- 1Laboratory of Retroviral Biochemistry, Department of Biochemistry and Molecular Biology, Faculty of Medicine, University of Debrecen, Debrecen, Hungary
- 2Doctoral School of Molecular Cell and Immune Biology, University of Debrecen, Debrecen, Hungary
- 3Laboratory of Inflammation-Physiology, Department of Physiology, Faculty of Medicine, University of Debrecen, Debrecen, Hungary
The severe acute respiratory syndrome coronavirus 2 (SARS-CoV-2) is the causative agent of coronavirus disease-19 (COVID-19). The spike protein (S) of SARS-CoV-2 plays a crucial role in mediating viral infectivity; hence, in an extensive effort to curb the pandemic, many urgently approved vaccines rely on the expression of the S protein, aiming to induce a humoral and cellular response to protect against the infection. Given the very limited information about the effects of intracellular expression of the S protein in host cells, we aimed to characterize the early cellular transcriptomic changes induced by expression of the S protein in THP-1-derived macrophage-like cells. Results showed that a wide variety of genes were differentially expressed, products of which are mainly involved in cell adhesion, homeostasis, and most notably, antiviral and immune responses, depicted by significant downregulation of protocadherins and type I alpha interferons (IFNAs). While initially, the levels of IFNAs were higher in the medium of S protein expressing cells, the downregulation observed on the transcriptomic level might have been reflected by no further increase of IFNA cytokines beyond the 5 h time-point, compared to the mock control. Our study highlights the intrinsic pathogenic role of the S protein and sheds some light on the potential drawbacks of its utilization in the context of vaccination strategies.
Introduction
As of the date of writing this manuscript, according to data from the World Health Organization (WHO), coronavirus disease of 2019 (COVID-19) caused by the severe acute respiratory syndrome coronavirus 2 (SARS-CoV-2) had claimed the lives of over 6.5 million individual worldwide, with more than 614 million infections reported to date. The spike protein (S) of SARS-CoV-2 is a class I, large trimeric fusion glycoprotein that mediates the initial vital contact with host target cells to establish the infection (1, 2). The protein is composed of two functional subunits (S1 and S2). While the S1 comprises the receptor binding domain (RBD) mediating interaction with the host target cellular surface receptor, the S2 mediates fusion between the viral and cellular membranes, similarly to other coronaviruses (1, 3). In the case of SARS-CoV-2 and the related SARS-CoV, the target cell receptor has been identified as the angiotensin-converting enzyme 2 (ACE2) (4), which is expressed in various tissues, primarily by the lung epithelial cells and in the intestine, and to a lesser extent in other tissues as well (5).
It is important to note that while the transmembrane protease serine 2 (TMPRSS2) has been found to facilitate cleavage at the S1/S2 and the S2’ site; hence enhancing fusion and infectivity (6), infection of cells with diminished or no expression of TMPRSS2 may still be accomplished utilizing endocytotic pathways or perhaps, other transmembrane proteases. Therefore, infection is not only limited to TMPRSS2 expressing cells (7, 8).
Given the crucial role of the S protein in mediating infectivity of SARS-CoV-2, vaccines aimed at inducing immunogenicity against the viral protein had been approved and are being widely deployed; most of which are based on the prototypical Wuhan-Hu-1 SARS-CoV-2 S protein sequence, in an effort to curb the pandemic (9). According to the WHO, almost 12.7 billion vaccine doses have been administered to date, with over 4.9 billion individuals fully vaccinated. While the clinical studies have shown a promising efficacy of many of the vaccines in inducing neutralizing antibodies and cellular immune responses against the Wuhan-Hu-1 and some other mutants, their efficacy appears limited in the face of new multi-mutant variants (10, 11), although, while neutralization studies against newly emerging variants are still ongoing, the final verdict is still underway.
Understanding the exact pathomechanism of the S protein and its interaction with host target receptors is of major importance. Despite the S protein of SARS-CoV-2 being the major target for vaccines, effects of the S protein on the cellular physiology are understudied.
Previously, it was reported that ACE2 in alveolar lung epithelium acts as a membrane receptor for cell signal transduction in response to SARS-CoV S protein, activating fibrosis-associated chemokine (C-C motif) ligand 2 expression through the Ras-ERK-AP-1 pathway (12). This finding was also verified recently in the context of SARS-CoV-2, wherein the S protein resulted in the activation of NF-κB and AP-1 transcription factors, promoting IL-6 trans-signaling by activation of angiotensin II receptor signaling in epithelial cells (13, 14). Moreover, this interaction between ACE2 and the S protein was found to trigger translational silencing of viral mRNA via DAP-kinase1 signaling pathway in an effort to perhaps relieve ER stress and unfolded protein response that is detrimental to viral replication (15). S protein of the Middle East respiratory syndrome coronavirus (MERS-CoV) was found to suppress macrophage responses, via the induction of interleukin-1 receptor associated kinase M (IRAK-M); a negative regulator of Toll-like receptors (TLR) signaling, in addition to induction of the transcriptional repressor peroxisome proliferator-activated receptor-γ (PPARγ) (16).
On the other hand, S protein was found to increase apoptosis, generation of reactive oxygen species, and intracellular calcium expression in macrophage-like cells, polarizing these cells towards a pro-inflammatory profile (17). Additionally, it was found to affect hematopoiesis and myeloid differentiation in vitro, and had a noticeable physiologic impact on peripheral blood cells ex vivo (18). A conserved 8-residues sequence (KWPWY/WVWL) in the S protein was found to be similar to a sequence typical in proteins involved in coagulation processes; such as von Willebrand factor, coagulation factor X, fibronectin and Notch (19). According to a study using scanning electron (SEM) and fluorescence microscopy as well as mass spectrometry (MS), S protein was found to directly interact with platelets and fibrin(ogen), which may perhaps contribute to the hypercoagulability state observed in COVID-19 (20).
Monocytes and monocyte-derived macrophages (MDMs) play a fundamental role in adaptive immunity against viral infection (21). Upon stimulation, they acquire inflammatory effector functions and aid in antigen-presentation and tissue repair (22). Given their expression of the ACE2 receptor, macrophages were found to be highly susceptible to SARS-CoV-2 infection (23), albeit recent studies have found that infection of monocytes and MDMs by SARS-CoV-2 is abortive, and the pathophysiology is mostly related to stimulation of the production of immunoregulatory cytokines (24). Nevertheless, there is emerging evidence from bulk as well as single cell transcriptomic profiling suggesting that alveolar macrophages from COVID-19 patients can be infected by SARS-CoV-2, resulting in progressive alveolar inflammation (25).
Following intramuscular injection, inflammatory cells dominated by neutrophils are derived to the site 2-6 hours after the injection, resulting in an extensive phagocytosis of vaccine particles (26). Given the inevitable involvement of tissue macrophages in the phagocytosis of vaccine particles, in this study, we were curious as to what transcriptomic changes may occur in monocyte-derived macrophages 5 hours post-transfection with the SARS-CoV-2 S protein, result of which may perhaps unravel its pathologic role in infection, and provide data on its effects as part of vaccination platforms. While most recent studies have carried out an in-depth analysis of cellular transcriptomic changes in target cells and MDMs in the context of SARS-CoV-2 infection (27, 28), our study focuses on the changes induced only by the S protein, which is more relevant given the majority of currently used vaccine formulations.
Materials and methods
Cell culturing
THP-1 human monocyte cell line was obtained from ATCC (TIB-202, USA) and cells were maintained in T-75 flask in 15 ml RPMI-1640 culture medium (Sigma-Aldrich, USA) containing 10% heat-inactivated fetal bovine serum (FBS, Gibco, UK), 1% L-glutamine (Sigma-Aldrich, USA) and 1% penicillin-streptomycin (Sigma-Aldrich, USA) in a humidified incubator with 5% CO2 at 37°C.
Plasmid constructs
A pUC57-amp vector encoding the prototypic Wuhan-Hu-1 SARS-CoV-2 S protein (codon optimized for human expression system, pUC57-2019-nCoV-S human) was obtained from GenScript Biotech (USA). The sequence was then cloned into a pcDNA3.1(+)-N-EGFP plasmid (GenScript Biotech, USA) using KpnI and ApaI restriction enzymes and T4 DNA ligase (NEB, UK). The resulting plasmid coded for the SARS-CoV-2 S protein fused to an N-terminal enhanced green fluorescent protein (EGFP) tag, the success of cloning was verified by agarose gel electrophoresis and PCR sequencing (Eurofins Genomics, Germany).
Activation of THP-1 cells
On the day of differentiation, THP-1 monocytes were plated onto 6-well plate (500.000 cells/well) in 2 ml RPMI-1640 culture medium supplemented with 10% FBS and 1% L-glutamine without antibiotics. After incubation for 3 hours, THP-1 monocytes were activated by 50 nM phorbol-12-myristate-13-acetate (PMA, Sigma-Aldrich, USA) for 1 hour, thereafter, PMA-containing medium was removed carefully, and the cells were incubated in fresh 2 ml antibiotic-free culture medium supplemented with 10% FBS, 1% L-glutamine for a further 24 hours.
