- 1Department of Nephrology, The Second Affiliated Hospital of Nanchang University, Nanchang, China
- 2Department of Blood Transfusion, The Second Affiliated Hospital of Nanchang University, Nanchang, China
Up to now, coronavirus disease 2019 (COVID-19) is still affecting worldwide due to its highly infectious nature anrapid spread. Diabetic kidney disease (DKD) is an independent risk factor for severe COVID-19 outcomes, and they have a certain correlation in some aspects. Particularly, the activated renin–angiotensin–aldosterone system, chronic inflammation, endothelial dysfunction, and hypercoagulation state play an important role in the underlying mechanism linking COVID-19 to DKD. The dipeptidyl peptidase-4 inhibitor is considered a potential therapy for COVID-19 and has similarly shown organ protection in DKD. In addition, neuropilin-1 as an alternative pathway for angiotensin-converting enzyme 2 also contributes to severe acute respiratory syndrome coronavirus 2 entering the host cells, and its decreased expression can affect podocyte migration and adhesion. Here, we review the pathogenesis and current evidence of the interaction of DKD and COVID-19, as well as focus on elevated blood glucose following vaccination and its possible mechanism. Grasping the pathophysiology of DKD patients with COVID-19 is of great clinical significance for the formulation of therapeutic strategies.
Introduction
The outbreak of the coronavirus disease 2019 (COVID-19) in Wuhan, China, in December 2019 is undoubtedly a serious blow to global health security and economic development. Several countries experienced a second or third wave, especially after the emergence of mutant viruses. This pandemic, caused by severe acute respiratory syndrome coronavirus 2 (SARS-CoV-2), enters host cells by binding to the angiotensin-converting enzyme 2 (ACE2) via a spike protein on its surface (1), initially presenting with fever, cough, and fatigue; as the condition progresses, hypoxemia, respiratory failure, and even death may occur (2). Meanwhile, the presence of comorbidities increases the risk of SARS-CoV-2 infection, including hypertension, obesity, and diabetes (3, 4).
Diabetic kidney disease (DKD) is one of the most common microvascular complications of both types of diabetes. The increasing prevalence of diabetes poses a major threat to DKD, especially an increase in type 2 diabetes (T2DM), and DKD is the most common cause of end-stage renal disease (5). During this pandemic, a study reported that DKD patients infected with SARS-CoV-2 require a longer hospital stay to recover and has the greatest impact on patients’ prognosis (6). Leon-Abarca et al. reported that patients with DKD are twice as likely to have COVID-19 pneumonia as patients with chronic kidney disease and have higher intubation rates and case-fatality rates, which may be associated with pro-inflammatory state and immune dysfunction (7). Notably, among the patients with maintenance hemodialysis (HD) with COVID-19, more than half of them were combined with DKD and they are more likely to be admitted to intensive care units (ICUs) and die (8). DKD patients are increasingly being appreciated as an infectable group for SARS-CoV-2, which is more prone to progress to a severe disease.
Hyperglycemia is the initial cause of the development of DKD; it can activate aldose reductase, protein kinase C, and advanced glycation end product (AGE) pathway, thus inducing the cell signal transduction network, resulting in inflammation response and cell damage. However, it has been reported that SARS-CoV-2 can infect pancreatic β-cells, leading to an imbalance in insulin homeostasis and apoptosis (9). Further research found that the concentrations of glucose, insulin, and insulin resistance were significantly increased in severe COVID-19 patients than in healthy people, resulting in increased oxidative and nitrosative stress (10), which may make it more difficult to treat and have serious consequences. Khunti et al. summarized the study of new-onset diabetes in COVID-19 (11). Thus, the disorder of glucose metabolism may cause a vicious circle in patients with DKD complicated with COVID-19.
Although the exact complex pathophysiology mechanism between COVID-19 and DKD is still being explored, the relationship between them seems to be traceable. In this review, we focus on DKD complicated with COVID-19 patients. We retrospect the pathogenesis of COVID-19 and DKD and put the spot on their correlation. Furthermore, we are also concerned about elevated blood glucose after COVID-19 vaccination.
Clinical characteristics of DKD complicated with COVID-19 patients
In this review, we performed a literature search through the electronic database, including Medline/PubMed, EMBASE, and Web of Science before 20 July 2022, using the following keywords: (“diabetic kidney disease” OR “diabetic nephropathy” OR “diabetic glomerulosclerosis”) AND (“COVID-19” OR “2019-nCoV” OR “novel corona virus” OR “SARS-CoV-2” OR “coronavirus”).
We found 11 cases of DKD complicated with COVID-19 (12–19) (Table 1), who were hospitalized with COVID-19. In the literature, six of them were men and two cases reported a history of contact with COVID-19 patients, four patients required endotracheal intubation, and two patients died. Fever and cough were the most common clinical symptoms. After receiving timely treatment, most of the patients’ biochemical indexes gradually improved and were finally discharged from the hospital. Among the nine patients treated with HD, we found that two patients did not need HD before admission but underwent HD after admission, and one patient did not recover from HD.
In the literature, four patients had reported lymphocytic counts decreased and three cases reported cytokine storm (12, 13). Similar laboratory results show progressive reduction of lymphocytes in COVID-19 patients; among the various cytokine changes, interleukin-6 (IL-6) and IL-8 were the most significant (20). Elevated cytokines and chemokines, known as cytokine storms, further lead to dysregulation of the immune response, which are a feature of severe COVID-19 and are thought to be a major determinant of disease progression (2, 21). Research found that plasma concentrations of IL-2, IL-7, IL-10, and tumor necrosis factor- α (TNF-α) of COVID-19 patients admitted to the ICU were higher than those of non-ICU patients (2).
Furthermore, four cases of elevated D-dimer levels have been reported in the literature (12, 14, 16). D-dimer is a fibrin degradation product, and the increase in this level indicates that a hypercoagulable state in vivo exists that may be associated with subsequent thrombosis (22). Sixteen percent of COVID-19 patients hospitalized in the New York Health system reported thrombotic events, and the level of d-dimer at the time of visit was independently associated with thrombotic events (23). Li et al. indicated that higher levels of D-dimer on admission were associated with increased odds of death and significantly elevated in patients with diabetes (24); thus, the use of anticoagulants also needs to attract the attention of clinicians.
DKD and COVID-19
The role of ACE2
Renin–angiotensin–aldosterone system (RAAS) activation is a central mechanism in the pathogenesis of DKD. ACE2 is considered a new therapeutic strategy for DKD due to its renoprotective effect by converting angiotensin II (Ang II) to angiotensin 1–7 (Ang 1–7), which acts as a vasodilator, anti-inflammatory, anti-fibrotic, and anti-proliferation agent via its specific Mas receptor to counteract the side effects of Ang II (25, 26). This fact is well established by the evidence of akita mice whose blood pressure was reduced, elevated protein kinase C level was attenuated, and glomerular hypertrophy was relieved after injection with ACE2 (27). Mizuiri et al. revealed that the high ACE/ACE2 ratio in DKD patients may lead to a decrease in the glomerular filtration rate and renal injury (28). Moreover, Ang 1-7 via Mas protein stimulates the production of nitric oxide (NO) in platelets to exert an antithrombotic role, and this effect is inhibited in Mas knockout mice who exhibit shorter bleeding times (29).
Similarly to SARS, SARS-CoV-2 enters host cells precisely through ACE2 and, because of its stronger affinity to ACE2, leads to a widespread infection worldwide (1). SARS-CoV-2 enters kidney cells and activate immune response to stimulate the production of the cytokine factor. The involvement of the immune system and inflammation in the pathogenesis of DKD has been reported (30, 31). DKD-induced chronic inflammation in combination with the inflammatory response by SARS-CoV-2 results in cytokine storm, which in turn exacerbates renal injury. Sultan et al. demonstrated that cytokine storm caused by the high expression of ACE2 in COVID-19 patients may be one of the causes of renal dysfunction (32). Interestingly, Menon and colleagues compared DKD ACE2+ characterized genes with the published SARS-CoV-2 genes and found that both two genes significantly overlap and nearly 30% of genes are functionally aligned with key processes of viral infection and immune response (33). It means that some gene expression procedures of DKD can interact with the virus infection to some extent. In addition, Gilbert et al.’s biopsy of 49 DKD patients and 12 healthy living kidney specimens showed that the ACE2 mRNA expression in the former was twice as high as that in the latter (34), implying that they are more vulnerable to being infected with COVID-19 and impose a double burden on the kidneys.
Notably, not only does ACE2 contribute to SARS-CoV-2 entering cells but also internalization decreases its expression on the cell membrane. This results in the accumulation of Ang II, which increases aldosterone secretion, resulting in subsequent hypokalemia, which inhibits insulin secretion (35) and exerts vasoconstriction, oxidative stress, inflammation, fibrosis, and pulmonary edema by combination with the AT1 receptor (AT1R). It has been proved that Ang II combined with AT1R increases pulmonary capillary permeability, leading to increased pulmonary edema and lung damage (21). Exogenous injection of recombinant human ACE2 protein reduces the extent of acute lung injury in acid-treated ACE2 knockout mice (36), showing that ACE2 has a protective effect on lungs. Taken together, downregulation of the ACE2/Ang 1-7/Mas receptor axis and amplification of the ACE/Ang II/AT1R axis are harmful to DKD complicated with COVID-19 patients.
