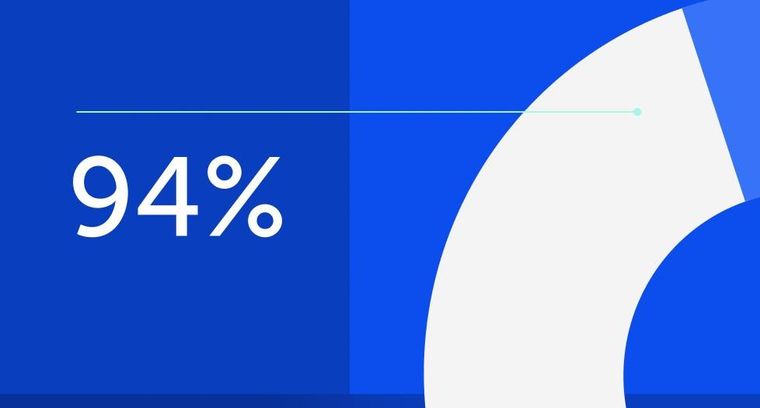
94% of researchers rate our articles as excellent or good
Learn more about the work of our research integrity team to safeguard the quality of each article we publish.
Find out more
ORIGINAL RESEARCH article
Front. Immunol., 24 January 2023
Sec. Vaccines and Molecular Therapeutics
Volume 14 - 2023 | https://doi.org/10.3389/fimmu.2023.1075291
Synthetic double-stranded RNA analogs recognized by Toll-like receptor 3 (TLR3) are an attractive adjuvant candidate for vaccines, especially against intracellular pathogens or tumors, because of their ability to enhance T cell and antibody responses. Although poly(I:C) is a representative dsRNA with potent adjuvanticity, its clinical application has been limited due to heterogeneous molecular size, inconsistent activity, poor stability, and toxicity. To overcome these limitations, we developed a novel dsRNA-based TLR3 agonist named NexaVant (NVT) by using PCR-coupled bidirectional in vitro transcription. Agarose gel electrophoresis and reverse phase-HPLC analysis demonstrated that NVT is a single 275-kDa homogeneous molecule. NVT appears to be stable since its appearance, concentration, and molecular size were unaffected under 6 months of accelerated storage conditions. Moreover, preclinical evaluation of toxicity under good laboratory practices showed that NVT is a safe substance without any signs of serious toxicity. NVT stimulated TLR3 and increased the expression of viral nucleic acid sensors TLR3, MDA-5, and RIG-1. When intramuscularly injected into C57BL/6 mice, ovalbumin (OVA) plus NVT highly increased the migration of dendritic cells (DCs), macrophages, and neutrophils into inguinal lymph node (iLN) compared with OVA alone. In addition, NVT substantially induced the phenotypic markers of DC maturation and activation including MHC-II, CD40, CD80, and CD86 together with IFN-β production. Furthermore, NVT exhibited an appropriate adjuvanticity because it elevated OVA-specific IgG, in particular, higher levels of IgG2c (Th1-type) but lower IgG1 (Th2-type). Concomitantly, NVT increased the levels of Th1-type T cells such as IFN-γ+CD4+ and IFN-γ+CD8+ cells in response to OVA stimulation. Collectively, we suggest that NVT with appropriate safety and effectiveness is a novel and promising adjuvant for vaccines, especially those requiring T cell mediated immunity such as viral and cancer vaccines.
Vaccine adjuvants are substances that improve the efficacy of vaccines by helping induce strong protective immune responses to vaccine antigens in humans or animals (1). Adjuvants can be used to (i) maximize the immune responses to vaccines, (ii) guide the type of adaptive immunity specific for each pathogen type, or (iii) alter the speed, generation, breadth, specificity, and affinity of the immune responses (2). So far, multiple mechanisms of action for adjuvants have been elucidated (3). One is the depot formation that holds and slowly releases antigens, prompting sustained stimulation of the immune system (4). Another is to promote the recruitment of the immune cells and maturation and activation of antigen-presenting cells (APCs) through the production of cytokines and chemokines (4). Mature APCs then generate an adaptive immune response with enhanced antigen processing and presentation capabilities (4). On the other hand, adjuvants can modulate the type of immunity, for example, dominating either cell-mediated immunity or humoral immunity by the induction of different types of cytokines (5). In fact, informed choice of vaccine adjuvant suitable for target antigens is essential for the promotion of the desired vaccine efficacy.
The first adjuvant used in human vaccines was aluminum salts (referred to as alum) such as aluminum hydroxide, aluminum phosphates, and aluminum potassium sulfate, mainly inducing robust Th2 and antibody responses (1, 6). Alum adjuvants have been widely used since 1930s for licensed vaccines such as diphtheria and tetanus vaccines targeting pathogens for which antibody responses protect the host (1). Therefore, alum adjuvants may not be a good choice for vaccines against intracellular pathogens which largely require the induction of cell-mediated immunity. Additionally, they often induce over activation of Th2-type immunity resulting in adverse effects including antibody-dependent enhancement of infection (7). These limitations have facilitated the development of new adjuvants. QS-21, a saponin compound extracted from the Chilean soapbark tree, increases both antigen-specific antibody and cellular responses (3). It has been used as an adjuvant in melanoma and prostate cancer vaccines (8), as well as combining AS01 for Shingrix (herpes zoster vaccine) and Mosquirix (malaria vaccine) (3, 9). MF59, an oil-in-water emulsion comprising squalene, has been used in influenza vaccines for decades because of strong T and B cell responses (9–11).
Recently, natural ligands or synthetic agents for pattern recognition receptors such as Toll-like receptors (TLRs) have been developed as novel adjuvants, either alone or in combination with various formulations. For example, TLR4 agonist monophosphoryl lipid A is a major component of AS04, an adjuvant for a licensed human papillomavirus vaccine (Cervarix) (6, 9). TLR9 agonist CpG 1018 was clinically applied as an effective adjuvant of the hepatitis B vaccine (Heplisav-B and Dynavax) eliciting a strong antigen-specific Th1 and cell-mediated immune response (12). Poly(I:C), a synthetic TLR3 agonist, has been tried for clinical application in anti-cancer vaccines because it can induce strong T cell-mediated immune responses (13, 14). However, its instability and toxicity have led to the generation of its derivatives (15). Currently, two analogs of poly(I:C) have been subjected to clinical trials (16). For instance, Ampligen (also known as polyI:C12U) induces type I IFN production and Th1 response with reduced toxicity compared to poly(I:C), and is under phase II clinical trials for vaccines against melanoma, colorectal cancer, and prostate cancer (16). The vaccine adjuvant Hiltonol (Poly-ICLC) has been reported to promote an anti-cancer immune response that lowers the risk of metastatic recurrence in breast cancer patients and four studies are under clinical phase I/II trials (16).