Transfection of differentiated THP-1-derived macrophage-like cells
Macrophage-like cellswere transfected with 2.5 µg of pcDNA3.1(+)-N-EGFP (mock control) or pcDNA3.1(+)-N-EGFP-S plasmids for 5 hours using Lipofectamine reagent (LTX) following the manufacturer’s protocol (Thermo Fisher Scientific, USA). Transfection control cells were only treated with LTX and native, non-transfected but in vitro-macrophage-like cells were prepared by replacing the medium with fresh one.
Determination of SARS-CoV-2 Spike protein expression in transfected cells
To verify expression of the GFP-Spike fusion protein in transfected cells 5 hours after transfection, the cells were washed twice with PBS and re-suspended in lysis buffer (50 mM Tris-HCl, 250 mM NaCl, 5 mM EDTA, 50 mM NaF, 0.5% NP-40, pH=7.4). Thereafter, the cell lysate was incubated on ice for 30 minutes and vortexed every 10 minutes. After incubation, the samples were sonicated (Realsonic Cleaner) and then centrifuged (11,000 g, 4°C, 30 min). The supernatant was transferred into new Eppendorf tubes and Human SARS-CoV-2 RBD ELISA Kit (Invitrogen, USA) was used to determine the amount of SARS-CoV-2 Spike, according to the manufacturer’s instruction.
Analysis of key pro-inflammatory cytokines by ELISA
5 hours after transfection, supernatants were collected, centrifuged and stored at -20°C until use for cytokine measurements. Cytokine levels of supernatants (IL-1β, TNFα, and IL-6) were determined by using ELISA kits (BD Biosciences, San Diego, CA, USA) according to the manufacturer’s instructions. Quantifications were performed by a FlexStation 3 Microplate Reader (Molecular Devices, Sunnyvale, CA, USA). The detection limits were 0.8 pg/ml for IL-1β, 2 pg/ml for TNFα and 2.2 pg/ml for IL-6. Additionally, levels of interferon alphas (IFNAs) was also determined from the medium at 2h, 4h, 5h, and 24h time-points, with the aid of Human IFN-Alpha ELISA Kit (TCM) (PBL Assay Science, NJ., USA). This time-point analysis was carried out on medium collected from cells transfected in 48-well plate (35,000 cells/well), utilizing 300 ng of plasmids for transfection, otherwise, all the steps of cell activation and transfection were similar to the ones mentioned above.
RNA isolation, preparation of library, and transcriptome sequencing
5 hours after transfection, the media was removed carefully and cells were washed with 500 µl PBS and homogenized in 500 µl TRIzol Reagent (Molecular Research Center, USA), then the RNA isolation was carried out following the manufacturer’s protocol. Agilent RNA 6000 Nano kit on Bioanalyzer 2100 (Agilent Technologies, Germany) was used to determine the quality and integrity of RNA. Thereafter, high-throughput sequencing was performed on MGI DNBSEQ G400 sequencer (MGI Tech Co., Ltd., China) using MGI Easy RNA Library Prep Set by the Genomic Medicine and Bioinformatics Core Facility of University of Debrecen (Hungary).
Analysis of transcriptomic data
Sequence quality was determined with FastQC (29). Trimming of adapter sequences and low quality reads was performed with Trimmomatic (version 0.36) (30). Fastq files were then aligned with HISAT2 (version 2.2.1) aligned against the GRCh38 version of the human reference sequence (31). After alignment, the sequences of the samples were quantified with featureCounts (version 1.6.2) (32). Principal component analysis (PCA) was performed and visualized by selecting the top two principal components to demonstrate that the samples are transcriptomically distinct populations. Differential gene expression analysis was carried out with DESeq2 R package (version 1.34.0) applying ‘ashr’ effect size estimator (33). The heatmap for expression profiling of DEGs was generated using pheatmap R package. Gene set enrichment analysis (GSEA) was performed using g:Profiler (34) to identify the biological processes (Gene Ontology BP) associated with the identified genes (p<0.05). We have applied Cytoscape (version 3.9.0) (35) to visualize the DEGs enrichment in various processes.
Results
In order to carry out our analysis, we have utilized a pcDNA3.1(+) plasmid coding for the SARS-CoV-2 S protein (pcDNA3.1(+)-N-EGFP-S) or the pcDNA3.1(+)-N-EGFP plasmid lacking insert as mock for transfection of the macrophage-like cells. Five hours post-transfection of the cells, total RNA was isolated from cells. In addition, total RNA was also isolated from cells that were treated with Lipofectamine (LTX) reagent without plasmid, and from non-transfected macrophage-like cells, as well. Expression of the S protein was verified by ELISA, and the concentration was found to be 40-70 pg/ml in the lysate of cells transfected with the GFP-Spike fusion protein-coding construct.
Alteration of genome expression profile by SARS-CoV-2 S
Overall, 2394 significantly altered transcripts were detected in mock-transfected macrophage-like cells, compared to those that were treated only with the LTX reagent. Similarly, significant alterations were detected between S protein and mock-transfected cells, compared to the LTX-treated ones. A full list of differentially expressed genes (DEGs) can be found in Supplementary Table S1 and Supplementary Figure S1.
Statistical analysis was then carried out to identify DEGs in the S protein-expressing cells compared to the mock controls, as depicted by the principal component analysis (PCA) (Figure 1).
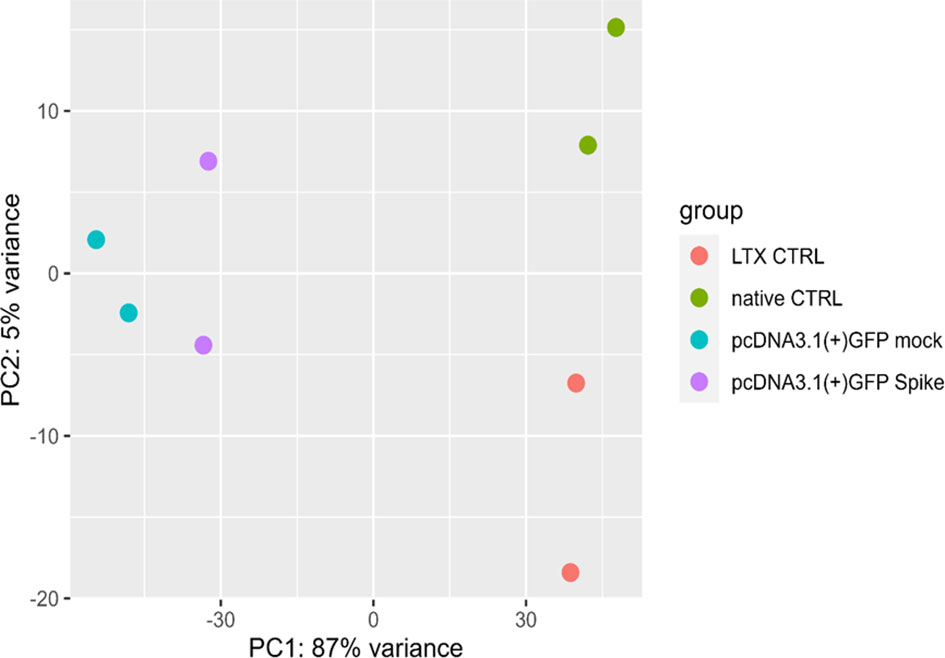
Figure 1 Principal component analysis (PCA). PCA of gene expression profiles in native (untreated) macrophage-like cells control (green, native CTRL), cells treated with only LTX reagent (orange, LTX CTRL), cells transfected with a pcDNA3.1(+)-N-EGFP plasmid (blue, mock) and cells transfected with S protein expressing plasmid (purple).
We have identified 165 DEGs (adjusted p-value < 0.1, shrunken log2(fold change) > 0.58), 142 of which were protein-coding, the most significantly differentially up- and downregulated genes in the S protein-expressing macrophage-like cells 5 hours post-transfection are depicted by the volcano plot in Figure 2.