Endothelial injury and thrombosis
Albuminuria is one of the earliest detectable abnormalities indicators of DKD. Thirty years ago, Deckert et al. hypothesized that proteinuria was a reflection of extensive microvascular injury (37) and was supported in subsequent results (38). Chronic hyperglycemia, reactive oxygen species (ROC), and inflammatory cytokines reduce the endothelial glycocalyx, thereby directly stimulating the contact between blood circulation substances and endothelium cells, causing structural disorders and proteinuria (39, 40). Increased oxidative stress reduces the production of NO in endothelial cells, and reduced NO availability further leads to endothelial dysfunction (38). Persistent endothelial dysfunction in turn causes changes in vascular tone and permeability, activation of abnormal coagulation function, and abnormal expression of inflammatory cytokines (41).
DKD-induced endothelial dysfunction further aggravates the serious risk of SARS-CoV-2 infection. Indeed, endothelial dysfunction is also a common feature of COVID-19. Endothelial damage and endothelial cell membrane disruption had been found in COVID-19 autopsies (42). Kidney damage in COVID-19 patients is dominated by elevated creatinine and albuminuria. An excess half of patients of COVID-19 had proteinuria after admission (43, 44). Both direct stimulation of SARS-CoV-2 invasion and indirect stimulation of cytokine storm result in vascular endothelial dysfunction and thus may activate the coagulation cascade (45, 46). Fibrinogen, D-dimer, factor VIII, von Willebrand factor (vWF), and neutrophil extracellular traps (NETs) were greatly increased in COVID-19 model experiment (47) and patients (22); these abnormal coagulation parameters indicating COVID-19 patients are in a hypercoagulable state. On the one hand, SARS-CoV-2 infection activates the complement system to generate pro-inflammatory peptides C3a and C5a and recruit neutrophils to release NETs; it induces thrombosis by activating the blood coagulation contact pathway, which in turn leads to excessive production of thrombin and C5a (46, 48). There seems to be a feedback pathway between the complement system and NETs, which continuously promotes thrombosis (48). On the other hand, NETs also combined vWF secreted by endothelium cells to help adhere and aggregate platelets (46). Damaged endothelial cells increase the release of plasminogen activator inhibitor-1, which inhibits the fibrinolytic system and aggravates thrombosis (48). Moreover, the previously mentioned increase in the ACE/ACE2 ratio was also involved in thrombosis (Figure 1). The accumulation of all factors leads to endothelium and vascular damage, which ultimately leads to the occurrence of the acute respiratory syndrome (ARDS) and multiple-organ failure.
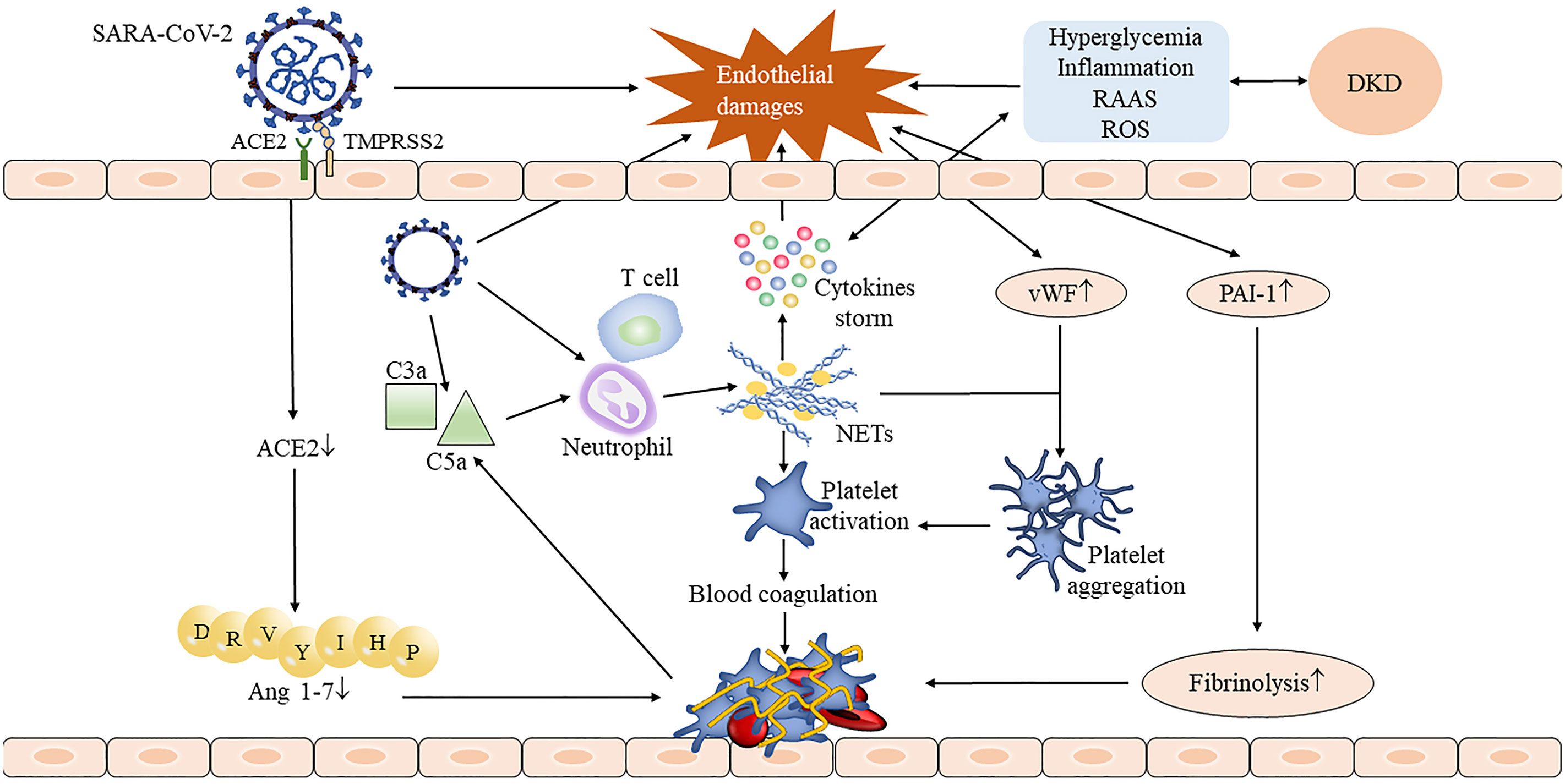
Figure 1 The process of thrombus formation in DKD complicated with COVID-19 patients. The invasion of SARS-CoV-2 damaged the integrity of the endothelium, and the injured endothelial cells stimulated the release of the von Willebrand factor (vWF) and plasminogen activator inhibitor-1 (PAI-1) and accelerated the formation of thrombosis. SARS-CoV-2 activates neutrophils by activating the complement system, thus causing the release of neutrophil extracellular traps (NETs) (48). NETs not only stimulate the occurrence of the coagulation cascade and leads to subsequent thrombosis but also stimulates cytokine storm to further aggravate endothelial damage. Downregulated angiotensin-converting enzyme 2 (ACE2) reduced angiotensin 1-7 production which also contributed to the thrombosis. The presence of DKD may aggravate endothelial damage through inflammatory stimulation, hyperglycemia, and oxidative stress, resulting in a more serious consequence.
The occurrence of COVID-19 with thrombotic events has been shown to have a higher rate of hospitalization and an incidence of ARDS (49) and is independently associated with the risk of death (23). Hertanto et al. reported a patient with a history of T2DM, DKD, and leg pain who was admitted with COVID-19. After the admission, dyspnea improved but the leg pain became progressively worse and computed tomography angiography showed thrombosis of popliteal arteries in both lower limbs (14). This patient has a markedly elevated D-dimer level on admission (14), suggesting that the patient may have been in a hypercoagulable state before admission. After infecting SARS-CoV-2, hyperinflammation, endothelium dysfunction, complement system activation, and hypercoagulable may aggravate limb ischemia, causing secondary harm to the patient. Altogether, endothelial damage and hypercoagulable state are key pathogenic mechanisms of COVID-19, and DKD may stimulate its progression and deterioration.
Dipeptidyl peptidase 4
Dipeptidyl peptidase 4 (DPP4), also known as CD26, is widely distributed in the kidney, usually present in membrane-bound and soluble forms, involved in the degradation of glucose-dependent insulinotropic polypeptide and glucagon-like peptide-1 (GLP-1), thus interfering with insulin secretion and disrupting glucose homeostasis (50). Tahara et al. noted that the level of DPP4 was correlated with AGEs; elevated AGEs increased the expression of DPP4 in renal tubular cells (51). It is well known that hyperglycemia can activate AGEs and induce subsequent oxidative stress and inflammation response. Various inflammatory cytokines associated with DKD contain truncation sites for DPP4 (52). A cross-sectional study in China shows increased DPP4 activities closely associated with T2DM-related DKD patients, whose oxidation stress, IL-6, and C-reactive protein (CRP) levels increased with the increase in the DPP4 quartile (53).
