- 1Department of Hand and Microsurgery, Xiangya Hospital of Central South University, Changsha, China
- 2National Clinical Research Center for Geriatric Disorders, Xiangya Hospital of Central South University, Changsha, China
Long-time ischemia worsening transplant outcomes in vascularized composite allotransplantation (VCA) is often neglected. Ischemia-reperfusion injury (IRI) is an inevitable event that follows reperfusion after a period of cold static storage. The pathophysiological mechanism activates local inflammation, which is a barrier to allograft long-term immune tolerance. The previous publications have not clearly described the relationship between the tissue damage and ischemia time, nor the rejection grade. In this review, we found that the rejection episodes and rejection grade are usually related to the ischemia time, both in clinical and experimental aspects. Moreover, we summarized the potential therapeutic measures to mitigate the ischemia-reperfusion injury. Compare to static preservation, machine perfusion is a promising method that can keep VCA tissue viability and extend preservation time, which is especially beneficial for the expansion of the donor pool and better MHC-matching.
Introduction
The world of reconstructive transplantation is mature (1). The challenges of allograft rejection have focused research on the long-term success of vascularized allograft transplantation (2, 3). Ischemia-reperfusion injury (IRI) is a potential threat to long-term allograft success, which is an inevitable event that follows reperfusion after a period of cold static storage (4, 5). This review summarizes the current clinical and laboratory aspects that discuss the relationship between transplant outcomes and IRI tissue damage. It can give some implications to reduce the IRI to achieve long-term VCA allograft survival.
Mechanisms of IRI
Ischemia leads to hypoxic anaerobic glycolysis and oxygen consumption, depleting adenosine triphosphate (ATP) and dysregulating ATP-dependent membrane ion exchangers (6, 7), reducing the activity of the Na+/K+/ATPase pump and increasing intracellular sodium concentration (8). Furthermore, the reduction in the intracellular concentration of ATP prevents the regeneration of glutathione, ascorbic acid and tocopherol that take part in detoxifying the metabolites present in the cytosol and the sarcoplasmic membrane. The accumulation of osmotically active particles such as lactate, sodium, inorganic phosphate and creatine leads to cell edema.
Moreover, cellular acidosis can stimulate the antiport Na+/H+ receptors, worsening the sodium overload and affecting the function of other membrane receptors such as the Na+/Ca2+ antiport. The Na+/Ca2+ antiport enables sodium exportation from cells based on the intracellular calcium concentration (9). Cellular hypercalcemia causes the breakdown of sarcoplasmic phospholipids and cytoskeleton protein, alters contractile protein’s efficiency and calcium affinity, and changes the tertiary structure of certain enzymes such as xanthine dehydrogenase to xanthine oxidase (10). These two enzymes have similar functions: the transformation of hypoxanthine in xanthine and xanthine in uric acid. Damage to calcium-dependent receptors increases cytosolic calcium, loss of homeostasis, activation of proteolytic enzymes, cell membrane disruption, and release of free fatty acids. Collectively, this dysfunction manifests as cell apoptosis or necrosis (11–13).
Reperfusion triggers a localized microvascular and systemic reaction, resulting in further tissue damage (14–16). Mitochondria respond to many different types of stress like oxidative and metabolic stresses (17–19). They are the primary source of reactive oxygen species (ROS), a by-product of respiration generated mainly at the electron transport chain complexes I and III (20). Ca2+ overload, with high ROS and Pi, changes mitochondrial membrane permeability and induces the opening of non-selective and high-conductance permeability transition pores (PTP) in the inner mitochondrial membrane (21–23). The PTP further compromises mitochondria’s bioenergetics function and structural integrity, leading to cell death (24–26). The release of ROS, mainly from mitochondria, forms the basis for IRI (27, 28).
How the IRI influences the transplant outcome
Experimental implications
IRI in the skin and subcutaneous tissue
IRI in the skin has been reported in several publications, not only in VCA but also in flap surgery (29–31). Skin and subcutaneous tissue are relatively resistant to the effects of anoxia, and intracellular pH changes are reversible for up to 24 h (32). Donski et al. (33) investigated the effect of cooling on the survival of free flaps in rabbit. They found 86% of flaps that were cooled for 1–3d survived. Meanwhile, other authors concluded that the maximum ischemia time of a rat flap was 6h at normal body temperatures and 48h if cooled (34). Thus, the warm IRI has more serious tissue damage than the cold IRI.
As VCA tissue is usually preserved at 4 °Cfor 6h, the warm ischemia time in VCA skin and subcutaneous tissue is pretty short. The tissue damage in the skin and subcutaneous tissue can be ignored. However, the IRI should be considered if warm ischemia is >6h or cold ischemia time >24h.
IRI in the skeleton muscle
Compared to the skin, mammalian skeletal muscle is substantially less tolerant to ischemia (35). Irreversible damage to the microcirculation of skeletal muscle in man begins at around 6 h (36). Wagh et al. (37, 38) found that skeletal muscle is much more susceptible to damage from cold (4°C) ischemic storage than skin, with an estimated critical ischemia time for rat gastrocnemius muscle flaps of approximately 16 h compared with approximately 3.5 days for rat epigastric skin flaps. Although measures have been taken to ameliorate the IRI in muscles, lots of results are based on short time warm-ischemia time (39–43). The data for VCA clinical usage is limited.
IRI in the vessel
The endothelium is very sensitive to I/R injuries (44, 45). It is essential to preserve the endothelium because endothelial cells have several vital functions, including controlling vascular tone and local blood flow, modulating coagulation and inflammation, participating in immune response, regulating micro and macromolecules’ movement towards the interstitium, and assisting in angiogenesis (46). Endothelium-dependent vasodilatation is more susceptible to IRI than vasoconstriction and endothelial-independent vasodilatation (47, 48). ROS and tumor necrosis factor-alpha(TNF-α) play a significant role in this process. Reperfusion also induces a critical inflammatory response, characterized by a massive production of free radicals and activation of the complement pathway, leucocytes and neutrophils (49). A little interaction between activated endothelium and neutrophils will result in a significant concentration of activated neutrophils in the interstitium, which release oxygen radicals and proteases, leading to the destruction of cells and the extracellular matrix. The migration of neutrophils from the intravascular bed to the interstitium involves several families of proteins such as selectins (P-selectin and L-selectin), integrins (intercellular adhesion molecule-1), and immunoglobulins (platelet-endothelial cell adhesion molecule-1). Lastly, oxidative stress, cytokine production, and the secondary mitochondrial lesions that occur with reperfusion induce apoptosis in parenchymal cells and the vascular structures.
In addition, vascular endothelial cells are the initial barrier to allograft-activated host immune rejection and are critical in triggering cell-mediated acute rejection (50). It has been found that circulating mitochondria in organ donors with prolonged ischemia may directly activate allograft vascular endothelial cells and promote graft rejection (51–53). Therefore, endothelial cells mediate acute graft rejection after IRI. The targeted intervention of mitochondrial damage in vascular endothelial cells, thereby reducing graft rejection events, has also been a research hot-spot in recent years.
IRI in the nerve
Although much is known, the precise pathophysiology of IRI in the peripheral nerve remains to be elucidated. Microvascular events, which may occur during reperfusion, may be important in amplifying the nerve fiber degeneration that is initiated during ischemia (54). Haruyasu Iida et al. (55) showed that reperfusion induced oxidative damage, which lowered nerve function and increased fiber deterioration, but extending the period of reperfusion to 42 days allowed for fiber regeneration. To reduce oxidative injury, Sang-Jin Shin et al. (56) investigated how inducible nitric oxide synthase (iNOS) inhibition affects the recovery of motor function in the rat sciatic nerve after IRI. Their study indicated that early inhibition of iNOS is vital for IRI reduction or prevention. Franka et al. (57) studied the critical ischemia times of individual tissues of a rat limb isograft. Histomorphometric investigation of the tibial nerve on POD 10 showed the typical signs of Wallerian degeneration in all transplanted animals and the nerve transection groups. The nerve of non-transplanted controls appeared to be normal in shape without signs of injury or cell infiltration. Overall, histopathological scores for nerve damage were significantly higher in the ischemia group than transection group. In general, nerve scores increased proportionally with the duration of ischemia time.
IRI in the bone
Compared to most other organs, the bone’s IRI is poorly understood, particularly from a mechanistic perspective. However, IRI of the bone is considered to occur in various diseases/situations (58–61). such as vascular disruption or compression, fractures, limb replantation/allotransplantation, and thromboembolic disorders. Moreover, some systemic diseases such as sickle cell anemia, Caisson disease, and Cushing’s disease may initiate IRI in the bone (62–64).
As systemic diseases influence many organs, it’s hard to investigate the mechanism of reperfusion injury in bones. Thus, bone IRI has been studied by interrupting blood supply through vascular compression (clamping) or dissection. In these studies, limb or bone graft replantation/transplantation was performed after preserving the limbs/grafts at 0 – 4 °C or room temperature (21 –25°C) (65–68). The studies concluded that significant retardation of bone growth/development occurs when critical ischemia lasts between 3 to 7 h at 37 °C. But the critical ischemia time increases with decreasing temperature. In some studies, even cold ischemia time (0 – 5 °C) of 25 h and above have been found to be tolerated (69).
The fact that therapy with antioxidants resulted in considerable protection proves that reperfusion injury of the bone, or extra injury during the reperfusion period, occurs (70). This reiterates the protective function of antioxidants against ROS. ROS can only be formed in the presence of O2, which means upon reperfusion. The available results, however, suggest some similarities to the mechanisms of IRI of other organs, such as the involvement of ROS (71, 72).
From a review of the literature, we have summarized the following critical ischemia time of VCA tissues (Table 1):
Many papers reported the IRI tissue damage in VCA. We briefly introduce the tissue damage in different types of VCA tissue. The lack of blood supply does not damage all tissues in the allografts to the same degree; some tissues are more susceptible than others. Those damaged tissues may release some molecules and activate the innate immune response, which is a barrier to long-term allograft survival (5, 73–75). In this review, we are not only focused on the relationship between the IRI and tissue damage but also discuss the relationship between IRI and transplant rejection caused by tissue damage (Table 2).
