton AM, Kox M, Abdo WF, Pickkers P. Precision immunotherapy for sepsis. Front Immunol (2018) 9:1926. doi: 10.3389/fimmu.2018.01926
36. Liu D, Huang SY, Sun JH, Zhang HC, Cai QL, Gao C, et al. Sepsis-induced immunosuppression: Mechanisms, diagnosis and current treatment options. Military Med Res (2022) 9(1):56. doi: 10.1186/s40779-022-00422-y
37. Danahy DB, Kurup SP, Winborn CS, Jensen IJ, Harty JT, Griffith TS, et al. Sepsis-induced state of immunoparalysis is defined by diminished CD8 T cell-mediated antitumor immunity. J Immunol (Baltimore Md 1950) (2019) 203(3):725–35. doi: 10.4049/jimmunol.1900435
38. McBride MA, Patil TK, Bohannon JK, Hernandez A, Sherwood ER, Patil NK. Immune checkpoints: Novel therapeutic targets to attenuate sepsis-induced immunosuppression. Front Immunol (2020) 11:624272. doi: 10.3389/fimmu.2020.624272
39. Patera AC, Drewry AM, Chang K, Beiter ER, Osborne D, Hotchkiss RS. Frontline science: Defects in immune function in patients with sepsis are associated with PD-1 or PD-L1 expression and can be restored by antibodies targeting PD-1 or PD-L1. J leukocyte Biol (2016) 100(6):1239–54. doi: 10.1189/jlb.4HI0616-255R
40. Hou H, Liu W, Wu S, Lu Y, Peng J, Zhu Y, et al. Tim-3 negatively mediates natural killer cell function in LPS-induced endotoxic shock. PLoS One (2014) 9(10):e110585. doi: 10.1371/journal.pone.0110585
41. Zhang W, Anyalebechi JC, Ramonell KM, Chen CW, Xie J, Liang Z, et al. Tigit modulates sepsis-induced immune dysregulation in mice with preexisting malignancy. JCI Insight (2021) 6(11):e139823. doi: 10.1172/jci.insight.139823
42. Neumann C, Scheffold A, Rutz S. Functions and regulation of T cell-derived interleukin-10. Semin Immunol (2019) 44:101344. doi: 10.1016/j.smim.2019.101344
43. Poujol F, Monneret G, Gallet-Gorius E, Pachot A, Textoris J, Venet F. Ex vivo stimulation of lymphocytes with IL-10 mimics sepsis-induced intrinsic T-cell alterations. Immunol investigations (2018) 47(2):154–68. doi: 10.1080/08820139.2017.1407786
44. Chen W, Frank ME, Jin W, Wahl SM. Tgf-beta released by apoptotic T cells contributes to an immunosuppressive milieu. Immunity (2001) 14(6):715–25. doi: 10.1016/s1074-7613(01)00147-9
45. Cavaillon JM, Adib-Conquy M. Determining the degree of immunodysregulation in sepsis. Contributions to Nephrol (2007) 156:101–11. doi: 10.1159/000102075
46. Mazer M, Unsinger J, Drewry A, Walton A, Osborne D, Blood T, et al. IL-10 has differential effects on the innate and adaptive immune systems of septic patients. J Immunol (Baltimore Md 1950) (2019) 203(8):2088–99. doi: 10.4049/jimmunol.1900637
47. Deng W, Zhu S, Zeng L, Liu J, Kang R, Yang M, et al. The circadian clock controls immune checkpoint pathway in sepsis. Cell Rep (2018) 24(2):366–78. doi: 10.1016/j.celrep.2018.06.026
48. Song GY, Chung CS, Chaudry IH, Ayala A. IL-4-Induced activation of the Stat6 pathway contributes to the suppression of cell-mediated immunity and death in sepsis. Surgery (2000) 128(2):133–8. doi: 10.1067/msy.2000.107282
49. Otto GP, Sossdorf M, Claus RA, Rödel J, Menge K, Reinhart K, et al. The late phase of sepsis is characterized by an increased microbiological burden and death rate. Crit Care (2011) 15(4):R183. doi: 10.1186/cc10332
50. Hamers L, Kox M, Pickkers P. Sepsis-induced immunoparalysis: Mechanisms, markers, and treatment options. Minerva anestesiologica (2015) 81(4):426–39.