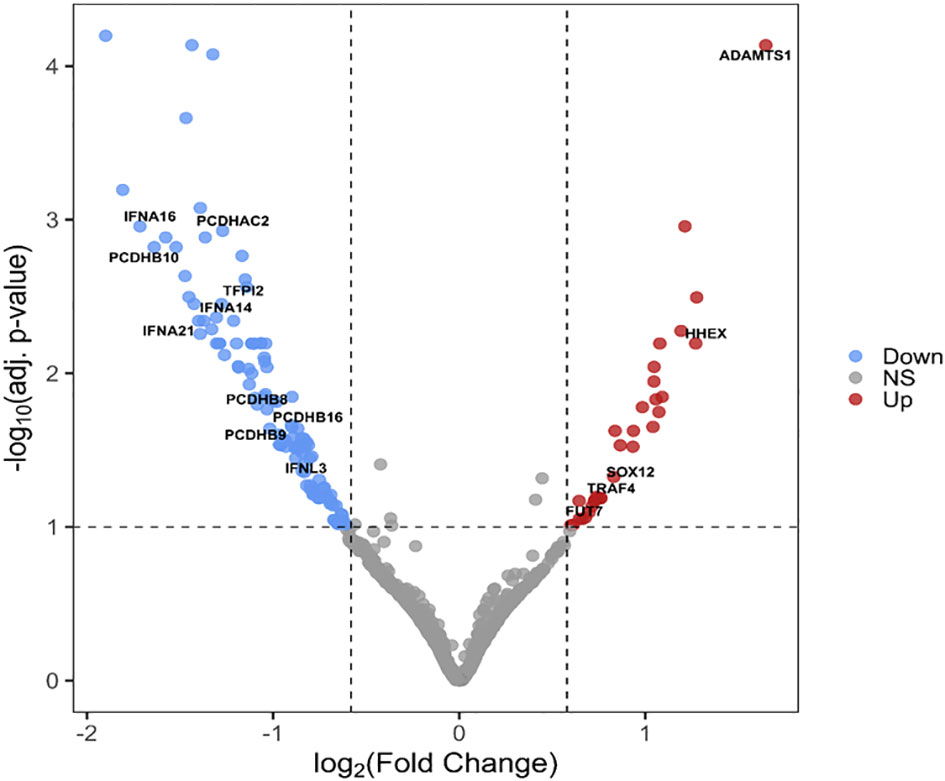
Figure 2 Volcano plot of DEGs between S protein expressing and mock-transfected macrophage-like cells. The -log10 adjusted p-values of the transcripts are plotted as a function of log2(fold change). Red and blue dots indicate the significantly up- and downregulated genes, respectively. Gray dots represent non-significant (NS) transcripts (p> 0.01 or LFC < 0.58 (= FC<1.5)).
When classified according to gene ontology (GO) - biological processes (BP), the products of the genes were found to play a role in 12 major processes: multi-organism process, response to stimulus, immune system process, locomotion, cell proliferation, localization, cellular process, multicellular organismal process, biological regulation, biological adhesion, developmental process and metabolic process (Figure 3). A comprehensive list of the detected GO terms can be found in Supplementary Table S1.
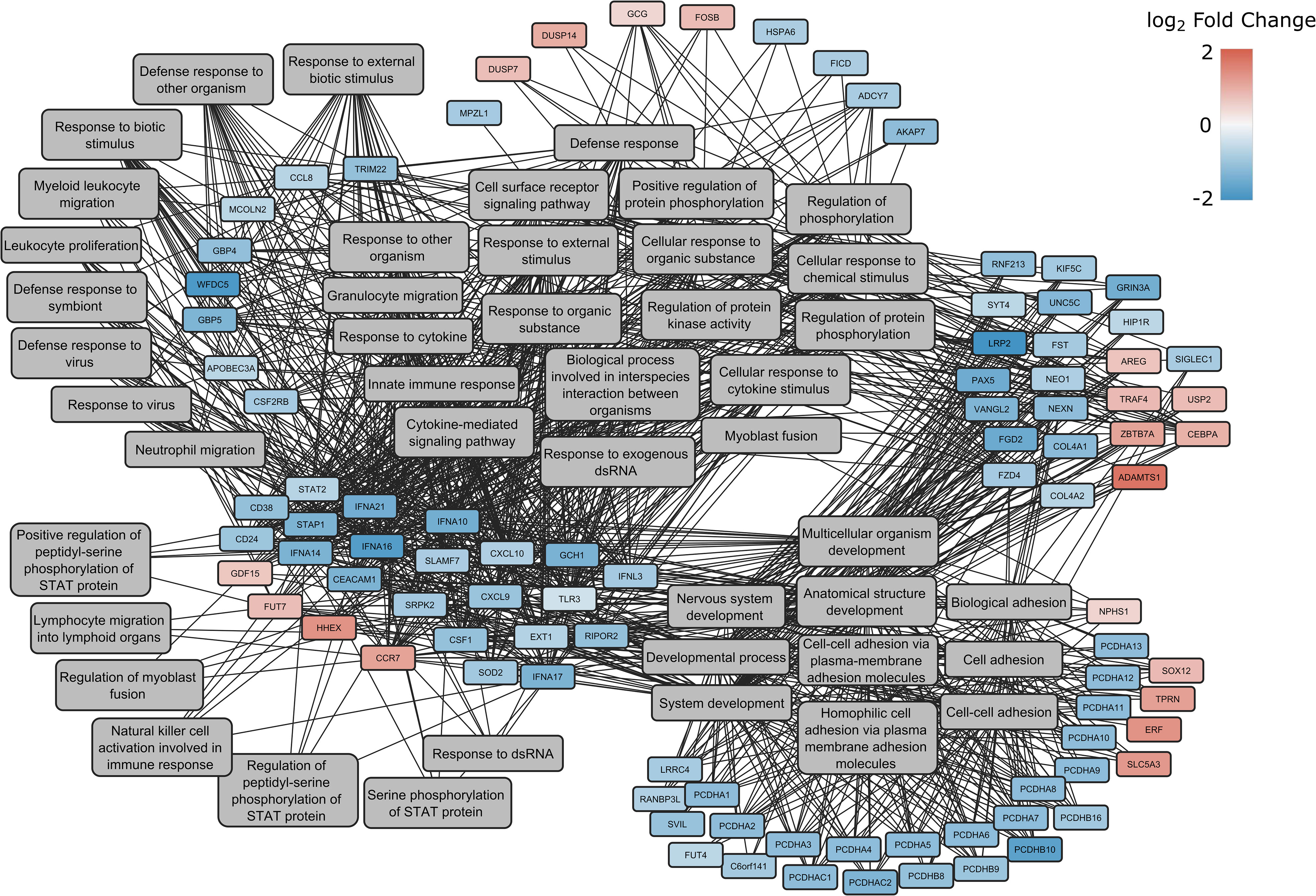
Figure 3 Enrichment network of DEGs between spike-transfected and mock-transfected samples in GO: Biological Processes. The log2(fold change) of the genes are indicated with red and blue colors, while involvement in a process is highlighted with gray lines connecting to the genes with the GO terms.
Next, GO analysis was performed on DEGs induced by the S protein in comparison to the mock control (Figure 4).
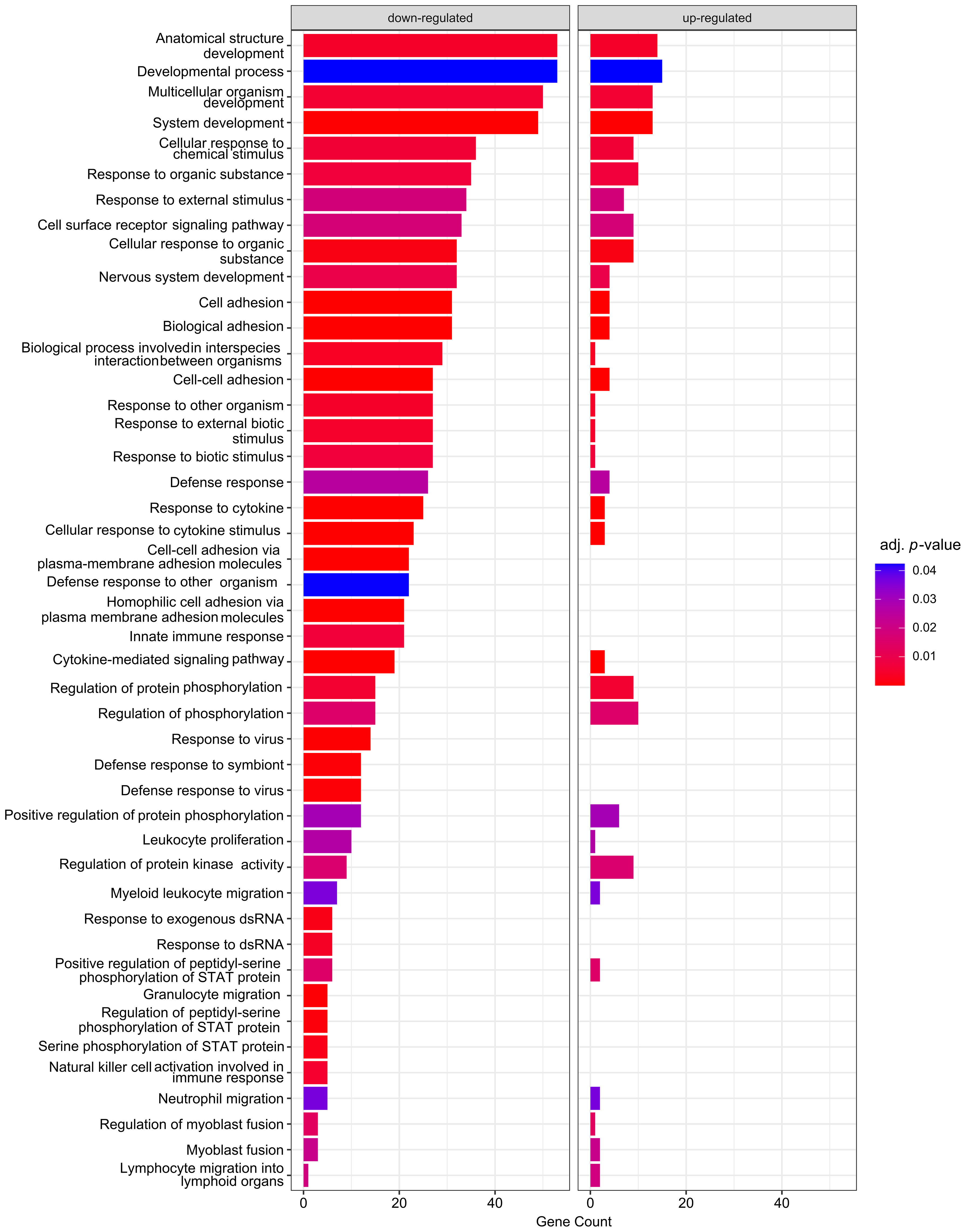
Figure 4 Enrichment analysis of DEGs according to GO: BPs. Analysis was carried out by comparison of S protein–expressing macrophage-like cells to the mock control. Size of the bars represent the genes enriched in a given term, and the colour corresponds of the adjusted p-value of the enrichment.
