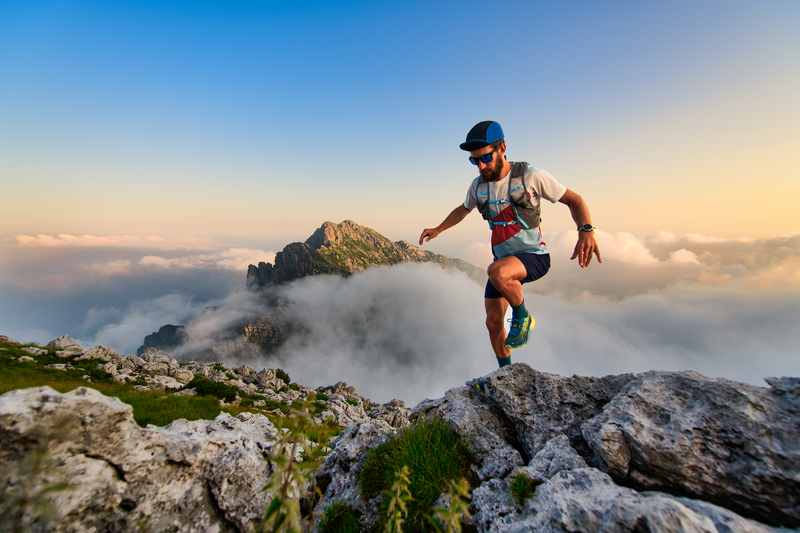
95% of researchers rate our articles as excellent or good
Learn more about the work of our research integrity team to safeguard the quality of each article we publish.
Find out more
REVIEW article
Front. Immunol. , 20 October 2022
Sec. Cancer Immunity and Immunotherapy
Volume 13 - 2022 | https://doi.org/10.3389/fimmu.2022.994319
Monocytes in peripheral blood circulation are the precursor of essential cells that control tumor progression, that include tumor-associated macrophages (TAMs), dendritic cells (DCs) and myeloid-derive suppressor cells (MDSC). Monocytes-derived cells orchestrate immune reactions in tumor microenvironment that control disease outcome and efficiency of cancer therapy. Four major types of anti-cancer therapy, surgery, radiotherapy, chemotherapy, and most recent immunotherapy, affect tumor-associated macrophage (TAM) polarization and functions. TAMs can also decrease the efficiency of therapy in a tumor-specific way. Monocytes is a major source of TAMs, and are recruited to tumor mass from the blood circulation. However, the mechanisms of monocyte programming in circulation by different therapeutic onsets are only emerging. In our review, we present the state-of-the art about the effects of anti-cancer therapy on monocyte progenitors and their dedifferentiation, on the content of monocyte subpopulations and their transcriptional programs in the circulation, on their recruitment into tumor mass and their potential to give origin for TAMs in tumor-specific microenvironment. We have also summarized very limited available knowledge about genetics that can affect monocyte interaction with cancer therapy, and highlighted the perspectives for the therapeutic targeting of circulating monocytes in cancer patients. We summarized the knowledge about the mediators that affect monocytes fate in all four types of therapies, and we highlighted the perspectives for targeting monocytes to develop combined and minimally invasive anti-cancer therapeutic approaches.
The immune systems can both cooperate with cancer therapy as well as interfere with its effects (1). Monocytes give origin to tumor-associated macrophages (TAM), dendritic cells (DC), and myeloid-derived suppressor cells (MDSC) that control immune reactions and cancer cell biology in tumor microenvironment (TME) (2–4). The functions of these cell types are controlled on the transcriptional, epigenetic, and metabolic levels (5). The differentiation of monocytes after their migration into tissues affects their descendants function and significantly affects efficiency of adaptive immune response, intramural immune status, level of angiogenesis, proliferation of cancer cells (6–9). TAMs are the most diverse and multifunctional orchestrators of cell-cell and cell-matrix interactions in TME. TAMs are programmed on the transcriptional, epigenetic and metabolic levels by multiple factors released by cancer cells, endothelial cells, fibroblasts, innate and adaptive immune cells in TME (5). In the majority of cancers, including breast, lung, prostate, and ovarian cancer, TAM substantially support tumor progression (6). TAMs secreted multiple angiogenic regulators that belong to growth factor family, S100 protein family, soluble ECM regulators, chitinase like proteins and others (10). TAMs can respond to and process number of chemotherapeutic agents, and significantly impair their effects on cancer cells (11). DCs are the main antigen-presenting cells for induction of adaptive immune responses (12). Monocytes can meet cancer-related circulating factors during the time they spend in blood, and can acquire detrimental for the patient programs transferred to tumor tissue and affecting differentiation of TAMs, DCs and MDSCs (13–15). Our understanding of monocytes has advanced when scientist started to consider these cells not as a homogenous population but as heterogeneous system that display responses to different stimuli (7, 16, 17). In cancer, monocyte subsets possess diverse activities that contribute to both pro‐ and anti-tumoral immune response, including phagocytosis, secretion of tumoricidal mediators, angiogenesis, remodeling of the extracellular matrix, recruitment of lymphocytes, as well as differentiation into TAM, MDSCs and DCs (2, 7–9, 18–21).
Human monocytes are a highly heterogenic population of cells, which can be defined by various markers, such as HLA-DR, CX3CR1, CCR2, CCR7, CD62L, Tie2, CD33, CD86, CD141, CD206, and others (22–24). The diversity of monocytes was firstly reflected in the nomenclature developed in the 1980s using 2‐color flow cytometric detection of two universal markers, CD14 and CD16, on human PBMCs (25–27). It allowed to identify 3 subpopulations – classical (CD14++16-), intermediate (CD14+16+) and non-classical (CD14+16++) monocytes (28–30). Later, other functional molecules were found that helped to identify further specific subpopulations of monocytes (Table 1). To date, the molecular and functional characteristics of monocyte subpopulations that give origin specifically to TAMs, DCs and/or MDSCs in tumor tissues remains to be unclear.
Multilevel molecular interactions control monocyte functions (55). Genetic alterations can affect pro- or anticancer potential of monocytes. Genetic polymorphisms of genes coding key cytokines and chemokines, such as factor for monocyte differentiation (macrophage colony-stimulating factor, M-CSF), factors for monocyte recruitment (monocyte chemoattractant protein-1 (MCP-1 or CCL2), CCR5 and CXCR4), are associated with breast, endometrial, oral, lung, prostate and bladder cancers (56–62).
As critical parameters of inflammation, amounts of monocytes or lymphocyte-monocytes ratio (LMR), and content of circulating monocytes subpopulations compositions can have diagnostic and prognostic significance (summarized in Table 1) (31, 32, 36–49, 63–68). Unambiguously attractive is the fact that monocytes circulate in blood that makes them minimally invasive biomarkers and accessible cell targets for therapy. Identification of critical molecular mechanisms regulating monocyte programs is needed to find new therapeutic targets. Recent studies using high throughput methods showed that transcriptional alterations in peripheral blood monocytes can serve as diagnostic, predictive and prognostic biomarkers in renal, colorectal, breast, cervical, skin, thyroid, hepatocellular and lung cancers (summarized in Table 1) (14, 15, 21, 49, 50, 69, 70).
The main goal of antitumor therapy is to achieve the maximal cytoreduction of primary tumor and metastatic foci (71). The increase of anti-tumor treatment efficiency is a major challenge in oncology. Drug resistance, related to tumor cell activity, is the main obstacle leading to inability to achieve pathological complete response (pCR). However, the outcome of cytostatic treatment significantly depends not only on the biological characteristics of tumor cells, but also on their interaction with components of TME (11, 72).
Similar to tumor cells, cells of immune system, which have a high proliferative potential, are also adversely affected by cytostatic treatment. Leukopenia and immunosuppression frequently occur after completion of anticancer treatment. However, there are also many reports indicating immunomodulatory effects of cytotoxic drugs (73–75). Under certain conditions, radiation and drug therapy can diminish tumor escape from immune surveillance, and restore anti-tumor immune response, resulting in increasing therapeutic efficiency (73–76). Several lines of evidences demonstrate that TAMs are accumulated in tumors after chemo- and radiotherapy and contribute to tumor recurrence inducing suppression of T cell immunity, the maintenance of tumor cell survival and activation of tumor vascularization (11). Moreover, TAMs often accumulate in hypoxic regions of solid tumors, since hypoxia mediates recruitment of monocytes and pro-tumor polarization of monocyte-derived cells, including TAMs (64, 77, 78). Thus, hypoxia-inducible factor 1α (HIF-1) directly regulates the function and differentiation of MDSCs in hypoxic zones (79). Monocytes, the main precursors of TAMs, DCs, and MDSCs are programmed by treatment in circulation and the TME. This fact can be used for the predicting anti-tumor therapeutic response and tumor-directed targeting (73–76). Immunotherapy is most recent cancer treatment that has shown good results for solid tumors with reduced side effects compared to conventional treatments (80). However, not all tumors sufficiently respond to immunotherapy (81). Current problem is to identify the criteria that predict efficiency of immunotherapy. Considering involvement of monocytes in tumor pathogenesis, the functional profile of monocytes can be an informative factor for immunotherapy outcome.
Below we summarize accumulated data, indicating how anti-cancer treatment alters monocyte programming in bone marrow and peripheral blood, as well as during the monocyte recruitment to tumor and differentiation towards TAMs, DCs, and MDSCs. We present the state of the art in our knowledge about the mediators that program monocytes during various types of cancer therapy. We highlight the necessity for deep investigation of molecular mechanisms of such programming in order to develop new anti-cancer treatment approaches and to enhance the efficiency of the existing approaches.
It is currently unclear whether tumor resection can affect tumor-associated phenotype of monocytes. The decrease in cytokine production observed during surgery may occur due to the effects of anaesthetics, and the increase in cytokine production after surgery may reflect surgical stress (82, 83). Surgical intervention leads to an increase in the level of IL-6 and IL-1 in peripheral blood after surgery (Figure 1A). TNF-α production is detected only during surgical interventions, that is associated with intestinal ischemia (82). In meta-analysis of 15 studies of breast cancer patients which compared methods of general anaesthesia and general anaesthesia combined with continuous paravertebral block, the level of CCL2, TNF-a and IL-8 was notably decreased in case of combined method (84). It can be assumed, that there is an effect of these cytokines on monocyte precursor cells in the bone marrow. However, the direct effect of surgical treatment on precursor cells has not been studied, and major focus was on the changes in the content of monocytes in blood.
Figure 1 Interaction of four major types of cancer therapy with monocyte programming. Effects of surgery (A), radiotherapy (B), chemotherapy (C) and immunotherapy (D) on the differentiation of the monocyte progenitors in bone marrow, monocyte amounts and content in the circulation, recruitment to tumor and differentiation into TAMs are separately presented. Soluble mediators of the therapy effects are listed in the grey arrows. GM-CSF- Granulocyte-macrophage colony-stimulating factor, M-CSF - Macrophage colony-stimulating factor, CCL2 - Monocyte chemoattractant protein 1 (MCP1), IL1 – Interleukin1, IL3 – Interleukin 3, IL4 – Interleukin 4, IL6 – Interleukin 6, IL10 – Interleukin 10, MCP5 - Monocyte chemotactic protein 5 (CCL12), MIFα- Macrophage Migration Inhibitory Factor α, ROS - , VEGF - Vascular endothelial growth factor α, INFy – Interferon γ, PGE2 - Prostaglandin E, IDO - indoleamine 2,3-dioxygenase, TNFa - tumor necrosis factor α, S100A9 - S100 calcium-binding protein A9.
The number of peripheral blood leukocytes is increased significantly, and the number of lymphocytes is decreased in patients with stage T3 of colon cancer after surgery, indicating that surgical trauma and stress trigger systemic inflammation and suppress immune defense mechanisms (85). It is known that monocytic MDCS are characterized by low HLA-DR expression, and are usually considered as immunosuppressive cells (86). In patients with prostate and colorectal cancer, the percentage of immunosuppressive CD14+HLA-DRlow monocytes was elevated compared to normal individuals (80). Postsurgical normalization of CD14+HLA-DRlow cells occurred in prostate cancer patients, but not in patients with colorectal cancer (87). In patients with both cancer types, the interferon-γ response of T lymphocytes to phorbolmyristate acetate-ionomycin was increased compared with normal donors, but it was further increased after tumor ablation only in prostate cancer patients (87). It remains to be questionable whether CD14+HLA-DRlow cells were able to suppress inflammatory reactions, or their elevated levels were rather an attempt of the organism to compensate the disbalanced immune status in cancer patients. After glioblastoma resection, HLA-DR expression on monocytes was dynamically decreasing during first days after surgery, and returned to pre-surgery level after day 8 (88). In patients with lung cancer, thoractomy induced an increase in monocytic MDCS with CD11b+CD14+CD33+HLA-DR- phenotype in the blood compared to the status before surgery, and their accumulation correlated linearly with an increase in Treg amounts in circulation (89). Moreover, these surgery-induced MDCSs were more potent in expending Treg when co-cultured with autologous T cells in vitro (89). Mean number of monocytes along with their phagocytic activity were increased after surgery reaching the control point before treatment in breast cancer patients (90). In colorectal cancer patients, surgical intervention led to the reversal expression of previously upregulated genes (ACP5, ADM, APP, BAX, CD68, CTSZ, CXCR4, FCER1A, FKBP5, GPER, HBA1, HBB, HLA-DQ1, HMOX1, HP, ILR2S100P, SLPI,SOCS3, TKT) to the levels comparable to healthy individuals (15). In gastric cancer patients, post-surgery increase in monocyte count was a marker for early relapse (91). However, in oesophageal squamous cell carcinoma and lung adenocarcinoma post-surgery monocyte count positively correlated with overall survival and disease-free survival (92, 93).
Postoperative monocytes migration is potentially important factor of recurrence-free period in cancer patients. Since tumor is eliminated by surgery, recruitment of monocytes into the site of surgery is not in focus of the investigators. However, it is reasonable to take under consideration the dynamics of circulating levels of major chemotactic factor for monocytes, CCL2 (Figure 1A). Thus, in patients with gastric cancer after palliative surgery, serum CCL2 levels decreased postoperatively, whereas in patients who underwent curative surgery, serum CCL2 level tended to increase (94). Surgery was shown to increase MDSC in circulation and in tumor tissues in animal tumor models and in patients with lung cancer, breast cancer, and melanoma (89, 95). Levels of major immunosuppressive mediators, indoleamine 2,3-dioxygenase (IDO) and arginase, was increased rapidly in circulating CD14+CD33+HLA-DR− cells after gastrectomy in gastric cancer (Figure 1A) (91). Ex vivo, isolated out of blood CD14+CD33+HLA-DR− cells suppressed INFγ production by CD8 T-cells (91). Gastric cancer patients with septic complications on 8th and 14th days after esophagectomy had alterations in monocyte phenotype and functions, such as increased H2O2 production and decreased HLA-DR expression ex vivo (85). After colon tumor resection, monocytes in ex vivo conditions had supressed ability to produce pro-inflammatory cytokines and chemokines directly after surgery (96). After three days ex vivo, reduced amount of IL1b was produced in response to LPS by patients’ monocytes. In addition, the authors detected increased expression levels of suppressors of cytokine signalling (SOCS)1 and SOCS3 mRNA (96).
Overall, in several types of solid tumors tolerogenic status of immune system, that is a natural way to resolve efficiently surgical trauma -induced inflammation, can affect both phenotypes of circulating monocytes or monocyte-derived MDSCs, as well as their ability to modulate inflammation and give signals to T-cells.
Radiation therapy (RT) is considered as a standard in the treatment of most of solid tumors (97). Radioresistance is a key factor for RT failure, and development of locoregional relapse and distant metastases are the major obstacles for the success of the treatment (98–101). The involvement of TME in radioresistance was demonstrated in several studies (102, 103). One of the first studies on the RT effect on monocyte differentiation was performed by E. S. Buescher and J. I.Gallin in 1984 (104). They found that radiation doses used to prevent graft-versus-host reactions during leukocyte transfusions have detrimental impact on the survival and function of human monocytes in vitro (104). The long-term effects of radiation on monocytopoiesis are characterized by prolonged increase in the number of circulating monocytes, as it was demonstrated in Hiroshima and Nagasaki citizens in 50 years observation (105). It results in severe hematopoietic dysfunction, concomitant with a reduction in phagocytosis and deficiency in antigen recognition by T cells, antibody production and impaired activation of neutrophils and monocytes (106, 107). Localized irradiation affects healthy tissue that are adjacent to the irradiation site, with about half of the localized dose being absorbed by healthy tissues and bones (108). Local tissue destruction has more pronounced extend compared to the systemic one. After irradiation, the production of cytokines IL-1α/β, IL-6, IL-17, TNF-α, and VEGF is increased, and cellular senescence in a blood is levated (Figure 1B) (109–111). Below we present accumulated data about the effect of irradiation on monocyte biology in peripheral blood and tissues.