Clinical experience
Ischemia is clinically an inevitable factor following donor organ procurement, cold preservation, and implantation. Though its specific role in VCA is occasionally underappreciated, the IRI can affect graft survival, function, and rejection. However, there is a paucity of studies examining IRI in VCA clinical usage. The experience in re-transplantation has opened a window for us to know the critical ischemia time related to VCA. The recommended ischemia times compatible with reliable success in replantation are 6 h of warm and 12 h of cold ischemia for major replants, although successful replantations have been reported after longer ischemia times (86–89). The ischemia time is largely influenced by skeleton muscle and causes it even more susceptible to IRI. Besides tissue damage, the literature in SOT has clearly demonstrated that IRI is a potent activator of the immune system and subsequently leads to occur rejection episodes. These clinical findings have also been testified in VCA. Based on the presence of a more aggressive diffuse lymphocytic infiltration and disruption of tissue architecture, Pradka et al. (90) showed that skin and muscle exposed to 3 h of ischemia had significantly higher rejection scores than when exposed to 1 h of ischemia.
To sum up, the IRI in VCA has the following effect on the graft and body.
Graft survival
Insult to the endothelium leads to an upregulation in the expression of bioactive agents (such as endothelin and thromboxane) and suppresses the release of nitric oxide (NO), increasing vascular tone. The presence of free radical accumulation, endothelial edema, and platelet activation can result in the cessation of blood flow through the graft. Thus, graft survival is threatened by IRI (91, 92).
Tissue damage
Muscle and nerve dysfunction in the donor limb has a significant impact even if the graft survives since their physiological integrity is crucial for maintaning physical function. We clearly described the IRI tissue damage in experimental implications. The muscle tissue is sensitive to the IRI, then the skin and subcutaneous, bone is more tolerant to IRI (93–95). However, the muscle normal structure and functional recovery are very important to the VCA surgical outcomes. Besides, the neuromuscular junctions are most sensitive to ischemia (96). Since most organs are still preserved in static preservation, muscle tissue is more sensitive to IRI than other organs, and it is not easy to regenerate after IRI, reducing IRI tissue damage has a positive effect on the functional recovery of VCA.
Graft rejection
As it is well known, there are 3 necessary steps for graft rejection. Step 1: Leukocyte infiltration (endothelial cells dysfunction, P-selectin and L-selectin, intercellular adhesion molecule-1, platelet-endothelial cell adhesion molecule-1). Step 2: Leukocyte activation (local inflammation). Step 3: Target organ (effective T cells and cytokines producing). Injured tissues activate the immune system by releasing damage-associated molecular patterns, which are endogenous molecules that mediate cellular injury. Their release upregulates endothelial adhesion molecules, migration of leukocytes into the graft and complement activation. This localized inflammatory response hampers the development of tolerance (97, 98). This explains why post-transplant graft dysfunction and rejection are proportional to the intensity and duration of IRI (Figure 1). Thus, ameliorating the IRI in VCA may reduce the incidence of VCA rejection (99).
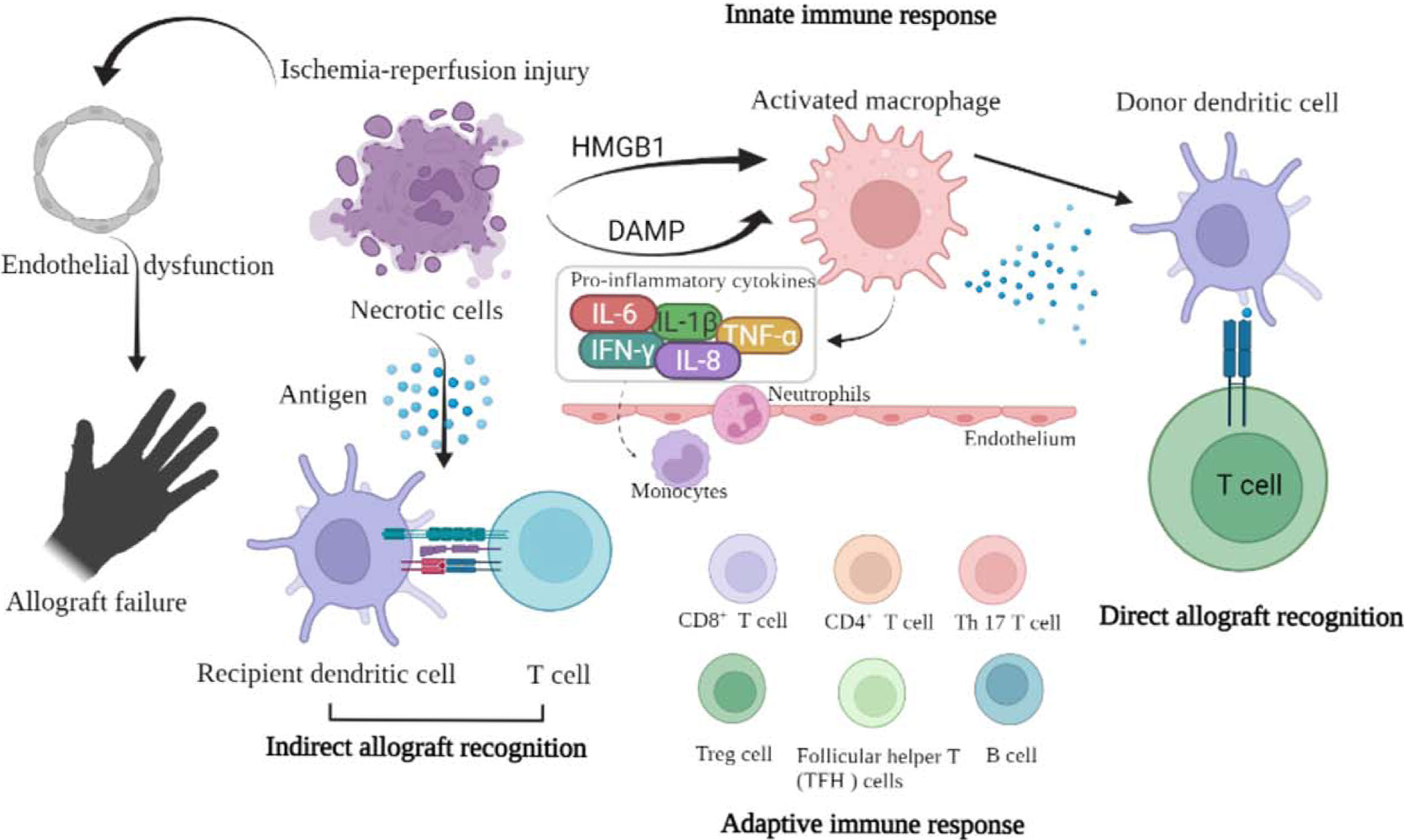
Figure 1 The schematic picture shows the relationship between ischemia-reperfusion injury and graft rejection.
Systemic reperfusion injury
Reperfusion injury may lead to systemic metabolic changes and the release of oxidized free radicals in patients, leading to cellular oxidative stress, systemic inflammatory response, multiple organ failure, and eventually death. Based on current arm replantation experiences, there is a chance of local or systemic complications, such as sepsis, remote organ failure, hyperkalemia, or acidaemia (100–104). Thus, the systemic reperfusion injury should be seriously evaluated prior to VCA surgery (105).
Strategies to reduce IRI
Over the past 20 years, a variety of drugs and interventions have been reported in clinical and basic research to alleviate IRI. Many treatment methods are based on limb IRI models, limb autograft models, and limb replantation. These interventions have shown good therapeutic effects, and the research results are worthy of reference by VCA. Table 3 summarizes the most commonly used treatments to reduce IRI, which include adenosine agonists, endothelin antagonists, antioxidants, complement activation inhibitors, apoptosis inhibitors, anti-inflammatory and proangiogenic, metabolic inhibitors, bioactive gases, traditional Chinese medicine, cell-based therapy, etc.
Despite the aforementioned therapeutic substances, the maneuver of postconditioning or remote postconditioning are effective therapies targeting IRI (142–145). Importantly, these strategies are simple, safe, and at least relatively harmless. Although the clinical trials of ischemic preconditioning or remote ischemic preconditioning have demonstrated favorable results in cardiac, hepatic, and pulmonary surgery, large, randomized, multi-center trials are required to verify the efficacy of these interventions in human skeletal muscle and skin. Recently, cutting-edge techniques have shown promising results, especially in muscle tissue preservation. The following paragraph describes these exciting methods.
Cryopreservation
Cryopreservation aims to slow the deterioration of graft tissue by reducing the rate of metabolism (146–149). This requires freezing of the graft to temperatures below 0°Cand offers the possibility of storage for many weeks. To preserve tissue viability by cryopreservation, careful control of the rate of cooling is necessary, as well as the addition of cryoprotectants to prevent intracellular ice crystal formation (150–152). Several studies have described the applicability of preserving single-cell systems, blood vessels, cutaneous tissues, bones, and nervous tissues by cryopreservation (153–155). In 2008, Rinker et al. (156) preserved rat epigastric flaps at −140°C for 2 weeks. The authors then performed isotransplantation using the flaps, which remained viable for up to 60 days, maintaining normal pigmentation and hair growth, and showing no histological signs of inflammation or necrosis. Arav et al. (157, 158) performed the first directional freezing and vitrification to preserve a syngeneic heterotopic rat hindlimb for 7 days. They demonstrated that myocytes, blood vessels, and skin layers of the hindlimb remained histologically viable 3 days after transplantation. Studies on the effects of cryopreservation on human VCA grafts are currently lacking. Although long-term VCA graft storage is possible with cryopreservation, it is still challenging to establish a standard preservation guideline because different tissues respond differently to freezing, thawing, and cryoprotectants (159).