The DPP4 inhibitor, such as linagliptin and alogliptin, can dramatically mitigate AGE and AGE receptor axis activity, thus alleviating the renal damage caused by inflammation, proteinuria, and oxidative stress in type 1 diabetes (T1DM) and T2DM (54, 55). The elevated concentration of the circulation soluble form of the AGE receptor in diabetics is correlated with the inflammatory marker and proteinuria, which can represent a biomarker for vascular injury in T2DM (56, 57). On the other hand, the DPP4 inhibitor also inhibits the fibrotic pathway mediated by transforming growth factor β (58), which is induced by the interaction between DPP4 and cation-independent mannose-6-phosphate receptor (59). The DPP4 inhibitor has gradually become the first choice treatment for patients with T2DM-related renal failure (60).
A recent paper suggests that the S1 domain of the spike glycoprotein of SARS-CoV-2 may interact with human CD26 to cause disease (61); blocking this connection may reduce the toxic effect of SARS-CoV-2 on the body. It has been well verified in T2DM patients infected with COVID-19, who have mild pneumonia, less non-invasive mechanical ventilation, and better prognosis (62), indicating a range of benefits of the drug for this group. Specifically, DPP4 plays a key role in T-cell activation and immune regulation; it not only modulates CD4+T cell maturation, migration, and cytokine secretion but also interacts with several molecules involved in T-cell function, including mannose 6-phosphate/insulin-like growth factor II receptor, which in turn increased the expression of the AGE receptor through activating reactive oxygen species (63, 64). DPP4 expression is upregulated following T-cell activation (65). Compared with CD28, the mRNA of TNF-α, interferon-γ, and Fas ligand are increased after CD26 co-stimulatory, manifesting greater cytotoxic effects (66). Human T helper type 17 cells also showed a high expression of enzymatically active CD26 (67), while T helper type 17 cells can release IL-17 to recruit monocytes, macrophages, and neutrophils and stimulate cytokines, including IL-1β and IL-6. Solerte et al. reported that T2DM patients infected with COVID-19 using the DPP4 inhibitor had elevated lymphocyte counts, and their CRP and procalcitonin levels were decreased compared to those on conventional treatment (68), suggesting that the anti-inflammatory effect may restore the efficiency of the immune response after SARS-CoV-2 infection. The DPP4 inhibitor also improves bronchoalveolar lavage IL-6 and TNF-α levels in LPS-induced mice and attenuates lung injury (69), meaning direct stimulation of anti-inflammatory activity in the lungs may help ameliorate lung damage from COVID-19. Meanwhile, the decrease in GLP-1 degradation alleviates insulin resistance and its anti-inflammatory can reduce lung injury caused by excessive production of cytokines (50) (Figure 2). As can be seen, the therapeutic benefits of DPP4 inhibitors may shed new light for DKD complicated with COVID-19 patients, and more research is warranted to fully evaluate their effect.
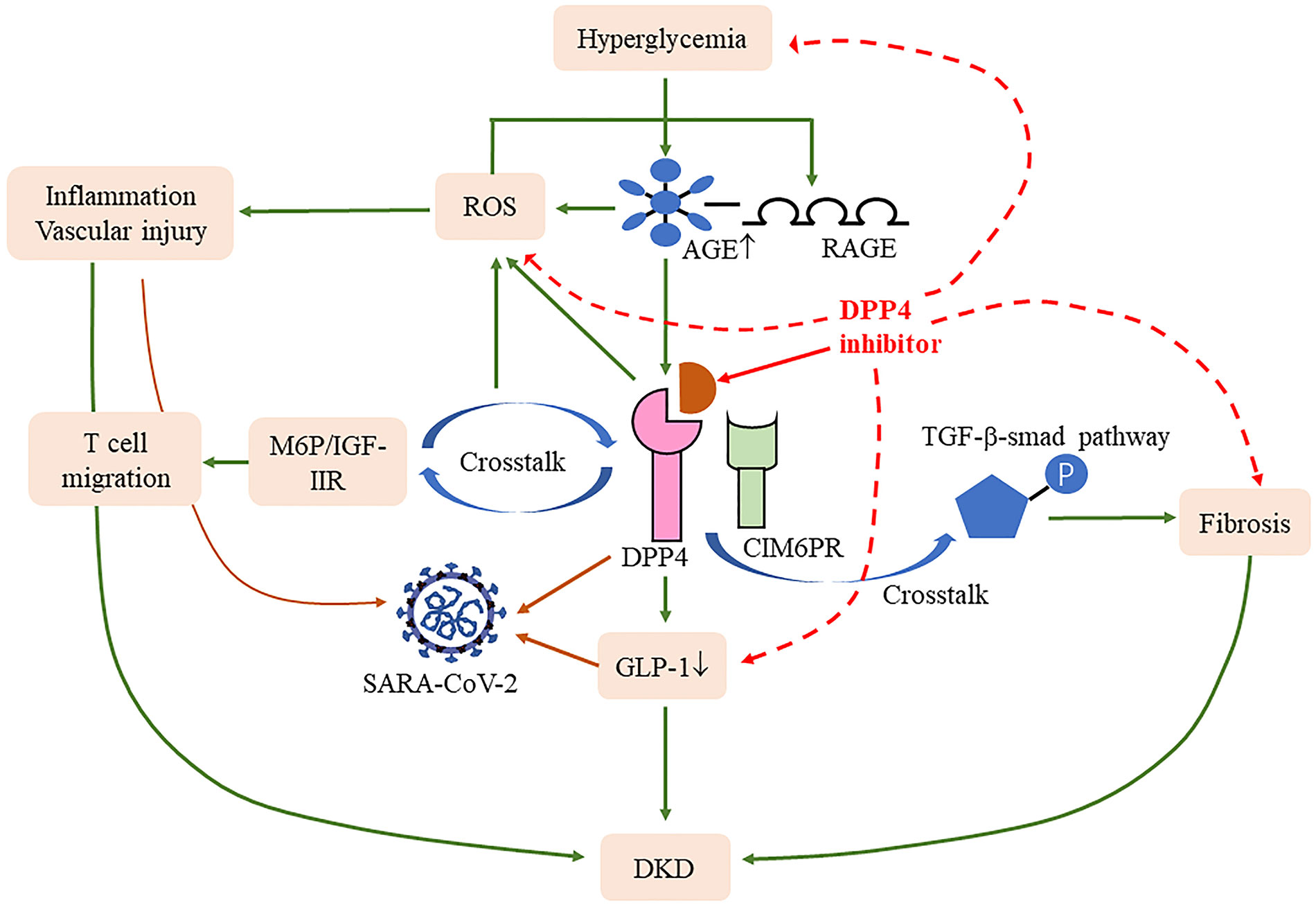
Figure 2 Effects of DPP4 and its inhibitors in COVID-19 and DKD. DPP4 cross talk with D-mannose-6-phosphate/insulin-like growth factor II receptor (M6P/IGF-IIR) (64) and cation-independent mannose-6-phosphate receptor (CIM6PR) (59) induced reactive oxygen species (ROS) and fibrosis, respectively. Meanwhile, DPP4 also induced the degradation of glucagon-like peptide-1 (GLP-1), all of which contribute to the progression of DKD and COVID-19. DPP4 inhibitions blocked these processes and thus improved the disease progression.
Neuropilin-1
It has been found that neuropilin-1 (NRP-1) mRNA protein is expressed in renal cells and highly located in differentiated podocytes (70, 71). Increased AGEs not only elevate the DPP4 release but also acts on the podocytes. AGE-modified bovine serum albumin downregulated the expression of NRP-1, which may be achieved by reducing the binding ability of the Sp1 transcription factor to the NRP1 promoter to the inhibit the transcriptional activity of the NRP1 promoter in podocytes (71), thus restraining podocyte migration, which leads to increases in “nude” areas of the glomerular basement membrane adhesion to the Bowman space and causes glomerulosclerosis (70). Furthermore, decreased NRP-1 partially inhibited the adhesion capacity of podocytes and impaired cellular reorganization skeleton, which may further influence podocyte injury in DKD and lead to proteinuria (72). Short-term erythropoietin treatment reversed NPR-1 expression, reduced proteinuria, and protected podocytes from AGE-mediated damage (73), which shows that a decreased expression of NPR-1 is one of the characteristics of DKD.
Besides ACE2 as a receptor of SARS-CoV-2 infection, NRP-1 also as an alternative pathway contributes to SARS-CoV-2 infection by binding to the S1 C-end rule motif cleaved by furin protein (74). The role of NPR-1 in COVID-19 has been well described (75). NRP-1 is considered an immune checkpoint for T-cell memory; its immunological role in COVID-19 is unquestionable (75). The Toll-like receptor (TLR) is a key modulator in innate immunity (76). Sultan et al. reported that both expressions of TLR2, TLR4 mRNA, and NRP-1 were elevated in moderate and severe COVID-19 patients, and the NRP-1 level was positively correlated with TLR2 and TLR4 (77). Upregulated NRP-1 is related to deterioration of renal function. This was verified by the significant correlation between NRP-1 expression and serum creatinine and urea levels in severe COVID-19 patients (32, 77). The consumption of NRP-1 after infection may lead to impaired podocyte function and aggravate DKD (78).
