- 1Center for Immunity and Inflammation, New Jersey Medical School, Rutgers-The State University of New Jersey, Newark, NJ, United States
- 2Department of Medicine, New Jersey Medical School, Rutgers-The State University of New Jersey, Newark, NJ, United States
Parasitic helminth infections remain a significant global health issue and are responsible for devastating morbidity and economic hardships. During infection, helminths migrate through different host organs, which results in substantial tissue damage and the release of diverse effector molecules by both hematopoietic and non-hematopoietic cells. Thus, host protective responses to helminths must initiate mechanisms that help to promote worm clearance while simultaneously mitigating tissue injury. The specialized immunity that promotes these responses is termed type 2 inflammation and is initiated by the recruitment and activation of hematopoietic stem/progenitor cells, mast cells, basophils, eosinophils, dendritic cells, neutrophils, macrophages, myeloid-derived suppressor cells, and group 2 innate lymphoid cells. Recent work has also revealed the importance of neuron-derived signals in regulating type 2 inflammation and antihelminth immunity. These studies suggest that multiple body systems coordinate to promote optimal outcomes post-infection. In this review, we will describe the innate immune events that direct the scope and intensity of antihelminth immunity. Further, we will highlight the recent progress made in our understanding of the neuro-immune interactions that regulate these pathways and discuss the conceptual advances they promote.
Introduction
Since Norman Stoll’s hallmark paper 75 years ago revealing the global burden of helminthiasis, various systemic- and meta-analyses have demonstrated that more than a quarter of the global population is infected with helminth parasites (1–5). Included among these infections are nematodes that can be categorized as roundworms (Ascaris lumbricoides, Trichinella spiralis, and Strongyloides stercoralis), whipworms (Trichuris trichiura) and hookworms (Ancylostoma duodenale and Necator americanus). Additionally, helminths are also comprised of platyhelminths, or flatworms, that include free-living turbellarian flatworms, land planarians, and the disease-related Neodermata, consisting of both flukes (schistosomes) and tapeworms (6). These diverse parasites can be transmitted by the consumption of food or water that is contaminated with eggs, via insect bite, or by the parasites directly penetrating the skin (4, 5). Despite their prevalence, helminth infections have long been considered as neglected tropical diseases (NTD) that result in malnutrition, significant morbidity, growth retardation, cognitive deficiencies, and immunopathology (3, 7–9). Control of these NTDs mainly relies on mass antihelmintic drug administrations (MDA) with compounds such as albendazole or mebendazole to reduce worm burdens. These treatments are often combined with improved sanitation measures to prevent future infections (10). Despite these efforts, reinfection rates remain extremely high, with studies showing that up to 60% of individuals can be reinfected within 6-12 months of receiving treatment (3, 10). The frequent use of MDA has also resulted in an increased risk of drug-resistant helminths, a trend that is already seen in livestock populations (11). These limitations highlight the significant need for the development of more dependable and enduring treatment strategies, such as effective immunotherapies. Unfortunately, the development of immune-based therapies has been fraught with difficulty due to the complexity of helminth life cycles and their stage-dependent antigenic variation (10, 12). Further, our incomplete understanding of how antihelminth immunity is initiated and regulated has proven to be another substantial hurdle. To address this, many groups have sought to better understand the innate immune events that promote host protective responses to helminths. Given that investigating helminth infections in patient populations is extremely challenging, many studies have employed animal models infected with Trichuris muris, Trichinella spiralis, Nippostrongylus brasiliensis, Heligmosomoides polygyrus, Strongyloides ratti, Strongyloides venezuelensis, Brugia malayi, and Schistosoma mansoni to study the mammalian immune response to these parasites [summarized in Table 1 (13–18), also reviewed by (20, 21)]. Collectively, these animal models have tremendously informed our understanding of the innate immune responses activated upon the initial exposure to these parasites (22, 23). As mentioned above, antihelminth immunity is primarily mediated by type 2 cytokine responses that are characterized by the development of type 2 helper T (TH2) cells. During a helminth infection, it is well appreciated that inflammation is initiated by the release of specific cytokines from immune cells and epithelial cells, such as, Tuft cells at barrier surfaces (24–29). Included among these rapidly released molecules are interleukin (IL)-25, IL-33, and thymic stromal lymphopoietin (TSLP) (30–32) that are produced in response to both the physical damage caused by the worms and also their release of excretory-secretory (ES) products (24, 26, 27, 33, 34). The production of these effector molecules mobilizes and activates diverse populations of innate immune cells that help to promote the development of TH2 cells (30, 35). Once activated, TH2 cells produce IL-13, influencing goblet cells within infected epithelial barriers to increase mucus production and facilitate worm expulsion (36, 37). Moreover, IL-13 from IL-25 activated ILC2 can regulate epithelial cell differentiation and drive a more secretory epithelial phenotype to facilitate intestinal remodeling and worm expulsion (33, 38). At the same time, TH2 cells produce IL-4 and IL-5 to promote the population expansion of alternatively activated (M2) macrophages and the migration of eosinophils to the affected tissues (39). Collectively, this cascade of events serves to clear worms, while also promoting wound healing once the worms are killed or expelled (40) (Summarized in Figure 1).
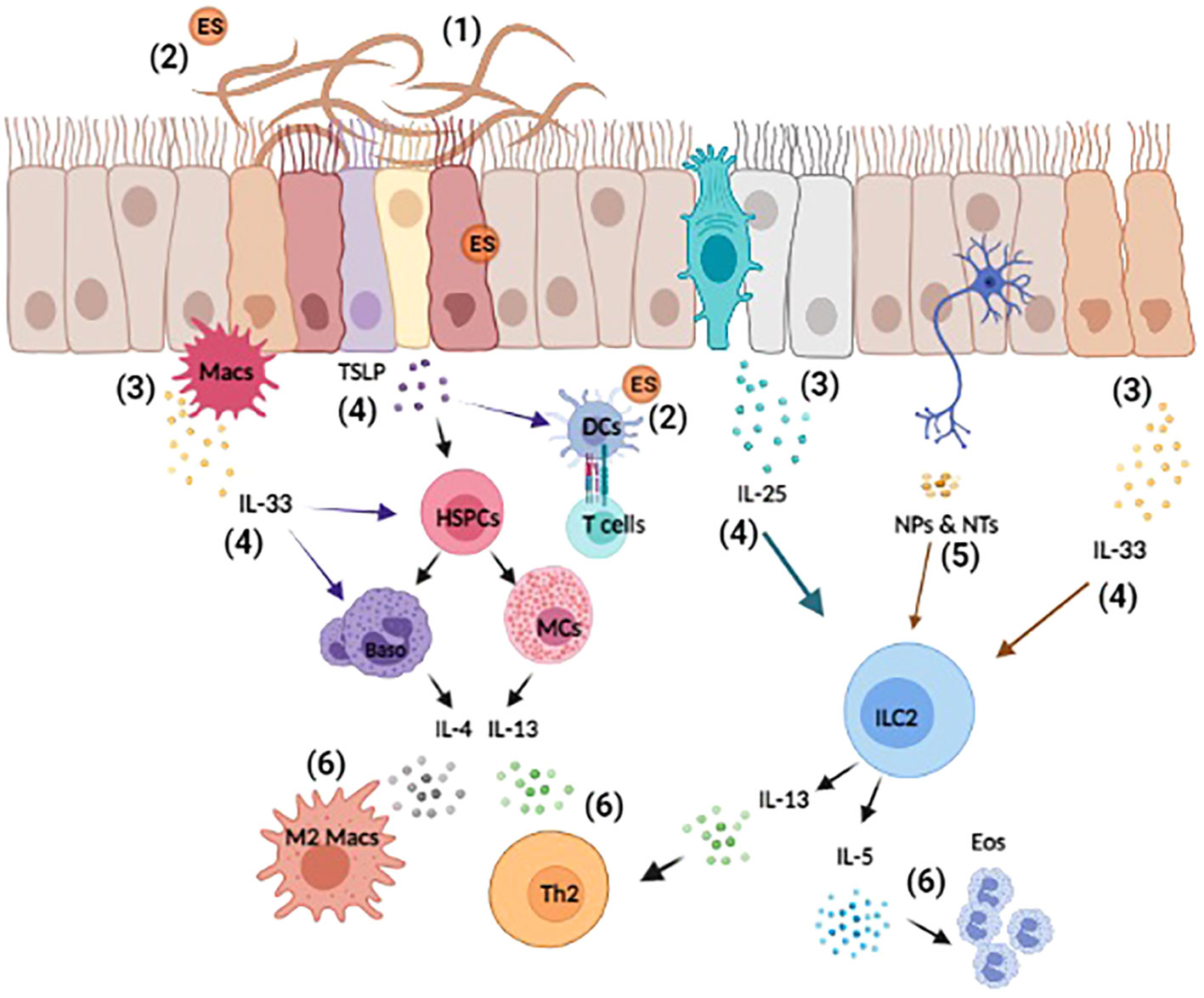
Figure 1 Overview of helminth-induced innate immune responses. Upon invasion, helminths cause substantial tissue damage as they burrow through various barriers and organs, such as the lungs, gut, and skin (1). Helminth also release excretory-secretory (ES) products that can act both locally and systemically (2). The damage-associated and helminth-derived signals promote the production of cytokine alarmins (IL-25, IL-33, and TSLP) from both hematopoietic and non-hematopoietic cells, such as macrophages (Macs) and epithelial cells (3). These early events drive the activation and expansion of innate immune cells, hematopoietic stem/progenitor (HSPCs), mast cells (MCs), basophils (Baso), dendritic cells (DCs), and ILC2s etc. (4). Moreover, innervating neurons can respond to helminth-derived signals by producing neuropeptides (NPs) and neurotransmitters (NTs) that directly influence immune cell activation and regulate inflammation (5). Collectively, these events induce the production of the type 2 cytokines IL-4, IL-5, and IL-13 that promote the polarization of type 2 T helper (TH2), the induction of M2 macrophages and eosinophilia (6). Reviewed in (9, 23, 41–43).
While many non-hematopoietic and adaptive immune cells play critical roles in the promoting host protection to helminth, this article will highlight important studies that have substantially increased our understanding of the various innate immune cells that initiate and regulate helminth-induced inflammation. We will first review recent reports demonstrating the crucial roles various myeloid cells play in promoting host protection to helminths. We will then emphasize the known contributions of innate lymphoid cells to antihelminth immunity. Finally, we will discuss emerging studies defining how these pathways can be regulated by neuro-immune communication occurring within the affected tissue and highlight how neuro-immune crosstalk appears to fine-tune antihelminth immunity to promote optimal outcomes (clearing the worms and restoring tissue homeostasis).
The contributions of mast cells to antihelminth immunity
Mast cells (MCs), having matured from progenitors in peripheral tissues, seed barrier surfaces and are ideally suited to respond to invading helminths. Once activated, MCs are well described for their ability to influence the development and persistence of TH2 cell-associated responses via their release of effector molecules including histamines, leukotrienes, prostaglandins, cytokines, and proteases (44, 45). Specifically, MCs can assist with the polarization of TH2 cells, in part, through the secretion of IL-4 and IL-13 (45). MCs can also influence the activation of antigen presenting cells (APCs), and some studies suggest that mast cells can act as APCs and thereby directly influence T-cell responses. However, the APC functions of MCs are debated and have been challenged by other reports (46–48). The below sections will summarize the known contributions of MCs to type 2 cytokine-mediated inflammation and antihelminth immunity.