Machine perfusion
The aim of machine perfusion is to preserve organ viability by supplying oxygen and nutrients and removing metabolic by-products (160–163). This way, grafts are preserved extracorporeally for extended periods, thereby significantly increasing their geographic accessibility (164–166). Grafts can be preserved under a variety of perfusion temperatures (167); these include hypothermic (0°C–12°C), mid-thermic (13°C–24°C), sub-normothermic (25°C–34°C), and normothermic (35°C–38°C) conditions. Studies utilizing small and large animal VCA models have shown that machine perfusion can effectively preserve transplant tissue for up to 24 hours (Table 4) (173, 187–191). Human limbs were preserved by Werner et al. (181) for 24 h using plasma-based sub-normothermic machine perfusion. After being preserved for 24 hours, the grafts were still functional and continued to respond to neuromuscular electrostimulation while exhibiting no evidence of myocyte damage.
Although recent studies have demonstrated the capability of machine perfusion in preserving graft tissue for an extended period (171, 192), some challenges still exist, such as (1) a paucity of studies utilizing allografts, (2) the absence of long-term follow-up data, and (3) lack of consensus on ideal temperature or perfusate for use in clinical settings. With the development of science and technology, machine perfusion combined with cryopreservation, CRISPR/Cas 9, stem cell therapy, siRNA, etc, to achieve in vitro editing of donor organs and modify the immunogenicity of donors, which can reduce IRI and immune rejection of the graft, and help the long-term survival of the graft (193–197).
Summary
Our retrospective review found that IRI not only causes tissue damage but also increases acute and chronic rejection events, with consistent results in organ transplantation and VCA. However, VCA contains different tissue components, and muscle is a highly metabolically active tissue that is most susceptible to reperfusion injury. The traditional static preservation method has been unable to meet clinical needs. Long-term cold ischemia causes great muscle damage, which is extremely detrimental to the functional recovery of VCA.
Advances in science and technology, such as cryopreservation technology, machine perfusion technology, etc, have significantly prolonged the preservation time of VCA. These effects are significantly better than static preservation. However, these technologies still need to be further improved, and certain consensus should be reached to standardize their clinical usage.
Future
Although VCA surgery is a life-improving, non-life-saving surgery, the ethics of surgery are still subject to academic controversy. The current focus of controversy is how to achieve a balance between patient cost and benefit. In order to improve the quality of life, patients need to take immunosuppressive drugs for a long time, and the side effects of these drugs greatly limit their clinical application. Recently, with the continuous deepening of basic research, the immune tolerance program of VCA has been successfully established in mice (198–201). But there are still many hurdles in translating it into large animals, even primates (202). IRI is one of the important factors that threaten the immune tolerance of VCA. In addition, improving the IRI could break geographic boundaries, expand the donor pool, increase organ utilization, and achieve better MHC-matching. At present, there are still few studies on IRI, and the pathophysiological mechanism of its tissue injury still needs to be further studied.
Author contributions
JH wrote the article and made the figure. UK, LQ, PW and JT proofread the manuscript. JT reviewed the article. All authors contributed to the article and approved the submitted version.
Conflict of interest
The authors declare that the research was conducted in the absence of any commercial or financial relationships that could be construed as a potential conflict of interest.
Publisher’s note
All claims expressed in this article are solely those of the authors and do not necessarily represent those of their affiliated organizations, or those of the publisher, the editors and the reviewers. Any product that may be evaluated in this article, or claim that may be made by its manufacturer, is not guaranteed or endorsed by the publisher.
References
1. Shores JT, Imbriglia JE, Lee WP. The current state of hand transplantation. J Handb Surg (2011) 36(11):1862–7. doi: 10.1016/j.jhsa.2011.09.001
2. Giannis D, Moris D, Cendales LC. Costimulation blockade in vascularized composite allotransplantation. Front Immunol (2020) 11:544186. doi: 10.3389/fimmu.2020.544186
3. Anggelia MR, Cheng HY, Lai PC, Hsieh YH, Lin CH, Lin CH. Cell therapy in vascularized composite allotransplantation. Biomed J (2022) 45(3):454–64. doi: 10.1016/j.bj.2022.01.005
4. Nakamura K, Kageyama S, Kupiec-Weglinski JW. Innate immunity in ischemia-reperfusion injury and graft rejection. Curr Opin Organ Transplant (2019) 24(6):687–93. doi: 10.1097/MOT.0000000000000709
5. Zhao H, Alam A, Soo AP, George AJT, Ma D. Ischemia-reperfusion injury reduces long term renal graft survival: Mechanism and beyond. EBioMedicine (2018) 28:31–42. doi: 10.1016/j.ebiom.2018.01.025
6. Fernandez AF, Liu Y, Ginet V, Shi M, Nah J, Zou Z, et al. Interaction between the autophagy protein beclin 1 and Na+,K+-ATPase during starvation, exercise, and ischemia. JCI Insight (2020) 5(1). doi: 10.1172/jci.insight.133282
7. Kalogeris T, Baines CP, Krenz M, Korthuis RJ. Ischemia/Reperfusion. Compr Physiol (2016) 7(1):113–70. doi: 10.1002/cphy.c160006
8. Wang R, Wang M, He S, Sun G, Sun X. Targeting calcium homeostasis in myocardial Ischemia/Reperfusion injury: An overview of regulatory mechanisms and therapeutic reagents. Front Pharmacol (2020) 11:872. doi: 10.3389/fphar.2020.00872
9. Pittas K, Vrachatis DA, Angelidis C, Tsoucala S, Giannopoulos G, Deftereos S. The role of calcium handling mechanisms in reperfusion injury. Curr Pharm design (2018) 24(34):4077–89.
10. Talaie T, DiChiacchio L, Prasad NK, Pasrija C, Julliard W, Kaczorowski DJ, et al. Ischemia-reperfusion injury in the transplanted lung: A literature review. Transplant Direct (2021) 7(2):e652. doi: 10.1097/TXD.0000000000001104
11. Pefanis A, Ierino FL, Murphy JM, Cowan PJ. Regulated necrosis in kidney ischemia-reperfusion injury. Kidney Int (2019) 96(2):291–301. doi: 10.1016/j.kint.2019.02.009
12. Philipponnet C, Aniort J, Garrouste C, Kemeny JL, Heng AE. Ischemia reperfusion injury in kidney transplantation: A case report. Med (Baltimore) (2018) 97(52):e13650. doi: 10.1097/MD.0000000000013650
13. Wu MY, Yiang GT, Liao WT, Tsai AP, Cheng YL, Cheng PW, et al. Current mechanistic concepts in ischemia and reperfusion injury. Cell Physiol Biochem (2018) 46(4):1650–67. doi: 10.1159/000489241
14. Ali M, Pham A, Wang X, Wolfram J, Pham S. Extracellular vesicles for treatment of solid organ ischemia–reperfusion injury. Am J Transplant (2020) 20(12):3294–307. doi: 10.1111/ajt.16164
15. Jimenez-Castro MB, Cornide-Petronio ME, Gracia-Sancho J, Peralta C. Inflammasome-mediated inflammation in liver ischemia-reperfusion injury. Cells-Basel (2019) 8(10):1131. doi: 10.3390/cells8101131
16. Yang W, Chen J, Meng Y, Chen Z, Yang J. Novel targets for treating ischemia-reperfusion injury in the liver. Int J Mol Sci (2018) 19(5):1302. doi: 10.3390/ijms19051302
17. Wu L, Xiong X, Wu X, Ye Y, Jian Z, Zhi Z, et al. Targeting oxidative stress and inflammation to prevent ischemia-reperfusion injury. Front Mol Neurosci (2020) 13:28. doi: 10.3389/fnmol.2020.00028
18. Cadenas S. ROS. And redox signaling in myocardial ischemia-reperfusion injury and cardioprotection. Free Radic Biol Med (2018) 117:76–89. doi: 10.1016/j.freeradbiomed.2018.01.024
19. Wang J, Zhou H. Mitochondrial quality control mechanisms as molecular targets in cardiac ischemia–reperfusion injury. Acta Pharm Sin B (2020) 10(10):1866–79. doi: 10.1016/j.apsb.2020.03.004
20. Chen Q, Younus M, Thompson J, Hu Y, Hollander JM, Lesnefsky EJ. Intermediary metabolism and fatty acid oxidation: novel targets of electron transport chain-driven injury during ischemia and reperfusion. Am J Physiol Heart Circ Physiol (2018) 314(4):H787–95. doi: 10.1152/ajpheart.00531.2017
21. Hurst S, Gonnot F, Dia M, Crola Da Silva C, Gomez L, Sheu SS. Phosphorylation of cyclophilin d at serine 191 regulates mitochondrial permeability transition pore opening and cell death after ischemia-reperfusion. Cell Death Dis (2020) 11(8):661. doi: 10.1038/s41419-020-02864-5
22. Parks RJ, Murphy E, Liu JC. Mitochondrial permeability transition pore and calcium handling. In: Mitochondrial bioenergetics. Springer (2018). p. 187–96.
23. Zhang H, Yan Q, Wang X, Chen X, Chen Y, Du J, et al. The role of mitochondria in liver ischemia-reperfusion injury: From aspects of mitochondrial oxidative stress, mitochondrial fission, mitochondrial membrane permeable transport pore formation, mitophagy, and mitochondria-related protective measures. Oxid Med Cell Longevity (2021) 2021:6670579. doi: 10.1155/2021/6670579
24. Sun T, Ding W, Xu T, Ao X, Yu T, Li M, et al. Parkin regulates programmed necrosis and myocardial Ischemia/Reperfusion injury by targeting cyclophilin-d. Antioxid Redox Signal (2019) 31(16):1177–93. doi: 10.1089/ars.2019.7734
25. Panel M, Ruiz I, Brillet R, Lafdil F, Teixeira-Clerc F, Nguyen CT, et al. Small-molecule inhibitors of cyclophilins block opening of the mitochondrial permeability transition pore and protect mice from hepatic Ischemia/Reperfusion injury. Gastroenterology (2019) 157(5):1368–82. doi: 10.1053/j.gastro.2019.07.026
26. Zhao Y, Guo Y, Chen Y, Liu S, Wu N, Jia D. Curculigoside attenuates myocardial ischemia−reperfusion injury by inhibiting the opening of the mitochondrial permeability transition pore. Int J Mol Med (2020) 45(5):1514–24.