51. Huang S, Liu D, Sun J, Zhang H, Zhang J, Wang Q, et al. Tim-3 regulates sepsis-induced immunosuppression by inhibiting the NF-κB signaling pathway in Cd4 T cells. Mol Ther J Am Soc Gene Ther (2022) 30(3):1227–38. doi: 10.1016/j.ymthe.2021.12.013
52. Leentjens J, Kox M, van der Hoeven JG, Netea MG, Pickkers P. Immunotherapy for the adjunctive treatment of sepsis: From immunosuppression to immunostimulation. Time Paradigm Change? Am J Respir Crit Care Med (2013) 187(12):1287–93. doi: 10.1164/rccm.201301-0036CP
53. Patil NK, Bohannon JK, Sherwood ER. Immunotherapy: A promising approach to reverse sepsis-induced immunosuppression. Pharmacol Res (2016) 111:688–702. doi: 10.1016/j.phrs.2016.07.019
54. Guo Y, Patil NK, Luan L, Bohannon JK, Sherwood ER. The biology of natural killer cells during sepsis. Immunology (2018) 153(2):190–202. doi: 10.1111/imm.12854
55. Peng H, Tian Z. Natural killer cell memory: Progress and implications. Front Immunol (2017) 8:1143. doi: 10.3389/fimmu.2017.01143
56. Sojka DK, Yang L, Yokoyama WM. Uterine natural killer cells. Front Immunol (2019) 10:960. doi: 10.3389/fimmu.2019.00960
57. Yokoyama WM, Sojka DK, Peng H, Tian Z. Tissue-resident natural killer cells. Cold Spring Harbor Symp quantitative Biol (2013) 78:149–56. doi: 10.1101/sqb.2013.78.020354
58. Sojka DK, Plougastel-Douglas B, Yang L, Pak-Wittel MA, Artyomov MN, Ivanova Y, et al. Tissue-resident natural killer (NK) cells are cell lineages distinct from thymic and conventional splenic nk cells. eLife (2014) 3:e01659. doi: 10.7554/eLife.01659
59. Carrega P, Ferlazzo G. Natural killer cell distribution and trafficking in human tissues. Front Immunol (2012) 3:347. doi: 10.3389/fimmu.2012.00347
60. Campbell KS, Hasegawa J. Natural killer cell biology: An update and future directions. J Allergy Clin Immunol (2013) 132(3):536–44. doi: 10.1016/j.jaci.2013.07.006
61. Adib Rad H, Basirat Z, Mostafazadeh A, Faramarzi M, Bijani A, Nouri HR, et al. Evaluation of peripheral blood NK cell subsets and cytokines in unexplained recurrent miscarriage. J Chin Med Assoc JCMA (2018) 81(12):1065–70. doi: 10.1016/j.jcma.2018.05.005
62. Euchner J, Sprissler J, Cathomen T, Fürst D, Schrezenmeier H, Debatin KM, et al. Natural killer cells generated from human induced pluripotent stem cells mature to CD56(Bright)CD16(+)Nkp80(+/-)in-Vitro and express Kir2dl2/Dl3 and Kir3dl1. Front Immunol (2021) 12:640672. doi: 10.3389/fimmu.2021.640672
63. Poli A, Michel T, Thérésine M, Andrès E, Hentges F, Zimmer J. Cd56bright natural killer (NK) cells: An important NK cell subset. Immunology (2009) 126(4):458–65. doi: 10.1111/j.1365-2567.2008.03027.x
64. Márquez ME, Millet C, Stekman H, Conesa A, Deglesne PA, Toro F, et al. CD16 cross-linking induces increased expression of Cd56 and production of IL-12 in peripheral NK cells. Cell Immunol (2010) 264(1):86–92. doi: 10.1016/j.cellimm.2010.05.002
65. Zamai L, Del Zotto G, Buccella F, Gabrielli S, Canonico B, Artico M, et al. Understanding the synergy of Nkp46 and Co-activating signals in various NK cell subpopulations: Paving the way for more successful NK-Cell-Based immunotherapy. Cells (2020) 9(3):753. doi: 10.3390/cells9030753
66. Lysakova-Devine T, O'Farrelly C. Tissue-specific NK cell populations and their origin. J leukocyte Biol (2014) 96(6):981–90. doi: 10.1189/jlb.1RU0514-241R
67. Perera Molligoda Arachchige AS. Human NK cells: From development to effector functions. Innate Immun (2021) 27(3):212–29. doi: 10.1177/17534259211001512
68. Cooper MA, Fehniger TA, Turner SC, Chen KS, Ghaheri BA, Ghayur T, et al. Human natural killer cells: A unique innate immunoregulatory role for the CD56(Bright) subset. Blood (2001) 97(10):3146–51. doi: 10.1182/blood.v97.10.3146
69. Sivori S, Vacca P, Del Zotto G, Munari E, Mingari MC, Moretta L. Human NK cells: Surface receptors, inhibitory checkpoints, and translational applications. Cell Mol Immunol (2019) 16(5):430–41. doi: 10.1038/s41423-019-0206-4
70. Sun C, Sun H, Zhang C, Tian Z. Nk cell receptor imbalance and NK cell dysfunction in hbv infection and hepatocellular carcinoma. Cell Mol Immunol (2015) 12(3):292–302. doi: 10.1038/cmi.2014.91
71. Chen Y, Lu D, Churov A, Fu R. Research progress on NK cell receptors and their signaling pathways. Mediators Inflammation (2020) 2020:6437057. doi: 10.1155/2020/6437057
72. Ma M, Wang Z, Chen X, Tao A, He L, Fu S, et al. NKG2C(+)NKG2A(-) natural killer cells are associated with a lower viral set point and may predict disease progression in individuals with primary hiv infection. Front Immunol (2017) 8:1176. doi: 10.3389/fimmu.2017.01176
