- Brain Tumor Research Center, Beijing Neurosurgical Institute, Beijing Laboratory of Biomedical Materials, Beijing, China
As one of the main components of the glioma immune microenvironment, glioma-associated macrophages (GAMs) have increasingly drawn research interest. Primarily comprised of resident microglias and peripherally derived mononuclear macrophages, GAMs are influential in a variety of activities such as tumor cell resistance to chemotherapy and radiotherapy as well as facilitation of glioma pathogenesis. In addition to in-depth research of GAM polarization, study of mechanisms relevant in tumor microenvironment recruitment has gradually increased. Suppression of GAMs at their source is likely to produce superior therapeutic outcomes. Here, we summarize the origin and recruitment mechanism of GAMs, as well as the therapeutic implications of GAM inhibition, to facilitate future glioma-related research and formulation of more effective treatment strategies.
Introduction
Gliomas are one of the most common adult brain tumors. According to the WHO classification of central nervous system tumors, gliomas are graded as levels 1, 2, 3 or 4 (1). Over recent decades, the treatment of CNS tumors has greatly improved, with therapeutic options currently including surgery, radiotherapy, chemotherapy, and targeted therapy (2, 3). However, median survival time among glioma patients continues to remain low relative to that of patients suffering malignancies such as those of the thyroid or breast. Unfortunately, the median survival of patients suffering glioblastoma (GBM), the most malignant astrocytoma, was recently reported to be approximately 15 months (4, 5).
The tumor immune microenvironment (TME) has long been a focus in oncological research. The TME mainly consists of tumor-associated macrophages, dendritic cells, neutrophils, lymphocytes, astrocytes, and other non-tumor-related cells, and plays a primary role in the promotion of glioma pathogenesis (Table 1) (13–16). Glioma-associated macrophages (GAMs) comprise approximately 25% of tumor volume (17) and primarily consist of microglia and macrophages. The presence of both cell types is understood to significantly positively correlate with the malignant progression of glioma (18) and is associated with the cellular acquisition of properties similar to the M2 macrophage phenotype (19, 20). Detailed study of GAM-TME interaction is thus warranted to facilitate development of novel glioma treatment methods and effectively improve glioma patient prognosis.
The origin and physiological function of glioma-associated macrophages in the tumor microenvironment
As the pathogenesis of malignancy progresses, interactions among tumor cells and adjacent tissues result in the formation of the TME, especially in the case of solid malignancy (21, 22). The TME provides a favorable environment for malignant cell growth and enables more effective proliferation as well as resistance to drugs and immunity (23). As the TME develops, a variety of immune cells including tumor-associated macrophages (TAMs), lymphocytes, dendritic cells, and neutrophils are recruited to the vicinity of the tumor (24). However, alterations in molecular interactions within the TME often result in an emergence of many immunosuppressive cells [such as regulatory T cells (25) and TAMs (26)] in and around the tumor. In concert with tumor-promoting molecules, these cells accelerate tumor progression (27, 28). Similarly, GAMs are abundant in and around gliomas (29). Prior studies have confirmed that GAMs in the central nervous system primarily originate from brain-resident microglia and peripherally-derived mononuclear macrophages that enter the central nervous system due to breakdown of the blood-brain barrier (Figure 1) (30). While peripherally-derived mononuclear macrophages are mainly distributed in the core region of the tumor, microglia are generally localized in the area surrounding the tumor (17). Of course, this distribution may be related to the recruitment characteristics of the corresponding cytokines. Studies have shown that some cytokines are more likely to recruit macrophages derived from monocytes in peripheral blood (31).
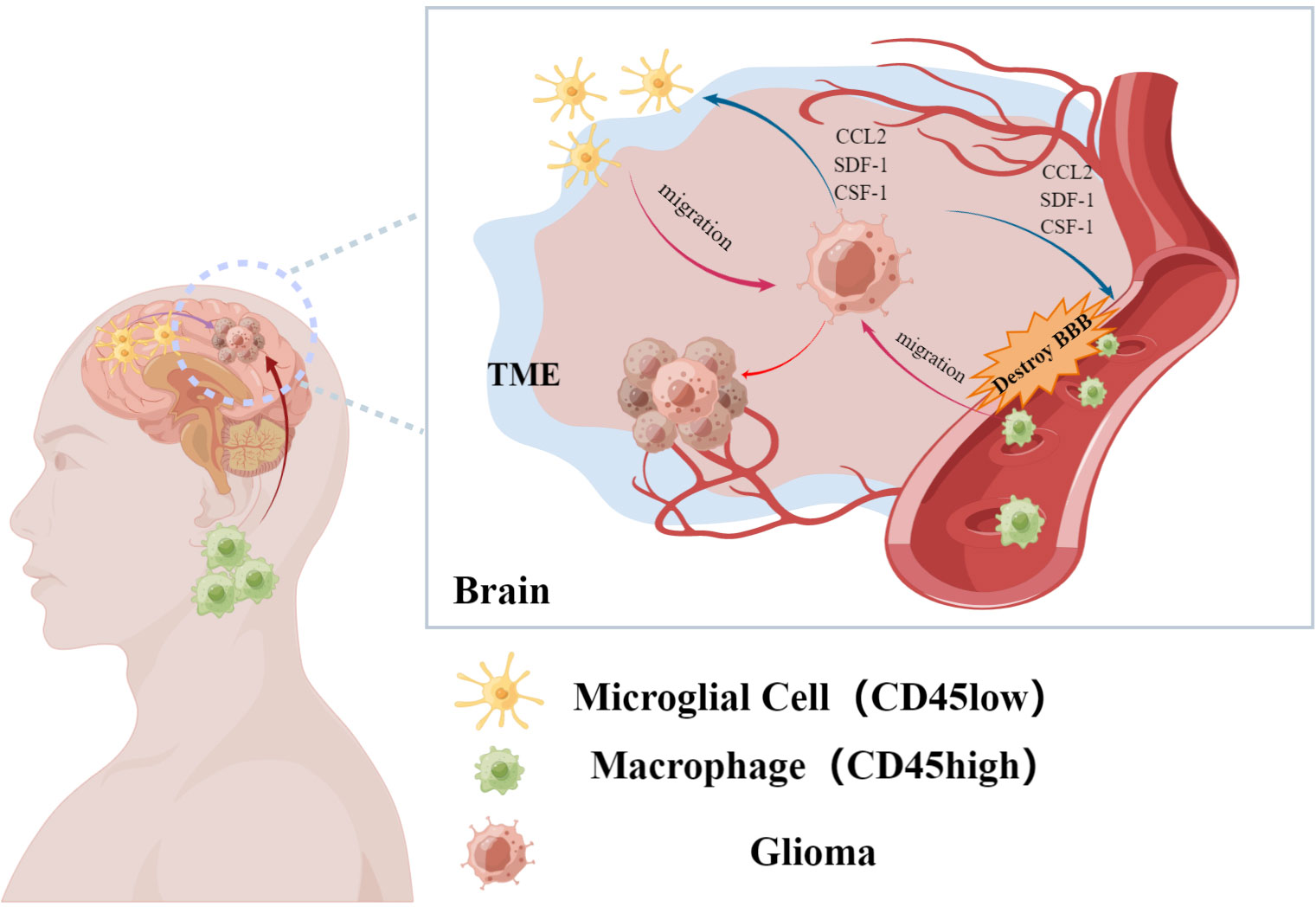
Figure 1 Glioma-associated macrophages are recruited by glioma cells to accumulate in the tumor microenvironment. It mainly includes resident macrophages in the brain and macrophages from peripheral sources, which affect the malignant process of glioma. (By Figdraw).
Microglia, derived from yolk sac progenitor cells, appear in the central nervous system at an early stage of ontogeny. The presence of microglia is detected in the brain as early as the ninth embryonic day (32). Traditionally, the distinction between microglia and peripherally-derived mononuclear macrophages is made based on differences in levels of CD45 expression; high levels of CD45 expression (CD45high) is considered a characteristic of peripherally-derived cells while low levels of CD45 expression (CD45low) is characteristic of microglia (33, 34). However, microglial expression of CD45 was reported to be up-regulated in the TME (35). Distinguishing between the two aforementioned cell subtypes, collectively referred to as GAMs, can thus be challenging. Of course, previous researches have shown that CXCR1+/CCR2- can be used for marking microglia, and CXCR1-/CCR2+ mark monocyte-derived macrophage. And this method is well accepted by the researchers (36). Despite their different origins, both microglia and peripherally-derived mononuclear macrophages play roles in promoting glioma progression (37)
Cytokines promote GAM migration to the site of the glioma and gradually increase the proportion of GAMs within brain tissue from 10-15% to 30-50%, eventually resulting in GAMs becoming the primary component of the glioma TME (20). Under the influence of glioma immune microenvironment, the polarization direction of Gams changed significantly, forming the GAM population dominated by M2 type (38). And as the grade of glioma increased, the proportion of M2 type GAM is also increasing. This type of GAMs produce cytokines such as TGF-β and IL-6, and generally promote tumor progression (39, 40). Such pathologic changes facilitate cellular invasion and angiogenesis, mediate tumor immune evasion, influence T cell infiltration and function, and induce Treg responses, thus significantly promoting malignant progression of glioma (26, 41). Furthermore, massive GAM infiltration of glioma tissue correlates with a poor patient prognosis; reduction of GAM infiltration often facilitates glioma treatment (26, 42). However, the distinction between M1 and M2 GAMs remains unclear. Frequent co-expression of M1 and M2 genes in the same cell suggests that these two subtypes may not be static (43). Furthermore, use of certain drugs (such as rapamycin), decreased M2-type GAM activation, and increased M1-type GAM activation were reported to restore GAM cytotoxic capabilities and result in glioma cell destruction (44). Although inducing changes in the polarization direction of macrophages may yield the greatest therapeutic benefits, due to the complexity of the in vivo system, it is difficult to simulate various conditions in vitro. Effectively reducing the number of immune microenvironments in glioma may be a more reasonable therapeutic strategy. However, a recent study on Liposomal honokiol (Lip-HNK) indicated that Lip-HNK repolarizes M2 macrophages into M1 phenotype, which effectively enhances the tumor inhibitory ability of GAM. This may be more reasonable and effective than simply inhibiting GAM. At present, Lip-HNK has entered the phase I clinical trial stage for glioma treatment, and various experiments for this drug are being improved step by step, and its further clinical therapeutic effect is also worth our expectation (45).