27. Thiele JR, Zeller J, Kiefer J, Braig D, Kreuzaler S, Lenz Y, et al. A conformational change in c-reactive protein enhances leukocyte recruitment and reactive oxygen species generation in Ischemia/Reperfusion injury. Front Immunol (2018) 9:675. doi: 10.3389/fimmu.2018.00675
28. Chen W, Li D. Reactive oxygen species (ROS)-responsive nanomedicine for solving ischemia-reperfusion injury. Front Chem (2020) 8:732. doi: 10.3389/fchem.2020.00732
29. Ballestin A, Casado JG, Abellan E, Vela FJ, Alvarez V, Uson A, et al. Ischemia-reperfusion injury in a rat microvascular skin free flap model: A histological, genetic, and blood flow study. PloS One (2018) 13(12):e0209624. doi: 10.1371/journal.pone.0209624
30. Coriddi M, Myers P, Mehrara B, Nelson J, Cordeiro PG, Disa J, et al. Management of postoperative microvascular compromise and ischemia reperfusion injury in breast reconstruction using autologous tissue transfer: Retrospective review of 2103 flaps. Microsurg (2022) 42(2):109–16. doi: 10.1002/micr.30845
31. Krag AE, Hvas CL, Kiil BJ, Eschen GT, Damsgaard TE, Hvas AM. Local and systemic coagulation marker response to musculocutaneous flap ischemia–reperfusion injury and remote ischemic conditioning: An experimental study in a porcine model. Microsurg (2018) 38(6):690–7. doi: 10.1002/micr.30287
32. Serafin D, Lesesne CB, Mullen RY, Georgiade NG. Transcutaneous PO2 monitoring for assessing viability and predicting survival of skin flaps: experimental and clinical correlations. J microsur (1981) 2(3):165–78. doi: 10.1002/micr.1920020303
33. Donski PK, Franklin JD, Hurley JV, O'Brien BM. The effects of cooling on experimental free flap survival. Br J Plast Surg (1980) 33(3):353–60. doi: 10.1016/S0007-1226(80)90082-X
34. Cooley BC, Hansen FC, Dellon AL. The effect of temperature on tolerance to ischemia in experimental free flaps. J microsur (1981) 3(1):11–4. doi: 10.1002/micr.1920030105
35. Thomason PR, Matzke HA. Effects of ischemia on the hind limb of the rat. Am J Phys Med (1975) 54(3):113–31.
36. Eriksson E, Anderson WA, Replogle RL. Effects of prolonged ischemia on muscle microcirculation in the cat. Surg Forum (1974) 25(0):254–5.
37. Wagh M, Pantazi G, Romeo R, Hurley JV, Morrison WA, Knight KR. Cold storage of rat skeletal muscle free flaps and pre-ischemic perfusion with modified UW solution. Microsurg (2000) 20(7):343–9. doi: 10.1002/1098-2752(2000)20:7<343::AID-MICR6>3.0.CO;2-C
38. Kerrigan CL, Daniel RK. Critical ischemia time and the failing skin flap. Plast reconstructive Surg (1982) 69(6):986–9. doi: 10.1097/00006534-198206000-00014
39. Yokoyama H, Tsujii M, Iino T, Nakamura T, Sudo A. Inhibitory effect of edaravone on systemic inflammation and local damage in skeletal muscles following long-term ischemia to murine hind limb. J orthopaedic Surg (Hong Kong) (2019) 27(3):2309499019874470. doi: 10.1177/2309499019874470
40. Li RW, Deng Y, Pham HN, Weiss S, Chen M, Smith PN. Riluzole protects against skeletal muscle ischaemia-reperfusion injury in a porcine model. Injury (2020) 51(2):178–84. doi: 10.1016/j.injury.2019.12.030
41. Furubeppu H, Ito T, Kakuuchi M, Yasuda T, Kamikokuryo C, Yamada S, et al. Differential regulation of damage-associated molecular pattern release in a mouse model of skeletal muscle Ischemia/Reperfusion injury. Front Immunol (2021) 12:628822. doi: 10.3389/fimmu.2021.628822
42. Messner F, Thurner M, Muller J, Blumer M, Hofmann J, Marksteiner R, et al. Myogenic progenitor cell transplantation for muscle regeneration following hindlimb ischemia and reperfusion. Stem Cell Res Ther (2021) 12(1):146. doi: 10.1186/s13287-021-02208-w
43. Tu H, Zhang D, Qian J, Barksdale AN, Pipinos II, Patel KP, et al. A comparison of acute mouse hindlimb injuries between tourniquet- and femoral artery ligation-induced ischemia-reperfusion. Injury (2021) 52(11):3217–26. doi: 10.1016/j.injury.2021.09.002
44. Yang Q, He GW, Underwood MJ, Yu CM. Cellular and molecular mechanisms of endothelial ischemia/reperfusion injury: perspectives and implications for postischemic myocardial protection. Am J Trans Res (2016) 8(2):765–77.
45. Wang J, Toan S, Zhou H. New insights into the role of mitochondria in cardiac microvascular ischemia/reperfusion injury. Angiogenesis (2020) 23(3):299–314. doi: 10.1007/s10456-020-09720-2
46. Nordling S, Brannstrom J, Carlsson F, Lu B, Salvaris E, Wanders A, et al. Enhanced protection of the renal vascular endothelium improves early outcome in kidney transplantation: Preclinical investigations in pig and mouse. Sci Rep (2018) 8(1):5220. doi: 10.1038/s41598-018-21463-1
47. Hemingway HW, Moore AM, Olivencia-Yurvati AH, Romero SA. Effect of endoplasmic reticulum stress on endothelial ischemia-reperfusion injury in humans. Am J Physiol Regulatory Integr Comp Physiol (2020) 319(6):R666–72. doi: 10.1152/ajpregu.00257.2020
48. Aboo Bakkar Z, Fulford J, Gates PE, Jackman SR, Jones AM, Bond B, et al. Prolonged forearm ischemia attenuates endothelium-dependent vasodilatation and plasma nitric oxide metabolites in overweight middle-aged men. Eur J Appl Physiol (2018) 118(8):1565–72. doi: 10.1007/s00421-018-3886-z
49. Wang Y, Liu Y. Neutrophil-induced liver injury and interactions between neutrophils and liver sinusoidal endothelial cells. Inflammation (2021) 44(4):1246–62. doi: 10.1007/s10753-021-01442-x
50. Cardinal H, Dieude M, Hebert MJ. Endothelial dysfunction in kidney transplantation. Front Immunol (2018) 9:1130. doi: 10.3389/fimmu.2018.01130
51. Tran DT, Esckilsen S, Mulligan J, Mehrotra S, Atkinson C, Nadig SN. Impact of mitochondrial permeability on endothelial cell immunogenicity in transplantation. Transplantation (2018) 102(6):935–44. doi: 10.1097/TP.0000000000002163
52. Lin L, Xu H, Bishawi M, Feng F, Samy K, Truskey G, et al. Circulating mitochondria in organ donors promote allograft rejection. Am J Transplant (2019) 19(7):1917–29. doi: 10.1111/ajt.15309
53. Gan I, Jiang J, Lian D, Huang X, Fuhrmann B, Liu W, et al. Mitochondrial permeability regulates cardiac endothelial cell necroptosis and cardiac allograft rejection. Am J Transplant (2019) 19(3):686–98. doi: 10.1111/ajt.15112
54. Saray A, Can B, Akbiyik F, Askar I. Ischaemia-reperfusion injury of the peripheral nerve: An experimental study. Microsurg (1999) 19(8):374–80. doi: 10.1002/(SICI)1098-2752(1999)19:8<374::AID-MICR5>3.0.CO;2-A
55. Iida H, Schmelzer JD, Schmeichel AM, Wang Y, Low PA. Peripheral nerve ischemia: reperfusion injury and fiber regeneration. Exp Neurol (2003) 184(2):997–1002. doi: 10.1016/S0014-4886(03)00385-6
56. Shin SJ, Qi WN, Cai Y, Rizzo M, Goldner RD, Nunley JA 2nd, et al. Inhibition of inducible nitric oxide synthase promotes recovery of motor function in rats after sciatic nerve ischemia and reperfusion. J Handb Surg (2005) 30(4):826–35. doi: 10.1016/j.jhsa.2005.03.003
57. Messner F, Hautz T, Blumer MJF, Bitsche M, Pechriggl EJ, Hermann M, et al. Critical ischemia times and the effect of novel preservation solutions HTK-n and TiProtec on tissues of a vascularized tissue isograft. Transplantation (2017) 101(9):e301–10. doi: 10.1097/TP.0000000000001845
58. Ballestin A, Casado JG, Abellan E, Vela FJ, Campos JL, Martinez-Chacon G, et al. A pre-clinical rat model for the study of ischemia-reperfusion injury in reconstructive microsurgery. Jove-J Vis Exp (2019) 153:e60292. doi: 10.3791/60292
59. Rostami S, Xu M, Datta S, Haykal S. Evaluation of early markers of ischemia-reperfusion injury and preservation solutions in a modified hindlimb model of vascularized composite allotransplantation. Transplant Direct (2022) 8(1):e1251. doi: 10.1097/TXD.0000000000001251
60. Huwae TECJ, Nurandani F RATNAWATI R. Reperfusion interval effect in prevention of ischemic-reperfusion injury due to tourniquet use on fracture healing. (2020).
61. Geng Y, Chen Z, Dai L, Liu G, He X. Recurrent arterial thrombosis of the lower extremity with secondary thrombocythemia due to reperfusion injury: a case report. Ann Palliat Med (2020) 9(5):3690–7. doi: 10.21037/apm-20-1649
62. Hebbel RP, Belcher JD, Vercellotti GM. The multifaceted role of ischemia/reperfusion in sickle cell anemia. J Clin Invest (2020) 130(3):1062–72. doi: 10.1172/JCI133639
63. Ikomi F. Recompression therapy for decompression sickness and arterial gas embolism. In: Hyperbaric oxygenation therapy. Springer (2020). p. 131–52.