73. Wicherska-Pawłowska K, Wróbel T, Rybka J. Toll-like receptors (TLRs), nod-like receptors (NLRs), and rig-I-Like receptors (RLRs) in innate immunity. TLRs, NLRs, and RLRs ligands as immunotherapeutic agents for hematopoietic diseases. Int J Mol Sci (2021) 22(24):13397. doi: 10.3390/ijms222413397
74. O'Neill LA. The interleukin-1 Receptor/Toll-like receptor superfamily: 10 years of progress. Immunol Rev (2008) 226:10–8. doi: 10.1111/j.1600-065X.2008.00701.x
75. Qiu Y, Yang J, Wang W, Zhao W, Peng F, Xiang Y, et al. Hmgb1-promoted and TLR2/4-dependent NK cell maturation and activation take part in rotavirus-induced murine biliary atresia. PLoS Pathog (2014) 10(3):e1004011. doi: 10.1371/journal.ppat.1004011
76. Strengell M, Matikainen S, Sirén J, Lehtonen A, Foster D, Julkunen I, et al. IL-21 in synergy with IL-15 or IL-18 enhances IFN-gamma production in human NK and T cells. J Immunol (Baltimore Md 1950) (2003) 170(11):5464–9. doi: 10.4049/jimmunol.170.11.5464
77. Patil NK, Luan L, Bohannon JK, Guo Y, Hernandez A, Fensterheim B, et al. IL-15 superagonist expands Mcd8+ T, NK and NKT cells after burn injury but fails to improve outcome during burn wound infection. PLoS One (2016) 11(2):e0148452. doi: 10.1371/journal.pone.0148452
78. Deuse T, Hu X, Agbor-Enoh S, Jang MK, Alawi M, Saygi C, et al. The sirpα-CD47 immune checkpoint in NK cells. J Exp Med (2021) 218(3):e20200839. doi: 10.1084/jem.20200839
79. Mahmoudzadeh S, Nozad Charoudeh H, Marques CS, Bahadory S, Ahmadpour E. The role of IL-12 in stimulating NK cells against toxoplasma gondii infection: A mini-review. Parasitol Res (2021) 120(7):2303–9. doi: 10.1007/s00436-021-07204-w
80. Choi YH, Lim EJ, Kim SW, Moon YW, Park KS, An HJ. IL-27 enhances IL-15/IL-18-Mediated activation of human natural killer cells. J immunotherapy Cancer (2019) 7(1):168. doi: 10.1186/s40425-019-0652-7
81. Vignali DA, Kuchroo VK. IL-12 family cytokines: Immunological playmakers. Nat Immunol (2012) 13(8):722–8. doi: 10.1038/ni.2366
82. Prager I, Watzl C. Mechanisms of natural killer cell-mediated cellular cytotoxicity. J leukocyte Biol (2019) 105(6):1319–29. doi: 10.1002/jlb.Mr0718-269r
83. Doss M, White MR, Tecle T, Hartshorn KL. Human defensins and ll-37 in mucosal immunity. J leukocyte Biol (2010) 87(1):79–92. doi: 10.1189/jlb.0609382
84. Ramírez-Labrada A, Pesini C, Santiago L, Hidalgo S, Calvo-Pérez A, Oñate C, et al. All about (NK cell-mediated) death in two acts and an unexpected encore: Initiation, execution and activation of adaptive immunity. Front Immunol (2022) 13:896228. doi: 10.3389/fimmu.2022.896228
85. Fehniger TA, Cai SF, Cao X, Bredemeyer AJ, Presti RM, French AR, et al. Acquisition of murine NK cell cytotoxicity requires the translation of a pre-existing pool of granzyme b and perforin mrnas. Immunity (2007) 26(6):798–811. doi: 10.1016/j.immuni.2007.04.010
86. Ernst WA, Thoma-Uszynski S, Teitelbaum R, Ko C, Hanson DA, Clayberger C, et al. Granulysin, a T cell product, kills bacteria by altering membrane permeability. J Immunol (Baltimore Md 1950) (2000) 165(12):7102–8. doi: 10.4049/jimmunol.165.12.7102
87. Endsley JJ, Torres AG, Gonzales CM, Kosykh VG, Motin VL, Peterson JW, et al. Comparative antimicrobial activity of granulysin against bacterial biothreat agents. Open Microbiol J (2009) 3:92–6. doi: 10.2174/1874285800903010092
88. Gonzales CM, Williams CB, Calderon VE, Huante MB, Moen ST, Popov VL, et al. Antibacterial role for natural killer cells in host defense to bacillus anthracis. Infection Immun (2012) 80(1):234–42. doi: 10.1128/iai.05439-11
89. Lu CC, Wu TS, Hsu YJ, Chang CJ, Lin CS, Chia JH, et al. NK cells kill mycobacteria directly by releasing perforin and granulysin. J leukocyte Biol (2014) 96(6):1119–29. doi: 10.1189/jlb.4A0713-363RR
90. Paolini R, Bernardini G, Molfetta R, Santoni A. NK cells and interferons. Cytokine Growth factor Rev (2015) 26(2):113–20. doi: 10.1016/j.cytogfr.2014.11.003
91. Horowitz A, Stegmann KA, Riley EM. Activation of natural killer cells during microbial infections. Front Immunol (2011) 2:88. doi: 10.3389/fimmu.2011.00088
92. Elemam NM, Ramakrishnan RK, Hundt JE, Halwani R, Maghazachi AA, Hamid Q. Innate lymphoid cells and natural killer cells in bacterial infections: Function, dysregulation, and therapeutic targets. Front Cell infection Microbiol (2021) 11:733564. doi: 10.3389/fcimb.2021.733564
93. Spörri R, Joller N, Albers U, Hilbi H, Oxenius A. Myd88-dependent IFN-gamma production by NK cells is key for control of legionella pneumophila infection. J Immunol (Baltimore Md 1950) (2006) 176(10):6162–71. doi: 10.4049/jimmunol.176.10.6162
94. Dahl CA, Schall RP, He HL, Cairns JS. Identification of a novel gene expressed in activated natural killer cells and T cells. J Immunol (Baltimore Md 1950) (1992) 148(2):597–603.