The effect of ionizing radiation depends on the total dose and fractionation rates (112). The direct action of radiation is related to the transfer of high energy to cells, resulting to membrane destruction and DNA damage followed by functional cell deficiency (113). Progenitor cells have high proliferative ability, and they are highly susceptible to irradiation. Overall, it was found that murine monocyte progenitors exhibit the impaired DNA repair activity and higher sensitivity to RT compared to macrophages. Total body irradiation in mice induced pronounced apoptosis in bone marrow-derived monocytes, but not in peritoneal macrophages (114).
Ionizing radiation promotes the suppression of immunity and subsequent bone marrow depression (Figure 1B) (115). Within first 24 hours after irradiation, active apoptotic death of hematopoietic cells results in monocytopenia (116). This effect is associated with a high sensitivity of monocyte progenitor cells to even low doses of irradiation (107). After irradiation at 10 Gy, only 1-3 out of 1000 stem cells, which are able for the regeneration, remain intact (117). Other stem cells in bone marrow are destroyed, and autologous recovery takes long time (117–119).
Irradiation toxicity can lead to spleen damage and decrease in the number of monocytes and macrophages contributing to tumor progression via reducing immune surveillance and pro-inflammatory stimulation (120, 121). Irradiation reduces not only spleen cellularity, but also the ability of survived lymphocytes and monocytes/macrophages to respond to mitogens (122). In spleen, the level of pSTAT1 and pSTAT4, which are activated by IFN-γ and IL-12, respectively, was significantly reduced by gamma irradiation, while an activation of STAT6 mediated by IL-4 receptor remained unchanged (Figure 1B) (123).
Analysis of RT effects on lymphoid and myeloid linage cells in healthy donors and patients with acute leukemia, who received allogeneic stem cell transplantation, revealed that monocytes are more resistant to RT than lymphocytes and granulocytes (106). The same tendency was found for patients with medulloblastoma (124). Irradiation negatively affects monocytes, significantly reducing their survival, which depends on the radiation doses. Thus, 2 Gy does not have significant impact on the number of circulating monocytes and monocyte-derived macrophages (125–127). In hybrid model of cross between female BALB/c and male DBA/2 CD2F1 mice, decrease in the percentage of circulating monocytes was detected four hours after irradiation at 8 Gy. After one month, monocytes were recovered and reached the pre-treatment level (128). Similar results were obtained in other studies. In vivo the number of circulating monocytes was noticeably decreased on the first days and was restored on days 21-28 after radiation (128–130) (Figure 1B). In patients with lung, breast, cervix and prostate cancers, who underwent RT (35 Gy), the number of circulating monocytes and eosinophils was significantly increased, that can be explained by the compensatory mechanisms activated by irradiation (131). In patients with larynx cancer, a decrease in the lymphocytes/monocytes index was observed from the point before RT to 6 weeks after RT (67). In patients with Hodgkin’s lymphoma, lymphocytes, neutrophils and monocytes are severely decreased immediately after irradiation at 35 Gy. Monocytes recovered quickly to ~ 50% pre-therapy level, and increased transiently to pre-therapy level up to 3 months after RT (132). Patients with medulloblastoma, who received a total of 35 Gy, had wave-like decrease and recovery of monocyte count that reached pre-treatment level to the end of treatment, maintaining this plateau for 2 months. In independent cohort, using a lower dose of 12.5 Gy leaded to increased amount of monocytes compared to pre-treatment level (132). In breast cancer patients, monocyte count was significantly higher 3 months after radiotherapy, compared to the pre-treatment level (133). These data indicate that depletion of monocytes is compensated during therapy.
As was mentioned above, peripheral blood monocytes are hypersensitive to IR and display high rate of apoptosis even after exposure at low doses (<2 Gy), compared to monocyte-derived macrophages which are hyposensitive. Monocytes have higher levels of single-chain and double-strain breaks and lower rate of DNA repair compared to macrophages (134). It can be explained by instability of monocytes to reactive oxygen species (ROS) impact, resulting in the protein oxidation and cell death independently of caspase-3 that induces monocyte necrosis (135). Monocytes and macrophages differentially respond to ROS-mediated oxidative stress. The sensitivity of monocytes to ROS is associated with phosphorylation of genes involved in DNA reparation, such as ATM, ATR, CHK1, CHK2 and p53, and activation of caspases 3, 7 and 8, which were not observed in macrophages and dendritic cells (Figure 1) (134).
Ionizing radiation can change migratory ability of monocytes via systemic stimulation of chemoattractant factors (136–140). Cytokine analysis in murine models for prostate and pancreatic cancer revealed elevated levels of M-CSF and CCL2 in the irradiated tumors and in circulation that stimulated recruitment of bone marrow and spleen-derived monocytes into tumor tissue (Figure 1B) (136, 141). Low-dose radiation increased serum level of anti-inflammatory cytokines IL-3 and IL-4, and pro-angiogenic vascular endothelial growth factor (VEGF) needed for efficient tumor vascularization (138). Circulating levels of chemotactic factors for monocytes and macrophages CCL2, MCP-5 and MIP-α were also elevated. At the same time, the levels of pro-inflammatory cytokines IL-12p70, IL-13, IL-17 and IFN-γ were decreased in mice circulation (138). This profile of circulating factors suggests that despite retaining high potential to be recruited into tumor mass, monocytes can be programmed in circulation to tolerate the tumor. Enhanced macrophage migration that was induced by conditioned medium from irradiated prostate cancer cells was completely blocked by a selective CSF1R inhibitor (136). In patients with prostate cancer, the level of CSF1 in blood serum was increased after RT (Figure 1) (136). The selective CSF1R inhibitor combined with RT suppressed tumor growth in vivo more effectively than RT alone (136). In murine models of head and neck tumor, breast cancer, and pancreatic ductal adenocarcinoma, response to RT was accompanied by CCL2 production, resulting in the recruitment of Ly6C+CCR2+ monocytes to support tumor proliferation and neovascularization after RT (139–141). The disruption of CCL2-CCR2 axis by ccl2 knock-out or by using CCL2 neutralizing antibody in combination with RT increased the efficiency of RT in head and neck, breast, and pancreatic ductal adenocarcinoma (Figure 1B) (139–141). Besides well-known CCL2/CCR2-mediated mechanism, monocyte infiltration into tumors after ionizing radiation depends on nerve damage-induced protein 1 gene (Ninjurin1, or Ninj1) which encodes a hemophilic adhesion molecule, and is activated in inflammatory lesions (142). Tumor hypoxia created by radiation-promoted vasculature disruption and enhanced activities of HIF-1/HIF-2 induced various cytokines/chemokines expression by residual tumor cells and tumor stromal (143). Also, hypoxia-induced HMGB1 facilitates the increase in the amounts of immunosuppressive cells such as MDSCs, and promotes the re-polarization of M1-type macrophages to M2-type macrophages (144). As a result, hypoxia enhances accumulation of immune cells and creates optimal conditions for the differentiation tumor-supporting monocyte-derived cells.
As described above, sensitivity of monocytes to irradiation is decreased during their differentiation into macrophages. This is associated with the ability of progenitor cells to actively proliferate compared to more differentiated cells (106). Human macrophages obtained from THP-1 monocytic cells are highly resistant to radiation-induced apoptosis compared to undifferentiated THP-1 cells in vitro (145). The phagocytic activity of human monocytes and macrophages in vitro was inhibited by irradiation at doses of 2500 and 5000 rad (104). After irradiation of 55 and 650 rad, phagocytic ability of human monocytes isolated from peripheral blood was not significantly affected by ionizing radiation, and monocytes were more radioresistant than lymphocytes (146). Phagocytic activity of peripheral blood monocytes remained intact in breast cancer patients undergoing RT at 5400-6640 Gy (147).
The influence of irradiation on immune cell activation can reflect hormesis phenomenon provided beneficial effects on organism (148). RT effect on the tumor-associated phenotype of macrophages was demonstrated in several studies, but the mechanism of this effect remains unclear. For example, increase in the expression of pro-inflammatory markers CD86, HLA-DR and CD80, but not CCR7, TNF and IL1B, and inhibition of the expression of anti-inflammatory markers CD163, MRC1, VCAN and IL10 were observed after 10 Gy exposure of human monocyte-derived macrophages, indicating a modulation of cell phenotype towards more pro-inflammatory one (149). These irradiated macrophages had increased phagocytic activity, and contributed to the invasion of cancer cells and angiogenesis in vitro (149). In THP-1 and RAW264.7 cell lines, radiation induced the expression of pro-inflammatory interferon-regulated factor 5 (IRF5) and an activation of a mutant kinase of telangiectasia ataxia (ATM), which are also crucial for the pro-inflammatory polarization of macrophages (150). Irradiation of human monocytic cell line U937 by γ-rays using a 137Cs source with a fixed dose rate of 148 or 531 cGy/min promoted antigen-presenting functional maturation by phosphorylating NFκB family member (151). In human monocytes isolated from healthy volunteers, low doses of irradiation (0, 05 and 0,1 Gy) activated MAPK pathways and positively regulated TLR signaling (152). Inhibition of NOS2 and ROS as well as an increase in arginase activity in bone-marrow macrophages were detected after the irradiation at dose 0.45 Gy/min in non-tumor immunodeficient C57BL/6 mice (153). In C57Bl/6J murine model of prostate cancer, irradiation induced upregulation of Arg-1 and COX-2 within 5 days and activation of iNOS-arginine pathway after 10 fractions of 25Gy irradiation in cells of TME, particularly in TAMs (Figure 1) (154). Expression of HLA-DR was up-regulated in monocytic DCs during hypofractionated RT treatment in colon cancer patients (155). Moreover, RT had two opposite effects on MDSCs, depending on the dose, fractionation scheme, and tumor model. Fractionated radiation therapy increased MDSCs amounts in tumor tissues, while ablative hypofractionated radiation therapy decreased MDSCs (156, 157). However, regardless of the stereotactic body RT regimen, several studies have shown that targeting MDSCs by Sunitinib, a multitargeted tyrosine kinase inhibitor of VEGFR1, VEGFR2, VEGFR3, PDGFR, c-kit, FLT3, and RET, increases the anti-tumor effect of RT (158).
Thus, radiation therapy impairs hematopoietic pool of progenitor cells due to their high proliferative activity. The damaging effect of RT declines from circulation monocytes to the differentiated monocyte-derived macrophages. Systemically, RT alters monocyte functions: proliferation, growth and phagocytic activity. Monocyte-derived macrophages, DCs and MDSCs are significantly more resistant to RT and respond to irradiation mainly by switching their phenotype toward tumor-supporting one.
Chemotherapy (CT) is one of the main anti-tumor treatment strategies, based on the cytotoxic effect of chemical drugs with different mechanisms of action on tumor cells suppressing their proliferation and inducing apoptosis (71). Systemic CT can affect hematopoietic progenitor cells, circulating immune cells and cells recruited from the bloodstream to tissues (1, 73). Collected data demonstrate that CT has an impact on the amount of circulating monocytes and tumor cell-TAM interactions. Understanding the dynamics of monocyte populations during CT can be helpful for the search of significant markers of therapy response and molecular targets to improve CT efficacy.
Monocyte recruitment both in peripheral blood and tumor tissue is multifunctional process related to chemotactic activity of tumor cells and components of TME, that can be modulated by chemotherapeutic impact (2, 19, 159). CT acts systemically, and cytostatic effect is directed to tumor cells and hematopoietic cells simultaneously. Progenitor cells have a greater ability to proliferate and are more sensitive to CT (1, 160).
Elevated TAM infiltration after taxane-based chemotherapy was demonstrated in patients with breast cancer and glioblastoma (161, 162). Monocyte recruitment to tumor site was increased in MMTV-PyMT (mouse model of breast cancer metastasis) treated with doxorubicin (163). Taxanes and anthracyclines promoted the secretion of tumor-derived extracellular vesicles, enriched with annexin A6 (ANXA6), stimulating CCL2 production and Ly6C+CCR2+ monocyte expansion in the pulmonary pre-metastatic niche in a breast cancer model (Figure 1C). Monocyte accumulation in turn facilitated the development of lung metastasis (164). The amount of monocyte progenitors in bone marrow and in spleen of Balb/c mice significantly decreased after 2 days of cyclophosphamide treatment. By day 7, the total amount of cells in bone marrow and spleen returned to the pre-treatment level (165). M-CSF (or CSF1) is a crucial factor of monocyte progenitors’ differentiation (166). Paclitaxel increased the expression of CSF1 and IL-34 in tumor cells, followed by the recruitment of large number of CSF1R-expressing monocytes in mouse mammary tumors (Figure 1C) (161). Treatment with paclitaxel and carboplatin increased the expression of CCL2, a key chemokine for monocyte recruitment, in ovarian cancer cells activating monocyte migration in vitro and in vivo (167). The combination of chemotherapy, irradiation and vascular destroying agents (such as combretastatin-A4-P) resulted in increased production of CSF1 and CCL2 along with CXCL12 by tumor cells, that induced monocyte recruitment in Lewis lung carcinoma (LCC) model and MMTV-PyMT model (168). In breast cancer patient-derived xenograft model, carboplatin and taxane-based CT stimulated monocytosis followed by systemic elevation of CCL2 level (169). Treatment with doxorubicin, docetaxel and SP600125, an c-Jun N-terminal kinases (JNK) inhibitor, stimulated CCL2-depended monocytosis that supported breast cancer stem cells proliferation (170). ShRNA and pharmacological suppression of the CCL2-CCR2 pathway diminished chemotherapeutic adverse effect on cancer stem cells (Figure 1C) (169, 170). YLK39, a novel strong chemotactic factor for primary human monocytes, whose expression in tumors after neoadjuvant chemotherapy predicted an increased risk of distant metastasis and poor response to preoperative CT (170).
Since monocytes replenish the pool of TAMs with tumor-supporting activity, blocking monocyte recruitment can be potentially used as a therapeutic strategy. Inhibition of monocyte renewal and recruitment by anti-chemotactic molecules improved the response to CT in numerous studies. Anti-CSF1R antibody depleted TAMs in mouse models of colorectal adenocarcinoma, fibrosarcoma, breast cancer, hepatocellular carcinoma, pancreatic cancer and glioma (171–175). Anti-CSF1R treatment enhanced response to clinically used chemotherapeutic agents such as paclitaxel and gemcitabine in mammary and pancreatic ductal adenocarcinoma murine tumors (161, 176). Similarly, the combination treatment with anti-CCL2 (carlumab, CNTO 888) and conventional chemotherapy (docetaxel, oxaliplatin and irinotecan plus folinic acid and 5-fluorouracil [5-FU]) resulted in the increase of the antitumor response in prostate and pancreatic cancers (177). Most of studies are limited to the analysis of CCL2/CCR2 interaction which was a target for a number of clinical trials. However, these clinical trials have never reached phase III level in cancer according to clinical trial data on ClinicalTrials.gov. Failure of clinical trials can be explained by number of redundantly acting chemokines attracting monocytes into pathologically change tissue, the mechanism that evolutionary needs high level of safety due to the key role in host response against pathogens.
Thus, CT induces production of factors that stimulate monocyte progenitors’ proliferation and monocyte entry into the circulation*. Since newborn monocytes are chemotherapy-intact, their repolarization after CT course can be promising approach for anti-tumor therapy in adjuvant regimen. This point needs to be investigated more thoroughly.
The dynamics of the count and subset composition of monocytes during CT treatment has been studying for several years due to easy access to monocytes in peripheral blood (178–180). Normal monocyte count is 400/µl (0.4 × 109/liter) for healthy adults. Men tend to have slightly higher monocyte counts compared to women. Increase in the number of blood monocytes correlates directly to the raise in a total pool of monocytes and a monocyte turnover rate. The pool of peripheral blood monocytes is renewed every 5 days (181).