64. Hasenmajer V, Sbardella E, Sciarra F, Minnetti M, Isidori AM, Venneri MA. The immune system in cushing’s syndrome. Trends Endocrinol Metab (2020) 31(9):655–69. doi: 10.1016/j.tem.2020.04.004
65. Krezdorn N, Macleod F, Tasigiorgos S, Turk M, Wo L, Kiwanuka H, et al. Twenty-four–hour ex vivo perfusion with acellular solution enables successful replantation of porcine forelimbs. Plast reconstructive Surg (2019) 144(4):608e–18e. doi: 10.1097/PRS.0000000000006084
66. Ozer K. Advances in limb preservation: From replantation to transplantation. J Handb Surg (2020) 45(7):626–37.e625. doi: 10.1016/j.jhsa.2020.04.006
67. Wang Z, Zhu L, Kou W, Sun W, He B, Wang C, et al. Replantation of cryopreserved fingers: An "Organ banking" breakthrough. Plast reconstructive Surg (2019) 144(3):679–83. doi: 10.1097/PRS.0000000000005979
68. Harrant AB, Wang Z, Anderson JB, Wang Y, Li B, Johnson AC, et al. Rat hind limb transplant model to assess vascularized composite allograft (VCA) ischemic reperfusion injury and inflammation. Plast Reconstructive Surg Global Open (2020) 8(7 Suppl). doi: 10.1097/01.GOX.0000696016.14930.a8
69. Berggren A, Weiland AJ, Dorfman H. The effect of prolonged ischemia time on osteocyte and osteoblast survival in composite bone grafts revascularized by microvascular anastomoses. Plast reconstructive Surg (1982) 69(2):290–8. doi: 10.1097/00006534-198202000-00019
70. Weiss AP, Carey LA, Randolph MA, Moore JR, Weiland AJ. Oxygen radical scavengers improve vascular patency and bone-muscle cell survival in an ischemic extremity replant model. Plast reconstructive Surg (1989) 84(1):117–23. doi: 10.1097/00006534-198907000-00021
71. Hsieh AS, Winet H, Bao JY, Glas H, Plenk H. Evidence for reperfusion injury in cortical bone as a function of crush injury ischemia duration: a rabbit bone chamber study. Bone (2001) 28(1):94–103. doi: 10.1016/S8756-3282(00)00415-4
72. Tamura Y, Inoue G, Miura T, Ishiguro N, Shimizu T. Reperfusion injury in bone: effects of CV-3611, a free radical scavenger, on ischemic revascularized bone grafts in rats. J reconstructive microsur (1992) 8(6):471–9. doi: 10.1055/s-2007-1006733
73. Hu S, Kuwabara R, Navarro Chica CE, Smink AM, Koster T, Medina JD, et al. Toll-like receptor 2-modulating pectin-polymers in alginate-based microcapsules attenuate immune responses and support islet-xenograft survival. Biomaterials (2021) 266:120460. doi: 10.1016/j.biomaterials.2020.120460
74. Ito T, Naini BV, Markovic D, Aziz A, Younan S, Lu M, et al. Ischemia-reperfusion injury and its relationship with early allograft dysfunction in liver transplant patients. Am J Transplant (2021) 21(2):614–25. doi: 10.1111/ajt.16219
75. Kaabak M, Babenko N, Shapiro R, Zokoyev A, Dymova O, Kim E. A prospective randomized, controlled trial of eculizumab to prevent ischemia-reperfusion injury in pediatric kidney transplantation. Pediatr Transplant (2018) 22(2):e13129. doi: 10.1111/petr.13129
76. Pradka SP, Ong YS, Zhang Y, Davis SJ, Baccarani A, Messmer C, et al. Increased signs of acute rejection with ischemic time in a rat musculocutaneous allotransplant model. Transplant Proc (2009) 41(2):531–6. doi: 10.1016/j.transproceed.2009.01.021
77. Xiao B, Xia W, Zhang J, Liu B, Guo S. Prolonged cold ischemic time results in increased acute rejection in a rat allotransplantation model. J Surg Res (2010) 164(2):e299–304. doi: 10.1016/j.jss.2010.08.012
78. Shimizu F, Okamoto O, Katagiri K, Fujiwara S, Wei FC. Prolonged ischemia increases severity of rejection in skin flap allotransplantation in rats. Microsurg (2010) 30(2):132–7. doi: 10.1002/micr.20728
79. Villamaria CY, Rasmussen TE, Spencer JR, Patel S, Davis MR. Microvascular porcine model for the optimization of vascularized composite tissue transplantation. J Surg Res (2012) 178(1):452–9. doi: 10.1016/j.jss.2012.03.051
80. Hautz T, Hickethier T, Blumer MJ, Bitsche M, Grahammer J, Hermann M, et al. Histomorphometric evaluation of ischemia-reperfusion injury and the effect of preservation solutions histidine-tryptophan-ketoglutarate and university of Wisconsin in limb transplantation. Transplantation (2014) 98(7):713–20. doi: 10.1097/TP.0000000000000300
81. Bonastre J, Landin L, Bolado P, Casado-Sanchez C, Lopez-Collazo E, Diez J. Effect of cold preservation on chronic rejection in a rat hindlimb transplantation model. Plast reconstructive Surg (2016) 138(3):628–37. doi: 10.1097/PRS.0000000000002461
82. Datta N, Devaney SG, Busuttil RW, Azari K, Kupiec-Weglinski JW. Prolonged cold ischemia time results in local and remote organ dysfunction in a murine model of vascularized composite transplantation. Am J Transplant (2017) 17(10):2572–9. doi: 10.1111/ajt.14290
83. Fries CA, Villamaria CY, Spencer JR, Rasmussen TE, Davis MR. C1 esterase inhibitor ameliorates ischemia reperfusion injury in a swine musculocutaneous flap model. Microsurg (2017) 37(2):142–7. doi: 10.1002/micr.30053
84. Robbins N, wordsworth Mj, Sippel MR, Parida BK, Gorantla VS, Breidenbach WC, et al. Prevention of ischemia-reperfusion injury and chronic rejection in a porcine vascularized composite allotransplantation model. Transplantation (2018) 102(Supplement 7):S706. doi: 10.1097/01.tp.0000543670.93080.cc
85. Gok E, Kubiak CA, Guy E, Kemp SWP, Ozer K. Effect of static cold storage on skeletal muscle after vascularized composite tissue allotransplantation. J reconstructive microsur (2020) 36(1):9–15. doi: 10.1055/s-0039-1693455
86. Wijayaratna SB, Suraweera HJ, Lamawansa MD, Mudalige SP, Esufali ST, Goonasekera CD. Post-operative critical care and outcomes of limb replantation: experience in a developing country. Injury (2008) 39(2):203–8. doi: 10.1016/j.injury.2007.09.009
87. Laing TA, Cassell O, O'Donovan D, Eadie P. Long term functional results from major limb replantations. J plastic reconstructive aesthetic Surg (2012) 65(7):931–4. doi: 10.1016/j.bjps.2012.01.012
88. Tantry TP, Kadam D, Shenoy SP, Bhandary S, Adappa KK. Perioperative evaluation and outcomes of major limb replantations with ischemia periods of more than 6 hours. J reconstructive microsur (2013) 29(3):165–72. doi: 10.1055/s-0032-1331143
89. Pet MA, Ko JH. Indications for replantation and revascularization in the hand. Handb Clinics (2019) 35(2):119–30. doi: 10.1016/j.hcl.2018.12.003
90. Pradka S, Ong Y, Zhang Y, Davis S, Baccarani A, Messmer C, et al. Increased signs of acute rejection with ischemic time in a rat musculocutaneous allotransplant model. In: Transplantation proceedings, vol. 2009. Elsevier (2009). p. 531–6.
91. Atkinson C, Lei B, Sleiman MM, Cheng Q, Tu Z, Zhu P, et al. In-situ pre-treatment of vascularized composite allografts with a targeted complement inhibitor protects against brain death and ischemia reperfusion induced injuries. Front Immunol (2021) 12:2583.
92. Zhou J, Chen J, Wei Q, Saeb-Parsy K, Xu X. The role of Ischemia/Reperfusion injury in early hepatic allograft dysfunction. Liver Transpl (2020) 26(8):1034–48. doi: 10.1002/lt.25779
93. Zhang M, Zhang M, Wang L, Yu T, Jiang S, Jiang P, et al. Activation of cannabinoid type 2 receptor protects skeletal muscle from ischemia-reperfusion injury partly via Nrf2 signaling. Life Sci (2019) 230:55–67. doi: 10.1016/j.lfs.2019.05.056
94. Kuroda Y, Togashi H, Uchida T, Haga K, Yamashita A, Sadahiro M. Oxidative stress evaluation of skeletal muscle in ischemia–reperfusion injury using enhanced magnetic resonance imaging. Sci Rep (2020) 10(1):1–9. doi: 10.1038/s41598-020-67336-4
95. Pottecher J, Adamopoulos C, Lejay A, Bouitbir J, Charles AL, Meyer A, et al. Diabetes worsens skeletal muscle mitochondrial function, oxidative stress, and apoptosis after lower-limb ischemia-reperfusion: Implication of the RISK and SAFE pathways? Front Physiol (2018) 9:579. doi: 10.3389/fphys.2018.00579
96. Lundborg G. Ischemic nerve injury. experimental studies on intraneural microvascular pathophysiology and nerve function in a limb subjected to temporary circulatory arrest. Scandinavian J Plast reconstructive Surg Supplementum (1970) 6:3–113.
97. Fernández AR, Sánchez-Tarjuelo R, Cravedi P, Ochando J, López-Hoyos M. Ischemia reperfusion injury–a translational perspective in organ transplantation. Int J Mol Sci (2020) 21(22):8549.