Mastocytosis is an established feature of helminth-induced inflammation (49–53) but the roles MCs play in promoting antihelminth immunity are still being elucidated (54–57). While the precise mechanisms that govern the expansion of MC populations remain to be fully defined, studies have reported that mast cell precursors respond to helminth-induced alarmins such as IL-25, IL-33, and TSLP (34). Specifically, IL-25 has been shown to induce a population of c-Kit-expressing progenitor cells that possess MC potential and support immunity to Trichuris muris (58–60). MC populations also expand and activate in response to cytokine alarmins following infection with Heligmosomoides polygyrus bakeri (60). The functions of MC(s) during helminth infections have long been studied using MC(s)-deficient Kitw/KitW-v mice and employing MC(s) stabilizers (60). Using these approaches, it has been shown that MC(s)-deficient Kitw/KitW-v mice infected with H. polygyrus exhibited elevated intestinal worm burdens, reduced production of type 2 cytokines, as well as decreased serum levels of MC protease-1 (Mcpt1) (60). Further, treatment of mice with the MC(s) stabilizer, cromolyn sodium resulted in suppressed type 2 cytokine production and reduced Mcpt1 levels post-H. polygyrus infection (60). Furthermore, MC(s)-deficient Kitw/KitW-v mice also showed delayed expulsion of Trichinella spiralis (61) and studies have demonstrated that Mcpt1 and mast cell-derived IL-4 are required for optimal clearance of T. spiralis (52, 56, 62, 63). Collectively, these studies highlight an important role for MCs in promoting immunity to several helminth parasites (31, 62, 63). In contrast, by taking similar loss-of-function approaches, it has been shown that MC(s) do not appear to promote worm expulsion in the context of Nippostrongylus brasiliensis or T. muris infection, suggesting that the roles MC(s) play are parasite-specific (31, 60, 62, 64, 65).
The contributions of hematopoietic stem/progenitors to host protection
It has traditionally been reported that the developmental pathways for red blood cells (RBCs) and MCs begin in the bone marrow with hematopoietic stem cells (HSCs), which can differentiate into a colony forming unit committed to the granulocyte, erythrocyte, monocyte, and megakaryocyte lineages (CFU-GEMM) (66) or follow the differentiation pathways of myeloid and lymphoid cells (39). Likely due to activation by circulating erythropoietin (EPO), CFU-GEMM can become burst-forming units-erythroid (BFU-E) (67) and thereby generate RBCs. In the case of MC development, instead of being influenced by EPO and entering erythropoiesis, the CFU-GEMM can be acted upon by stem-cell factor to develop down the granulocyte pathway (53). During this process, CFU-GEMMs increase their CD34 expression and become multipotent progenitors (MPP) (67). MPPs can then progress to become common myeloid progenitors (CMP) followed by granulocyte/monocyte progenitors (GMP) that can ultimately become a committed MC progenitor (MCp) (68–70). These MCps can then mature into MCs with heterogeneous properties depending on factors such as their tissue location (70). Additionally, emerging studies investigating MC and erythrocyte development now suggest that they may share more developmental similarities than previously appreciated.
Recent single cell-based work in humans and mice have determined that RBCs and MCs are directly linked and arise from a common progenitor cell (56, 57, 71, 72). Consistent with a link between these distinct lineages, a progenitor cell with dual MC and RBC potential was also identified in the context of a T. spiralis challenge (57). This erythrocyte/mast cell progenitor was defined by its expression of the metabolic enzyme carbonic anhydrase 1 (Car1) and was sufficient to promote type 2 cytokine responses and RBC development post-T. spiralis infection (56, 57). This work suggests that in addition to supporting protective immunity via promoting MC development, Car1-expressing progenitor cells may also combat helminth-induced wounding by supporting RBC development and thereby help to mitigate blood loss, a common feature of infection.
These studies complement earlier work that further suggest important roles for hematopoietic stem/progenitors (HSPCs) in promoting antihelminth immunity. The tissue-derived cytokines (IL25, IL33, and TSLP) can promote the populations expansion of other multipotent progenitor cells that have varied expression of CD34 and c-Kit. These specialized progenitors can develop into several innate immune (MCs, basophils, and macrophages) and thereby promote antihelminth immunity (31, 58, 73). After entering the periphery with an immature phenotype, helminth-mobilized HSPCs can undergo extramedullary hematopoiesis and support innate immune responses at the host parasite interface (31, 58, 73). Collectively, these studies demonstrate that the egress of HSPCs from the bone marrow is an important component of host protection (31, 56–58, 73).
The contributions of basophils to antihelminth immunity
Although the contributions of basophils to antihelminth immunity and type 2 inflammation have long been studied, their diverse functions are still being elucidated. At baseline, basophils are extremely rare and represent the least prevalent granulocyte in the blood. However, peripheral basophilia is a hallmark of several helminth infections (74, 75). While basophil development has traditionally been reported to occur in the bone marrow, recent work also suggests that basophils can develop from mobilized progenitor cells that enter the periphery in the context of helminth-induced inflammation (75–77). Similar to MCs, basophils can produce robust amounts of effector molecules including type 2 cytokines (IL-4 and IL-13), histamines, platelet-activating factor, and lipid mediators (prostaglandins and leukotrienes) that allow them to promote worm clearance (75, 78). Additionally, their production of growth factors like amphiregulin and macrophage colony-stimulating factor are also thought to promote tissue reparative pathways (79, 80).
The most effective systems for studying basophil function have been genetic mouse models targeting the basophil-specific protease MCs protease 8 (Mcpt8) and basophil-specific IL-4 enhancer elements (75, 81). Using these systems, loss-of-function studies have indicated a non-redundant role for basophils in promoting worm expulsion following T. spiralis, T. muris, and H. polygyrus infections (82–84). However, basophil depletion had no effect on worm burdens following a primary infection with N. brasiliensis or S. ratti (85–87). Additionally, depleting basophils post-Strongyloides venezuelensis infection resulted in lower S. venezuelensis egg production, suggesting that basophils regulate parasite fitness (88). In summary, like many other innate immune cells, the functions of basophils appear to be highly parasite-specific.
As mentioned above, host protective responses to helminths involves both promoting worm expulsion and mitigating helminth-induced tissue damage. While loss-of-function studies targeting basophils revealed no effect in regulating N. brasiliensis worm burdens, additional work has now revealed that basophils depletion results in dysregulated lung inflammation. N. brasiliensis-induced ILC2 responses were found to be exaggerated in the absence of basophils, resulting in increased lung pathology and reduced pulmonary function (78). The inhibitory effect of basophils was mediated by neuro-immune interactions; the nature of these signals will be discussed in greater detail below. Conceptually, these studies suggest that basophils can also promote host protection by restricting helminth-induced inflammation and preventing excessive tissue damage.
The contributions of eosinophils to antihelminth immunity
Similar to MCs and basophils, peripheral eosinophilia is a common feature of parasitic helminth infections (89). Eosinophils traffic to helminth-affected tissues where they are reported to contribute to worm killing and various aspects of tissue remodeling (90–93). Eosinophils produce numerous effector molecules including eosinophil-derived neurotoxin, major basic protein, and eosinophil peroxidase that can contribute to type 2 cytokine responses and simultaneously promote extracellular matrix deposition and wound healing (93, 94). Further, recent work also suggest that eosinophils can inhibit the mobility of parasitic larvae in an antibody-dependent manner (95).
While eosinophils can be recruited by both chemokines and cytokine alarmins (31, 96, 97), IL-5 produced by helminth-activated ILC2s and TH2 cells is a dominant regulator of infection-induced eosinophilia (98, 99). Consistent with these reports, mice lacking productive IL-5/IL-5R signaling are less able to mount an eosinophilic response and are less efficient at clearing T. spiralis (92, 98). Recent work has also showed that both mouse and human eosinophils can respond directly to parasite antigens isolated from Strongyloides stercoralis and S. mansoni respectively (100, 101). Although eosinophils produce numerous effector molecules, the mechanisms eosinophils employ to kill parasitic worms remain to be fully defined. It has been hypothesized that the release of chromatin and DNA extracellular traps may be one killing mechanism eosinophils use to combat large extracellular pathogens, but more work is required to support this hypothesis (102). Further, serum levels of eosinophil granular proteins are reported to be elevated in individuals infected with helminths such as S. stercoralis, indicating eosinophils may also activate and degranulate at distal sites (93). These data suggest that eosinophils may contribute to host protection beyond their roles in killing worms at the host parasite interface.
Contributions of dendritic cells to antihelminth immunity
Dendritic cells (DCs), known for their professional antigen presenting cell (APC) capacities, are appreciated as important liaisons that bridge the gap between innate and adaptive immunity. As such, DCs are known for their pivotal roles in the recognition, capture, processing, and presentation of helminth-derived antigens to T cells (103–105). Many studies have reported that helminth ES products can activate DCs via toll-like receptor 2 (TLR2), TLR4, or C-type lectin receptors (103). Moreover, it has also been shown that helminth infections can promote non-classical DC maturation which is reported to dramatically influence T cell activation [reviewed in (103)]. For example, T. spiralis ES antigens and Glutathione-S-transferase can suppress DC maturation (106, 107) and T. spiralis-conditioned DCs can alleviate 2,4,6-trinitrobenzene sulfonic acid (TNBS)- induced colitis in mice (108). More recently, Ding et al. also reported that DCs stimulated by T. spiralis ES products were able to significantly inhibit tumor growth in H22 tumor-bearing mice (109). Interestingly, Connor and Webb et al. also found that DCs adopt a type 1 interferon (IFN-I) signature when stimulated with S. mansoni or N. brasiliensis antigens (110, 111). Of note, this IFN-I responsiveness was required for DCs to prime TH2 immune activation in these contexts (110, 111).
It is well established that conventional DCs (cDCs) can be subdivided into cDC1s and cDC2s that possess unique effector functions and abilities to polarize T cells (112). Specifically, cDC1s (CD8α+CD103+) are reported to specialize in antigen cross-presentation and promote TH1 cell development that supports immunity to intracellular pathogens. In contrast, cDC2s (CD4+CD11b+) that express interferon regulatory factor 4 (IRF4) specialize in presenting antigen to CD4+ T cells and possess a unique ability to promote TH2 or TH17 responses (112, 113). Given the plasticity of these DC subsets, it is perhaps not surprising that specialized DCs have also been reported to promote antihelminth responses. Cook et al., revealed that DC production of RELMα is required for optimal TH2 priming post-S. mansoni egg challenge (114). Moreover, TH2-inducing DCs expressing OX40 ligand, CD301b and programmed death ligand-2 (PDL2), are required for optimal TH2 cell development post-N. brasiliensis infection (115–117). More recently, Halim et al. showed that IRF4+CD11b+CD103− DCs produce the TH2 cell-attracting chemokine CCL17 post-N. brasiliensis challenge (118). Studies by Mayer et al., also identified a role for IRF4-expressing DCs in priming TH2 cell responses following S. mansoni egg challenge (119).
Interestingly, CD11b+CD103+ DCs were shown to promote TH2 responses in the small intestine, while CD11b+CD103- DCs appear to play similar roles in the colon, suggesting functional-specificity for DCs in different anatomical compartments (119). Collectively, these studies demonstrate that highly specialized DC subsets play important roles in promoting antihelminth immunity and suggest that CD103 expression may dictate the tissue specificity of these APCs.
Unlike conventional DCs, the roles of plasmacytoid DCs (pDCs) in antihelminth immunity remain less defined. While studies suggest that pDCs are dispensable for hepatic TH2 responses during acute S. mansoni infection (23, 120), other reports suggest that pDCs are required for optimal TH2 cytokine production in response to S. mansoni eggs in the intestinal-draining mesenteric lymph nodes (120). Furthermore, pDC depletion at chronic stages of infection resulted in increased hepatic and splenic pathology as well as suboptimal TH2 cytokine production in the liver. However, further studies are needed to better define the role pDCs play in promoting antihelminth immunity and regulating tissue pathology.
The contributions of neutrophils to antihelminth immunity
Although neutrophils are best known for their roles in antiviral and antibacterial immunity, recent studies have begun to define a role for these dynamic cells in the context of type 2 inflammation (121–123). For instance, recent reports have demonstrated that neutrophils can inhibit the mobility of S. ratti larvae via their release of myeloperoxidase and matrix metalloproteinase-9. Additionally, studies have also highlighted that neutrophils are recruited to the lung post-N. brasiliensis infection where they contribute to tissue damage and hemorrhaging (124). It is reported that N. brasiliensis-induced neutrophils are recruited by local production of IL-17A from activated γδT cells in response to chitinase-like proteins (CLPs), such as Ym1 (121, 122). Additionally, it has been shown that soluble extracts from S. stercoralis promote neutrophil recruitment through CXCR2, rather than IL-17, suggesting helminth-induced neutrophils may be regulated by distinct signals (125). Even though γδ+ intraepithelial lymphocyte populations are found to be expanded post-T. muris and -T. spiralis infection (126, 127), whether neutrophil recruitment is initiated in the context of these infections remains unknown.