Furthermore, NRP-1 contributes to the SARS-CoV-2 infection of pancreatic β-cells and impairs cell function; NRP-1 inhibition can rescue this process (9). Severe COVID-19 may induce blood clotting dysfunction, which leads to the release of various factors from the endothelium. NPR-1 also plays a role in the coagulation process by controlling the adhesion and permeability of endothelial cells (75). Given the above characteristics, severing the combination of the SARS-CoV-2 S1 and NRP-1 could be a new therapeutic target for COVID-19 (79). However, there are few available data on NRP-1 in DKD complicated with COVID-19 patients. Whether the level will be changed in this population remains to be confirmed by further study.
COVID-19 vaccination
The rapid rollout of vaccines has significantly reduced morbidity and mortality associated with COVID-19 (80). However, associated adverse reactions have been reported; we summarize the cases of hyperglycemia and related complications following COVID-19 vaccination in people with or without diabetes (Table 2) (81–97). The majority of cases occur with nausea, polydipsia, and polyuria after 1–4 weeks of COVID-19 vaccination and diagnosed as diabetic ketoacidosis (DKA), hyperosmolar hyperglycemic syndrome, or new-onset diabetes. Of the reported glycated hemoglobin concentrations, those following vaccination were almost higher than baseline; although the exact mechanism is unclear, it may have a similar pathophysiology to the generation of immune and cytokines responses following COVID-19 infection.
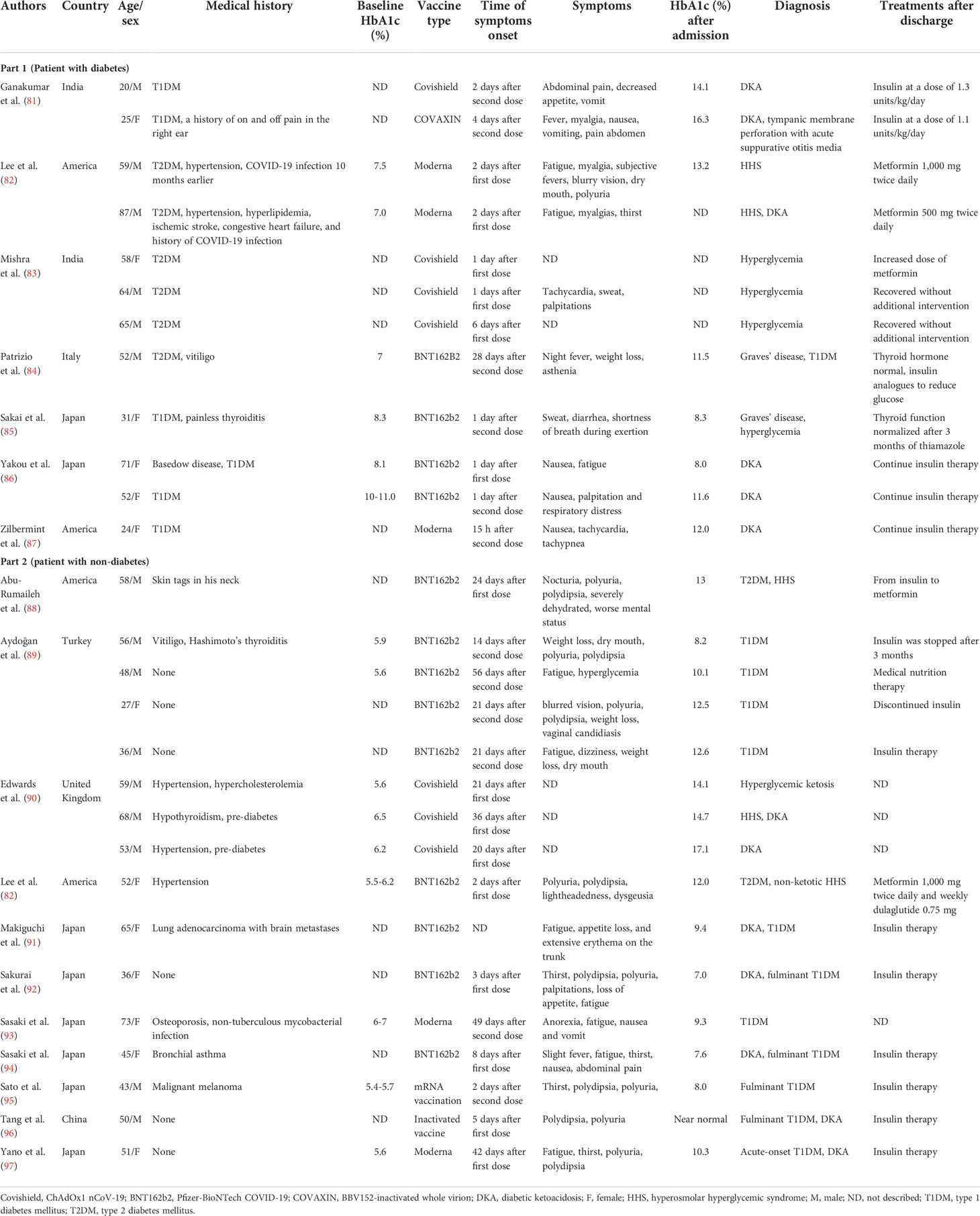
Table 2 Summary cases of hyperglycemia following COVID-19 vaccination in patients with or without diabetes.
SARS-CoV-2 invades pancreatic β-cells through ACE2 and NRP-1, thereby destroying insulin secretion, and Müller et al. demonstrated the presence of SARS-CoV-2 antigens in the pancreas (98). Subsequently, downregulated ACE2 elevates the ACE/ACE2 ratio, which in turn activates inflammatory pathways and hypercytokinemia can directly damage the insulin signal; it is reasonable to assume that similar reactions may occur after SARS-CoV-2 antigen presentation following vaccination (90, 99). The impairment of the insulin signal leads to the increase in gluconeogenesis and the decomposition of adipose tissue, which further increases the production of ketone bodies (100) and subsequent hyperglycemia complications. Many cases have reported symptoms of nausea and vomiting after vaccination (101), resulting in patients being unable to consume carbohydrates and history of diabetes-inducing insufficient insulin, which together leads to ketosis; in turn, ketosis aggravates nausea. This vicious cycle leads to the development of DKA in patients (86).
In the literature, of 16 patients with new-onset diabetes after vaccination, 11 of them were diagnosed with T1DM. Adjuvants are a key component of vaccine by secreting TNF-α, interferon-α, and other cytokines by immune cells to activate more robust and long-lasting specific immune responses, leading to autoimmune/inflammatory syndrome (84, 102, 103). There have been reports of autoimmune diseases caused by COVID-19 vaccination (104). mRNA seems to have self-adjuvant properties that induce immune response. A severe immune response leads to a rapid destruction of cells and severe loss of insulin secretion, which may be one of the causes of T1DM production. Melanoma differentiation‐associated protein 5 (MDA5), as a receptor for recognition of congenital pathogens, induces interferon synthesis, which impairs β-cell insulin production, proinsulin transformation, and mitochondrial function (105) and may recognize RNA of RNA-based COVID-19 vaccines, thus promoting the development of T1DM (92, 106) (Figure 3). Meanwhile, for patients with genetic susceptibility, vaccination may aggravate irreversible changes in autoimmune diseases (84, 85). T1DM-related alleles were reported in two patients (94, 97). Therefore, genetically susceptible people need to be more cautious after vaccination. However, it also needs more research to investigate the relationship between T1DM and COVID-19 vaccination. Fortunately, although they need drug treatment, the prognosis was relatively good in all of these cases. Therefore, timely monitoring of blood glucose after vaccination is necessary, regardless of prior diabetes.