Initial dampening of innate immune responses, among others by the S protein
In total, 21 differentially downregulated genes were found to belong to the innate immune response (GO:0045087) group including interferon alpha isoforms (IFNAs, IFN-alpha 10, 14, 16, 17 and 21), interferon lambda 3 (IFNL3), signal transducer and activator of transcription 2 (STAT2), Toll-like receptor 3 (TLR3), tripartite motif-containing 22 (TRIM22), C-C motif chemokine 8 (CCL8), C-X-C motif chemokine 10 (CXCL10), apolipoprotein B MRNA editing enzyme catalytic subunit 3A (APOBEC3A), carcinoembryonic antigen-related cell adhesion molecule 1 (CEACAM1), SLAM family member 7 (SLAMF7), WAP four-disulfide core domain protein 5 (WFDC5), guanylate-binding protein 4 and 5 (GBP4 and GBP5), colony stimulation factor 1 (CSF1), GTP cyclohydrolase 1 (GCH1), mucolipin TRP cation channel 2 (MCOLN2), and SRSF protein kinase 2 (SRPK2) (Figure 5A).
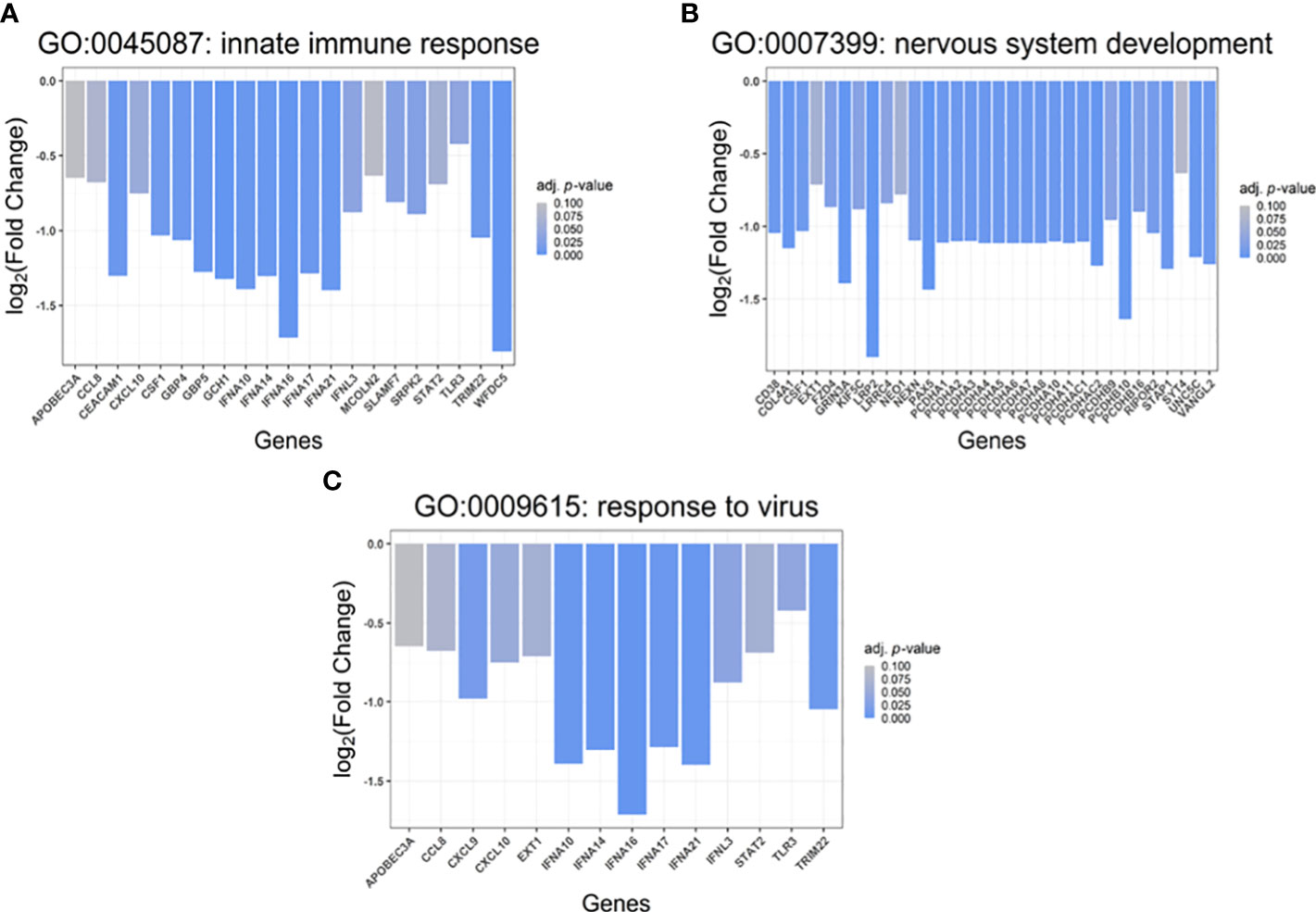
Figure 5 Gene set enrichment analysis (GSEA). Biological processes (Gene Ontology, GO: BP) associated with the identified, differentially up- and downregulated genes (p<0.05) in cells transfected with S protein-coding construct compared to mock control. (A) Genes involved in Innate immune response (GO:0045087), (B) Genes involved in “nervous system development” (GO:0007399), (C) Genes involved in “response to virus” (GO:0009615). The X-axis shows the names of the involved genes while the Y-axis denotes the log2(fold change) of the transcripts.
Moreover, 32 down-regulated genes were detected belonging to the nervous system development (GO:0007399) group (Figure 5B), including protocadherin alphas 1-11 (PCDHA 1-11), PCDHA subfamily C1-2 (PCDHAC1-C2), protocadherin betas 9, 10 and 16 (PCDHB9/10/16), LDL receptor-related protein 2 (LRP2), CD38 molecule, neogenin (NEO1), leucine-rich repeat-containing 4 (LRRC4), frizzled family receptor 4 (FZD4), paired box 5 (PAX5), synaptotagmin 4 (SYT4), neogenin 1 (NEO1), glutamate ionotropic receptor NMDA type subunit 3A (GRIN3A) as well as nexilin (F-actin-binding protein, NEXN). On the other hand, amphiregulin (AREG), solute carrier family 5 member 3 (SLC5A3), SRY-box transcription factor 12 (SOX12), pseudogene similar to part of protein tyrosine phosphatase receptor type N (PTPRN) were found to be up-regulated in the S protein-expressing cells.
5 hours after transfection with the pcDNA3.1(+)-N-EGFP-S plasmid, we observed downregulation of 14 genes whose products play a crucial role in the antiviral response (GO:0009615); such as IFNA 10, 14, 16, 17 and 21, IFNL3, TLR3, TRIM22, CCL8, C-X-C motif chemokine 9 (CXCL9), CXCL10, glycosyltransferase exostosin 1 (EXT1), APOBEC3A, and STAT2 (Figure 5C).
Early changes in the cytokine profile induced by the S protein
Five hours post-transfection, the cell culture media were collected to analyze changes in the levels of key cytokines (IL-1β, IL-6, TNFα). Transfection with the S protein resulted in a significant elevation of all measured cytokine levels, most notably, level of TNFα was increased more than 2.5 fold compared to the mock control-transfected cells (Figure 6A). Moreover, we carried out analysis of IFNAs level in the media of transfected cells at 2 h, 4 h, 5 h, and 24 h time-points. At 2 hours, no IFNAs were detected in the cell culture media of either sample, and increase in IFNA level thereafter, was only detected in the mock control; and the cells transfected with the GFP-Spike fusion protein-coding construct. At 4 h time-point, levels of IFNAs were significantly higher than that of the mock control, thereafter, there was no significant difference in IFNAs level between the two (Figure 6B).