Chemotherapy seems to have less effect on mature monocytes. The count of these monocytes can decrease due to a lifespan termination in peripheral blood leading to complete exhaustion called the nadir. Renewed monocyte population after CT treatment may have a different functional profile with the loss of pro-tumor programming in case of favorable outcome.
In cancer patients, nadir point for monocytes is observed at 5-7 day after CT drug injection (178–180) (Table 2). In patients with pancreatic cancer, the number of CD14+ cells has been significantly decreased at day 14 after gemcitabine treatment, and then recovered until day 55th (178) (Table 2). In BALB/c mice, that received 4 doses (on day 1, 7, 14 and 21) of doxorubicin or docetaxel, monocyte count was decreased from day 6 to day 21, and then raised up until day 45 (185). Absolute number of white blood cells was decreased, but monocyte absolute count had no significant changes during five cycles of doxorubicin plus cyclophosphamide treatment in luminal B and Her2+ breast cancer patients. After five CT cycles, monocyte absolute count was drastically decreased, concomitant to the switch to paclitaxel treatment (183) (Table 2). In the same patients, decrease in absolute neutrophil and leukocyte counts as well as leukocytes-monocytes ratio was observed from 1st to 5th cycle of CT treatment with significant increase to the end of 8th cycle (183). Due to having a common progenitor, monocyte and neutrophil counts correlated during CT (186). Wherein monocytopenia preceded neutropenia by 4-6 days, that was a predictive factor for side effects after docetaxel, cisplatin and fluorouracil-based therapy in patients with head and neck cancer and with lung cancer (186). Considering that the vulnerability to infectious and febrile neutropenia are frequently the limiting adverse effects of anti-cancer CT and can cause life-threatening events, they can be useful indicators for modulating treatment regimens (187).
Chemotherapeutic agents can affect monocyte subpopulations in circulation blood (160, 188, 189). In breast cancer cohort, the amount of CD3-CD14+CD16- monocytes was increased during 5 cycles of doxorubicin, dramatically decreased after 5th cycle, but then has reсovered after following 3 cycles of paclitaxel treatment (183). For another breast cancer cohort, gradual decrease in CD14+ and CD14+HLA-DRlow subsets during 6 cycles of 5-FU, epirubicin, cyclophosphamide or capecitabine treatment was found (190). Non-classical subset (CD3-CD14+CD16High) of monocytes demonstrated the opposite trend in this study (190). In HER2+ breast cancer patients, a positive correlation between the amount of classical and non-classical monocytes and plasma IL-10 level was found during neoadjuvant chemotherapy (Figure 1C) (183). In patients with triple-negative breast cancer, significant decreased in classical, non-classical and intermediate monocytes was found after 12 weeks of treatment with nanoparticle albumin-bound paclitaxel. The monocyte count for all subsets increased after two weeks of treatment with epirubicin and cyclophosphamide, with maximum at week 8 (Table 2) (184). 5FU-based CT treatment of liver metastases in colorectal cancer patients induced rapid decrease in CD14+CD16+ intermediate subset of peripheral blood monocytes that predicted poor response to NAC (41). Etoposide plus cisplatin combination treatment elevated the amount of IL-10+CD206+CD14+ M2-like monocytes in peripheral blood of patients with small cell lung cancer (SCLC) compared to non-treated patients (191). In non-small cell lung cancer (NSCLC) patients, both frequency and absolute number of CD14+HLA-DR-/low cells were significantly increased in the peripheral blood compared to healthy control. Increased amount of CD14+HLA-DR-/low monocytes was indicative for poor response to platinum-based chemotherapy and short period of PFS (192). In advanced-stage ovarian cancer patients, CD14+HLA‐DRlow/− monocytes were diminished by platinum and taxane-based chemotherapy (193).
In addition to the repopulation of monocytes in peripheral blood, their proprieties can also be changed during CT treatment. In cyclophosphamide-treated mice, monocytes possessed gene expression signature specific for neutrophil precursors, defined by increased proliferative capability and elevated amounts of multiple granules and suppressed T-cell proliferation in vitro (165). Two days after CTX there was a sharp decrease in cellularity in both bone marrow and spleen, and the total number of cells returned to a baseline level by day 7 (165). In mice, HIF-1α–deficient tumors had reduced numbers of proangiogenic TEMs, and tumor were also significantly smaller after docetaxel chemotherapy (194).
The prognostic and predictive values have been demonstrated for lymphocyte to monocyte ratio (LMR). Increased pre-treatment absolute lymphocyte count and high LMR were independent favourable prognostic factors in patients with metastatic nasopharyngeal carcinoma (67). Positive correlation between pre-treatment LMR and pCR was observed in patients with locally advanced oesophageal squamous cell carcinoma who underwent neoadjuvant platinum-based chemoradiotherapy (195). Significant correlation between lower monocyte count after NAC and pCR was found in triple-negative breast cancer patients (196). Increased IL-10 level and monocyte counts correlated with lower rates of pCR (183). High percentage of CD11b+CD14+ and CD11b+CD14+S100A9+ cells predicted poor response to platinum-based chemotherapy and negatively correlated with PFS in patients with NSCLC (197). Oppositely, in triple negative breast cancer patients who received non-platinum-containing treatment, monocyte counts positively correlated with disease-free survival (196).
Thus, similar kinetics of increase in monocyte amounts in circulation was observed in cancer patients treated by various chemotherapeutic drugs. The nadir point for monocytes precedes the one for neutrophils. After CT application, the number of circulation monocytes can come down to the original level within several week. It can be assumed that newly differentiated monocytes carry genetic, epigenetic and transcriptional modifications developed under the influence of cytostatic drugs. Most advanced single cell resolution technology provides now a useful tool to decipher the impact of each programming level on functionality of monocytes when they enter tumor mass.
The most important stage of the ontogenesis of monocyte-derived TAMs, DCs and MDCSs which can be modulated by CT treatment is tumor-supporting differentiation (11, 198, 199). In MDA-MB435 breast carcinoma mouse model, paclitaxel+albumin treatment resulted in increased infiltration of tumor by CD45+CD169+ and F4/80+ macrophages (200). The expression of GM-CSF, a crucial growth factor for monocyte to macrophage differentiation, was significantly upregulated in various pancreatic ductal adenocarcinoma cell lines or human pancreatic ductal adenocarcinoma tissues after gemcitabine treatment. In patients with pancreatic ductal adenocarcinoma, GM-CSF expression correlated to poor prognosis after CT treatment (Figure 1) (201). The blockade of GM-CSF with monoclonal antibodies facilitated T-cell proliferation in co-culture of monocytes stimulated by tumor cell-conditioned supernatants and T lymphocytes (202). Most chemotherapeutic agents used in conventional cancer chemotherapy reduce the number of MDSCs in tumor tissues and peripheral lymphoid organs, and inhibit differentiation in MDSCs (203). Gemcitabine treatment stimulated the immunosuppressive activity of monocytic MDSC and, accordingly, decreased the amount of IFN-γ-producing CD4+ and CD8+ T cells in mammary tumor-bearing mice (204). Cyclophosphamide treatment induced the expansion of inflammatory monocytic myeloid cells in vitro that attenuated antitumor CD4+ T-cell response through the PD-1-PD-L1 axis (165). Treatment with cisplatin or carboplatin increased the production of IL-6 and PGE2 by cervical cancer cells, head and neck cancer cells, and myeloma cell lines thus promoting STAT3-dependent IL-10 production by M2 macrophages with tumor-supporting activity (Figure 1C) (205). In patients with breast cancer, high expression of monocyte chemotactic factor YKL-39, specifically expressed by TAMs, correlated with increased risk of distant metastasis and poor response to PTX- or taxotere-based NAC (170). In breast cancer patients, CT did not affect monocyte amounts, but phagocytic index of monocyte-derived macrophages was diminished (90). In breast cancer patients who underwent anthracycline-containing NAC, the absence of clinical response was associated with the presence of M2-like macrophages with YKL-39-CCL18+ or YKL-39+CCL18- phenotypes (206). These data indicate that CT aggravates immunosuppression by differentiation of monocytes toward tumor-promoting macrophages.
Thus, cytostatic agents are able to modulate the recruitment of monocytes into the tumor, their differentiation into specific TAM populations, and their regulation of adaptive antitumor immune responses. Such mode of action can dramatically affect tumor progression after chemotherapy, contributing to poor response and poor outcome. In future, combined chemotherapeutic and immunomodulatory approaches based on the modulation of monocyte-TAM activity can help to increase CT efficacy.
Immunotherapy is one of the promising targeted strategies for the treatment of malignant neoplasms (207–211). Immunotherapy can be divided into two main types - active or passive. Active immunotherapy uses CAR-T cells, cancer vaccines and monoclonal antibodies (mAbs) that specifically target tumor cells through the immune system. Passive immunotherapy does not directly affect tumor cells, but increases the ability of the immune system to attack them. The latter type includes checkpoint inhibitors and cytokines. Patients treated with immunotherapy showed different response rates depending on cancer type and cohort composition with the same malignancy (212, 213). The impaired anti-tumor immune response observed in patients with decreased immunotherapy efficacy is likely a consequence of immune system dysfunction: diminished antigen presentation/detection, leukopenia, and coordinated network of immunosuppressive cell surface proteins, cytokines and cellular mediators (214). The use of autologous monocytes, and interferons alpha and gamma for local-regional administration directly into the peritoneal cavity demonstrated first promising results for improving immune response (215).
Immune checkpoints are inhibitory receptors and pathways that are responsible for maintaining self-tolerance and modulating immune response (216). One of the most used immune checkpoint inhibitors (ICI) include anti-PD-1 or anti-PD-L1 molecules, which prevent the blocking of the immune system and activate attacking of tumor cells by T lymphocytes. PD-1 is an inhibitory receptor expressed on activated T cells, B-cells and monocytes (217). Drugs in this category currently include nivolumab (Opdivo), atezolizumab (Tecentriq), pembrolizumab (Keytruda), avelumab (Bavencio), durvalumab (Imfinzi) and cemiplimab-rwlc (Libtayo). Despite that these drugs target T-cells, they are not fully effective in the absence of myeloid cells such as monocytes and macrophages (218, 219). There is only limited information about IT influence to monocytes progenitors today. The combination of oxaliplatin, thymidine-based antitumor nucleoside analogue trifluridine and thymidine phosphorylase inhibitor tipiracil, which suppresses bone marrow, induced immune cell death in vivo, providing a rationale way for the use of these drugs to eliminate immunosuppressive cells in patients with metastatic colorectal cancer (Figure 1D) (220). We describe bellow the available data for IT effect on circulating monocytes and their differentiation in the tumor tissues.
In patients with nasopharynx cancer, breast cancer and esophageal cancer, increased absolute monocyte count and/or CD14+16- monocyte percentage in a pool of peripheral blood mononuclear cells correlated with worse response to anti-PD-1/PD-L1 therapy and decreased PFS (221). In melanoma, a higher content of CD14+CD16−HLA-DRhigh monocytes before anti-PD-1 therapy is a strong predictor for PFS and OS (Figure 1D) (222). The average number of monocytes remained unchanged after ICI-based therapy compared to pre-treatment amount (223). Another ICI therapy is directed at CTLA-4, a cytotoxic protein 4 associated with T lymphocytes. Ipilimumab blocks the activity of CTLA-4 facilitating the elimination of cancer cells by the immune system (224). The amount of classical monocytes (CD14+CD16−) that express high levels of HLA-DR before treatment was predictive for improved OS in neuroblastoma patients (225). The majority of CD14+HLA-DRlow/neg cells are classical monocytes, so the higher expression of HLA-DR may reflect lower levels of immunosuppressive monocytic MDSCs (226). High level of CD14+HLA-DRlow/neg monocytes in peripheral blood of melanoma patients can be indicative for poor response to immunotherapy with anti-CTLA4 agent (Figure 1D) (227–229). Patients with lung and gastric cancers who have high PD-L1 expression on monocytes rarely respond to anti-PD1 therapy and have poor PFS and OS (230, 231).
Successful outcome of mAb therapy can depend on the initial number of monocytes in peripheral blood (226). At a primary low level of monocytes, the myelosuppression or decrease in the number of monocytes in tumor tissue after treatment may occur (226). Monocytes provide an essential mechanism for the depletion of malignant B cells under successful anti-CD20 therapy (232). Therefore, there is an urgent need to monitor the number and function of monocytes in patients with lymphoma undergoing anti-CD20 and other mAb-based therapies (232).
Some drugs designed to inhibit monocytes/macrophages migration into tumor are also under investigations (233, 234). However, clinical trials were not as successful compared to CTLA-4 and PD-1/PD-L1 targeting (233, 234). Anti-S100A9 (Tasquinimod) treatment reduced tumor growth partially by reducing the recruitment of Ly6Chigh cells from circulation to tumor tissue in mammary and melanoma mouse model (233). Anti-CCL2 antibodies were also proposed as a targeted immunotherapy for cancer. The main idea of using the drug was to block the recruitment of monocytes into the tumor. Anti-CCL2 CNTO 888 (carlumab) has demonstrated antitumor activity and good tolerance in cancer patients, but clinical trials on carlumab are not completed (235). Combination of carlumab with conventional chemotherapy are under clinical trials (236). However, recent results showed that increased metastasis rate was reported following anti-CCL2 therapy (237). In breast cancer model, the discontinuation of anti-CCL2 therapy was associated with descendance of monocytes from the bone marrow, increased mobilization of tumor cells from the primary site, an increase in their infiltration into the metastatic loci and increased angiogenesis promoted by IL-6 and VEGF (Figure 1D) (237).
Chemotherapy in combination with ICI therapy induces upregulation of PD-L1 on cancer cells, facilitates infiltration of CD8+ T cells and NK cells, increases maturation of antigen-presenting cells including DCs and TAMs, and promotes MDSC activation (217). Mononuclear cells isolated from peripheral blood of healthy donors decreased the proliferation of nivolumab-treated T cells in vitro (225). Increased interactions of therapeutic mAb with monocyte receptors can enhance antibody dependent cellular cytotoxicity in vivo (238). Although most anti-CD20 antibodies activated the complement system in vitro, B-cell depletion was completely effective in mice with genetic insufficiency of C3, C4, or C1q complement components (232). The fact that monocytes deplete B cells via FcyR-dependent pathway during anti-CD20 immunotherapy has important clinical implications for tumor treatment with anti-CD20 mAbs (Rituximab) and other antibody-based drugs (239). Anti-CD20 mAbs induce the production of pro-inflammatory chemokines by immune cells inside the tumor, in particular by infiltrating macrophages (240). Rituximab can also lead to the release of pro-inflammatory cytokines, including TNF-α and IL-6, which can further enhance the immune response (Figure 1D) (240). Monocytes and macrophages induce antibody-dependent cytotoxicity and phagocytosis of tumor cells in the presence of antitumor mAb IgG (241). In pancreatic ductal adenocarcinoma model, macrophages activated by anti-CD40 mAb quickly penetrated tumor, acquired tumor-like phenotype and contributed to the depletion of tumor stroma via blocking CD40-expressing macrophages (242). Treatment with antibodies against immune checkpoint inhibitors a-PD-1 + a-CTLA-4 resulted in a dynamic remodeling of intra-tumoral monocyte-derived macrophages (241, 243). CyTOF and scRNASeq analysis identified 5 and 8 subsets of monocytes/macrophages respectively with difference functional direction that correlated with tumor progression (243).
Bispecific antibodies are proteins that target specific tumor antigens (similar to mAb) and bind proteins on the surface of immune cells (66). This allows T cells to kill cancer cells (244). Blinatumomab (Blincyto) is an example of a bispecific antibody (anti-CD3—anti-CD19) that recruits T cells to B cells. The drug was approved for the treatment of recurrent and refractory forms of B-cell malignancies, such as B-cell acute lymphoblastic leukemia and non-Hodgkin’s lymphoma (245). Catumaxomab targets EpCAM-positive carcinomas (246). A complex mechanism of action is related to an effective destruction of cancer cells, but excessive inflammatory background can be developed as a result of the pro-inflammatory cytokine production by blood monocytes (246).
