- 1Department of Medical Biochemistry, College of Health Sciences, Debre Tabor University, Debre Tabor, Ethiopia
- 2Department of Medical Biochemistry, College of Medicine and Health Sciences, University of Gondar, Gondar, Ethiopia
Neutralizing antibodies (NAbs) are central players in the humoral immunity that defends the body from SARS-CoV-2 infection by blocking viral entry into host cells and neutralizing their biological effects. Even though NAbs primarily work by neutralizing viral antigens, on some occasions, they may also combat the SARS-CoV-2 virus escaping neutralization by employing several effector mechanisms in collaboration with immune cells like natural killer (NK) cells and phagocytes. Besides their prophylactic and therapeutic roles, antibodies can be used for COVID-19 diagnosis, severity evaluation, and prognosis assessment in clinical practice. Furthermore, the measurement of NAbs could have key implications in determining individual or herd immunity against SARS-CoV-2, vaccine effectiveness, and duration of the humoral protective response, as well as aiding in the selection of suitable individuals who can donate convalescent plasma to treat infected people. Despite all these clinical applications of NAbs, using them in clinical settings can present some challenges. This review discusses the protective functions, possible protective mechanisms against SARS-CoV-2, and potential clinical applications of NAbs in COVID-19. This article also highlights the possible challenges and solutions associated with COVID-19 antibody-based prophylaxis, therapy, and vaccination.
1 Introduction
The coronavirus disease of 2019 (COVID-19) is a highly contagious and disastrous disease that has been declared a global pandemic since early 2020 (1). COVID-19, which is caused by severe acute respiratory syndrome coronavirus 2 (SARS-CoV-2), has significantly hit the world with enormous morbidity and mortality while inflicting economic, health, and social impacts since its inception (2, 3). Following SARS-CoV-2 infections, several immune cascades can be triggered that, when effective and balanced, are important to control the pathogenesis of COVID-19 (4, 5). It has been demonstrated that humoral immunity is an essential component of the adaptive immunity that fights against SARS-CoV-2 (6). Antibodies are central players in humoral immune responses produced by the B lymphocytes in response to SARS-CoV-2 infection or vaccinations (7, 8). These antibodies, which recognize and confer protection against foreign objects (antigens), can be non-neutralizing or neutralizing antibodies. Non-neutralizing antibodies (nNAbs), also known as binding or sub-neutralizing antibodies, identify and bind to foreign invaders without interfering with microbial infectivity (9, 10). They do not directly combat pathogens; instead, they alert the body to the presence of viruses so that immune cells can find and kill them. nNAbs exert their effects via their conserved crystallizable region (Fc), which interacts with various components of the immune system (11, 12).
Neutralizing antibodies (NAbs) are integral parts of the humoral immune response that defends the body cells from microbial infections, such as SARS-CoV-2 infection, by blocking their entry into a cell and neutralizing their biological action (13). NAbs mediate their function through a variable region of the antibody that binds to the antigen, known as the fragment antigen-binding (Fab) area (11). NAbs, which can be induced by vaccines or prior infection, play a critical role in conferring defense against SARS-CoV-2 infections (14). Clinically, NAbs are currently employed as a key therapeutic component in humans for the treatment of a number of serious pathogenic infections, including SARS-CoV-2 infections (15). The current vaccines, biologic therapy with monoclonal antibodies (mAbs), and convalescent plasma to control SARS-CoV-2 infection are all based on the use of NAbs capable of preventing infection by interfering with the steps of the viral replicative cycle (14, 15). Nonetheless, humoral immunity mediated by NAbs is being challenged by the adverse effects associated with mAbs or COVID-19 vaccines and the advent of new SARS-CoV-2 variants associated with immune evasion. Therefore, a comprehensive understanding of NAb-mediated immune responses to SARS-CoV-2 infection is of paramount importance to guide the development of more potent COVID-19 vaccines and antibody-mediated treatments. This review points out the current knowledge on the role of NAbs in the protection of SARS-CoV-2 infection and their potential clinical implications in COVID-19, as well as summarizes the possible challenges associated with COVID-19 antibody-based prophylaxis, therapy, and vaccinations.
2 Protective roles of NAbs in SARS-CoV-2 infection
2.1 Protection against primary SARS-CoV-2 infection
Humoral immune responses, most notably those mediated by NAbs, have been amply demonstrated to have a role in host protection against SARS-CoV-2 infections. Several studies confirmed that NAbs are highly predictive of immune protection from SARS-CoV-2 infection (16, 17). Congruently, another report showed that protection against SARS-CoV-2 exposure is positively associated with the development of high neutralizing titers of antibodies. It has been shown that NAbs are correlated with defense against viral infection and provide the best proof that protective immunity is established (18). A plethora of studies has also highlighted that NAbs induced by natural infection or vaccination play a pivotal role in viral clearance during acute SARS-CoV-2 infection (16, 19–23). This was further confirmed by other studies reporting that passive transfer of convalescent animals sera or neutralizing mAbs to naïve animals can have a key defensive role against viral infection (19, 24). Besides their neutralizing role during acute infection, high-affinity SARS-CoV-2 NAbs control disease progression to a more severe type during the chronic phase by facilitating the polarization of the T-cell response towards protective type 1 immunity and preventing the virus-induced pathological type 2 eosinophilic inflammation (25–27).
A growing body of evidence reveals that the protective antibodies produced in response to SARS-CoV-2 infection or vaccination can be immunoglobulin (Ig) G, IgM, and IgA, although IgM and IgA appear in the circulation of many cases of SARS-CoV-2 infection (28, 29). IgG antibodies are considered the main antibodies providing defensive immune responses to fight off the SARS-CoV-2 infection (30). The antibody levels of IgG have been shown to correlate with neutralization and act as excellent predictors of neutralization, though the former has a stronger correlation and is more specific at predicting neutralization (100%), and the latter is a more sensitive predictor of neutralization (98%) (9). When compared to other IgG subclasses, IgG1 and IgG3 have been discovered to have a significant protective role, with IgG3 having up to 50-fold greater neutralizing potency (31). A growing body of research suggests that SARS-CoV-2 infection induces neutralizing IgA that could aid in sero-neutralization as strongly as IgG (32–34). Besides, secretory IgA(sIgA) antibodies in response to SARS-CoV-2 infection are produced and enhance mucosal immunity beneficial against the virus targeting mucosal surfaces (5, 35, 36).
Although it is still a matter of debate on how long the NAbs will last, many different types of literature have reported that anti-SARS-CoV-2 antibodies, which may appear as early as one week after the viral invasion, may persist for several months (37, 38). Consistent findings have also been reported by a recent meta-analysis demonstrating that NAbs can be detected 7-15 days after symptom onset in individuals with naturally acquired SARS-CoV-2 infection, while their neutralizing activity gradually declines between 1.3 month to 6.2 months (39, 40). Particularly, anti-SARS-CoV-2 S-specific IgM antibodies become detectable after the fourth day of the infection, peak during the first week of disease onset, and persist for 20 days to a month before gradually declining (41, 42). Plasma IgGs, on the other hand, are detected within the first two weeks of symptom onset, but reach their maximum quantities between the 16th and 50th day, and then decline thereafter (42–44). But it has been demonstrated that IgG can persist in the blood for several months and can still be seen at least a year after infection (45–47). Neutralizing IgA antibodies against SARS-CoV-2 can be detected as early as a week after symptoms appear, they peak between the 15th and 30th day, and disappear at 45–50 days later (32, 44, 48).
2.2 Protection against SARS-CoV-2 reinfection
In addition to their defensive function against primary infection, antibody-mediated immunity acquired through natural infection or vaccination can offer some protection against reinfection and/or lower the risk of clinically significant outcomes (23, 49–51). Nonetheless, whether an individual is protected from SARS-CoV-2 infection after recovery depends on the quantity and quality of the antibodies produced. Antibodies of adequate quality (neutralizing) and sufficient quantity in a person recovering from SARS-CoV-2 infection are protective against re-infection (49). A strong correlation exists between the level of neutralization and immunological defense against SARS-CoV-2, with higher levels indicating higher-quality antibodies and protection (17). The presence of adequate antibodies in response to prior infection is also strongly associated with resistance to reinfection, whereas insufficient humoral defenses cause reinfection (23).
Several investigations involving non-human primate models challenged with SARS-CoV-2 developed potent antibody responses and are protected from reinfection by the same strains for at least some time (50, 51). Notably, an observational study showed that the majority of patients with COVID-19 seroconversion potentially provide immunity to reinfection (38). Consistently, individuals who have recovered from mild COVID-19 develop reasonably robust antibody responses to S protein, which correlate significantly with neutralization of re-infection with SARS-CoV-2 (38). It has also been demonstrated that seropositive recovered subjects have 89% protection from reinfection. Furthermore, recent publications reported that people infected with wild-type SARS-CoV-2 may produce potent human broadly SARS-CoV-2–neutralizing IgA and IgG antibodies effective against Omicron BA.1 and BA.2 (5, 17).
Nevertheless, it is not clearly known how long protective immunity against prior infection will last and confer protection combating re-infection. Studies have reported that SARS-CoV-2 antibody responses may persist for several months after infection and provide protection against the second bout of infection (52). But the primary immune responses show an ineluctably gradual waning in convalescent patients, with a possible risk of re-infection (17, 53). In agreement, another report showed low antibody titers or quick fading of antibodies against SARS-CoV-2 in recovering patients (48). Zhang et al. also demonstrated that reinfections may occur during the convalescent stage in patients who had SARS-CoV-2 infection-induced humoral immunity (54). Notably, suboptimal antibody-mediated immune responses to prior infection or vaccination that may increase the risk of reinfection have been prominently observed in those older or immunocompromised individuals (55). Collectively, NAb levels have been shown to decline from 2-3 months after initial infection in some reports, while significantly falling after 6-8 months after the onset of symptoms in other studies (56–58). This reveals the possibility of reinfection with a second bout of SARS-CoV-2 infection, even shortly after recovery from the primary infection.
Recent real-world data from vaccine-rollout programs have shown the protective cut-off titer of antibodies induced by COVID-19 vaccines or infection (Table 1). Both nNAbs and NAbs have been demonstrated to be potential correlates of protection (CoP) against SARS-CoV-2 infection, although diverse lines of evidence have established postvaccination NAb titers as a better CoP, which is an immunological metric used to assess vaccine efficacy against SARS-CoV-2 infection (63, 66). Vaccine efficacy is the percentage reduction in the average risk of COVID-19 among vaccine recipients as opposed to the risk among placebo receivers (66).
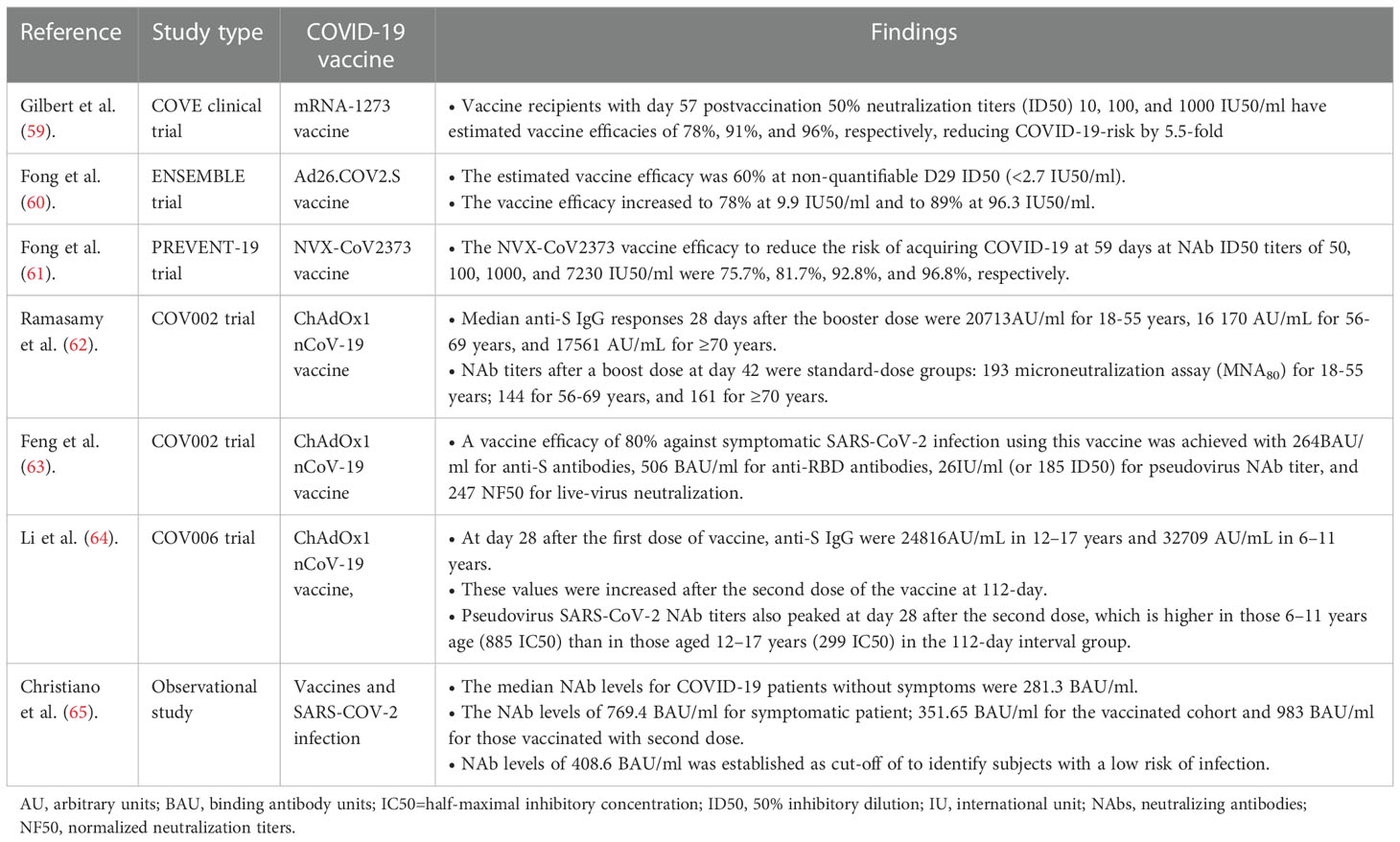
Table 1 A summary table on the protective antibody titer and vaccine efficacy against SARS-CoV-2 infections.