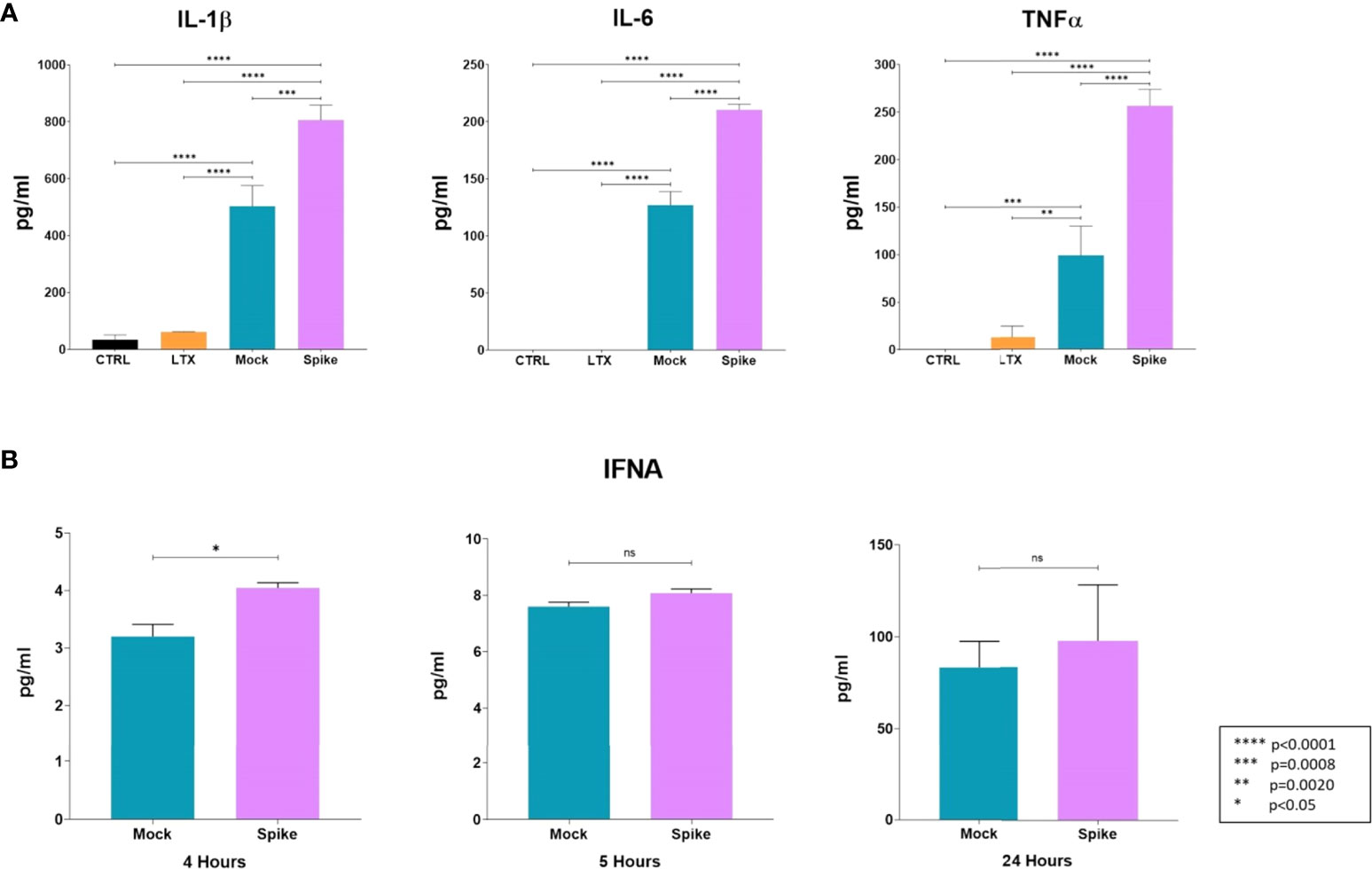
Figure 6 Early changes in the cytokine profile induced by the S protein. (A) Protein levels of IL-1β, IL-6, and TNF-α in medium of transfected cells. Results are concluded from triplicate measurements. (B) Time-point analysis of levels of IFNAs from media of transfected cells. Results are concluded form duplicate measurements. Error bars indicate SEM. ns, non-significant.
Discussion
In this study, we investigated the transcriptomic changes in THP-1-derived macrophage-like cells induced by the expression of SARS-CoV-2 S protein, the gene expression profile was investigated 5 hours post-transfection. THP-1 is an acute monocytic leukemia cell line, that has long been considered suitable substitutes for human monocytes, with similar viability, cytokine profile and host response (36–38). Activation with PMA induces their differentiation into macrophage-like phenotype, mimicking primary human macrophages (39). Being an immortalized malignant cell line, their response to stimuli may not completely overlap with that of their primary counterparts (37), and some have found significant differences at the level of chromatin structure and topologically associated domains between the two (40). However, the homogeneity of the genetic background of the THP-1 cells (41) minimizes variability. This was advantageous for the purpose of this study, since the presence of variability precipitated by age, gender, and previous infections in peripheral blood mononuclear cell (PBMC)-derived macrophages might have hindered our analysis of the intrinsic pathogenic role of the S protein, although, one might argue that such variability is essential to understanding the pathogenicity in the context of infection with SARS-CoV-2 in different populations.
Our results indicate that expression of the S protein in cells induced significant changes in the transcription profiles of genes that are involved in cell adhesion, homeostasis and most notably, antiviral and immune response.
When we classified the detected transcripts according to GO-term biological processes, transcripts of genes that were involved in cell and biological adhesion were most significantly altered. Members of the clustered protocadherins (PCDH) subfamily of the cadherin superfamily are known to play a major role in cell adhesion, through interaction with β1-integrins (42). PCDHA was shown to be mainly expressed in neuronal cells; such as the olfactory bulb, cerebellum, hippocampus and cerebral cortex (43). In mice model, clustered PCDHA was found to be important for sensory integration and short-term memory (44), others also found that their downregulation or knock-out may predispose to learning defects and aberrant distribution of serotonergic projections in the brain (44, 45). Interestingly, disruption of PCDH diverse expression was found to play a major role in olfactory neural circuit assembly in mice (46). In our experiment, cells transfected with S protein-coding construct showed a marked downregulation of PCDHA 1-11, PCDHA superfamily C-isoforms C1-C2 (PCDHAC1, PCDHAC2) as well as PCDHB 9, 10, 16. Upon treatment of human pulmonary microvascular endothelial cells with thrombin, PCDHA members were among the top up-regulated genes (47), hinting to an uncertain significance in the coagulation cascade and haematological diseases (48).
Of major interest was the downregulation of IFNAs (10, 14, 16, 17, and 21), IFNL3, TRIM22, tripartite motif containing 66 (TRIM66), TLR3, CD38 and CXCL9 induced by S protein expression.
Type I interferons; of which IFNAs are major constituents, play a pivotal role in the early host defense against viral infection. Through binding to IFN receptors, they initiate the JAK/STAT signaling pathway and transcription factors of STAT family, resulting in the transcription of interferon-stimulated genes (ISGs) which act directly against the invading virus (49). It is worth noting that while some ISGs possess antiviral properties, others were found to promote viral replication (50). Of the ISGs that were reported to have potent antiviral response, DDX60, GBP1, IFI44L were also downregulated. These were found to suppress the replication of hepatitis C virus (HCV), human papilloma virus (HPV), encephalomyocarditis virus (EMCV) and the vesicular stomatitis virus (VSV) (50, 51).
While some IFNA genes are rapidly induced by viral infection without the need for ongoing protein synthesis, others show delayed induction in response to ongoing viral protein synthesis (52). IFNA 10, 14, 16 and 17 were found to have high anti-proliferative and antiviral effect in cell culture (53). IFNAs also play an important role in the inhibition of viral reactivation. Previous studies have demonstrated that treatment of Epstein-Barr (EBV)-infected cells with type I interferon inhibits reactivation of the virus (54), similar effects were also observed in the case of cytomegalovirus (CMV) and the herpes simplex virus (HSV) (55).
It has been established that SARS-CoV-2 is a weak inducer of type I interferons in cell culture and animal models (56, 57), moreover, viral proteins such as the accessory protein 9b (ORF9b), non-structural proteins (nsp) 1, 13, and 15 were found to evade and antagonize the induction of type I interferons (58–60). Additionally, pretreatment of kidney epithelial Vero E6 and lung cancer Calu3 cell line with IFN-I resulted in substantially attenuated replication of SARS-CoV-2 (61).
Of the transcripts that were significantly upregulated were the pleckstrin homology domain-containing family A member 6 (PLEKHA6), a disintegrin and metalloproteinase with thrombospondin motif (ADAMTS1) and transcription factors; such as hematopoietically-expressed Homeobox protein (HHEX), FosB proto-oncogene (AP-1 transcription factor subunit), FUT7, SOX12 and CCAAT-enhance-binding protein alpha (CEBPA).
ADAMTS1 was shown to remodel the extracellular matrix and has been implicated in angiogenesis, embryogenesis, wound repair, and cancer development (62, 63).
Interestingly, we found that transfection with the S protein encoding plasmid resulted in the up-regulation of FUT7, product of which catalyzes the addition of a fucose in the sialyl Lewis X (sLeX)-characteristic alpha-(1,3) linkage. Viruses, such as the human T-cell leukemia virus type 1 (HTLV-1) were found to induce sLeX synthesis in a similar manner, through up-regulation of FUT7, which contribute immensely to the pathogenesis of the virus (64).