Thus, immunotherapy effects can be mediated by monocyte responses. The effect of immunotherapy on monocyte activity can have both direct and indirect mode of action. The first one related to monocyte-specific alterations, and the second one is based on monocyte interactions with other cell types, e.g., CD8 T-cells or DC. The development of novel immunotherapeutic approaches aimed at molecular targets in monocytes can be also a promising strategy for improvement therapeutic effect.
Constitutive features are one of the factors that can define the immune responses in general, as well as antitumor response of immune system. To date, there is no comprehensive view of the importance of gene polymorphic variants of myeloid cell in oncogenesis. It is known that the presence of single nucleotide polymorphisms (SNP) of the CCL2 gene is associated with breast, oral, endometrial, non-small cell lung, prostate and bladder cancers (56, 57, 60, 61, 247). Data on the correlation of SNP on monocytes with an influence of antitumor treatment are discrete and provide information about individual genes. We have summarized the monocyte-related SNP variants and their chromosomal localization on Figure 2. Thus, in monocytes, the Ala134Thr variant (rs2072443) in TMEM176B is associated with poor gene expression and with an increased activation of NLRP3 inflammasome. This SNP correlates with longer overall survival in colorectal cancer cohort (Table 3) (248). Patients with nasopharyngeal carcinoma with AA and AG gene variants showed a significant correlation with the development of distant metastasis after the initial RT (251). Moreover, rs4986790 and rs4986791 of TLR4 significantly correlated with poor efficacy and early relapse in patients with breast cancer, that was confirmed using mice models and in vitro tests on human monocyte-derived DCs (Table 3) (250). SNP of CCL2 is associated with longer PFS after conventional CT with anti-VEGF drug Bevacizumab compared to wild variants (Table 3) (249). Analysis of seven SNPs of CCL2, NOS3, IL6R, IL12B, IL1RN and CXCR3 genes from patient cohort with different cancer types showed that SNP carriers have better response to anti-PD-1/PD-L1 treatment (Table 3) (253). Evidently, CCR2-depend pathway defines the response of brain tumor cells to radiotherapy (Table 3) (254). Thus, several studies about single polymorphic gene variants describe correlations between monocyte SNPs and CT and RT efficacy. Therefore, new technologies are required to clarify the issue of the influence of genetic features on the reactions of monocytes to anti-cancer therapy.
Figure 2 Chromosomal localisation of SNPs associated with the monocytes’ involvement in the cancer therapy effects. Figure created in biorender (http://biorender.io).
Programming of monocyte progenitors in the bone marrow and in the circulation is essential for their potential to give rise to TAMs, DCs and MDSCs that are mostly cancer promoting, however, in some cancers (for example, in colorectal cancer) can have anti-cancer activities. Cancer therapy can interact with monocytes on the level of programming their dividing precursors in bone marrow, can affect efficiency of monocyte entry into the circulation, affect their subpopulational content and transcriptome, and can also affect their recruitment into tumor sites and their potential to develop cancer-specific TAMs, DCs and MDCSs.
However, the mechanisms that mediate cancer therapy programming of monocytes are only emerging, and large effort is needed to create a comprehensive picture for programming of major TAM, DC and MDSC precursors-monocytes in each type of cancer and by each type of therapy or their combination.
Rapidly developing single cell analytic technologies provide a highly useful tool here, since monocytes are much easier to examine on a single cell level compared to TAMs, DCs and MDSCs that need sophisticated approaches to be isolated out of heterogeneous tumor tissues without being damaged and without losing of highly adherent subpopulations. Moreover, in perspective, multiomics approaches have to be applied in order to combine the information not only about transcriptome and surface markers, but also about the metabolic status of monocytes, their genome and epigenome. Overall, the impact of genetic diversity of monocytes is underestimated when we examine the mechanisms of innate immune control in cancer. Such complex analysis is needed to design monocyte-targeted therapies, that due to the minimal invasiveness is a very promising direction to instruct human innate immunity to fight against cancer.
Conceptualization: MP and JK. Writing—original draft preparation: MP, AF, IL, JK, SA, AT, NC. Writing— review and editing: MP, IL, JK. Figure preparation: AF, MP, JK. Supervision: NC and JK. Funding acquisition: JK. All authors contributed to the article and approved the submitted version.
This research was funded state contract of the Ministry of Science and Higher Education of the Russian Federation “Genetic and epigenetic editing of tumor cells and microenvironment in order to block metastasis” o. 075-15-2021-1073.
The authors declare that the research was conducted in the absence of any commercial or financial relationships that could be construed as a potential conflict of interest.
All claims expressed in this article are solely those of the authors and do not necessarily represent those of their affiliated organizations, or those of the publisher, the editors and the reviewers. Any product that may be evaluated in this article, or claim that may be made by its manufacturer, is not guaranteed or endorsed by the publisher.
1. Galluzzi L, Buque A, Kepp O, Zitvogel L, Kroemer G. Immunological effects of conventional chemotherapy and targeted anticancer agents. Cancer Cell (2015) 28(6):690–714. doi: 10.1016/j.ccell.2015.10.012
2. Robinson A, Han CZ, Glass CK, Pollard JW. Monocyte regulation in homeostasis and malignancy. Trends Immunol (2021) 42(2):104–19. doi: 10.1016/j.it.2020.12.001
3. Olingy CE, Dinh HQ, Hedrick CC. Monocyte heterogeneity and functions in cancer. J Leukocyte Biol (2019) 106(2):309–22. doi: 10.1002/JLB.4RI0818-311R
4. Engblom C, Pfirschke C, Pittet MJ. The role of myeloid cells in cancer therapies. Nat Rev Cancer (2016) 16(7):447–62. doi: 10.1038/nrc.2016.54
5. Larionova I, Kazakova E, Patysheva M, Kzhyshkowska J. Transcriptional, epigenetic and metabolic programming of tumor-associated macrophages. Cancers (2020) 12(6):1411. doi: 10.3390/cancers12061411
6. Larionova I, Tuguzbaeva G, Ponomaryova A, Stakheyeva M, Cherdyntseva N, Pavlov V, et al. Tumor-associated macrophages in human breast, colorectal, lung, ovarian and prostate cancers. Front Oncol (2020) 10:566511. doi: 10.3389/fonc.2020.566511
7. Lee J, Geng S, Li S, Li LW. Single cell RNA-seq and machine learning reveal novel subpopulations in low-grade inflammatory monocytes with unique regulatory circuits. Front Immunol (2021) 12. doi: 10.3389/fimmu.2021.627036
8. Porrello A, Leslie PL, Harrison EB, Gorentla BK, Kattula S, Ghosh SK, et al. Factor XIIIA-expressing inflammatory monocytes promote lung squamous cancer through fibrin cross-linking. Nat Commun (2018) 9(1):1988. doi: 10.1038/s41467-018-04355-w
9. Madsen DH, Jürgensen HJ, Siersbæk MS, Kuczek DE, Grey Cloud L, Liu S, et al. Tumor-associated macrophages derived from circulating inflammatory monocytes degrade collagen through cellular uptake. Cell Rep (2017) 21(13):3662–71. doi: 10.1016/j.celrep.2017.12.011
10. Larionova I, Kazakova E, Gerashchenko T, Kzhyshkowska J. New angiogenic regulators produced by TAMs: Perspective for targeting tumor angiogenesis. Cancers (2021) 13(13):3253. doi: 10.3390/cancers13133253
11. Larionova I, Cherdyntseva N, Liu T, Patysheva M, Rakina M, Kzhyshkowska J. Interaction of tumor-associated macrophages and cancer chemotherapy. Oncoimmunology (2019) 8(7):1596004. doi: 10.1080/2162402X.2019.1596004
12. Gren ST, Rasmussen TB, Janciauskiene S, Hakansson K, Gerwien JG, Grip O. A single-cell gene-expression profile reveals inter-cellular heterogeneity within human monocyte subsets. PloS One (2015) 10(12):e0144351. doi: 10.1371/journal.pone.0144351
13. Kiss M, Caro AA, Raes G, Laoui D. Systemic reprogramming of monocytes in cancer. Front Oncol (2020) 10:1399. doi: 10.3389/fonc.2020.01399
14. Cassetta L, Fragkogianni S, Sims AH, Swierczak A, Forrester LM, Zhang H, et al. Human tumor-associated macrophage and monocyte transcriptional landscapes reveal cancer-specific reprogramming, biomarkers, and therapeutic targets. Cancer Cell (2019) 35(4):588–+. doi: 10.1016/j.ccell.2019.02.009
15. Hamm A, Prenen H, Van Delm W, Di Matteo M, Wenes M, Delamarre E, et al. Tumour-educated circulating monocytes are powerful candidate biomarkers for diagnosis and disease follow-up of colorectal cancer. Gut (2016) 65(6):990–1000. doi: 10.1136/gutjnl-2014-308988
16. Taylor PR, Gordon S. Monocyte heterogeneity and innate immunity. Immunity (2003) 19(1):2–4. doi: 10.1016/S1074-03)00178-X
17. Bodey B, Siegel SE, Kaiser HE. Antigen presentation by dendritic cells and their significance in antineoplastic immunotherapy. Vivo (Athens Greece) (2004) 18(1):81–100. doi: 10.1007/1-4020-2804-0_7
18. Kross KW, Heimdal JH, Aarstad HJ. Mononuclear phagocytes in head and neck squamous cell carcinoma. Eur Arch Oto-rhino-laryngol (2010) 267(3):335–44. doi: 10.1007/s00405-009-1153-y
19. Qian B-Z, Li J, Zhang H, Kitamura T, Zhang J, Campion LR, et al. CCL2 recruits inflammatory monocytes to facilitate breast-tumour metastasis. Nature (2011) 475(7355):222–U129. doi: 10.1038/nature10138
20. Olingy CE, Dinh HQ, Hedrick CC. Monocyte heterogeneity and functions in cancer. J Leukocyte Biol (2019) 106(2):309–22. doi: 10.1002/JLB.4RI0818-311R
21. Kiss M, Caro AA, Raes G, Laoui D. Systemic reprogramming of monocytes in cancer. Front Oncol (2020) 10. doi: 10.3389/fonc.2020.01399
22. Kapellos TS, Bonaguro L, Gemünd I, Reusch N, Saglam A, Hinkley ER, et al. Human monocyte subsets and phenotypes in major chronic inflammatory diseases. Front Immunol (2019) 10:2035. doi: 10.3389/fimmu.2019.02035
23. Ong SM, Teng K, Newell E, Chen H, Chen J, Loy T, et al. A novel, five-marker alternative to CD16-CD14 gating to identify the three human monocyte subsets. Front Immunol (2019) 10:1761. doi: 10.3389/fimmu.2019.01761
24. Merah-Mourah F, Cohen SO, Charron D, Mooney N, Haziot A. Identification of novel human monocyte subsets and evidence for phenotypic groups defined by interindividual variations of expression of adhesion molecules. Sci Rep (2020) 10(1):4397. doi: 10.1038/s41598-020-61022-1
25. Zieglerheitbrock HWL, Passlick B, Flieger D. The monoclonal antimonocyte antibody my4 stains lymphocytes-b and 2 distinct monocyte subsets in human peripheral-blood. Hybridoma (1988) 7(6):521–7. doi: 10.1089/hyb.1988.7.521
26. Passlick B, Flieger D, Zieglerheitbrock HWL. Identification and characterization of a novel monocyte subpopulation in human peripheral-blood. Blood (1989) 74(7):2527–34. doi: 10.1182/blood.V74.7.2527.2527
27. Andreesen R, Brugger W, Thomssen C, Rehm A, Speck B, Lohr GW. Defective monocyte-to-macrophage maturation in patients with aplastic-anemia. Blood (1989) 74(6):2150–6. doi: 10.1182/blood.V74.6.2150.2150
28. Ziegler-Heitbrock L, Ancuta P, Crowe S, Dalod M, Grau V, Hart DN, et al. Nomenclature of monocytes and dendritic cells in blood. Blood (2010) 116(16):E74–80. doi: 10.1182/blood-2010-02-258558
29. Nockher WA, Scherberich JE. Expanded CD14(+) CD16(+) monocyte subpopulation in patients with acute and chronic infections undergoing hemodialysis. Infect Immun (1998) 66(6):2782–90. doi: 10.1128/IAI.66.6.2782-2790.1998
30. Murphy FJ, Reen DJ. Differential expression of function-related antigens on newborn and adult monocyte subpopulations. Immunology (1996) 89(4):587–91. doi: 10.1046/j.1365-2567.1996.d01-788.x
31. Bobdey S, Ganesh B, Mishra P, Jain A. Role of monocyte count and neutrophil-to-Lymphocyte ratio in survival of oral cancer patients. Int Arch Otorhinolaryngol (2017) 21(1):21–7. doi: 10.1055/s-0036-1587318
32. Lin GN, Peng JW, Liu DY, Xiao JJ, Chen YQ, Chen XQ. Increased lymphocyte to monocyte ratio is associated with better prognosis in patients with newly diagnosed metastatic nasopharyngeal carcinoma receiving chemotherapy. Tumour Biol (2014) 35(11):10849–54. doi: 10.1007/s13277-014-2362-6
33. Li K, Xia XF, Su M, Zhang H, Chen H, Zou SCL. Predictive value of lymphocyte-to-monocyte ratio (LMR) and neutrophil-to-lymphocyte ratio (NLR) in patients with oesophageal cancer undergoing concurrent chemoradiotherapy. BMC Cancer (2019) 19(1):1004. doi: 10.1186/s12885-019-6157-4
34. Pan YC, Jia ZF, Cao DH, Wu YH, Jiang J, Wen SM, et al. Preoperative lymphocyte-to-monocyte ratio (LMR) could independently predict overall survival of resectable gastric cancer patients. Medicine (2018) 97(52):e13896. doi: 10.1097/MD.0000000000013896
35. Xu BB, Xu Y, Lu J, Wu Y, Wang JB, Lin JX, et al. Prognostic significance of combined lymphocyte-monocyte ratio and tumor-associated macrophages in gastric cancer patients after radical resection. J Cancer (2020) 11(17):5078–87. doi: 10.7150/jca.44440
36. Choi YH, Lee JW, Lee SH, Choi JH, Kang J, Lee BS, et al. A high monocyte-to-Lymphocyte ratio predicts poor prognosis in patients with advanced gallbladder cancer receiving chemotherapy. Cancer Epidemiol Biomarkers Prev (2019) 28(6):1045–51. doi: 10.1158/1055-9965.EPI-18-1066
37. Xiang J, Zhou L, Li X, Bao W, Chen T, Xi X, et al. Preoperative monocyte-to-Lymphocyte ratio in peripheral blood predicts stages, metastasis, and histological grades in patients with ovarian cancer. Trans Oncol (2017) 10(1):33–9. doi: 10.1016/j.tranon.2016.10.006
38. Lu C, Zhou L, Ouyang J, Yang H. Prognostic value of lymphocyte-to-monocyte ratio in ovarian cancer: A meta-analysis. Medicine (2019) 98(24):e15876. doi: 10.1097/MD.0000000000015876
39. Hayashi T, Fujita K, Tanigawa G, Kawashima A, Nagahara A, Ujike T, et al. Serum monocyte fraction of white blood cells is increased in patients with high Gleason score prostate cancer. Oncotarget (2017) 8(21):35255–61. doi: 10.18632/oncotarget.13052
40. Peng Y, Chen R, Qu F, Ye Y, Fu Y, Tang Z, et al. Low pretreatment lymphocyte/monocyte ratio is associated with the better efficacy of neoadjuvant chemotherapy in breast cancer patients. Cancer Biol Ther (2020) 21(2):189–96. doi: 10.1080/15384047.2019.1680057
41. Marín Hernández C, Piñero Madrona A, Gil Vázquez PJ, Galindo Fernández PJ, Ruiz Merino G, Alonso Romero JL, et al. Usefulness of lymphocyte-to-monocyte, neutrophil-to-monocyte and neutrophil-to-lymphocyte ratios as prognostic markers in breast cancer patients treated with neoadjuvant chemotherapy. Clin Trans Oncol (2018) 20(4):476–83. doi: 10.1007/s12094-017-1732-0
42. Sekine K, Kanda S, Goto Y, Horinouchi H, Fujiwara Y, Yamamoto N, et al. Change in the lymphocyte-to-monocyte ratio is an early surrogate marker of the efficacy of nivolumab monotherapy in advanced non-small-cell lung cancer. Lung Cancer (Amsterdam Netherlands) (2018) 124:179–88. doi: 10.1016/j.lungcan.2018.08.012
43. Zhu X, Wu SQ, Xu R, Wang YH, Zhong ZH, Zhang L, et al. The evaluation of monocyte lymphocyte ratio as a preoperative predictor in urothelial malignancies: a pooled analysis based on comparative studies. Sci Rep (2019) 9(1):6280. doi: 10.1038/s41598-019-42781-y
44. Kwiecień I, Rutkowska E, Polubiec-Kownacka M, Raniszewska A, Rzepecki P, Domagała-Kulawik J. Blood monocyte subsets with activation markers in relation with macrophages in non-small cell lung cancer. Cancers (2020) 12(9):2513. doi: 10.3390/cancers12092513
45. Schauer D, Starlinger P, Reiter C, Jahn N, Zajc P, Buchberger E, et al. Intermediate monocytes but not TIE2-expressing monocytes are a sensitive diagnostic indicator for colorectal cancer. PloS One (2012) 7(9):e44450. doi: 10.1371/journal.pone.0044450
46. Zhang B, Cao M, He Y, Liu Y, Zhang G, Yang C, et al. Increased circulating M2-like monocytes in patients with breast cancer. Tumour Biol (2017) 39(6):1010428317711571. doi: 10.1177/1010428317711571
47. Vuk-Pavlović S, Bulur PA, Lin Y, Qin R, Szumlanski CL, Zhao X, et al. Immunosuppressive CD14+HLA-DRlow/- monocytes in prostate cancer. Prostate (2010) 70(4):443–55. doi: 10.1002/pros.21078
48. Brooks N, Stojanovska L, Grant P, Apostolopoulos V, McDonald CF, Pouniotis DS. Characterization of blood monocyte phenotype in patients with endometrial cancer. Int J Gynecol Cancer (2012) 22(9):1500–8. doi: 10.1097/IGC.0b013e3182249273
49. Chittezhath M, Dhillon MK, Lim JY, Laoui D, Shalova IN, Teo YL, et al. Molecular profiling reveals a tumor-promoting phenotype of monocytes and macrophages in human cancer progression. Immunity (2014) 41(5):815–29. doi: 10.1016/j.immuni.2014.09.014
50. Patysheva M, Larionova I, Stakheyeva M, Grigoryeva E, Iamshchikov P, Tarabanovskaya N, et al. Effect of Early-Stage Human Breast Carcinoma on Monocyte Programming. Front Oncol (2022) 11:800235. doi: 10.3389/fonc.2021.800235
51. Zilionis R, Engblom C, Pfirschke C, Savova V, Zemmour D, Saatcioglu HD, et al. Single-cell transcriptomics of human and mouse lung cancers reveals conserved myeloid populations across individuals and species. Immunity (2019) 50(5):1317–34.e10. doi: 10.1016/j.immuni.2019.03.009
52. Kemp SB, Steele NG, Carpenter ES, Donahue KL, Bushnell GG, Morris AH, et al. Pancreatic cancer is marked by complement-high blood monocytes and tumor-associated macrophages. Life Sci Alliance (2021) 4(6):e202000935. doi: 10.26508/lsa.20200093
53. Thomas J, Torok MA, Agrawal K, Pfau T, Vu TT, Lyberger J, et al. The Neonatal Fc Receptor Is Elevated in Monocyte-Derived Immune Cells in Pancreatic Cancer. Int J Mol Sci (2022) 23(13):7066. doi: 10.3390/ijms23137066
54. Ding K, Kong X, Xu J, Jiao Y, Hu Y, Xiao Q, et al. Circulation immune landscape in canonical pathogenesis of colorectal cancer by CyTOF analysis, in: Abstracts: AACR Virtual Special Conference: Tumor Immunology and Immunotherapy; 2021,. p. 016. Philadelphia: AACR (2022). doi: 10.1158/2326-6074.TUMIMM21-P016
55. Robinson A, Han CZ, Glass CK, Pollard JW. Monocyte regulation in homeostasis and malignancy. Trends Immunol (2021) 42(2):104–19. doi: 10.1016/j.it.2020.12.001
56. Attar R, Agachan B, Kuran SB, Cacina C, Sozen S, Yurdum LM, et al. Association of CCL2 and CCR2 gene variants with endometrial cancer in Turkish women. Vivo (Athens Greece) (2010) 24(2):243–8.