3 Protective mechanisms of NAbs against SARS-CoV-2 infection
NAbs employ a range of proposed defensive mechanisms to eradicate SARS-CoV-2, including direct inhibition of viral entry to host cells (neutralization) and post-binding inhibition (effector functions) (Figure 1) (67). Neutralization, which typically blocks the early steps of the viral replication cycle, is the primary defensive mechanism of NAbs to prevent SARS-CoV-2 (68, 69). It is achieved by the binding of antibodies through their Fab region to viral surface proteins, blocking their interaction with the host cell receptor and disabling the viral infectivity without the involvement of any other antibody or immune cell activity (15, 70). This process of losing the virus’s ability to replicate and cause severe infection and rendering the infectious agent no longer infectious or pathogenic to cause disease is known as neutralization. However, the types of targeted viral proteins have been found to influence the production of potent antibodies that are potentially neutralizing SARS-CoV-2 infection. Although anti-SARS-CoV-2 host antibodies may target both the structural and non-structural proteins, two structural proteins, the nucleoprotein (N) and spike (S) protein, have been discovered to be highly immunogenic viral antigens recognized by antibodies (20, 37, 38).
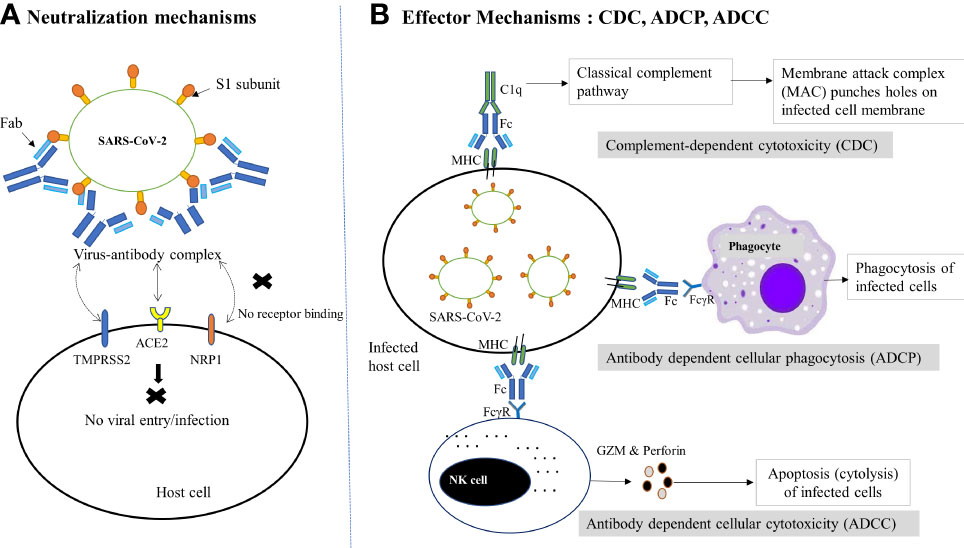
Figure 1 Schematic diagram of NAb-mediated protective mechanisms against SARS-CoV-2 infection. (A) Neutralization mechanisms: Following the binding of NAbs (via Fab) to the RBD of the S1 subunit of SARS-CoV-2, the virus-antibody complex is formed, which prevents the viral binding to host receptor (ACE2) or coreceptors (TMPRSS2, NRP1 etc.) or changes the conformation of the S protein to block viral entry into host cells, thereby preventing subsequent membrane fusion or infection. (B) Effector mechanisms: The binding of antibody-bound infected host cells (via MHC and Fc) to the FcγR of the immune cells and complements (C1q) trigger several effector mechanisms to kill the virus infected cells. In the CDC pathway, complement activates the classical complement pathway and induces cell death by the formation of MAC. On the other hand, phagocytes interact with MHC and result in phagocytosis of infected cells via ADCP. Similarly, the interaction of FcγR-expressing NK cells with the Fc of the antibody complex leads to cytotoxic granule release (GZM and perforin) and hence cytolysis (ADCC). ACE2, Angiotensin-converting enzyme 2; ADCC, Antibody-dependent cellular cytotoxicity; ADCP, Antibody-dependent cellular phagocytosis; CDC, complement-dependent cytotoxicity; Fab, fragment antigen-binding region; Fc, crystallizable fragment; FcγR, Fc gamma receptor; GZM, Granzyme; MAC, Membrane attack complex; MHC, major histocompatibility complex; NAbs, Neutralizing antibodies; NK, Natural killer cell; NRP1, neuropilin 1;TMPRSS2, transmembrane serine protease 2.
S protein is a large trimeric glycoprotein integrated over the viral surface and possesses S1 and S2 subunits. The S subunit contains a 223 amino acid long immunologically important domain found near its C-terminus called the receptor-binding domain (RBD). During host cell infections, the SARS-CoV-2 uses RBD to bind to the host cell surface receptors, the angiotensin-converting enzyme 2 (ACE2) receptor, and mediate subsequent membrane fusion (20, 71–74). The disruption of this RBD–ACE2 interaction prevents SARS-CoV-2 entry into host cells, making RBD a prime target to induce robust antibody-mediated immune responses that are potentially neutralizing and protective of SARS-CoV-2 infection (38, 71, 72). On the other hand, N protein is a structural protein that is found inside the SARS-CoV-2 virus or infected cells and involved in RNA transcription, replication, and packaging of the encapsidated genome into virions. Although N protein is shown to be a highly immunogenic viral protein abundantly expressed during infections, the antibodies produced against this protein are unlikely to directly neutralize SARS-CoV-2. This might be due to the biological function and hidden location of N protein, which induce little or no NAb (38, 75, 76). In comparison, patients with antibodies against the N protein have a weaker neutralizing potential than those with antibodies against the S1-RBD. Besides, the antibodies that bind N proteins do not correlate with those that bind S1-RBD or have neutralizing properties, revealing that, unlike anti-S1-RBD antibodies, anti-N protein antibodies failed to elicit NAb production (77, 78). Moreover, compared to anti-S antibodies, anti-N antibodies showed lower persistence following SARS-CoV-2 infection for up to a year (58).
In addition, NAb binding to the viral protein epitopes that interact with host cell coreceptors is another suggested protective mechanism against viral infection (75). Although many NAbs can block the attachment of the RBD with ACE2, there is the possibility that several NAbs bind to the non-RBD segment of the S protein or the RBD region that do not compete with the ACE2 receptor but recognize epitopes other than the ACE2-binding motif (9, 71, 79). A number of host cell coreceptors or alternative receptors that may be involved in SARS-CoV-2 infectivity and targeted by NAbs include glucose-regulating protein 78 (GRP78), dipeptidyl peptidase 4 (DPP4), AXL, CD147, neuropilin 1(NRP1), TM serine protease 2 (TMPRSS2), lectins, and vimentin (80–86). Therefore, NAb-mediated interruption of the host coreceptor-viral protein interactions could be an alternative mechanism by which antibodies defend the host cells from viral infection. Besides viral entry blocking, NAbs have been found effective as virus-neutralizers by modifying the conformation of the S protein and blocking membrane fusion. NAbs dock to viral epitopes that are not essential for host cell receptor binding but are necessary for conformational changes needed for virus-host cell membrane fusion required for the virus to reach the cytoplasm of host cells (72). Moreover, binding of NAbs to viral proteins necessary for host cell receptor attachment, specifically distal epitopes or internalized portion of fusogenic proteins, to block complete membrane fusion is another way antibodies shield host cells from viral infection. This mechanism of neutralization can occur once the virus is inside endosomes; the antibodies’ interaction with viral surface proteins prevents the modifications required for the viral membrane fusion, which prevents viral infection (11).
In some instances, when viruses escape neutralization and infect host cells, the elimination of infected cells could be accomplished through a variety of antibody-mediated effector mechanisms, involving antibody-dependent cell cytotoxicity(ADCC), antibody-dependent cellular phagocytosis (ADCP), and antibody-mediated complement-dependent cytotoxicity (CDC) (67, 87). NAbs employ these non-neutralizing effector mechanisms by directly docking through their Fc area to the specific Fcγ receptors (FcγR) expressed on the surface of many immune cells like natural killer (NK) cells, macrophages, and neutrophils. Once these human immune cells have bound to the FcγR of antibodies, such as FcγRI, IIA, IIC, IIIA, and IIIB, they get activated in the battle against infectious agents, leading to ADCC, ADCP, and CDC by NK cells, phagocytes, and complement systems, respectively (9, 79). In these effector mechanisms, nNAbs may work alongside NAbs to signal the immune cells to harmful invaders that need to be eliminated. Besides, nNAbs can also exert these Fc-dependent antiviral effector functions, although they bind the viruses differently and hence are unable to neutralize them effectively and necessarily decrease the infection (88–90). Cumulatively, despite the fact that nNAbs are beneficial, NAbs are more effective at battling infections, including SARS-COV-2.
4 Clinical implications of antibodies in COVID-19
In addition to their biological role, antibodies have been observed to play a crucial role in clinical settings, including as markers for COVID-19 diagnosis, determining the immune status and duration of post-COVID-19 infection/vaccination, and serving as disease severity, and prognosis markers. Besides, antibodies have essential implications in designing COVID-19 therapy, prophylaxis, and vaccines, as discussed in this part of the review.
4.1 Diagnostic value of antibodies in COVID-19
In the current COVID-19 pandemic situation, different diagnostic modalities are being used to detect whether an individual is infected with SARS-CoV-2 or not. A number of serological assays have already been approved for clinical practice to detect antibodies against SARS-CoV-2, identify individuals infected with the virus, and thus diagnose COVID-19 infection (9, 38). In serological tests, antibodies against the highly immunogenic N and S viral proteins are frequently used. But due to a conserved N protein across the various coronaviruses (including non-SARS-CoV-2), N protein-based antibody assays have a greater false-positive rate (less specificity) and lesser performance than anti-S antibodies in differentiating COVID-19 patients from controls (6, 20, 38).
Because anti-SARS-CoV-2 antibodies can be found in the blood as early as 10 days after symptom onset, and because COVID-19 vaccination is now widely used, serological tests are nowadays rarely used to diagnose acute infections on their own. Instead, those tests using anti-SARS-CoV-2 antibodies could be potential diagnostic tools to complement a real-time polymerase chain reaction (RT-PCR)-based diagnosis to enhance the detection window of SARS-CoV-2 infection and reduce false-negative RT-PCR testing (20). Moreover, with the emergence of SARS-CoV-2 variants, concerns have also been raised over the ability of serological assays to detect antibodies to the new variants. The Food and Drug Administration (FDA) also cautions against using antibody-based tests to identify an active infection because they only identify the antibodies that the immune system produces in response to a virus, not the virus itself (91).
4.2 Antibody’s role in determining the levels and duration of protective immunity
Antibodies could have an essential utility for the evaluation of protective individual or herd immunity produced against SARS-CoV-2 infection or vaccination (67). In response to SARS-CoV-2 infections or vaccinations, a person may generate antibodies that may offer protective immunity to fend against re/infection. The presence of antibodies does not necessarily indicate immunity against potential re-infection; rather, neutralization titer is highly predictive of immune protection, providing evidence on SARS-CoV-2 immune protection and vaccine efficacy (17). Moreover, the evaluation of NAb levels is useful for the determination of the longevity of the protective humoral response and supporting donor selection criteria for convalescent plasma therapy (67). This reveals that measuring NAb titer is crucial to ascertain whether antibodies are virus-neutralizing and aid in viral clearance and whether they can confer long-lasting protective immunity.
A variety of serology assays involving anti-N or anti-S antibodies can be employed for measuring the humoral response to SARS-CoV-2, including antibody levels, subclasses, avidity, and neutralization activity (92–94). Anti-N serology tests can be used in settings where prior infection with SARS-CoV-2 is suspected. However, serology tests using anti-N protein portray not just the presence of antibodies from prior exposure to the SARS-CoV-2 but also to other related viruses; hence, they may not be a reliable indicator of the presence of NAbs. Thus, using anti-N protein-based serology tests to evaluate long-term COVID-19 immunity may potentially be misleading. Contrarily, anti-S serology assays, especially those involving the anti-S1 subunit and anti-RBD antibodies, detect antibodies produced after prior SARS-CoV-2 infection and vaccination and thus better indicate the neutralizing capacity of antibodies (78, 95).
The antibody-based detection methods can be qualitative, semi-quantitative, or quantitative (96). Qualitative serological tests reveal the existence of antibodies that may help determine whether the patient has developed a humoral immune response against SARS-CoV-2. This type of test measures the host immune response in the form of IgM, IgA, or IgG following infection or vaccination. However, qualitative serologic tests do not provide information on the quantity of antibodies present in a patient. Hence, this test should not be used to assess the immune status of individuals post-COVID-19 infection/vaccination (97). Whereas, semi-quantitative serological assays quantify estimated antibody concentrations rather than their precise values and report the results using arbitrary units. Despite the fact that semi-quantitative assays aid in the diagnosis of COVID-19 and the determination of antibody and neutralization titers, their clinical applicability in the study of the immune status of SARS-CoV-2 infected or vaccinated people remains theoretical due to the test’s semi-quantitative nature (96). Quantitative serologic tests are the type of assays that are lately developed to precisely measure antibody levels in COVID-19 patients. Remarkably, high-throughput quantitative serological tests using the S1 subunit and RBD are becoming increasingly important to determine antibody levels, neutralization titers, and neutralization potency index (97, 98).
Although numerous serological tests are developed to evaluate the binding antibodies, there are only a few neutralizations assays that measure the functional ability of NAbs to block SARS-CoV-2 entry into host cells. The reference test for assessing the ability of antibodies to prevent the virus from entering host cells is the virus neutralization test (VNT) (99). In particular, plaque reduction neutralization tests (PRNT) or conventional neutralization tests (cVNT) are considered the gold standard test for evaluating antibody neutralization capacity against SARS-CoV-2 (100, 101). But this test is a labor-intensive assay that demands a high degree of expertise and exposes laboratory professionals to infection risks as live virus and cell culture are involved. Besides, PRNT has very a low throughput, a long turnaround time, and is difficult to automate. Due to these limitations, it is unsuitable and less reproducible for use in large-scale screening programs (24, 102). Consequently, VNTs are nowadays being replaced by a number of other cost-effective and feasible serological assays, which are frequently used as part of research and diagnostics to identify NAbs and determine potential protective antibody titers after infection or vaccination.