GAM recruitment, activation, and polarization
As mentioned above, 10-15% of brain tissue is normally composed of microglia. Effectively regulate the development and physiological functions of the central nervous system. As glioma pathogenesis progresses, weakening of blood-brain barrier function contributes to TME formation and significantly increased the proportion of GAM in TME (46).
Within the TME, a variety of factors such as cytokines and growth factors activate GAMs and influence cellular polarization. GAMs are generally classified into M0, M1 and M2 subtypes; M2 GAM can be subclassified into functionally different M2a, M2b and M2c cells. Interactions among the aforementioned GAMs phenotypes and glioma cells influence tumor cell invasion and migration, angiogenesis, the tumor-mesenchymal transition as well as immunosuppression (Figure 2) (47).
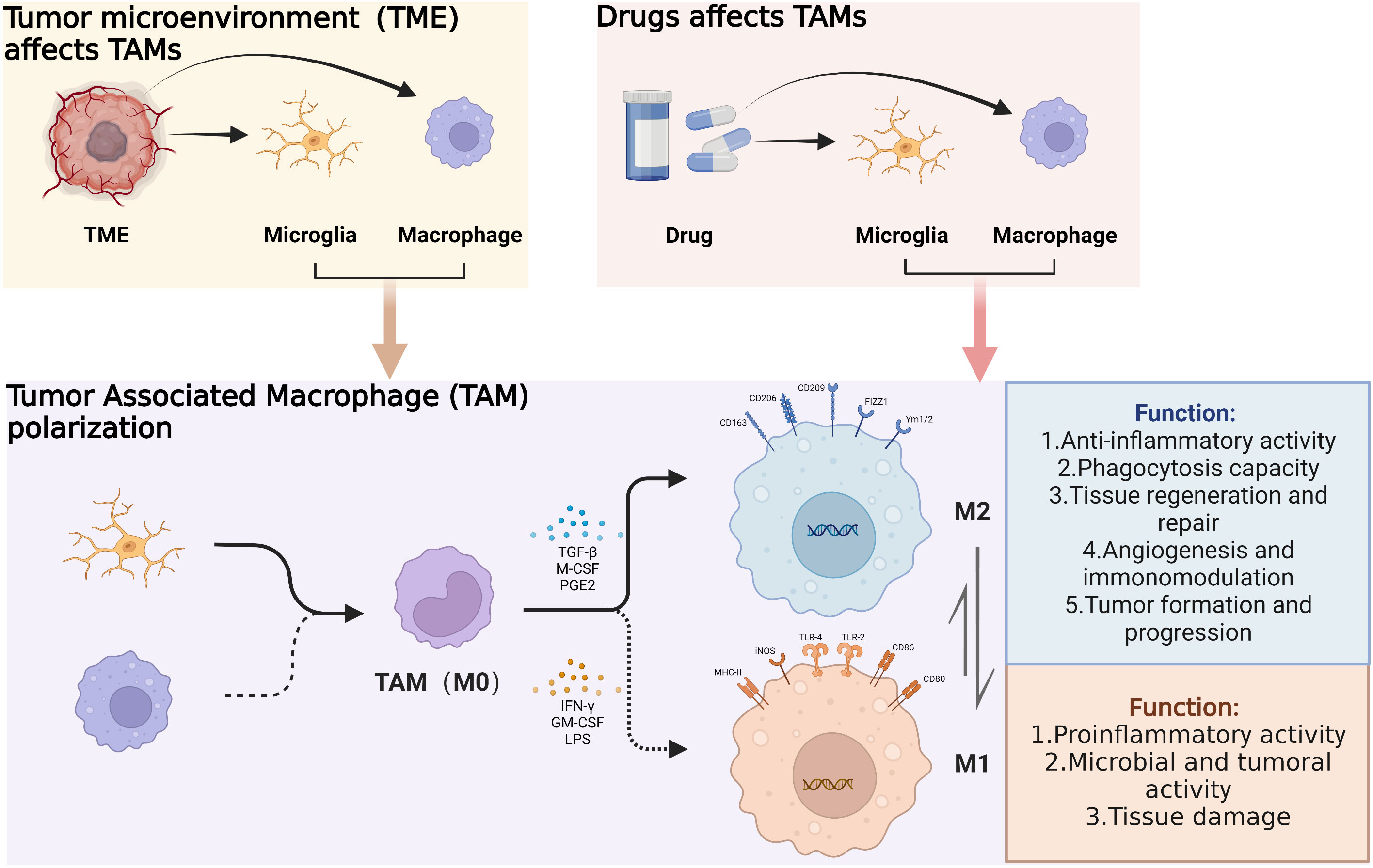
Figure 2 Under the influence of the glioma microenvironment and corresponding drugs, tumor-associated macrophages are recruited toward the tumor. And under the action of certain cytokines, the polarization direction of tumor-associated macrophages changes, resulting in different physiological functions. (By Biorender).
Among the primary factors influencing glioma pathogenesis, GAM accumulation within the TME has long been a research focus. Chemokines, complement receptor ligands and miRNAs all function to recruit macrophages toward the glioma (31, 48). Numerous studies of chemokines have revealed them to play a leading role in the directional migration of microglia. The most extensively studied of these have been CC chemokine ligand 2 (CCL2; MCP-1), stromal cell-derived factor 1 (SDF-1), colony stimulating factor 1 (CSF-1), granulocyte-macrophage colony stimulating factor (GM-CSF), tumor necrosis factor (TNF) and glial cell derived neurotrophic factor (GDNF). Some of these chemokines additionally influence subsequent activation and polarization of GAMs, thereby significantly affecting TME function (49). In this review, major chemokines previously identified to function in macrophage recruitment are summarized to provide foundations for future research directions.
Major immune cell recruitment factors within the glioma microenvironment
I. CCL2/CCR2 axis
Also known as MCP-1, CCL2 plays an important role in tumor growth and macrophage recruitment in the setting of a number of malignancies (Figure 3A) (50). For example, breast cancer, gastric cancer, ovarian cancer, etc. (51–53) CCL2 is also present in the central nervous system. A potent chemoattractant, CCL2 is produced by many central nervous system cells such as astrocytes, endothelial cells and microglia (54). Glioma cells also express high levels of CCL2 (17). This was reported to significantly positively correlate with GAM quantity within the glioma TME. However, whether CCL2 directly acts on tumor cells remains unclear. An earlier study of the U87 glioma cell line utilized flow cytometry to confirm that these cells lack CCR2, the CCL2 receptor. Moreover, CCL2 did not appear to markedly influence proliferation or migration of U87 cells (55). A different study of the U251 glioma cell line, however, suggested differently: after CCL2 knockdown, glioma cells exhibited significantly decreased proliferation and migration, and significantly increased apoptotic activity, as compared to control cells (56). Effects of CCL2 on glioma cells thus warrant further clarification.