Although dsRNA including poly(I:C) are promising adjuvant candidates, especially for anti-viral or anti-cancer vaccines, because of enhancing cell-mediated immunity as well as humoral immunity, there are serious shortcomings to overcome. Firstly, poly(I:C) is inherently not homogenous due to technical limitations in the manufacturing process (17). For the development of pharmaceuticals, it is necessary to define each active ingredient, but there are difficulties in identifying the exact size, molecular weight, and structure of poly(I:C) due to its heterogeneous characteristics. Secondly, since the biological activity of dsRNAs such as poly(I:C) varies depending on their molecular size (18), heterogeneity of poly(I:C) may lead to inadequacies in pharmacokinetic tests required for the development of drugs such as anti-cancer agents (19). In fact, batch-to-batch variations have been reported in the pharmacological action of poly(I:C) (20). Thirdly, the heterogenic nature of poly(I:C) is associated with toxic effects (21). Many studies have reported that poly(I:C) could cause autoimmune diseases such as primary biliary cirrhosis and lupus nephritis due to non-specific immune responses and systemic allergic reactions (22, 23). Fourthly, the production process is too complicated and inefficient to go to quality-controlled mass production. Indeed, poly(I:C) is synthesized through enzymatic synthesis using polyribonucleotide nucleotidyltransferase, but complicated reactions using the enzyme make it difficult to control the length of poly-I and poly-C molecules (20). Commercially-available poly(I:C) and its derivatives are highly heterogenous and lead to lot-to-lot variation (24). Moreover, heating and slow cooling many times are required for reannealing the poly-I and poly-C strands, which makes its reconstitution difficult (15). Thus, an advanced TLR3-based adjuvant would be an excellent next-generation adjuvant to retain the adjuvanticity of poly(I:C) but overcome its limitations. In the current study, by using polymerase-chain reaction (PCR)-coupled bidirectional in vitro transcription (IVT), we developed a homogeneous and quality-controlled dsRNA-based TLR3 agonist as an effective vaccine adjuvant and named it NexaVant (NVT).
Antibodies used in this study were purchased from BioLegend (San Diego, CA, USA) or Southern Biotechnology (Birmingham, AL, USA), and listed in Table S1. Specific pathogen-free C57BL/6 or BALB/c female mice at 6 weeks of age were purchased from Samtako Bio Korea (Kyounggi, Korea) and maintained at the NA Vaccine Institute (NAVI) animal facility (Seoul, Korea). The mice were fed a sterile, commercial mouse diet and provided with water ad libitum. The experimental protocols used in this study were reviewed and approved by the Ethics Committee and Institutional Animal Care and Use Committee (Permit Number: NAVI-2019-0002) of the NAVI.
As a template for IVT, the nucleotide segment (1,701-3,360; 1,660 nucleotides) from CSBV genome (GenBank accession number KF960044.1) was cloned into the pUC-GW-Amp vector and the template was amplified by PCR using primer pairs containing the T7 RNA promoter sequence. PCR cycling conditions were as follows: 95°C for 5 min, followed by 35 cycles of 95°C for 30 s, 60°C for 30 s, and 72°C for 1 min. A final elongation step was carried out for 5 min at 72°C. Synthesis and purification of dsRNA were performed using a MEGAscript RNAi Kit (Thermo Fisher Scientific, Waltham, MA, USA) according to the manufacturer’s instructions. Briefly, IVT reactions were carried out by incubating the PCR products with 10× T7 Reaction Buffer, 4 ribonucleotide solutions, and T7 Enzyme Mix at 37°C for 2-4 h. The IVT products were incubated at 75°C for 5 min and then the complementary RNAs were allowed to anneal to each other at room temperature to form dsRNA. The template DNAs and unannealed ssRNAs were removed by treatment with DNase I and RNase A. Lastly, each dsRNA sample was purified with 100% ethanol and eluted with a solution containing 10 mM Tris-HCl (pH 7) and 1 mM EDTA. For the synthesis of NVT used as a vaccine adjuvant, partial nucleotide segment (1,701-2,112; 412 nucleotides) was selectively cloned, and ssRNAs were digested by treatment with RNase T1 instead of RNase A. Template and primer details for NVT synthesis are shown in Table S2.
The purified NVT sample was analyzed by RP-HPLC on the Waters Alliance HPLC System (Waters, Milford, MA, USA) using a Waters XBridge OST C18 Column with 130 Å, 2.5 μm, and 4.6 × 50 mm (Waters, Milford, MA, USA). Two eluent-buffers were used to perform the chromatography. Buffer A is an aqueous solution containing 0.1 M triethylammonium acetate (TEAA), pH 7.0, and buffer B is an aqueous solution of 0.1 M TEAA, pH 7.0, containing 25% (v/v) acetonitrile. The whole procedure was undertaken at 50°C which is a non-denaturing temperature. Ion-pair RP-HPLC analysis was performed using the following linear gradient condition: flow rate 0.9 ml/min, 40–70% buffer B over 15 min. Peak quantification was performed by recording chromatograms at 260 nm and integrating peak areas.
Human TLR3-expressing HEK293 cells (InvivoGen, San Diego, CA, USA) were cultured in Dulbecco’s modified Eagle medium (DMEM) supplemented with 4.5 g/l glucose, 2 mM L-glutamine, 10% heat-inactivated fetal bovine serum (FBS), 1% penicillin/streptomycin in the presence of blasticidin (Sigma-Aldrich, St Louis, MO, USA) (30 μg/ml), zeocin (InvivoGen) (100 μg/ml), and normocin (InvivoGen) (100 μg/ml). For stimulation, the cells were seeded on a 96-well culture plate at 5 × 104 cells/well and incubated overnight at 37°C. The attached cells were treated with various concentrations of dsRNA, NVT, or poly (I:C) (InvivoGen) and CpG (InvivoGen) for 24 h, and 20 μl of supernatant and 180 μl of Quanti-Blue were reacted in a 96-well enzyme-linked immunosorbent assay (ELISA) plate. While incubating at 37°C for 1-3 h, the absorbance was measured at 655 nm when the most color development occurred.
C57BL/6 mice were injected intramuscularly with optimal dose (10 μg) of NVT, poly(I:C), or CpG (25). Five hours later, total RNA was isolated from the local iLN of the immunized mice using TRIzol reagent (Thermo Fisher Scientific). Briefly, the cells from iLN were lysed by treatment with 1 ml of TRIzol, and 0.2 ml of chloroform was added to the lysate. After centrifugation at 12,000 × g for 15 min at 4°C, the aqueous phase containing RNA was transferred to a new tube. Then, the same amount of isopropanol was added and incubated for 10 min at room temperature, followed by centrifugation for 10 min at 12,000 × g at 4°C. The RNA pellets were washed with 75% ethanol, air-dried, and resuspended in RNase-free water. The amount of total RNA was measured at 260 nm with NanoDrop (Molecular Devices, San Jose, CA, USA). The purity was confirmed at 260/280 nm (a ratio between 1.8 - 2.0 is considered pure) and 260/230 nm (a ratio between 2.0 - 2.2 is considered pure).