Importantly, work by Chen et al. showed that neutrophils sort-purified from the lungs of N. brasiliensis-infected mice had a distinct transcriptional signature compared to lipopolysaccharides-activated neutrophils (121). One dominant feature of N. brasiliensis -induced neutrophils was their increased expression of the type 2 cytokine IL-13, prompting them to be named N2 neutrophils. Moreover, it was shown that neutrophil-derived IL-13 promoted M2 macrophage polarization (121). In addition to IL-13, neutrophils have also been shown to release neutrophil extracellular DNA traps (NETs) to promote antihelminth immunity. Specifically, NETs were found to be released upon contact with S. stercoralis larvae (128). Although the NETs failed to kill the larvae, they helped immobilize parasites (129) and may assist with “starving” the worms by trapping them in a nutrient-deficient microenvironment similar to what has recently been shown by macrophages (130). Further, Bouchery et al., showed that NETs released following an N. brasiliensis challenge can directly impair larval viability and the parasites combat this response by secreting DNAse II (123). The ability of helminth-derived products to inhibit NET formation was also shown by Chauhan et al. that demonstrated that Mesocestoides corti ES products were sufficient to inhibit NET formation in the context of bacterial peritonitis (131). Moreover, it was reported that neutrophils and eosinophils require myeloperoxidase and major basic protein to kill S. stercoralis larvae in vitro (93). Therefore, it is possible that the NETs immobilize the parasites and thereby maximize the parasitic exposure to antihelminth products secreted by activated granulocytes and macrophages. Taken together, these studies suggest that neutrophils promote host protective responses to helminths via a variety of effector functions, however, further work is required to better understand how parasite-specific these responses are, and the full range of effector functions neutrophils employ in these contexts.
The contributions of monocytes and macrophages to antihelminth immunity
It is well established that the IL-4 and/or IL-13-mediated activation of macrophages results in their polarization to what has traditionally been termed as an alternatively activated or M2 phenotype. The importance of M2s and their roles in promoting host protection to helminths is well established and has been extensively reviewed elsewhere (132–135). Therefore, we will briefly highlight this impressive body of literature and largely focus our discussion on recent studies describing heterogeneity within tissue-specific macrophage responses.
The induction of M2s has been demonstrated in the context of numerous helminth infections including N. brasiliensis, S. mansoni, H. polygyrus, Taenia crassiceps, T. spiralis, Fasciola hepatica, Ascaris suum, and filarial parasites (136–143). While IL-4 and/or IL-13 produced by various myeloid and lymphoid cells are known to promote M2 responses (124, 132, 137, 144–146), additional factors can also facilitate M2 activation including antibodies (IgG), collectins (surfactant protein A & D), complement components, helminth ES products (34, 132), TLR and CLR ligands, macrophage migration inhibitory factor (MIF), macrophage-derived protease inhibitor (serpinB2), cytokine alarmins, and metabolic cues (vitamin A) (58, 147–150). M2s are known to promote host protection via several mechanisms including the release of effector molecules and chemokines to promote type 2 responses, directly or indirectly killing parasitic larvae, promoting wound healing by stimulating collagen deposition, and angiogenesis (114, 132, 133, 135, 150, 151).
Macrophages reside in every organ and mucosal surface and exhibit distinct phenotypes and effector functions depending on their tissue-specific niche (132). Importantly, recent studies have also revealed that macrophages are specially programmed to operate in an organ-specific manner (152–157). Tissue-resident macrophages (TRMs) that are derived from embryonic precursors, seed the tissues during early stages of development and are tailored to perform tissue-specific tasks. While TRMs have been shown to proliferate in the context of inflammation (158), additional monocyte-derived macrophages can enter these niches to supplement TRM responses. Upon entering the tissue, monocyte-derived macrophages receive tissue-specific signals and begin to acquire a TRM-like phenotype (115, 159, 160). These studies strongly suggest that M2 responses occurring post-helminth infection are comprised of a heterogenous group of cells. Additionally, recent work has shown that TRMs and monocyte-derived macrophages can perform unique antihelminth functions. For instance, monocyte-derived alveolar macrophages induced post-N. brasiliensis infection are more effective at killing parasites than TRMs. The heightened ability of monocyte-derived alveolar macrophages to kill parasitic larvae was mediated by their enhanced expression of arginase 1 which allowed them to deplete local arginine (130). Further, by comparing macrophages from S. mansoni or Litomosoides sigmodontis-infected mice, along with IL-4 and anti-IL-4 antibody complexes (IL-4c) and thioglycolate-treated mice, Gundra et al. reported that monocyte-derived macrophages are more immunoregulatory than TRMs (161). Moreover, additional work identified that vitamin A was essential to instruct the tissue programming of macrophages from a monocyte-derived phenotype to a more TRM-like phenotype (149). Collectively, these emerging studies suggest that antihelminth macrophage responses are more heterogeneous than previously appreciated. Further, this important work suggests that several factors dictate how M2 macrophages are regulated, including the nature of the parasite, the origin of the cells (monocyte-derived versus tissue-resident) and the signals they receive from the tissue microenvironment.
Contributions of myeloid-derived suppressor cells to antihelminth immunity
Myeloid-derived suppressor cells (MDSCs) were initially described in cancer for their ability to inhibit anti-tumor T cells but have subsequently been appreciated for their immunosuppressive roles in response to pathogens including helminth (162, 163). MDSCs are a heterogenous group of cells that can be divided into two major groups, granulocytic/polymorphornuclear MDSCs (PMN-MDSCs, Gr1+CD11b+Ly6G+Ly6Clo) and monocytic MDSCs (M-MDSCs, Gr1+CD11b+Ly6G-Ly6Chi). Infections with S. mansoni, S. japonicum, T. crassicepts, Brugia malayi, N. brasiliensis, and H. polygyrus have all been shown to induce MDSCs that are thought to play important immunoregulatory roles (162). The diverse roles MDSCs play in regulating helminth-induced inflammation is discussed in great depth by Stevenson et al. in a recent review article and therefore will not be discussed in depth here (162).
The contributions of innate lymphoid cells to antihelminth immunity
Innate lymphoid cells (ILCs) are tissue-resident cells that lack adaptive antigen receptors and are considered the non-specific counterparts of T lymphocytes. They reside in various tissues including the lung, intestine, mesenteric fat associated lymphoid cluster, liver, skin, and kidney, and are appreciated for their pivotal roles in promoting immunity to bacteria, viruses, and parasitic infections. ILCs are classified into 5 distinct subsets – nature killer (NK) cells, ILC1s, ILC2s, ILC3s, and lymphoid tissue inducer cells based on their developmental origins, transcriptional and surface marker phenotypes, as well as functional differences (164). Importantly, various subsets of ILCs have been shown to play diverse roles in regulating antihelminth immunity which will be highlighted in the below sections.
NK cells have been shown to accumulate during the early phases of H. polygyrus infection in an IFNγ receptor-dependent manner where they are thought to promote tissue protection (165). NK cells have also been shown to be activated following S. japonicum infection, S. mansoni infection, and S. mansoni egg challenge (166, 167). Consistent with animal models, human studies have also indicated that NK cells appear to respond to helminths (168, 169). However, future studies are needed to better elucidate the functions of NK cells in these contexts.
ILC2s are well described for their ability to respond to cytokine alarmins (IL-25, TSLP, and IL-33) and as such become rapidly activated in the context of helminth infections (34, 170, 171). In addition to alarmins, ILC2s are also regulated by type 2 cytokines (IL-4 and IL-9) and inflammatory lipid mediators that are hallmarks of type 2 inflammation (172, 173). Once activated, ILC2s are reported to produce robust levels of IL-5 and IL-13 and thereby support the population expansion and recruitment of eosinophils, the M2 polarization of macrophages, and mucus production by goblet cells (28, 32, 33, 82, 121, 124, 132, 170, 171, 174, 175). Helminth activated ILC2s have also been shown to produce IL-4 and IL-9, although these cytokines appear to be less prominent (171, 176). These studies are excessively discussed in review articles by Herbert et al., Bouchery et al., and Miller et al. (177–179). Additionally, growing evidence suggests that the antihelminth functions of ILC2s are regulated, in part, by neuron-derived signals. The importance of these pathways will be discussed in greater depth below.
Neuro-immune communication during helminth infections
The central nervous system is responsible for maintaining homeostasis during steady state conditions and in the context of infection and inflammation (180, 181). To accomplish this, complicated cellular and molecular networks have been established to allow highly coordinated communication between the nervous and immune system to occur (75, 78, 182–186). Given the intricate relationship helminths have developed with their mammalian hosts, it is not surprising that many of these pathways play critical roles in promoting host protection and regulating antihelminth immunity. Emerging studies have significantly advanced our understanding of these intricate networks and have encouraged more interdisciplinary collaborations to better understand neuro-immune interactions in the context of helminth-induced inflammation. The following section will highlight pathways that are known, or likely, to regulate neuro-immune communication during helminth infections. Additionally, we will comment on the need for future studies to further determine how these pathways operate in response to this diverse class of pathogens.
Novel transgenic animal models, precise activation techniques (chemogenetics and optogenetics), and other emerging technologies have greatly facilitated our ability to interrogate the pathways that regulate rare immune cells located within helminth-affected tissues (23, 75, 186). These intricate studies have recently revealed several neuron-derived signals that regulate ILC2 responses post-N. brasiliensis infection (Summarized in Figure 2). For instance, the neuropeptide neuromedin U (NMU) was recently shown to directly activate ILC2s through its receptor NMUR1 to drive antiparasitic immunity post-N. brasiliensis infection. In the intestine, a subset of enteric neurons express NMU (185, 187) and colocalize with ILC2s. NMU induces ILC2 proliferation and production of type 2 cytokines, such as IL-5, IL-9, and IL-13. Additionally, Chu et al. found that activated ILC2s upregulate choline acetyltransferase to generate more acetylcholine (Ach) following N. brasiliensis infection or treatment with cytokine alarmins (184). Importantly, Ach was sufficient to promote ILC2 cytokine production and their expulsion of N. brasiliensis (184). Another neuropeptide calcitonin gene-related peptide (CGRP), expressed by nociceptor neurons was shown to inhibit ILC2 activation and thereby limit antihelminth responses (183). Similarly, neuromedin B (NMB) was also shown to restrict ILC2 activation in the lungs as part of a basophils-dependent feedback loop following N. brasiliensis infection (78). Interrupting NMB-NMBR interactions was also shown to result in substantially increased lung pathology and reduced lung function post-infection, suggesting that its inhibitory effects are required to maintain tissue integrity. This work also showed that prostaglandin E (PGE), one of several basophil-derived lipid mediators, can stimulate NMBR expression on ILC2s and thereby prime them for NMB-mediated inhibition (Figure 1). Finally, sympathetic neurons can also inhibit ILC2 responses and helminth clearance by activating beta-2 adrenergic receptor, which are expressed by ILC2s (182).