Currently, several serological assays have been approved for clinical practice by the FDA Emergency Use Authorization (EUA) (Table 2). Collectively, they are instrumental for long-term monitoring of humoral immune response to infection or vaccines, for measuring antibody-longevity and waning, determining threshold values that can correlate to protection, quantifying seroprevalence in the community to estimate herd immunity, and determining the fatality rate of infection. Besides, serological tests are crucial for recruiting suitable individuals for clinical trials of vaccine or therapy development, and for evaluating the efficacy of different vaccine candidates. and for collecting plasma donations from convalescent COVID-19 patients with the highest levels of antibodies (9, 111, 112).
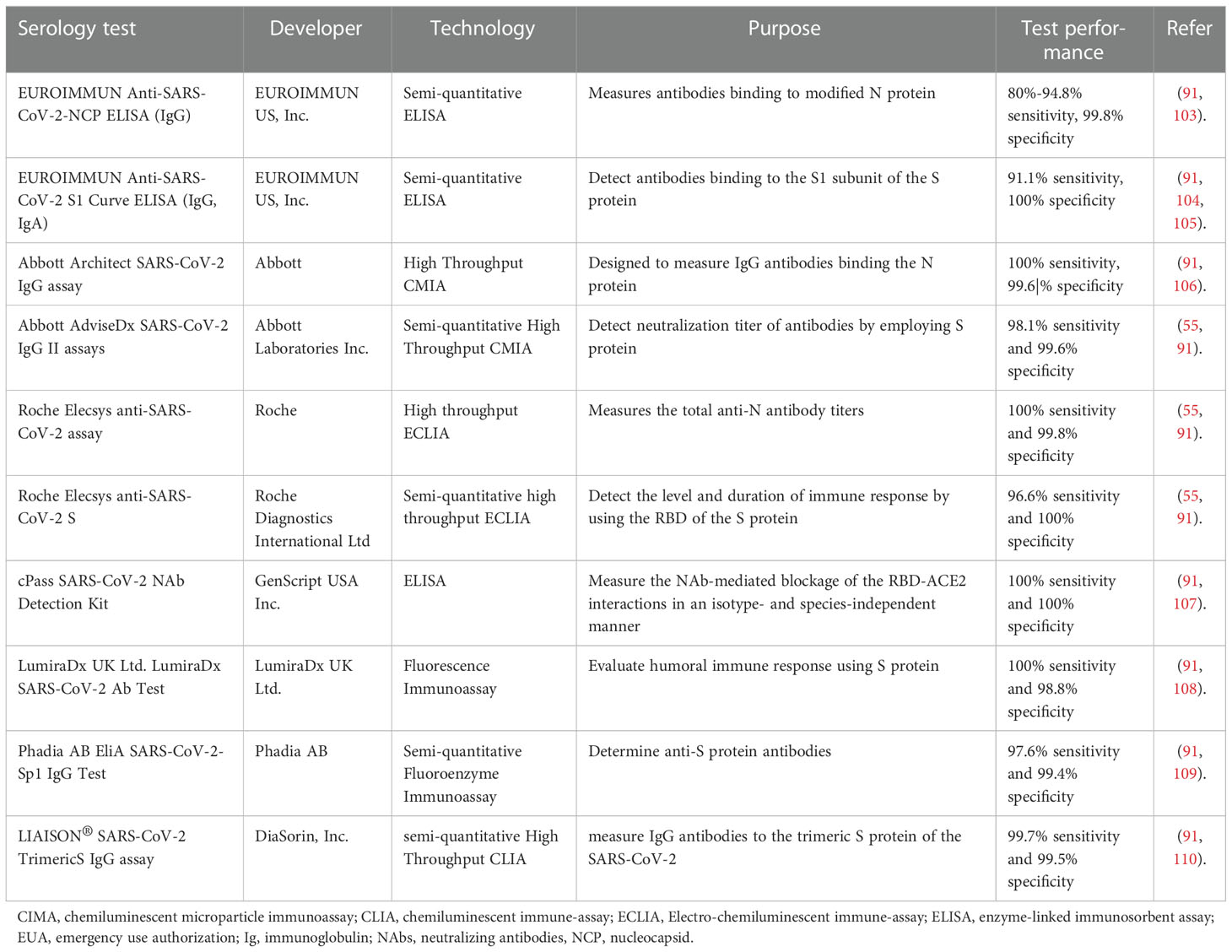
Table 2 Some EUA authorized serological assays measuring humoral immune response or antibody levels.
4.3 Antibodies as severity and prognostic markers of COVID-19
Accumulated evidence revealed that antibodies may be used as severity and prognostic markers in COVID-19. A study by Garcia-Beltran and his coworkers demonstrated that antibody levels, neutralization titer, and neutralization potency are associated with disease severity and may predict patient clinical outcomes (survival) (9). Higher antibody titers have been found to have a significant association with severe disease and worse outcomes (9). Yamayoshi et al. also found higher antibody titers in patients with severe infections than in those with mild or moderate infections (113). Consistently, several other studies have shown that more severe COVID-19 cases are associated with higher anti-RBD and anti-S antibody levels (114, 115). In line with this, another study found that immune activation and high antibody production from extrafollicular B cells play a pathogenic role in severely ill patients (116). This could be due to antibody overproduction that results in hyperactive inflammatory responses and uncontrolled viral replication via FcR-or complement-dependent effector mechanisms known as antibody-dependent enhancement (ADE). Moreover, many publications have reported that neutralization titers, in addition to antibody levels, can dictate the clinical outcomes of COVID-19 patients, with higher neutralizing titers being linked with worse patient outcomes (114, 115, 117–119). The correlation of higher antibody and neutralization titers with more intense COVID-19 symptoms suggests that robust antibody responses may not be sufficient enough to fend off serious illnesses. Antibody and neutralization titers, on the other hand, may have clinical relevance as severity and prognostic markers in COVID-infected patients.
Additionally, Garcia-Beltran et al. showed that the neutralization potency index may serve as a better severity and prognostic marker to assess disease severity and patient outcomes in COVID-19 (9). Quantification of neutralization potency is used to assess the quality of anti-RBD IgG antibodies regardless of their amount. Intriguingly, the neutralization potency of anti-RBD antibodies has been found to be a strong indicator of patient outcomes or survival in which patients with more potent virus-neutralizing antibodies appear to have better clinical outcomes or are more likely to survive. Further analysis of this study demonstrated that an index of 100 or above predicts 100% 30-day survival, whereas an index of less than 100 predicts 87% 30-day survival (9). Besides, the neutralization potency index can be used to predict disease severity in that patients with more severe or fatal COVID-19 appear to have substantially less effective NAbs than those with milder or asymptomatic cases (9). The worsening of disease severity is postulated to be caused by high levels of antibodies and sub-optimal neutralization potency that leads to excessive inflammatory responses and viral over-replication via ADE (20). Altogether, measuring a patient’s anti-RBD antibody neutralization potency index is a promising biomarker of severity that could be employed as a valuable tool for physicians seeking COVID-19 patient risk assessment to identify patients at risk of severe disease or death and guide treatment options.
4.4 Potential therapeutic and prophylactic roles of antibodies in COVID-19
Multiple studies have shown that passive antibody transfer has important implications for conferring a state of protective immunity in prophylactic or therapeutic settings (120–123). This involves the passive transfusion of recombinantly produced antibodies or antibodies from the plasma of convalescent patients to a non-immune person to provide immediate protection against severe SARS-CoV-2 infection (111, 124). In the absence of effective antiviral therapeutics, convalescent sera therapy has been used to treat patients with the severe disease during many viral disease outbreaks (125). Prior studies have demonstrated that plasma NAbs from recovered patients were successfully employed in the passive antibody therapy for patients infected with SARS-CoV, influenza virus A, and Ebola virus (76, 126–130). Similarly, because severe COVID-19 urges rapid intervention during a pandemic, sera from recovered patients have been studied for the discovery of possibly effective therapy against SARS-CoV-2 for severe patients (20).
Accumulating data shows that the use of recovered sera from COVID-19 patients to treat critically ill patients has been observed to be successful with minimal or no adverse effects (131–134). This agrees with another study indicating a higher discharge rate and no adverse effects among patients treated with convalescent sera earlier in infection (131). Furthermore, several prior small and large-scale studies on severe COVID-19 patients have shown that convalescent sera, which are safe and potently neutralizing, may improve patient outcomes in the absence of effective antiviral therapeutics (9, 16, 131–138). According to a Chinese pilot investigation by Chen et al, transfusion of convalescent sera with NAb titers above 1:640 (in 200ml of plasma) is well tolerated and may improve patient outcomes through neutralizing viremia in severe COVID-19 cases (123). Whereas a case report from Indonesia recommends that the titer of SARS-CoV-2 NAb should be greater than 1:320 to effectively treat COVID-19 patients (120). Another recent randomized clinical trial also revealed that the use of high-titer convalescent plasma is beneficial in immunocompromised patients who have not yet had an antibody response (122). Convalescent plasma immunotherapy has been demonstrated to result in a longer survival time and a shorter hospital stay, especially when it is given early to COVID-19 patients (120). FDA has authorized convalescent plasma for emergency use in the treatment of hospitalized COVID-19 patients (139).
On the contrary, a large clinical study conducted by Sullivan et al. reported that patients who received convalescent plasma transfusions and control groups had an insignificant difference in the rate of COVID-19-related hospitalization within 28 days, revealing the minimal benefit of convalescent plasma (140). Similarly, high-titer convalescent plasma that is collected from donors after natural infection does not have a benefit in unselected patients who visit the emergency room or are admitted to the hospital (141). Another recent randomized clinical trial also reports that convalescent plasma does not improve survival or decrease the requirement for mechanical ventilation while having large expenses (142). Taken together, the plasma of recovered patients has less benefit in critically ill patients or non-severe COVID-19 patients.
Passive immunotherapy using engineered anti-SARS-CoV-2 mAbs that target the S protein has been shown to have clinical benefits in treating patients with COVID-19 (143, 144). SARS-CoV-2-infected mice receiving mAbs against the S protein were observed to reduce viral titers and lung pathology, suggesting the protective effect of antibodies against SARS-CoV-2 (19). Recently, the mAbs engineered from NAbs isolated initially from convalescent COVID-19 patients are advancing into antiviral treatment. It has been shown that mAb therapy more effectively improves patient outcomes when given to outpatients early in the course of COVID-19 or to those without an antibody response (145, 146). To date, although there is no currently available mAb product for post-exposure prophylaxis of SARS-CoV-2 infection, a few mAbs have received EUAs from the FDA for the treatment of selected hospitalized COVID-19 patients. Bebtelovimab, tixagevimab plus cilgavimab, bamlanivimab plus etesevimab, casirivimab plus imdevimab, and sotrovimab are the current approved mAbs for COVID-19 therapy (147).
However, the anticipated activity of different anti-SARS-CoV-2 mAb therapies varies significantly depending on the circulating variant. Presently, because of the high global prevalence of the omicron variant, bamlanivimab plus etesevimab, casirivimab plus imdevimab, and sotrovimab have been reported to provide little clinical benefit for patients with COVID-19 caused by the omicron variant (147, 148). This suggests the need for more effective mAb therapies against SARS-CoV-2, including emerging variants. A recent report indicates that IgM or IgA mAbs, which possess different Fab domains to interact with multiple antigen-binding sites, have been shown to have a dramatically higher efficiency of neutralization against SARS-CoV-2 than the currently approved IgG mAbs with the same Fab domain (149). This exciting finding highlights that IgM and IgA mAbs could be used as effective and affordable therapeutic options for COVID-19, including those caused by new variants, although further supporting evidence is required.
4.5 Roles of antibodies in vaccine development
Since the outbreak of COVID-19, scientists have been working tirelessly to develop an effective vaccine against SARS-CoV-2. COVID-19 vaccine development is currently advancing at an unheard-of rate, with numerous vaccines in the early phases of preclinical and clinical studies, some of which have already received approval for use. These COVID-19 vaccines can be mRNA vaccines, viral vector vaccines, protein-based vaccines, or attenuated coronavirus vaccines that induce protective NAbs (150–155). The mRNA vaccines are designed from mRNA that forms genetic instructions for producing the S protein of SARS-CoV-2. The S protein then gets released into the body and elicits humoral immune responses (or NAbs). The mRNA-1273 (from Moderna) and BNT162b2 (from Pfizer-BioNTech) vaccines are lipid nanoparticle encapsulated mRNA vaccines that encode the full-length S protein of SARS-CoV-2 (156, 157). The mRNA-1273 vaccine is recommended for those aged 6 months or older, with two intramuscular doses given at 4-8 weeks’ interval, depending on their age. Similarly, the BNT162b2 vaccine is authorized for those aged 6 months and older using two or three doses, with an adjustment in the recommended dosage for those between 6 months to 11 years. Full vaccination with these two vaccines is shown to have an efficacy above 95% in preventing COVID-19. But the booster doses are required after 4-6 months of completion of the primary series of these vaccines using the same or another COVID-19 vaccine (150, 152).
In addition to mRNA vaccines, COVID-19 vaccines can be viral vector vaccines, including the AZD1222 from Oxford-AstraZeneca, the JNJ-78436735 from Johnson and Johnson, and the Sputnik V from the Gamaleya Research Institute.COVID-19 viral vector vaccines are made from modified versions of various harmless viral vectors, such as adenoviruses, to deliver important instructions to host cells. After getting into a human cell, these vaccines will use the cell’s machinery to express a harmless piece of the virus or the S protein and trigger the immune system to produce NAbs and stimulate other immune cells to fight off what it thinks is an infection (151, 153, 158–161). Specifically, the AstraZeneca (AZD-1222) vaccine is a non-replicative chimpanzee adenoviral vector ChAdOx1 containing S protein (159). As per recent recommendations, it is administered as a 2-dose intramuscular injection spaced 8 to 12 weeks apart and has achieved nearly 79% efficacy against COVID-19 (151, 158, 159). On the other hand, the JNJ-78436735 (Ad26.COV2.S) vaccine is designed using adenovirus 26 (Ad26) and administered as a single intramuscular shot to provide 66-9%-76.7% of protective efficacy after two weeks of vaccination (162). The Sputnik V vaccine is also a vector vaccine using Ad26 and Ad5 as vectors for the expression of the S protein of SARS-CoV-2. This vaccine is given at two different times to achieve 91.6% efficacy and is now authorized for emergency use in many other countries (160, 161).