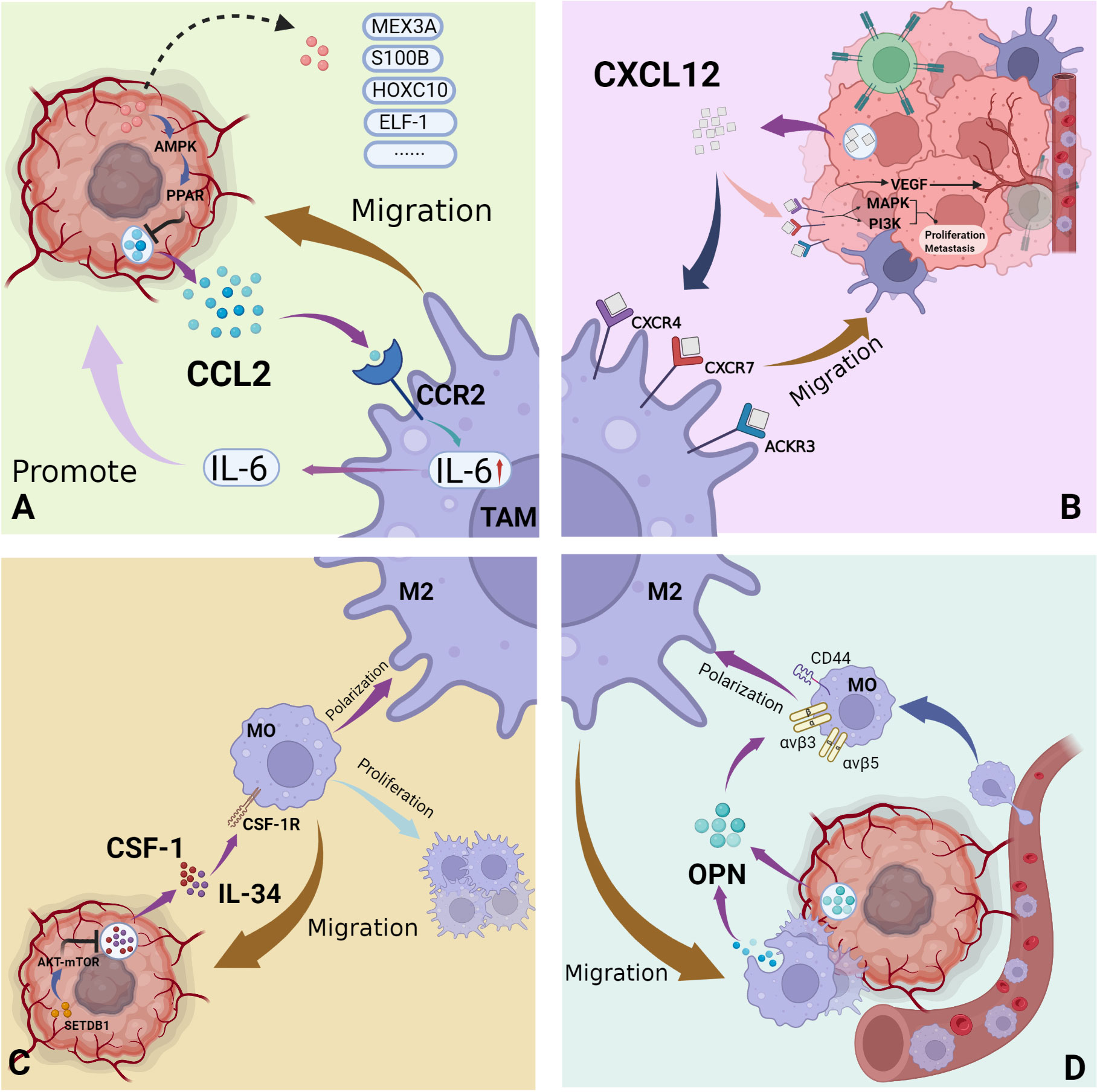
Figure 3 Major recruitment factors of GAMs in the glioma immune microenvironment. (A) CCL2 secreted by glioma cells acts on its receptor CCR2 on macrophages, effectively mediating the migration of macrophages to tumor sites and the secretion of tumor-promoting cytokines; (B) The three main receptors of CXCL12 secreted by gliomas: CXCR4, CXCR7, ACKR3, exist on the surface of both tumor cells and macrophages. Mediates the recruitment of macrophages, as well as tumor cell proliferation, metastasis and angiogenesis. (C) As ubiquitous cytokines, CSF-1 and IL-34 act on the receptor CSF-1R to mediate the recruitment of macrophages and the proliferation and differentiation of macrophages. (D) OPN is secreted by tumor cells and macrophages and induces the recruitment and polarization of macrophages. (By Biorender).
A number of molecules also influence macrophage infiltration of the central nervous system via interactions with CCL2 and thereby affect glioma pathogenesis. Among these, MEX3A expression was found to be significantly increased in the setting of glioma and significantly positively correlated with the degree of malignancy as well as poor prognosis. A likely downstream target of MEX3A, CCL2 is also regulated by this protein. Possible relevant pathways include PPARα/RXRα activation and AMPK signaling (57). Under CCL2 knockout conditions, the tumor-promoting effect of MEX3A was found to be significantly attenuated (56). Homeobox C10 (HOXC10), known to induce angiogenesis via VEGFR upregulation, is also highly expressed in the setting of glioma and exerts a similar regulatory effect on CCL2 (58). As HOXC10 knockdown was confirmed to inhibit CCL2 expression, it thus likely plays an important role in CCL2-mediated macrophage recruitment (59). Proteins such as ELF-1 and Notch1 also exert upstream regulatory effects on CCL2, thus affecting macrophage recruitment (60–62).
Upon CCL2 interaction with CCR2, release of IL-6 from microglia is promoted in addition to cellular recruitment. Greater IL-6 levels further increase TME microglia, reduce cytotoxic CD8+ T cells within the TME, and effectively promote malignant proliferation and metastasis (63). Interestingly, a number of studies have reported that CCL-7 (MCP-3) plays a more significant role in GAM infiltration in glioma as compared to CCL2 (64). However, a relatively early publication year of this study, as well as a lack of relevant follow-up research, necessitates verification.
II. The SDF-1 (CXCL12)-CXCR4/CXCR7 axis
Stromal cell-derived factor-1 (SDF-1), also known as chemokine CXC ligand 12 (CXCL12), is expressed and secreted by a variety of cells including myeloid, endothelial, epithelial and tumor cells (Figure 3B) (65). CXCR4, expressed primarily by monocytes and neutrophils in the immune microenvironment, is the primary receptor for CXCL12, but there are others (66). For example, CXCR7 and atypical chemokine receptor 3 (ACKR3) also bind CXCL12 and exert corresponding downstream regulatory effects. Signaling pathways such as the mitogen-activated protein kinase ACCRA (MAPK)/extracellular signal-regulated kinase (ERK) and phosphatidylinositol 3-kinase (PI3K)/protein kinase B (PKB) are activated under the influence of the CXCL12-CXCR4/CXCR7 axis, thus affecting cellular proliferation and metastasis (67, 68). The regulation of angiogenesis by the CXCL12-CXCR4/CXCR7 axis attracts increasing research attention. Studies have reported that the CXCL12/CXCR4 axis effectively upregulates VEGF expression within the TME, thereby promoting angiogenesis. Inhibition of VEGF alone, however, is known to increase CXCR4 expression. Existing experimental treatment methods therefore utilize CXCR4 and VEGF inhibitors in combination have achieved good therapeutic effect (66). The CXCL12-CXCR4/CXCR7 axis also influences resistance to radiotherapy and chemotherapy, immune cell infiltration into the TME and tumor stem cell proliferation. Although the relevant mechanisms of these phenomena are not described in detail in this review, such activity greatly affects malignant pathogenesis (69–73).
In glioma, CXCL12-CXCR4/CXCR7 was reported to be significantly increased. Indeed, increased expression of CXCR4 is considered to be one hallmark of malignant glioblastoma (74). Its ligand CXCL12 was further identified as a potential biomarker of cancer stem cell resistance to radiotherapy, likely via induction of autophagy among malignant cells (75, 76). CXCR4 was preferentially expressed in glioma stem cells. As the differentiation degree of glioma increased, the expression level of CXCR4 decreased gradually, instead, the expression level of CXCR7 increased (77, 78). This axis is involved in malignant processes such as tumor angiogenesis, inflammatory response, immunosuppression and reprogramming (79). Recruitment of monocytes is one of the many tumor-promoting effects exerted by CXCR4.
A study of tumor-associated fibroblast (CAF)-induced monocyte migration revealed that CXCL12-supplemented media significantly increased monocyte chemotaxis (80). In glioma, the CXCL12-CXCR4/CXCR7 axis similarly plays a decisive role in macrophage recruitment (77). CXCR4 and CXCR7 are highly expressed on the surface of both microglia and glioma cells (78). As CXCL12 concentration within the TME increases, microglia (mainly M2 macrophages) are increasingly recruited toward the vicinity of the malignancy.
Hypoxia, an essential characteristic of the TME, results in enhanced resistance to damage by tumor cells due to an adaptive modulatory response (81). In the setting of glioma, hypoxia further promotes malignant pathogenesis as well as drug resistance (82). The hypoxic microenvironment upregulates members of the hypoxia-inducible factor (HIF) family, which influence glioma phenotype via effects on angiogenesis, cellular resistance to therapy and enhanced metastasis (83). Members of the HIF family also affect macrophage recruitment via CXCL12 regulation. An earlier study showed that HIF-1α, as an upstream regulator of CXCL12, effectively influences CXCL12 release (84).While HIF-1α regulates CXCL12, it is also activated by CXCL12 via RAS/ERK1-2 and PI3K/AKT signaling (85). In another study of a mouse model of astrocytoma revealed that SDF-1 (CXCL12) effectively promotes GAM recruitment to the vicinity of the malignancy in a concentration-dependent fashion within the hypoxic TME. Co-localization of HIF-1 and CXCL12 by immunofluorescence subsequently complemented the aforementioned findings (86).
In the setting of the hypoxic TME, HIF members play regulatory roles in the CXCL12-CXCR4/CXCR7 axis along with other cytokines such as VEGF and CD26 (87, 88). A 2006 study of the U251 glioma cell line revealed that VEGF increases expression of both SDF-1 and CXCR4 mRNA, thus effectively improving metastatic capabilities (89). In addition, CXCL12 induces macrophage polarization toward the M2 subtype, thus further promoting malignant process (73).
III. The CSF-1/IL-34-CSF-1R axis
Colony-stimulating factor 1 (CSF-1) and IL-34 are ubiquitous cytokines of great significance to the regulation of monocyte function. Their common ligand, CSF-1R, is a transmembrane tyrosine kinase receptor widely expressed on the surface of cells such as monocytes and marrow-derived macrophages. Ligand-receptor interaction results in effective promotion of monocyte proliferation and differentiation via downstream signaling pathways such as JAK-STAT and PI3K/AKT (Figure 3C) (90–93).