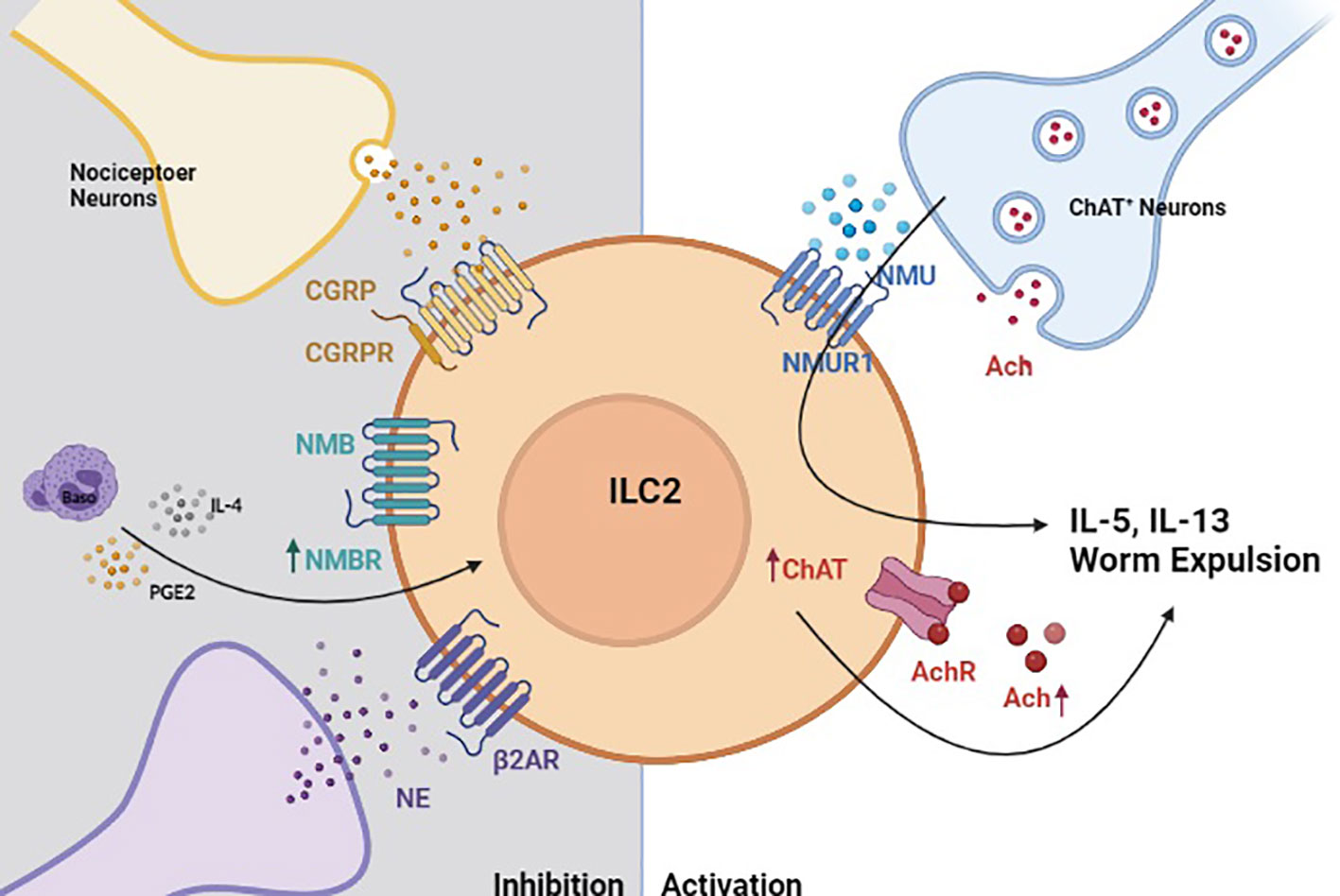
Figure 2 Neuro-Immune crosstalk regulates helminth-induced inflammation. In the context of helminth-induced inflammation, group 2 innate lymphoid cells (ILC2s) are activated by neuromedin U (NMU) that is released by choline acetyltransferase positive (ChAT+) neurons (185). Activated ILC2s also upregulate ChAT to promote acetylcholine biosynthesis, which serves to further amplify their production of type 2 cytokines (184). Helminth-activated ILC2s are also restricted by neuron-derived signals. Calcitonin gene-related peptide (CGRP), neuromedin B (NMB) and sympathetic neuron-derived norepinephrine (NE) inhibit ILC2 response in a manner that properly regulated helminth-induced inflammation (78, 182, 183).
In addition to ILC2s, MCs have long been observed to be anatomically and functionally associated with neurons and neuronal processes throughout the body. Additionally, neural regulation of MCs has been described in disease models of atopic dermatitis, allergic asthma, and chronic obstructive pulmonary disease (160, 188). MCs express a variety of neuron-related receptors, transmitters and peptides, such as Ach, substance P, and CGRP among others (189). Recent studies have also highlighted a pivotal role of Mas-related G-protein-coupled receptors (Mrgprs) in neuro-immune crosstalk (188, 190). Mrgprs are highly expressed in MCs and are reported to mediate MC degranulation in an IgE-independent manner (191, 192). More recently, Arifuzzaman et al., found that cutaneous bacterial infection can activate MC activation through an MrgprB2/MRGPRX2-mediated pathway, which leads to enhanced recruitment of neutrophils and wound-healing CD301b+ DCs (193), both cell types that are known to promote antihelminth immunity. Zhang et al. also reported that MrgprD-expressing neurons can suppress MC hyperresponsiveness and skin inflammation by releasing glutamate (194). Interestingly, one recent study showed that tick peptides can cause histamine-independent itch, by directly activating MrgprC11/MRGPRX1 on the dorsal root ganglion and MrgprB2/MRGPRX2 on MCs (195). Furthermore, other non-helminth models have revealed that MCs can stimulate itch sensory and nociceptor neurons to promote itch sensation and type 2 inflammation in the skin (168, 196, 197). Collectively, these studies highlight important roles for MCs in mediating neuro-immune communication at barrier surface. The ability of MCs and other tissue-resident cell types such as macrophages to participate in neuro-immune mechanisms that regulate antihelminth responses is an active and exciting area of study that will further inform our understanding of how inflammation is regulated in the tissue microenvironment. Further, whether neuro-immune communication is bidirectional and also involves helminth-activated immune cells regulating the functions of the central nervous system is also an area of great interest.
Summary
A robust body of literature has highlighted the important functions of various innate immune cells in regulating host protective responses to helminths. Further, it is now appreciated that many of these responses are regulated in both parasite- and tissue-specific manners. Given the ancient and complex relationship between helminth and their mammalian hosts, studying these infections provides a unique lens into the factors that regulate tissue-specific immunity. This work has begun to highlight the importance of the peripheral nervous system in positively or negatively regulating helminth-induced inflammation. The ability of neuron-derived signals to amplify or restrict antihelminth responses may allow them to tailor the inflammation to promote optimal outcomes (inflammation strong enough to promote worm expulsion, but tightly regulate to prevent excessive tissue damage). However, future studies are required to better understand how these seemingly opposing signals operate post-infection and to determine whether other tissue-resident cell types such MCs, TRM, and monocyte-derived macrophages are similarly involved in these processes. Gaining a better understanding of these pathways may inform therapeutic strategies to treat a myriad of inflammatory conditions and reveal more efficient ways to treat tissue-specific pathology.
Author contributions
JP and MCS contributed to the generation of the manuscript, in terms of material development, content creation, and proofreading. HGF and CMH contributed to the generation of certain paragraphs, related literature search, and proof reading of the manuscript. All authors contributed to the article and approved the submitted version.
Conflict of interest
MCS is the founder and president of NemaGen Discoveries.
The remaining authors declare that the research was conducted in the absence of any commercial or financial relationships that could be construed as a potential conflict of interest.
Publisher’s note
All claims expressed in this article are solely those of the authors and do not necessarily represent those of their affiliated organizations, or those of the publisher, the editors and the reviewers. Any product that may be evaluated in this article, or claim that may be made by its manufacturer, is not guaranteed or endorsed by the publisher.
References
1. de Silva NR, Brooker S, Hotez PJ, Montresor A, Engels D, Savioli L. Soil-transmitted helminth infections: updating the global picture. Trends Parasitol (2003) 19:547–51. doi: 10.1016/j.pt.2003.10.002
2. Steinmann P, Zhou XN, Li YL, Li HJ, Chen SR, Yang Z, et al. Helminth infections and risk factor analysis among residents in Eryuan county, Yunnan province, China. Acta Trop (2007) 104:38–51. doi: 10.1016/j.actatropica.2007.07.003
3. Jia TW, Melville S, Utzinger J, King CH, Zhou XN. Soil-transmitted helminth reinfection after drug treatment: a systematic review and meta-analysis. PloS Negl Trop Dis (2012) 6:e1621. doi: 10.1371/journal.pntd.0001621
4. WHO. Schistosomiasis and soil-transmitted helminthiases: number of people treated in 2015. Wkly Epidemiol Rec (2016) 91:585–95.
5. Jourdan PM, Lamberton PHL, Fenwick A, Addiss DG. Soil-transmitted helminth infections. Lancet (2018) 391:252–65. doi: 10.1016/S0140-6736(17)31930-X
7. Cox FE. History of human parasitology. Clin Microbiol Rev (2002) 15:595–612. doi: 10.1128/CMR.15.4.595-612.2002
8. Dean AS, Crump L, Greter H, Hattendorf J, Schelling E, Zinsstag J. Clinical manifestations of human brucellosis: a systematic review and meta-analysis. PloS Negl Trop Dis (2012) 6:e1929. doi: 10.1371/journal.pntd.0001929
9. Gause WC, Wynn TA, Allen JE. Type 2 immunity and wound healing: evolutionary refinement of adaptive immunity by helminths. Nat Rev Immunol (2013) 13:607–14. doi: 10.1038/nri3476
10. Zawawi A, Else KJ. Soil-Transmitted Helminth Vaccines: Are We Getting Closer? Front Immunol (2020) 11:576748. doi: 10.3389/fimmu.2020.576748
11. Waller PJ, Echevarria F, Eddi C, Maciel S, Nari A, Hansen JW. The prevalence of anthelmintic resistance in nematode parasites of sheep in southern Latin America: general overview. Vet Parasitol (1996) 62:181–7. doi: 10.1016/0304-4017(95)00909-4
12. McNeilly TN, Nisbet AJ. Immune modulation by helminth parasites of ruminants: implications for vaccine development and host immune competence. Parasite (2014) 21:51. doi: 10.1051/parasite/2014051
13. Klementowicz JE, Travis MA, Grencis RK. Trichuris muris: a model of gastrointestinal parasite infection. Semin Immunopathol (2012) 34:815–28. doi: 10.1007/s00281-012-0348-2
16. Brooker S, Bethony J, Hotez PJ. Human hookworm infection in the 21st century. Adv Parasitol (2004) 58:197–288. doi: 10.1016/S0065-308X(04)58004-1
17. Buonfrate D, Bisanzio D, Giorli G, Odermatt P, Furst T, Greenaway C, et al. The Global Prevalence of Strongyloides stercoralis Infection. Pathog 9 (2020) 9(6):468. doi: 10.3390/pathogens9060468
18. Mak JW. Epidemiology of lymphatic filariasis. Ciba Found Symp (1987) 127:5–14. doi: 10.1002/9780470513446.ch2.
19. Bocanegra C, Gallego S, Mendioroz J, Moreno M, Sulleiro E, Salvador F, et al. Epidemiology of Schistosomiasis and Usefulness of Indirect Diagnostic Tests in School-Age Children in Cubal, Central Angola. PloS Negl Trop Dis (2015) 9:e0004055. doi: 10.1371/journal.pntd.0004055
20. Eberle JU, Voehringer D. Role of basophils in protective immunity to parasitic infections. Semin Immunopathol (2016) 38:605–13. doi: 10.1007/s00281-016-0563-3
21. Obata-Ninomiya K, Domeier PP, Ziegler SF. Basophils and Eosinophils in Nematode Infections. Front Immunol (2020) 11:583824. doi: 10.3389/fimmu.2020.583824
22. Loke P, Lee SC, Oyesola OO. Effects of helminths on the human immune response and the microbiome. Mucosal Immunol (2022). doi: 10.1038/s41385-022-00532-9
23. Webb LM, Tait Wojno ED. The role of rare innate immune cells in Type 2 immune activation against parasitic helminths. Parasitology (2017) 144:1288–301. doi: 10.1017/S0031182017000488
24. Gerbe F, Sidot E, Smyth DJ, Ohmoto M, Matsumoto I, Dardalhon V, et al. Intestinal epithelial tuft cells initiate type 2 mucosal immunity to helminth parasites. Nature (2016) 529:226–30. doi: 10.1038/nature16527
25. Kotas ME, Mroz NM, Koga S, Liang HE, Schroeder AW, Ricardo-Gonzalez RR, et al. CISH constrains the tuft-ILC2 circuit to set epithelial and immune tone. Mucosal Immunol (2021) 14:1295–305. doi: 10.1038/s41385-021-00430-6
26. Luo XC, Chen ZH, Xue JB, Zhao DX, Lu C, Li YH, et al. Infection by the parasitic helminth Trichinella spiralis activates a Tas2r-mediated signaling pathway in intestinal tuft cells. Proc Natl Acad Sci U.S.A (2019) 116:5564–9.