One Step TB Green PrimeScript RT-PCR kit (Takara Bio, Shiga, Japan) was used according to the manufacturer’s specifications. Briefly, RNA samples were mixed in a 96-well reaction plate with 2× One Step TB Green RT-PCR Buffer 4, PrimeScript 1 step Enzyme Mix 2, forward and reverse primers (10 μM), and RNase-free dH2O. Then, PCR reactions were performed with pre-incubation at 42°C for 5 min, followed by 40 thermal cycles at 95°C for 10 s and 55°C for 30 s using a CFX real-time PCR system (Bio-Rad, Hercules, CA, USA). The expression level of target genes was normalized to the reference gene HPRT and calculated using the 2−ΔΔCt method as previously described with minor modifications (26). Primers were selected from previous study (27) and synthesized by Cosmogenetech (Seoul, Korea). The specific primer sequences are shown in Table S2.
To test NVT for long-term stability, the substance was stored in an accelerated condition at 25 ± 2°C and relative humidity of 60 ± 5% under the ICH guideline (https://www.ich.org/page/quality-guidelines) on “Stability testing of new drug substances and drug products”. Endotoxin test was performed using the Pierce™ LAL Chromogenic Endotoxin Quantitation Kit (Thermo Fisher Scientific, Waltham, MA, USA) according to the manufacturer’s instructions. Their appearance and concentration were determined for each period, and the stability was monitored by electrophoresis on an 1% agarose gel.
Preclinical GLP toxicity profiling experiments of the NVT administered through intramuscular and subcutaneous routes were undertaken at a GLP-certified organization (Biotoxtech, Cheongju, Korea) according to the Organisation for Economic Co-operation and Development (OECD) protocol (https://www.oecd.org/chemicalsafety/testing/good-laboratory-practiceglp.htm). The test profiles include single dose, dose range finding test, repeated dose toxicity tests (two doses, four doses, and recovery test) for Sprague Dawley rats and New Zealand white rabbits, antigenicity assays [passive cutaneous anaphylaxis (PCA) and active systemic anaphylaxis (ASA)] for guinea pigs, and in vivo micronucleus assay for ICR mice. Also, in vitro chromosomal aberration assay using a Chinese hamster lung cell line, and in vitro bacterial reverse mutation test (Ames test) for Salmonella typhimurium and Escherichia coli were performed (Table 1).
C57BL/6 female mice (n=4 per group) were administered either with 2 μg of ovalbumin (OVA; InvivoGen) alone or formulated with 10 μg of NVT or10 μg of poly(I:C) as an adjuvant via the intramuscular route. At 0, 6 or 24 h, the draining iLN was harvested to dissociate into a single cell suspension. The cells were labeled with a fixable viability dye to distinguish live cells from dead cells followed by surface staining for macrophage (CD11b+F4/80+), neutrophil (CD11b+Ly6G+), and DCs (CD11chiMHC-IIhi) (Supplementary Figure 1). The specificity of staining was confirmed by fluorescence minus one and the migration of innate cells was expressed as an absolute number after flow cytometric analysis. To analyze the activation of DCs, CD11chiMHC-IIhi cells were gated and then the intensity of the surface expression of CD40, CD80, CD86, and MHC class II was determined by NovoCyte flow cytometry (ACEA Biosciences, San Diego, CA, USA). IFN-β was measured by sandwich ELISA according to the manufacturer’s instructions (BioLegend, San Diego, CA, USA).
To explore the efficacy of the adjuvants, C57BL/6 mice (n=5 per group, female, 6-8 weeks old) were immunized intramuscularly twice at two-week intervals with 2 μg of OVA with either alone or adjuvanted with 10 μg of NVT or 10 μg of poly (I:C). No severe clinical signs including weight loss were observed after immunization. Two weeks later, blood was collected to determine OVA-specific antibodies and the spleen was isolated to analyze OVA-specific T cell responses.
The 96-well microplates were coated with either 2 μg/ml of goat anti-mouse IgG antibody for standard curve construction or 5 μg/ml of OVA for OVA-specific antibody titer overnight at 4°C and then non-specific sites were blocked with 1% skim milk. Serially diluted mouse IgG antibodies or serum samples were incubated for 2 h at room temperature. For the OVA-specific Ig isotypes, horseradish peroxidase-conjugated anti-mouse IgG-, IgG1-, and IgG2c-antibodies were used as secondary antibodies and developed by using 3,3′,5,5′-tetramethylbenzidine substrate solution. The reaction was stopped with 0.5 M HCl and the absorbance was measured at 450-590 nm using a Multiskan Sky microplate reader (Thermo Fisher Scientific). A standard curve using mouse IgG was generated and antibody titers of serum samples were calculated as arbitrary units relative to the standard curve.
Two weeks after the last vaccination, spleens were obtained from immunized mice and dissociated into single cells. To examine OVA-specific T cell responses, the cell suspensions were restimulated with 20 μg/ml of OVA peptides (OVA257-264 and OVA323-339) (28) and incubated overnight at 37°C. Then, 1 μg/ml of a protein transport inhibitor GolgiPlug (BD Bioscience, Franklin Lakes, NJ, USA) was added and further cultured for 4-6 h. After washing with phosphate-buffered saline (PBS), the cells were stained with a viability dye, anti-CD4, anti-CD8, and anti-CD44 antibodies for 20 min at 4°C. For intracellular staining, the cells were permeabilized and fixed using a Cytofix/Cytoperm solution (BD Bioscience) and stained with anti-IFN-γ and anti-IL-4 antibodies for 20 min at 4°C. The cells were washed and resuspended with FACS buffer (PBS containing 1% FBS and 0.1% NaN3) and analyzed by flow cytometry.
Experimental results were expressed as the mean value ± standard deviation (S.D.). The Kruskal-Wallis test followed by Dunn’s post-test was used for multiple comparison of more than three groups by using GraphPad Prism version 6.00 for Windows (GraphPad Software, La Jolla, CA, USA, www.graphpad.com). *p<0.05 was considered statistically significant.
The nucleotide segment (1,701-3,360; 1,660 nucleotides) from CSBV genome (GenBank accession number KF960044.1), which does not match with a human DNA sequence, was cloned into a vector. Then, PCR products of various sizes were produced by 3′-serial deletion and used to synthesize dsRNAs of 319, 397, 466, 508, 664, 733, 822, 885, 1,032, 1,153, or 1,648 bp length by IVT. When each dsRNA was electrophoresed on an 1% agarose gel, dsRNA longer than 508 bp showed polymeric impurities similar to poly(I:C), indicating the need to further select dsRNA of less than 500 bp (data not shown). Since dsRNA tend to activate TLR3 signaling, we tested whether the synthetic dsRNA could act as a TLR3 agonist. To this end, the TLR3-expressing reporter cell line was stimulated with dsRNA of 319 bp to 508 bp in length. The induction of TLR3 activation was similar regardless of the dsRNA size (Supplementary Figure 2A). To select a specific length of dsRNA that was the most effective as an adjuvant, mice were intramuscularly injected with OVA formulated with each of the dsRNAs twice at intervals of 2 weeks, and OVA-specific IgG levels in each serum were measured 2 weeks after boosting. Unlike the results of in vitro assays, dsRNAs shorter than 300 bp were found to be less effective in inducing antigen-specific antibodies as vaccine adjuvants in vivo, suggesting that candidate molecules were further selected from dsRNAs with lengths of 400 to 500 bp (Supplementary Figure 2B). Next, to determine the dsRNA size for optimal production efficiency, dsRNAs of various lengths were synthesized under the same conditions and their yields were compared on an 1% agarose gel. The yield of dsRNA synthesis was highest at 424 bp to 460 bp, about 120 μg per 20 μl reaction (Supplementary Figure 2C). In consideration of adjuvanticity, production efficiency, and manufacturing cost, dsRNA with a length of 424 bp (approximately 275 kDa) was selected as a final candidate for product analyses, and the partial genomic sequence (1,701-2,112; 412 nucleotides) of CSBV was used for cloning to synthesize it (Figure 1).