95. Park MH, Song MJ, Cho MC, Moon DC, Yoon DY, Han SB, et al. Interleukin-32 enhances cytotoxic effect of natural killer cells to cancer cells Via activation of death receptor 3. Immunology (2012) 135(1):63–72. doi: 10.1111/j.1365-2567.2011.03513.x
96. Khawar B, Abbasi MH, Sheikh N. A panoramic spectrum of complex interplay between the immune system and IL-32 during pathogenesis of various systemic infections and inflammation. Eur J Med Res (2015) 20(1):7. doi: 10.1186/s40001-015-0083-y
97. Kobayashi H, Huang J, Ye F, Shyr Y, Blackwell TS, Lin PC. Interleukin-32beta propagates vascular inflammation and exacerbates sepsis in a mouse model. PLoS One (2010) 5(3):e9458. doi: 10.1371/journal.pone.0009458
98. Kim EY, Ner-Gaon H, Varon J, Cullen AM, Guo J, Choi J, et al. Post-sepsis immunosuppression depends on NKT cell regulation of Mtor/IFN-γ in NK cells. J Clin Invest (2020) 130(6):3238–52. doi: 10.1172/jci128075
99. Perona-Wright G, Mohrs K, Szaba FM, Kummer LW, Madan R, Karp CL, et al. Systemic but not local infections elicit immunosuppressive IL-10 production by natural killer cells. Cell Host Microbe (2009) 6(6):503–12. doi: 10.1016/j.chom.2009.11.003
100. Yang HL, Zhou WJ, Chang KK, Mei J, Huang LQ, Wang MY, et al. The crosstalk between endometrial stromal cells and macrophages impairs cytotoxicity of NK cells in endometriosis by secreting IL-10 and TGF-β. Reprod (Cambridge England) (2017) 154(6):815–25. doi: 10.1530/rep-17-0342
101. Jiang Y, Yang M, Sun X, Chen X, Ma M, Yin X, et al. IL-10(+) NK and TGF-β(+) NK cells play negative regulatory roles in HIV infection. BMC Infect Dis (2018) 18(1):80. doi: 10.1186/s12879-018-2991-2
102. Clark SE, Filak HC, Guthrie BS, Schmidt RL, Jamieson A, Merkel P, et al. Bacterial manipulation of NK cell regulatory activity increases susceptibility to listeria monocytogenes infection. PLoS Pathog (2016) 12(6):e1005708. doi: 10.1371/journal.ppat.1005708
103. Ali AK, Komal AK, Almutairi SM, Lee SH. Natural killer cell-derived IL-10 prevents liver damage during sustained murine cytomegalovirus infection. Front Immunol (2019) 10:2688. doi: 10.3389/fimmu.2019.02688
104. Jensen IJ, McGonagill PW, Butler NS, Harty JT, Griffith TS, Badovinac VP. NK cell-derived IL-10 supports host survival during sepsis. J Immunol (Baltimore Md 1950) (2021) 206(6):1171–80. doi: 10.4049/jimmunol.2001131
105. Highton AJ, Schuster IS, Degli-Esposti MA, Altfeld M. The role of natural killer cells in liver inflammation. Semin immunopathology (2021) 43(4):519–33. doi: 10.1007/s00281-021-00877-6
106. Zitti B, Bryceson YT. Natural killer cells in inflammation and autoimmunity. Cytokine Growth factor Rev (2018) 42:37–46. doi: 10.1016/j.cytogfr.2018.08.001
107. Liu M, Liang S, Zhang C. Nk cells in autoimmune diseases: Protective or pathogenic? Front Immunol (2021) 12:624687. doi: 10.3389/fimmu.2021.624687
108. Newman KC, Korbel DS, Hafalla JC, Riley EM. Cross-talk with myeloid accessory cells regulates human natural killer cell interferon-gamma responses to malaria. PLoS Pathog (2006) 2(12):e118. doi: 10.1371/journal.ppat.0020118
109. Nomura T, Kawamura I, Tsuchiya K, Kohda C, Baba H, Ito Y, et al. Essential role of interleukin-12 (IL-12) and IL-18 for gamma interferon production induced by listeriolysin O in mouse spleen cells. Infection Immun (2002) 70(3):1049–55. doi: 10.1128/iai.70.3.1049-1055.2002
110. Enoh VT, Fairchild CD, Lin CY, Varma TK, Sherwood ER. Differential effect of imipenem treatment on wild-type and NK cell-deficient CD8 knockout mice during acute intra-abdominal injury. Am J Physiol Regulatory Integr Comp Physiol (2006) 290(3):R685–93. doi: 10.1152/ajpregu.00678.2005
111. Sherwood ER, Enoh VT, Murphey ED, Lin CY. Mice depleted of CD8+ T and NK cells are resistant to injury caused by cecal ligation and puncture. Lab investigation; J Tech Methods Pathol (2004) 84(12):1655–65. doi: 10.1038/labinvest.3700184
112. Sherwood ER, Lin CY, Tao W, Hartmann CA, Dujon JE, French AJ, et al. Beta 2 microglobulin knockout mice are resistant to lethal intraabdominal sepsis. Am J Respir Crit Care Med (2003) 167(12):1641–9. doi: 10.1164/rccm.200208-950OC
113. Tao W, Sherwood ER. Beta2-microglobulin knockout mice treated with anti-Asialogm1 exhibit improved hemodynamics and cardiac contractile function during acute intra-abdominal sepsis. Am J Physiol Regulatory Integr Comp Physiol (2004) 286(3):R569–75. doi: 10.1152/ajpregu.00470.2003