To gain insight into the changes of key cytokines on the protein level induced by transfection with the S protein-coding construct, we carried out analysis of the medium of transfected cells, and determined the concentration of IL-1β, IL-6, TNF-α, and IFNAs. Rather unsurprisingly, S protein resulted in the upregulation of IL-1β, IL-6, and TNFα protein levels, compared to the mock control. It has been previously documented that the S protein induces IL-1β in a dose-dependent manner (65), and elevated levels of IL-6 and TNF-α were also found to accompany induction with the S protein in a mouse model (66). Given the downregulation of IFNA levels by the S protein on the transcriptomic level, we wanted to explore as to what extent that might influence the cytokine levels, therefore, we conducted time analysis of IFNAs protein level at 2, 4, 5, and 24h time-points. Although initially the levels of IFNAs were higher in the medium of S protein expressing cells, there was no significant difference in their levels after the 5h time-point, compared to the mock control.
Correlation between transcriptomic changes and protein levels cannot always be drawn, and while genomic regulation of certain cytokines; such as TNF-α, may closely mirror their protein levels, other cytokines such as IL-6 may not show a similar pattern (67). In regards to IFNAs, the downregulation of 1-1.5 log-fold changes observed on the transcriptomic level might have been reflected by no further increase of IFNA cytokines beyond the 5 h time-point, compared to the mock control.
In conclusion, our study shows that SARS-CoV-2 S induces complex and orchestrated cellular transcriptomic changes in human monocyte-derived macrophages that undoubtedly contribute to the pathogenesis of infection. According to our knowledge, downregulation of protocadherins and type I interferons by the S protein at an early (5 hours) time-point post-transfection is a novel finding, and should be taken into consideration in the context of vaccination strategies, as this may result in the activation of other latent viral infections; such as EBV infection in vaccinated individuals or during the course of COVID-19 (68, 69).
However, our study is not without limitations. First, expression of the S protein by our plasmid was in fusion with and N-terminal EGFP tag, which may have affected the localization of the S protein, although, the significantly altered transcripts were compared to transfection with the GFP-only expressing plasmid utilized as mock. Also, it would be of interest to see whether or not the differential expression of genes persists beyond the 5 hours’ time-point. Moreover, additional studies utilizing proteomic techniques and proximity ligation assays to study intracellular interactions of the S protein would have been advantageous; however, this may perhaps be the subject of a follow-up study.
Another limitation of our study is that polarization into classically activated (M1) and alternatively activated (M2) macrophages was not carried out, which could have provided important information on the distinct alteration of cytokine response induced by the S protein. Recent studies have shown that distinct macrophage phenotypes show differential immune response patterns to infection (70), and M1 and M2 phenotypes showed noticeable difference in uptake, amplification, and release of SARS-CoV-2 (71). This analysis however, was beyond the scope of our current study, and shall be explored in the future. In this study, we analyzed the transcriptomic response and key cytokine levels in PMA-differentiated THP-1 cells. Cluster of differentiation (CD) marker analysis showed that > 50% of the cells were at M0 stage, as they expressed CD11b, but not CD80 or CD206. This is in line with literature, since THP-1 cells apparently do not express CD80 or CD206, neither in resting nor activated state, but they express CD11b upon stimulation with PMA (72). Therefore, we were not able to verify the rest of the phenotypes, and were not able to ascertain or control for the transcriptomic changes and cytokine profile between M2 and M1 phenotypes.
All in all, our results shed light on the complex pathophysiology of SARS-CoV-2, focusing on the S protein given its wide utilization in vaccination strategies, and despite the current limitations of this study, the findings presented are nevertheless important, providing an insight into how the S protein alters the cellular milieu and transcriptomic profile, in order to establish an optimal cellular environment for viral infection and production.
Data availability statement
Data has been submitted to GEO repository under GSE208320 identifier.
Author contributions
Conceptualization: MM, JT. Methodology: MM, NM, AA, HA, and TL. Software: VA. Validation: VA, MM, JT. Formal Analysis: MM, JT, NM and TL. Investigation: MM, NM, AA, HA, TL and VA. Resources: JT, SB. Data Curation: VA. Writing – original draft preparation: MM, NM, TL, VA. Writing – review and editing: MM, NM, JM, JT, SB. Visualization: MM, JT. Supervision: MM. Project administration: JT. Funding Acquisition: JT. All authors contributed to the article and approved the submitted version.
Funding
Project no. TKP2021-EGA-20 (Biotechnology) has been implemented with the support provided from the National Research, Development and Innovation Fund of Hungary, financed under the TKP2021-EGA funding scheme. This project was supported by the grant for high-risk research in the post-COVID field of the Hungarian Academy of Sciences (POST-COVID2021-16), and by the Hungarian National Scientific Research Fund (NKFIH-OTKA Grant No. K131844) to SB. HA and AA hold a Stipendium Hungaricum Scholarship from the Government of Hungary.
Acknowledgments
The authors are grateful to the members of Laboratory of Retroviral Biochemistry research group at the Department of Biochemistry and Molecular Biology (University of Debrecen). The authors are grateful for the high-throughput sequencing service of Genomic Medicine and Bioinformatics Core Facility of University of Debrecen (Hungary).
Conflict of interest
The authors declare that the research was conducted in the absence of any commercial or financial relationships that could be construed as a potential conflict of interest.
Publisher’s note
All claims expressed in this article are solely those of the authors and do not necessarily represent those of their affiliated organizations, or those of the publisher, the editors and the reviewers. Any product that may be evaluated in this article, or claim that may be made by its manufacturer, is not guaranteed or endorsed by the publisher.
Supplementary material
The Supplementary Material for this article can be found online at: https://www.frontiersin.org/articles/10.3389/fimmu.2022.999233/full#supplementary-material
Supplementary Table 1 | List of GO enrichment terms of RNA transcripts detected from control, mock and spike-transfected THP1 cells.
Supplementary Figure 1 | Cluster heatmap of differently regulated genes among the spike-transfected and mock samples.
References
1. Walls AC, Park YJ, Tortorici MA, Wall A, McGuire AT, Veesler D. Structure, function, and antigenicity of the SARS-CoV-2 spike glycoprotein. Cell (2020) 183:1735. doi: 10.1016/j.cell.2020.11.032
2. Belouzard S, Millet JK, Licitra BN, Whittaker GR. Mechanisms of coronavirus cell entry mediated by the viral spike protein. Viruses (2012) 4:1011–33. doi: 10.3390/v4061011
3. Belouzard S, Chu VC, Whittaker GR. Activation of the SARS coronavirus spike protein via sequential proteolytic cleavage at two distinct sites. Proc Natl Acad Sci USA (2009) 106:5871–6. doi: 10.1073/pnas.0809524106
4. Hoffmann M, Kleine-Weber H, Schroeder S, Kruger N, Herrler T, Erichsen S, et al. SARS-CoV-2 cell entry depends on ACE2 and TMPRSS2 and is blocked by a clinically proven protease inhibitor. Cell (2020) 181:271–280 e8. doi: 10.1016/j.cell.2020.02.052
5. Lukassen S, Chua RL, Trefzer T, Kahn NC, Schneider MA, Muley T, et al. SARS-CoV-2 receptor ACE2 and TMPRSS2 are primarily expressed in bronchial transient secretory cells. EMBO J (2020) 39:e105114. doi: 10.15252/embj.2020105114