Figure 1 Schematic illustration of NexaVant (NVT) synthesis. The partial nucleotide segment (1701-2112; 412 nucleotides) from the CSBV genome was cloned into the vector. Forward and reverse PCR primers containing the T7 RNA promoter sequence were designed for the DNA template. After in vitro transcription (IVT) on the DNA template, complementary RNA strands were annealed to form dsRNA. Template DNAs and non-specific ssRNAs were removed with the treatment of DNase I and RNase T1 to generate a dsRNA structure having UAUAG-3′ at both ends and the final product was purified through column purification. nt, nucleotide.
When dsRNA is synthesized with a commercial kit, ssRNA and DNA template are removed by treating the product with RNase A and DNase I. However, since RNase A can partially or completely degrade dsRNA (29), there is a possibility that it may affect the stability of the product after synthesis or purification. Considering that RNase T1 specifically degrades ssRNA at G residues and cannot cleave dsRNA, we used RNase T1 instead of RNase A for dsRNA purification. This step generated 424 bp dsRNA with UAUAG-3′ at both ends (Figure 1). We named this RNase T1-treated final product NexaVant (NVT). Treatment of NVT with RNase III alone or in combination with RNase T1 generated approximately 25 bp dsRNAs, indicating that NVT consists of dsRNA that forms a completely complementary pairing (Supplementary Figure 3). We also found the importance of RNase T1 use for the synthesis and purification of NVT by confirming that NVT is completely degraded by RNase A (Supplementary Figure 3).
NVT was tested for stability under accelerated storage conditions (25 ± 2°C). The result showed that the appearance, concentration, and molecular size of NVT were unaffected for 6 months (Figure 2A and Table 2). Next, an RP-HPLC assay was used to confirm that the finally-produced NVT is a single compound. The RP-HPLC study confirmed that NVT was eluted as a single peak at a retention time of 17.601 min and accordingly exhibited almost 100% purity, demonstrating that NVT exhibits an extreme homogeneity (Figure 2B). To determine whether NVT acts as a TLR3 ligand, human TLR3-expressing HEK 293 cells were stimulated with 10, 50, or 100 μg/ml of NVT, or 100 μg/ml of CpG (negative control), or poly(I:C) (positive control), and NF-κB/AP-1 activation was measured. NVT induced TLR3 activation in a dose-dependent manner in vitro (Figure 2C). To determine whether the expression of viral nucleic acid sensors in vivo is increased by NVT, mice were injected intramuscularly with 10 μg of NVT, CpG, or poly(I:C), then local draining iLN were isolated and the expression of TLR3, MDA5, and RIG-I was measured by RT-qPCR analysis. We found that NVT promoted the expression of MDA5 and RIG-I as well as TLR3 to the same level as poly(I:C) (Supplementary Figure 4). For biomedical product development, the in vivo kinetics of the candidate molecule is essential. Notably, pharmacokinetic data of poly(I:C) were difficult to obtain due to the lack of analytical methods for such a heterogeneous mixture (15). In contrast, RT-qPCR reactions can be used to determine the exact copy number of NVT in blood. Indeed, we were able to quantify the content of NVT in blood by collecting blood from rats injected subcutaneously with NVT, extracting RNA, and performing RT-qPCR. The pharmacokinetic analysis showed that the amount of NVT present in the blood decreased rapidly (Figure 2D), indicating that unpredictable responses by NVT caused by long-term retention in the body are unlikely.
Figure 2 Stability and biological characteristics of NVT (A) NVT was stored at 25 ± 2°C for up to 6 months. NVT bands at various concentrations were identified by electrophoresis on 1% agarose gel in each month indicated. (B) Homogeneity of NVT samples was analyzed by RP-HPLC using a two-eluent buffer system. Peak quantification was performed by recording chromatograms at 260 nm and integrating peak areas. (C) Human TLR3-expressing HEK293 cells were stimulated with 10, 50, or 100 μg/ml of NVT, or 100 μg/ml of CpG (negative control) or poly(I:C) (positive control). After 24 h, the supernatant and Quanti-Blue solution were mixed and reacted at 37°C for 1-3 h. The change in absorbance was measured at 655 nm and the TLR3 activity of the samples was normalized relative to non-treated (NT) control. (D) Rats (n=6 per group) were subcutaneously injected with 375 μg of NVT. Blood was collected at each indicated time point, total RNA was extracted, and RT-qPCR for NVT was performed to determine in vivo pharmacokinetics of NVT. *, P < 0.05; ns: not significant.
To evaluate the role of NVT in the induction of an immune response as an adjuvant, C57BL/6 mice were intramuscularly injected with OVA either alone (control) or formulated with NVT or poly(I:C) as an adjuvant, and draining iLNs were collected 0, 6 and 24 h after injection to analyze innate immune cell migration and DC activation (Figure 3A). Compared to the data at 0 h, NVT efficiently promoted the migration of macrophages (CD11b+F4/80+) and neutrophils (CD11b+Ly6G+) as well as DCs (CD11chiMHC-IIhi) into draining iLN soon after the injection as poly(I:C) did (Figures 3B-D). Next, to measure the expression of DC maturation, the mean fluorescence intensity of CD40, CD80, CD86, and MHC-II in CD11chiMHC-IIhi DCs was analyzed by flow cytometry, and we found both NVT and poly(I:C) promoted the activation of DCs. NVT significantly increased CD40 at 6 h compared to the control (OVA alone). After that, the expression of CD40 decreased and became similar to control at 24 h (Figure 3E). NVT enhanced the expression of the costimulatory molecules CD80 and CD86 at 24 h after injection, significantly higher than the control and poly(I:C) groups (Figure 3F, G). NVT also significantly promoted an increase in MHC class II expression compared to control at 24 h (Figure 3H). In addition to DC activation, NVT substantially promoted the production of IFN-β, the major effector cytokine induced by TLR3 agonists, compared to poly(I:C) at 6 h after injection, indicating that NVT provides strong in vivo activation of type I IFN signaling cascades (Figure 3I). Taken together, these results suggest NVT works as a potential adjuvant that can activate adaptive immunity through migration of APCs to LN, induction of DC activation, and promotion of IFN-β production.