Expression of CSF-1 and IL-34 in various tumors is significantly increased. In breast cancer, significant upregulation of CSF-1 effectively promotes migration of non-resident macrophages to the TME and induces their polarization toward the M2 subtype (94). In melanoma, the ERK pathway-mediated RUNX1 transcription factor promotes CSF-1R expression, increasing tumor cell survival and malignancy (95).
In normal brain tissue, CSF-1 and IL-34 are respectively secreted by resident microglia and neurons (96). Both cytokines significantly affect microglial development, maturation and function (97). However, in glioma, especially glioblastoma, these cytokines influence tumor progression. While CSF-1R, CSF-1 and IL-34 directly support tumor cell growth, CSF-1, highly expressed by glioma cells, effectively promotes macrophage recruitment and indirectly promotes malignant pathogenesis via GAM interactions (98). One study of the SETDB1 enzyme revealed significantly increased expression in glioma and resultant promotion of CSF-1 secretion via AKT/mTOR signaling, thereby enhancing macrophage recruitment and polarization (99). Inhibition of CSF-1R signaling in vivo was similarly reported to reduce macrophage infiltration of the TME, further validating the roles CSF-1 and IL-34 play in immune cell recruitment (100).
A number of studies have investigated macrophage recruitment by CSF-1. Although administration of anti-CSF-1R therapy to glioma experimental animals exhibited an excellent initial reaction, nearly half of experimental animals eventually developed drug resistance and tumor recurrence. PTEN/PI3K pathway activation and increased levels of IGF-1 were found in the drug-resistant glioma setting. As such, targeting of the above two mechanisms with a combined therapeutic strategy involving CSF-1R inhibition was reported to improve therapeutic outcome (101). Although these findings suggest that CSF-1 and IL-34 are susceptible to regulation, anti-CSF-1R therapy warrants further study for effective use in glioma treatment.
IV. OPN/SPP1
Osteopontin (OPN), also known as secreted phosphoprotein-1(SPP1), is highly expressed in microglia of the early postnatal brain and injured adults (102). It is an exocrine immunoregulatory protein involved in the inflammatory process, and also expressed in fibroblasts, dendritic cells and macrophages. It is involved in both physiological and pathological processes such as bone formation, osteoarthritis, obesity and Alzheimer’s disease, as well as carcinogenesis and metastasis (103–106). In the central nervous system, OPN effectively monitors acute or chronic injuries in the central nervous system, such as inflammation (102). In various pathological settings, OPN plays roles in mediating inflammation, inducing immune cell proliferation and attracting mature macrophage migration to the vicinity of the lesion (Figure 3D) (103, 107, 108).
A component of the extracellular matrix, OPN primarily binds three receptors relevant in the TME including α4β1, αvβ3 and CD44. Activation of corresponding pathways promotes macrophage recruitment, angiogenesis and T cell inhibition, thereby promoting malignant progression (109). Increased expression of OPN in glioma was reported to significantly positively correlate with the degree of malignancy (110). It has been reported that the increased expression of OPN in glioma is significantly positively correlated with the degree of malignancy, and its expression is effective in maintaining the survival and angiogenesis of glioma cells. Furthermore, OPN increases secretion of metalloproteinase-2 (MMP-2), thereby promoting glioma metastasis. OPN also effectively reduces glioma cell sensitivity to the immune system (106, 111). Selective inhibition of OPN expression in glioma was noted to significantly reduce malignant cell proliferation (112).
As previously mentioned, in various diseases, the secreted glycoprotein OPN effectively induces macrophage migration to the lesion site in a dose-dependent manner (104). Recruitment of M0 and M2 (but not M1) macrophages to tumor tissue via αvβ5-integrin signaling facilitates a continued increase in OPN secretion and further enhances macrophage recruitment (111). Interestingly, existing literature is inconsistent regarding effects of OPN on macrophage polarization within the TME. Studies focusing on obesity, liver cancer and colitis underscored that apart from promoting macrophage recruitment, OPN effectively induces macrophage polarization to the M2 phenotype via the αvβ3 and CD44 receptors, and activates the downstream STAT3/PPARg signaling pathway (103, 113, 114). However, a glioma study reported that despite regulation of macrophage recruitment via CD44, OPN does not significantly influence macrophage polarization (111). Mechanisms relevant to the function of OPN in malignancy thus require further study.
OPN offers unique immunotherapeutic prospects. After OPN knockout, significantly decreased levels of M2 macrophages and tumor cell expression of PD-L1 were reported. These phenomena are not due to direct effects of OPN on tumor cells, but rather due to indirect regulatory effects that induce secretion of CSF-1 by macrophages via the PI3K/AKT/NF-κB/p65 pathway. After CSF-1R inhibition, anti-PD-L1 treatment in a mouse model of OPN-expressing malignancy was significantly improved (113). However, these findings are limited to liver cancer, and CSF-1R inhibition therapy needs further investigation.
Significance of macrophage-targeting treatment in the management of glioma
Although glioma treatment has significantly improved over recent decades, surgical resection, adjuvant radiotherapy and temozolomide-based chemotherapy remain gold-standard treatments (115). Median patient survival time, however, has continued to remain relatively low (4). Furthermore, temozolomide treatment was reported to negatively influence myeloid-derived suppressor cells within the glioma immune TME, such as by increasing M2 macrophage phenotypic markers (115). As such, strategies that target GAM recruitment factors in the setting of glioma continue to draw increasing research interest.
Studies have confirmed that anti-CCL2 antibody treatment of glioma effectively reduces immune cell accumulation within the TME, improves the therapeutic effect of temozolomide and prolongs survival among tumor-bearing mice (116). Inhibition of CCL2 was also found to significantly reduce angiogenesis (117). In a study centered on the combination of CCR2 inhibition and PD-1 blocking, the use of a CCR2 antagonist (CCX872) in combination with anti-PD-1 therapy further improved the median survival of tumor-bearing mice (118).
Focus on the therapeutic potential of the CXCL12-CXCR4/CXCR7 axis revealed FTY720, an immunomodulatory drug used in multiple sclerosis treatment, to possess therapeutic activity against various tumors and improving sensitivity to temozolomide therapy (119, 120). Importantly, FTY720 was found to effectively regulate interactions between glioma cells and GAMs primarily via promotion of CXCR4 uptake and inhibition of MAPK-mediated IL-6 secretion.
Significant decreases in glioma volume after administration of CSF-1R inhibitors have also been reported (120, 121). As CSF-1R promotes maintenance of malignant cellular characteristics in the setting of glioma via ERK1/2 activation, use of the ERK1/2 inhibitor SCH772984 was reported to effectively reduce subsequent malignant progression (122).
Given the role of OPN/SPP1 on macrophage recruitment and promotion of malignancy, OPN inhibition and antagonism of its corresponding receptors (e.g. CD44) are potential therapeutic strategies that warrant investigation in the context of glioma. The proliferative capacity of glioma cells was reported to be significantly reduced in the setting of CD44 knockout and OPN silencing (123). Relevant upstream and downstream regulatory mechanisms similarly warrant detailed study.
Conclusion
As knowledge concerning glioma pathology has advanced, the role played by GAMs has drawn increasing interest. Although significant progress regarding GAM origin, polarization and function has been made, mechanisms involved in GAM recruitment remain unclear. Here, we review known GAM functions with the aim of facilitating development of future research focused on elucidation of macrophage recruitment mechanisms as well as effective therapeutic strategies.
Author contributions
XZ and GJ performed conception, data acquisition, data analysis and wrote the manuscript. FL and JZ reviewed and edited the manuscript, secured funding and supervision. All authors contributed to the article and approved the submitted version.
Funding
This work was supported by grants from the Beijing Natural Science Foundation Program and Scientific Research Key Program of the Beijing Municipal Commission of Education (KZ202010025034), Capital’s Funds for Health Improvement and Research (CFH, 2020-1-1071), the Natural Science Foundation of Beijing Municipality (No. 7202020), and the Beijing Laboratory of Biomedical Materials Foundation.
Acknowledgments
We thank the Brain Tumor Research Center (Beijing Laboratory of Biomedical Materials) at Beijing Neurosurgical Institute for support.
Conflict of interest
The authors declare that the research was conducted in the absence of any commercial or financial relationships that could be construed as a potential conflict of interest.
The reviewer WL declared a shared affiliation with the authors to the handling editor at the time of review.
Publisher’s note
All claims expressed in this article are solely those of the authors and do not necessarily represent those of their affiliated organizations, or those of the publisher, the editors and the reviewers. Any product that may be evaluated in this article, or claim that may be made by its manufacturer, is not guaranteed or endorsed by the publisher.