6. Djomkam ALZ, Olwal CO, Sala TB, Paemka L. Commentary: SARS-CoV-2 cell entry depends on ACE2 and TMPRSS2 and is blocked by a clinically proven protease inhibitor. Front Oncol (2020) 10:1448. doi: 10.3389/fonc.2020.01448
7. Kawase M, Shirato K, van der Hoek L, Taguchi F, Matsuyama S. Simultaneous treatment of human bronchial epithelial cells with serine and cysteine protease inhibitors prevents severe acute respiratory syndrome coronavirus entry. J Virol (2012) 86:6537–45. doi: 10.1128/JVI.00094-12
8. Murgolo N, Therien AG, Howell B, Klein D, Koeplinger K, Lieberman LA, et al. SARS-CoV-2 tropism, entry, replication, and propagation: Considerations for drug discovery and development. PloS Pathog (2021) 17:e1009225. doi: 10.1371/journal.ppat.1009225
9. Kyriakidis NC, Lopez-Cortes A, Gonzalez EV, Grimaldos AB, Prado EO. SARS-CoV-2 vaccines strategies: a comprehensive review of phase 3 candidates. NPJ Vaccines (2021) 6:28. doi: 10.1038/s41541-021-00292-w
10. Zhou D, Dejnirattisai W, Supasa P, Liu C, Mentzer AJ, Ginn HM, et al. Evidence of escape of SARS-CoV-2 variant B.1.351 from natural and vaccine-induced sera. Cell (2021) 184:2348–2361 e6. doi: 10.1016/j.cell.2021.02.037
11. Hoffmann M, Kruger N, Schulz S, Cossmann A, Rocha C, Kempf A, et al. The omicron variant is highly resistant against antibody-mediated neutralization: Implications for control of the COVID-19 pandemic. Cell (2022) 185:447–56.e11. doi: 10.1016/j.cell.2021.12.032
12. Chen IY, Chang SC, Wu HY, Yu TC, Wei WC, Lin S, et al. Upregulation of the chemokine (C-c motif) ligand 2 via a severe acute respiratory syndrome coronavirus spike-ACE2 signaling pathway. J Virol (2010) 84:7703–12. doi: 10.1128/JVI.02560-09
13. Patra T, Meyer K, Geerling L, Isbell TS, Hoft DF, Brien J, et al. SARS-CoV-2 spike protein promotes IL-6 trans-signaling by activation of angiotensin II receptor signaling in epithelial cells. PloS Pathog (2020) 16:e1009128. doi: 10.1371/journal.ppat.1009128
14. Suzuki YJ, Gychka SG. SARS-CoV-2 spike protein elicits cell signaling in human host cells: Implications for possible consequences of COVID-19 vaccines. Vaccines (2021) 9(1):36. doi: 10.3390/vaccines9010036
15. Basu A, Penumutchu S, Nguyen K, Mbonye U, Tolbert BS, Karn J, et al. A structurally conserved RNA element within SARS-CoV-2 ORF1a RNA and s mRNA regulates translation in response to viral s protein-induced signaling in human lung cells. J Virol (2022) 96:e0167821. doi: 10.1128/JVI.01678-21
16. Al-Qahtani AA, Lyroni K, Aznaourova M, Tseliou M, Al-Anazi MR, Al-Ahdal MN, et al. Middle east respiratory syndrome corona virus spike glycoprotein suppresses macrophage responses via DPP4-mediated induction of IRAK-m and PPARgamma. Oncotarget (2017) 8:9053–66. doi: 10.18632/oncotarget.14754
17. Barhoumi T, Alghanem B, Shaibah H, Mansour FA, Alamri HS, Akiel MA, et al. SARS-CoV-2 coronavirus spike protein-induced apoptosis, inflammatory, and oxidative stress responses in THP-1-Like-Macrophages: Potential role of angiotensin-converting enzyme inhibitor (Perindopril). Front Immunol (2021) 12:728896. doi: 10.3389/fimmu.2021.728896
18. Ropa J, Cooper S, Capitano ML, Van’t Hof W, Broxmeyer HE. Human hematopoietic stem, progenitor, and immune cells respond ex vivo to SARS-CoV-2 spike protein. Stem Cell Rev Rep (2021) 17:253–65. doi: 10.1007/s12015-020-10056-z
19. Buonvino S, Melino S. New consensus pattern in spike CoV-2: potential implications in coagulation process and cell-cell fusion. Cell Death Discov (2020) 6:134. doi: 10.1038/s41420-020-00372-1
20. Abou-Ismail MY, Diamond A, Kapoor S, Arafah Y, Nayak L. The hypercoagulable state in COVID-19: Incidence, pathophysiology, and management. Thromb Res (2020) 194:101–15. doi: 10.1016/j.thromres.2020.06.029
21. Gauthier T, Chen W. Modulation of macrophage immunometabolism: A new approach to fight infections. Front Immunol (2022) 13:780839. doi: 10.3389/fimmu.2022.780839
22. Guilliams M, Mildner A, Yona S. Developmental and functional heterogeneity of monocytes. Immunity (2018) 49:595–613. doi: 10.1016/j.immuni.2018.10.005
23. Nikitina E, Larionova I, Choinzonov E, Kzhyshkowska J. Monocytes and macrophages as viral targets and reservoirs. Int J Mol Sci (2018) 19(9):2821. doi: 10.3390/ijms19092821
24. Boumaza A, Gay L, Mezouar S, Bestion E, Diallo AB, Michel M, et al. Monocytes and macrophages, targets of severe acute respiratory syndrome coronavirus 2: The clue for coronavirus disease 2019 immunoparalysis. J Infect Dis (2021) 224:395–406. doi: 10.1093/infdis/jiab044
25. Grant RA, Morales-Nebreda L, Markov NS, Swaminathan S, Guzman ER, Abbott DA, et al. Alveolitis in severe SARS-CoV-2 pneumonia is driven by self-sustaining circuits between infected alveolar macrophages and T cells. bioRxiv: preprint Server Biol (2020). doi: 10.1101/2020.08.05.238188
26. Lu F, Hogenesch H. Kinetics of the inflammatory response following intramuscular injection of aluminum adjuvant. Vaccine (2013) 31:3979–86. doi: 10.1016/j.vaccine.2013.05.107
27. Kulasinghe A, Tan CW, Dos Santos Miggiolaro AFR, Monkman J, SadeghiRad H, Bhuva DD, et al. Profiling of lung SARS-CoV-2 and influenza virus infection dissects virus-specific host responses and gene signatures. Eur Respir J (2021) 59(6):2101881. doi: 10.1101/2020.11.04.20225557
28. Abdelmoaty MM, Yeapuri P, Machhi J, Olson KE, Shahjin F, Kumar V, et al. Defining the innate immune responses for SARS-CoV-2-Human macrophage interactions. Front Immunol (2021) 12:741502. doi: 10.3389/fimmu.2021.741502
30. Bolger AM, Lohse M, Usadel B. Trimmomatic: a flexible trimmer for illumina sequence data. Bioinformatics (2014) 30:2114–20. doi: 10.1093/bioinformatics/btu170
31. Kim D, Langmead B, Salzberg SL. HISAT: a fast spliced aligner with low memory requirements. Nat Methods (2015) 12:357–60. doi: 10.1038/nmeth.3317
32. Langmead B, Trapnell C, Pop M, Salzberg SL. Ultrafast and memory-efficient alignment of short DNA sequences to the human genome. Genome Biol (2009) 10:R25. doi: 10.1186/gb-2009-10-3-r25
33. Love MI, Huber W, Anders S. Moderated estimation of fold change and dispersion for RNA-seq data with DESeq2. Genome Biol (2014) 15:550. doi: 10.1186/s13059-014-0550-8
34. Raudvere U, Kolberg L, Kuzmin I, Arak T, Adler P, Peterson H, et al. g:Profiler: a web server for functional enrichment analysis and conversions of gene lists (2019 update). Nucleic Acids Res (2019) 47:W191–8. doi: 10.1093/nar/gkz369
35. Shannon P, Markiel A, Ozier O, Baliga NS, Wang JT, Ramage D, et al. Cytoscape: a software environment for integrated models of biomolecular interaction networks. Genome Res (2003) 13:2498–504. doi: 10.1101/gr.1239303
36. Madhvi A, Mishra H, Leisching GR, Mahlobo PZ, Baker B. Comparison of human monocyte derived macrophages and THP1-like macrophages as in vitro models for m. tuberculosis infection. Comp Immunol Microbiol Infect Dis (2019) 67:101355. doi: 10.1016/j.cimid.2019.101355
37. Tedesco S, De Majo F, Kim J, Trenti A, Trevisi L, Fadini GP, et al. Convenience versus biological significance: Are PMA-differentiated THP-1 cells a reliable substitute for blood-derived macrophages when studying in vitro polarization? Front Pharmacol (2018) 9:71. doi: 10.3389/fphar.2018.00071
38. Qin Z. The use of THP-1 cells as a model for mimicking the function and regulation of monocytes and macrophages in the vasculature. Atherosclerosis (2012) 221:2–11. doi: 10.1016/j.atherosclerosis.2011.09.003
39. Lund ME, To J, O’Brien BA, Donnelly S. The choice of phorbol 12-myristate 13-acetate differentiation protocol influences the response of THP-1 macrophages to a pro-inflammatory stimulus. J Immunol Methods (2016) 430:64–70. doi: 10.1016/j.jim.2016.01.012
40. Liu Y, Li H, Czajkowsky DM, Shao Z. Monocytic THP-1 cells diverge significantly from their primary counterparts: a comparative examination of the chromosomal conformations and transcriptomes. Hereditas (2021) 158:43. doi: 10.1186/s41065-021-00205-w
41. Chanput W, Peters V, Wichers H. THP-1 and U937 cells. In: Verhoeckx K, Cotter P, Lopez-Exposito I, Kleiveland C, Lea T, Mackie A, Requena T, Swiatecka D, Wichers H, editors. The impact of food bioactives on health: in vitro and ex vivo models. Cham (CH): Springer (2015). p. 147–59.