Figure 3 NVT prompts innate immune responses. (A) Experimental scheme. C57BL/6 female mice (n=4 per group) were injected into the thigh muscles of the right hind leg with OVA (2 μg) either alone or together with NVT (10 μg) or poly(I:C) (10 μg). At 0, 6 and 24 h, draining inguinal lymph node (iLN) on the right hind leg was collected, dissociated into single cells, and whole cells were counted. (B-D) Macrophages (CD11b+F4/80+), neutrophils (CD11b+Ly6G+) and DCs (CD11chiMHC-IIhi) were analyzed by flow cytometry, and the absolute numbers were calculated by multiplying the total iLN cells by their percentage. (E-H) The mean fluorescence intensity of CD40, CD80, CD86, and MHC-II expression on CD11chiMHC-IIhi DCs was determined by flow cytometric analysis. (I) The concentration of IFN-β in serum was measured by ELISA. All data were expressed as the mean values ± S.D. *, P < 0.05; N.D., not detected.
Next, we examined the ability of NVT to induce antigen-specific antibody responses. C57BL/6 mice were immunized intramuscularly twice at two-week intervals with either OVA alone or adjuvanted with NVT or poly(I:C) according to the schedule (Figure 4A). Then, OVA-specific total IgG, IgG1, and IgG2c were measured in sera from mice two weeks after the last immunization. The groups in which OVA was formulated with an adjuvant such as NVT or poly(I:C) showed significant increases in antigen-specific IgG. Interestingly, NVT had higher levels of Th1-type IgG2c antibody but lower levels of Th2-type IgG1 antibody compared to poly(I:C) (Figures 4B-D). These results suggest that NVT is a strong effective vaccine adjuvant that can induce a potent Th1-biased antibody response.
Figure 4 NVT potently enhances Th1-skewed antibody and T cell response. (A) C57BL/6 mice (n=5 per group) were primed and boosted via an intramuscular route with OVA (2 μg) either alone or adjuvanted with NVT (10 μg) or poly(I:C) (10 μg). (B-D) The levels of OVA-specific total IgG, IgG1, and IgG2c antibodies in the serum were measured at 2 weeks post-boosting. (E, F) Spleen cells were obtained from mice 2 weeks after boosting and restimulated with OVA peptides (OVA257-264 and OVA323-339). After blocking cytokine secretion with protein transport inhibitor, surface staining was performed with anti-CD4, anti-CD8, and anti-CD44 antibodies, followed by intracellular staining with anti-IFN-γ and anti-IL-4 antibodies. Flow cytometric analysis showed the proportion of IFN-γ+-expressing CD4 and CD8 T cells in the draining iLN. Data are presented as the mean values ± S.D. *, P < 0.05; ns, not significant; N.D., not detected.
To further explore whether NVT could promote the antigen-specific T cell response, C57BL/6 mice were vaccinated twice with either OVA alone or together with NVT or poly(I:C). Two weeks after boosting, splenocytes isolated from the mice were restimulated with epitopes of the OVA protein, OVA257-264 and OVA323-339, to analyze OVA-specific T cell responses (28). We found that OVA alone is insufficient to induce OVA-specific CD4+ T cell responses. NVT enhanced OVA-specific IFN-γ-producing CD4+ T cells to a level similar to that of poly(I:C) (Figure 4E) whereas OVA-specific IL-4-producing CD4+ T cells responses were not observed in any group (data not shown). Moreover, NVT promoted an OVA-specific cytotoxic T lymphocyte (CTL) response in the spleen to a level comparable to that of poly(I:C) (Figure 4F). Taken together, NVT would be an effective vaccine adjuvant that promotes potent Th1 and CTL responses, especially for vaccines against intracellular pathogens or cancers.
Pharmaceutical development includes GLP preclinical toxicology studies as required by regulatory agencies (30). Thus, we performed a GLP toxicity test to see if NVT is a safe substance without causing severe toxicity, and to obtain the clinical starting dose and safety margin we determined the maximum tolerated dose (MTD) and no observed adverse effect level (NOAEL). We first determined the MTD and lethal dose of NVT in rats, which is a standard toxicological test animal model (30), by subcutaneously administering doses of 0, 10, 20, and 40 mg/kg. No severe adverse effects were observed even at the highest dose and therefore lethal dose and MTD were considered as >40 mg/kg. In addition, when a repeated dose toxicity test (subcutaneous route in rats, 4 doses and recovery for 2 weeks) was performed, there was no mortality or serious symptoms, and NOAEL and MTD were confirmed to be 3 mg/kg for males and 6 mg/kg for females. Next, according to the standard protocol of the OECD, NVT (1.6, 0.8, 0.4, and 0.2 mg) was injected into ICR mice through the intramuscular route, and the effect on micronuclei induction was confirmed. There was no abnormality in the generation of micronuclear polychromatic red blood cells (MNPCE), indicating that NVT did not cause chromosomal damage, consistent with the in vitro chromosomal aberration assay. Lastly, the results of antigenicity (guinea pig) and Ames test (Salmonella) confirmed that NVT was a non-antigenic and non-mutagenic substance, respectively. Therefore, NVT is expected to be a safe vaccine adjuvant without serious toxicity in the range below the MTD and NOAEL (Table 1).
Although poly(I:C) has been a vaccine adjuvant candidate of interest for increasing the effectiveness of anti-viral and anti-cancer vaccines (22, 31), its toxicity problems and difficulties in quality-controlled manufacture and pharmacokinetic analysis due to its molecular nature (i.e., mixed compounds with heterogeneous molecular structures) remain to be resolved for clinical application (15, 31). To overcome these problems, we developed a novel TLR3 agonist, NVT, and investigated its physicochemical properties, adjuvanticity, and safety. NVT displayed high purity, molecular homogeneity, measurable pharmacokinetics, long-term stability, and non-toxicity in various animals (Table 1). Mechanism studies demonstrated that NVT is effective for the migration of innate immune cells into local draining lymph node and the activation of DCs. Furthermore, NVT induced a Th1-biased antibody response superior to poly(I:C). Therefore, NVT would be a promising adjuvant for anti-viral or anti-cancer vaccines, successfully overcoming the problems of poly(I:C) as an adjuvant candidate.
To produce the intended effects an active pharmaceutical ingredient should possess consistent molecular characteristics, defined pharmacokinetics, and appropriate stability. The heterogeneous structure of poly(I:C) made exact pharmacokinetic analysis difficult and induced many unpredictable side effects in clinical trials (15). The current study demonstrated that NVT is a homogeneous molecule with a confirmed molecular structure that enables accurate pharmacokinetic analysis. Manufacturing of NVT is highly reproducible without sequence errors in PCR and IVT steps (data not shown), indicating that NVT can be mass-produced as an identical material with batch-to-batch consistency. Considering that the physical instability of the adjuvant to temperature changes can lead to loss of vaccine efficacy even within the cold chains (32), the long-term stability of NVT as determined by the accelerated stability test condition will be a huge advantage for commercial manufacturing, storage, and shipping of the final vaccine product.