References
1. Weller M, Wick W, Aldape K, Brada M, Berger M, Pfister SM, et al. Glioma. Nat Rev Dis Primers. (2015) 1:15017. doi: 10.1038/nrdp.2015.17
2. Stupp R, Mason WP, van den Bent MJ, Weller M, Fisher B, Taphoorn MJ, et al. Radiotherapy plus concomitant and adjuvant temozolomide for glioblastoma. N Engl J Med (2005) 352(10):987–96. doi: 10.1056/NEJMoa043330
3. Li S, Wang C, Chen J, Lan Y, Zhang W, Kang Z, et al. Signaling pathways in brain tumors and therapeutic interventions. Signal Transduct Target Ther (2023) 8(1):8. doi: 10.1038/s41392-022-01260-z
4. Chen J, Li Y, Yu TS, McKay RM, Burns DK, Kernie SG, et al. A restricted cell population propagates glioblastoma growth after chemotherapy. Nature (2012) 488(7412):522–6. doi: 10.1038/nature11287
5. Lim M, Xia Y, Bettegowda C, Weller M. Current state of immunotherapy for glioblastoma. Nat Rev Clin Oncol (2018) 15(7):422–42. doi: 10.1038/s41571-018-0003-5
6. Gutmann DH, McLellan MD, Hussain I, Wallis JW, Fulton LL, Fulton RS, et al. Somatic neurofibromatosis type 1 (NF1) inactivation characterizes NF1-associated pilocytic astrocytoma. Genome Res (2013) 23(3):431–9. doi: 10.1101/gr.142604.112
7. Morantz RA, Wood GW, Foster M, Clark M, Gollahon K. Macrophages in experimental and human brain tumors. part 1: Studies of the macrophage content of experimental rat brain tumors of varying immunogenicity. J Neurosurg (1979) 50(3):298–304. doi: 10.3171/jns.1979.50.3.0298
8. Friedrich M, Hahn M, Michel J, Sankowski R, Kilian M, Kehl N, et al. Dysfunctional dendritic cells limit antigen-specific T cell response in glioma. Neuro Oncol (2022) 25(2):263–76. doi: 10.1093/neuonc/noac138
9. Mishalian I, Bayuh R, Levy L, Zolotarov L, Michaeli J, Fridlender ZG. Tumor-associated neutrophils (TAN) develop pro-tumorigenic properties during tumor progression. Cancer Immunol Immunother. (2013) 62(11):1745–56. doi: 10.1007/s00262-013-1476-9
10. Granot Z, Henke E, Comen EA, King TA, Norton L, Benezra R. Tumor entrained neutrophils inhibit seeding in the premetastatic lung. Cancer Cell (2011) 20(3):300–14. doi: 10.1016/j.ccr.2011.08.012
11. Lupo KB, Matosevic S. CD155 immunoregulation as a target for natural killer cell immunotherapy in glioblastoma. J Hematol Oncol (2020) 13(1):76. doi: 10.1186/s13045-020-00913-2
12. Mathewson ND, Ashenberg O, Tirosh I, Gritsch S, Perez EM, Marx S, et al. Inhibitory CD161 receptor identified in glioma-infiltrating T cells by single-cell analysis. Cell (2021) 184(5):1281–1298 e1226. doi: 10.1016/j.cell.2021.01.022
13. Bowman RL, Klemm F, Akkari L, Pyonteck SM, Sevenich L, Quail DF, et al. Macrophage ontogeny underlies differences in tumor-specific education in brain malignancies. Cell Rep (2016) 17(9):2445–59. doi: 10.1016/j.celrep.2016.10.052
14. Hinshaw DC, Shevde LA. The tumor microenvironment innately modulates cancer progression. Cancer Res (2019) 79(18):4557–66. doi: 10.1158/0008-5472.CAN-18-3962
15. Nielsen SR, Strobech JE, Horton ER, Jackstadt R, Laitala A, Bravo MC, et al. Suppression of tumor-associated neutrophils by lorlatinib attenuates pancreatic cancer growth and improves treatment with immune checkpoint blockade. Nat Commun (2021) 12(1):3414. doi: 10.1038/s41467-021-23731-7
16. Wang H, Franco F, Tsui YC, Xie X, Trefny MP, Zappasodi R, et al. CD36-mediated metabolic adaptation supports regulatory T cell survival and function in tumors. Nat Immunol (2020) 21(3):298–308. doi: 10.1038/s41590-019-0589-5
17. Chen Z, Feng X, Herting CJ, Garcia VA, Nie K, Pong WW, et al. Cellular and molecular identity of tumor-associated macrophages in glioblastoma. Cancer Res (2017) 77(9):2266–78. doi: 10.1158/0008-5472.CAN-16-2310
18. Thomas AA, Fisher JL, Rahme GJ, Hampton TH, Baron U, Olek S, et al. Regulatory T cells are not a strong predictor of survival for patients with glioblastoma. Neuro Oncol (2015) 17(6):801–9. doi: 10.1093/neuonc/nou363
19. Ugel S, De Sanctis F, Mandruzzato S, Bronte V. Tumor-induced myeloid deviation: when myeloid-derived suppressor cells meet tumor-associated macrophages. J Clin Invest. (2015) 125(9):3365–76. doi: 10.1172/JCI80006
20. Hambardzumyan D, Gutmann DH, Kettenmann H. The role of microglia and macrophages in glioma maintenance and progression. Nat Neurosci (2016) 19(1):20–7. doi: 10.1038/nn.4185
21. Song M, He J, Pan QZ, Yang J, Zhao J, Zhang YJ, et al. Cancer-associated fibroblast-mediated cellular crosstalk supports hepatocellular carcinoma progression. Hepatology (2021) 73(5):1717–35. doi: 10.1002/hep.31792
22. Zhang M, Di Martino JS, Bowman RL, Campbell NR, Baksh SC, Simon-Vermot T, et al. Adipocyte-derived lipids mediate melanoma progression via FATP proteins. Cancer Discovery (2018) 8(8):1006–25. doi: 10.1158/2159-8290.CD-17-1371
23. Liu YT, Goel S, Kai M, Moran Guerrero JA, Nguyen T, Mai J, et al. Seed- and soil-dependent differences in murine breast tumor microenvironments dictate anti-PD-L1 IgG delivery and therapeutic efficacy. Pharmaceutics (2021) 13(4):530. doi: 10.3390/pharmaceutics13040530
24. Sathe A, Grimes SM, Lau BT, Chen J, Suarez C, Huang RJ, et al. Single-cell genomic characterization reveals the cellular reprogramming of the gastric tumor microenvironment. Clin Cancer Res (2020) 26(11):2640–53. doi: 10.1158/1078-0432.CCR-19-3231
25. Watson MJ, Vignali PDA, Mullett SJ, Overacre-Delgoffe AE, Peralta RM, Grebinoski S, et al. Metabolic support of tumour-infiltrating regulatory T cells by lactic acid. Nature (2021) 591(7851):645–51. doi: 10.1038/s41586-020-03045-2
26. Saha D, Martuza RL, Rabkin SD. Macrophage polarization contributes to glioblastoma eradication by combination immunovirotherapy and immune checkpoint blockade. Cancer Cell (2017) 32(2):253–267.e255. doi: 10.1016/j.ccell.2017.07.006
27. Angelin A, Gil-de-Gomez L, Dahiya S, Jiao J, Guo L, Levine MH, et al. Foxp3 reprograms T cell metabolism to function in low-glucose, high-lactate environments. Cell Metab (2017) 25(6):1282–1293.e1287. doi: 10.1016/j.cmet.2016.12.018
28. Tammela T, Sanchez-Rivera FJ, Cetinbas NM, Wu K, Joshi NS, Helenius K, et al. A wnt-producing niche drives proliferative potential and progression in lung adenocarcinoma. Nature (2017) 545(7654):355–9. doi: 10.1038/nature22334
29. Preusser M, Lim M, Hafler DA, Reardon DA, Sampson JH. Prospects of immune checkpoint modulators in the treatment of glioblastoma. Nat Rev Neurol (2015) 11(9):504–14. doi: 10.1038/nrneurol.2015.139
30. Yu-Ju Wu C, Chen CH, Lin CY, Feng LY, Lin YC, Wei KC, et al. CCL5 of glioma-associated microglia/macrophages regulates glioma migration and invasion via calcium-dependent matrix metalloproteinase 2. Neuro Oncol (2020) 22(2):253–66. doi: 10.1093/neuonc/noz189
31. Zhou W, Ke SQ, Huang Z, Flavahan W, Fang X, Paul J, et al. Periostin secreted by glioblastoma stem cells recruits M2 tumour-associated macrophages and promotes malignant growth. Nat Cell Biol (2015) 17(2):170–82. doi: 10.1038/ncb3090
32. Kierdorf K, Katzmarski N, Haas CA, Prinz M. Bone marrow cell recruitment to the brain in the absence of irradiation or parabiosis bias. PloS One (2013) 8(3):e58544. doi: 10.1371/journal.pone.0058544
33. Badie B, Schartner JM. Flow cytometric characterization of tumor-associated macrophages in experimental gliomas. Neurosurgery (2000) 46(4):957–61. doi: 10.1097/00006123-200004000-00035
34. Muller A, Brandenburg S, Turkowski K, Muller S, Vajkoczy P. Resident microglia, and not peripheral macrophages, are the main source of brain tumor mononuclear cells. Int J Cancer. (2015) 137(2):278–88. doi: 10.1002/ijc.29379