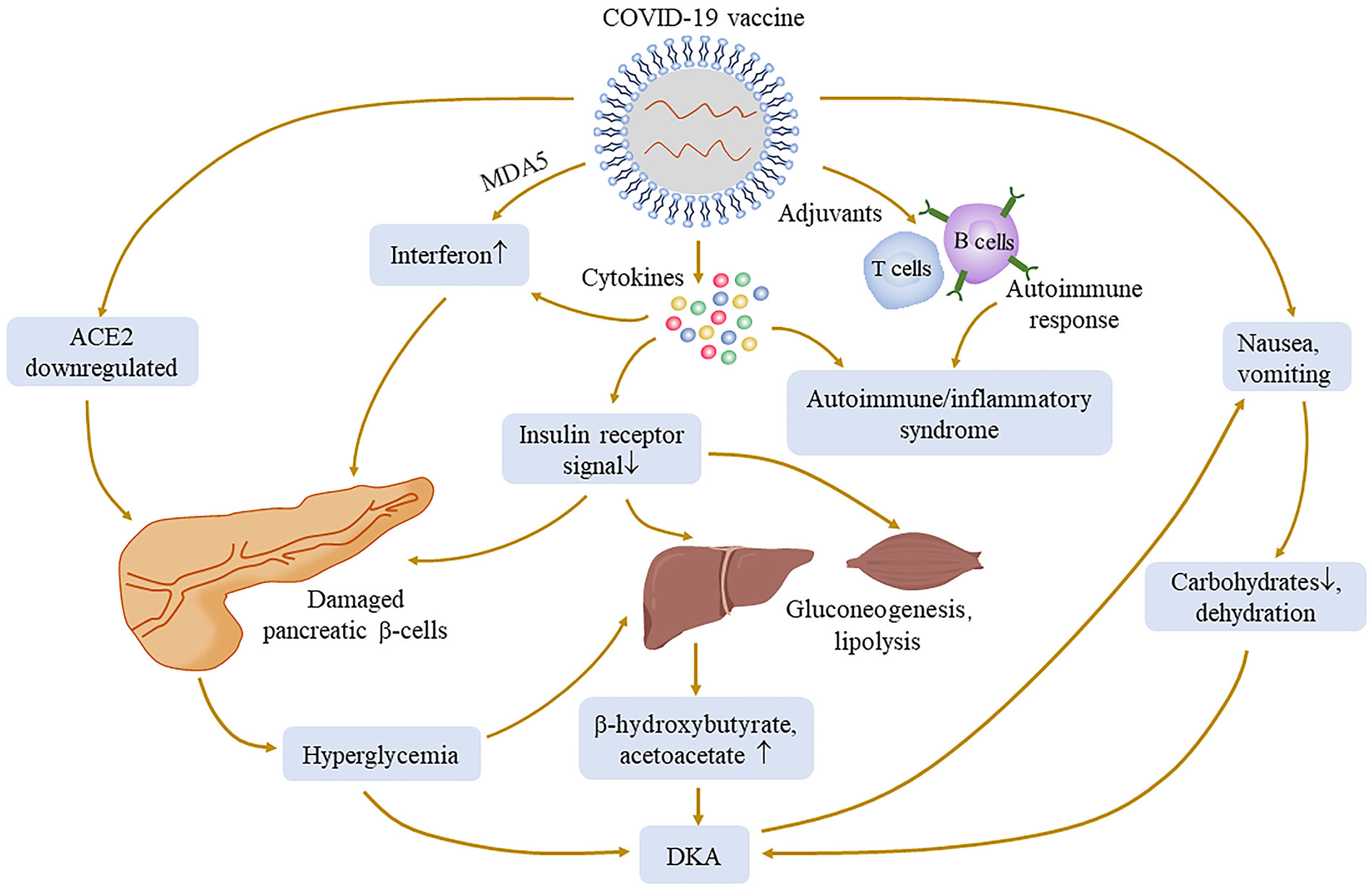
Figure 3 Possible mechanisms of hyperglycemia and its complication associated with COVID-19 vaccination. The injection of COVID-19 vaccines may cause a pathophysiological process similar to that of COVID-19 infection, such as ACE2 downregulation, cytokine production, and immune response. Adjuvants can activate more powerful and lasting specific immune responses to cause autoimmune diseases. The production of cytokines may damage the insulin receptor signal, lead to gluconeogenesis and lipolysis, and increase the substrate concentration of ketone bodies. Melanoma differentiation‐associated protein 5 (MDA5), as an innate pathogen recognition receptor, may interact with vaccines and damage pancreatic β-cells. All of the above processes may cause an abnormal increase of blood glucose.
Conclusion
The presence of DKD has been reported to make COVID-19 potentially more severe and fatal, ultimately resulting in ARDS and multiple-organ failure. The relationship between COVID-19 and DKD is complex and bidirectional. RAAS activation, chronic inflammation, and endothelial dysfunction are the core components linking COVID-19 and DKD. Chronic endothelial dysfunction promotes the procoagulant and anti-fibrinolytic state. SARS-COV-2 disrupts the glucose–insulin axis, which increases oxidative stress and exacerbates the progression of DKD. The anti-inflammatory effect of DPP4 inhibitors shows the protective effect on organs. The role of NRP-1 still needs to be further explored. The development of vaccines has greatly suppressed the spread of disease; although there are some adverse reactions, early detection may reduce the harm caused by adverse reactions. Of course, due to the lack of relevant experimental models, more research is needed to illustrate the relationship and prognosis between DKD and COVID-19.
Author contributions
YY: conducted the data collection and wrote the manuscript. SZ: conducted the data collection and wrote the manuscript. GX: was responsible for the idea and paper revision. All authors contributed to the article and approved the submitted version.
Funding
The Kidney Disease Engineering Technology Research Centre Foundation of Jiangxi Province (No. 20164BCD40095).
Conflict of interest
The authors declare that the research was conducted in the absence of any commercial or financial relationships that could be construed as a potential conflict of interest.
Publisher’s note
All claims expressed in this article are solely those of the authors and do not necessarily represent those of their affiliated organizations, or those of the publisher, the editors and the reviewers. Any product that may be evaluated in this article, or claim that may be made by its manufacturer, is not guaranteed or endorsed by the publisher.
Abbreviations
ACE2, angiotensin-converting enzyme 2; AGEs, advanced glycation end products; Ang II, angiotensin II; Ang 1-7, angiotensin 1-7; ARDS, acute respiratory syndrome; AT1R, AT1 receptor; COVID-19, coronavirus disease 2019; CRP, C-reactive protein; DKA, diabetic ketoacidosis; DKD, diabetic kidney disease; DPP4, dipeptidyl peptidase 4; GLP-1, glucagon-like peptide-1; HD, hemodialysis; ICU, intensive care unit; IL-6, interleukin-6; MDA5, melanoma differentiation‐associated protein 5; NETs, neutrophil extracellular traps; NO, nitric oxide; NRP-1, neuropilin-1; RAAS, renin–angiotensin–aldosterone system; SARS-CoV-2, severe acute respiratory syndrome coronavirus 2; T1DM, type 1 diabetes; T2DM, type 2 diabetes; TNF-α, tumor necrosis factor-α; vWF, von Willebrand factor.
References
1. Shang J, Wan Y, Luo C, Ye G, Geng Q, Auerbach A, et al. Cell entry mechanisms of sars-Cov-2. Proc Natl Acad Sci U.S.A. (2020) 117(21):11727–34. doi: 10.1073/pnas.2003138117
2. Huang C, Wang Y, Li X, Ren L, Zhao J, Hu Y, et al. Clinical features of patients infected with 2019 novel coronavirus in wuhan, China. Lancet (2020) 395(10223):497–506. doi: 10.1016/s0140-6736(20)30183-5
3. Ng WH, Tipih T, Makoah NA, Vermeulen JG, Goedhals D, Sempa JB, et al. Comorbidities in sars-Cov-2 patients: A systematic review and meta-analysis. mBio (2021) 12(1):e03647–20. doi: 10.1128/mBio.03647-20
4. Martos-Benitez FD, Soler-Morejon CD, Garcia-Del Barco D. Chronic comorbidities and clinical outcomes in patients with and without covid-19: A Large population-based study using national administrative healthcare open data of Mexico. Intern Emerg Med (2021) 16(6):1507–17. doi: 10.1007/s11739-020-02597-5
5. Tuttle KR, Bakris GL, Bilous RW, Chiang JL, de Boer IH, Goldstein-Fuchs J, et al. Diabetic kidney disease: A report from an ada consensus conference. Am J Kidney Dis (2014) 64(4):510–33. doi: 10.1053/j.ajkd.2014.08.001
6. Schiller M, Solger K, Leipold S, Kerl HU, Kick W. Diabetes-associated nephropathy and obesity influence covid-19 outcome in type 2 diabetes patients. J Community Hosp Intern Med Perspect (2021) 11(5):590–6. doi: 10.1080/20009666.2021.1957555
7. Leon-Abarca JA, Memon RS, Rehan B, Iftikhar M, Chatterjee A. The impact of covid-19 in diabetic kidney disease and chronic kidney disease: A population-based study. Acta BioMed (2020) 91(4):e2020161. doi: 10.23750/abm.v91i4.10380
8. Can O, Bilek G, Sahan S. Risk factors for infection and mortality among hemodialysis patients during covid-19 pandemic. Int Urol Nephrol (2022) 54(3):661–9. doi: 10.1007/s11255-021-02926-3
9. Wu CT, Lidsky PV, Xiao Y, Lee IT, Cheng R, Nakayama T, et al. Sars-Cov-2 infects human pancreatic beta cells and elicits beta cell impairment. Cell Metab (2021) 33(8):1565–76.e5. doi: 10.1016/j.cmet.2021.05.013
10. Soto ME, Guarner-Lans V, Diaz-Diaz E, Manzano-Pech L, Palacios-Chavarria A, Valdez-Vazquez RR, et al. Hyperglycemia and loss of redox homeostasis in covid-19 patients. Cells (2022) 11(6):932. doi: 10.3390/cells11060932
11. Khunti K, Del Prato S, Mathieu C, Kahn SE, Gabbay RA, Buse JB. Covid-19, hyperglycemia, and new-onset diabetes. Diabetes Care (2021) 44(12):2645–55. doi: 10.2337/dc21-1318
12. Abe T, Izumo T, Ueda A, Hayashi M, Ishibashi Y. Successful treatment of two Japanese esrd cases with severe covid-19 pneumonia. CEN Case Rep (2021) 10(1):42–5. doi: 10.1007/s13730-020-00512-7
13. Al Bishawi A, Abdel Hadi H, Elmekaty E, Al Samawi M, Nair A, Abou Kamar M, et al. Remdesivir for covid-19 pneumonia in patients with severe chronic kidney disease: A case series and review of the literature. Clin Case Rep (2022) 10(2):e05467. doi: 10.1002/ccr3.5467
14. Hertanto DM, Sutanto H, Adi S. Case report: Diabetic nephropathy aggravates the progression and prognosis of covid-19-Associated acute limb ischemia. F1000Res (2021) 10:584. doi: 10.12688/f1000research.54193.2
15. Hirai D, Yamashita D, Seta K. Favipiravir for covid-19 in a patient on hemodialysis. Am J Kidney Dis (2021) 77(1):153–4. doi: 10.1053/j.ajkd.2020.09.007