NVT has excellent adjuvanticity in that it potently enhanced OVA-specific antibody responses; in particular, the production of IgG2c is significantly higher than poly(I:C). Notably, the production of IgG2c in C57BL/6 mice is associated with a Th1-type cellular immune response (33). Concomitantly with the antibody responses, NVT promoted the induction of antigen-specific Th1 and CTL responses rather than Th2-type responses. Since Th1-type immunity is essential for the removal of intracellular pathogens or tumors that are difficult to eliminate through antibody-mediated responses (34), NVT could be suitable as a vaccine adjuvant requiring cell-mediated responses for protection. Considering the possibility of antibody-dependent enhancement of infection and allergic reactions by Th2-biased vaccines (7, 35, 36), Th1-biased NVT could be selected for many vaccine applications.
We found that NVT could activate TLR3 and increase the expression of the viral RNA sensors MDA5 and RIG-I as much as poly(I:C) (Supplementary Figure 4). The increased expression of those viral RNA sensors may promote the production of type I IFNs and thereby potentiate anti-viral host defense by increasing recognition of and response to intracellular viral pathogens (37). Indeed, type I IFNs have broad biological effects on the defense against viral infection in the host (38). Type I IFNs are essential for DC maturation to promote Th1 cell differentiation (13). Type I IFNs also promote the formation of a germinal center (GC) and leads to selective amplification of the IgG2c+ GC B cells through Th1-dependent pathways (38). Consequently, type I IFNs augment IgG2c production with class switching (38, 39), and deficiency of IFNR results in a significant decrease in the production of Ag-specific IgG2a with an increase in IgG1 (40). These results imply that IFN-β induced by NVT would play a key role in the formation of GC B cells and the production of IgG2c as well as Th1 cellular immunity.
We demonstrated that NVT is a vaccine adjuvant that does not cause serious problems in GLP toxicity tests according to the OECD guidelines. Despite the potential of poly(I:C) as an effective vaccine adjuvant, its clinical application has been limited due to the possibility of various toxic side effects (22).. For instance, poly(I:C) can stimulate the production of factors that can exacerbate lupus nephritis in the nephritic kidney in a mouse animal model (41). Poly(I:C) can also cause abnormal expression of MHC class I molecules on beta islet cells and activate potentially autoreactive T cells in the pancreas, which can lead to autoimmune diseases such as diabetes in mice (42). In a human study, intravenous injection of poly(I:C) triggered toxicity such as fever, mild elevation of liver enzymes, and coagulation abnormalities (43). Moreover, a phase I clinical trial in advanced cancer reported that poly(I:C) complexes developed systemic allergic reactions in 2 of 32 patients (23). On the other hand, NVT is considered to be non-allergic because no inflammatory responses or abnormalities were observed in the PCA and ASA tests using the guinea pigs (Table 1). GLP toxicity results also showed that NVT does not cause mortality or severe problems in rats up to approximately 40 mg/kg via the subcutaneous route, and did not induce adverse effects in toxicity tests according to the OECD protocol in various animal models (Table 1). In addition, since NVT disappears rapidly after proper stimulation within the body, it is considered to be less likely to cause an abnormal response. A safety assessment of NVT in humans will be needed for future clinical applications.
Despite the excellent immunostimulatory potential of NVT, there is the possibility of non-delivery issue due to RNA degradation as RNA can be easily degraded by various physical or chemical factors (44). In particular, rapid degradation by enzymes such as ribonucleases in vivo may prevent delivery to APCs or lymphoid organs, limiting their role as immune stimulators (22). Poly(I:C) showed enhanced stability in vivo by combining poly-L-lysine and carboxymethyl cellulose (poly-ICLC) (45), implying that the stability of NVT can be further improved. Currently, many studies have demonstrated that combination of vaccines with emulsions or cationic liposomes not only protects the substance but also induces a more efficient immune response (46, 47). Thus, the formulation of the NVT with the chemicals or delivery system is expected to contribute to more effective vaccine efficacy as well as stability in vivo.
In conclusion, we demonstrated that NVT in the presence of an antigen successfully elicits antigen-specific T cell and antibody response. More importantly, NVT had no observed toxicity on administration to various animal models by various routes. In addition, NVT has a single molecular weight and defined structure and is feasible for mass production under quality-controlled manufacturing. Therefore, NVT could be a novel, effective, and safe adjuvant for human vaccines, especially for anti-viral or anti-cancer vaccines. In the future, optimized formulation of vaccine antigen and NVT is expected to increase antigen persistence and potentiate protective immune response.
The original contributions presented in the study are included in the article/Supplementary Materials. Further inquiries can be directed to the corresponding author.
The animal study was reviewed and approved by The Ethics Committee and Institutional Animal Care and Use Committee of the NA Vaccine Institute (Permit Number: NAVI-2019-0002).
SH and KK designed this work. KK, SC, HB and CH carried out all experiments. KK, M-GL and SH analyzed and/or interpreted data. KK, SC, D-HK and SH prepared and reviewed the manuscript. All authors contributed to the article and approved the submitted version.
This work was supported by the National Research Foundation of Korea (NRF) funded by the Ministry of Science and ICT (NRF-2018R1A5A2024418, NRF-2019R1A2C2007041, NRF-2022M3A9F3082330, and RS-2022-00164722), the Technology Development Program of MSS (S3030897), and the Korea Health Technology R&D Project through the Korea Health Industry Development Institute (KHIDI), funded by the Ministry of Health & Welfare, Republic of Korea (HI22C1234).
Author KK, SC, S-HL, HB, CH, M-GL and D-HK are employed by NA Vaccine Institute Research and Development Center.
The remaining author declare that the research was conducted in the absence of any commercial or financial relationships that could be construed as a potential conflict of interest.
All claims expressed in this article are solely those of the authors and do not necessarily represent those of their affiliated organizations, or those of the publisher, the editors and the reviewers. Any product that may be evaluated in this article, or claim that may be made by its manufacturer, is not guaranteed or endorsed by the publisher.