35. Wei J, Chen P, Gupta P, Ott M, Zamler D, Kassab C, et al. Immune biology of glioma-associated macrophages and microglia: functional and therapeutic implications. Neuro Oncol (2020) 22(2):180–94. doi: 10.1093/neuonc/noz212
36. Mizutani M, Pino PA, Saederup N, Charo IF, Ransohoff RM, Cardona AE. The fractalkine receptor but not CCR2 is present on microglia from embryonic development throughout adulthood. J Immunol (2012) 188(1):29–36. doi: 10.4049/jimmunol.1100421
37. Hutter G, Theruvath J, Graef CM, Zhang M, Schoen MK, Manz EM, et al. Microglia are effector cells of CD47-SIRPalpha antiphagocytic axis disruption against glioblastoma. Proc Natl Acad Sci U S A. (2019) 116(3):997–1006. doi: 10.1073/pnas.1721434116
38. Yin J, Kim SS, Choi E, Oh YT, Lin W, Kim TH, et al. ARS2/MAGL signaling in glioblastoma stem cells promotes self-renewal and M2-like polarization of tumor-associated macrophages. Nat Commun (2020) 11(1):2978. doi: 10.1038/s41467-020-16789-2
39. Ye XZ, Xu SL, Xin YH, Yu SC, Ping YF, Chen L, et al. Tumor-associated microglia/macrophages enhance the invasion of glioma stem-like cells via TGF-beta1 signaling pathway. J Immunol (2012) 189(1):444–53. doi: 10.4049/jimmunol.1103248
40. Hori T, Sasayama T, Tanaka K, Koma Y-I, Nishihara M, Tanaka H, et al. Tumor-associated macrophage related interleukin-6 in cerebrospinal fluid as a prognostic marker for glioblastoma. J Clin Neurosci (2019) 68:281–9. doi: 10.1016/j.jocn.2019.07.020
41. Chen P, Zhao D, Li J, Liang X, Li J, Chang A, et al. Symbiotic macrophage-glioma cell interactions reveal synthetic lethality in PTEN-null glioma. Cancer Cell (2019) 35(6):868–884.e866. doi: 10.1016/j.ccell.2019.05.003
42. Li J, Kaneda MM, Ma J, Li M, Shepard RM, Patel K, et al. PI3Kgamma inhibition suppresses microglia/TAM accumulation in glioblastoma microenvironment to promote exceptional temozolomide response. Proc Natl Acad Sci U S A. (2021) 118(16):e2009290118. doi: 10.1073/pnas.2009290118
43. Muller S, Kohanbash G, Liu SJ, Alvarado B, Carrera D, Bhaduri A, et al. Single-cell profiling of human gliomas reveals macrophage ontogeny as a basis for regional differences in macrophage activation in the tumor microenvironment. Genome Biol (2017) 18(1):234. doi: 10.1186/s13059-017-1362-4
44. Lisi L, Laudati E, Navarra P, Dello Russo C. The mTOR kinase inhibitors polarize glioma-activated microglia to express a M1 phenotype. J Neuroinflammation. (2014) 11:125. doi: 10.1186/1742-2094-11-125
45. Li S, Li L, Chen J, Fan Y, Wang C, Du Y, et al. Liposomal honokiol inhibits glioblastoma growth through regulating macrophage polarization. Ann Transl Med (2021) 9(22):1644. doi: 10.21037/atm-21-1836
46. Griveau A, Seano G, Shelton SJ, Kupp R, Jahangiri A, Obernier K, et al. A glial signature and Wnt7 signaling regulate glioma-vascular interactions and tumor microenvironment. Cancer Cell (2018) 33(5):874–889.e877. doi: 10.1016/j.ccell.2018.03.020
47. Vitale I, Manic G, Coussens LM, Kroemer G, Galluzzi L. Macrophages and metabolism in the tumor microenvironment. Cell Metab (2019) 30(1):36–50. doi: 10.1016/j.cmet.2019.06.001
48. Frank AC, Ebersberger S, Fink AF, Lampe S, Weigert A, Schmid T, et al. Apoptotic tumor cell-derived microRNA-375 uses CD36 to alter the tumor-associated macrophage phenotype. Nat Commun (2019) 10(1):1135. doi: 10.1038/s41467-019-08989-2
49. Sierra-Filardi E, Nieto C, Dominguez-Soto A, Barroso R, Sanchez-Mateos P, Puig-Kroger A, et al. CCL2 shapes macrophage polarization by GM-CSF and m-CSF: identification of CCL2/CCR2-dependent gene expression profile. J Immunol (2014) 192(8):3858–67. doi: 10.4049/jimmunol.1302821
50. Li X, Yao W, Yuan Y, Chen P, Li B, Li J, et al. Targeting of tumour-infiltrating macrophages via CCL2/CCR2 signalling as a therapeutic strategy against hepatocellular carcinoma. Gut (2017) 66(1):157–67. doi: 10.1136/gutjnl-2015-310514
51. Rogic A, Pant I, Grumolato L, Fernandez-Rodriguez R, Edwards A, Das S, et al. High endogenous CCL2 expression promotes the aggressive phenotype of human inflammatory breast cancer. Nat Commun (2021) 12(1):6889. doi: 10.1038/s41467-021-27108-8
52. Ma L, Jiang Y, Wu N. Long non-coding RNA CCL2 promoted gastric cancer function via miR-128/PARP2 signal pathway. Bioengineered (2022) 13(1):1602–11. doi: 10.1080/21655979.2021.2020548
53. Miyamoto T, Murakami R, Hamanishi J, Tanigaki K, Hosoe Y, Mise N, et al. B7-H3 suppresses antitumor immunity via the CCL2-CCR2-M2 macrophage axis and contributes to ovarian cancer progression. Cancer Immunol Res (2022) 10(1):56–69. doi: 10.1158/2326-6066.CIR-21-0407
54. Cherry JD, Meng G, Daley S, Xia W, Svirsky S, Alvarez VE, et al. CCL2 is associated with microglia and macrophage recruitment in chronic traumatic encephalopathy. J Neuroinflammation. (2020) 17(1):370. doi: 10.1186/s12974-020-02036-4
55. Zhang J, Sarkar S, Cua R, Zhou Y, Hader W, Yong VW. A dialog between glioma and microglia that promotes tumor invasiveness through the CCL2/CCR2/interleukin-6 axis. Carcinogenesis (2012) 33(2):312–9. doi: 10.1093/carcin/bgr289
56. Yang C, Zhan H, Zhao Y, Wu Y, Li L, Wang H. MEX3A contributes to development and progression of glioma through regulating cell proliferation and cell migration and targeting CCL2. Cell Death Dis (2021) 12(1):14. doi: 10.1038/s41419-020-03307-x
57. Jordan S, Tung N, Casanova-Acebes M, Chang C, Cantoni C, Zhang D, et al. Dietary intake regulates the circulating inflammatory monocyte pool. Cell (2019) 178(5):1102–1114 e1117. doi: 10.1016/j.cell.2019.07.050
58. Tan Z, Chen K, Wu W, Zhou Y, Zhu J, Wu G, et al. Overexpression of HOXC10 promotes angiogenesis in human glioma via interaction with PRMT5 and upregulation of VEGFA expression. Theranostics (2018) 8(18):5143–58. doi: 10.7150/thno.27310
59. Li S, Zhang W, Wu C, Gao H, Yu J, Wang X, et al. HOXC10 promotes proliferation and invasion and induces immunosuppressive gene expression in glioma. FEBS J (2018) 285(12):2278–91. doi: 10.1111/febs.14476
60. Du S, Wu S, Feng X, Wang B, Xia S, Liang L, et al. A nerve injury-specific long noncoding RNA promotes neuropathic pain by increasing Ccl2 expression. J Clin Invest. (2022) 132(13):e153563. doi: 10.1172/JCI153563
61. Geng Y, Fan J, Chen L, Zhang C, Qu C, Qian L, et al. A notch-dependent inflammatory feedback circuit between macrophages and cancer cells regulates pancreatic cancer metastasis. Cancer Res (2021) 81(1):64–76. doi: 10.1158/0008-5472.CAN-20-0256
62. Guo H, Wang F, Diao Y, Zhang Z, Chen Q, Qian CN, et al. Knockdown of Notch1 inhibits nasopharyngeal carcinoma cell growth and metastasis via downregulation of CCL2, CXCL16, and uPA. Mol Carcinog. (2019) 58(10):1886–96. doi: 10.1002/mc.23082
63. Yang F, He Z, Duan H, Zhang D, Li J, Yang H, et al. Synergistic immunotherapy of glioblastoma by dual targeting of IL-6 and CD40. Nat Commun (2021) 12(1):3424. doi: 10.1038/s41467-021-23832-3
64. Okada M, Saio M, Kito Y, Ohe N, Yano H, Yoshimura S, et al. Tumor-associated macrophage/microglia infiltration in human gliomas is correlated with MCP-3, but not MCP-1. Int J Oncol (2009) 34(6):1621–7. doi: 10.3892/ijo_00000292
65. Alghamri MS, Banerjee K, Mujeeb AA, Mauser A, Taher A, Thalla R, et al. Systemic delivery of an adjuvant CXCR4-CXCL12 signaling inhibitor encapsulated in synthetic protein nanoparticles for glioma immunotherapy. ACS Nano. (2022) 16(6):8729–50. doi: 10.1021/acsnano.1c07492
66. Jung K, Heishi T, Incio J, Huang Y, Beech EY, Pinter M, et al. Targeting CXCR4-dependent immunosuppressive Ly6C(low) monocytes improves antiangiogenic therapy in colorectal cancer. Proc Natl Acad Sci U S A. (2017) 114(39):10455–60. doi: 10.1073/pnas.1710754114