57. Banin-Hirata BK, Losi-Guembarovski R, Oda JM, de Oliveira CE, Campos CZ, Mazzuco TL, et al. CCR2-V64I genetic polymorphism: a possible involvement in HER2+ breast cancer. Clin Exp Med (2016) 16(2):139–45. doi: 10.1007/s10238-015-0342-9
58. Chen MK, Yeh KT, Chiou HL, Lin CW, Chung TT, Yang SF. CCR2-64I gene polymorphism increase susceptibility to oral cancer. Oral Oncol (2011) 47(7):577–82. doi: 10.1016/j.oraloncology.2011.04.008
59. Chen Z, Yin S, Zheng L, Tang W, Kang M, Wei W, et al. Relationship between the monocyte chemo-attractant protein-1 gene rs1024611 A>G polymorphism and cancer susceptibility: A meta-analysis involving 14,617 subjects. Immunol Investigations (2021) 50(5):461–77. doi: 10.1080/08820139.2020.1776726
60. Zhang XW, Qin X, Qin CY, Yin YL, Chen Y, Zhu HL. Expression of monocyte chemoattractant protein-1 and CC chemokine receptor 2 in non-small cell lung cancer and its significance. Cancer Immunol Immunother: CII (2013) 62(3):563–70. doi: 10.1007/s00262-012-1361-y
61. Kucukgergin C, Isman FK, Dasdemir S, Cakmakoglu B, Sanli O, Gokkusu C, et al. The role of chemokine and chemokine receptor gene variants on the susceptibility and clinicopathological characteristics of bladder cancer. Gene (2012) 511(1):7–11. doi: 10.1016/j.gene.2012.09.011
62. Bianconi V, Sahebkar A, Atkin SL, Pirro M. The regulation and importance of monocyte chemoattractant protein-1. Curr Opin Hematol (2018) 25(1):44–51. doi: 10.1097/MOH.0000000000000389
63. Huang A, Zhang B, Wang B, Zhang F, Fan KX, Guo YJ. Increased CD14(+)HLA-DR-/low myeloid-derived suppressor cells correlate with extrathoracic metastasis and poor response to chemotherapy in non-small cell lung cancer patients. Cancer Immunol Immunother (2013) 62(9):1439–51. doi: 10.1007/s00262-013-1450-6
64. Chen XJ, Wu S, Yan RM, Fan LS, Yu L, Zhang YM, et al. The role of the hypoxia-Nrp-1 axis in the activation of M2-like tumor-associated macrophages in the tumor microenvironment of cervical cancer. Mol Carcinogenesis (2019) 58(3):388–97. doi: 10.1002/mc.22936
65. Tadmor T, Bari A, Marcheselli L, Sacchi S, Aviv A, Baldini L, et al. Absolute monocyte count and lymphocyte-monocyte ratio predict outcome in nodular sclerosis Hodgkin lymphoma: Evaluation based on data from 1450 patients. Mayo Clin Proc (2015) 90(6):756–64. doi: 10.1016/j.mayocp.2015.03.025
66. Ma WT, Gao F, Gu K, Chen DK. The role of monocytes and macrophages in autoimmune diseases: A comprehensive review. Front Immunol (2019) 10:1140. doi: 10.3389/fimmu.2019.01140
67. Lin CH, Chou WC, Wu YY, Lin CY, Chang KP, Liao CT, et al. Prognostic significance of dynamic changes in lymphocyte-to-monocyte ratio in patients with head and neck cancer treated with radiotherapy: results from a large cohort study. Radiother Oncol (2021) 154:76–86. doi: 10.1016/j.radonc.2020.09.012
68. Schauer D, Starlinger P, Alidzanovic L, Zajc P, Maier T, Feldman A, et al. Chemotherapy of colorectal liver metastases induces a rapid rise in intermediate blood monocytes which predicts treatment response. Oncoimmunology (2016) 5(6):e1160185. doi: 10.1080/2162402X.2016.1160185
69. Wang L, Simons DL, Lu X, Tu TY, Avalos C, Chang AY, et al. Breast cancer induces systemic immune changes on cytokine signaling in peripheral blood monocytes and lymphocytes. Ebiomedicine (2020) 52:102361. doi: 10.1016/j.ebiom.2020.102631
70. Zhang W, Ruan J, Zhou DB, Han X, Zhang Y, Wang W, et al. Predicting worse survival for newly diagnosed T cell lymphoma based on the decreased baseline CD16-/CD16+monocyte ratio. Sci Rep (2020) 10(1):7757. doi: 10.1038/s41598-020-64579-z
71. Markham MJ, Wachter K, Agarwal N, Bertagnolli MM, Chang SM, Dale W, et al. Clinical cancer advances 2020: Annual report on progress against cancer from the American society of clinical oncology. J Clin Oncol (2020) 38(10):1081–+. doi: 10.1200/JCO.19.03141
72. Stakheyeva M, Riabov V, Mitrofanova I, Litviakov N, Choynzonov E, Cherdyntseva N, et al. Role of the immune component of tumor microenvironment in the efficiency of cancer treatment: Perspectives for the personalized therapy. Curr Pharm Design (2017) 23(32):4807–26. doi: 10.2174/1381612823666170714161703
73. Zitvogel L, Galluzzi L, Smyth MJ, Kroemer G. Mechanism of action of conventional and targeted anticancer therapies: Reinstating immunosurveillance. Immunity (2013) 39(1):74–88. doi: 10.1016/j.immuni.2013.06.014
74. Coffelt SB, Kersten K, Doornebal CW, Weiden J, Vrijland K, Hau CS, et al. IL-17-producing γδ T cells and neutrophils conspire to promote breast cancer metastasis. Nature (2015) 522(7556):345–8. doi: 10.1038/nature14282
75. Galluzzi L, Humeau J, Buque A, Zitvogel L, Kroemer G. Immunostimulation with chemotherapy in the era of immune checkpoint inhibitors. Nat Rev Clin Oncol (2020) 17(12):725–41. doi: 10.1038/s41571-020-0413-z
76. Grassberger C, Ellsworth SG, Wilks MQ, Keane FK, Loeffler JS. Assessing the interactions between radiotherapy and antitumour immunity. Nat Rev Clin Oncol (2019) 16(12):729–45. doi: 10.1038/s41571-019-0238-9
77. Murdoch C, Giannoudis A, Lewis CE. Mechanisms regulating the recruitment of macrophages into hypoxic areas of tumors and other ischemic tissues. Blood (2004) 104(8):2224–34. doi: 10.1182/blood-2004-03-1109
78. Henze AT, Mazzone M. The impact of hypoxia on tumor-associated macrophages. J Clin Invest (2016) 126(10):3672–9. doi: 10.1172/JCI84427
79. Noman MZ, Desantis G, Janji B, Hasmim M, Karray S, Dessen P, et al. PD-L1 is a novel direct target of HIF-1α, and its blockade under hypoxia enhanced MDSC-mediated T cell activation. J Exp Med (2014) 211(5):781–90. doi: 10.1084/jem.20131916
80. Salas-Benito D, Pérez-Gracia JL, Ponz-Sarvisé M, Rodriguez-Ruiz ME, Martínez-Forero I, Castañón E, et al. Paradigms on immunotherapy combinations with chemotherapy. Cancer Discovery (2021) 11(6):1353–67. doi: 10.1158/2159-8290.CD-20-1312
81. Rizzo A, Ricci AD. Biomarkers for breast cancer immunotherapy: PD-L1, TILs, and beyond. Expert Opin Investigational Drugs (2022) 31(6):549–55. doi: 10.1080/13543784.2022.2008354
82. Cabié A, Fitting C, Farkas JC, Laurian C, Cormier JM, Carlet J, et al. Influence of surgery on in-vitro cytokine production by human monocytes. Cytokine (1992) 4(6):576–80. doi: 10.1016/1043-4666(92)90022-J
83. Gessler P, Pretre R, Bürki C, Rousson V, Frey B, Nadal D. Monocyte function-associated antigen expression during and after pediatric cardiac surgery. J Thorac Cardiovasc Surg (2005) 130(1):54–60. doi: 10.1016/j.jtcvs.2005.01.008
84. Feng C, Qian D, Chen C. A systematic review and meta-analysis of the effects of general anesthesia combined with continuous paravertebral block in breast cancer surgery and postoperative analgesia. Gland Surg (2021) 10(5):1713–25. doi: 10.21037/gs-21-272
85. Chen WK, Ren L, Wei Y, Zhu DX, Miao CH, Xu JM. General anesthesia combined with epidural anesthesia ameliorates the effect of fast-track surgery by mitigating immunosuppression and facilitating intestinal functional recovery in colon cancer patients. Int J Colorectal Dis (2015) 30(4):475–81. doi: 10.1007/s00384-014-2098-1
86. Kusmartsev S, Gabrilovich DI. Immature myeloid cells and cancer-associated immune suppression. Cancer Immunol Immunother: CII (2002) 51(6):293–8. doi: 10.1007/s00262-002-0280-8
87. Brusa D, Simone M, Gontero P, Spadi R, Racca P, Micari J, et al. Circulating immunosuppressive cells of prostate cancer patients before and after radical prostatectomy: Profile comparison. Int J Urol (2013) 20(10):971–8. doi: 10.1111/iju.12086
88. Woiciechowsky C, Asadullah K, Nestler D, Eberhardt B, Platzer C, Schöning B, et al. Sympathetic activation triggers systemic interleukin-10 release in immunodepression induced by brain injury. Nat Med (1998) 4(7):808–13. doi: 10.1038/nm0798-808
89. Wang J, Yang L, Yu L, Wang YY, Chen R, Qian J, et al. Surgery-induced monocytic myeloid-derived suppressor cells expand regulatory T cells in lung cancer. Oncotarget (2017) 8(10):17050–8. doi: 10.18632/oncotarget.14991
90. Arsenijević N, Baskić D, Popović S, Ristić P, Aćimović L. Preliminary study of mononuclear phagocytosis during breast cancer therapy. J BUON: Off J Balkan Union Oncol (2005) 10(1):105–9.
91. Urakawa S, Yamasaki M, Goto K, Haruna M, Hirata M, Morimoto-Okazawa A, et al. Peri-operative monocyte count is a marker of poor prognosis in gastric cancer: increased monocytes are a characteristic of myeloid-derived suppressor cells. Cancer Immunol Immunother (2019) 68(8):1341–50. doi: 10.1007/s00262-019-02366-0
92. Song Q, Wu JZ, Wang S. Postoperative monocyte count change is a better predictor of survival than preoperative monocyte count in esophageal squamous cell carcinoma. BioMed Res Int (2019) 2019:2702719. doi: 10.1155/2019/2702719
93. Hai Y, Chen N, Wu WW, Wang ZH, Lin F, Guo CL, et al. High postoperative monocyte indicates inferior clinicopathological characteristics and worse prognosis in lung adenocarcinoma or squamous cell carcinoma after lobectomy. BMC Cancer (2018) 18:1011. doi: 10.1186/s12885-018-4909-1
94. Tonouchi H, Miki C, Tanaka K, Kusunoki M. Profile of monocyte chemoattractant protein-1 circulating levels in gastric cancer patients. Scandinavian J Gastroenterol (2002) 37(7):830–3. doi: 10.1080/gas.37.7.830.833
95. Bennett S, Tai L, Alkayyal A, Sahi S, Zhang J, Ananth AA, et al. Surgery-induced expansion of myeloid derived suppressor cells leads to natural killer cell dysfunction and postoperative metastases. Canadian Society of Surgical Oncology (2014).