The Supplementary Material for this article can be found online at: https://www.frontiersin.org/articles/10.3389/fimmu.2023.1075291/full#supplementary-material
1. Di Pasquale A, Preiss S, Tavares Da Silva F, Garcon N. Vaccine adjuvants: From 1920 to 2015 and beyond. Vaccines (Basel) (2015) 3(2):320–43. doi: 10.3390/vaccines3020320
2. Rapaka RR, Cross AS, McArthur MA. Using adjuvants to drive T cell responses for next-generation infectious disease vaccines. Vaccines (Basel) (2021) 9(8):820. doi: 10.3390/vaccines9080820
3. Pulendran B, SA P, O'Hagan DT. Emerging concepts in the science of vaccine adjuvants. Nat Rev Drug Discovery (2021) 20(6):454–75. doi: 10.1038/s41573-021-00163-y
4. Awate S, Babiuk LA, Mutwiri G. Mechanisms of action of adjuvants. Front Immunol (2013) 4:114. doi: 10.3389/fimmu.2013.00114
5. Morrow MP, Yan J, Pankhong P, Ferraro B, Lewis MG, Khan AS, et al. Unique Th1/Th2 phenotypes induced during priming and memory phases by use of interleukin-12 (IL-12) or IL-28B vaccine adjuvants in rhesus macaques. Clin Vaccine Immunol (2010) 17(10):1493–9. doi: 10.1128/CVI.00181-10
6. Didierlaurent AM, Morel S, Lockman L, Giannini SL, Bisteau M, Carlsen H, et al. AS04, an aluminum salt- and TLR4 agonist-based adjuvant system, induces a transient localized innate immune response leading to enhanced adaptive immunity. J Immunol (2009) 183(10):6186–97. doi: 10.4049/jimmunol.0901474
7. Tseng CT, Sbrana E, Iwata-Yoshikawa N, Newman PC, Garron T, Atmar RL, et al. Immunization with sars coronavirus vaccines leads to pulmonary immunopathology on challenge with the sars virus. PloS One (2012) 7(4):e35421. doi: 10.1371/journal.pone.0035421
8. Gin DY, Slovin SF. Enhancing immunogenicity of cancer vaccines: QS-21 as an immune adjuvant. Curr Drug Ther (2011) 6(3):207–12. doi: 10.2174/157488511796391988
9. Bonam SR, Partidos CD, Halmuthur SKM, Muller S. An overview of novel adjuvants designed for improving vaccine efficacy. Trends Pharmacol Sci (2017) 38(9):771–93. doi: 10.1016/j.tips.2017.06.002
10. O'Hagan DT. MF59 is a safe and potent vaccine adjuvant that enhances protection against influenza virus infection. Expert Rev Vaccines (2007) 6(5):699–710. doi: 10.1586/14760584.6.5.699
11. Wilkins AL, Kazmin D, Napolitani G, Clutterbuck EA, Pulendran B, Siegrist CA, et al. AS03- and MF59-adjuvanted influenza vaccines in children. Front Immunol (2017) 8:1760. doi: 10.3389/fimmu.2017.01760
12. Champion CR. Heplisav-b: A hepatitis b vaccine with a novel adjuvant. Ann Pharmacother (2021) 55(6):783–91. doi: 10.1177/1060028020962050
13. Longhi MP, Trumpfheller C, Idoyaga J, Caskey M, Matos I, Kluger C, et al. Dendritic cells require a systemic type I interferon response to mature and induce CD4+ Th1 immunity with poly ic as adjuvant. J Exp Med (2009) 206(7):1589–602. doi: 10.1084/jem.20090247
14. Rouas R, Lewalle P, El Ouriaghli F, Nowak B, Duvillier H, Martiat P. Poly(I:C) used for human dendritic cell maturation preserves their ability to secondarily secrete bioactive IL-12. Int Immunol (2004) 16(5):767–73. doi: 10.1093/intimm/dxh077
15. Naumann K, Wehner R, Schwarze A, Petzold C, Schmitz M, Rohayem J. Activation of dendritic cells by the novel toll-like receptor 3 agonist RGC100. Clin Dev Immunol (2013) 2013:283649. doi: 10.1155/2013/283649
16. Le Naour J, Galluzzi L, Zitvogel L, Kroemer G, Vacchelli E. Trial watch: TLR3 agonists in cancer therapy. Oncoimmunology (2020) 9(1):1771143. doi: 10.1080/2162402X.2020.1771143
17. Grunberg-Manago M, Oritz PJ, Ochoa S. Enzymatic synthesis of nucleic acidlike polynucleotides. Science (1955) 122(3176):907–10. doi: 10.1126/science.122.3176.907
18. Machida H, Kuninaka A, Yoshino H. Relationship between the molecular size of poly I-poly c and its biological activity. Jpn J Microbiol (1976) 20(2):71–6. doi: 10.1111/j.1348-0421.1976.tb00911.x
19. Marchetti S, Schellens JH. The impact of FDA and EMEA guidelines on drug development in relation to phase 0 trials. Br J Cancer (2007) 97(5):577–81. doi: 10.1038/sj.bjc.6603925
20. Nakano T, Yamamura ET, Fujita H, Sone T, Asano K. Novel methods for nucleotide length control in double-stranded polyinosinic-polycytidylic acid production using uneven length components. Biosci Biotechnol Biochem (2018) 82(11):1889–901. doi: 10.1080/09168451.2018.1501264
21. Komal A, Noreen M, El-Kott AF. TLR3 agonists: RGC100, ARNAX, and poly-IC: A comparative review. Immunol Res (2021) 69(4):312–22. doi: 10.1007/s12026-021-09203-6
22. Hafner AM, Corthesy B, Merkle HP. Particulate formulations for the delivery of Poly(I:C) as vaccine adjuvant. Adv Drug Delivery Rev (2013) 65(10):1386–99. doi: 10.1016/j.addr.2013.05.013
23. Krown SE, Kerr D, Stewart WE 2nd, Field AK, Oettgen HF. Phase I trials of Poly(I,C) complexes in advanced cancer. J Biol Response Mod (1985) 4(6):640–9.