Furthermore, several COVID-19 vaccines contain coronavirus whole proteins or fragments of them with no genetic material, which can be with or without nanoparticle packaging, known as protein-based COVID-19 vaccines. The adjuvanted NVX-CoV2373 vaccine from Novavax is a protein-based vaccine that incorporates the S protein of SARS-CoV-2 as well as matrix (M) adjuvant that enhances the immune response of the vaccinated people (154). Currently, the FDA has granted an expanded EUA for the adjuvanted Novavax (NVX-CoV2373) vaccine to be administered as a two-dose primary series (with an 8-week interval) for active immunization against COVID-19 in people aged 12 and older (163). Evidence from different phases of the clinical trials indicates that the efficacy of the Novavax vaccine against mild, moderate, and severe diseases is about 90% (164). However, the NAbs produced against this vaccine cannot last longer than 6 months, and hence, a booster dose is generally recommended after 4-6 months of the completion of primary vaccination with an authorized COVID-19 vaccine (165). Moreover, COVID-19 vaccines can also be created from weakened, inactivated, or chemically killed coronaviruses to produce NAbs against the viruses in vaccinated people. Sinovac, BBIBP-CorV, sinopharm-Wuhan, and Bharar Biotech are some examples of attenuated COVID-19 vaccines. CoronaVac (Sinovac) is produced by Sinovac Biotech and given in two intramuscular injections with 14 days gap (166). Whereas, BBIBP-CorV is produced by a Chinese company (Sinopharm) and administered intramuscularly in two doses separated by 21 days to bring nearly 79.3% efficacy. This vaccine is currently authorized for emergency use in several countries (166).
Overall, COVID-19 vaccines are thought to produce a neutralizing type of antibodies and promote T-cell response skewing towards Th1 polarization and blocking Th2 response against COVID-19 (111). NAbs have been shown to be the main correlate of protection for COVID-19 vaccines (59, 167, 168). An increasing body of evidence demonstrates that vaccines against SARS-CoV-2 have been shown to elicit neutralization titer comparable to those observed in naturally infected persons (75, 169). Moreover, a number of successful COVID-19 vaccines with a protection rate greater than 90%, such as BNT162b2, Moderna, and NVX-CoV2373 vaccines, were reported to exhibit Th1-cell-skewed responses of their S protein antigens during preclinical and clinical studies (170). However, while a plethora of studies have demonstrated good humoral responses against SARS-CoV-2 after a few days of vaccination, they do not last long and gradually wane (171). This is evident from a considerable loss of protection or neutralization levels measured from the post-immunization neutralization titer, highlighting the need for booster immunization (167). Currently, WHO recommends at least one booster dose after completion of the primary cycle of many of the COVID-19 vaccines in individuals immunized a few months before.
5 Challenges of antibody-based COVID-19 prophylaxis, therapy, and vaccination
5.1 Challenges associated with convalescent plasma and mAbs and possible solutions
Available evidence indicates that the prophylactic or therapeutic use of convalescent plasma may trigger the production of antibodies that could then facilitate immunopathology such as type 2 inflammatory response, leading to more serious illness or death (111, 172). This adverse condition may happen when a large number of antibodies with less potent neutralizing ability is administered and result in the deterioration of the disease condition or death. Similarly, another report observed ADE with sera from COVID-19 convalescents in an FcR- and ACE2-dependent manner (173). The FDA-approved mAb therapies, such as casirivimab, imdevimab, and sotrovimab, have been reported to cause ADE in FcR- and ACE2-positive cells (174). Several recent studies have also shown that anti-S-protein mAbs can function as ADE-causing antibodies (175, 176). This raises a serious concern over the use of convalescent plasma or mAbs as a treatment or prophylactic strategy. Thus, measuring the neutralization titers and potency of the patient’s antibodies to determine the efficacy and safety of convalescent plasma is required before the transfusion of the convalescent plasma to reduce the risk of potential adverse reactions. Besides, the convalescent plasma of those patients with comorbidities and those who have a high risk of developing immunopathology should be excluded from transfusion (79).
5.2 Challenges associated with COVID-19 vaccines and possible solutions
Despite the prophylactic advantage, COVID-19 vaccines have some downsides, as demonstrated by several publications. A number of reports showed that the emergence of ADE is a potential clinical safety risk associated with COVID-19 vaccines (173, 176, 177). COVID-19 vaccines that contain non-neutralizing antigen targets are proposed to instigate non protective antibodies that derive pathologic ADE. Early research on COVID-19 vaccines revealed that subunit vaccines that can elicit S-specific NAbs, particularly against S stabilized in the prefusion conformation, diminish the presentation of non-neutralizing epitopes and are hence associated with lower ADE risks (73). A recent study by Wang et al., however, has indicated that the full-length S protein of SARS-CoV-2 used for vaccine development induces nNAbs that increase the rate of ADE of infection by facilitating virus entrance into host cells that possess complement or Fc receptors and exacerbating the viral infection (178). This suggests that approaches for developing vaccines should lessen any potential immunopathology linked to nNAbs (125).
On the other hand, COVID-19 immunization strategies that use the RBD of the S protein have been demonstrated to stimulate high titers of NAbs and long-lasting humoral protective immunity, providing a high chance of success with minimal risk of ADE (179, 180). This highlights that vaccines containing RBD directly or vectors encoding the RBD sequence may be considered an ideal, safe vaccine for protection against SARS-CoV-2 infection. This is in agreement with other studies showing that replacing the full-length S protein with RBD as the antigen lowers the risk of ADE while improving the immunogenicity of the candidate vaccine (125, 181). However, opposing results have been reported by Walsh and colleagues, who compared two mRNA-based COVID-19 vaccine and showed that the BNT162b2 vaccine, encoding the entire length-S protein was associated with a lower incidence and severity of systemic reactions than the BNT162b1encoding the RBD of the S protein (182). These counterintuitive results support the necessity for additional research to pinpoint the best potential viral antigen for designing safer COVID-19 vaccines.
Furthermore, a pathological type 2 inflammatory response, which is accompanied by a eosinophilic pulmonary immune pathology, could also be a concern during COVID-19 vaccination, as reported by some studies (25–27). Nonetheless, several other preclinical studies of COVID-19 vaccines found no evidence of increased respiratory disease typical of an increased eosinophilic proinflammatory pulmonary response upon challenge in preclinical studies of COVID-19 vaccines (27, 183). But the risk of Th-2-oriented immune serum following COVID-19 vaccination that contains polyclonal antibodies directing the S protein to boost virus infection needs to be further evaluated by taking into account the stated risk of ADE of infection. To improve the efficacies and safety of COVID-19 vaccines, careful selection of antigens during vaccine development to induce high-affinity NAbs polarizing the T-cell response towards type 1 immunity while suppressing unwanted type 2 immunity may be critical (26). In addition, adopting adjuvants that promote Th1 response polarization, reduce the risk of undesired type 2 immune response, and hence improve the protective effect of COVID-19 vaccines have been shown to have promising outcomes (25).
Even though all currently authorized COVID-19 vaccines are ideally safe, effective, and less risky of developing severe COVID-19 illness, there are some associated mild symptoms such as pain, redness, swelling, tiredness, headache, muscle pain, chills, fever, and nausea after vaccination (184). Rarely, some severe and even fatal symptoms such as blood clotting may happen following the administration of a few vaccines. For instance, AstraZeneca and JNJ-78436735 vaccines are recently reported to be associated with very rare cases of blood clotting with low blood platelets (thrombocytopenia) with or without bleeding, including rare cases of clots in the vessels draining blood from the brain, particularly in women younger than 50 years old. But many countries continue to use the vaccines since this fatal type of side effect has an extremely rare occurrence and the benefits of the vaccines outweigh the risk of side effects (185–188). Moreover, Guillain-Barre Syndrome was reported following JNJ-78436735 and Pfizer COVID-19 vaccination (189, 190).
The other challenge associated with compromised vaccine efficacy is the emergence of new SARS-CoV-2 variants. So far, numerous SARS-CoV-2 variants have been reported to appear and spread globally, including B.1.1.7, B.1.351, P.1, P.2, B.1.427, B.1.429, B.1.525, B.1.526, B.1.617, B.1.1.298, B.1.1.207, and B.1.1.529 (191, 192). Naturally occurring mutations in the new SARS-CoV-2 variants span the whole length of S protein but mainly occur in S1 and RBD which are the main targets of NAbs and may alter their interaction with the ACE2 receptor (156, 193). The main RBD mutations of concern, namely N501Y, E484K, K417N, and K417T, have been identified as antigenically important and reported as escape mutations for several mAbs. The B.1.1.7, P.1, and B.1.351 variants have the N501Y and E484K substitution in S protein when compared to the WIV04/2019 (or the reference or original) sequence. In addition, a K417T substitution is present in both B.1.351 and P1 lineages, and K417N mutation occurs in the B.1.351 variant (193–195). The current suite of antibody vaccines was designed with S protein based on strains circulating during the early phases of the pandemic. This raised a concern as to whether the currently approved vaccines will be effective against the new variants of SARS-CoV-2. Thus, the emerging strains of SARS-CoV-2 could pose another challenge to developing an effective COVID-19 vaccine, as has happened with other viral vaccines (193–195).
According to available data, antibodies developed in response to the original SARS-CoV-2 infection or vaccines found to provide protection (neutralization) against some strains, such as D614G, and antibodies developed in the majority of patients can cross-neutralize D614 or G614 strains, lowering the risk of re-infection (9, 130, 196).In contrast, preliminary studies with pseudo viruses suggest that neutralization by most convalescent sera and vaccine-induced immune sera is largely ineffective and has limited cross-protection against the recently emerged novel SARS-CoV-2 variants (193–195). Some of the novel SARS-CoV-2 variants that have particular importance, known as variants of concern (VOC), generally increase the transmission dynamics, virulence, and disease severity as well as significantly suppress the host immune response, such as neutralization by antibodies produced during prior infection or vaccination, decreasing therapy or vaccine effectiveness against them, or causing diagnostic detection failure (197). Variants with multiple substitutions in S protein, including in the NTD and RBD, enhance transmissibility, which may explain why SARS-CoV-2 transmission is still uncontrollable and the world is in the midst of a COVID-19 pandemic. Besides, the novel SARS-CoV-2 variants containing K417N, K417T, E484K, and N501Y RBD mutations in the S protein gene, namely B.1.1.7, B.1.351, and P.1 have been shown to reduce the overall neutralization efficacy of the current COVID-19 vaccines, such as AZD1222, JNJ-78436735, NVX-CoV2373, and Coronavac (153, 154, 193, 198).
Generally, these variants may significantly reduce neutralization, even in fully vaccinated individuals (199–201). This could be due to the higher immune evasion potential of SARS-CoV-2 amplifying the waning of humoral immunity produced in response to vaccination. Therefore, the reformulation of existing vaccines to incorporate diverse spike sequences should be considered during vaccine development to produce new vaccines capable of eliciting broadly neutralizing antibodies (bNAbs) that may be necessary to resolve the ongoing pandemic as well as prevent future pandemics from novel strains. Other studies have suggested that waning immunity, rather than immunological escape, may be to blame for the gradual decline in vaccine effectiveness (202, 203). This suggests that a booster dose of the COVID-19 vaccine appeared to increase neutralizing activity and confer prolonged protective immunity against SARS-CoV-2 variants. Mounting body of evidence shows that a booster dose of the mRNA vaccine appears to increase neutralizing activity against the omicron variant, reducing symptomatic disease and/or hospitalization; although protection against symptomatic omicron infection is less effective than with Delta, and the immunity may fade over time (204–207). Therefore, additional booster doses (two or above doses) may reestablish antibody levels and increase effectiveness against all COVID-19-related outcomes; however, the protection against infection remains short-lived (208, 209).
6 Conclusion
In summary, NAbs are components of the humoral immune response produced against the S protein of SARS-CoV-2, particularly the RBD of the S1 subunit that has a key role in viral entry and infection of target cells. NAbs bound to RBD block the SARS-CoV-2 from invading the host cells and its replication with or with no other immune cell involvement. This process of viral clearance by disabling its infectivity is called neutralization. Potent NAbs are essential to protect the body from SARS-CoV-2 infection or reinfection. NAbs have also potential application in clinical settings and can be generally helpful in the diagnosis of COVID-19, determination of immune status and duration of protection against SARS-CoV-2, as well as assessment of disease severity, clinical outcome, and vaccine effectiveness of COVID-19. Moreover, NAbs have been reported to play a therapeutic and prophylactic role in COVID-19 if the antibodies have high potency and are safe with a low risk of developing adverse events. They are also crucial in COVID-19 vaccine development, with several of them having received approval currently in many countries. Despite all these implications, COVID-19 antibody-based prophylaxis, therapy, and vaccination are possibly associated with unwanted immune responses such as eosinophilic type 2 immune response and ADE. In addition, the newly emerged variants of SARS-CoV-2 may reduce vaccine efficacy and challenges in developing an effective COVID-19 vaccine. Thus, producing new vaccines by incorporating diverse spike sequences to elicit bNAbs may be needed.
Author contributions
The first draft was designed by EC and both EC and TA made a significant contribution to the work reported, whether that is in the compilation, editing, and finalizing the review. All authors contributed to the article and approved the submitted version.
Conflict of interest
The authors declare that the research was conducted in the absence of any commercial or financial relationships that could be construed as a potential conflict of interest.
Publisher’s note
All claims expressed in this article are solely those of the authors and do not necessarily represent those of their affiliated organizations, or those of the publisher, the editors and the reviewers. Any product that may be evaluated in this article, or claim that may be made by its manufacturer, is not guaranteed or endorsed by the publisher.
Abbreviations
ACE2, Angiotensin-converting enzyme 2; ADCC, Antibody-dependent cellular cytotoxicity; ADCP, Antibody-dependent cellular phagocytosis; bNAbs, broadly neutralizing antibodies; COVID 19, coronavirus disease 2019; ELISA, Enzyme-linked immunosorbent assay; FcγR, Fcγ receptor; FDA, Food and Drug Administration; Ig, Immunoglobulin; mAbs, monoclonal antibodies; MERS, middle east respiratory syndrome; NAbs; Neutralizing antibodies; NK cells, Natural killer cells; N protein, Nucleocapsid protein; RT-PCR, Real time polymerase chain reaction; RBD, Receptor binding domain; SARS, severe acute respiratory syndrome; SARS-CoV, Severe acute respiratory syndrome coronavirus; SARS-CoV-2, Severe acute respiratory syndrome coronavirus 2; S protein, Spike protein; Th1, T-helper cell 1.