27. Drurey C, Lindholm HT, Coakley G, Poveda MC, Loser S, Doolan R, et al. Intestinal epithelial tuft cell induction is negated by a murine helminth and its secreted products. J Exp Med 219 (2022) 219(1):e20211140. doi: 10.1084/jem.20211140
28. Varyani F, Loser S, Filbey KJ, Harcus Y, Drurey C, Poveda MC, et al. The IL-25-dependent tuft cell circuit driven by intestinal helminths requires macrophage migration inhibitory factor (MIF). Mucosal Immunol (2022). doi: 10.1038/s41385-022-00496-w
29. Schneider C, O'Leary CE, von Moltke J, Liang HE, Ang QY, Turnbaugh PJ, et al. A Metabolite-Triggered Tuft Cell-ILC2 Circuit Drives Small Intestinal Remodeling. Cell (2018) 174:271–284 e14. doi: 10.1016/j.cell.2018.05.014
30. Allen JE, Sutherland TE. Host protective roles of type 2 immunity: parasite killing and tissue repair, flip sides of the same coin. Semin Immunol (2014) 26:329–40. doi: 10.1016/j.smim.2014.06.003
31. Siracusa MC, Saenz SA, Wojno ED, Kim BS, Osborne LC, Ziegler CG, et al. Thymic stromal lymphopoietin-mediated extramedullary hematopoiesis promotes allergic inflammation. Immunity (2013) 39:1158–70. doi: 10.1016/j.immuni.2013.09.016
32. Hung LY, Tanaka Y, Herbine K, Pastore C, Singh B, Ferguson A, et al. Cellular context of IL-33 expression dictates impact on anti-helminth immunity. Sci Immunol 5 (2020) 5(53):eabc6259. doi: 10.1126/sciimmunol.abc6259
33. von Moltke J, Ji M, Liang HE, Locksley RM. Tuft-cell-derived IL-25 regulates an intestinal ILC2-epithelial response circuit. Nature (2016) 529:221–5. doi: 10.1038/nature16161
34. Inclan-Rico JM, Siracusa MC. First Responders: Innate Immunity to Helminths. Trends Parasitol (2018) 34:861–80. doi: 10.1016/j.pt.2018.08.007
35. Ehsan M, Gadahi JA, Hasan MW, Haseeb M, Ali H, Yan R, et al. Characterization of Haemonchus contortus Excretory/Secretory Antigen (ES-15) and Its Modulatory Functions on Goat Immune Cells In Vitro. Pathog 9 (2020) 9(3):162. doi: 10.3390/pathogens9030162
36. Patel N, Kreider T, Urban JF Jr., Gause WC. Characterisation of effector mechanisms at the host:parasite interface during the immune response to tissue-dwelling intestinal nematode parasites. Int J Parasitol (2009) 39:13–21. doi: 10.1016/j.ijpara.2008.08.003
37. Birchenough GM, Johansson ME, Gustafsson JK, Bergstrom JH, Hansson GC. New developments in goblet cell mucus secretion and function. Mucosal Immunol (2015) 8:712–9. doi: 10.1038/mi.2015.32
38. Rajeev S, Sosnowski O, Li S, Allain T, Buret AG, McKay DM. Enteric Tuft Cells in Host-Parasite Interactions. Pathog 10 (2021) 10(9):1163. doi: 10.3390/pathogens10091163
40. Sorobetea D, Svensson-Frej M, Grencis R. Immunity to gastrointestinal nematode infections. Mucosal Immunol (2018) 11:304–15. doi: 10.1038/mi.2017.113
41. Palker TJ, Dong G, Leitner WW. Mast cells in innate and adaptive immunity to infection. Eur J Immunol (2010) 40:13–8. doi: 10.1002/eji.200990325
42. Kawakami T, Galli SJ. Regulation of mast-cell and basophil function and survival by IgE. Nat Rev Immunol (2002) 2:773. doi: 10.1038/nri914
43. Henz B, Maurer M, Lippert U, Worm M, Babina M. Mast cells as initiators of immunity and host defense. Exp Dermatol (2001) 10:1–10. doi: 10.1034/j.1600-0625.2001.100101.x
44. Lotfi-Emran S, Ward BR, Le QT, Pozez AL, Manjili MH, Woodfolk JA, et al. Human mast cells present antigen to autologous CD4(+) T cells. J Allergy Clin Immunol (2018) 141:311–321.e10. doi: 10.1016/j.jaci.2017.02.048
45. Carroll-Portillo A, Cannon JL, te Riet J, Holmes A, Kawakami Y, Kawakami T, et al. Mast cells and dendritic cells form synapses that facilitate antigen transfer for T cell activation. J Cell Biol (2015) 210:851–64. doi: 10.1083/jcb.201412074
46. Grencis R, Else K, Huntley J, Nishikawa S. The in vivo role of stem cell factor (c-kit ligand) on mastocytosis and host protective immunity to the intestinal nematode Trichinella spiralis in mice. Parasite Immunol (1993) 15:55–9. doi: 10.1111/j.1365-3024.1993.tb00572.x
47. Newlands G, Miller H, MacKellar A, Galli SJ. Stem cell factor contributes to intestinal mucosal mast cell hyperplasia in rats infected with Nippostrongylus brasiliensis or Trichinella spiralis, but anti-stem cell factor treatment decreases parasite egg production during N brasiliensis infection. (1995). doi: 10.1182/blood.V86.5.1968.bloodjournal8651968
48. Anthony RM, Rutitzky LI, Urban JF, Stadecker MJ, Gause WC. Protective immune mechanisms in helminth infection. Nat Rev Immunol (2007) 7:975–87. doi: 10.1038/nri2199
49. Galli SJ, Tsai M. Mast cells in allergy and infection: versatile effector and regulatory cells in innate and adaptive immunity. Eur J Immunol (2010) 40:1843–51. doi: 10.1002/eji.201040559
50. Mendez-Enriquez E, Hallgren J. Mast Cells and Their Progenitors in Allergic Asthma. Front Immunol (2019) 10:821. doi: 10.3389/fimmu.2019.00821
51. Jamur MC, Oliver C. Origin, maturation and recruitment of mast cell precursors. Front Biosci (Schol Ed) (2011) 3:1390–406. doi: 10.2741/231
52. Mukai K, Tsai M, Saito H, Galli SJ. Mast cells as sources of cytokines, chemokines, and growth factors. Immunol Rev (2018) 282:121–50. doi: 10.1111/imr.12634
53. Henry EK, Sy CB, Inclan-Rico JM, Espinosa V, Ghanny SS, Dwyer DF, et al. Carbonic anhydrase enzymes regulate mast cell-mediated inflammation. J Exp Med (2016) 213:1663–73. doi: 10.1084/jem.20151739
54. Inclan-Rico JM, Hernandez CM, Henry EK, Federman HG, Sy CB, Ponessa JJ, et al. Trichinella spiralis-induced mastocytosis and erythropoiesis are simultaneously supported by a bipotent mast cell/erythrocyte precursor cell. PloS Pathog (2020) 16:e1008579. doi: 10.1371/journal.ppat.1008579
55. Fallon PG, Ballantyne SJ, Mangan NE, Barlow JL, Dasvarma A, Hewett DR, et al. Identification of an interleukin (IL)-25–dependent cell population that provides IL-4, IL-5, and IL-13 at the onset of helminth expulsion. J Exp Med (2006) 203:1105–16. doi: 10.1084/jem.20051615
56. Hepworth MR, Daniłowicz-Luebert E, Rausch S, Metz M, Klotz C, Maurer M, et al. Mast cells orchestrate type 2 immunity to helminths through regulation of tissue-derived cytokines. Proc Natl Acad Sci (2012) 109:6644–9. doi: 10.1073/pnas.1112268109
57. Saenz SA, Siracusa MC, Perrigoue JG, Spencer SP, Urban JF Jr., Tocker JE, et al. IL25 elicits a multipotent progenitor cell population that promotes T(H)2 cytokine responses. Nature (2010) 464:1362–6. doi: 10.1038/nature08901
58. Knight PA, Wright SH, Lawrence CE, Paterson YY, Miller HR. Delayed Expulsion of the Nematode Trichinella spiralisIn Mice Lacking the Mucosal Mast Cell–Specific Granule Chymase, Mouse Mast Cell Protease-1. J Exp Med (2000) 192:1849–56. doi: 10.1084/jem.192.12.1849
59. Urban JF, Schopf L, Morris SC, Orekhova T, Madden KB, Betts CJ, et al. Stat6 signaling promotes protective immunity against Trichinella spiralis through a mast cell-and T cell-dependent mechanism. J Immunol (2000) 164:2046–52. doi: 10.4049/jimmunol.164.4.2046
60. Siracusa MC, Saenz SA, Wojno EDT, Kim BS, Osborne LC, Ziegler CG, et al. Thymic stromal lymphopoietin-mediated extramedullary hematopoiesis promotes allergic inflammation. Immunity (2013) 39:1158–70. doi: 10.1016/j.immuni.2013.09.016
61. Crowle PK, Reed ND. Rejection of the intestinal parasite Nippostrongylus brasiliensis by mast cell-deficient W/Wv anemic mice. Infection Immun (1981) 33:54–8. doi: 10.1128/iai.33.1.54-58.1981
62. Betts CJ, Else KJ. Mast cells, eosinophils and antibody-mediated cellular cytotoxicity are not critical in resistance to Trichuris muris. Parasite Immunol (1999) 21:45–52. doi: 10.1046/j.1365-3024.1999.00200.x
63. Macri S, Pavesi E, Crescitelli R, Aspesi A, Vizziello C, Botto C, et al. Immunophenotypic Profiling of Erythroid Progenitor-Derived Extracellular Vesicles in Diamond-Blackfan Anaemia: A New Diagnostic Strategy. PloS One (2015) 10:e0138200. doi: 10.1371/journal.pone.0138200
64. Challen GA, Boles N, Lin KK, Goodell MA. Mouse hematopoietic stem cell identification and analysis. Cytometry A (2009) 75:14–24. doi: 10.1002/cyto.a.20674
65. Akashi K, Traver D, Miyamoto T, Weissman IL. A clonogenic common myeloid progenitor that gives rise to all myeloid lineages. Nature (2000) 404:193–7. doi: 10.1038/35004599
66. Franco CB, Chen CC, Drukker M, Weissman IL, Galli SJ. Distinguishing mast cell and granulocyte differentiation at the single-cell level. Cell Stem Cell (2010) 6:361–8. doi: 10.1016/j.stem.2010.02.013
67. Dahlin JS, Heyman B, Hallgren J. Committed mast cell progenitors in mouse blood differ in maturity between Th1 and Th2 strains. Allergy (2013) 68:1333–7. doi: 10.1111/all.12223
68. Harris NL, Loke P. Recent Advances in Type-2-Cell-Mediated Immunity: Insights from Helminth Infection. Immunity (2017) 47:1024–36. doi: 10.1016/j.immuni.2017.11.015
69. Oyesola OO, Fruh SP, Webb LM, Tait Wojno ED. Cytokines and beyond: Regulation of innate immune responses during helminth infection. Cytokine (2020) 133:154527. doi: 10.1016/j.cyto.2018.08.021
70. Maizels RM. Regulation of immunity and allergy by helminth parasites. Allergy (2020) 75:524–34. doi: 10.1111/all.13944
71. Zheng S, Papalexi E, Butler A, Stephenson W, Satija R. Molecular transitions in early progenitors during human cord blood hematopoiesis. Mol Syst Biol (2018) 14:e8041. doi: 10.15252/msb.20178041
72. Gentek R, Ghigo C, Hoeffel G, Bulle MJ, Msallam R, Gautier G, et al. Hemogenic Endothelial Fate Mapping Reveals Dual Developmental Origin of Mast Cells. Immunity (2018) 48:1160–1171.e5. doi: 10.1016/j.immuni.2018.04.025
73. Saenz SA, Siracusa MC, Monticelli LA, Ziegler CG, Kim BS, Brestoff JR, et al. IL-25 simultaneously elicits distinct populations of innate lymphoid cells and multipotent progenitor type 2 (MPPtype2) cells. J Exp Med (2013) 210:1823–37. doi: 10.1084/jem.20122332
74. Giacomin PR, Siracusa MC, Walsh KP, Grencis RK, Kubo M, Comeau MR, et al. Thymic stromal lymphopoietin-dependent basophils promote Th2 cytokine responses following intestinal helminth infection. J Immunol (2012) 189:4371–8. doi: 10.4049/jimmunol.1200691
75. Peng J, Siracusa MC. Basophils in antihelminth immunity. Semin Immunol (2021) 53:101529. doi: 10.1016/j.smim.2021.101529
76. Arinobu Y, Iwasaki H, Gurish MF, Mizuno S, Shigematsu H, Ozawa H, et al. Developmental checkpoints of the basophil/mast cell lineages in adult murine hematopoiesis. Proc Natl Acad Sci U.S.A (2005) 102:18105–10.