24. Mian MF, Ahmed AN, Rad M, Babaian A, Bowdish D, Ashkar AA. Length of dsrna (Poly I:C) drives distinct innate immune responses, depending on the cell type. J Leukoc Biol (2013) 94(5):1025–36. doi: 10.1189/jlb.0312125
25. Ahn SY, Le CTT, Ko EJ. Monophosphoryl lipid a and poly I:C combination adjuvant promoted ovalbumin-specific cell mediated immunity in mice model. Biol (Basel) (2021) 10(9):908. doi: 10.3390/biology10090908
26. Katragadda V, Adem M, Mohammad RA, Sri Bhasyam S, Battini K. Testosterone recuperates deteriorated Male fertility in cypermethrin intoxicated rats. Toxicol Res (2021) 37(1):125–34. doi: 10.1007/s43188-020-00046-1
27. Araya RE, Jury J, Bondar C, Verdu EF, Chirdo FG. Intraluminal administration of poly I:C causes an enteropathy that is exacerbated by administration of oral dietary antigen. PloS One (2014) 9(6):e99236. doi: 10.1371/journal.pone.0099236
28. Arnaboldi PM, Roth-Walter F, Mayer L. Suppression of Th1 and Th17, but not Th2, responses in a CD8(+) T cell-mediated model of oral tolerance. Mucosal Immunol (2009) 2(5):427–38. doi: 10.1038/mi.2009.93
29. Edy VG, Szekely M, Loviny T, Dreyer C. Action of nucleases on double-stranded RNA. Eur J Biochem (1976) 61(2):563–72. doi: 10.1111/j.1432-1033.1976.tb10051.x
30. Piwoni K, Jaeckel G, Rasa A, Alberts P. 4-week repeated dose rat glp toxicity study of oncolytic echo-7 virus rigvir administered intramuscularly with a 4-week recovery period. Toxicol Rep (2021) 8:230–8. doi: 10.1016/j.toxrep.2021.01.009
31. Qu J, Hou Z, Han Q, Zhang C, Tian Z, Zhang J. Poly(I:C) exhibits an anti-cancer effect in human gastric adenocarcinoma cells which is dependent on RLRs. Int Immunopharmacol (2013) 17(3):814–20. doi: 10.1016/j.intimp.2013.08.013
32. Darriba ML, Cerutti ML, Bruno L, Cassataro J, Pasquevich KA. Stability studies of the vaccine adjuvant U-Omp19. J Pharm Sci (2021) 110(2):707–18. doi: 10.1016/j.xphs.2020.10.011
33. Nazeri S, Zakeri S, Mehrizi AA, Sardari S, Djadid ND. Measuring of IgG2c isotype instead of IgG2a in immunized C57bl/6 mice with plasmodium vivax trap as a subunit vaccine candidate in order to correct interpretation of Th1 versus Th2 immune response. Exp Parasitol (2020) 216:107944. doi: 10.1016/j.exppara.2020.107944
34. Knutson KL, Disis ML. Tumor antigen-specific T helper cells in cancer immunity and immunotherapy. Cancer Immunol Immunother (2005) 54(8):721–8. doi: 10.1007/s00262-004-0653-2
35. Xu L, Ma Z, Li Y, Pang Z, Xiao S. Antibody dependent enhancement: Unavoidable problems in vaccine development. Adv Immunol (2021) 151:99–133. doi: 10.1016/bs.ai.2021.08.003
36. Ebenig A, Muraleedharan S, Kazmierski J, Todt D, Auste A, Anzaghe M, et al. Vaccine-associated enhanced respiratory pathology in covid-19 hamsters after T(H)2-biased immunization. Cell Rep (2022) 40(7):111214. doi: 10.1016/j.celrep.2022.111214
37. Banos-Lara Mdel R, Ghosh A, Guerrero-Plata A. Critical role of MDA5 in the interferon response induced by human metapneumovirus infection in dendritic cells and in vivo. J Virol (2013) 87(2):1242–51. doi: 10.1128/JVI.01213-12
38. Dahlgren MW, Plumb AW, Niss K, Lahl K, Brunak S, Johansson-Lindbom B. Type I interferons promote germinal centers through b cell intrinsic signaling and dendritic cell dependent Th1 and tfh cell lineages. Front Immunol (2022) 13:932388. doi: 10.3389/fimmu.2022.932388
39. Swanson CL, Wilson TJ, Strauch P, Colonna M, Pelanda R, Torres RM. Type I IFN enhances follicular b cell contribution to the T cell-independent antibody response. J Exp Med (2010) 207(7):1485–500. doi: 10.1084/jem.20092695
40. Coro ES, Chang WL, Baumgarth N. Type I IFN receptor signals directly stimulate local b cells early following influenza virus infection. J Immunol (2006) 176(7):4343–51. doi: 10.4049/jimmunol.176.7.4343
41. Patole PS, Grone HJ, Segerer S, Ciubar R, Belemezova E, Henger A, et al. Viral double-stranded RNA aggravates lupus nephritis through toll-like receptor 3 on glomerular mesangial cells and antigen-presenting cells. J Am Soc Nephrol (2005) 16(5):1326–38. doi: 10.1681/ASN.2004100820
42. Lang KS, Recher M, Junt T, Navarini AA, Harris NL, Freigang S, et al. Toll-like receptor engagement converts T-cell autoreactivity into overt autoimmune disease. Nat Med (2005) 11(2):138–45. doi: 10.1038/nm1176
43. Freeman AI, Al-Bussam N, O'Malley JA, Stutzman L, Bjornsson S, Carter WA. Pharmacologic effects of polyinosinic-polycytidylic acid in man. J Med Virol (1977) 1(2):79–93. doi: 10.1002/jmv.1890010202
44. Stahl-Hennig C, Eisenblatter M, Jasny E, Rzehak T, Tenner-Racz K, Trumpfheller C, et al. Synthetic double-stranded RNAs are adjuvants for the induction of T helper 1 and humoral immune responses to human papillomavirus in rhesus macaques. PloS Pathog (2009) 5(4):e1000373. doi: 10.1371/journal.ppat.1000373
45. Diaz-San Segundo F, Dias CC, Moraes MP, Weiss M, Perez-Martin E, Salazar AM, et al. Poly ICLC increases the potency of a replication-defective human adenovirus vectored foot-and-Mouth disease vaccine. Virology (2014) 468-470:283–92. doi: 10.1016/j.virol.2014.08.012
46. Zaks K, Jordan M, Guth A, Sellins K, Kedl R, Izzo A, et al. Efficient immunization and cross-priming by vaccine adjuvants containing TLR3 or TLR9 agonists complexed to cationic liposomes. J Immunol (2006) 176(12):7335–45. doi: 10.4049/jimmunol.176.12.7335
47. Nordly P, Rose F, Christensen D, Nielsen HM, Andersen P, Agger EM, et al. Immunity by formulation design: Induction of high CD8+ T-cell responses by Poly(I:C) incorporated into the CAF01 adjuvant Via a double emulsion method. J Control Release (2011) 150(3):307–17. doi: 10.1016/j.jconrel.2010.11.021
Keywords: vaccine adjuvant, dsRNA, in vitro transcription, TLR3 agonist, Th1 response
Citation: Ko KH, Cha SB, Lee S-H, Bae HS, Ham CS, Lee M-G, Kim D-H and Han SH (2023) A novel defined TLR3 agonist as an effective vaccine adjuvant. Front. Immunol. 14:1075291. doi: 10.3389/fimmu.2023.1075291
Received: 20 October 2022; Accepted: 06 January 2023;
Published: 24 January 2023.
Edited by:
Masashi Tachibana, Osaka University, JapanReviewed by:
Bert Devriendt, Ghent University, BelgiumCopyright © 2023 Ko, Cha, Lee, Bae, Ham, Lee, Kim and Han. This is an open-access article distributed under the terms of the Creative Commons Attribution License (CC BY). The use, distribution or reproduction in other forums is permitted, provided the original author(s) and the copyright owner(s) are credited and that the original publication in this journal is cited, in accordance with accepted academic practice. No use, distribution or reproduction is permitted which does not comply with these terms.
*Correspondence: Seung Hyun Han, c2hoYW4tbWlAc251LmFjLmty
Disclaimer: All claims expressed in this article are solely those of the authors and do not necessarily represent those of their affiliated organizations, or those of the publisher, the editors and the reviewers. Any product that may be evaluated in this article or claim that may be made by its manufacturer is not guaranteed or endorsed by the publisher.
Research integrity at Frontiers
Learn more about the work of our research integrity team to safeguard the quality of each article we publish.