96. Kolseth IB, Førland DT, Risøe PK, Flood-Kjeldsen S, Ågren J, Reseland JE, et al. Human monocyte responses to lipopolysaccharide and 9-cis retinoic acid after laparoscopic surgery for colon cancer. Scandinavian J Clin Lab Invest (2012) 72(8):593–601. doi: 10.3109/00365513.2012.721520
97. Burnette B, Weichselbaum RR. Radiation as an immune modulator. Semin Radiat Oncol (2013) 23(4):273–80. doi: 10.1016/j.semradonc.2013.05.009
98. Huang RX, Zhou PK. And targets for radiotherapy sensitization in cancer. Signal Transduction Targeted Ther (2020) 5(1):60. doi:
99. Larionova I, Rakina M, Ivanyuk E, Trushchuk Y, Chernyshova A, Denisov E. Radiotherapy resistance: identifying universal biomarkers for various human cancers. J Cancer Res Clin Oncol (2022) 148(5):1015–31. doi: 10.1007/s00432-022-03923-4
100. Poon I, Erler D, Dagan R, Redmond KJ, Foote M, Badellino S, et al. Evaluation of definitive stereotactic body radiotherapy and outcomes in adults with extracranial oligometastasis. JAMA Netw Open (2020) 3(11):e2026312. doi: 10.1001/jamanetworkopen.2020.26312
101. Alamilla-Presuel JC, Burgos-Molina AM, González-Vidal A, Sendra-Portero F, Ruiz-Gómez MJ. Factors and molecular mechanisms of radiation resistance in cancer cells. Int J Radiat Biol (2022) 98(8):1–15. doi: 10.1080/09553002.2022.2047825
102. Krisnawan VE, Stanley JA, Schwarz JK, DeNardo DG. Tumor microenvironment as a regulator of radiation therapy: New insights into stromal-mediated radioresistance. Cancers (2020) 12(10). doi: 10.3390/cancers12102916
103. Suwa T, Kobayashi M, Nam JM, Harada H. Tumor microenvironment and radioresistance. Exp Mol Med (2021) 53(6):1029–35. doi: 10.1038/s12276-021-00640-9
104. Buescher ES, Gallin JI. Radiation effects on cultured human-monocytes and on monocyte-derived macrophages. Blood (1984) 63(6):1402–7. doi: 10.1182/blood.V63.6.1402.1402
105. Yoshida K, French B, Yoshida N, Hida A, Ohishi W, Kusunoki Y. Radiation exposure and longitudinal changes in peripheral monocytes over 50 years: the adult health study of atomic-bomb survivors. Br J Haematol (2019) 185(1):107–15. doi: 10.1111/bjh.15750
106. Heylmann D, Ponath V, Kindler T, Kaina B. Comparison of DNA repair and radiosensitivity of different blood cell populations. Sci Rep (2021) 11(1):2478. doi: 10.1038/s41598-021-81058-1
107. Heylmann D, Rodel F, Kindler T, Kaina B. Radiation sensitivity of human and murine peripheral blood lymphocytes, stem and progenitor cells. Biochim Et Biophys Acta-Rev Cancer (2014) 1846(1):121–9. doi: 10.1016/j.bbcan.2014.04.009
108. Zou Q, Hong W, Zhou Y, Ding Q, Wang J, Jin W, et al. Bone marrow stem cell dysfunction in radiation-induced abscopal bone loss. J Orthopaedic Surg Res (2016) 11:3. doi: 10.1186/s13018-015-0339-9
109. Linard C, Marquette C, Mathieu J, Pennequin A, Clarençon D, Mathé D. Acute induction of inflammatory cytokine expression after gamma-irradiation in the rat: effect of an NF-kappaB inhibitor. Int J Radiat Oncol Biol Physics (2004) 58(2):427–34. doi: 10.1016/j.ijrobp.2003.09.039
110. Chandra A, Park SS, Pignolo RJ. Potential role of senescence in radiation-induced damage of the aged skeleton. Bone (2019) 120:423–31. doi: 10.1016/j.bone.2018.12.006
111. Elgström E, Ohlsson TG, Eriksson SE. Cytokine evaluation in untreated and radioimmunotherapy-treated tumors in an immunocompetent rat model. Tumour Biol (2017) 39(4):1010428317697550. doi: 10.1177/1010428317697550
112. Hall EJ. Cancer caused by x-rays - a random event? Lancet Oncol (2007) 8(5):369–70. doi: 10.1016/S1470-2045(07)70113-4
113. Reisz JA, Bansal N, Qian J, Zhao WL, Furdui CM. Effects of ionizing radiation on biological molecules-mechanisms of damage and emerging methods of detection. Antioxid Redox Signaling (2014) 21(2):260–92. doi: 10.1089/ars.2013.5489
114. Berte N, Eich M, Heylmann D, Koks C, Van Gool SW, Kaina B. Impaired DNA repair in mouse monocytes compared to macrophages and precursors. DNA Repair (2021) 98. doi: 10.1016/j.dnarep.2020.103037
115. Akeem S, Lukman O, Eltahir K, Fatai O, Abiola B, Khadijat O. Bone marrow and peripheral blood cells toxicity of a single 2.0 gy Cobalt(60) ionizing radiation: An animal model. Ethiopian J Health Sci (2019) 29(2):195–202. doi: 10.4314/ejhs.v29i2.6
116. Costa S, Reagan MR. Therapeutic irradiation: Consequences for bone and bone marrow adipose tissue. Front Endocrinol (2019) 10. doi: 10.3389/fendo.2019.00587
117. Fliedner TM, Graessle D, Paulsen C, Reimers K. Structure and function of bone marrow hemopoiesis: Mechanisms of response to ionizing radiation exposure. Cancer Biother Radiopharmaceut (2002) 17(4):405–26. doi: 10.1089/108497802760363204
118. Green DE, Rubin CT. Consequences of irradiation on bone and marrow phenotypes, and its relation to disruption of hematopoietic precursors. Bone (2014) 63:87–94. doi: 10.1016/j.bone.2014.02.018
119. Benna M, Guy JB, Bosacki C, Jmour O, Ben Mrad M, Ogorodniitchouk O, et al. Chemoradiation and granulocyte-colony or granulocyte macrophage-colony stimulating factors (G-c SF or GM-CSF): time to think out of the box? Br J Radiol (1109) 2020:93. doi: 10.1259/bjr.20190147
120. Katz MS. Bystander effects and unintended consequences: Time to include the spleen in radiation therapy planning. Front Oncol (2020) 10:1171. doi: 10.3389/fonc.2020.01171
121. Chin AL, Aggarwal S, Pradhan P, Bush K, von Eyben R, Koong AC, et al. The role of bone marrow and spleen irradiation in the development of acute hematologic toxicity during chemoradiation for esophageal cancer. Adv Radiat Oncol (2018) 3(3):297–304. doi: 10.1016/j.adro.2018.02.005
122. Harrington NP, Chambers KA, Ross WM, Filion LG. Radiation damage and immune suppression in splenic mononuclear cell populations. Clin Exp Immunol (1997) 107(2):417–24. doi: 10.1111/j.1365-2249.1997.272-ce1158.x
123. Han SK, Song JY, Yun YS, Yi SY. Effect of gamma radiation on cytokine expression and cytokine-receptor mediated STAT activation. Int J Radiat Biol (2006) 82(9):686–97. doi: 10.1080/09553000600930699
124. Yang FE, Vaida F, Ignacio L, Houghton A, Nauityal J, Halpern H, et al. Analysis of weekly complete blood counts in patients receiving standard fractionated partial body radiation therapy. Int J Radiat Oncol Biol Physics (1995) 33(3):617–17. doi: 10.1016/0360-3016(95)00255-W
125. Anderson RE, Warner NL. Ionizing radiation and the immune response. Adv Immunol (1976) 24:215–335. doi: 10.1016/S0065-2776(08)60331-4
126. Jung K, Sabri S, Hanson J, Xu Y, Wang YW, Lai R, et al. Elevated ARG1 expression in primary monocytes-derived macrophages as a predictor of radiation-induced acute skin toxicities in early breast cancer patients. Cancer Biol Ther (2015) 16(9):1281–8. doi: 10.1080/15384047.2015.1056945
127. Maeng HG, Kim DN, Cho SK, Cha JH, Kim TY, Lee YS, et al. Altered immune cell proportions in the radiodermatitis induced hairless mice-1 (HR-1). J Radiat Res (2006) 47(1):9–17. doi: 10.1269/jrr.47.9
128. Garg S, Boerma M, Wang J, Fu Q, Loose DS, Kumar KS, et al. Influence of sublethal total-body irradiation on immune cell populations in the intestinal mucosa. Radiat Res (2010) 173(4):469–78. doi: 10.1667/RR1742.1
129. Loinard C, Vilar J, Milliat F, Lévy B, Benderitter M. Monocytes/Macrophages mobilization orchestrate neovascularization after localized colorectal irradiation. Radiat Res (2017) 187(5):549–61. doi: 10.1667/RR14398.1
130. Li X, Cui W, Hull L, Smith JT, Kiang JG, Xiao M. Effects of low-to-Moderate doses of gamma radiation on mouse hematopoietic system. Radiat Res (2018) 190(6):612–22. doi: 10.1667/RR15087.1
131. Rotman M, Rogow L, DeLeon G, Heskel N. Supportive therapy in radiation oncology. Cancer (1977) 39(2 Suppl):744–50. doi: 10.1002/1097-0142(197702)39:2+<744::AID-CNCR2820390709>3.0.CO;2-R
132. Plowman PN. The effects of conventionally fractionated, extended portal radiotherapy on the human peripheral blood count. Int J Radiat Oncol Biol Physics (1983) 9(6):829–39. doi: 10.1016/0360-3016(83)90008-1
133. Smit B, Stjernswärd J, Dowdle E, Sealy GR, Wilson E, Beatty D, et al. The lymphocyte: monocyte ratio: B- and T-cell ratio after radiotherapy, chemotherapy and surgery. Int J Radiat Oncol Biol Physics (1979) 5(10):1841–7. doi: 10.1016/0360-3016(79)90569-8
134. Bauer M, Goldstein M, Christmann M, Becker H, Heylmann D, Kaina B. Human monocytes are severely impaired in base and DNA double-strand break repair that renders them vulnerable to oxidative stress. Proc Natl Acad Sci United States America (2011) 108(52):21105–10. doi: 10.1073/pnas.1111919109
135. Kappler M, Gerry AB, Brown E, Reid L, Leake DS, Gieseg SP. Aqueous peroxyl radical exposure to THP-1 cells causes glutathione loss followed by protein oxidation and cell death without increased caspase-3 activity. Biochim Biophys Acta (2007) 1773(6):945–53. doi: 10.1016/j.bbamcr.2007.04.001
136. Xu JY, Escamilla J, Mok S, David J, Priceman S, West B, et al. CSF1R signaling blockade stanches tumor-infiltrating myeloid cells and improves the efficacy of radiotherapy in prostate cancer. Cancer Res (2013) 73(9):2782–94. doi: 10.1158/0008-5472.CAN-12-3981
137. Kalbasi A, Komar C, Tooker GM, Liu M, Lee JW, Gladney WL, et al. Tumor-derived CCL2 mediates resistance to radiotherapy in pancreatic ductal adenocarcinoma. Clin Cancer Res (2017) 23(1):137–48. doi: 10.1158/1078-0432.CCR-16-0870
138. Shin SC, Lee KM, Kang YM, Kim K, Kim CS, Yang KH, et al. Alteration of cytokine profiles in mice exposed to chronic low-dose ionizing radiation. Biochem Biophys Res Commun (2010) 397(4):644–9. doi: 10.1016/j.bbrc.2010.05.121
139. Mondini M, Loyher PL, Hamon P, de Thore MG, Laviron M, Berthelot K, et al. CCR2-dependent recruitment of tregs and monocytes following radiotherapy is associated with TNF alpha-mediated resistance. Cancer Immunol Res (2019) 7(3):376–87. doi: 10.1158/2326-6066.CIR-18-0633
140. Vempati P, Puckett L, Evans C, Dassler-Plenker J, Curtis J, Egeblad M. A potential synergistic role of radiation therapy with targeting of the CCL2-CCR2 signaling axis in a murine model of breast cancer. Int J Radiat Oncol Biol Physics (2020) 108(3):E554–E5. doi: 10.1016/j.ijrobp.2020.07.1719
141. Kalbasi A, Komar C, Tooker GM, Liu M, Lee JW, Gladney WL, et al. Tumor-derived CCL2 mediates resistance to radiotherapy in pancreatic ductal adenocarcinoma. Clin Cancer Res (2017) 23(1):137–48. doi: 10.1158/1078-0432.CCR-16-0870
142. Kang JH, Woo JK, Jang YS, Oh SH. Radiation potentiates monocyte infiltration into tumors by Ninjurin1 expression in endothelial cells. Cells (2020) 9(5):1086. doi: 10.3390/cells9051086
143. Suwa T, Kobayashi M, Nam JM, Harada H. Tumor microenvironment and radioresistance. Exp Mol Med (2021) 53(6):1029–35. doi: 10.1038/s12276-021-00640-9
144. Lv W, Chen N, Lin Y, Ma H, Ruan Y, Li Z, et al. Macrophage migration inhibitory factor promotes breast cancer metastasis via activation of HMGB1/TLR4/NF kappa b axis. Cancer Lett (2016) 375(2):245–55. doi: 10.1016/j.canlet.2016.02.005
145. Yoshino H, Chiba K, Saitoh T, Kashiwakura I. Ionizing radiation affects the expression of toll-like receptors 2 and 4 in human monocytic cells through c-jun n-terminal kinase activation. J Radiat Res (2014) 55(5):876–84. doi: 10.1093/jrr/rru040
146. Kwan DK, Norman A. Radiosensitivity of large human monocytes. Radiat Res (1978) 75(3):556–62. doi: 10.2307/3574842
147. Standish LJ, Torkelson C, Hamill FA, Yim D, Hill-Force A, Fitzpatrick A, et al. Immune defects in breast cancer patients after radiotherapy. J Soc Integr Oncol (2008) 6(3):110–21.