67. Janssens R, Struyf S, Proost P. The unique structural and functional features of CXCL12. Cell Mol Immunol (2018) 15(4):299–311. doi: 10.1038/cmi.2017.107
68. Ma J, Sun X, Wang Y, Chen B, Qian L, Wang Y. Fibroblast-derived CXCL12 regulates PTEN expression and is associated with the proliferation and invasion of colon cancer cells via PI3k/Akt signaling. Cell Commun Signal (2019) 17(1):119. doi: 10.1186/s12964-019-0432-5
69. Heckmann D, Maier P, Laufs S, Li L, Sleeman JP, Trunk MJ, et al. The disparate twins: A comparative study of CXCR4 and CXCR7 in SDF-1alpha-induced gene expression, invasion and chemosensitivity of colon cancer. Clin Cancer Res (2014) 20(3):604–16. doi: 10.1158/1078-0432.CCR-13-0582
70. Goffart N, Lombard A, Lallemand F, Kroonen J, Nassen J, Di Valentin E, et al. CXCL12 mediates glioblastoma resistance to radiotherapy in the subventricular zone. Neuro Oncol (2017) 19(1):66–77. doi: 10.1093/neuonc/now136
71. Yu X, Wang D, Wang X, Sun S, Zhang Y, Wang S, et al. CXCL12/CXCR4 promotes inflammation-driven colorectal cancer progression through activation of RhoA signaling by sponging miR-133a-3p. J Exp Clin Cancer Res (2019) 38(1):32. doi: 10.1186/s13046-018-1014-x
72. Durr C, Pfeifer D, Claus R, Schmitt-Graeff A, Gerlach UV, Graeser R, et al. CXCL12 mediates immunosuppression in the lymphoma microenvironment after allogeneic transplantation of hematopoietic cells. Cancer Res (2010) 70(24):10170–81. doi: 10.1158/0008-5472.CAN-10-1943
73. Wang D, Wang X, Si M, Yang J, Sun S, Wu H, et al. Exosome-encapsulated miRNAs contribute to CXCL12/CXCR4-induced liver metastasis of colorectal cancer by enhancing M2 polarization of macrophages. Cancer Lett (2020) 474:36–52. doi: 10.1016/j.canlet.2020.01.005
74. Truong D, Fiorelli R, Barrientos ES, Melendez EL, Sanai N, Mehta S, et al. A three-dimensional (3D) organotypic microfluidic model for glioma stem cells - vascular interactions. Biomaterials (2019) 198:63–77. doi: 10.1016/j.biomaterials.2018.07.048
75. Trautmann F, Cojoc M, Kurth I, Melin N, Bouchez LC, Dubrovska A, et al. CXCR4 as biomarker for radioresistant cancer stem cells. Int J Radiat Biol (2014) 90(8):687–99. doi: 10.3109/09553002.2014.906766
76. Wang Y, Gan G, Wang B, Wu J, Cao Y, Zhu D, et al. Cancer-associated fibroblasts promote irradiated cancer cell recovery through autophagy. EBioMedicine (2017) 17:45–56. doi: 10.1016/j.ebiom.2017.02.019
77. Mercurio L, Ajmone-Cat MA, Cecchetti S, Ricci A, Bozzuto G, Molinari A., et al. Targeting CXCR4 by a selective peptide antagonist modulates tumor microenvironment and microglia reactivity in a human glioblastoma model. J Exp Clin Cancer Res (2016) 35:55. doi: 10.1186/s13046-016-0326-y
78. Hattermann K, Held-Feindt J, Lucius R, Muerkoster SS, Penfold ME, Schall TJ, et al. The chemokine receptor CXCR7 is highly expressed in human glioma cells and mediates antiapoptotic effects. Cancer Res (2010) 70(8):3299–308. doi: 10.1158/0008-5472.CAN-09-3642
79. Liu Y, Cao X. Characteristics and significance of the pre-metastatic niche. Cancer Cell (2016) 30(5):668–81. doi: 10.1016/j.ccell.2016.09.011
80. Li X, Bu W, Meng L, Liu X, Wang S, Jiang L, et al. CXCL12/CXCR4 pathway orchestrates CSC-like properties by CAF recruited tumor associated macrophage in OSCC. Exp Cell Res (2019) 378(2):131–8. doi: 10.1016/j.yexcr.2019.03.013
81. Lodhi T, Song YP, West C, Hoskin P, Choudhury A. Hypoxia and its modification in bladder cancer: Current and future perspectives. Clin Oncol (R Coll Radiol). (2021) 33(6):376–90. doi: 10.1016/j.clon.2021.03.001
82. Su H, Zou D, Sun Y, Dai Y. Hypoxia-associated circDENND2A promotes glioma aggressiveness by sponging miR-625-5p. Cell Mol Biol Lett (2019) 24:24. doi: 10.1186/s11658-019-0149-x
83. Liu J, Gao L, Zhan N, Xu P, Yang J, Yuan F, et al. Hypoxia induced ferritin light chain (FTL) promoted epithelia mesenchymal transition and chemoresistance of glioma. J Exp Clin Cancer Res (2020) 39(1):137. doi: 10.1186/s13046-020-01641-8
84. Tabatabai G, Frank B, Mohle R, Weller M, Wick W. Irradiation and hypoxia promote homing of haematopoietic progenitor cells towards gliomas by TGF-beta-dependent HIF-1alpha-mediated induction of CXCL12. Brain (2006) 129(Pt 9):2426–35. doi: 10.1093/brain/awl173
85. Vitale C, Griggio V, Riganti C, Todaro M, Kopecka J, Jones R, et al. Targeting HIF-1alpha regulatory pathways as a strategy to hamper tumor-microenvironment interactions in CLL. Cancers (Basel). (2021) 13(12):2883. doi: 10.3390/cancers13122883
86. Wang SC, Hong JH, Hsueh C, Chiang CS. Tumor-secreted SDF-1 promotes glioma invasiveness and TAM tropism toward hypoxia in a murine astrocytoma model. Lab Invest. (2012) 92(1):151–62. doi: 10.1038/labinvest.2011.128
87. Zhuo W, Jia L, Song N, Lu XA, Ding Y, Wang X, et al. The CXCL12-CXCR4 chemokine pathway: A novel axis regulates lymphangiogenesis. Clin Cancer Res (2012) 18(19):5387–98. doi: 10.1158/1078-0432.CCR-12-0708
88. Freitas C, Desnoyer A, Meuris F, Bachelerie F, Balabanian K, Machelon V. The relevance of the chemokine receptor ACKR3/CXCR7 on CXCL12-mediated effects in cancers with a focus on virus-related cancers. Cytokine Growth Factor Rev (2014) 25(3):307–16. doi: 10.1016/j.cytogfr.2014.04.006
89. Hong X, Jiang F, Kalkanis SN, Zhang ZG, Zhang XP, DeCarvalho AC, et al. SDF-1 and CXCR4 are up-regulated by VEGF and contribute to glioma cell invasion. Cancer Lett (2006) 236(1):39–45. doi: 10.1016/j.canlet.2005.05.011
90. Boulakirba S, Pfeifer A, Mhaidly R, Obba S, Goulard M, Schmitt T, et al. IL-34 and CSF-1 display an equivalent macrophage differentiation ability but a different polarization potential. Sci Rep (2018) 8(1):256. doi: 10.1038/s41598-017-18433-4
91. Chitu V, Gokhan S, Nandi S, Mehler MF, Stanley ER. Emerging roles for CSF-1 receptor and its ligands in the nervous system. Trends Neurosci (2016) 39(6):378–93. doi: 10.1016/j.tins.2016.03.005
92. Patel S, Player MR. Colony-stimulating factor-1 receptor inhibitors for the treatment of cancer and inflammatory disease. Curr Top Med Chem (2009) 9(7):599–610. doi: 10.2174/156802609789007327
93. Elmore MR, Najafi AR, Koike MA, Dagher NN, Spangenberg EE, Rice RA, et al. Colony-stimulating factor 1 receptor signaling is necessary for microglia viability, unmasking a microglia progenitor cell in the adult brain. Neuron (2014) 82(2):380–97. doi: 10.1016/j.neuron.2014.02.040
94. Su S, Liu Q, Chen J, Chen J, Chen F, He C, et al. A positive feedback loop between mesenchymal-like cancer cells and macrophages is essential to breast cancer metastasis. Cancer Cell (2014) 25(5):605–20. doi: 10.1016/j.ccr.2014.03.021
95. Giricz O, Mo Y, Dahlman KB, Cotto-Rios XM, Vardabasso C, Nguyen H, et al. The RUNX1/IL-34/CSF-1R axis is an autocrinally regulated modulator of resistance to BRAF-V600E inhibition in melanoma. JCI Insight (2018) 3(14):e120422. doi: 10.1172/jci.insight.120422
96. Stanley ER, Chitu V. CSF-1 receptor signaling in myeloid cells. Cold Spring Harb Perspect Biol (2014) 6(6):a021857. doi: 10.1101/cshperspect.a021857
97. Dai XM, Ryan GR, Hapel AJ, Dominguez MG, Russell RG, Kapp S, et al. Targeted disruption of the mouse colony-stimulating factor 1 receptor gene results in osteopetrosis, mononuclear phagocyte deficiency, increased primitive progenitor cell frequencies, and reproductive defects. Blood (2002) 99(1):111–20. doi: 10.1182/blood.V99.1.111