References
1. Abebe EC, Dejenie TA, Shiferaw MY, Malik T. The newly emerged COVID-19 disease: a systemic review. Virol J (2020) 17(1):1–8. doi: 10.1186/s12985-020-01363-5
2. Martin A, Markhvida M, Hallegatte S, Walsh B. Socio-economic impacts of COVID-19 on household consumption and poverty. Econ disast Climate change (2020) 4(3):453–79. doi: 10.1007/s41885-020-00070-3
3. Dietz W, Santos-Burgoa C. Obesity and its implications for COVID-19 mortality. Obesity (2020) 28(6):1005. doi: 10.1002/oby.22818
4. Focosi D, Maggi F, Casadevall A. Mucosal vaccines, sterilizing immunity, and the future of sars-cov-2 virulence. Viruses (2022) 14(2):187. doi: 10.3390/v14020187
5. Planchais C, Fernández I, Bruel T, de Melo GD, Prot M, Beretta M, et al. Potent human broadly SARS-CoV-2 neutralizing IgA and IgG antibodies effective against omicron BA. 1 and BA. 2. bioRxiv (2022). doi: 10.1101/2022.04.01.486719
6. Tang MS, Case JB, Franks CE, Chen RE, Anderson NW, Henderson JP, et al. Association between SARS-CoV-2 neutralizing antibodies and commercial serological assays. Clin Chem (2020) 66(12):1538–47. doi: 10.1093/clinchem/hvaa211
7. Reth M. Matching cellular dimensions with molecular sizes. Nat Immunol (2013) 14(8):765–7. doi: 10.1038/ni.2621
8. Murphy K, Weaver C. Janeway's immunobiology: Garland science;. (2016). doi: 10.1201/9781315533247
9. Garcia-Beltran WF, Lam EC, Astudillo MG, Yang D, Miller TE, Feldman J, et al. COVID-19-neutralizing antibodies predict disease severity and survival. Cell (2021) 184(2):476–88:e11. doi: 10.1016/j.cell.2020.12.015
10. Schmaljohn A L. Protective antiviral antibodies that lack neutralizing activity: precedents and evolution of concepts. Curr HIV Res (2013) 11(5):345–53. doi: 10.2174/1570162X113116660057
11. Jiang S, Du L. Effect of low-pathogenic human coronavirus-specific antibodies on SARS-CoV-2. Trends Immunol (2020) 41(10):853–4. doi: 10.1016/j.it.2020.08.003
12. Sedova E, Scherbinin D, Lysenko A, Alekseeva S, Artemova E, Shmarov M. Non-neutralizing antibodies directed at conservative influenza antigens. Acta Naturae (2019) 11(4(4 (43):22–32. doi: 10.32607/20758251-2019-11-4-22-32
13. Dutta A, Huang C-T, Lin C-Y, Chen T-C, Lin Y-C, Chang C-S, et al. Sterilizing immunity to influenza virus infection requires local antigen-specific T cell response in the lungs. Sci Rep (2016) 6(1):1–14. doi: 10.1038/srep32973
14. Katz LM. (A little) clarity on convalescent plasma for covid-19. Mass Med Soc; (2021) p:666–8. doi: 10.1056/NEJMe2035678
15. Zhou G, Zhao Q. Perspectives on therapeutic neutralizing antibodies against the novel coronavirus SARS-CoV-2. Int J Biol Sci (2020) 16(10):1718–23. doi: 10.7150/ijbs.45123
16. Brouwer PJ, Caniels TG, van der Straten K, Snitselaar JL, Aldon Y, Bangaru S, et al. Potent neutralizing antibodies from COVID-19 patients define multiple targets of vulnerability. Science (2020) 369(6504):643–50. doi: 10.1126/science.abc5902
17. McDade TW, Demonbreun AR, Sancilio A, Mustanski B, D’Aquila RT, McNally EM. Durability of antibody response to vaccination and surrogate neutralization of emerging variants based on SARS-CoV-2 exposure history. Sci Rep (2021) 11(1):1–6. doi: 10.1038/s41598-021-96879-3
18. Plotkin SA. Correlates of protection induced by vaccination. Clin Vaccine Immunol (2010) 17(7):1055–65. doi: 10.1128/CVI.00131-10
19. Hassan AO, Case JB, Winkler ES, Thackray LB, Kafai NM, Bailey AL, et al. A SARS-CoV-2 infection model in mice demonstrates protection by neutralizing antibodies. Cell (2020) 182(3):744–53:e4. doi: 10.1016/j.cell.2020.06.011
20. Siracusano G, Pastori C, Lopalco L. Humoral immune responses in COVID-19 patients: a window on the state of the art. Front Immunol (2020) 11. doi: 10.3389/fimmu.2020.01049
21. Mercado NB, Zahn R, Wegmann F, Loos C, Chandrashekar A, Yu J, et al. Single-shot Ad26 vaccine protects against SARS-CoV-2 in rhesus macaques. Nature (2020) 586(7830):583–8. doi: 10.1038/s41586-020-2607-z
22. Yu J, Tostanoski LH, Peter L, Mercado NB, McMahan K, Mahrokhian SH, et al. DNA Vaccine protection against SARS-CoV-2 in rhesus macaques. Science (2020) 369(6505):806–11. doi: 10.1126/science.abc6284
23. Addetia A, Crawford KH, Dingens A, Zhu H, Roychoudhury P, Huang M-L, et al. Neutralizing antibodies correlate with protection from SARS-CoV-2 in humans during a fishery vessel outbreak with a high attack rate. J Clin Microbiol (2020) 58(11):e02107-20. doi: 10.1128/JCM.02107-20
24. Rogers TF, Zhao F, Huang D, Beutler N, Burns A, He W-t, et al. Isolation of potent SARS-CoV-2 neutralizing antibodies and protection from disease in a small animal model. Science (2020) 369(6506):956–63. doi: 10.1126/science.abc7520
25. Al Kaabi N, Zhang Y, Xia S, Yang Y, Al Qahtani MM, Abdulrazzaq N, et al. Effect of 2 inactivated SARS-CoV-2 vaccines on symptomatic COVID-19 infection in adults: a randomized clinical trial. Jama (2021) 326(1):35–45. doi: 10.1001/jama.2021.8565
26. Luan N, Li T, Wang Y, Cao H, Yin X, Lin K, et al. Th2-oriented immune serum after SARS-CoV-2 vaccination does not enhance infection in vitro. Front Immunol (2022) 13. doi: 10.3389/fimmu.2022.882856
27. Bewley KR, Gooch K, Thomas KM, Longet S, Wiblin N, Hunter L, et al. Immunological and pathological outcomes of SARS-CoV-2 challenge following formalin-inactivated vaccine in ferrets and rhesus macaques. Sci Adv (2021) 7(37):eabg7996. doi: 10.1126/sciadv.abg7996
28. Murin CD, Wilson IA, Ward AB. Antibody responses to viral infections: a structural perspective across three different enveloped viruses. Nat Microbiol (2019) 4(5):734–47. doi: 10.1038/s41564-019-0392-y
29. Ruggiero A, Piubelli C, Calciano L, Accordini S, Valenti MT, Dalle Carbonare L, et al. SARS-CoV-2 vaccination elicits unconventional IgM specific responses in naïve and previously COVID-19-infected individuals. EBioMedicine (2022) 77:103888. doi: 10.1016/j.ebiom.2022.103888
30. Piccoli L, Park Y-J, Tortorici MA, Czudnochowski N, Walls AC, Beltramello M, et al. Mapping neutralizing and immunodominant sites on the SARS-CoV-2 spike receptor-binding domain by structure-guided high-resolution serology. Cell (2020) 183(4):1024–42.e21. doi: 10.1016/j.cell.2020.09.037
31. Kallolimath S, Sun L, Palt R, Stiasny K, Mayrhofer P, Gruber C, et al. Highly active engineered IgG3 antibodies against SARS-CoV-2. Proc Natl Acad Sci (2021) 118(42):e2107249118. doi: 10.1073/pnas.2107249118
32. Sterlin D, Mathian A, Miyara M, Mohr A, Anna F, Claër L, et al. IgA dominates the early neutralizing antibody response to SARS-CoV-2. Sci Trans Med (2021) 13(577):eabd2223. doi: 10.1126/scitranslmed.abd2223
33. Wang Z, Lorenzi JC, Muecksch F, Finkin S, Viant C, Gaebler C, et al. Enhanced SARS-CoV-2 neutralization by dimeric IgA. Sci Trans Med (2021) 13(577):eabf1555. doi: 10.1126/scitranslmed.abf1555
34. Isho B, Abe KT, Zuo M, Jamal AJ, Rathod B, Wang JH, et al. Persistence of serum and saliva antibody responses to SARS-CoV-2 spike antigens in COVID-19 patients. Sci Immunol (2020) 5(52):eabe5511. doi: 10.1126/sciimmunol.abe5511
35. Cagigi A, Yu M, Österberg B, Svensson J, Falck-Jones S, Vangeti S, et al. Airway antibodies emerge according to COVID-19 severity and wane rapidly but reappear after SARS-CoV-2 vaccination. JCI Insight (2021) 6(22):e151463. doi: 10.1172/jci.insight.151463
36. Molaei S, Dadkhah M, Asghariazar V, Karami C, Safarzadeh E. The immune response and immune evasion characteristics in SARS-CoV, MERS-CoV, and SARS-CoV-2: Vaccine design strategies. Int Immunopharmacol (2020), 107051. doi: 10.1016/j.intimp.2020.107051
37. Hashem AM, Algaissi A, Almahboub SA, Alfaleh MA, Abujamel TS, Alamri SS, et al. Early humoral response correlates with disease severity and outcomes in COVID-19 patients. Viruses (2020) 12(12):1390. doi: 10.3390/v12121390
38. Wajnberg A, Amanat F, Firpo A, Altman DR, Bailey MJ, Mansour M, et al. Robust neutralizing antibodies to SARS-CoV-2 infection persist for months. Science (2020) 370(6521):1227–30. doi: 10.1126/science.abd7728
39. Post N, Eddy D, Huntley C, Van Schalkwyk MC, Shrotri M, Leeman D, et al. Antibody response to SARS-CoV-2 infection in humans: A systematic review. PloS One (2020) 15(12):e0244126. doi: 10.1371/journal.pone.0244126
40. L'Huillier AG, Meyer B, Andrey DO, Arm-Vernez I, Baggio S, Didierlaurent A, et al. Antibody persistence in the first 6 months following SARS-CoV-2 infection among hospital workers: a prospective longitudinal study. Clin Microbiol Infect (2021) 27(5):784.e1–.e8 doi: 10.1016/j.cmi.2021.01.005
41. Liu X, Wang J, Xu X, Liao G, Chen Y, Hu C-H. Patterns of IgG and IgM antibody response in COVID-19 patients. Emerg Microbes infect (2020) 9(1):1269–74. doi: 10.1080/22221751.2020.1773324
42. Assadiasl S, Fatahi Y, Zavvar M, Nicknam MH. COVID-19: Significance of antibodies. Hum Antib (2020) 28(4):287–97. doi: 10.3233/HAB-200429
43. Ma H, Zeng W, He H, Zhao D, Jiang D, Zhou P, et al. Serum IgA, IgM, and IgG responses in COVID-19. Cell Mol Immunol (2020) 17(7):773–5. doi: 10.1038/s41423-020-0474-z
44. Scourfield DO, Reed SG, Quastel M, Alderson J, Bart VM, Teijeira Crespo A, et al. The role and uses of antibodies in COVID-19 infections: A living review. Oxford Open Immunol (2021) 2(1):iqab003. doi: 10.1093/oxfimm/iqab003
45. Wang Z, Muecksch F, Schaefer-Babajew D, Finkin S, Viant C, Gaebler C, et al. Naturally enhanced neutralizing breadth against SARS-CoV-2 one year after infection. Nature (2021) 595(7867):426–31. doi: 10.1038/s41586-021-03696-9
46. Ortega N, Ribes M, Vidal M, Rubio R, Aguilar R, Williams S, et al. Seven-month kinetics of SARS-CoV-2 antibodies and role of pre-existing antibodies to human coronaviruses. Nat Commun (2021) 12(1):1–10. doi: 10.1038/s41467-021-24979-9
47. Chan ASW, Ho JMC, Li JSF, Tam HL, Tang PMK. Impacts of COVID-19 pandemic on psychological well-being of older chronic kidney disease patients. Front Med (2021) 8:666973. doi: 10.3389/fmed.2021.666973
48. Lou B, Li T-D, Zheng S-F, Su Y-Y, Li Z-Y, Liu W, et al. Serology characteristics of SARS-CoV-2 infection after exposure and post-symptom onset. Eur Respir J (2020) 56(2):2000763. doi: 10.1183/13993003.00763-2020
49. Kadkhoda K. COVID-19: are neutralizing antibodies neutralizing enough? Transfusion (2020) 60 (7):1602–3. doi: 10.1111/trf.15897
50. Deng W, Bao L, Liu J, Xiao C, Liu J, Xue J, et al. Primary exposure to SARS-CoV-2 protects against reinfection in rhesus macaques. Science (2020) 369(6505):818–23. doi: 10.1126/science.abc5343
51. Chandrashekar A, Liu J, Martinot AJ, McMahan K, Mercado NB, Peter L, et al. SARS-CoV-2 infection protects against rechallenge in rhesus macaques. Science (2020) 369(6505):812–7. doi: 10.1126/science.abc4776
52. Altawalah H. Antibody responses to natural SARS-CoV-2 infection or after COVID-19 vaccination. Vaccines (2021) 9(8):910. doi: 10.3390/vaccines9080910
53. Saadat S, Tehrani ZR, Logue J, Newman M, Frieman MB, Harris AD, et al. Binding and neutralization antibody titers after a single vaccine dose in health care workers previously infected with SARS-CoV-2. Jama (2021) 325(14):1467–9. doi: 10.1001/jama.2021.3341
54. Zhang J, Ding N, Ren L, Song R, Chen D, Zhao X, et al. COVID-19 reinfection in the presence of neutralizing antibodies. Natl Sci Rev (2021) 8(4):nwab006. doi: 10.1093/nsr/nwab006
55. Bradley BT, Bryan A, Fink SL, Goecker EA, Roychoudhury P, Huang M-L, et al. Anti-SARS-CoV-2 antibody levels measured by the AdviseDx SARS-CoV-2 assay are concordant with previously available serologic assays but are not fully predictive of sterilizing immunity. J Clin Microbiol (2021) 59(9):e00989-21. doi: 10.1128/JCM.00989-21
56. Dan JM, Mateus J, Kato Y, Hastie KM, Yu ED, Faliti CE, et al. Immunological memory to SARS-CoV-2 assessed for up to 8 months after infection. Science (2021) 371(6529):eabf4063. doi: 10.1126/science.abf4063
57. Marot S, Malet I, Leducq V, Zafilaza K, Sterlin D, Planas D, et al. Rapid decline of neutralizing antibodies against SARS-CoV-2 among infected healthcare workers. Nat Commun (2021) 12(1):1–7. doi: 10.1038/s41467-021-21111-9
58. Van Elslande J, Oyaert M, Lorent N, Weygaerde YV, Van Pottelbergh G, Godderis L, et al. Lower persistence of anti-nucleocapsid compared to anti-spike antibodies up to one year after SARS-CoV-2 infection. Diagn Microbiol Infect dis (2022) 103(1):115659. doi: 10.1016/j.diagmicrobio.2022.115659
59. Gilbert PB, Montefiori DC, McDermott AB, Fong Y, Benkeser D, Deng W, et al. Immune correlates analysis of the mRNA-1273 COVID-19 vaccine efficacy clinical trial. Science (2022) 375(6576):43–50. doi: 10.1126/science.abm3425
60. Fong Y, McDermott AB, Benkeser D, Roels S, Stieh DJ, Vandebosch A, et al. Immune correlates analysis of the ENSEMBLE single Ad26. COV2. s dose vaccine efficacy clinical trial. Nat Microbiol (2022), 7(12):1996–2010. doi: 10.1038/s41564-022-01262-1