148. Calabrese EJ, Mattson MP. How does hormesis impact biology, toxicology, and medicine? NPJ Aging Mech Dis (2017) 3:13. doi: 10.1038/s41514-017-0013-z
149. Teresa Pinto A, Laranjeiro Pinto M, Patrícia Cardoso A, Monteiro C, Teixeira Pinto M, Filipe Maia A, et al. Ionizing radiation modulates human macrophages towards a pro-inflammatory phenotype preserving their pro-invasive and pro-angiogenic capacities. Sci Rep (2016) 6:18765. doi: 10.1038/srep18765
150. Wu Q, Allouch A, Paoletti A, Leteur C, Mirjolet C, Martins I, et al. NOX2-dependent ATM kinase activation dictates pro-inflammatory macrophage phenotype and improves effectiveness to radiation therapy. Cell Death Differentiation (2017) 24(9):1632–44. doi: 10.1038/cdd.2017.91
151. Parker JJ, Jones JC, Strober S, Knox SJ. Characterization of direct radiation-induced immune function and molecular signaling changes in an antigen presenting cell line. Clin Immunol (2013) 148(1):44–55. doi: 10.1016/j.clim.2013.03.008
152. El-Saghire H, Michaux A, Thierens H, Baatout S. Low doses of ionizing radiation induce immune-stimulatory responses in isolated human primary monocytes. Int J Mol Med (2013) 32(6):1407–14. doi: 10.3892/ijmm.2013.1514
153. Coates PJ, Rundle JK, Lorimore SA, Wright EG. Indirect macrophage responses to ionizing radiation: Implications for genotype-dependent bystander signaling. Cancer Res (2008) 68(2):450–6. doi: 10.1158/0008-5472.CAN-07-3050
154. Tsai CS, Chen FH, Wang CC, Huang HL, Jung SM, Wu CJ, et al. Macrophages from irradiated tumors express higher levels of iNOS, arginase-I and COX-2, and promote tumor growth. Int J Radiat Oncol Biol Physics (2007) 68(2):499–507. doi: 10.1016/j.ijrobp.2007.01.041
155. Frey B, Rückert M, Weber J, Mayr X, Derer A, Lotter M, et al. Hypofractionated irradiation has immune stimulatory potential and induces a timely restricted infiltration of immune cells in colon cancer tumors. Front Immunol (2017) 8:231. doi: 10.3389/fimmu.2017.00231
156. Kang C, Jeong SY, Song SY, Choi EK. The emerging role of myeloid-derived suppressor cells in radiotherapy. Radiat Oncol J (2020) 38(1):1–10. doi: 10.3857/roj.2019.00640
157. Ostrand-Rosenberg S, Horn LA, Ciavattone NG. Radiotherapy both promotes and inhibits myeloid-derived suppressor cell function: Novel strategies for preventing the tumor-protective effects of radiotherapy. Front Oncol (2019) 9:215. doi: 10.3389/fonc.2019.00215
158. Chen HM, Ma G, Gildener-Leapman N, Eisenstein S, Coakley BA, Ozao J, et al. Myeloid-derived suppressor cells as an immune parameter in patients with concurrent sunitinib and stereotactic body radiotherapy. Clin Cancer Res (2015) 21(18):4073–85. doi: 10.1158/1078-0432.CCR-14-2742
159. Mantovani A, Marchesi F, Malesci A, Laghi L, Allavena P. Tumour-associated macrophages as treatment targets in oncology. Nat Rev Clin Oncol (2017) 14(7):399–416. doi: 10.1038/nrclinonc.2016.217
160. Petroni G, Buque A, Zitvogel L, Kroemer G, Galluzzi L. Immunomodulation by targeted anticancer agents. Cancer Cell (2020) 39(3):310–45. doi: 10.1016/j.ccell.2020.11.009
161. DeNardo DG, Brennan DJ, Rexhepaj E, Ruffell B, Shiao SL, Madden SF, et al. Leukocyte complexity predicts breast cancer survival and functionally regulates response to chemotherapy. Cancer Discov (2011) 1(1):54–67. doi: 10.1158/2159-8274.CD-10-0028
162. Kioi M, Shimamura T, Nakashima H, Hirota M, Tohnai I, Husain SR, et al. IL-13 cytotoxin has potent antitumor activity and synergizes with paclitaxel in a mouse model of oral squamous cell carcinoma. Int J Cancer (2009) 124(6):1440–8. doi: 10.1002/ijc.24067
163. Nakasone ES, Askautrud HA, Kees T, Park JH, Plaks V, Ewald AJ, et al. Imaging tumor-stroma interactions during chemotherapy reveals contributions of the microenvironment to resistance. Cancer Cell (2012) 21(4):488–503. doi: 10.1016/j.ccr.2012.02.017
164. Keklikoglou I, Cianciaruso C, Güç E, Squadrito ML, Spring LM, Tazzyman S, et al. Chemotherapy elicits pro-metastatic extracellular vesicles in breast cancer models. Nat Cell Biol (2019) 21(2):190–202. doi: 10.1038/s41556-018-0256-3
165. Ding ZC, Aboelella NS, Bryan L, Shi H, Zhou G. The monocytes that repopulate in mice after cyclophosphamide treatment acquire a neutrophil precursor gene signature and immunosuppressive activity. Front Immunol (2020) 11:594540. doi: 10.3389/fimmu.2020.594540
166. Ushach I, Zlotnik A. Biological role of granulocyte macrophage colony-stimulating factor (GM-CSF) and macrophage colony-stimulating factor (M-CSF) on cells of the myeloid lineage. J Leukoc Biol (2016). doi: 10.1189/jlb.3RU0316-144R
167. Geller MA, Bui-Nguyen TM, Rogers LM, Ramakrishnan S. Chemotherapy induces macrophage chemoattractant protein-1 production in ovarian cancer. Int J Gynecol Cancer (2010) 20(6):918–25. doi: 10.1111/IGC.0b013e3181e5c442
168. Hughes R, Qian BZ, Rowan C, Muthana M, Keklikoglou I, Olson OC, et al. Perivascular M2 macrophages stimulate tumor relapse after chemotherapy. Cancer Res (2015) 75(17):3479–91. doi: 10.1158/0008-5472.CAN-14-3587
169. Liu L, Yang L, Yan W, Zhai J, Pizzo DP, Chu PG, et al. Chemotherapy induces breast cancer stemness in association with dysregulated monocytosis. Clin Cancer Res (2018) 24(10):2370–82. doi: 10.1158/1078-0432.CCR-17-2545
170. Liu TF, Larionova I, Litviakov N, Riabov V, Zavyalova M, Tsyganov M, et al. Tumor-associated macrophages in human breast cancer produce new monocyte attracting and pro-angiogenic factor YKL-39 indicative for increased metastasis after neoadjuvant chemotherapy. Oncoimmunology (2018) 7(6):e1436922. doi: 10.1080/2162402X.2018.1436922
171. Swierczak A, Cook AD, Lenzo JC, Restall CM, Doherty JP, Anderson RL, et al. The promotion of breast cancer metastasis caused by inhibition of CSF-1R/CSF-1 signaling is blocked by targeting the G-CSF receptor. Cancer Immunol Res (2014) 2(8):765–76. doi: 10.1158/2326-6066.CIR-13-0190
172. Ao JY, Zhu XD, Chai ZT, Cai H, Zhang YY, Zhang KZ, et al. Colony-stimulating factor 1 receptor blockade inhibits tumor growth by altering the polarization of tumor-associated macrophages in hepatocellular carcinoma. Mol Cancer Ther (2017) 16(8):1544–54. doi: 10.1158/1535-7163.MCT-16-0866
173. Li M, Li MM, Yang YL, Liu YK, Xie HB, Yu QW, et al. Remodeling tumor immune microenvironment via targeted blockade of PI3K-gamma and CSF-1/CSF-1R pathways in tumor associated macrophages for pancreatic cancer therapy. J Controlled Release (2020) 321:23–35. doi: 10.1016/j.jconrel.2020.02.011
174. Przystal JM, Becker H, Canjuga D, Tsiami F, Anderle N, Keller AL, et al. Targeting CSF1R alone or in combination with PD1 in experimental glioma. Cancers (2021) 13(10):2400. doi: 10.3390/cancers13102400
175. Ries CH, Cannarile MA, Hoves S, Benz J, Wartha K, Runza V, et al. Targeting tumor-associated macrophages with anti-CSF-1R antibody reveals a strategy for cancer therapy. Cancer Cell (2014) 25(6):846–59. doi: 10.1016/j.ccr.2014.05.016
176. Mitchem JB, Brennan DJ, Knolhoff BL, Belt BA, Zhu Y, Sanford DE, et al. Targeting tumor-infiltrating macrophages decreases tumor-initiating cells, relieves immunosuppression, and improves chemotherapeutic responses. Cancer Res (2013) 73(3):1128–41. doi: 10.1158/0008-5472.CAN-12-2731
177. Loberg RD, Ying C, Craig M, Day LL, Sargent E, Neeley C, et al. Targeting CCL2 with systemic delivery of neutralizing antibodies induces prostate cancer tumor regression in vivo. Cancer Res (2007) 67(19):9417–24. doi: 10.1158/0008-5472.CAN-07-1286
178. Soeda A, Morita-Hoshi Y, Makiyama H, Morizane C, Ueno H, Ikeda M, et al. Regular dose of gemcitabine induces an increase in CD14+ monocytes and CD11c+ dendritic cells in patients with advanced pancreatic cancer. Japanese J Clin Oncol (2009) 39(12):797–806. doi: 10.1093/jjco/hyp112
179. Moriyama Y, Horita N, Kudo M, Shinkai M, Fujita H, Yamanaka T, et al. Monocyte nadir is a possible indicator for neutrophil nadir during lung cancer chemotherapy. Clin Respir J (2017) 11(4):453–8. doi: 10.1111/crj.12358
180. Ouyang W, Liu Y, Deng D, Zhou F, Xie C. The change in peripheral blood monocyte count: A predictor to make the management of chemotherapy-induced neutropenia. J Cancer Res Ther (2018) 14(Supplement):S565–s70. doi: 10.4103/0973-1482.177502
181. Kzhyshkowska J, Gudima A, Moganti K, Gratchev A, Orekhov A. Perspectives for Monocyte/Macrophage-based diagnostics of chronic inflammation. Transfusion Med Hemother (2016) 43(2):66–77. doi: 10.1159/000444943
182. Moriyama Y, Horita N, Kudo M, Shinkai M, Fujita H, Yamanaka T, et al. Monocyte nadir is a possible indicator for neutrophil nadir during lung cancer chemotherapy. Clin Respir J (2017) 11(4):453–8. doi: 10.1111/crj.12358
183. Valdés-Ferrada J, Muñoz-Durango N, Pérez-Sepulveda A, Muñiz S, Coronado-Arrázola I, Acevedo F, et al. Peripheral blood classical monocytes and plasma interleukin 10 are associated to neoadjuvant chemotherapy response in breast cancer patients. Front Immunol (2020) 11:1413. doi: 10.3389/fimmu.2020.01413
184. Massa C, Karn T, Denkert C, Schneeweiss A, Hanusch C, Blohmer JU, et al. Differential effect on different immune subsets of neoadjuvant chemotherapy in patients with TNBC. J Immunother Cancer (2020) 8(2):e001261. doi: 10.1136/jitc-2020-001261
185. Liu L, Yang L, Yan W, Zhai J, Pizzo DP, Chu P, et al. Chemotherapy induces breast cancer stemness in association with dysregulated monocytosis. Clin Cancer Res (2018) 24(10):2370–82. doi: 10.1158/1078-0432.CCR-17-2545
186. Shimanuki M, Imanishi Y, Sato Y, Nakahara N, Totsuka D, Sato E, et al. Pretreatment monocyte counts and neutrophil counts predict the risk for febrile neutropenia in patients undergoing TPF chemotherapy for head and neck squamous cell carcinoma. Oncotarget (2018) 9(27):18970–84. doi: 10.18632/oncotarget.24863
187. Schirrmacher V. From chemotherapy to biological therapy: A review of novel concepts to reduce the side effects of systemic cancer treatment. Int J Oncol (2018) 54(2):407–19. doi: 10.3892/ijo.2018.4661
188. Cherdyntseva NV, Mitrofanova IV, Buldakov MA, Stakheeva MN, Patysheva MR, Zavjalova MV, et al. Macrophages and tumor progression: on the way to macrophage-specif therapy. Byulleten Sibirskoy Meditsiny (2017) 16(4):61–74. doi: 10.20538/1682-0363-2017-4-61-74
189. Patysheva M, Stakheyeva M, Larionova I, Fedorov A, Kzhyshkowska J, N. C. Cytostatic cancer therapy modulates monocyte-macrophage cell functions: how it impacts on treatment outcomes. Exp Oncol (2019). doi: 10.32471/exp-oncology.2312-8852.vol-41-no-3.13597
190. Larsson AM, Roxå A, Leandersson K, Bergenfelz C. Impact of systemic therapy on circulating leukocyte populations in patients with metastatic breast cancer. Sci Rep (2019) 9(1):13451. doi: 10.1038/s41598-019-49943-y
191. Hu X, Gu Y, Zhao S, Hua S, Jiang Y. Increased IL-10+CD206+CD14+M2-like macrophages in alveolar lavage fluid of patients with small cell lung cancer. Cancer Immunol Immunother: CII (2020) 69(12):2547–60. doi: 10.1007/s00262-020-02639-z
192. Huang A, Zhang B, Wang B, Zhang F, Fan KX, Guo YJ. Increased CD14(+)HLA-DR (-/low) myeloid-derived suppressor cells correlate with extrathoracic metastasis and poor response to chemotherapy in non-small cell lung cancer patients. Cancer Immunol Immunother: CII (2013) 62(9):1439–51. doi: 10.1007/s00262-013-1450-6
193. Stenzel AE, Abrams SI, Joseph JM, Goode EL, Tario JD Jr., Wallace PK, et al. Circulating CD14(+) HLA-DR(lo/-) monocytic cells as a biomarker for epithelial ovarian cancer progression. Am J Reprod Immunol (New York NY: 1989) (2021) 85(3):e13343. doi: 10.1111/aji.13343
194. Steinberger KJ, Forget MA, Bobko AA, Mihalik NE, Gencheva M, Roda JM, et al. Hypoxia-inducible factor α subunits regulate Tie2-expressing macrophages that influence tumor oxygen and perfusion in murine breast cancer. J Immunol (Baltimore Md: 1950) (2020) 205(8):2301–11. doi: 10.4049/jimmunol.2000185
195. Zhao K, Wang C, Shi F, Li M, Yu J. Lymphocyte-monocyte ratio as a predictive marker for pathological complete response to neoadjuvant therapy in esophageal squamous cell carcinoma. Trans Cancer Res (2020) 9(6):3842–53. doi: 10.21037/tcr-19-2849
196. Talamantes S, Xie E, Costa RLB, Chen M, Rademaker A, Santa-Maria CA. Circulating immune cell dynamics in patients with triple negative breast cancer treated with neoadjuvant chemotherapy. Cancer Med (2020) 9(19):6954–60. doi: 10.1002/cam4.3358
197. Feng PH, Lee KY, Chang YL, Chan YF, Kuo LW, Lin TY, et al. CD14(+)S100A9(+) monocytic myeloid-derived suppressor cells and their clinical relevance in non-small cell lung cancer. Am J Respir Crit Care Med (2012) 186(10):1025–36. doi: 10.1164/rccm.201204-0636OC
198. De Palma M, Lewis CE. Macrophage regulation of tumor responses to anticancer therapies. Cancer Cell (2013) 23(3):277–86. doi: 10.1016/j.ccr.2013.02.013
199. Mantovani A, Allavena P. The interaction of anticancer therapies with tumor-associated macrophages. J Exp Med (2015) 212(4):435–45. doi: 10.1084/jem.20150295
200. Cao Q, Yan X, Chen K, Huang Q, Melancon MP, Lopez G, et al. Macrophages as a potential tumor-microenvironment target for noninvasive imaging of early response to anticancer therapy. Biomaterials (2018) 152:63–76. doi: 10.1016/j.biomaterials.2017.10.036
201. Takeuchi S, Baghdadi M, Tsuchikawa T, Wada H, Nakamura T, Abe H, et al. Chemotherapy-derived inflammatory responses accelerate the formation of immunosuppressive myeloid cells in the tissue microenvironment of human pancreatic cancer. Cancer Res (2015) 75(13):2629–40. doi: 10.1158/0008-5472.CAN-14-2921
202. Lotfi N, Zhang GX, Esmaeil N, Rostami A. Evaluation of the effect of GM-CSF blocking on the phenotype and function of human monocytes. Sci Rep (2020) 10(1):1567. doi: 10.1038/s41598-020-58131-2
203. Wang Z, Liu Y, Zhang Y, Shang Y, Gao Q. MDSC-decreasing chemotherapy increases the efficacy of cytokine-induced killer cell immunotherapy in metastatic renal cell carcinoma and pancreatic cancer. Oncotarget (2016) 7(4):4760–9. doi: 10.18632/oncotarget.6734
204. Wu C, Tan X, Hu X, Zhou M, Yan J, Ding C. Tumor microenvironment following gemcitabine treatment favors differentiation of immunosuppressive Ly6C(high) myeloid cells. J Immunol (Baltimore Md: 1950) (2020) 204(1):212–23. doi: 10.4049/jimmunol.1900930
205. Dijkgraaf EM, Heusinkveld M, Tummers B, Vogelpoel LT, Goedemans R, Jha V, et al. Chemotherapy alters monocyte differentiation to favor generation of cancer-supporting M2 macrophages in the tumor microenvironment. Cancer Res (2013) 73(8):2480–92. doi: 10.1158/0008-5472.CAN-12-3542
206. Litviakov N, Tsyganov M, Larionova I, Ibragimova M, Deryusheva I, Kazantseva P, et al. Expression of M2 macrophage markers YKL-39 and CCL18 in breast cancer is associated with the effect of neoadjuvant chemotherapy. Cancer Chemother Pharmacol (2018) 82(1):99–109. doi: 10.1007/s00280-018-3594-8
207. Koprowski H, Steplewski Z, Herlyn D, Herlyn M. Study of antibodies against human melanoma produced by somatic-cell hybrids. Proc Natl Acad Sci United States America (1978) 75(7):3405–9. doi: 10.1073/pnas.75.7.3405
208. Nadler LM, Stashenko P, Hardy R, Kaplan WD, Button LN, Kufe DW, et al. Serotherapy of a patient with a monoclonal-antibody directed against a human lymphoma-associated antigen. Cancer Res (1980) 40(9):3147–54.