98. De I, Steffen MD, Clark PA, Patros CJ, Sokn E, Bishop SM, et al. CSF1 overexpression promotes high-grade glioma formation without impacting the polarization status of glioma-associated microglia and macrophages. Cancer Res (2016) 76(9):2552–60. doi: 10.1158/0008-5472.CAN-15-2386
99. Zhu W, Zhao Z, Chou F, Zuo L, Liu T, Yeh S, et al. Loss of the androgen receptor suppresses intrarenal calcium oxalate crystals deposition via altering macrophage recruitment/M2 polarization with change of the miR-185-5p/CSF-1 signals. Cell Death Dis (2019) 10(4):275. doi: 10.1038/s41419-019-1358-y
100. Coniglio SJ, Eugenin E, Dobrenis K, Stanley ER, West BL, Symons MH, et al. Microglial stimulation of glioblastoma invasion involves epidermal growth factor receptor (EGFR) and colony stimulating factor 1 receptor (CSF-1R) signaling. Mol Med (2012) 18:519–27. doi: 10.2119/molmed.2011.00217
101. Quail DF, Bowman RL, Akkari L, Quick ML, Schuhmacher AJ, Huse JT, et al. The tumor microenvironment underlies acquired resistance to CSF-1R inhibition in gliomas. Science (2016) 352(6288):aad3018. doi: 10.1126/science.aad3018
102. Yim A, Smith C, Brown AM. Osteopontin/secreted phosphoprotein-1 harnesses glial-, immune-, and neuronal cell ligand-receptor interactions to sense and regulate acute and chronic neuroinflammation. Immunol Rev (2022) 311(1):224–33. doi: 10.1111/imr.13081
103. Tardelli M, Zeyda K, Moreno-Viedma V, Wanko B, Grun NG, Staffler G, et al. Osteopontin is a key player for local adipose tissue macrophage proliferation in obesity. Mol Metab (2016) 5(11):1131–7. doi: 10.1016/j.molmet.2016.09.003
104. Rentsendorj A, Sheyn J, Fuchs DT, Daley D, Salumbides BC, Schubloom HE, et al. A novel role for osteopontin in macrophage-mediated amyloid-beta clearance in alzheimer’s models. Brain Behav Immun (2018) 67:163–80. doi: 10.1016/j.bbi.2017.08.019
105. Li L, Lv G, Wang B, Kuang L. XIST/miR-376c-5p/OPN axis modulates the influence of proinflammatory M1 macrophages on osteoarthritis chondrocyte apoptosis. J Cell Physiol (2020) 235(1):281–93. doi: 10.1002/jcp.28968
106. Jan HJ, Lee CC, Shih YL, Hueng DY, Ma HI, Lai JH, et al. Osteopontin regulates human glioma cell invasiveness and tumor growth in mice. Neuro Oncol (2010) 12(1):58–70. doi: 10.1093/neuonc/nop013
107. Rittling SR. Osteopontin in macrophage function. Expert Rev Mol Med (2011) 13:e15. doi: 10.1017/S1462399411001839
108. Lu C, Liu Z, Klement JD, Yang D, Merting AD, Poschel D, et al. WDR5-H3K4me3 epigenetic axis regulates OPN expression to compensate PD-L1 function to promote pancreatic cancer immune escape. J Immunother Cancer. (2021) 9(7):e002624. doi: 10.1136/jitc-2021-002624
109. Moorman HR, Poschel D, Klement JD, Lu C, Redd PS, Liu K. Osteopontin: A key regulator of tumor progression and immunomodulation. Cancers (Basel). (2020) 12(11):3379. doi: 10.3390/cancers12113379
110. Lu DY, Yeh WL, Huang SM, Tang CH, Lin HY, Chou SJ. Osteopontin increases heme oxygenase-1 expression and subsequently induces cell migration and invasion in glioma cells. Neuro Oncol (2012) 14(11):1367–78. doi: 10.1093/neuonc/nos262
111. Wei J, Marisetty A, Schrand B, Gabrusiewicz K, Hashimoto Y, Ott M, et al. Osteopontin mediates glioblastoma-associated macrophage infiltration and is a potential therapeutic target. J Clin Invest. (2019) 129(1):137–49. doi: 10.1172/JCI121266
112. Lamour V, Le Mercier M, Lefranc F, Hagedorn M, Javerzat S, Bikfalvi A, et al. Selective osteopontin knockdown exerts anti-tumoral activity in a human glioblastoma model. Int J Cancer. (2010) 126(8):1797–805. doi: 10.1002/ijc.24751
113. Zhu Y, Yang J, Xu D, Gao XM, Zhang Z, Hsu JL, et al. Disruption of tumour-associated macrophage trafficking by the osteopontin-induced colony-stimulating factor-1 signalling sensitises hepatocellular carcinoma to anti-PD-L1 blockade. Gut (2019) 68(9):1653–66. doi: 10.1136/gutjnl-2019-318419
114. Yuan Q, Gu J, Zhang J, Liu S, Wang Q, Tian T, et al. MyD88 in myofibroblasts enhances colitis-associated tumorigenesis via promoting macrophage M2 polarization. Cell Rep (2021) 34(5):108724. doi: 10.1016/j.celrep.2021.108724
115. Foray C, Valtorta S, Barca C, Winkeler A, Roll W, Muther M, et al. Imaging temozolomide-induced changes in the myeloid glioma microenvironment. Theranostics (2021) 11(5):2020–33. doi: 10.7150/thno.47269
116. Zhu X, Fujita M, Snyder LA, Okada H. Systemic delivery of neutralizing antibody targeting CCL2 for glioma therapy. J Neurooncol. (2011) 104(1):83–92. doi: 10.1007/s11060-010-0473-5
117. Cho HR, Kumari N, Thi Vu H, Kim H, Park CK, Choi SH. Increased antiangiogenic effect by blocking CCL2-dependent macrophages in a rodent glioblastoma model: correlation study with dynamic susceptibility contrast perfusion MRI. Sci Rep (2019) 9(1):11085. doi: 10.1038/s41598-019-47438-4
118. Flores-Toro JA, Luo D, Gopinath A, Sarkisian MR, Campbell JJ, Charo IF, et al. CCR2 inhibition reduces tumor myeloid cells and unmasks a checkpoint inhibitor effect to slow progression of resistant murine gliomas. Proc Natl Acad Sci U S A. (2020) 117(2):1129–38. doi: 10.1073/pnas.1910856117
119. Zhang L, Wang H. FTY720 inhibits the Nrf2/ARE pathway in human glioblastoma cell lines and sensitizes glioblastoma cells to temozolomide. Pharmacol Rep (2017) 69(6):1186–93. doi: 10.1016/j.pharep.2017.07.003
120. Pyonteck SM, Akkari L, Schuhmacher AJ, Bowman RL, Sevenich L, Quail DF, et al. CSF-1R inhibition alters macrophage polarization and blocks glioma progression. Nat Med (2013) 19(10):1264–72. doi: 10.1038/nm.3337
121. Hume DA, MacDonald KP. Therapeutic applications of macrophage colony-stimulating factor-1 (CSF-1) and antagonists of CSF-1 receptor (CSF-1R) signaling. Blood (2012) 119(8):1810–20. doi: 10.1182/blood-2011-09-379214
122. Sun L, Liang H, Yu W, Jin X. Increased invasive phenotype of CSF-1R expression in glioma cells via the ERK1/2 signaling pathway. Cancer Gene Ther (2019) 26(5-6):136–44. doi: 10.1038/s41417-018-0053-y
123. Nishikawa M, Inoue A, Ohnishi T, Yano H, Ozaki S, Kanemura Y, et al. Hypoxia-induced phenotypic transition from highly invasive to less invasive tumors in glioma stem-like cells: significance of CD44 and osteopontin as therapeutic targets in glioblastoma. Transl Oncol (2021) 14(8):101137. doi: 10.1016/j.tranon.2021.101137
Keywords: glioma, glioma-associated microglia/macrophages, recruitment of macrophages, cytokine, tumor microenvironment
Citation: Zhou X, Jin G, Zhang J and Liu F (2023) Recruitment mechanisms and therapeutic implications of tumor-associated macrophages in the glioma microenvironment. Front. Immunol. 14:1067641. doi: 10.3389/fimmu.2023.1067641
Received: 12 October 2022; Accepted: 03 April 2023;
Published: 20 April 2023.
Edited by:
Liangxue Zhou, Sichuan University, ChinaReviewed by:
Sheila Mansouri, University Health Network (UHN), CanadaWenbin Li, Beijing Tiantan Hospital, Capital Medical University, China
Bharati Mehani, National Cancer Institute (NIH), United States
Pierluigi Navarra, Catholic University of the Sacred Heart, Italy
Copyright © 2023 Zhou, Jin, Zhang and Liu. This is an open-access article distributed under the terms of the Creative Commons Attribution License (CC BY). The use, distribution or reproduction in other forums is permitted, provided the original author(s) and the copyright owner(s) are credited and that the original publication in this journal is cited, in accordance with accepted academic practice. No use, distribution or reproduction is permitted which does not comply with these terms.
*Correspondence: Junwen Zhang, amV3emhhbmdAaG90bWFpbC5jb20=; Fusheng Liu, bGl1ZnVzaGVuZ0BjY211LmVkdS5jbg==