16. Koshi E, Saito S, Okazaki M, Toyama Y, Ishimoto T, Kosugi T, et al. Efficacy of favipiravir for an end stage renal disease patient on maintenance hemodialysis infected with novel coronavirus disease 2019. CEN Case Rep (2021) 10(1):126–31. doi: 10.1007/s13730-020-00534-1
17. Kuroki Y, Hiyama K, Minami J, Takeuchi M, Shojima M, Matsueda S, et al. The first case of covid-19 pneumonia in a hemodialysis patient in Japan. CEN Case Rep (2020) 9(4):404–8. doi: 10.1007/s13730-020-00495-5
18. Tang B, Li S, Xiong Y, Tian M, Yu J, Xu L, et al. Covid-19 pneumonia in a hemodialysis patient. Kidney Med (2020) 2(3):354–8. doi: 10.1016/j.xkme.2020.03.001
19. Yuan H, Guo E, Gao Z, Hu F, Lu L. Coronavirus disease 2019 in a hemodialysis patient. Blood Purif (2020) 49(6):761–4. doi: 10.1159/000507877
20. Zhang X, Tan Y, Ling Y, Lu G, Liu F, Yi Z, et al. Viral and host factors related to the clinical outcome of covid-19. Nature (2020) 583(7816):437–40. doi: 10.1038/s41586-020-2355-0
21. Chen R, Lan Z, Ye J, Pang L, Liu Y, Wu W, et al. Cytokine storm: The primary determinant for the pathophysiological evolution of covid-19 deterioration. Front Immunol (2021) 12:589095. doi: 10.3389/fimmu.2021.589095
22. Panigada M, Bottino N, Tagliabue P, Grasselli G, Novembrino C, Chantarangkul V, et al. Hypercoagulability of covid-19 patients in intensive care unit: A report of thromboelastography findings and other parameters of hemostasis. J Thromb Haemost (2020) 18(7):1738–42. doi: 10.1111/jth.14850
23. Bilaloglu S, Aphinyanaphongs Y, Jones S, Iturrate E, Hochman J, Berger JS. Thrombosis in hospitalized patients with covid-19 in a new York city health system. Jama (2020) 324(8):799–801. doi: 10.1001/jama.2020.13372
24. Li G, Deng Q, Feng J, Li F, Xiong N, He Q. Clinical characteristics of diabetic patients with covid-19. J Diabetes Res (2020) 2020:1652403. doi: 10.1155/2020/1652403
25. Nomura H, Kuruppu S, Rajapakse NW. Stimulation of angiotensin converting enzyme 2: A novel treatment strategy for diabetic nephropathy. Front Physiol (2021) 12:813012. doi: 10.3389/fphys.2021.813012
26. Malek V, Suryavanshi SV, Sharma N, Kulkarni YA, Mulay SR, Gaikwad AB. Potential of renin-Angiotensin-Aldosterone system modulations in diabetic kidney disease: Old players to new hope! Rev Physiol Biochem Pharmacol (2021) 179:31–71. doi: 10.1007/112_2020_50
27. Oudit GY, Liu GC, Zhong J, Basu R, Chow FL, Zhou J, et al. Human recombinant Ace2 reduces the progression of diabetic nephropathy. Diabetes (2010) 59(2):529–38. doi: 10.2337/db09-1218
28. Mizuiri S, Hemmi H, Arita M, Ohashi Y, Tanaka Y, Miyagi M, et al. Expression of ace and Ace2 in individuals with diabetic kidney disease and healthy controls. Am J Kidney Dis (2008) 51(4):613–23. doi: 10.1053/j.ajkd.2007.11.022
29. Fraga-Silva RA, Pinheiro SV, Goncalves AC, Alenina N, Bader M, Santos RA. The antithrombotic effect of angiotensin-(1-7) involves mas-mediated no release from platelets. Mol Med (2008) 14(1-2):28–35. doi: 10.2119/2007-00073.Fraga-Silva
30. Wada J, Makino H. Innate immunity in diabetes and diabetic nephropathy. Nat Rev Nephrol (2016) 12(1):13–26. doi: 10.1038/nrneph.2015.175
31. Pichler R, Afkarian M, Dieter BP, Tuttle KR. Immunity and inflammation in diabetic kidney disease: Translating mechanisms to biomarkers and treatment targets. Am J Physiol Renal Physiol (2017) 312(4):F716–F31. doi: 10.1152/ajprenal.00314.2016
32. Sultan RH, Abdallah M, Ali TM, Ahmed AE, Assal HH, Elesawy BH, et al. The associations between cytokine levels, kidney and heart function biomarkers, and expression levels of angiotensin-converting enzyme-2 and neuropilin-1 in covid-19 patients. Vaccines (Basel) (2022) 10(7):1045. doi: 10.3390/vaccines10071045
33. Menon R, Otto EA, Sealfon R, Nair V, Wong AK, Theesfeld CL, et al. Sars-Cov-2 receptor networks in diabetic and covid-19-Associated kidney disease. Kidney Int (2020) 98(6):1502–18. doi: 10.1016/j.kint.2020.09.015
34. Gilbert RE, Caldwell L, Misra PS, Chan K, Burns KD, Wrana JL, et al. Overexpression of the severe acute respiratory syndrome coronavirus-2 receptor, angiotensin-converting enzyme 2, in diabetic kidney disease: Implications for kidney injury in novel coronavirus disease 2019. Can J Diabetes (2021) 45(2):162–6.e1. doi: 10.1016/j.jcjd.2020.07.003
35. Pal R, Banerjee M. Covid-19 and the endocrine system: Exploring the unexplored. J Endocrinol Invest (2020) 43(7):1027–31. doi: 10.1007/s40618-020-01276-8
36. Imai Y, Kuba K, Rao S, Huan Y, Guo F, Guan B, et al. Angiotensin-converting enzyme 2 protects from severe acute lung failure. Nature (2005) 436(7047):112–6. doi: 10.1038/nature03712
37. Deckert T, Feldt-Rasmussen B, Borch-Johnsen K, Jensen T, Kofoed-Enevoldsen A. Albuminuria reflects widespread vascular damage. Steno Hypothesis Diabetol (1989) 32(4):219–26. doi: 10.1007/bf00285287
38. Goligorsky MS. Vascular endothelium in diabetes. Am J Physiol Renal Physiol (2017) 312(2):F266–F75. doi: 10.1152/ajprenal.00473.2016
39. Henry CB, Duling BR. Tnf-alpha increases entry of macromolecules into luminal endothelial cell glycocalyx. Am J Physiol Heart Circ Physiol (2000) 279(6):H2815–23. doi: 10.1152/ajpheart.2000.279.6.H2815
40. Satchell SC, Tooke JE. What is the mechanism of microalbuminuria in diabetes: A role for the glomerular endothelium? Diabetologia (2008) 51(5):714–25. doi: 10.1007/s00125-008-0961-8
41. van den Oever IA, Raterman HG, Nurmohamed MT, Simsek S. Endothelial dysfunction, inflammation, and apoptosis in diabetes mellitus. Mediators Inflammation (2010) 2010:792393. doi: 10.1155/2010/792393
42. Ackermann M, Verleden SE, Kuehnel M, Haverich A, Welte T, Laenger F, et al. Pulmonary vascular endothelialitis, thrombosis, and angiogenesis in covid-19. N Engl J Med (2020) 383(2):120–8. doi: 10.1056/NEJMoa2015432
43. Cheng Y, Luo R, Wang K, Zhang M, Wang Z, Dong L, et al. Kidney disease is associated with in-hospital death of patients with covid-19. Kidney Int (2020) 97(5):829–38. doi: 10.1016/j.kint.2020.03.005
44. Morell-Garcia D, Ramos-Chavarino D, Bauca JM, Argente Del Castillo P, Ballesteros-Vizoso MA, Garcia de Guadiana-Romualdo L, et al. Urine biomarkers for the prediction of mortality in covid-19 hospitalized patients. Sci Rep (2021) 11(1):11134. doi: 10.1038/s41598-021-90610-y
45. Amraei R, Rahimi N. Covid-19, renin-angiotensin system and endothelial dysfunction. Cells (2020) 9(7):1652. doi: 10.3390/cells9071652
46. Bonaventura A, Vecchie A, Dagna L, Martinod K, Dixon DL, Van Tassell BW, et al. Endothelial dysfunction and immunothrombosis as key pathogenic mechanisms in covid-19. Nat Rev Immunol (2021) 21(5):319–29. doi: 10.1038/s41577-021-00536-9
47. Sasidharakurup H, Kumar G, Nair B, Diwakar S. Mathematical modeling of severe acute respiratory syndrome coronavirus 2 infection network with cytokine storm, oxidative stress, thrombosis, insulin resistance, and nitric oxide pathways. OMICS (2021) 25(12):770–81. doi: 10.1089/omi.2021.0155
48. Java A, Apicelli AJ, Liszewski MK, Coler-Reilly A, Atkinson JP, Kim AH, et al. The complement system in covid-19: Friend and foe? JCI Insight (2020) 5(15):e140711. doi: 10.1172/jci.insight.140711