114. Badgwell B, Parihar R, Magro C, Dierksheide J, Russo T, Carson WE 3rd. Natural killer cells contribute to the lethality of a murine model of escherichia coli infection. Surgery (2002) 132(2):205–12. doi: 10.1067/msy.2002.125311
115. Goldmann O, Chhatwal GS, Medina E. Contribution of natural killer cells to the pathogenesis of septic shock induced by streptococcus pyogenes in mice. J Infect Dis (2005) 191(8):1280–6. doi: 10.1086/428501
116. Christaki E, Diza E, Giamarellos-Bourboulis EJ, Papadopoulou N, Pistiki A, Droggiti DI, et al. NK and NKT cell depletion alters the outcome of experimental pneumococcal pneumonia: Relationship with regulation of interferon-Γ production. J Immunol Res (2015) 2015:532717. doi: 10.1155/2015/532717
117. Barkhausen T, Frerker C, Pütz C, Pape HC, Krettek C, van Griensven M. Depletion of NK cells in a murine polytrauma model is associated with improved outcome and a modulation of the inflammatory response. Shock (Augusta Ga) (2008) 30(4):401–10. doi: 10.1097/SHK.0b013e31816e2cda
118. Zhang S, Zhao J, Bai X, Handley M, Shan F. Biological effects of IL-15 on immune cells and its potential for the treatment of cancer. Int Immunopharmacol (2021) 91:107318. doi: 10.1016/j.intimp.2020.107318
119. Guo Y, Luan L, Rabacal W, Bohannon JK, Fensterheim BA, Hernandez A, et al. IL-15 superagonist-mediated immunotoxicity: Role of NK cells and IFN-γ. J Immunol (Baltimore Md 1950) (2015) 195(5):2353–64. doi: 10.4049/jimmunol.1500300
120. Guo Y, Luan L, Patil NK, Wang J, Bohannon JK, Rabacal W, et al. IL-15 enables septic shock by maintaining NK cell integrity and function. J Immunol (Baltimore Md 1950) (2017) 198(3):1320–33. doi: 10.4049/jimmunol.1601486
121. Böning MAL, Trittel S, Riese P, van Ham M, Heyner M, Voss M, et al. Adap promotes degranulation and migration of NK cells primed during in vivo listeria monocytogenes infection in mice. Front Immunol (2019) 10:3144. doi: 10.3389/fimmu.2019.03144
122. Castriconi R, Carrega P, Dondero A, Bellora F, Casu B, Regis S, et al. Molecular mechanisms directing migration and retention of natural killer cells in human tissues. Front Immunol (2018) 9:2324. doi: 10.3389/fimmu.2018.02324
123. Herzig DS, Driver BR, Fang G, Toliver-Kinsky TE, Shute EN, Sherwood ER. Regulation of lymphocyte trafficking by CXC chemokine receptor 3 during septic shock. Am J Respir Crit Care Med (2012) 185(3):291–300. doi: 10.1164/rccm.201108-1560OC
124. Marquardt N, Wilk E, Pokoyski C, Schmidt RE, Jacobs R. Murine CXCR3+CD27bright NK cells resemble the human CD56bright NK-cell population. Eur J Immunol (2010) 40(5):1428–39. doi: 10.1002/eji.200940056
125. Herzig DS, Guo Y, Fang G, Toliver-Kinsky TE, Sherwood ER. Therapeutic efficacy of CXCR3 blockade in an experimental model of severe sepsis. Crit Care (2012) 16(5):R168. doi: 10.1186/cc11642
126. Anthony DA, Andrews DM, Chow M, Watt SV, House C, Akira S, et al. A role for granzyme m in TLR4-driven inflammation and endotoxicosis. J Immunol (Baltimore Md 1950) (2010) 185(3):1794–803. doi: 10.4049/jimmunol.1000430
127. Andaluz-Ojeda D, Iglesias V, Bobillo F, Almansa R, Rico L, Gandía F, et al. Early natural killer cell counts in blood predict mortality in severe sepsis. Crit Care (2011) 15(5):R243. doi: 10.1186/cc10501
128. de Pablo R, Monserrat J, Torrijos C, Martín M, Prieto A, Alvarez-Mon M. The predictive role of early activation of natural killer cells in septic shock. Crit Care (2012) 16(2):413. doi: 10.1186/cc11204
129. Nilsson N, Bremell T, Tarkowski A, Carlsten H. Protective role of NK1.1+ cells in experimental staphylococcus aureus arthritis. Clin Exp Immunol (1999) 117(1):63–9. doi: 10.1046/j.1365-2249.1999.00922.x
130. Small CL, McCormick S, Gill N, Kugathasan K, Santosuosso M, Donaldson N, et al. NK cells play a critical protective role in host defense against acute extracellular staphylococcus aureus bacterial infection in the lung. J Immunol (Baltimore Md 1950) (2008) 180(8):5558–68. doi: 10.4049/jimmunol.180.8.5558
131. Hiraki S, Ono S, Kinoshita M, Tsujimoto H, Takahata R, Miyazaki H, et al. Neutralization of IL-10 restores the downregulation of IL-18 receptor on natural killer cells and interferon-γ production in septic mice, thus leading to an improved survival. Shock (Augusta Ga) (2012) 37(2):177–82. doi: 10.1097/SHK.0b013e31823f18ad
132. Hall LJ, Murphy CT, Hurley G, Quinlan A, Shanahan F, Nally K, et al. Natural killer cells protect against mucosal and systemic infection with the enteric pathogen citrobacter rodentium. Infection Immun (2013) 81(2):460–9. doi: 10.1128/iai.00953-12