61. Fong Y, Huang Y, Benkeser D, Carpp LN, Áñez G, Woo W, et al. Immune correlates analysis of the PREVENT-19 COVID-19 vaccine efficacy clinical trial. medRxiv (2022). doi: 10.1101/2022.06.22.22276362
62. Ramasamy MN, Minassian AM, Ewer KJ, Flaxman AL, Folegatti PM, Owens DR, et al. Safety and immunogenicity of ChAdOx1 nCoV-19 vaccine administered in a prime-boost regimen in young and old adults (COV002): a single-blind, randomised, controlled, phase 2/3 trial. Lancet (2020) 396(10267):1979–93. doi: 10.1016/S0140-6736(20)32466-1
63. Feng S, Phillips DJ, White T, Sayal H, Aley PK, Bibi S, et al. Correlates of protection against symptomatic and asymptomatic SARS-CoV-2 infection. Nat Med (2021) 27(11):2032–40. doi: 10.1038/s41591-021-01540-1
64. Li G, Cappuccini F, Marchevsky NG, Aley PK, Aley R, Anslow R, et al. Safety and immunogenicity of the ChAdOx1 nCoV-19 (AZD1222) vaccine in children aged 6–17 years: a preliminary report of COV006, a phase 2 single-blind, randomised, controlled trial. Lancet (2022) 399(10342):2212–25. doi: 10.1016/S0140-6736(22)00770-X
65. Cristiano A, Nuccetelli M, Pieri M, Sarubbi S, Pelagalli M, Calugi G, et al. Serological anti-SARS-CoV-2 neutralizing antibodies association to live virus neutralizing test titers in COVID-19 paucisymptomatic/symptomatic patients and vaccinated subjects. Int Immunopharmacol (2021) 101:108215. doi: 10.1016/j.intimp.2021.108215
66. Gilbert PB, Donis RO, Koup RA, Fong Y, Plotkin SA, Follmann D. A covid-19 milestone attained–a correlate of protection for vaccines. New Engl J Med (2022) 387(24):2203–6. doi: 10.1056/NEJMp2211314
67. Morales-Núñez JJ, Muñoz-Valle JF, Torres-Hernández PC, Hernández-Bello J. Overview of neutralizing antibodies and their potential in COVID-19. Vaccines (2021) 9(12):1376. doi: 10.3390/vaccines9121376
68. Cheedarla N, Hanna LE. Functional and protective role of neutralizing antibodies (NAbs) against viral infections. Recent Develop Appl Microbiol Biochem: Elsevier (2019) p:83–93. doi: 10.1016/B978-0-12-816328-3.00007-6
69. Klasse P. Neutralization of virus infectivity by antibodies: old problems in new perspectives. Adv Biol (2014) 2014:157895. doi: 10.1155/2014/157895
70. Zheng Q, Jiang J, He M, Zheng Z, Yu H, Li T, et al. Viral neutralization by antibody-imposed physical disruption. Proc Natl Acad Sci (2019) 116(52):26933–40. doi: 10.1073/pnas.1916028116
71. Yan R, Zhang Y, Li Y, Xia L, Guo Y, Zhou Q. Structural basis for the recognition of SARS-CoV-2 by full-length human ACE2. Science (2020) 367(6485):1444–8. doi: 10.1126/science.abb2762
72. Du L, He Y, Zhou Y, Liu S, Zheng B-J, Jiang S. The spike protein of SARS-CoV–a target for vaccine and therapeutic development. Nat Rev Microbiol (2009) 7(3):226–36. doi: 10.1038/nrmicro2090
73. Wrapp D, Wang N, Corbett KS, Goldsmith JA, Hsieh C-L, Abiona O, et al. Cryo-EM structure of the 2019-nCoV spike in the prefusion conformation. Science (2020) 367(6483):1260–3. doi: 10.1126/science.abb2507
74. Letko M, Marzi A, Munster V. Functional assessment of cell entry and receptor usage for SARS-CoV-2 and other lineage b betacoronaviruses. Nat Microbiol (2020) 5(4):562–9. doi: 10.1038/s41564-020-0688-y
75. Ju B, Zhang Q, Ge J, Wang R, Sun J, Ge X, et al. Human neutralizing antibodies elicited by SARS-CoV-2 infection. Nature (2020) 584(7819):115–9. doi: 10.1038/s41586-020-2380-z
76. Van Den Brink EN, Ter Meulen J, Cox F, Jongeneelen MA, Thijsse A, Throsby M, et al. Molecular and biological characterization of human monoclonal antibodies binding to the spike and nucleocapsid proteins of severe acute respiratory syndrome coronavirus. J virol (2005) 79(3):1635–44. doi: 10.1128/JVI.79.3.1635-1644.2005
77. Lee N, Jeong S, Lee SK, Cho E-J, Hyun J, Park M-J, et al. Quantitative analysis of anti-n and anti-s antibody titers of SARS-CoV-2 infection after the third dose of CoVid-19 vaccination. Vaccines (2022) 10(7):1143. doi: 10.3390/vaccines10071143
78. Brochot E, Demey B, Touzé A, Belouzard S, Dubuisson J, Schmit J-L, et al. Anti-spike, anti-nucleocapsid and neutralizing antibodies in SARS-CoV-2 inpatients and asymptomatic individuals. Front Microbiol (2020) 2468. doi: 10.3389/fmicb.2020.584251
79. Jiang S, Zhang X, Yang Y, Hotez PJ, Du L. Neutralizing antibodies for the treatment of COVID-19. Nat Biomed Eng (2020) 4(12):1134–9. doi: 10.1038/s41551-020-00660-2
80. Fu J, Wei C, He J, Zhang L, Zhou J, Balaji KS, et al. Evaluation and characterization of HSPA5 (GRP78) expression profiles in normal individuals and cancer patients with COVID-19. Int J Biol Sci (2021) 17(3):897. doi: 10.7150/ijbs.54055
81. Vankadari N, Wilce JA. Emerging COVID-19 coronavirus: glycan shield and structure prediction of spike glycoprotein and its interaction with human CD26. Emerg Microbes infect (2020) 9(1):601–4. doi: 10.1080/22221751.2020.1739565
82. Wang S, Qiu Z, Hou Y, Deng X, Xu W, Zheng T, et al. AXL is a candidate receptor for SARS-CoV-2 that promotes infection of pulmonary and bronchial epithelial cells. Cell Res (2021) 31(2):126–40. doi: 10.1038/s41422-020-00460-y
83. Radzikowska U, Ding M, Tan G, Zhakparov D, Peng Y, Wawrzyniak P, et al. Distribution of ACE2, CD147, CD26, and other SARS-CoV-2 associated molecules in tissues and immune cells in health and in asthma, COPD, obesity, hypertension, and COVID-19 risk factors. Allergy (2020) 75(11):2829–45. doi: 10.1111/all.14429
84. Shen S, Zhang J, Fang Y, Lu S, Wu J, Zheng X, et al. SARS-CoV-2 interacts with platelets and megakaryocytes via ACE2-independent mechanism. J Hematol Oncol (2021) 14(1):1–5. doi: 10.1186/s13045-021-01082-6
85. Cantuti-Castelvetri L, Ojha R, Pedro LD, Djannatian M, Franz J, Kuivanen S, et al. Neuropilin-1 facilitates SARS-CoV-2 cell entry and infectivity. Science (2020) 370(6518):856–60. doi: 10.1126/science.abd2985
86. Abebe EC, Ayele TM, Muche ZT, Dejenie TA. Neuropilin 1: a novel entry factor for SARS-CoV-2 infection and a potential therapeutic target. Biol: Targets Ther (2021) 15:143. doi: 10.2147/BTT.S307352
87. Lu LL, Suscovich TJ, Fortune SM, Alter G. Beyond binding: antibody effector functions in infectious diseases. Nat Rev Immunol (2018) 18(1):46–61. doi: 10.1038/nri.2017.106
88. Stein SR, Ramelli SC, Grazioli A, Chung JY, Singh M, Yinda CK, et al. NIH COVID-19 Autopsy Consortium; Peterson KE, Cohen JI, de Wit E, Vannella KM, Hewitt SM, Kleiner DE, Chertow DS. SARS-CoV-2 infection and persistence in the human body and brain at autopsy. Nature (2022) 612(7941):758–63. doi: 10.1038/s41586-022-05542-y
89. Dufloo J, Grzelak L, Staropoli I, Madec Y, Tondeur L, Anna F, et al. Asymptomatic and symptomatic SARS-CoV-2 infections elicit polyfunctional antibodies. Cell Rep Med (2021) 2(5):100275. doi: 10.1016/j.xcrm.2021.100275
90. Schäfer A, Muecksch F, Lorenzi JC, Leist SR, Cipolla M, Bournazos S, et al. Antibody potency, effector function, and combinations in protection and therapy for SARS-CoV-2 infection in vivo. J Exp Med (2021) 218(3). doi: 10.1084/jem.20201993
91. The US Food and Drug Adminstration. EUA authorized serology test performance. FDA (2022). Available at: https://www.fda.gov/medical-devices/coronavirus-disease-2019-covid-19-emergency-use-authorizations-medical-devices/eua-authorized-serology-test-performance
92. Pegu A, O’Connell SE, Schmidt SD, O’Dell S, Talana CA, Lai L, et al. Durability of mRNA-1273 vaccine–induced antibodies against SARS-CoV-2 variants. Science (2021) 373(6561):1372–7. doi: 10.1126/science.abj4176
93. Trombetta CM, Marchi S, Viviani S, Manenti A, Benincasa L, Ruello A, et al. Serum neutralizing activity against b. 1.1. 7, b. 1.351 and p. 1 SARS-CoV-2 variants of concern in hospitalized COVID-19 patients. Viruses (2021) 13(7):1347. doi: 10.3390/v13071347
94. Luo YR, Chakraborty I, Yun C, Wu AH, Lynch KL. Kinetics of SARS-CoV-2 antibody avidity maturation and association with disease severity. MedRXiv (2020). doi: 10.1101/2020.07.30.20165522
95. Rikhtegaran Tehrani Z, Saadat S, Saleh E, Ouyang X, Constantine N, DeVico AL, et al. Performance of nucleocapsid and spike-based SARS-CoV-2 serologic assays. PloS One (2020) 15(11):e0237828. doi: 10.1101/2020.08.05.20168476
96. Peghin M, Bontempo G, De Martino M, Palese A, Gerussi V, Graziano E, et al. Evaluation of qualitative and semi-quantitative cut offs for rapid diagnostic lateral flow test in relation to serology for the detection of SARS-CoV-2 antibodies: findings of a prospective study. BMC Infect Dis (2022) 22(1):1–9. doi: 10.1186/s12879-022-07786-5
98. Higgins V, Fabros A, Kulasingam V. Quantitative measurement of anti-SARS-CoV-2 antibodies: analytical and clinical evaluation. J Clin Microbiol (2021) 59(4):e03149-20. doi: 10.1128/JCM.03149-20
99. Legros V, Denolly S, Vogrig M, Boson B, Siret E, Rigaill J, et al. A longitudinal study of SARS-CoV-2-infected patients reveals a high correlation between neutralizing antibodies and COVID-19 severity. Cell Mol Immunol (2021) 18(2):318–27. doi: 10.1038/s41423-020-00588-2
100. Mendoza EJ, Manguiat K, Wood H, Drebot M. Two detailed plaque assay protocols for the quantification of infectious SARS-CoV-2. Curr Protoc Microbiol (2020) 57(1):cpmc105. doi: 10.1002/cpmc.105
101. Chen W, Zhang J, Qin X, Wang W, Xu M, Wang L-F, et al. SARS-CoV-2 neutralizing antibody levels are correlated with severity of COVID-19 pneumonia. Biomed Pharmacother (2020) 130:110629. doi: 10.1016/j.biopha.2020.110629
102. Payne S. Viruses: from understanding to investigation. Elsevier (2022). Available at: https://www.elsevier.com/books/viruses/payne/978-0-323-90385-1
103. Luo J, Brakel A, Krizsan A, Ludwig T, Mötzing M, Volke D, et al. Sensitive and specific serological ELISA for the detection of SARS-CoV-2 infections. Virol J (2022) 19(1):1–10. doi: 10.1186/s12985-022-01768-4
104. Okba NM, Müller MA, Li W, Wang C, GeurtsvanKessel CH, Corman VM, et al. SARS-CoV-2 specific antibody responses in COVID-19 patients. MedRxiv (2020). doi: 10.1101/2020.03.18.20038059
105. Gededzha MP, Mampeule N, Jugwanth S, Zwane N, David A, Burgers WA, et al. Performance of the EUROIMMUN anti-SARS-CoV-2 ELISA assay for detection of IgA and IgG antibodies in south Africa. PloS One (2021) 16(6):e0252317. doi: 10.1371/journal.pone.0252317
106. Bryan A, Pepper G, Wener MH, Fink SL, Morishima C, Chaudhary A, et al. Performance characteristics of the Abbott architect SARS-CoV-2 IgG assay and seroprevalence in Boise, Idaho. J Clin Microbiol (2020) 58(8):e00941-20. doi: 10.1128/JCM.00941-20
107. Meyer B, Reimerink J, Torriani G, Brouwer F, Godeke G-J, Yerly S, et al. Validation and clinical evaluation of a SARS-CoV-2 surrogate virus neutralisation test (sVNT). Emerg Microbes infect (2020) 9(1):2394–403. doi: 10.1080/22221751.2020.1835448
108. Ellis JE, Guest P, Lawson V, Loecherbach J, Lindner N, McCulloch A. Performance evaluation of the microfluidic antigen LumiraDx SARS-CoV-2 and flu A/B test in diagnosing COVID-19 and influenza in patients with respiratory symptoms. Infect Dis Ther (2022) 11(6):2099–109. doi: 10.1007/s40121-022-00696-8
109. Wiwe EF, Carlsson ER, Rasmussen CL, Rasmussen P, Ougaard R, Hansen SI, et al. Long-term comparison of 7 SARS-CoV-2 antibody assays in the north Zealand covid-19 cohort. J Appl Lab Med (2022) 7(3):711–26. doi: 10.1093/jalm/jfab173
110. Bonelli F, Blocki FA, Bunnell T, Chu E, De La OA, Grenache DG, et al. Evaluation of the automated LIAISON® SARS-CoV-2 TrimericS IgG assay for the detection of circulating antibodies. Clin Chem Lab Med (2021) 59(8):1463–7. doi: 10.1515/cclm-2021-0023
111. Simon H-U, Karaulov AV, Bachmann MF. Strategies to prevent SARS-CoV-2-mediated eosinophilic disease in association with COVID-19 vaccination and infection. Int Arch Allergy Immunol (2020) 181(8):624–8. doi: 10.1159/000509368
112. Bergwerk M, Gonen T, Lustig Y, Amit S, Lipsitch M, Cohen C, et al. Covid-19 breakthrough infections in vaccinated health care workers. New Engl J Med (2021) 385(16):1474–84. doi: 10.1056/NEJMoa2109072
113. Yamayoshi S, Yasuhara A, Ito M, Akasaka O, Nakamura M, Nakachi I, et al. Antibody titers against SARS-CoV-2 decline, but do not disappear for several months. EClinicalMedicine (2021) 32:100734. doi: 10.1016/j.eclinm.2021.100734
114. Secchi M, Bazzigaluppi E, Brigatti C, Marzinotto I, Tresoldi C, Rovere-Querini P, et al. COVID-19 survival associates with the immunoglobulin response to the SARS-CoV-2 spike receptor binding domain. J Clin Invest (2020) 130(12). doi: 10.1172/JCI142804
115. Shrock E, Fujimura E, Kula T, Timms RT, Lee I-H, Leng Y, et al. Viral epitope profiling of COVID-19 patients reveals cross-reactivity and correlates of severity. Science (2020) 370(6520):eabd4250.