49. Mukherjee A, Pakhchanian H, Raiker R, Singh S, Chatterjee A. Burden of thrombotic events in coronavirus disease-19 (Covid-19) patients and effect on outcomes (from a multicenter electronic health record database). Am J Cardiol (2021) 147:150–2. doi: 10.1016/j.amjcard.2021.03.003
50. Kim W, Egan JM. The role of incretins in glucose homeostasis and diabetes treatment. Pharmacol Rev (2008) 60(4):470–512. doi: 10.1124/pr.108.000604
51. Tahara N, Yamagishi S, Takeuchi M, Tahara A, Kaifu K, Ueda S, et al. Serum levels of advanced glycation end products (Ages) are independently correlated with circulating levels of dipeptidyl peptidase-4 (Dpp-4) in humans. Clin Biochem (2013) 46(4-5):300–3. doi: 10.1016/j.clinbiochem.2012.11.023
52. Cho EH, Kim SW. Soluble dipeptidyl peptidase-4 levels are associated with decreased renal function in patients with type 2 diabetes mellitus. Diabetes Metab J (2019) 43(1):97–104. doi: 10.4093/dmj.2018.0030
53. Zheng T, Liu Y, Qin S, Liu H, Zhang X, Zhao H. Increased plasma dipeptidyl peptidase-4 activities are associated with high prevalence of diabetic nephropathy in Chinese patients with newly diagnosed type 2 diabetes: A cross-sectional study. Diabetes Vasc Dis Res (2016) 13(2):127–36. doi: 10.1177/1479164115615356
54. Sakata K, Hayakawa M, Yano Y, Tamaki N, Yokota N, Eto T, et al. Efficacy of alogliptin, a dipeptidyl peptidase-4 inhibitor, on glucose parameters, the activity of the advanced glycation end product (Age) - receptor for age (Rage) axis and albuminuria in Japanese type 2 diabetes. Diabetes Metab Res Rev (2013) 29(8):624–30. doi: 10.1002/dmrr.2437
55. Nakashima S, Matsui T, Takeuchi M, Yamagishi SI. Linagliptin blocks renal damage in type 1 diabetic rats by suppressing advanced glycation end products-receptor axis. Horm Metab Res (2014) 46(10):717–21. doi: 10.1055/s-0034-1371892
56. Yamagishi S, Matsui T, Nakamura K. Kinetics, role and therapeutic implications of endogenous soluble form of receptor for advanced glycation end products (Srage) in diabetes. Curr Drug Targets (2007) 8(10):1138–43. doi: 10.2174/138945007782151298
57. Humpert PM, Djuric Z, Kopf S, Rudofsky G, Morcos M, Nawroth PP, et al. Soluble rage but not endogenous secretory rage is associated with albuminuria in patients with type 2 diabetes. Cardiovasc Diabetol (2007) 6:9. doi: 10.1186/1475-2840-6-9
58. Gangadharan Komala M, Gross S, Zaky A, Pollock C, Panchapakesan U. Linagliptin limits high glucose induced conversion of latent to active tgfss through interaction with Cim6pr and limits renal tubulointerstitial fibronectin. PloS One (2015) 10(10):e0141143. doi: 10.1371/journal.pone.0141143
59. Gupta S, Sen U. More than just an enzyme: Dipeptidyl peptidase-4 (Dpp-4) and its association with diabetic kidney remodelling. Pharmacol Res (2019) 147:104391. doi: 10.1016/j.phrs.2019.104391
60. Danta CC. Dipeptidyl peptidase-4: A potential therapeutic target in diabetic kidney disease with sars-Cov-2 infection. ACS Pharmacol Transl Sci (2020) 3(5):1020–2. doi: 10.1021/acsptsci.0c00097
61. Vankadari N, Wilce JA. Emerging wuhan (Covid-19) coronavirus: Glycan shield and structure prediction of spike glycoprotein and its interaction with human Cd26. Emerg Microbes Infect (2020) 9(1):601–4. doi: 10.1080/22221751.2020.1739565
62. Mirani M, Favacchio G, Carrone F, Betella N, Biamonte E, Morenghi E, et al. Impact of comorbidities and glycemia at admission and dipeptidyl peptidase 4 inhibitors in patients with type 2 diabetes with covid-19: A case series from an academic hospital in Lombardy, Italy. Diabetes Care (2020) 43(12):3042–9. doi: 10.2337/dc20-1340
63. Matteucci E, Giampietro O. Dipeptidyl peptidase-4 (Cd26): Knowing the function before inhibiting the enzyme. Curr Med Chem (2009) 16(23):2943–51. doi: 10.2174/092986709788803114
64. Ishibashi Y, Matsui T, Maeda S, Higashimoto Y, Yamagishi S. Advanced glycation end products evoke endothelial cell damage by stimulating soluble dipeptidyl peptidase-4 production and its interaction with mannose 6-Phosphate/Insulin-Like growth factor ii receptor. Cardiovasc Diabetol (2013) 12:125. doi: 10.1186/1475-2840-12-125
65. Tanaka T, Camerini D, Seed B, Torimoto Y, Dang NH, Kameoka J, et al. Cloning and functional expression of the T cell activation antigen Cd26. J Immunol (1992) 149(2):481–6.
66. Hatano R, Ohnuma K, Yamamoto J, Dang NH, Morimoto C. Cd26-mediated Co-stimulation in human Cd8(+) T cells provokes effector function Via pro-inflammatory cytokine production. Immunology (2013) 138(2):165–72. doi: 10.1111/imm.12028
67. Bengsch B, Seigel B, Flecken T, Wolanski J, Blum HE, Thimme R. Human Th17 cells express high levels of enzymatically active dipeptidylpeptidase iv (Cd26). J Immunol (2012) 188(11):5438–47. doi: 10.4049/jimmunol.1103801
68. Solerte SB, D'Addio F, Trevisan R, Lovati E, Rossi A, Pastore I, et al. Sitagliptin treatment at the time of hospitalization was associated with reduced mortality in patients with type 2 diabetes and covid-19: A multicenter, case-control, retrospective, observational study. Diabetes Care (2020) 43(12):2999–3006. doi: 10.2337/dc20-1521
69. Kawasaki T, Chen W, Htwe YM, Tatsumi K, Dudek SM. Dpp4 inhibition by sitagliptin attenuates lps-induced lung injury in mice. Am J Physiol Lung Cell Mol Physiol (2018) 315(5):L834–L45. doi: 10.1152/ajplung.00031.2018
70. Bondeva T, Ruster C, Franke S, Hammerschmid E, Klagsbrun M, Cohen CD, et al. Advanced glycation end-products suppress neuropilin-1 expression in podocytes. Kidney Int (2009) 75(6):605–16. doi: 10.1038/ki.2008.603
71. Bondeva T, Wolf G. Advanced glycation end products suppress neuropilin-1 expression in podocytes by a reduction in Sp1-dependent transcriptional activity. Am J Nephrol (2009) 30(4):336–45. doi: 10.1159/000227762
72. Bondeva T, Wojciech S, Wolf G. Advanced glycation end products inhibit adhesion ability of differentiated podocytes in a neuropilin-1-Dependent manner. Am J Physiol Renal Physiol (2011) 301(4):F852–70. doi: 10.1152/ajprenal.00575.2010
73. Loeffler I, Ruster C, Franke S, Liebisch M, Wolf G. Erythropoietin ameliorates podocyte injury in advanced diabetic nephropathy in the Db/Db mouse. Am J Physiol Renal Physiol (2013) 305(6):F911–8. doi: 10.1152/ajprenal.00643.2012
74. Cantuti-Castelvetri L, Ojha R, Pedro LD, Djannatian M, Franz J, Kuivanen S, et al. Neuropilin-1 facilitates sars-Cov-2 cell entry and infectivity. Science (2020) 370(6518):856–60. doi: 10.1126/science.abd2985
75. Mayi BS, Leibowitz JA, Woods AT, Ammon KA, Liu AE, Raja A. The role of neuropilin-1 in covid-19. PloS Pathog (2021) 17(1):e1009153. doi: 10.1371/journal.ppat.1009153
76. Brodin P. Immune determinants of covid-19 disease presentation and severity. Nat Med (2021) 27(1):28–33. doi: 10.1038/s41591-020-01202-8
77. Sultan RH, Elesawy BH, Ali TM, Abdallah M, Assal HH, Ahmed AE, et al. Correlations between kidney and heart function bioindicators and the expressions of toll-like, Ace2, and nrp-1 receptors in covid-19. Vaccines (Basel) (2022) 10(7):1106. doi: 10.3390/vaccines10071106