77. Huang H, Li Y. Mechanisms controlling mast cell and basophil lineage decisions. Curr Allergy Asthma Rep (2014) 14:457. doi: 10.1007/s11882-014-0457-1
78. Inclan-Rico JM, Ponessa JJ, Valero-Pacheco N, Hernandez CM, Sy CB, Lemenze AD, et al. Basophils prime group 2 innate lymphoid cells for neuropeptide-mediated inhibition. Nat Immunol (2020) 21:1181–93. doi: 10.1038/s41590-020-0753-y
79. Pellefigues C, Naidoo K, Mehta P, Schmidt AJ, Jagot F, Roussel E, et al. Basophils promote barrier dysfunction and resolution in the atopic skin. J Allergy Clin Immunol (2021) 148:799–812 e10. doi: 10.1016/j.jaci.2021.02.018
80. Meulenbroeks C, van Weelden H, Schwartz C, Voehringer D, Redegeld FAM, Rutten V, et al. Basophil-derived amphiregulin is essential for UVB irradiation-induced immune suppression. J Invest Dermatol (2015) 135:222–8. doi: 10.1038/jid.2014.329
81. Sawaguchi M, Tanaka S, Nakatani Y, Harada Y, Mukai K, Matsunaga Y, et al. Role of mast cells and basophils in IgE responses and in allergic airway hyperresponsiveness. J Immunol (2012) 188:1809–18. doi: 10.4049/jimmunol.1101746
82. Webb LM, Oyesola OO, Fruh SP, Kamynina E, Still KM, Patel RK, et al. The Notch signaling pathway promotes basophil responses during helminth-induced type 2 inflammation. J Exp Med (2019) 216:1268–79. doi: 10.1084/jem.20180131
83. Siracusa MC, Saenz SA, Hill DA, Kim BS, Headley MB, Doering TA, et al. TSLP promotes interleukin-3-independent basophil haematopoiesis and type 2 inflammation. Nature (2011) 477:229–33. doi: 10.1038/nature10329
84. Schwartz C, Turqueti-Neves A, Hartmann S, Yu P, Nimmerjahn F, Voehringer D. Basophil-mediated protection against gastrointestinal helminths requires IgE-induced cytokine secretion. Proc Natl Acad Sci U.S.A (2014) 111:E5169–77. doi: 10.1073/pnas.1412663111
85. Reitz M, Brunn ML, Voehringer D, Breloer M. Basophils are dispensable for the establishment of protective adaptive immunity against primary and challenge infection with the intestinal helminth parasite Strongyloides ratti. PloS Negl Trop Dis (2018) 12:e0006992. doi: 10.1371/journal.pntd.0006992
86. Reitz M, Brunn ML, Rodewald HR, Feyerabend TB, Roers A, Dudeck A, et al. Mucosal mast cells are indispensable for the timely termination of Strongyloides ratti infection. Mucosal Immunol (2017) 10:481–92. doi: 10.1038/mi.2016.56
87. Ohnmacht C, Schwartz C, Panzer M, Schiedewitz I, Naumann R, Voehringer D. Basophils orchestrate chronic allergic dermatitis and protective immunity against helminths. Immunity (2010) 33:364–74. doi: 10.1016/j.immuni.2010.08.011
88. Mukai K, Karasuyama H, Kabashima K, Kubo M, Galli SJ. Differences in the Importance of Mast Cells, Basophils, IgE, and IgG versus That of CD4(+) T Cells and ILC2 Cells in Primary and Secondary Immunity to Strongyloides venezuelensis. Infect Immun 85 (2017) 85(5):e00053–17. doi: 10.1128/IAI.00053-17
89. Huang L, Appleton JA. Eosinophils in Helminth Infection: Defenders and Dupes. Trends Parasitol (2016) 32:798–807. doi: 10.1016/j.pt.2016.05.004
90. Mitre E, Klion AD. Eosinophils and helminth infection: protective or pathogenic? Semin Immunopathol (2021) 43:363–81. doi: 10.1007/s00281-021-00870-z
91. Maxwell C, Hussain R, Nutman TB, Poindexter RW, Little MD, Schad GA, et al. The clinical and immunologic responses of normal human volunteers to low dose hookworm (Necator americanus) infection. Am J Trop Med Hyg (1987) 37:126–34. doi: 10.4269/ajtmh.1987.37.126
92. Lammas DA, Wakelin D, Mitchell LA, Tuohy M, Else KJ, Grencis RK. Genetic influences upon eosinophilia and resistance in mice infected with Trichinella spiralis. Parasitology 105 ( Pt (1992) 1):117–24. doi: 10.1017/S0031182000073765
93. Rajamanickam A, Munisankar S, Bhootra Y, Dolla CK, Nutman TB, Babu S. Elevated Systemic Levels of Eosinophil, Neutrophil, and Mast Cell Granular Proteins in Strongyloides Stercoralis Infection that Diminish following Treatment. Front Immunol (2018) 9:207. doi: 10.3389/fimmu.2018.00207
94. Kita H. Eosinophils: multifaceted biological properties and roles in health and disease. Immunol Rev (2011) 242:161–77. doi: 10.1111/j.1600-065X.2011.01026.x
95. Ehrens A, Rudiger N, Heepmann L, Linnemann L, Hartmann W, Hubner MP, et al. Eosinophils and Neutrophils Eliminate Migrating Strongyloides ratti Larvae at the Site of Infection in the Context of Extracellular DNA Trap Formation. Front Immunol (2021) 12:715766. doi: 10.3389/fimmu.2021.715766
96. Inclan-Rico JM, Ponessa JJ, Siracusa MC. Contributions of innate lymphocytes to allergic responses. Curr Opin Allergy Clin Immunol (2019) 19:175–84. doi: 10.1097/ACI.0000000000000515
97. Hung LY, Lewkowich IP, Dawson LA, Downey J, Yang Y, Smith DE, et al. IL-33 drives biphasic IL-13 production for noncanonical Type 2 immunity against hookworms. Proc Natl Acad Sci U.S.A (2013) 110:282–7.
98. Vallance BA, Matthaei KI, Sanovic S, Young IG, Collins SM. Interleukin-5 deficient mice exhibit impaired host defence against challenge Trichinella spiralis infections. Parasite Immunol (2000) 22:487–92. doi: 10.1046/j.1365-3024.2000.00328.x
99. Knott ML, Matthaei KI, Giacomin PR, Wang H, Foster PS, Dent LA. Impaired resistance in early secondary Nippostrongylus brasiliensis infections in mice with defective eosinophilopoeisis. Int J Parasitol (2007) 37:1367–78. doi: 10.1016/j.ijpara.2007.04.006
100. Stein LH, Redding KM, Lee JJ, Nolan TJ, Schad GA, Lok JB, et al. Eosinophils utilize multiple chemokine receptors for chemotaxis to the parasitic nematode Strongyloides stercoralis. J Innate Immun (2009) 1:618–30. doi: 10.1159/000233235
101. Magalhaes KG, Luna-Gomes T, Mesquita-Santos F, Correa R, Assuncao LS, Atella GC, et al. Schistosomal Lipids Activate Human Eosinophils via Toll-Like Receptor 2 and PGD2 Receptors: 15-LO Role in Cytokine Secretion. Front Immunol (2018) 9:3161. doi: 10.3389/fimmu.2018.03161
102. Mukherjee M, Lacy P, Ueki S. Eosinophil Extracellular Traps and Inflammatory Pathologies-Untangling the Web! Front Immunol (2018) 9:2763. doi: 10.3389/fimmu.2018.02763
103. Motran CC, Ambrosio LF, Volpini X, Celias DP, Cervi L. Dendritic cells and parasites: from recognition and activation to immune response instruction. Semin Immunopathol (2017) 39:199–213. doi: 10.1007/s00281-016-0588-7
104. Kumar S, Jeong Y, Ashraf MU, Bae YS. Dendritic Cell-Mediated Th2 Immunity and Immune Disorders. Int J Mol Sci (2019) 20(9):2159. doi: 10.3390/ijms20092159
105. Mendez-Samperio P. Molecular events by which dendritic cells promote Th2 immune protection in helmith infection. Infect Dis (Lond) (2016) 48:715–20. doi: 10.1080/23744235.2016.1194529
106. Langelaar M, Aranzamendi C, Franssen F, van der Giessen J, Rutten V, van der Ley P, et al. Suppression of dendritic cell maturation by Trichinella spiralis excretory/secretory products. Parasite Immunol (2009) 31:641–5. doi: 10.1111/j.1365-3024.2009.01136.x
107. Jin X, Yang Y, Liu X, Shi H, Cai X, Luo X, et al. Glutathione-S-transferase of Trichinella spiralis regulates maturation and function of dendritic cells. Parasitology (2019) 146:1725–32. doi: 10.1017/S003118201900115X
108. Jin X, Yang Y, Bai X, Shi H, Zhang W, Zhang Z, et al. Dendritic cells treated by Trichinella spiralis muscle larval excretory/secretory products alleviate TNBS-induced colitis in mice. Int Immunopharmacol (2019) 70:378–86. doi: 10.1016/j.intimp.2019.02.028
109. Ding J, Liu X, Tang B, Bai X, Wang Y, Li S, et al. Murine hepatoma treatment with mature dendritic cells stimulated by Trichinella spiralis excretory/secretory products. Parasite (2020) 27:47. doi: 10.1051/parasite/2020045
110. Connor LM, Tang SC, Cognard E, Ochiai S, Hilligan KL, Old SI, et al. Th2 responses are primed by skin dendritic cells with distinct transcriptional profiles. J Exp Med (2017) 214:125–42. doi: 10.1084/jem.20160470
111. Webb LM, Lundie RJ, Borger JG, Brown SL, Connor LM, Cartwright AN, et al. Type I interferon is required for T helper (Th) 2 induction by dendritic cells. EMBO J (2017) 36:2404–18. doi: 10.15252/embj.201695345
112. Yin X, Chen S, Eisenbarth SC. Dendritic Cell Regulation of T Helper Cells. Annu Rev Immunol (2021) 39:759–90. doi: 10.1146/annurev-immunol-101819-025146
113. Schlitzer A, Sivakamasundari V, Chen J, Sumatoh HR, Schreuder J, Lum J, et al. Identification of cDC1- and cDC2-committed DC progenitors reveals early lineage priming at the common DC progenitor stage in the bone marrow. Nat Immunol (2015) 16:718–28. doi: 10.1038/ni.3200
114. Cook PC, Jones LH, Jenkins SJ, Wynn TA, Allen JE, MacDonald AS. Alternatively activated dendritic cells regulate CD4+ T-cell polarization in vitro and in vivo. Proc Natl Acad Sci U.S.A (2012) 109:9977–82.