209. Coiffier B, Lepretre S, Pedersen LM, Gadeberg O, Fredriksen H, van Oers MHJ, et al. Safety and efficacy of ofatumumab, a fully human monoclonal anti-CD20 antibody, in patients with relapsed or refractory b-cell chronic lymphocytic leukemia: a phase 1-2 study. Blood (2008) 111(3):1094–100. doi: 10.1182/blood-2007-09-111781
210. Sofia F, Diamandis EP, Blasutig IM. Cancer immunotherapy: the beginning of the end of cancer? Cancer Discov (2016). doi: 10.1186/s12916-016-0623-5
211. Peters S, Mok T, Passaro A, Jänne PA. The promising evolution of targeted therapeutic strategies in cancer. (2021). doi: 10.1158/2159-8290.CD-21-0124
212. Duffy MJ, Crown J. Biomarkers for predicting response to immunotherapy with immune checkpoint inhibitors in cancer patients. Clin Chem (2019) 65(10):1228–38. doi: 10.1373/clinchem.2019.303644
213. Leon-Mateos L, Garcia-Velloso MJ, García-Figueiras R, Rodriguez-Moreno JF, Vercher-Conejero JL, Sánchez M, et al. A multidisciplinary consensus on the morphological and functional responses to immunotherapy treatment. Clin Trans Oncol (2021) 23(3):434–49. doi: 10.1007/s12094-020-02442-3
214. Fu Y, Liu S, Zeng S, Shen H. From bench to bed: the tumor immune microenvironment and current immunotherapeutic strategies for hepatocellular carcinoma. J Exp Clin Cancer Res (2019) 38(1):396. doi: 10.1186/s13046-019-1396-4
215. Green DS, Nunes AT, David-Ocampo V, Ekwede IB, Houston ND, Highfill SL, et al. A phase 1 trial of autologous monocytes stimulated ex vivo with sylatron(®) (Peginterferon alfa-2b) and actimmune(®) (Interferon gamma-1b) for intra-peritoneal administration in recurrent ovarian cancer. J Trans Med (2018) 16(1):196. doi: 10.1186/s12967-018-1569-5
216. Pardoll DM. The blockade of immune checkpoints in cancer immunotherapy. Nat Rev Cancer (2012) 12(4):252–64. doi: 10.1038/nrc3239
217. Kiaie SH, Sanaei MJ, Heshmati M, Asadzadeh Z, Azimi I, Hadidi S, et al. Immune checkpoints in targeted-immunotherapy of pancreatic cancer: New hope for clinical development. Acta Pharm Sin B (2021) 11(5):1083–97. doi: 10.1016/j.apsb.2020.12.011
218. Xiong H, Mittman S, Rodriguez R, Moskalenko M, Pacheco-Sanchez P, Yang Y, et al. Anti-PD-L1 treatment results in functional remodeling of the macrophage compartment. Cancer Res (2019) 79(7):1493–506. doi: 10.1158/0008-5472.CAN-18-3208
219. Schetters STT, Rodriguez E, Kruijssen LJW, Crommentuijn MHW, Boon L, Van den Bossche J, et al. Monocyte-derived APCs are central to the response of PD1 checkpoint blockade and provide a therapeutic target for combination therapy. J Immunother Cancer (2020) 8(2):e000588. doi: 10.1136/jitc-2020-000588
220. Limagne E, Thibaudin M, Nuttin L, Spill A, Derangère V, Fumet JD, et al. Trifluridine/Tipiracil plus oxaliplatin improves PD-1 blockade in colorectal cancer by inducing immunogenic cell death and depleting macrophages. Cancer Immunol Res (2019) 7(12):1958–69. doi: 10.1158/2326-6066.CIR-19-0228
221. Shao Y, Lin S, Zhang P, Zhang J, Ji D, Tao Z, et al. Baseline monocyte and its classical subtype may predict efficacy of PD-1/PD-L1 inhibitor in cancers. Biosci Rep (2021) 41(1):BSR20202613. doi: 10.1042/BSR20202613
222. Krieg C, Nowicka M, Guglietta S, Schindler S, Hartmann FJ, Weber LM, et al. High-dimensional single-cell analysis predicts response to anti-PD-1 immunotherapy. Nat Med (2018) 24(2):144–53. doi: 10.1038/nm.4466
223. Kiaie SH, Sanaei MJ, Heshmati M, Asadzadeh Z, Azimi I, Hadidi S, et al. Immune checkpoints in targeted-immunotherapy of pancreatic cancer: New hope for clinical development. Acta Pharm Sin B (2021) 11(5):1083–97. doi: 10.1016/j.apsb.2020.12.011
224. Peggs KS, Quezada SA, Allison JP. Cell intrinsic mechanisms of T-cell inhibition and application to cancer therapy. Immunol Rev (2008) 224:141–65. doi: 10.1111/j.1600-065X.2008.00649.x
225. Eissler N, Mao Y, Brodin D, Reuterswärd P, Andersson Svahn H, Johnsen JI, et al. Regulation of myeloid cells by activated T cells determines the efficacy of PD-1 blockade. Oncoimmunology (2016) 5(12):e1232222. doi: 10.1080/2162402X.2016.1232222
226. Mengos AE, Gastineau DA, Gustafson MP. The CD14(+)HLA-DRlo/neg monocyte: An immunosuppressive phenotype that restrains responses to cancer immunotherapy. Front Immunol (2019) 10. doi: 10.3389/fimmu.2019.01147
227. Meyer C, Cagnon L, Costa-Nunes CM, Baumgaertner P, Montandon N, Leyvraz L, et al. Frequencies of circulating MDSC correlate with clinical outcome of melanoma patients treated with ipilimumab. Cancer Immunol Immunother: CII (2014) 63(3):247–57. doi: 10.1007/s00262-013-1508-5
228. Kitano S, Postow MA, Ziegler CG, Kuk D, Panageas KS, Cortez C, et al. Computational algorithm-driven evaluation of monocytic myeloid-derived suppressor cell frequency for prediction of clinical outcomes. Cancer Immunol Res (2014) 2(8):812–21. doi: 10.1158/2326-6066.CIR-14-0013
229. Gebhardt C, Sevko A, Jiang H, Lichtenberger R, Reith M, Tarnanidis K, et al. Myeloid cells and related chronic inflammatory factors as novel predictive markers in melanoma treatment with ipilimumab. Clin Cancer Res: Off J Am Assoc Cancer Res (2015) 21(24):5453–9. doi: 10.1158/1078-0432.CCR-15-0676
230. Ando K, Hamada K, Shida M, Ohkuma R, Kubota Y, Horiike A, et al. A high number of PD-L1(+) CD14(+) monocytes in peripheral blood is correlated with shorter survival in patients receiving immune checkpoint inhibitors. Cancer Immunol Immunother: CII (2021) 70(2):337–48. doi: 10.1007/s00262-020-02686-6
231. Riemann D, Schütte W, Turzer S, Seliger B, Möller M. High PD-L1/CD274 expression of monocytes and blood dendritic cells is a risk factor in lung cancer patients undergoing treatment with PD1 inhibitor therapy. Cancers (2020) 12(10):2966. doi: 10.3390/cancers12102966
232. Minard-Colin V, Xiu Y, Poe JC, Horikawa M, Magro CM, Hamaguchi Y, et al. Lymphoma depletion during CD20 immunotherapy in mice is mediated by macrophage FcgammaRI, FcgammaRIII, and FcgammaRIV. Blood (2008) 112(4):1205–13. doi: 10.1182/blood-2008-01-135160
233. Deronic A, Leanderson T, Ivars F. The anti-tumor effect of the quinoline-3-carboxamide tasquinimod: blockade of recruitment of CD11b(+) Ly6C(hi) cells to tumor tissue reduces tumor growth. BMC Cancer (2016) 16:440. doi: 10.1186/s12885-016-2481-0
234. Limagne E, Thibaudin M, Nuttin L, Spill A, Derangere V, Fumet J-D, et al. Trifluridine/Tipiracil plus oxaliplatin improves PD-1 blockade in colorectal cancer by inducing immunogenic cell death and depleting macrophages. Cancer Immunol Res (2019) 7(12):1958–69. doi: 10.1158/2326-6066.CIR-19-0228
235. Pienta KJ, Machiels JP, Schrijvers D, Alekseev B, Shkolnik M, Crabb SJ, et al. Phase 2 study of carlumab (CNTO 888), a human monoclonal antibody against CC-chemokine ligand 2 (CCL2), in metastatic castration-resistant prostate cancer. Investigational New Drugs (2013) 31(3):760–8. doi: 10.1007/s10637-012-9869-8
236. Brana I, Calles A, LoRusso PM, Yee LK, Puchalski TA, Seetharam S, et al. Carlumab, an anti-C-C chemokine ligand 2 monoclonal antibody, in combination with four chemotherapy regimens for the treatment of patients with solid tumors: an open-label, multicenter phase 1b study. Targeted Oncol (2015) 10(1):111–23. doi: 10.1007/s11523-014-0320-2
237. Bonapace L, Coissieux MM, Wyckoff J, Mertz KD, Varga Z, Junt T, et al. Cessation of CCL2 inhibition accelerates breast cancer metastasis by promoting angiogenesis. Nature (2014) 515(7525):130–3. doi: 10.1038/nature13862
238. Zitvogel L, Kepp O, Kroemer G. Immune parameters affecting the efficacy of chemotherapeutic regimens. Nat Rev Clin Oncol (2011) 8(3):151–60. doi: 10.1038/nrclinonc.2010.223
239. Uchida J, Hamaguchi Y, Oliver JA, Ravetch JV, Poe JC, Haas KM, et al. The innate mononuclear phagocyte network depletes b lymphocytes through fc receptor-dependent mechanisms during anti-CD20 antibody immunotherapy. J Exp Med (2004) 199(12):1659–69. doi: 10.1084/jem.20040119
240. Cittera E, Leidi M, Buracchi C, Pasqualini F, Sozzani S, Vecchi A, et al. The CCL3 family of chemokines and innate immunity cooperate in vivo in the eradication of an established lymphoma xenograft by rituximab. J Immunol (Baltimore Md: 1950) (2007) 178(10):6616–23. doi: 10.4049/jimmunol.178.10.6616
241. Braster R, O’Toole T, van Egmond M. Myeloid cells as effector cells for monoclonal antibody therapy of cancer. Methods (San Diego Calif) (2014) 65(1):28–37. doi: 10.1016/j.ymeth.2013.06.020
242. Beatty GL, Chiorean EG, Fishman MP, Saboury B, Teitelbaum UR, Sun W, et al. CD40 agonists alter tumor stroma and show efficacy against pancreatic carcinoma in mice and humans. Sci (New York NY) (2011) 331(6024):1612–6. doi: 10.1126/science.1198443
243. Gubin MM, Esaulova E, Ward JP, Malkova ON, Runci D, Wong P, et al. High-dimensional analysis delineates myeloid and lymphoid compartment remodeling during successful immune-checkpoint cancer therapy. Cell (2018) 175(4):1014–30.e19. doi: 10.1016/j.cell.2018.09.030
244. Elsässer D, Valerius T, Repp R, Weiner GJ, Deo Y, Kalden JR, et al. HLA class II as potential target antigen on malignant b cells for therapy with bispecific antibodies in combination with granulocyte colony-stimulating factor. Blood (1996) 87(9):3803–12. doi: 10.1182/blood.V87.9.3803.bloodjournal8793803
245. Sedykh SE, Prinz VV, Buneva VN, Nevinsky GA. Bispecific antibodies: design, therapy, perspectives. Drug Design Dev Ther (2018) 12:195–208. doi: 10.2147/DDDT.S151282
246. Borlak J, Länger F, Spanel R, Schöndorfer G, Dittrich C. Immune-mediated liver injury of the cancer therapeutic antibody catumaxomab targeting EpCAM, CD3 and fcγ receptors. Oncotarget (2016) 7(19):28059–74. doi: 10.18632/oncotarget.8574
247. Wu HH, Lee TH, Tee YT, Chen SC, Yang SF, Lee SK, et al. Relationships of single nucleotide polymorphisms of monocyte chemoattractant protein 1 and chemokine receptor 2 with susceptibility and clinicopathologic characteristics of neoplasia of uterine cervix in Taiwan women. Reprod Sci (Thousand Oaks Calif) (2013) 20(10):1175–83. doi: 10.1177/1933719113477481
248. Cambui RAG, Fernandes FP, Leal VNC, Reis EC, de Lima DS, do Espírito Santo GF, et al. The Ala134Thr variant in TMEM176B exerts a beneficial role in colorectal cancer prognosis by increasing NLRP3 inflammasome activation. J Cancer Res Clin Oncol (2022) 1:1–10. doi: 10.1007/s00432-022-04284-8
249. Siamak S, Sunakawa Y, Loupakis F, Yang D, Zhang W, Ning Y, et al. CCL2 polymorphism as a predictive marker for bevacizumab (Bev) in combination with FOLFIRI as first-line treatment in metastatic colorectal cancer (mCRC) patients (pts). J Clin Oncol (2014) 32(15_suppl):e14556–e. doi: 10.1200/jco.2014.32.15_suppl.e14556
250. Apetoh L, Ghiringhelli F, Tesniere A, Obeid M, Ortiz C, Criollo A, et al. Toll-like receptor 4-dependent contribution of the immune system to anticancer chemotherapy and radiotherapy. Nat Med (2007) 13(9):1050–9. doi: 10.1038/nm1622
251. Yang S, Fu ZZ, Zhang YQ, Fu BH, Dong LX. Rs7853346 polymorphism in lncRNA-PTENP1 and rs1799864 polymorphism in CCR2 are associated with radiotherapy-induced cognitive impairment in subjects with glioma Via regulating PTENP1/miR-19b/CCR2 signaling pathway. Biochem Genet (2022) 60(4):1159–76. doi: 10.1007/s10528-021-10145-9
252. Tse KP, Tsang NM, Chen KD, Li HP, Liang Y, Hsueh C, et al. MCP-1 promoter polymorphism at 2518 is associated with metastasis of nasopharyngeal carcinoma after treatment. Clin Cancer Res: Off J Am Assoc Cancer Res (2007) 13(21):6320–6. doi: 10.1158/1078-0432.CCR-07-1029
253. Refae S, Gal J, Ebran N, Otto J, Borchiellini D, Peyrade F, et al. Germinal immunogenetics predict treatment outcome for PD-1/PD-L1 checkpoint inhibitors. Investigational New Drugs (2020) 38(1):160–71. doi: 10.1007/s10637-019-00845-w
254. Yang S, Fu ZZ, Zhang YQ, Fu BH, Dong LX. Rs7853346 polymorphism in lncRNA-PTENP1 and rs1799864 polymorphism in CCR2 are associated with radiotherapy-induced cognitive impairment in subjects with glioma Via regulating PTENP1/miR-19b/CCR2 signaling pathway. Biochem Genet (2022) 60(4):1159–76. doi: 10.1007/s10528-021-10145-9
Keywords: monocyte, anti-cancer treatment, surgery, chemotherapy, radiotherapy, immunotherapy, genotype
Citation: Patysheva M, Frolova A, Larionova I, Afanas'ev S, Tarasova A, Cherdyntseva N and Kzhyshkowska J (2022) Monocyte programming by cancer therapy. Front. Immunol. 13:994319. doi: 10.3389/fimmu.2022.994319
Received: 14 July 2022; Accepted: 27 September 2022;
Published: 20 October 2022.
Edited by:
Sukh Mahendra Singh, Banaras Hindu University, IndiaReviewed by:
Armando Rojas, Catholic University of the Maule, ChileCopyright © 2022 Patysheva, Frolova, Larionova, Afanas'ev, Tarasova, Cherdyntseva and Kzhyshkowska. This is an open-access article distributed under the terms of the Creative Commons Attribution License (CC BY). The use, distribution or reproduction in other forums is permitted, provided the original author(s) and the copyright owner(s) are credited and that the original publication in this journal is cited, in accordance with accepted academic practice. No use, distribution or reproduction is permitted which does not comply with these terms.
*Correspondence: Julia Kzhyshkowska, anVsaWEua3poeXNoa293c2thQG1lZG1hLnVuaS1oZWlkZWxiZXJnLmRl
Disclaimer: All claims expressed in this article are solely those of the authors and do not necessarily represent those of their affiliated organizations, or those of the publisher, the editors and the reviewers. Any product that may be evaluated in this article or claim that may be made by its manufacturer is not guaranteed or endorsed by the publisher.
Research integrity at Frontiers
Learn more about the work of our research integrity team to safeguard the quality of each article we publish.