78. Mourad D, Azar NS, Azar ST. Diabetic nephropathy and covid-19: The potential role of immune actors. Int J Mol Sci (2021) 22(15):7762. doi: 10.3390/ijms22157762
79. Charoute H, Elkarhat Z, Elkhattabi L, El Fahime E, Oukkache N, Rouba H, et al. Computational screening of potential drugs against covid-19 disease: The neuropilin-1 receptor as molecular target. Virusdisease (2022) 33(1):23–31. doi: 10.1007/s13337-021-00751-x
80. Baden LR, El Sahly HM, Essink B, Kotloff K, Frey S, Novak R, et al. Efficacy and safety of the mrna-1273 sars-Cov-2 vaccine. N Engl J Med (2021) 384(5):403–16. doi: 10.1056/NEJMoa2035389
81. Ganakumar V, Jethwani P, Roy A, Shukla R, Mittal M, Garg MK. Diabetic ketoacidosis (Dka) in type 1 diabetes mellitus (T1dm) temporally related to covid-19 vaccination. Diabetes Metab Syndr (2022) 16(1):102371. doi: 10.1016/j.dsx.2021.102371
82. Lee HJ, Sajan A, Tomer Y. Hyperglycemic emergencies associated with covid-19 vaccination: A case series and discussion. J Endocr Soc (2021) 5(11):bvab141. doi: 10.1210/jendso/bvab141
83. Mishra A, Ghosh A, Dutta K, Tyagi K, Misra A. Exacerbation of hyperglycemia in patients with type 2 diabetes after vaccination for Covid19: Report of three cases. Diabetes Metab Syndr (2021) 15(4):102151. doi: 10.1016/j.dsx.2021.05.024
84. Patrizio A, Ferrari SM, Antonelli A, Fallahi P. A case of graves' disease and type 1 diabetes mellitus following sars-Cov-2 vaccination. J Autoimmun (2021) 125:102738. doi: 10.1016/j.jaut.2021.102738
85. Sakai M, Takao K, Kato T, Ito K, Kubota S, Hirose T, et al. Graves' disease after administration of severe acute respiratory syndrome coronavirus 2 (Sars-Cov-2) vaccine in a type 1 diabetes patient. Intern Med (2022) 61(10):1561–5. doi: 10.2169/internalmedicine.9231-21
86. Yakou F, Saburi M, Hirose A, Akaoka H, Hirota Y, Kobayashi T, et al. A case series of ketoacidosis after coronavirus disease 2019 vaccination in patients with type 1 diabetes. Front Endocrinol (Lausanne) (2022) 13:840580. doi: 10.3389/fendo.2022.840580
87. Zilbermint M, Demidowich AP. Severe diabetic ketoacidosis after the second dose of mrna-1273 covid-19 vaccine. J Diabetes Sci Technol (2022) 16(1):248–9. doi: 10.1177/19322968211043552
88. Abu-Rumaileh MA, Gharaibeh AM, Gharaibeh NE. Covid-19 vaccine and hyperosmolar hyperglycemic state. Cureus (2021) 13(3):e14125. doi: 10.7759/cureus.14125
89. Aydogan BI, Unluturk U, Cesur M. Type 1 diabetes mellitus following sars-Cov-2 mrna vaccination. Endocrine (2022) 78(1):42–6. doi: 10.1007/s12020-022-03130-8
90. Edwards AE, Vathenen R, Henson SM, Finer S, Gunganah K. Acute hyperglycaemic crisis after vaccination against covid-19: A case series. Diabetes Med (2021) 38(11):e14631. doi: 10.1111/dme.14631
91. Makiguchi T, Fukushima T, Tanaka H, Taima K, Takayasu S, Tasaka S. Diabetic ketoacidosis shortly after covid-19 vaccination in a non-Small-Cell lung cancer patient receiving combination of pd-1 and ctla-4 inhibitors: A case report. Thorac Cancer (2022) 13(8):1220–3. doi: 10.1111/1759-7714.14352
92. Sakurai K, Narita D, Saito N, Ueno T, Sato R, Niitsuma S, et al. Type 1 diabetes mellitus following covid-19 rna-based vaccine. J Diabetes Investig (2022) 13(7):1290–2. doi: 10.1111/jdi.13781
93. Sasaki H, Itoh A, Watanabe Y, Nakajima Y, Saisho Y, Irie J, et al. Newly developed type 1 diabetes after coronavirus disease 2019 vaccination: A case report. J Diabetes Investig (2022) 13(6):1105–8. doi: 10.1111/jdi.13757
94. Sasaki K, Morioka T, Okada N, Natsuki Y, Kakutani Y, Ochi A, et al. New-onset fulminant type 1 diabetes after severe acute respiratory syndrome coronavirus 2 vaccination: A case report. J Diabetes Investig (2022) 13(7):1286–9. doi: 10.1111/jdi.13771
95. Sato T, Kodama S, Kaneko K, Imai J, Katagiri H. Type 1 diabetes mellitus associated with nivolumab after second sars-Cov-2 vaccination, Japan. Emerg Infect Dis (2022) 28(7):1518–20. doi: 10.3201/eid2807.220127
96. Tang X, He B, Liu Z, Zhou Z, Li X. Fulminant type 1 diabetes after covid-19 vaccination. Diabetes Metab (2022) 48(2):101324. doi: 10.1016/j.diabet.2022.101324
97. Yano M, Morioka T, Natsuki Y, Sasaki K, Kakutani Y, Ochi A, et al. A case of new-onset type 1 diabetes after covid-19 mrna vaccination. Intern Med (2022) 61(8):1197–200. doi: 10.2169/internalmedicine.9004-21
98. Muller JA, Gross R, Conzelmann C, Kruger J, Merle U, Steinhart J, et al. Sars-Cov-2 infects and replicates in cells of the human endocrine and exocrine pancreas. Nat Metab (2021) 3(2):149–65. doi: 10.1038/s42255-021-00347-1
99. Samuel SM, Varghese E, Triggle CR, Busselberg D. Covid-19 vaccines and hyperglycemia-is there a need for postvaccination surveillance? Vaccines (Basel) (2022) 10(3):454. doi: 10.3390/vaccines10030454
100. Dhatariya KK, Glaser NS, Codner E, Umpierrez GE. Diabetic ketoacidosis. Nat Rev Dis Primers (2020) 6(1):40. doi: 10.1038/s41572-020-0165-1
101. Chapin-Bardales J, Gee J, Myers T. Reactogenicity following receipt of mrna-based covid-19 vaccines. Jama (2021) 325(21):2201–2. doi: 10.1001/jama.2021.5374
102. Liang Z, Zhu H, Wang X, Jing B, Li Z, Xia X, et al. Adjuvants for coronavirus vaccines. Front Immunol (2020) 11:589833. doi: 10.3389/fimmu.2020.589833
103. Shoenfeld Y, Agmon-Levin N. 'Asia' - Autoimmune/Inflammatory syndrome induced by adjuvants. J Autoimmun (2011) 36(1):4–8. doi: 10.1016/j.jaut.2010.07.003
104. Ishay Y, Kenig A, Tsemach-Toren T, Amer R, Rubin L, Hershkovitz Y, et al. Autoimmune phenomena following sars-Cov-2 vaccination. Int Immunopharmacol (2021) 99:107970. doi: 10.1016/j.intimp.2021.107970
105. Blum SI, Tse HM. Innate viral sensor Mda5 and coxsackievirus interplay in type 1 diabetes development. Microorganisms (2020) 8(7):993. doi: 10.3390/microorganisms8070993
Keywords: COVID-19, SARS-CoV-2, diabetic kidney disease, ACE2, DPP4, NRP-1, vaccination
Citation: Yang Y, Zou S and Xu G (2022) An update on the interaction between COVID-19, vaccines, and diabetic kidney disease. Front. Immunol. 13:999534. doi: 10.3389/fimmu.2022.999534
Received: 22 July 2022; Accepted: 03 October 2022;
Published: 20 October 2022.
Edited by:
Kari Ann Shirey, University of Maryland, United StatesReviewed by:
Huibin Huang, The Second Affiliated Hospital of Fujian Medical University, ChinaWaleed Mahallawi, Taibah University, Saudi Arabia
Copyright © 2022 Yang, Zou and Xu. This is an open-access article distributed under the terms of the Creative Commons Attribution License (CC BY). The use, distribution or reproduction in other forums is permitted, provided the original author(s) and the copyright owner(s) are credited and that the original publication in this journal is cited, in accordance with accepted academic practice. No use, distribution or reproduction is permitted which does not comply with these terms.
*Correspondence: Gaosi Xu, Z2Fvc2l4dUAxNjMuY29t
†These authors have contributed equally to this work and share first authorship