115. Jenkins SJ, Allen JE. The expanding world of tissue-resident macrophages. Eur J Immunol (2021) 51:1882–96. doi: 10.1002/eji.202048881
116. Gao Y, Nish SA, Jiang R, Hou L, Licona-Limon P, Weinstein JS, et al. Control of T helper 2 responses by transcription factor IRF4-dependent dendritic cells. Immunity (2013) 39:722–32. doi: 10.1016/j.immuni.2013.08.028
117. Kumamoto Y, Linehan M, Weinstein JS, Laidlaw BJ, Craft JE, Iwasaki A. CD301b(+) dermal dendritic cells drive T helper 2 cell-mediated immunity. Immunity (2013) 39:733–43. doi: 10.1016/j.immuni.2013.08.029
118. Halim TY, Hwang YY, Scanlon ST, Zaghouani H, Garbi N, Fallon PG, et al. Group 2 innate lymphoid cells license dendritic cells to potentiate memory TH2 cell responses. Nat Immunol (2016) 17:57–64. doi: 10.1038/ni.3294
119. Mayer JU, Demiri M, Agace WW, MacDonald AS, Svensson-Frej M, Milling SW. Different populations of CD11b(+) dendritic cells drive Th2 responses in the small intestine and colon. Nat Commun (2017) 8:15820. doi: 10.1038/ncomms15820
120. Webb LM, Phythian-Adams AT, Costain AH, Brown SL, Lundie RJ, Forde-Thomas J, et al. Plasmacytoid Dendritic Cells Facilitate Th Cell Cytokine Responses throughout Schistosoma mansoni Infection. Immunohorizons (2021) 5:721–32. doi: 10.4049/immunohorizons.2100071
121. Chen F, Wu W, Millman A, Craft JF, Chen E, Patel N, et al. Neutrophils prime a long-lived effector macrophage phenotype that mediates accelerated helminth expulsion. Nat Immunol (2014) 15:938–46. doi: 10.1038/ni.2984
122. Sutherland TE, Logan N, Ruckerl D, Humbles AA, Allan SM, Papayannopoulos V, et al. Chitinase-like proteins promote IL-17-mediated neutrophilia in a tradeoff between nematode killing and host damage. Nat Immunol (2014) 15:1116–25. doi: 10.1038/ni.3023
123. Bouchery T, Moyat M, Sotillo J, Silverstein S, Volpe B, Coakley G, et al. Hookworms Evade Host Immunity by Secreting a Deoxyribonuclease to Degrade Neutrophil Extracellular Traps. Cell Host Microbe (2020) 27:277–289 e6. doi: 10.1016/j.chom.2020.01.011
124. Chen F, Liu Z, Wu W, Rozo C, Bowdridge S, Millman A, et al. An essential role for TH2-type responses in limiting acute tissue damage during experimental helminth infection. Nat Med (2012) 18:260–6. doi: 10.1038/nm.2628
125. O'Connell AE, Redding KM, Hess JA, Lok JB, Nolan TJ, Abraham D. Soluble extract from the nematode Strongyloides stercoralis induces CXCR2 dependent/IL-17 independent neutrophil recruitment. Microbes Infect (2011) 13:536–44. doi: 10.1016/j.micinf.2011.01.016
126. Little MC, Bell LV, Cliffe LJ, Else KJ. The characterization of intraepithelial lymphocytes, lamina propria leukocytes, and isolated lymphoid follicles in the large intestine of mice infected with the intestinal nematode parasite Trichuris muris. J Immunol (2005) 175:6713–22. doi: 10.4049/jimmunol.175.10.6713
127. Bozic F, Marinculic A, Durakovic E. Analysis of intestinal intraepithelial lymphocyte populations in experimental Trichinella spiralis infection of mice. Folia Parasitol (Praha) (2000) 47:55–9. doi: 10.14411/fp.2000.010
128. Bonne-Annee S, Kerepesi LA, Hess JA, O'Connell AE, Lok JB, Nolan TJ, et al. Human and mouse macrophages collaborate with neutrophils to kill larval Strongyloides stercoralis. Infect Immun (2013) 81:3346–55. doi: 10.1128/IAI.00625-13
129. Bonne-Annee S, Kerepesi LA, Hess JA, Wesolowski J, Paumet F, Lok JB, et al. Extracellular traps are associated with human and mouse neutrophil and macrophage mediated killing of larval Strongyloides stercoralis. Microbes Infect (2014) 16:502–11. doi: 10.1016/j.micinf.2014.02.012
130. Chen F, El-Naccache DW, Ponessa JJ, Lemenze A, Espinosa V, Wu W, et al. Helminth resistance is mediated by differential activation of recruited monocyte-derived alveolar macrophages and arginine depletion. Cell Rep (2022) 38:110215. doi: 10.1016/j.celrep.2021.110215
131. Chauhan A, Sharma A, Tripathi JK, Sun Y, Sukumran P, Singh BB, et al. Helminth derived factors inhibit neutrophil extracellular trap formation and inflammation in bacterial peritonitis. Sci Rep (2021) 11:12718. doi: 10.1038/s41598-021-92001-9
132. Coakley G, Harris NL. Interactions between macrophages and helminths. Parasite Immunol (2020) 42:e12717. doi: 10.1111/pim.12717
133. Cortes-Selva D, Fairfax K. Schistosome and intestinal helminth modulation of macrophage immunometabolism. Immunology (2021) 162:123–34. doi: 10.1111/imm.13231
134. Wolde M, Laan LC, Medhin G, Gadissa E, Berhe N, Tsegaye A. Human Monocytes/Macrophage Inflammatory Cytokine Changes Following in vivo and in vitro Schistomam manoni Infection. J Inflammation Res (2020) 13:35–43. doi: 10.2147/JIR.S233381
135. Lechner A, Bohnacker S, Esser-von Bieren J. Macrophage regulation & function in helminth infection. Semin Immunol (2021) 53:101526. doi: 10.1016/j.smim.2021.101526
136. Reece JJ, Siracusa MC, Scott AL. Innate immune responses to lung-stage helminth infection induce alternatively activated alveolar macrophages. Infect Immun (2006) 74:4970–81. doi: 10.1128/IAI.00687-06
137. Herbert DR, Holscher C, Mohrs M, Arendse B, Schwegmann A, Radwanska M, et al. Alternative macrophage activation is essential for survival during schistosomiasis and downmodulates T helper 1 responses and immunopathology. Immunity (2004) 20:623–35. doi: 10.1016/S1074-7613(04)00107-4
138. Anthony RM, Urban JF Jr., Alem F, Hamed HA, Rozo CT, Boucher JL, et al. Memory T(H)2 cells induce alternatively activated macrophages to mediate protection against nematode parasites. Nat Med (2006) 12:955–60. doi: 10.1038/nm1451
139. Rodriguez-Sosa M, Satoskar AR, Calderon R, Gomez-Garcia L, Saavedra R, Bojalil R, et al. Chronic helminth infection induces alternatively activated macrophages expressing high levels of CCR5 with low interleukin-12 production and Th2-biasing ability. Infect Immun (2002) 70:3656–64. doi: 10.1128/IAI.70.7.3656-3664.2002
140. Dzik JM, Golos B, Jagielska E, Zielinski Z, Walajtys-Rode E. A non-classical type of alveolar macrophage response to Trichinella spiralis infection. Parasite Immunol (2004) 26:197–205. doi: 10.1111/j.0141-9838.2004.00700.x
141. Donnelly S, O'Neill SM, Sekiya M, Mulcahy G, Dalton JP. Thioredoxin peroxidase secreted by Fasciola hepatica induces the alternative activation of macrophages. Infect Immun (2005) 73:166–73. doi: 10.1128/IAI.73.1.166-173.2005
142. Oshiro TM, Macedo MS, Macedo-Soares MF. Anti-inflammatory activity of PAS-1, a protein component of Ascaris suum. Inflammation Res (2005) 54:17–21. doi: 10.1007/s00011-004-1316-7
143. Nair MG, Cochrane DW, Allen JE. Macrophages in chronic type 2 inflammation have a novel phenotype characterized by the abundant expression of Ym1 and Fizz1 that can be partly replicated in vitro. Immunol Lett (2003) 85:173–80. doi: 10.1016/S0165-2478(02)00225-0
144. Svensson M, Bell L, Little MC, DeSchoolmeester M, Locksley RM, Else KJ. Accumulation of eosinophils in intestine-draining mesenteric lymph nodes occurs after Trichuris muris infection. Parasite Immunol (2011) 33:1–11. doi: 10.1111/j.1365-3024.2010.01246.x
145. van Panhuys N, Prout M, Forbes E, Min B, Paul WE, Le Gros G. Basophils are the major producers of IL-4 during primary helminth infection. J Immunol (2011) 186:2719–28. doi: 10.4049/jimmunol.1000940
146. Koyasu S, Moro K, Tanabe M, Takeuchi T. Natural helper cells: a new player in the innate immune response against helminth infection. Adv Immunol (2010) 108:21–44. doi: 10.1016/B978-0-12-380995-7.00002-1
147. Jackson-Jones LH, Ruckerl D, Svedberg F, Duncan S, Maizels RM, Sutherland TE, et al. IL-33 delivery induces serous cavity macrophage proliferation independent of interleukin-4 receptor alpha. Eur J Immunol (2016) 46:2311–21. doi: 10.1002/eji.201646442
148. Schroder WA, Le TT, Major L, Street S, Gardner J, Lambley E, et al. A physiological function of inflammation-associated SerpinB2 is regulation of adaptive immunity. J Immunol (2010) 184:2663–70. doi: 10.4049/jimmunol.0902187
149. Gundra UM, Girgis NM, Gonzalez MA, San Tang M, van der Zande HJP, et al. Vitamin A mediates conversion of monocyte-derived macrophages into tissue-resident macrophages during alternative activation. Nat Immunol (2017) 18:642–53. doi: 10.1038/ni.3734
150. Damle SR, Martin RK, Cross JV, Conrad DH. Macrophage migration inhibitory factor deficiency enhances immune response to Nippostrongylus brasiliensis. Mucosal Immunol (2017) 10:205–14. doi: 10.1038/mi.2016.29
151. Bouchery T, Kyle R, Camberis M, Shepherd A, Filbey K, Smith A, et al. ILC2s and T cells cooperate to ensure maintenance of M2 macrophages for lung immunity against hookworms. Nat Commun (2015) 6:6970. doi: 10.1038/ncomms7970
152. Schyns J, Bai Q, Ruscitti C, Radermecker C, De Schepper S, Chakarov S, et al. Non-classical tissue monocytes and two functionally distinct populations of interstitial macrophages populate the mouse lung. Nat Commun (2019) 10:3964. doi: 10.1038/s41467-019-11843-0
153. Chakarov S, Lim HY, Tan L, Lim SY, See P, Lum J, et al. Two distinct interstitial macrophage populations coexist across tissues in specific subtissular niches. Sci 363 (2019) 363(6432):eaau0964. doi: 10.1126/science.aau0964
154. Lavin Y, Winter D, Blecher-Gonen R, David E, Keren-Shaul H, Merad M, et al. Tissue-resident macrophage enhancer landscapes are shaped by the local microenvironment. Cell (2014) 159:1312–26. doi: 10.1016/j.cell.2014.11.018
155. Gosselin D, Link VM, Romanoski CE, Fonseca GJ, Eichenfield DZ, Spann NJ, et al. Environment drives selection and function of enhancers controlling tissue-specific macrophage identities. Cell (2014) 159:1327–40. doi: 10.1016/j.cell.2014.11.023
156. Gautier EL, Shay T, Miller J, Greter M, Jakubzick C, Ivanov S, et al. Gene-expression profiles and transcriptional regulatory pathways that underlie the identity and diversity of mouse tissue macrophages. Nat Immunol (2012) 13:1118–28. doi: 10.1038/ni.2419