116. Woodruff MC, Ramonell RP, Nguyen DC, Cashman KS, Saini AS, Haddad NS, et al. Extrafollicular b cell responses correlate with neutralizing antibodies and morbidity in COVID-19. Nat Immunol (2020) 21(12):1506–16. doi: 10.1038/s41590-020-00814-z
117. Long Q-X, Tang X-J, Shi Q-L, Li Q, Deng H-J, Yuan J, et al. Clinical and immunological assessment of asymptomatic SARS-CoV-2 infections. Nat Med (2020) 26(8):1200–4. doi: 10.1038/s41591-020-0965-6
118. Liu Z-L, Liu Y, Wan L-G, Xiang T-X, Le A-P, Liu P, et al. Antibody profiles in mild and severe cases of COVID-19. Clin Chem (2020) 66(8):1102–4. doi: 10.1093/clinchem/hvaa137
119. Wang P, Liu L, Nair MS, Yin MT, Luo Y, Wang Q, et al. SARS-CoV-2 neutralizing antibody responses are more robust in patients with severe disease. Emerg Microbes infect (2020) 9(1):2091–3. doi: 10.1080/22221751.2020.1823890
120. Thabrani A, Hadi WS, Thobari JA, Novianti Z, Juliana I, Suharto A, et al. Convalescent plasma as a treatment modality for coronavirus disease 2019 in Indonesia: A case reports. Ann Med Surg (2021) 66:102444. doi: 10.1016/j.amsu.2021.102444
121. Estcourt L, Callum J. Convalescent plasma for Covid-19–making sense of the inconsistencies. Mass Med Soc; (2022) p:1753–4. doi: 10.1056/NEJMe2204332
122. Libster R, Pérez Marc G, Wappner D, Coviello S, Bianchi A, Braem V, et al. Early high-titer plasma therapy to prevent severe covid-19 in older adults. New Engl J Med (2021) 384(7):610–8. doi: 10.1056/NEJMoa2033700
123. Chen L, Xiong J, Bao L, Shi Y. Convalescent plasma as a potential therapy for COVID-19. Lancet Infect dis (2020) 20(4):398–400. doi: 10.1016/S1473-3099(20)30141-9
124. Weisblum Y, Schmidt F, Zhang F, DaSilva J, Poston D, Lorenzi JC, et al. Escape from neutralizing antibodies by SARS-CoV-2 spike protein variants. Elife (2020) 9:e61312. doi: 10.7554/eLife.61312
125. Lee WS, Wheatley AK, Kent SJ, DeKosky BJ. Antibody-dependent enhancement and SARS-CoV-2 vaccines and therapies. Nat Microbiol (2020) 5(10):1185–91. doi: 10.1038/s41564-020-00789-5
126. Wong V, Dai D, Wu A, Sung J. Treatment of severe acute respiratory syndrome with convalescent plasma. Hong Kong Med J (2003) 9(3):199–201.
127. Zhou B, Zhong N, Guan Y. Treatment with convalescent plasma for influenza a (H5N1) infection. New Engl J Med (2007) 357(14):1450–1. doi: 10.1056/NEJMc070359
128. Van Griensven J, Edwards T, de Lamballerie X, Semple MG, Gallian P, Baize S, et al. Evaluation of convalescent plasma for Ebola virus disease in Guinea. New Engl J Med (2016) 374(1):33–42. doi: 10.1056/NEJMoa1511812
129. Sui J, Li W, Murakami A, Tamin A, Matthews LJ, Wong SK, et al. Potent neutralization of severe acute respiratory syndrome (SARS) coronavirus by a human mAb to S1 protein that blocks receptor association. Proc Natl Acad Sci (2004) 101(8):2536–41. doi: 10.1073/pnas.0307140101
130. Zhu Z, Chakraborti S, He Y, Roberts A, Sheahan T, Xiao X, et al. Potent cross-reactive neutralization of SARS coronavirus isolates by human monoclonal antibodies. Proc Natl Acad Sci (2007) 104(29):12123–8. doi: 10.1073/pnas.0701000104
131. Duan K, Liu B, Li C, Zhang H, Yu T, Qu J, et al. Effectiveness of convalescent plasma therapy in severe COVID-19 patients. Proc Natl Acad Sci (2020) 117(17):9490–6. doi: 10.1073/pnas.2004168117
132. Shen C, Wang Z, Zhao F, Yang Y, Li J, Yuan J, et al. Treatment of 5 critically ill patients with COVID-19 with convalescent plasma. Jama (2020) 323(16):1582–9. doi: 10.1001/jama.2020.4783
133. Bloch EM, Shoham S, Casadevall A, Sachais BS, Shaz B, Winters JL, et al. Deployment of convalescent plasma for the prevention and treatment of COVID-19. J Clin Invest (2020) 130(6):2757–65. doi: 10.1172/JCI138745
134. Casadevall A, Joyner MJ, Pirofski L-A. A randomized trial of convalescent plasma for COVID-19–potentially hopeful signals. Jama (2020) 324(5):455–7. doi: 10.1001/jama.2020.10218
135. Wu Y, Wang F, Shen C, Peng W, Li D, Zhao C, et al. A noncompeting pair of human neutralizing antibodies block COVID-19 virus binding to its receptor ACE2. Science (2020) 368(6496):1274–8. doi: 10.1126/science.abc2241
136. Joyner MJ, Wright RS, Fairweather D, Senefeld JW, Bruno KA, Klassen SA, et al. Early safety indicators of COVID-19 convalescent plasma in 5000 patients. J Clin Invest (2020) 130(9).
137. Ahn JY, Sohn Y, Lee SH, Cho Y, Hyun JH, Baek YJ, et al. Use of convalescent plasma therapy in two COVID-19 patients with acute respiratory distress syndrome in Korea. J Korean Med Sci (2020) 35(14):e149. doi: 10.3346/jkms.2020.35.e149
138. Zhang B, Liu S, Tan T, Huang W, Dong Y, Chen L, et al. Treatment with convalescent plasma for critically ill patients with severe acute respiratory syndrome coronavirus 2 infection. Chest (2020) 158(1):e9–e13. doi: 10.1016/j.chest.2020.03.039
139. Pau AK, Aberg J, Baker J, Belperio PS, Coopersmith C, Crew P, et al. Convalescent plasma for the treatment of COVID-19: perspectives of the national institutes of health COVID-19 treatment guidelines panel. Ann Internal Med (2021) 174(1):93–9. doi: 10.7326/M20-6448
140. Sullivan DJ, Gebo KA, Shoham S, Bloch EM, Lau B, Shenoy AG, et al. Early outpatient treatment for covid-19 with convalescent plasma. New Engl J Med (2022) 386(18):1700–11. doi: 10.1056/NEJMoa2119657
141. Piechotta V, Iannizzi C, Chai KL, Valk SJ, Kimber C, Dorando E, et al. Convalescent plasma or hyperimmune immunoglobulin for people with COVID-19: a living systematic review. Cochrane Database Syst Rev (2021) 5(5):CD013600. doi: 10.1002/14651858.CD013600.pub4
142. Alemany A, Millat-Martinez P, Corbacho-Monné M, Malchair P, Ouchi D, Ruiz-Comellas A, et al. High-titre methylene blue-treated convalescent plasma as an early treatment for outpatients with COVID-19: a randomised, placebo-controlled trial. Lancet Respir Med (2022) 10(3):278–88. doi: 10.1016/S2213-2600(21)00545-2
143. Tuccori M, Ferraro S, Convertino I, Cappello E, Valdiserra G, Blandizzi C, et al. Anti-SARS-CoV-2 neutralizing monoclonal antibodies: clinical pipeline. MAbs. (2020) 12(1):1854149. doi: 10.1080/19420862.2020.1854149
144. Renn A, Fu Y, Hu X, Hall MD, Simeonov A. Fruitful neutralizing antibody pipeline brings hope to defeat SARS-Cov-2. Trends Pharmacol Sci (2020) 41(11):815–29. doi: 10.1016/j.tips.2020.07.004
145. Mussini C, Cozzi-Lepri A. Another piece in the COVID-19 treatment puzzle. Lancet (London England) (2022) 399(10325):609. doi: 10.1016/S0140-6736(22)00154-4
146. Lin WT, Hung SH, Lai CC, Wang CY, Chen CH. The impact of neutralizing monoclonal antibodies on the outcomes of COVID-19 outpatients: A systematic review and meta-analysis of randomized controlled trials. J Med virol (2022) 94(5):2222–9. doi: 10.1002/jmv.27623
147. FDA U. Coronavirus (COVID-19) update: FDA limits use of certain monoclonal antibodies to treat COVID-19 due to the omicron variant. FDA (2022).
148. THE FDA Fact Sheet. Emergency REGEN-COV usage revision. Emergency REGEN-COV usage revision (2022). Available at: https://www.regencov.com/.
149. Pisil Y, Yazici Z, Shida H, Miura T. Is SARS-CoV-2 neutralized more effectively by IgM and IgA than IgG having the same fab region? Pathogens (2021) 10(6):751. doi: 10.3390/pathogens10060751
150. Baden LR, El Sahly HM, Essink B, Kotloff K, Frey S, Novak R, et al. Efficacy and safety of the mRNA-1273 SARS-CoV-2 vaccine. New Engl J Med (2021) 384(5):403–16.
151. Voysey M, Clemens SAC, Madhi SA, Weckx LY, Folegatti PM, Aley PK, et al. Safety and efficacy of the ChAdOx1 nCoV-19 vaccine (AZD1222) against SARS-CoV-2: an interim analysis of four randomised controlled trials in Brazil, south Africa, and the UK. Lancet (2021) 397(10269):99–111.
152. Polack FP, Thomas SJ, Kitchin N, Absalon J, Gurtman A, Lockhart S, et al. Safety and efficacy of the BNT162b2 mRNA covid-19 vaccine. New Engl J Med (2020) 383(27):2603–15.
156. Corbett KS, Edwards DK, Leist SR, Abiona OM, Boyoglu-Barnum S, Gillespie RA, et al. SARS-CoV-2 mRNA vaccine design enabled by prototype pathogen preparedness. Nature (2020) 586(7830):567–71. doi: 10.1038/s41586-020-2622-0
157. Mulligan MJ, Lyke KE, Kitchin N, Absalon J, Gurtman A, Lockhart S, et al. Phase I/II study of COVID-19 RNA vaccine BNT162b1 in adults. Nature (2020) 586(7830):589–93. doi: 10.1038/s41586-020-2639-4
158. Ewer KJ, Barrett JR, Belij-Rammerstorfer S, Sharpe H, Makinson R, Morter R, et al. T Cell and antibody responses induced by a single dose of ChAdOx1 nCoV-19 (AZD1222) vaccine in a phase 1/2 clinical trial. Nat Med (2021) 27(2):270–8. doi: 10.1038/s41591-020-01194-5
159. Folegatti PM, Ewer KJ, Aley PK, Angus B, Becker S, Belij-Rammerstorfer S, et al. Safety and immunogenicity of the ChAdOx1 nCoV-19 vaccine against SARS-CoV-2: a preliminary report of a phase 1/2, single-blind, randomised controlled trial. Lancet (2020) 396(10249):467–78. doi: 10.1016/S0140-6736(20)31604-4
160. Jones I, Roy P. Sputnik V COVID-19 vaccine candidate appears safe and effective. Lancet (2021) 397(10275):642–3. doi: 10.1016/S0140-6736(21)00191-4
162. Tostanoski LH, Wegmann F, Martinot AJ, Loos C, McMahan K, Mercado NB, et al. Ad26 vaccine protects against SARS-CoV-2 severe clinical disease in hamsters. Nat Med (2020) 26(11):1694–700. doi: 10.1038/s41591-020-1070-6
163. FDA U. Coronavirus (COVID-19) update: FDA authorizes emergency use of novavax COVID-19 vaccine, adjuvanted. FDA (2022).