133. Pastille E, Pohlmann S, Wirsdörfer F, Reib A, Flohé SB. A disturbed interaction with accessory cells upon opportunistic infection with pseudomonas aeruginosa contributes to an impaired IFN-γ production of NK cells in the lung during sepsis-induced immunosuppression. Innate Immun (2015) 21(2):115–26. doi: 10.1177/1753425913517274
134. Lai HC, Chang CJ, Lin CS, Wu TR, Hsu YJ, Wu TS, et al. NK cell-derived IFN-γ protects against nontuberculous mycobacterial lung infection. J Immunol (Baltimore Md 1950) (2018) 201(5):1478–90. doi: 10.4049/jimmunol.1800123
135. Inoue S, Unsinger J, Davis CG, Muenzer JT, Ferguson TA, Chang K, et al. IL-15 prevents apoptosis, reverses innate and adaptive immune dysfunction, and improves survival in sepsis. J Immunol (Baltimore Md 1950) (2010) 184(3):1401–9. doi: 10.4049/jimmunol.0902307
136. Zhao X, Qi H, Zhou J, Xu S, Gao Y. Treatment with recombinant interleukin-15 (IL-15) increases the number of T cells and natural killer (NK) cells and levels of interferon-γ (IFN-γ) in a rat model of sepsis. Med Sci monitor Int Med J Exp Clin Res (2019) 25:4450–6. doi: 10.12659/msm.914026
137. Gogos C, Kotsaki A, Pelekanou A, Giannikopoulos G, Vaki I, Maravitsa P, et al. Early alterations of the innate and adaptive immune statuses in sepsis according to the type of underlying infection. Crit Care (2010) 14(3):R96. doi: 10.1186/cc9031
138. Giannikopoulos G, Antonopoulou A, Kalpakou G, Makaritsis K, Panou C, Papadomichelakis E, et al. The functional role of natural killer cells early in clinical sepsis. APMIS Acta pathologica microbiologica immunologica Scandinavica (2013) 121(4):329–36. doi: 10.1111/apm.12002
139. Garzón-Tituaña M, Sierra-Monzón JL, Comas L, Santiago L, Khaliulina-Ushakova T, Uranga-Murillo I, et al. Granzyme a inhibition reduces inflammation and increases survival during abdominal sepsis. Theranostics (2021) 11(8):3781–95. doi: 10.7150/thno.49288
140. Giamarellos-Bourboulis EJ, Tsaganos T, Spyridaki E, Mouktaroudi M, Plachouras D, Vaki I, et al. Early changes of CD4-positive lymphocytes and NK cells in patients with severe gram-negative sepsis. Crit Care (2006) 10(6):R166. doi: 10.1186/cc5111
141. Boomer JS, Shuherk-Shaffer J, Hotchkiss RS, Green JM. A prospective analysis of lymphocyte phenotype and function over the course of acute sepsis. Crit Care (2012) 16(3):R112. doi: 10.1186/cc11404
142. Holub M, Klucková Z, Beneda B, Hobstová J, Huzicka I, Prazák J, et al. Changes in lymphocyte subpopulations and CD3+/Dr+ expression in sepsis. Clin Microbiol infection Off Publ Eur Soc Clin Microbiol Infect Dis (2000) 6(12):657–60. doi: 10.1046/j.1469-0691.2000.00175.x
143. Darden DB, Dong X, Brusko MA, Kelly L, Fenner B, Rincon JC, et al. A novel single cell rna-seq analysis of non-myeloid circulating cells in late sepsis. Front Immunol (2021) 12:696536. doi: 10.3389/fimmu.2021.696536
144. Huang C, Wang Y, Li X, Ren L, Zhao J, Hu Y, et al. Clinical features of patients infected with 2019 novel coronavirus in wuhan, China. Lancet (London England) (2020) 395(10223):497–506. doi: 10.1016/s0140-6736(20)30183-5
145. Guan WJ, Ni ZY, Hu Y, Liang WH, Ou CQ, He JX, et al. Clinical characteristics of coronavirus disease 2019 in China. New Engl J Med (2020) 382(18):1708–20. doi: 10.1056/NEJMoa2002032
146. Karakike E, Giamarellos-Bourboulis EJ, Kyprianou M, Fleischmann-Struzek C, Pletz MW, Netea MG, et al. Coronavirus disease 2019 as cause of viral sepsis: A systematic review and meta-analysis. Crit Care Med (2021) 49(12):2042–57. doi: 10.1097/CCM.0000000000005195
147. Wilk AJ, Rustagi A, Zhao NQ, Roque J, Martínez-Colón GJ, McKechnie JL, et al. A single-cell atlas of the peripheral immune response in patients with severe COVID-19. Nat Med (2020) 26(7):1070–6. doi: 10.1038/s41591-020-0944-y
148. Jiang Y, Wei X, Guan J, Qin S, Wang Z, Lu H, et al. Covid-19 pneumonia: CD8(+) T and NK cells are decreased in number but compensatory increased in cytotoxic potential. Clin Immunol (Orlando Fla) (2020) 218:108516. doi: 10.1016/j.clim.2020.108516
149. Huang W, Li M, Luo G, Wu X, Su B, Zhao L, et al. The inflammatory factors associated with disease severity to predict COVID-19 progression. J Immunol (Baltimore Md 1950) (2021) 206(7):1597–608. doi: 10.4049/jimmunol.2001327