42. Morishita H, Yagi T. Protocadherin family: diversity, structure, and function. Curr Opin Cell Biol (2007) 19:584–92. doi: 10.1016/j.ceb.2007.09.006
43. Hirayama T, Yagi T. The role and expression of the protocadherin-alpha clusters in the CNS. Curr Opin Neurobiol (2006) 16:336–42. doi: 10.1016/j.conb.2006.05.003
44. Yamagishi T, Yoshitake K, Kamatani D, Watanabe K, Tsukano H, Hishida R, et al. Molecular diversity of clustered protocadherin-alpha required for sensory integration and short-term memory in mice. Sci Rep (2018) 8:9616. doi: 10.1038/s41598-018-28034-4
45. Katori S, Noguchi-Katori Y, Okayama A, Kawamura Y, Luo W, Sakimura K, et al. Protocadherin-alphaC2 is required for diffuse projections of serotonergic axons. Sci Rep (2017) 7:15908. doi: 10.1038/s41598-017-16120-y
46. Mountoufaris G, Chen WV, Hirabayashi Y, O’Keeffe S, Chevee M, Nwakeze CL, et al. Multicluster pcdh diversity is required for mouse olfactory neural circuit assembly. Science (2017) 356:411–4. doi: 10.1126/science.aai8801
47. Zhang LQ, Cheranova D, Gibson M, Ding S, Heruth DP, Fang D, et al. RNA-Seq reveals novel transcriptome of genes and their isoforms in human pulmonary microvascular endothelial cells treated with thrombin. PloS One (2012) 7:e31229. doi: 10.1164/ajrccm-conference.2012.185.1_MeetingAbstracts.A1874
48. Henrie A, Hemphill SE, Ruiz-Schultz N, Cushman B, DiStefano MT, Azzariti D, et al. ClinVar miner: Demonstrating utility of a web-based tool for viewing and filtering ClinVar data. Hum Mutat (2018) 39:1051–60. doi: 10.1002/humu.23555
49. Du Y, Yang F, Wang Q, Xu N, Xie Y, Chen S, et al. Influenza a virus antagonizes type I and type II interferon responses via SOCS1-dependent ubiquitination and degradation of JAK1. Virol J (2020) 17:74. doi: 10.1186/s12985-020-01348-4
50. Schoggins JW, Rice CM. Interferon-stimulated genes and their antiviral effector functions. Curr Opin Virol (2011) 1:519–25. doi: 10.1016/j.coviro.2011.10.008
51. Vestal DJ, Jeyaratnam JA. The guanylate-binding proteins: emerging insights into the biochemical properties and functions of this family of large interferon-induced guanosine triphosphatase. J Interferon Cytokine Res (2011) 31:89–97. doi: 10.1089/jir.2010.0102
52. Marie I, Durbin JE, Levy DE. Differential viral induction of distinct interferon-alpha genes by positive feedback through interferon regulatory factor-7. EMBO J (1998) 17:6660–9. doi: 10.1093/emboj/17.22.6660
53. Lavoie TB, Kalie E, Crisafulli-Cabatu S, Abramovich R, DiGioia G, Moolchan K, et al. Binding and activity of all human alpha interferon subtypes. Cytokine (2011) 56:282–9. doi: 10.1016/j.cyto.2011.07.019
54. Liu X, Sadaoka T, Krogmann T, Cohen JI. Epstein-Barr Virus (EBV) tegument protein BGLF2 suppresses type I interferon signaling to promote EBV reactivation. J Virol (2020) 94(11):e00258-20. doi: 10.1128/JVI.00258-20
55. Sainz B Jr., LaMarca HL, Garry RF, Morris CA. Synergistic inhibition of human cytomegalovirus replication by interferon-alpha/beta and interferon-gamma. Virol J (2005) 2:14. doi: 10.1186/1743-422X-2-14
56. Blanco-Melo D, Nilsson-Payant BE, Liu WC, Uhl S, Hoagland D, Moller R, et al. Imbalanced host response to SARS-CoV-2 drives development of COVID-19. Cell (2020) 181:1036–1045 e9. doi: 10.1016/j.cell.2020.04.026
57. Chu H, Chan JF, Wang Y, Yuen TT, Chai Y, Hou Y, et al. Comparative replication and immune activation profiles of SARS-CoV-2 and SARS-CoV in human lungs: An ex vivo study with implications for the pathogenesis of COVID-19. Clin Infect Dis (2020) 71:1400–9. doi: 10.1093/cid/ciaa410
58. Shi CS, Qi HY, Boularan C, Huang NN, Abu-Asab M, Shelhamer JH, et al. SARS-coronavirus open reading frame-9b suppresses innate immunity by targeting mitochondria and the MAVS/TRAF3/TRAF6 signalosome. J Immunol (2014) 193:3080–9. doi: 10.4049/jimmunol.1303196
59. Gordon DE, Jang GM, Bouhaddou M, Xu J, Obernier K, White KM, et al. A SARS-CoV-2 protein interaction map reveals targets for drug repurposing. Nature (2020) 583:459–68. doi: 10.1038/s41586-020-2286-9
60. Sa Ribero M, Jouvenet N, Dreux M, Nisole S. Interplay between SARS-CoV-2 and the type I interferon response. PloS Pathog (2020) 16:e1008737. doi: 10.1371/journal.ppat.1008737
61. Lokugamage KG, Hage A, de Vries M, Valero-Jimenez AM, Schindewolf C, Dittmann M, et al. Type I interferon susceptibility distinguishes SARS-CoV-2 from SARS-CoV. J Virol (2020) 94(23):e01410-20. doi: 10.1128/JVI.01410-20
62. Shindo T, Kurihara H, Kuno K, Yokoyama H, Wada T, Kurihara Y, et al. ADAMTS-1: a metalloproteinase-disintegrin essential for normal growth, fertility, and organ morphology and function. J Clin Invest (2000) 105:1345–52. doi: 10.1172/JCI8635
63. Tan Ide A, Ricciardelli C, Russell DL. The metalloproteinase ADAMTS1: a comprehensive review of its role in tumorigenic and metastatic pathways. Int J Cancer (2013) 133:2263–76. doi: 10.1002/ijc.28127
64. Norden R, Nystrom K, Aurelius J, Brisslert M, Olofsson S. Virus-induced appearance of the selectin ligand sLeX in herpes simplex virus type 1-infected T-cells: involvement of host and viral factors. Glycobiology (2013) 23:310–21. doi: 10.1093/glycob/cws160
65. Zhao Y, Kuang M, Li J, Zhu L, Jia Z, Guo X, et al. SARS-CoV-2 spike protein interacts with and activates TLR41. Cell Res (2021) 31:818–20. doi: 10.1038/s41422-021-00495-9
66. Gu T, Zhao S, Jin G, Song M, Zhi Y, Zhao R, et al. Cytokine signature induced by SARS-CoV-2 spike protein in a mouse model. Front Immunol (2020) 11:621441. doi: 10.3389/fimmu.2020.621441
67. Shebl FM, Pinto LA, Garcia-Pineres A, Lempicki R, Williams M, Harro C, et al. Comparison of mRNA and protein measures of cytokines following vaccination with human papillomavirus-16 L1 virus-like particles. Cancer Epidemiol Biomarkers Prev (2010) 19:978–81. doi: 10.1158/1055-9965.EPI-10-0064
68. Chen T, Song J, Liu H, Zheng H, Chen C. Positive Epstein-Barr virus detection in coronavirus disease 2019 (COVID-19) patients. Sci Rep (2021) 11:10902. doi: 10.1038/s41598-021-90351-y
69. Gold JE, Okyay RA, Licht WE, Hurley DJ. Investigation of long COVID prevalence and its relationship to Epstein-Barr virus reactivation. Pathogens (2021) 10(6):763. doi: 10.3390/pathogens10060763
70. Lian Q, Zhang K, Zhang Z, Duan F, Guo L, Luo W, et al. Differential effects of macrophage subtypes on SARS-CoV-2 infection in a human pluripotent stem cell-derived model. Nat Commun (2022) 13:2028. doi: 10.1038/s41467-022-29731-5
71. Lv J, Wang Z, Qu Y, Zhu H, Zhu Q, Tong W, et al. Distinct uptake, amplification, and release of SARS-CoV-2 by M1 and M2 alveolar macrophages. Cell Discov (2021) 7:24. doi: 10.1038/s41421-021-00258-1
72. Forrester MA, Wassall HJ, Hall LS, Cao H, Wilson HM, Barker RN, et al. Similarities and differences in surface receptor expression by THP-1 monocytes and differentiated macrophages polarized using seven different conditioning regimens. Cell Immunol (2018) 332:58–76. doi: 10.1016/j.cellimm.2018.07.008
Keywords: SARS-CoV-2, coronavirus, spike protein, transcriptomics, COVID-19
Citation: Miltner N, Linkner TR, Ambrus V, Al-Muffti AS, Ahmad H, Mótyán JA, Benkő S, Tőzsér J and Mahdi M (2022) Early suppression of antiviral host response and protocadherins by SARS-CoV-2 Spike protein in THP-1-derived macrophage-like cells. Front. Immunol. 13:999233. doi: 10.3389/fimmu.2022.999233
Received: 20 July 2022; Accepted: 05 October 2022;
Published: 20 October 2022.
Edited by:
Jacqueline Valverde-Villegas, UMR5535 Institut de Génétique Moléculaire de Montpellier (IGMM), FranceReviewed by:
Qizhou Lian, The University of Hong Kong, Hong Kong SAR, ChinaTlili Barhoumi, King Abdullah International Medical Research Center (KAIMRC), Saudi Arabia
Copyright © 2022 Miltner, Linkner, Ambrus, Al-Muffti, Ahmad, Mótyán, Benkő, Tőzsér and Mahdi. This is an open-access article distributed under the terms of the Creative Commons Attribution License (CC BY). The use, distribution or reproduction in other forums is permitted, provided the original author(s) and the copyright owner(s) are credited and that the original publication in this journal is cited, in accordance with accepted academic practice. No use, distribution or reproduction is permitted which does not comply with these terms.
*Correspondence: Mohamed Mahdi, bW9oYW1lZEBtZWQudW5pZGViLmh1; József Tőzsér, dG96c2VyQG1lZC51bmlkZWIuaHU=
†These authors share first authorship