98. Nieuwenhuijs-Moeke GJ, Pischke SE, Berger SP, Sanders JSF, Pol RA, Struys M, et al. Ischemia and reperfusion injury in kidney transplantation: Relevant mechanisms in injury and repair. J Clin Med (2020) 9(1):253. doi: 10.3390/jcm9010253
99. Patel PM, Connolly MR, Coe TM, Calhoun A, Pollok F, Markmann JF, et al. Minimizing ischemia reperfusion injury in xenotransplantation. Front Immunol (2021) 12:681504. doi: 10.3389/fimmu.2021.681504
100. McCutcheon C, Hennessy B. Systemic reperfusion injury during arm replantation requiring intraoperative amputation. Anaesthesia Intensive Care (2002) 30(1):71–3. doi: 10.1177/0310057X0203000113
101. Yassin MM, Harkin DW, Barros D'Sa AA, Halliday MI, Rowlands BJ. Lower limb ischemia-reperfusion injury triggers a systemic inflammatory response and multiple organ dysfunction. World J Surg (2002) 26(1):115–21. doi: 10.1007/s00268-001-0169-2
102. Steinau H-U. Major limb replantation and postischemia syndrome: investigation of acute ischemia-induced myopathy and reperfusion injury. Springer Science & Business Media (2013).
103. Leclere FM, Mathys L, Juon B, Franz T, Unglaub F, Vogelin E. Macroreplantations of the upper extremity: a series of 11 patients. Arch orthopaedic Trauma Surg (2012) 132(12):1797–805. doi: 10.1007/s00402-012-1590-8
104. Kovačević P, Kozarski J, Đorđević D, Janković I, Pavlović D. Upper limb replantation: Surgical strategy and the prophylaxis of acute renal failure in ischemia reperfusion injury: A report of two cases. Vojnosanitetski pregled (2021) 00:120–0.
105. Koul AR, Cyriac A, Khaleel VM, Vinodan K. Bilateral high upper limb replantation in a child. Plast reconstructive Surg (2004) 113(6):1734–8 discussion 1739-1741. doi: 10.1097/01.PRS.0000117369.43393.98
106. Rowlands TE, Gough MJ, Homer-Vanniasinkam S. Do prostaglandins have a salutary role in skeletal muscle ischaemia-reperfusion injury? Eur J Vasc endovascular Surg (1999) 18(5):439–44. doi: 10.1053/ejvs.1999.0929
107. Luyt CE, Lepailleur-Enouf D, Gaultier CJ, Valdenaire O, Steg G, Michel JB. Involvement of the endothelin system in experimental critical hind limb ischemia. Mol Med (2000) 6(11):947–56. doi: 10.1007/BF03401829
108. Herbert KJ, Hickey MJ, Lepore DA, Knight KR, Morrison WA, Stewart AG. Effects of the endothelin receptor antagonist bosentan on ischaemia/reperfusion injury in rat skeletal muscle. Eur J Pharmacol (2001) 424(1):59–67. doi: 10.1016/S0014-2999(01)01133-5
109. Frassdorf J, Luther B, Mullenheim J, Otto F, Preckel B, Schlack W, et al. Influence of groin incision, duration of ischemia, and prostaglandin E1 on ischemia-reperfusion injury of the lower limb. J cardiothoracic Vasc Anesth (2006) 20(2):187–95. doi: 10.1053/j.jvca.2005.11.001
110. Zheng J, Wang R, Zambraski E, Wu D, Jacobson KA, Liang BT. Protective roles of adenosine A1, A2A, and A3 receptors in skeletal muscle ischemia and reperfusion injury. Am J Physiol Heart Circ Physiol (2007) 293(6):H3685–3691. doi: 10.1152/ajpheart.00819.2007
111. Duehrkop C, Banz Y, Spirig R, Miescher S, Nolte MW, Spycher M, et al. C1 esterase inhibitor reduces lower extremity ischemia/reperfusion injury and associated lung damage. PloS One (2013) 8(8):e72059. doi: 10.1371/journal.pone.0072059
112. Zhang S, Shaw-Boden J, Banz Y, Bongoni AK, Taddeo A, Spirig R, et al. Effects of C1 inhibitor on endothelial cell activation in a rat hind limb ischemia-reperfusion injury model. J Vasc Surg (2018) 68(6S):209S–221S.e202. doi: 10.1016/j.jvs.2017.10.072
113. Masa I, Casado-Sanchez C, Crespo-Lora V, Ballestin A. Effects of ischemic preconditioning and C1 esterase inhibitor administration following ischemia-reperfusion injury in a rat skin flap model. J reconstructive microsur (2021) 37(3):242–8. doi: 10.1055/s-0040-1717102
114. Bolcal C, Yildirim V, Doganci S, Sargin M, Aydin A, Eken A, et al. Protective effects of antioxidant medications on limb ischemia reperfusion injury. J Surg Res (2007) 139(2):274–9. doi: 10.1016/j.jss.2006.10.043
115. Medling BD, Bueno R, Chambers C, Neumeister MW. The effect of vitamin e succinate on ischemia reperfusion injury. Handb (New York NY) (2010) 5(1):60–4. doi: 10.1007/s11552-009-9196-5
116. Avci G, Kadioglu H, Sehirli AO, Bozkurt S, Guclu O, Arslan E, et al. Curcumin protects against ischemia/reperfusion injury in rat skeletal muscle. J Surg Res (2012) 172(1):e39–46. doi: 10.1016/j.jss.2011.08.021
117. Muneuchi G, Hossain A, Yamaguchi F, Ueno M, Tanaka Y, Suzuki S, et al. The rare sugar d-allose has a reducing effect against ischemia-reperfusion injury on the rat abdominal skin island flap model. J Surg Res (2013) 183(2):976–81. doi: 10.1016/j.jss.2013.03.006
118. Dong X, Xing Q, Li Y, Han X, Sun L. Dexmedetomidine protects against ischemia-reperfusion injury in rat skeletal muscle. J Surg Res (2014) 186(1):240–5. doi: 10.1016/j.jss.2013.07.052
119. Yin Z, Ren H, Liu L, Chen W, Gan C, Jiao H, et al. Thioredoxin protects skin flaps from ischemia-reperfusion injury: A novel prognostic and therapeutic target. Plast reconstructive Surg (2016) 137(2):511–21. doi: 10.1097/01.prs.0000475768.68654.03
120. Seyid M, Tiftikcioglu Y, Erdem M, Akdemir O, Tatar BE, Uyanikgil Y, et al. The effect of ceruloplasmin against ischemia-reperfusion injury in epigastric island flap in rats. J Surg Res (2021) 267:627–35. doi: 10.1016/j.jss.2021.05.045
121. Song K, Zhang M, Hu J, Liu Y, Liu Y, Wang Y, et al. Methane-rich saline attenuates ischemia/reperfusion injury of abdominal skin flaps in rats via regulating apoptosis level. BMC Surg (2015) 15:92. doi: 10.1186/s12893-015-0075-4
122. Cheng Y, Di S, Fan C, Cai L, Gao C, Jiang P, et al. SIRT1 activation by pterostilbene attenuates the skeletal muscle oxidative stress injury and mitochondrial dysfunction induced by ischemia reperfusion injury. Apoptosis an Int J programmed Cell Death (2016) 21(8):905–16. doi: 10.1007/s10495-016-1258-x
123. Xin D, Quan R, Zeng L, Xu C, Tang Y. Lipoxin A4 protects rat skin flaps against ischemia-reperfusion injury through inhibiting cell apoptosis and inflammatory response induced by endoplasmic reticulum stress. Ann Trans Med (2020) 8(17):1086. doi: 10.21037/atm-20-5549
124. Zhang EW, Fang T, Arnold PB, Songcharoen SJ, Lineaweaver WC, Zhang F. The effect of activated protein c on attenuation of ischemia-reperfusion injury in a rat muscle flap model. Ann Plast Surg (2015) 75(4):448–54. doi: 10.1097/SAP.0000000000000118
125. Rah DK, Min HJ, Kim YW, Cheon YW. Effect of platelet-rich plasma on ischemia-reperfusion injury in a skin flap mouse model. Int J Med Sci (2017) 14(9):829–39. doi: 10.7150/ijms.19573
126. Nam SY, Shin BH, Lee M, Lee S, Heo CY. NecroX-5 ameliorates inflammation by skewing macrophages to the M2 phenotype. Int Immunopharmacol (2019) 66:139–45.
127. Henderson PW, Weinstein AL, Sung J, Singh SP, Nagineni V, Spector JA. Hydrogen sulfide attenuates ischemia-reperfusion injury in in vitro and in vivo models of intestine free tissue transfer. Plast reconstructive Surg (2010) 125(6):1670–8.
128. Zhao G, Zhang X, Xu P, Mi JY, Rui YJ. The protective effect of irisin against ischemia-reperfusion injury after perforator flap grafting in rats. Injury (2018) 49(12):2147–53.
129. Chen G, Shen H, Zang L, Su Z, Huang J, Sun Y, et al. Protective effect of luteolin on skin ischemia-reperfusion injury through an AKT-dependent mechanism. Int J Mol Med (2018) 42(6):3073–82.
130. Ren H, Meng X, Yin J, Sun J, Huang Q, Yin Z. Ganoderma lucidum polysaccharide peptide attenuates skin flap ischemia-reperfusion injury in a thioredoxin-dependent manner. Plast reconstructive Surg (2018) 142(1):23e–33e.