150. Liao M, Liu Y, Yuan J, Wen Y, Xu G, Zhao J, et al. Single-cell landscape of bronchoalveolar immune cells in patients with COVID-19. Nat Med (2020) 26(6):842–4. doi: 10.1038/s41591-020-0901-9
151. Carsetti R, Zaffina S, Piano Mortari E, Terreri S, Corrente F, Capponi C, et al. Different innate and adaptive immune responses to sars-Cov-2 infection of asymptomatic, mild, and severe cases. Front Immunol (2020) 11:610300. doi: 10.3389/fimmu.2020.610300
152. Koutsakos M, Rowntree LC, Hensen L, Chua BY, van de Sandt CE, Habel JR, et al. Integrated immune dynamics define correlates of COVID-19 severity and antibody responses. Cell Rep Med (2021) 2(3):100208. doi: 10.1016/j.xcrm.2021.100208
153. Liu C, Martins AJ, Lau WW, Rachmaninoff N, Chen J, Imberti L, et al. Time-resolved systems immunology reveals a late juncture linked to fatal COVID-19. Cell (2021) 184(7):1836–57.e22. doi: 10.1016/j.cell.2021.02.018
154. Sahoo D, Katkar GD, Khandelwal S, Behroozikhah M, Claire A, Castillo V, et al. Ai-guided discovery of the invariant host response to viral pandemics. EBioMedicine (2021) 68:103390. doi: 10.1016/j.ebiom.2021.103390
155. Maucourant C, Filipovic I, Ponzetta A, Aleman S, Cornillet M, Hertwig L, et al. Natural killer cell immunotypes related to COVID-19 disease severity. Sci Immunol (2020) 5(50):eabd6832. doi: 10.1126/sciimmunol.abd6832
156. Yao C, Bora SA, Parimon T, Zaman T, Friedman OA, Palatinus JA, et al. Cell-Type-Specific immune dysregulation in severely ill COVID-19 patients. Cell Rep (2021) 34(1):108590. doi: 10.1016/j.celrep.2020.108590
157. Zheng M, Gao Y, Wang G, Song G, Liu S, Sun D, et al. Functional exhaustion of antiviral lymphocytes in COVID-19 patients. Cell Mol Immunol (2020) 17(5):533–5. doi: 10.1038/s41423-020-0402-2
158. Yaqinuddin A, Kashir J. Innate immunity in covid-19 patients mediated by NKG2A receptors, and potential treatment using monalizumab, cholroquine, and antiviral agents. Med Hypotheses (2020) 140:109777. doi: 10.1016/j.mehy.2020.109777
159. Nguyen R, Wu H, Pounds S, Inaba H, Ribeiro RC, Cullins D, et al. A phase II clinical trial of adoptive transfer of haploidentical natural killer cells for consolidation therapy of pediatric acute myeloid leukemia. J immunotherapy Cancer (2019) 7(1):81. doi: 10.1186/s40425-019-0564-6
160. Dolstra H, Roeven MWH, Spanholtz J, Hangalapura BN, Tordoir M, Maas F, et al. Successful transfer of umbilical cord blood CD34(+) hematopoietic stem and progenitor-derived NK cells in older acute myeloid leukemia patients. Clin Cancer Res an Off J Am Assoc Cancer Res (2017) 23(15):4107–18. doi: 10.1158/1078-0432.Ccr-16-2981
161. Bachanova V, Sarhan D, DeFor TE, Cooley S, Panoskaltsis-Mortari A, Blazar BR, et al. Haploidentical natural killer cells induce remissions in non-Hodgkin lymphoma patients with low levels of immune-suppressor cells. Cancer immunology immunotherapy CII (2018) 67(3):483–94. doi: 10.1007/s00262-017-2100-1
162. Modak S, Le Luduec JB, Cheung IY, Goldman DA, Ostrovnaya I, Doubrovina E, et al. Adoptive immunotherapy with haploidentical natural killer cells and anti-Gd2 monoclonal antibody M3f8 for resistant neuroblastoma: Results of a phase I study. Oncoimmunology (2018) 7(8):e1461305. doi: 10.1080/2162402x.2018.1461305
163. Szmania S, Lapteva N, Garg T, Greenway A, Lingo J, Nair B, et al. Ex vivo-expanded natural killer cells demonstrate robust proliferation in vivo in high-risk relapsed multiple myeloma patients. J immunotherapy (Hagerstown Md 1997) (2015) 38(1):24–36. doi: 10.1097/cji.0000000000000059
164. Liu E, Marin D, Banerjee P, Macapinlac HA, Thompson P, Basar R, et al. Use of CAR-transduced natural killer cells in CD19-positive lymphoid tumors. New Engl J Med (2020) 382(6):545–53. doi: 10.1056/NEJMoa1910607
165. Gras Navarro A, Kmiecik J, Leiss L, Zelkowski M, Engelsen A, Bruserud Ø, et al. NK cells with Kir2ds2 immunogenotype have a functional activation advantage to efficiently kill glioblastoma and prolong animal survival. J Immunol (Baltimore Md 1950) (2014) 193(12):6192–206. doi: 10.4049/jimmunol.1400859
166. Lee SJ, Kang WY, Yoon Y, Jin JY, Song HJ, Her JH, et al. Natural killer (NK) cells inhibit systemic metastasis of glioblastoma cells and have therapeutic effects against glioblastomas in the brain. BMC Cancer (2015) 15:1011. doi: 10.1186/s12885-015-2034-y
167. Geller MA, Knorr DA, Hermanson DA, Pribyl L, Bendzick L, McCullar V, et al. Intraperitoneal delivery of human natural killer cells for treatment of ovarian cancer in a mouse xenograft model. Cytotherapy (2013) 15(10):1297–306. doi: 10.1016/j.jcyt.2013.05.022