157. Dick SA, Wong A, Hamidzada H, Nejat S, Nechanitzky R, Vohra S, et al. Three tissue resident macrophage subsets coexist across organs with conserved origins and life cycles. Sci Immunol 7 (2022):eabf7777. doi: 10.1126/sciimmunol.abf7777
158. Jenkins SJ, Ruckerl D, Cook PC, Jones LH, Finkelman FD, van Rooijen N, et al. Local macrophage proliferation, rather than recruitment from the blood, is a signature of TH2 inflammation. Science (2011) 332:1284–8. doi: 10.1126/science.1204351
159. Loke P, Lin JD. Redefining inflammatory macrophage phenotypes across stages and tissues by single-cell transcriptomics. Sci Immunol 7 (2022):eabo4652. doi: 10.1126/sciimmunol.abo4652
160. Thapaliya M, Chompunud Na Ayudhya C, Amponnawarat A, Roy S, Ali H. Mast Cell-Specific MRGPRX2: a Key Modulator of Neuro-Immune Interaction in Allergic Diseases. Curr Allergy Asthma Rep (2021) 21:3. doi: 10.1007/s11882-020-00979-5
161. Gundra UM, Girgis NM, Ruckerl D, Jenkins S, Ward LN, et al. Alternatively activated macrophages derived from monocytes and tissue macrophages are phenotypically and functionally distinct. Blood (2014) 123:e110–22. doi: 10.1182/blood-2013-08-520619
162. Stevenson MM, Valanparambil RM, Tam M. Myeloid-Derived Suppressor Cells: The Expanding World of Helminth Modulation of the Immune System. Front Immunol (2022) 13:874308. doi: 10.3389/fimmu.2022.874308
163. Veglia F, Sanseviero E, Gabrilovich DI. Myeloid-derived suppressor cells in the era of increasing myeloid cell diversity. Nat Rev Immunol (2021) 21:485–98. doi: 10.1038/s41577-020-00490-y
164. Vivier E, Artis D, Colonna M, Diefenbach A, Di Santo JP, Eberl G, et al. Innate Lymphoid Cells: 10 Years On. Cell (2018) 174:1054–66. doi: 10.1016/j.cell.2018.07.017
165. Gentile ME, Li Y, Robertson A, Shah K, Fontes G, Kaufmann E, et al. NK cell recruitment limits tissue damage during an enteric helminth infection. Mucosal Immunol (2020) 13:357–70. doi: 10.1038/s41385-019-0231-8
166. Hu Y, Wang X, Wei Y, Liu H, Zhang J, Shen Y, et al. Functional Inhibition of Natural Killer Cells in a BALB/c Mouse Model of Liver Fibrosis Induced by Schistosoma japonicum Infection. Front Cell Infect Microbiol (2020) 10:598987. doi: 10.3389/fcimb.2020.598987
167. Faveeuw C, Mallevaey T, Trottein F. Role of natural killer T lymphocytes during helminthic infection. Parasite (2008) 15:384–8. doi: 10.1051/parasite/2008153384
168. X B, Huang H, Gao S, Xu X. Echinococcus multilocularis induces surface high expression of inhibitory killer immunoglobulin-like receptor on natural killer cells. Allergol Immunopathol (Madr) (2021) 49:78–86. doi: 10.15586/aei.v49i5.465
169. Khetsuphan T, Chaisri U, Dechkhajorn W, Benjathummarak S, Dekumyoy P, Ampawong S, et al. Effects of Gnathostoma spinigerum infective stage larva excretory-secretory products on NK cells in peripheral blood mononuclear cell culture: focused on expressions of IFN-gamma and killer cell lectin-like receptors. Parasitol Res (2020) 119:1011–21. doi: 10.1007/s00436-019-06593-3
170. Douglas B, Oyesola O, Cooper MM, Posey A, Tait Wojno E, Giacomin PR, et al. Immune System Investigation Using Parasitic Helminths. Annu Rev Immunol (2021) 39:639–65. doi: 10.1146/annurev-immunol-093019-122827
171. Pelly VS, Kannan Y, Coomes SM, Entwistle LJ, Ruckerl D, Seddon B, et al. IL-4-producing ILC2s are required for the differentiation of TH2 cells following Heligmosomoides polygyrus infection. Mucosal Immunol (2016) 9:1407–17. doi: 10.1038/mi.2016.4
172. Turner JE, Morrison PJ, Wilhelm C, Wilson M, Ahlfors H, Renauld JC, et al. IL-9-mediated survival of type 2 innate lymphoid cells promotes damage control in helminth-induced lung inflammation. J Exp Med (2013) 210:2951–65. doi: 10.1084/jem.20130071
173. Karagiannis F, Masouleh SK, Wunderling K, Surendar J, Schmitt V, Kazakov A, et al. Lipid-Droplet Formation Drives Pathogenic Group 2 Innate Lymphoid Cells in Airway Inflammation. Immunity (2020) 52:620–634 e6. doi: 10.1016/j.immuni.2020.03.003
174. Siracusa MC, Reece JJ, Urban JF Jr., Scott AL. Dynamics of lung macrophage activation in response to helminth infection. J Leukoc Biol (2008) 84:1422–33. doi: 10.1189/jlb.0308199
175. Lloyd CM, Snelgrove RJ. Type 2 immunity: Expanding our view. Sci Immunol 3 (2018) 3(25):eaat1604. doi: 10.1126/sciimmunol.aat1604
176. Doherty TA, Khorram N, Lund S, Mehta AK, Croft M, Broide DH. Lung type 2 innate lymphoid cells express cysteinyl leukotriene receptor 1, which regulates TH2 cytokine production. J Allergy Clin Immunol (2013) 132:205–13. doi: 10.1016/j.jaci.2013.03.048
177. Herbert DR, Douglas B, Zullo K. Group 2 Innate Lymphoid Cells (ILC2): Type 2 Immunity and Helminth Immunity. Int J Mol Sci 20 (2019) 20(9):2276. doi: 10.3390/ijms20092276
178. Bouchery T, Le Gros G, Harris N. ILC2s-Trailblazers in the Host Response Against Intestinal Helminths. Front Immunol (2019) 10:623. doi: 10.3389/fimmu.2019.00623
179. Miller MM, Reinhardt RL, Heterogeneity T. Origins, and Impact of Migratory iILC2 Cells in Anti-helminth Immunity. Front Immunol (2020) 11:1594. doi: 10.3389/fimmu.2020.01594
180. Tan CL, Knight ZA. Regulation of Body Temperature by the Nervous System. Neuron (2018) 98:31–48. doi: 10.1016/j.neuron.2018.02.022
181. Myers MG Jr., Affinati AH, Richardson N, Schwartz MW. Central nervous system regulation of organismal energy and glucose homeostasis. Nat Metab (2021) 3:737–50. doi: 10.1038/s42255-021-00408-5
182. Moriyama S, Brestoff JR, Flamar AL, Moeller JB, Klose CSN, Rankin LC, et al. beta2-adrenergic receptor-mediated negative regulation of group 2 innate lymphoid cell responses. Science (2018) 359:1056–61. doi: 10.1126/science.aan4829
183. Nagashima H, Mahlakoiv T, Shih HY, Davis FP, Meylan F, Huang Y, et al. Neuropeptide CGRP Limits Group 2 Innate Lymphoid Cell Responses and Constrains Type 2 Inflammation. Immunity (2019) 51:682–695 e6. doi: 10.1016/j.immuni.2019.06.009
184. Chu C, Parkhurst CN, Zhang W, Zhou L, Yano H, Arifuzzaman M, et al. The ChAT-acetylcholine pathway promotes group 2 innate lymphoid cell responses and anti-helminth immunity. Sci Immunol 6 (2021) 6(57):eabe3218. doi: 10.1126/sciimmunol.abe3218
185. Klose CSN, Mahlakoiv T, Moeller JB, Rankin LC, Flamar AL, Kabata H, et al. The neuropeptide neuromedin U stimulates innate lymphoid cells and type 2 inflammation. Nature (2017) 549:282–6. doi: 10.1038/nature23676
186. Chu C, Artis D, Chiu IM. Neuro-immune Interactions in the Tissues. Immunity (2020) 52:464–74. doi: 10.1016/j.immuni.2020.02.017
187. Cardoso V, Chesne J, Ribeiro H, Garcia-Cassani B, Carvalho T, Bouchery T, et al. Neuronal regulation of type 2 innate lymphoid cells via neuromedin U. Nature (2017) 549:277–81. doi: 10.1038/nature23469
188. McNeil BD, Pundir P, Meeker S, Han L, Undem BJ, Kulka M, et al. Identification of a mast-cell-specific receptor crucial for pseudo-allergic drug reactions. Nature (2015) 519:237–41. doi: 10.1038/nature14022
189. Xu H, Shi X, Li X, Zou J, Zhou C, Liu W, et al. Neurotransmitter and neuropeptide regulation of mast cell function: a systematic review. J Neuroinflamm (2020) 17:356. doi: 10.1186/s12974-020-02029-3
190. Inclan-Rico JM, Kim BS, Abdus-Saboor I. Beyond somatosensation: Mrgprs in mucosal tissues. Neurosci Lett (2021) 748:135689. doi: 10.1016/j.neulet.2021.135689
191. Tatemoto K, Nozaki Y, Tsuda R, Konno S, Tomura K, Furuno M, et al. Immunoglobulin E-independent activation of mast cell is mediated by Mrg receptors. Biochem Biophys Res Commun (2006) 349:1322–8. doi: 10.1016/j.bbrc.2006.08.177
192. Babina M, Guhl S, Artuc M, Zuberbier T. Allergic FcepsilonRI- and pseudo-allergic MRGPRX2-triggered mast cell activation routes are independent and inversely regulated by SCF. Allergy (2018) 73:256–60. doi: 10.1111/all.13301
193. Arifuzzaman M, Mobley YR, Choi HW, Bist P, Salinas CA, Brown ZD, et al. MRGPR-mediated activation of local mast cells clears cutaneous bacterial infection and protects against reinfection. Sci Adv 5 (2019):eaav0216. doi: 10.1126/sciadv.aav0216
194. Zhang S, Edwards TN, Chaudhri VK, Wu J, Cohen JA, Hirai T, et al. Nonpeptidergic neurons suppress mast cells via glutamate to maintain skin homeostasis. Cell (2021) 184:2151–2166 e16. doi: 10.1016/j.cell.2021.03.002
195. Li X, Yang H, Han Y, Yin S, Shen B, Wu Y, et al. Tick peptides evoke itch by activating MrgprC11/MRGPRX1 to sensitize TRPV1 in pruriceptors. J Allergy Clin Immunol (2021) 147:2236–2248 e16. doi: 10.1016/j.jaci.2020.12.626
196. Meixiong J, Anderson M, Limjunyawong N, Sabbagh MF, Hu E, Mack MR, et al. Activation of Mast-Cell-Expressed Mas-Related G-Protein-Coupled Receptors Drives Non-histaminergic Itch. Immunity (2019) 50:1163–1171 e5. doi: 10.1016/j.immuni.2019.03.013
Keywords: innate immune cells, neuro-immune crosstalk, innate immunity, antihelminth immunity, host protection
Citation: Peng J, Federman HG, Hernandez CM and Siracusa MC (2022) Communication is key: Innate immune cells regulate host protection to helminths. Front. Immunol. 13:995432. doi: 10.3389/fimmu.2022.995432
Received: 15 July 2022; Accepted: 30 August 2022;
Published: 26 September 2022.
Edited by:
Lauren Webb, University of Washington, United StatesReviewed by:
Manuel Ritter, University Hospital Bonn, GermanyCaroline Sokol, Massachusetts General Hospital and Harvard Medical School, United States
Copyright © 2022 Peng, Federman, Hernandez and Siracusa. This is an open-access article distributed under the terms of the Creative Commons Attribution License (CC BY). The use, distribution or reproduction in other forums is permitted, provided the original author(s) and the copyright owner(s) are credited and that the original publication in this journal is cited, in accordance with accepted academic practice. No use, distribution or reproduction is permitted which does not comply with these terms.
*Correspondence: Mark C. Siracusa, mark.siracusa@njms.rutgers.edu