131. Xiang Y, Ye S, Cai C, Chen J, Zhao X, Zhu N, et al. Salvianolic acid a attenuates limb ischemia/reperfusion injury in skeletal muscle of rats. Biomedicine pharmacother = Biomedecine pharmacotherapie (2018) 97:551–6. doi: 10.1016/j.biopha.2017.10.094
132. Zhao Y, Liu X, Fu X, Mo Z, Jiang Y, Yan Y. Protective effects of epigallocatechin gallate against ischemia reperfusion injury in rat skeletal muscle via activating Nrf2/HO-1 signaling pathway. Life Sci (2019) 239:117014. doi: 10.1016/j.lfs.2019.117014
133. Hong JP, Kwon H, Chung YK, Jung SH. The effect of hyperbaric oxygen on ischemia-reperfusion injury: an experimental study in a rat musculocutaneous flap. Ann Plast Surg (2003) 51(5):478–87. doi: 10.1097/01.sap.0000095651.05156.0f
134. Bihari A, Cepinskas G, Forbes TL, Potter RF, Lawendy AR. Systemic application of carbon monoxide-releasing molecule 3 protects skeletal muscle from ischemia-reperfusion injury. J Vasc Surg (2017) 66(6):1864–71. doi: 10.1016/j.jvs.2016.11.065
135. Elsurer C, Onal M, Selimoglu N, Erdur O, Yilmaz M, Erdogan E, et al. Postconditioning ozone alleviates ischemia-reperfusion injury and enhances flap endurance in rats. J Invest Surg (2020) 33:15–24. doi: 10.1080/08941939.2018.1473901
136. Cui H, Feng Y, Shu C, Yuan R, Bu L, Jia M, et al. Dietary nitrate protects against skin flap ischemia-reperfusion injury in rats via modulation of antioxidative action and reduction of inflammatory responses. Front Pharmacol (2019) 10:1605. doi: 10.3389/fphar.2019.01605
137. Tong J, Zhang Y, Yu P, Liu J, Mei X, Meng J. Protective effect of hydrogen gas on mouse hind limb ischemia-reperfusion injury. J Surg Res (2021) 266:148–59. doi: 10.1016/j.jss.2021.03.046
138. Hammers DW, Rybalko V, Merscham-Banda M, Hsieh PL, Suggs LJ, Farrar RP. Anti-inflammatory macrophages improve skeletal muscle recovery from ischemia-reperfusion. J Appl Physiol (Bethesda Md 1985) (2015) 118(8):1067–74. doi: 10.1152/japplphysiol.00313.2014
139. Ballestin A, Casado JG, Abellan E, Vela FJ, Alvarez V, Uson A, et al. Adipose-derived stem cells ameliorate ischemia-reperfusion injury in a rat skin free flap model. J reconstructive microsur (2018) 34(8):601–9.
140. Bai Y, Han YD, Yan XL, Ren J, Zeng Q, Li XD, et al. Adipose mesenchymal stem cell-derived exosomes stimulated by hydrogen peroxide enhanced skin flap recovery in ischemia-reperfusion injury. Biochem Biophys Res Commun (2018) 500(2):310–7. doi: 10.1016/j.bbrc.2018.04.065
141. Orfany A, Arriola CG, Doulamis IP, Guariento A, Ramirez-Barbieri G, Moskowitzova K, et al. Mitochondrial transplantation ameliorates acute limb ischemia. J Vasc Surg (2020) 71(3):1014–26. doi: 10.1016/j.jvs.2019.03.079
142. Landman T, Schoon Y, Warle M, De Leeuw FE, Thijssen D. The effect of repeated remote ischemic postconditioning on infarct size in patients with an ischemic stroke (REPOST): study protocol for a randomized clinical trial. Trials (2019) 20(1):167. doi: 10.1186/s13063-019-3264-0
143. Yasojima EY, Domingues RJS, Silva RC, Sousa LFF, Trindade Junior SC. Comparison of remote and local postconditioning against hepatic ischemic-reperfusion injury in rats. Acta Cir Bras (2021) 36(1):e360101. doi: 10.1590/acb360101
144. Chen G, Thakkar M, Robinson C, Dore S. Limb remote ischemic conditioning: Mechanisms, anesthetics, and the potential for expanding therapeutic options. Front Neurol (2018) 9:40. doi: 10.3389/fneur.2018.00040
145. Zhao JJ, Xiao H, Zhao WB, Zhang XP, Xiang Y, Ye ZJ, et al. Remote ischemic postconditioning for ischemic stroke: A systematic review and meta-analysis of randomized controlled trials. Chin Med J (Engl) (2018) 131(8):956–65. doi: 10.4103/0366-6999.229892
146. He B, Su S, Yuan G, Duan J, Zhu Z, Wang Z. Clinical guideline for vascularized composite tissue cryopreservation. J Tissue Eng Regener Med (2021) 15(6):527–33. doi: 10.1002/term.3190
147. Tian Y, Li N, Wang W, Li N. Application of cryopreservation technique in the preservation of rat limbs. In: Transplantation proceedings, vol. 2021. Elsevier (2021). p. 2816–9.
148. He B, Su S, Lu Y, Wen X, Duan J, Liu X, et al. Effects of cryopreservation and replantation on muscles: Application scope of limb cryopreservation. Ann Plast Surg (2020) 84(5S Suppl 3):S208–14. doi: 10.1097/SAP.0000000000002366
149. Finger EB, Bischof JC. Cryopreservation by vitrification: a promising approach for transplant organ banking. Curr Opin Organ Transplant (2018) 23(3):353–60. doi: 10.1097/MOT.0000000000000534
150. Chang T, Zhao G. Ice inhibition for cryopreservation: Materials, strategies, and challenges. Adv Sci (Weinh) (2021) 8(6):2002425. doi: 10.1002/advs.202002425
151. Chang T, Moses OA, Tian C, Wang H, Song L, Zhao G. Synergistic ice inhibition effect enhances rapid freezing cryopreservation with low concentration of cryoprotectants. Adv Sci (Weinh) (2021) 8(6):2003387. doi: 10.1002/advs.202003387
152. Fahy GM, Wowk B. Principles of ice-free cryopreservation by vitrification. In: Cryopreservation and freeze-drying protocols. Springer (2021). p. 27–97.
153. Taylor MJ, Weegman BP, Baicu SC, Giwa SE. New approaches to cryopreservation of cells, tissues, and organs. Transfus Med Hemother (2019) 46(3):197–215. doi: 10.1159/000499453
154. Gal S, Pu LLQ. An update on cryopreservation of adipose tissue. Plast reconstructive Surg (2020) 145(4):1089–97. doi: 10.1097/PRS.0000000000006699
155. Bojic S, Murray A, Bentley BL, Spindler R, Pawlik P, Cordeiro JL, et al. Winter is coming: the future of cryopreservation. BMC Biol (2021) 19(1):1–20. doi: 10.1186/s12915-021-00976-8
156. Rinker B, Cui XD, Cibull ML, Fink BF, Gao DY, Vasconez HC. Cryopreservation of composite tissue transplants. Handb (New York NY) (2008) 3(1):17–23. doi: 10.1007/s11552-007-9062-2
157. Arav A, Friedman O, Natan Y, Gur E, Shani N. Rat hindlimb cryopreservation and transplantation: A step toward "Organ banking". Am J Transplant (2017) 17(11):2820–8. doi: 10.1111/ajt.14320
158. Shani N, Friedman O, Arav A, Natan Y, Gur E. Cryopreservation and transplantation of vascularized composite transplants: Unique challenges and opportunities. Plast reconstructive Surg (2019) 143(5):1074e–80e. doi: 10.1097/PRS.0000000000005541
159. Jungare KA, Radha R, Sreekanth D. Cryopreservation of biological samples–a short review. In: Materials today: Proceedings (2021).
160. Ceresa CDL, Nasralla D, Pollok JM, Friend PJ. Machine perfusion of the liver: applications in transplantation and beyond. Nat Rev Gastroenterol Hepatol (2022) 19(3):199–209. doi: 10.1038/s41575-021-00557-8
161. Pavicevic S, Uluk D, Reichelt S, Fikatas P, Globke B, Raschzok N, et al. Hypothermic oxygenated machine perfusion for extended criteria donor allografts: Preliminary experience with extended organ preservation times in the setting of organ reallocation. Artif Organs (2022) 46(2):306–11. doi: 10.1111/aor.14103
162. Jochmans I, Brat A, Davies L, Hofker HS, van de Leemkolk FEM, Leuvenink HGD, et al. Oxygenated versus standard cold perfusion preservation in kidney transplantation (COMPARE): a randomised, double-blind, paired, phase 3 trial. Lancet (2020) 396(10263):1653–62. doi: 10.1016/S0140-6736(20)32411-9
163. Husen P, Boffa C, Jochmans I, Krikke C, Davies L, Mazilescu L, et al. Oxygenated end-hypothermic machine perfusion in expanded criteria donor kidney transplant: A randomized clinical trial. JAMA Surg (2021) 156(6):517–25. doi: 10.1001/jamasurg.2021.0949
164. Fodor M, Cardini B, Peter W, Weissenbacher A, Oberhuber R, Hautz T, et al. Static cold storage compared with normothermic machine perfusion of the liver and effect on ischaemic-type biliary lesions after transplantation: a propensity score-matched study. Br J Surg (2021) 108(9):1082–9. doi: 10.1093/bjs/znab118
165. Amin KR, Stone JP, Kerr J, Geraghty A, Joseph L, Montero-Fernandez A, et al. Randomized preclinical study of machine perfusion in vascularized composite allografts. Br J Surg (2021) 108(5):574–82.
166. Raigani S, De Vries RJ, Uygun K, Yeh H. Pumping new life into old ideas: Preservation and rehabilitation of the liver using ex situ machine perfusion. Artif Organs (2020) 44(2):123–8. doi: 10.1111/aor.13579
167. Bonaccorsi-Riani E, Brüggenwirth I, Buchwald J, Iesari S, Martins P. Machine perfusion: cold versus warm, versus neither. update on clinical trials. In: Seminars in liver disease, vol. 2020. Thieme Medical Publishers (2020). p. 264–81.