168. Veluchamy JP, Lopez-Lastra S, Spanholtz J, Bohme F, Kok N, Heideman DA, et al. In vivo efficacy of umbilical cord blood stem cell-derived NK cells in the treatment of metastatic colorectal cancer. Front Immunol (2017) 8:87. doi: 10.3389/fimmu.2017.00087
169. Myers JA, Miller JS. Exploring the NK cell platform for cancer immunotherapy. Nat Rev Clin Oncol (2021) 18(2):85–100. doi: 10.1038/s41571-020-0426-7
170. Parkhurst MR, Riley JP, Dudley ME, Rosenberg SA. Adoptive transfer of autologous natural killer cells leads to high levels of circulating natural killer cells but does not mediate tumor regression. Clin Cancer Res an Off J Am Assoc Cancer Res (2011) 17(19):6287–97. doi: 10.1158/1078-0432.Ccr-11-1347
171. Veluchamy JP, Kok N, van der Vliet HJ, Verheul HMW, de Gruijl TD, Spanholtz J. The rise of allogeneic natural killer cells as a platform for cancer immunotherapy: Recent innovations and future developments. Front Immunol (2017) 8:631. doi: 10.3389/fimmu.2017.00631
172. Heipertz EL, Zynda ER, Stav-Noraas TE, Hungler AD, Boucher SE, Kaur N, et al. Current perspectives on "Off-the-Shelf" allogeneic nk and CAR-NK cell therapies. Front Immunol (2021) 12:732135. doi: 10.3389/fimmu.2021.732135
173. Verneris MR, Miller JS. The phenotypic and functional characteristics of umbilical cord blood and peripheral blood natural killer cells. Br J haematology (2009) 147(2):185–91. doi: 10.1111/j.1365-2141.2009.07768.x
174. Karagiannis P, Kim SI. Ipsc-derived natural killer cells for cancer immunotherapy. Molecules Cells (2021) 44(8):541–8. doi: 10.14348/molcells.2021.0078
175. Herrera L, Santos S, Vesga MA, Anguita J, Martin-Ruiz I, Carrascosa T, et al. Adult peripheral blood and umbilical cord blood nk cells are good sources for effective car therapy against CD19 positive leukemic cells. Sci Rep (2019) 9(1):18729. doi: 10.1038/s41598-019-55239-y
176. Kundu S, Gurney M, O'Dwyer M. Generating natural killer cells for adoptive transfer: Expanding horizons. Cytotherapy (2021) 23(7):559–66. doi: 10.1016/j.jcyt.2020.12.002
177. Condiotti R, Zakai YB, Barak V, Nagler A. Ex vivo expansion of CD56+ cytotoxic cells from human umbilical cord blood. Exp Hematol (2001) 29(1):104–13. doi: 10.1016/s0301-472x(00)00617-2
178. Zhu H, Kaufman DS. An improved method to produce clinical-scale natural killer cells from human pluripotent stem cells. Methods Mol Biol (Clifton NJ) (2019) 2048:107–19. doi: 10.1007/978-1-4939-9728-2_12
179. Woll PS, Grzywacz B, Tian X, Marcus RK, Knorr DA, Verneris MR, et al. Human embryonic stem cells differentiate into a homogeneous population of natural killer cells with potent in vivo antitumor activity. Blood (2009) 113(24):6094–101. doi: 10.1182/blood-2008-06-165225
180. Ames E, Murphy WJ. Advantages and clinical applications of natural killer cells in cancer immunotherapy. Cancer immunology immunotherapy CII (2014) 63(1):21–8. doi: 10.1007/s00262-013-1469-8
181. Caruso S, De Angelis B, Carlomagno S, Del Bufalo F, Sivori S, Locatelli F, et al. NK cells as adoptive cellular therapy for hematological malignancies: Advantages and hurdles. Semin Hematol (2020) 57(4):175–84. doi: 10.1053/j.seminhematol.2020.10.004
Keywords: natural killer cells, sepsis, immunotherapy, protective effect, detrimental effect
Citation: Wang F, Cui Y, He D, Gong L and Liang H (2023) Natural killer cells in sepsis: Friends or foes? Front. Immunol. 14:1101918. doi: 10.3389/fimmu.2023.1101918
Received: 18 November 2022; Accepted: 09 January 2023;
Published: 26 January 2023.
Edited by:
Luc Van Kaer, Vanderbilt University Medical Center, United StatesReviewed by:
Youcai Deng, Army Medical University, ChinaSeokmann Hong, Sejong University, Republic of Korea
Lan Wu, Vanderbilt University Medical Center, United States
Copyright © 2023 Wang, Cui, He, Gong and Liang. This is an open-access article distributed under the terms of the Creative Commons Attribution License (CC BY). The use, distribution or reproduction in other forums is permitted, provided the original author(s) and the copyright owner(s) are credited and that the original publication in this journal is cited, in accordance with accepted academic practice. No use, distribution or reproduction is permitted which does not comply with these terms.
*Correspondence: Huaping Liang, MTM2MzgzNTY3MjhAMTYzLmNvbQ==
†These authors have contributed equally to this work