164. Dunkle LM, Kotloff KL, Gay CL, Áñez G, Adelglass JM, Barrat Hernández AQ, et al. Efficacy and safety of NVX-CoV2373 in adults in the united states and Mexico. New Engl J Med (2022) 386(6):531–43. doi: 10.1056/NEJMoa2116185
165. WHO. NVX-CoV2373 recombinant, adjuvanted COVID-19 vaccine. WHO (2022). Available at: https://www.who.int/publications/m/item/nvx-cov2373-recombinant-adjuvanted-covid19-vaccine.
166. Mallapaty S. China COVID vaccine reports mixed results–what does that mean for the pandemic? Nature (2021) 15. doi: 10.1038/d41586-021-00094-z
167. Khoury DS, Cromer D, Reynaldi A, Schlub TE, Wheatley AK, Juno JA, et al. Neutralizing antibody levels are highly predictive of immune protection from symptomatic SARS-CoV-2 infection. Nat Med (2021) 27(7):1205–11. doi: 10.1038/s41591-021-01377-8
168. Krammer F. A correlate of protection for SARS-CoV-2 vaccines is urgently needed. Nat Med (2021) 27(7):1147–8. doi: 10.1038/s41591-021-01432-4
169. Liu L, Wang P, Nair MS, Yu J, Rapp M, Wang Q, et al. Potent neutralizing antibodies against multiple epitopes on SARS-CoV-2 spike. Nature (2020) 584(7821):450–6. doi: 10.1038/s41586-020-2571-7
170. Keech C, Albert G, Cho I, Robertson A, Reed P, Neal S, et al. Phase 1–2 trial of a SARS-CoV-2 recombinant spike protein nanoparticle vaccine. New Engl J Med (2020) 383(24):2320–32. doi: 10.1056/NEJMoa2026920
171. Rodda LB, Netland J, Shehata L, Pruner KB, Morawski PA, Thouvenel CD, et al. Functional SARS-CoV-2-specific immune memory persists after mild COVID-19. Cell (2021) 184(1):169–83.e17. doi: 10.1016/j.cell.2020.11.029
172. Wen J, Cheng Y, Ling R, Dai Y, Huang B, Huang W, et al. Antibody-dependent enhancement of coronavirus. Int J Infect Dis (2020) 100:483–9. doi: 10.1016/j.ijid.2020.09.015
173. Shimizu J, Sasaki T, Koketsu R, Morita R, Yoshimura Y, Murakami A, et al. Reevaluation of antibody-dependent enhancement of infection in anti-SARS-CoV-2 therapeutic antibodies and mRNA-vaccine antisera using FcR-and ACE2-positive cells. Sci Rep (2022) 12(1):1–9. doi: 10.1038/s41598-022-19993-w
174. Gupta A, Gonzalez-Rojas Y, Juarez E, Crespo Casal M, Moya J, Falci DR, et al. Early treatment for covid-19 with SARS-CoV-2 neutralizing antibody sotrovimab. New Engl J Med (2021) 385(21):1941–50. doi: 10.1056/NEJMoa2107934
175. Li D, Edwards RJ, Manne K, Martinez DR, Schäfer A, Alam SM, et al. In vitro and in vivo functions of SARS-CoV-2 infection-enhancing and neutralizing antibodies. Cell (2021) 184(16):4203–19.e32. doi: 10.1016/j.cell.2021.06.021
176. Zhou Y, Liu Z, Li S, Xu W, Zhang Q, Silva IT, et al. Enhancement versus neutralization by SARS-CoV-2 antibodies from a convalescent donor associates with distinct epitopes on the RBD. Cell Rep (2021) 34(5):108699. doi: 10.1016/j.celrep.2021.108699
177. Liu Y, Soh WT, Kishikawa J-i, Hirose M, Nakayama EE, Li S, et al. An infectivity-enhancing site on the SARS-CoV-2 spike protein targeted by antibodies. Cell (2021) 184(13):3452–66.e18. doi: 10.1016/j.cell.2021.05.032
178. Wang Z, Deng T, Zhang Y, Niu W, Nie Q, Yang S, et al. ACE2 can act as the secondary receptor in the FcγR-dependent ADE of SARS-CoV-2 infection. Iscience (2022) 25(1):103720. doi: 10.1016/j.isci.2021.103720
179. Abayasingam A, Balachandran H, Agapiou D, Hammoud M, Rodrigo C, Keoshkerian E, et al. Long-term persistence of RBD+ memory b cells encoding neutralizing antibodies in SARS-CoV-2 infection. Cell Rep Med (2021) 2(4):100228. doi: 10.1016/j.xcrm.2021.100228
180. Li Y, Ma M-l, Lei Q, Wang F, Hong W, Lai D-y, et al. Linear epitope landscape of the SARS-CoV-2 spike protein constructed from 1,051 COVID-19 patients. Cell Rep (2021) 34(13):108915. doi: 10.1016/j.celrep.2021.108915
181. Bayarri-Olmos R, Idorn M, Rosbjerg A, Pérez-Alós L, Hansen CB, Johnsen LB, et al. SARS-CoV-2 neutralizing antibody responses towards full-length spike protein and the receptor-binding domain. J Immunol (2021) 207(3):878–87. doi: 10.4049/jimmunol.2100272
182. Walsh EE, Frenck RW Jr., Falsey AR, Kitchin N, Absalon J, Gurtman A, et al. Safety and immunogenicity of two RNA-based covid-19 vaccine candidates. New Engl J Med (2020) 383(25):2439–50. doi: 10.1056/NEJMoa2027906
183. Yuan L, Tang Q, Zhu H, Guan Y, Cheng T, Xia N. SARS-CoV-2 infection and disease outcomes in non-human primate models: advances and implications. Emerg Microbes infect (2021) 10(1):1881–9. doi: 10.1080/22221751.2021.1976598
184. Haynes BF, Corey L, Fernandes P, Gilbert PB, Hotez PJ, Rao S, et al. Prospects for a safe COVID-19 vaccine. Sci Trans Med (2020) 12(568):eabe0948. doi: 10.1126/scitranslmed.abe0948
185. Mahase E. Covid-19: WHO says rollout of AstraZeneca vaccine should continue, as Europe divides over safety. Br Med J Publishing Group (2021). doi: 10.1136/bmj.n728
186. Pai M, Grill A, Ivers N. Vaccine-induced prothrombotic immune thrombocytopenia VIPIT following AstraZeneca COVID-19 vaccination. Sci Briefs Ontario COVID-19 Sci Advisory Table (2021) 1(17). doi: 10.47326/ocsat.2021.02.17.1.0
187. Mahase E. Covid-19: Unusual blood clots are “very rare side effect” of janssen vaccine, says EMA. Br Med J Publishing Group (2021). doi: 10.1136/bmj.n1046
188. Oliver SE, Shimabukuro TT. Johnson & Johnson/Janssen COVID-19 vaccine and cerebral venous sinus thrombosis with thrombocytopenia–update for clinicians on early detection and treatment. (2021).
189. Waheed S, Bayas A, Hindi F, Rizvi Z, Espinosa PS. Neurological complications of COVID-19: Guillain-barre syndrome following pfizer COVID-19 vaccine. Cureus (2021) 13(2):e13426. doi: 10.7759/cureus.13426
190. Hanson KE, Goddard K, Lewis N, Fireman B, Myers TR, Bakshi N, et al. Incidence of Guillain-Barré syndrome after COVID-19 vaccination in the vaccine safety datalink. JAMA netw Open (2022) 5(4):e228879–e. doi: 10.1001/jamanetworkopen.2022.8879
191. Garcia-Beltran WF, Lam EC, Denis KS, Nitido AD, Garcia ZH, Hauser BM, et al. Multiple SARS-CoV-2 variants escape neutralization by vaccine-induced humoral immunity. Cell (2021). 184(9):2372–83.e9. doi: 10.1016/j.cell.2021.03.013
192. Chekol Abebe E, Tiruneh G, Medhin M, Behaile T, Mariam A, Asmamaw Dejenie T, et al. Mutational pattern, impacts and potential preventive strategies of omicron SARS-CoV-2 variant infection. Infect Drug Resist (2022) 15:1871–87. doi: 10.2147/IDR.S360103
193. Collier DA, De Marco A, Ferreira IA, Meng B, Datir R, Walls AC, et al. SARS-CoV-2 b. 1.1. 7 escape from mRNA vaccine-elicited neutralizing antibodies. MedRxiv (2021).
194. Kemp S, Harvey W, Datir R, Collier D, Ferreira I, Carabelii A, et al. Recurrent emergence and transmission of a SARS-CoV-2 spike deletion ΔH69/V70. bioRxiv (2020). doi: 10.1101/2020.12.14.422555
195. Tegally H, Wilkinson E, Giovanetti M, Iranzadeh A, Fonseca V, Giandhari J, et al. Emergence and rapid spread of a new severe acute respiratory syndrome-related coronavirus 2 (SARS-CoV-2) lineage with multiple spike mutations in south Africa. medRxiv (2020). doi: 10.1101/2020.12.21.20248640
196. Zhou B, Thao TTN, Hoffmann D, Taddeo A, Ebert N, Labroussaa F, et al. SARS-CoV-2 spike D614G variant confers enhanced replication and transmissibility. bioRxiv (2020). doi: 10.1101/2020.10.27.357558
197. Kupferschmidt K. New coronavirus variants could cause more reinfections, require updated vaccines. Sci COVID-19 Rep (2021). doi: 10.1126/science.abg6028
198. Herper M, Branswell H. New data shed light on efficacy of J&J’s single-dose covid vaccine. (2021).
199. Wang Z, Schmidt F, Weisblum Y, Muecksch F, Barnes CO, Finkin S, et al. mRNA vaccine-elicited antibodies to SARS-CoV-2 and circulating variants. Nature (2021) 592(7855):616–22. doi: 10.1038/s41586-021-03324-6
200. Wang P, Casner RG, Nair MS, Wang M, Yu J, Cerutti G, et al. Increased resistance of SARS-CoV-2 variant p. 1 to antibody neutralization. Cell Host Microbe (2021). d29(5):747–51.e4. doi: 10.1016/j.chom.2021.04.007
201. Tada T, Dcosta BM, Samanovic-Golden M, Herati RS, Cornelius A, Mulligan MJ, et al. Neutralization of viruses with European, south African, and united states SARS-CoV-2 variant spike proteins by convalescent sera and BNT162b2 mRNA vaccine-elicited antibodies. bioRxiv (2021). doi: 10.1101/2021.02.05.430003
202. Tartof SY, Slezak JM, Fischer H, Hong V, Ackerson BK, Ranasinghe ON, et al. Effectiveness of mRNA BNT162b2 COVID-19 vaccine up to 6 months in a large integrated health system in the USA: a retrospective cohort study. Lancet (2021) 398(10309):1407–16. doi: 10.1016/S0140-6736(21)02183-8
203. Rosenberg ES, Dorabawila V, Easton D, Bauer UE, Kumar J, Hoen R, et al. Covid-19 vaccine effectiveness in new York state. New Engl J Med (2022) 386(2):116–27. doi: 10.1056/NEJMoa2116063
204. Garcia-Beltran WF, Denis KJS, Hoelzemer A, Lam EC, Nitido AD, Sheehan ML, et al. mRNA-based COVID-19 vaccine boosters induce neutralizing immunity against SARS-CoV-2 omicron variant. Cell (2022) 185(3):457–66.e4. doi: 10.1016/j.cell.2021.12.033
205. Gruell H, Vanshylla K, Tober-Lau P, Hillus D, Schommers P, Lehmann C, et al. mRNA booster immunization elicits potent neutralizing serum activity against the SARS-CoV-2 omicron variant. Nat Med (2022) 28(3):477–80. doi: 10.1038/s41591-021-01676-0
206. Abu-Raddad LJ, Chemaitelly H, Ayoub HH, AlMukdad S, Yassine HM, Al-Khatib HA, et al. Effect of mRNA vaccine boosters against SARS-CoV-2 omicron infection in Qatar. New Engl J Med (2022) 386(19):1804–16. doi: 10.1056/NEJMoa2200797
207. Andrews N, Stowe J, Kirsebom F, Toffa S, Rickeard T, Gallagher E, et al. Covid-19 vaccine effectiveness against the omicron (B. 1.1. 529) variant. New Engl J Med (2022) 386(16):1532–46. doi: 10.1056/NEJMoa2119451
208. Regev-Yochay G, Gonen T, Gilboa M, Mandelboim M, Indenbaum V, Amit S, et al. Efficacy of a fourth dose of COVID-19 mRNA vaccine against omicron. New Engl J Med (2022) 386(14):1377–80. doi: 10.1056/NEJMc2202542
Keywords: neutralizing antibodies, protective role, clinical application, COVID 19 vaccine, SARS-COV-2 variants
Citation: Abebe EC and Dejenie TA (2023) Protective roles and protective mechanisms of neutralizing antibodies against SARS-CoV-2 infection and their potential clinical implications. Front. Immunol. 14:1055457. doi: 10.3389/fimmu.2023.1055457
Received: 27 September 2022; Accepted: 03 January 2023;
Published: 19 January 2023.
Edited by:
Juarez Antonio Simões Quaresma, Federal University of Pará, BrazilReviewed by:
Eakachai Prompetchara, Chulalongkorn University, ThailandIrene Ramos, Icahn School of Medicine at Mount Sinai, United States
Copyright © 2023 Abebe and Dejenie. This is an open-access article distributed under the terms of the Creative Commons Attribution License (CC BY). The use, distribution or reproduction in other forums is permitted, provided the original author(s) and the copyright owner(s) are credited and that the original publication in this journal is cited, in accordance with accepted academic practice. No use, distribution or reproduction is permitted which does not comply with these terms.
*Correspondence: Endeshaw Chekol Abebe, ZW5kZXNoYXdjaGVrb2xlQGdtYWlsLmNvbQ==