168. Rezaei M, Ordenana C, Figueroa BA, Said SA, Fahradyan V, Dalla Pozza E, et al. Ex vivo normothermic perfusion of human upper limbs. Transplantation (2022) 106(8):1638–46. doi: 10.1097/TP.0000000000004045
169. Goutard M, de Vries RJ, Tawa P, Pendexter CA, Rosales IA, Tessier SN, et al. Exceeding the limits of static cold storage in limb transplantation using subnormothermic machine perfusion. J reconstructive microsur (2022). doi: 10.1055/a-1886-5697
170. Figueroa BA, Said SA, Ordenana C, Rezaei M, Orfahli LM, Dube GP, et al. Ex vivo normothermic preservation of amputated limbs with a hemoglobin-based oxygen carrier perfusate. J Trauma acute Care Surg (2022) 92(2):388–97. doi: 10.1097/TA.0000000000003395
171. Burlage LC, Lellouch AG, Taveau CB, Tratnig-Frankl P, Pendexter CA, Randolph MA, et al. Optimization of ex vivo machine perfusion and transplantation of vascularized composite allografts. J Surg Res (2022) 270:151–61. doi: 10.1016/j.jss.2021.09.005
172. Kruit AS, Brouwers K, van Midden D, Zegers H, Koers E, van Alfen N, et al. Successful 18-h acellular extracorporeal perfusion and replantation of porcine limbs - histology versus nerve stimulation. Transpl Int (2021) 34(2):365–75. doi: 10.1111/tri.13802
173. Amin KR, Stone JP, Kerr JC, Wong JK, Fildes JE. Normothermic ex vivo perfusion of the limb allograft depletes donor leukocytes prior to transplantation. J plastic reconstructive aesthetic Surg JPRAS (2021) 74(11):2969–76. doi: 10.1016/j.bjps.2021.03.071
174. Said SA, Ordenana CX, Rezaei M, Figueroa BA, Dasarathy S, Brunengraber H, et al. Ex-vivo normothermic limb perfusion with a hemoglobin-based oxygen carrier perfusate. Military Med (2020) 185(Suppl 1):110–20. doi: 10.1093/milmed/usz314
175. Haug V, Kollar B, Tasigiorgos S, Endo Y, Kauke M, Safi AF, et al. Hypothermic ex situ perfusion of human limbs with acellular solution for 24 hours. Transplantation (2020) 104(9):e260–70. doi: 10.1097/TP.0000000000003221
176. Haug V, Kollar B, Endo Y, Kadakia N, Veeramani A, Kauke M, et al. Comparison of acellular solutions for ex-situ perfusion of amputated limbs. In: Military medicine, vol. 185. (2020). p. e2004–12.
177. Fahradyan V, Said SA, Ordenana C, Dalla Pozza E, Frautschi R, Duraes EFR, et al. Extended ex vivo normothermic perfusion for preservation of vascularized composite allografts. Artif Organs (2020) 44(8):846–55.
178. Krezdorn N, Macleod F, Tasigiorgos S, Turk MDM, Wo L, Kiwanuka BAH, et al. Twenty-Four-Hour ex vivo perfusion with acellular solution enables successful replantation of porcine forelimbs. Plast reconstructive Surg (2019) 144(4):608e–18e.
179. Gok E, Alghanem F, Moon R, Guy E, Rojas-Pena A, Bartlett RH, et al. Development of an ex-situ limb perfusion system for a rodent model. ASAIO J (American Soc Artif Internal Organs 1992) (2019) 65(2):167–72.
180. Krezdorn N, Sakthivel D, Turk M, Aycart MA, Tasigiorgos S, Bueno EM, et al. Reduced hypoxia-related genes in porcine limbs in ex vivo hypothermic perfusion versus cold storage. J Surg Res (2018) 232:137–45.
181. Werner NL, Alghanem F, Rakestraw SL, Sarver DC, Nicely B, Pietroski RE, et al. Ex situ perfusion of human limb allografts for 24 hours. Transplantation (2017) 101(3):e68–74.
182. Kueckelhaus M, Dermietzel A, Alhefzi M, Aycart MA, Fischer S, Krezdorn N, et al. Acellular hypothermic extracorporeal perfusion extends allowable ischemia time in a porcine whole limb replantation model. Plast reconstructive Surg (2017) 139(4):922e–32e.
183. Duraes EFR, Madajka M, Frautschi R, Soliman B, Cakmakoglu C, Barnett A, et al. Developing a protocol for normothermic ex-situ limb perfusion. Microsurg (2018) 38(2):185–94.
184. Ozer K, Rojas-Pena A, Mendias CL, Bryner BS, Toomasian C, Bartlett RH. The effect of ex situ perfusion in a swine limb vascularized composite tissue allograft on survival up to 24 hours. J Handb Surg (2016) 41(1):3–12.
185. Ozer K, Rojas-Pena A, Mendias CL, Bryner B, Toomasian C, Bartlett RH. Ex situ limb perfusion system to extend vascularized composite tissue allograft survival in swine. Transplantation (2015) 99(10):2095–101.
186. Araki J, Sakai H, Takeuchi D, Kagaya Y, Tashiro K, Naito M, et al. Normothermic preservation of the rat hind limb with artificial oxygen-carrying hemoglobin vesicles. Transplantation (2015) 99(4):687–92.
187. Matar AJ, Crepeau RL, Mundinger GS, Cetrulo CL Jr, Torabi R. Large Animal models of vascularized composite allotransplantation: A review of immune strategies to improve allograft outcomes. Front Immunol (2021) 12:664577. doi: 10.3389/fimmu.2021.664577
188. Kueckelhaus M, Puscz F, Dermietzel A, Dadras M, Fischer S, Krezdorn N, et al. Extracorporeal perfusion in vascularized composite allotransplantation: Current concepts and future prospects. Ann Plast Surg (2018) 80(6):669–78. doi: 10.1097/SAP.0000000000001477
189. Rezaei M, Figueroa B, Orfahli LM, Ordenana C, Brunengraber H, Dasarathy S, et al. Composite vascularized allograft machine preservation: State of the art. Curr Transplant Rep (2019) 6(4):265–76. doi: 10.1007/s40472-019-00263-0
190. Gok E, Kubiak CA, Guy E, Ponder M, Hoenerhoff MJ, Rojas-Pena A, et al. Long-term effects of hypothermic ex situ perfusion on skeletal muscle metabolism, structure, and force generation after transplantation. Transplantation (2019) 103(10):2105–12. doi: 10.1097/TP.0000000000002800
191. Kaltenborn A, Krezdorn N, Hoffmann S, Gutcke A, Haastert-Talini K, Vogt PM, et al. Ex vivo limb perfusion for traumatic amputation in military medicine. Mil Med Res (2020) 7(1):21. doi: 10.1186/s40779-020-00250-y
192. Tingle SJ, Figueiredo RS, Moir JA, Goodfellow M, Talbot D, Wilson CH. Machine perfusion preservation versus static cold storage for deceased donor kidney transplantation. Cochrane Database Syst Rev (2019) 3(3):CD011671. doi: 10.1002/14651858.CD011671.pub2
193. Wang Y, Wang S, Gu C, Xiong Y, Shen H, Liu F, et al. Ex-vivo treatment of allografts using adipose-derived stem cells induced prolonged rejection-free survival in an allogenic hind-limb transplantation model. Ann Trans Med (2020) 8(14):867. doi: 10.21037/atm.2019.12.141
194. Hosgood SA, Hoff M, Nicholson ML. Treatment of transplant kidneys during machine perfusion. Transpl Int (2021) 34(2):224–32. doi: 10.1111/tri.13751
195. Cao H, Wu L, Tian X, Zheng W, Yuan M, Li X, et al. HO-1/BMMSC perfusion using a normothermic machine perfusion system reduces the acute rejection of DCD liver transplantation by regulating NKT cell co-inhibitory receptors in rats. Stem Cell Res Ther (2021) 12(1):587. doi: 10.21203/rs.3.rs-380793/v1
196. Carlson K, Barbas A, Goldaracena N, Fernandez L, Al-Adra DP. Immunological organ modification during ex vivo machine perfusion: The future of organ acceptance. Transplant Rev (Orlando) (2021) 35(2):100586. doi: 10.1016/j.trre.2020.100586
197. Samoylova ML, Nash A, Kuchibhatla M, Barbas AS, Brennan TV. Machine perfusion of donor kidneys may reduce graft rejection. Clin Transplant (2019) 33(10):e13716.
198. Anggelia MR, Cheng HY, Chuang WY, Hsieh YH, Wang AYL, Lin CH, et al. Unraveling the crucial roles of FoxP3+ regulatory T cells in vascularized composite allograft tolerance induction and maintenance. Transplantation (2021) 105(6):1238–49.
199. Fisher JD, Zhang W, Balmert SC, Aral AM, Acharya AP, Kulahci Y, et al. In situ recruitment of regulatory T cells promotes donor-specific tolerance in vascularized composite allotransplantation. Sci Adv (2020) 6(11):eaax8429.
200. Lin CH, Anggelia MR, Cheng HY, Wang AYL, Chuang WY, Lin CH, et al. The intragraft vascularized bone marrow component plays a critical role in tolerance induction after reconstructive transplantation. Cell Mol Immunol (2021) 18(2):363–73.
201. Oh BC, Furtmuller GJ, Fryer ML, Guo Y, Messner F, Krapf J, et al. Vascularized composite allotransplantation combined with costimulation blockade induces mixed chimerism and reveals intrinsic tolerogenic potential. JCI Insight (2020) 5(7).
Keywords: ischemia-reperfusion injury (IRI), vascularized composite allotransplantation (VCA), tissue damage, transplant rejection, innate immunity, adaptive immunity
Citation: He J, Khan UZ, Qing L, Wu P and Tang J (2022) Improving the ischemia-reperfusion injury in vascularized composite allotransplantation: Clinical experience and experimental implications. Front. Immunol. 13:998952. doi: 10.3389/fimmu.2022.998952
Received: 20 July 2022; Accepted: 29 August 2022;
Published: 16 September 2022.
Edited by:
Lin Zhong, Shanghai General Hospital, ChinaReviewed by:
Maximilian Kueckelhaus, University Hospital Münster, GermanyJun Chen, Hubei University of Medicine, China
Copyright © 2022 He, Khan, Qing, Wu and Tang. This is an open-access article distributed under the terms of the Creative Commons Attribution License (CC BY). The use, distribution or reproduction in other forums is permitted, provided the original author(s) and the copyright owner(s) are credited and that the original publication in this journal is cited, in accordance with accepted academic practice. No use, distribution or reproduction is permitted which does not comply with these terms.
*Correspondence: Juyu Tang, dGFuZ2p1eXVAY3N1LmVkdS5jbg==