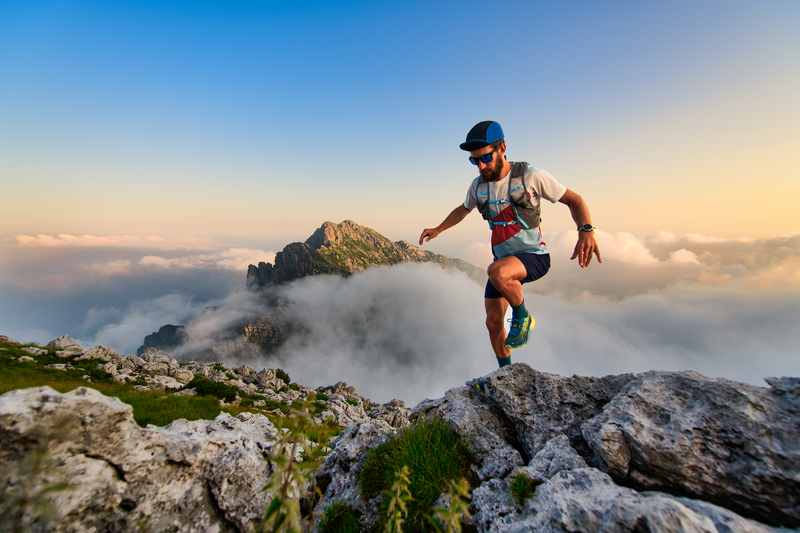
95% of researchers rate our articles as excellent or good
Learn more about the work of our research integrity team to safeguard the quality of each article we publish.
Find out more
REVIEW article
Front. Immunol. , 14 September 2022
Sec. Cancer Immunity and Immunotherapy
Volume 13 - 2022 | https://doi.org/10.3389/fimmu.2022.988849
This article is part of the Research Topic Interplay Between Oncomicrobes, the Microbiota and the Immune System: Impact on Responses to Cancer Immunotherapy View all 7 articles
With the arrival of the era of tumor immunotherapy, Immune Checkpoint Inhibitors have benefited countless tumor patients. However, the emergence of Immune-Related Adverse Events, especially Immune Checkpoint Inhibitor-Mediated Colitis (IMC), has become an important obstacle to immunotherapy. Therefore, it is very important to clarify the mechanism and influencing factors of IMC. The effect of gut microbiota on IMC is gradually becoming a research hotspot. Gut microbiota from different phyla can affect IMC by regulating innate and acquired immunity of tumor patients in various ways. In this review, we make a systematic and comprehensive introduction of the effect of gut microbiota on IMC. Through understanding the specific effects of gut microbiota on IMC, and then exploring the possibility of reducing IMC by regulating gut microbiota.
In recent years, the emergence of Immune Checkpoint Inhibitors (ICIs) has revolutionized the clinical treatment of malignant tumors. In particular, Cytotoxic T Lymphocyte Antigen-4 (CTLA-4) and Programmed Death-1/Programmed Death Ligand-1 (PD-1/PD-L1) have shown significant efficacy in the treatment of various tumors, thus becoming a new treatment method to prolong the survival of cancer patients (1). However, only a few patients obtained long-term clinical benefits, and many patients stopped treatment due to Immune-Related Adverse Events(irAEs), indicating irAEs are an important obstacle to immunotherapy (2). When ICIs launch immune attacks on normal tissues, irAEs will occur. These autoimmune toxicities can affect almost all organs. Different from other adverse drug reactions, irAEs have a broader time range of onset cycle, irAEs can occur as early as the first dose and a few months after treatment and even several months after the cessation of immunotherapy (3). Common irAEs include dermatitis, colitis, and thyroiditis, while others include pneumonia, hepatitis, nephritis, pituitary dysfunction, adrenal inflammation, and myositis. They also have terrible effects on the heart and central nervous system, and may even lead to death (2).CTLA-4 inhibitors and PD-1/PD-L1 inhibitors show different patterns of adverse events, indicating that they are different in maintaining immune tolerance (4). CTLA-4 inhibitors are prone to colitis, while PD-1/PD-L1 inhibitors have a high incidence rate of pneumonia and thyroiditis (5). Among irAEs, gastrointestinal irAEs are the most common cause of drug withdrawal in ICIs (6). Overall, the prevalence and severity of colitis caused by CTLA-4 inhibitors are higher than those caused by PD-1/PD-L1 inhibitors (7). However, with the more and more extensive use of combined immunotherapy, the frequency and severity of IMC have also greatly increased. For example, the incidence rate of IMC caused by CTLA-4 inhibitors and PD-1/PD-L1 inhibitors is 5.7%-39.1% and 0.7%-31.6% respectively, while the incidence rate of IMC caused by combination immunotherapy can reach 40.4% (8, 9). Although most irAEs, including IMC, can be alleviated to varying degrees by corticosteroid and biological agents, such as TNF-α antagonist. The dose and duration of drug use are positively correlated with the severity of IMC. But there are still many patients who are insensitive to corticosteroid and biological agents. Approximately 30%–60% of IMC patients appear recalcitrant to first-line glucocorticoid, showing no response to high-dose steroid within 3 days of onset or no complete response in 7 days (10). In addition, long-term corticosteroid exposure cause infection, hypertension, bone fracture, cataract, nausea, vomiting, and other gastrointestinal conditions, and metabolic issues and other side effects (11).To prevent side effects, the dosage and duration of corticosteroid and biological agents are strictly regulated and limited (12). Long-term and massive application of corticosteroid and biological agents also attenuates the antitumor effect of ICI (13, 14). Therefore, there is an urgent need for effective indicators of IMC and methods that can replace corticosteroid to improve patients’ compliance with immunotherapy.
Human gut microbiota is a complex system that affects the host’s nutrition acquisition, organ development, immune regulation, and other functions. With the development of analytical technology, people have further discovered the role of gut microbiota in maintaining the balance between human health and disease, particularly in promoting the development of the mucosal immune system and maintaining intestinal immune homeostasis (15–17). The composition of human gut microbiota remains relatively stable throughout life. Bacteroidetes and Firmicutes are the most abundant, accounting for about 90%, while Proteobacteria, Actinobacteria, Fusobacteria, and Verrucomicrobia are less abundant (18). The change of gut microbiota has a profound impact on the host, not only affecting the intestinal tract but also affecting many organs of the whole body (19). Generally speaking, the highly diverse gut microbiota establishes a symbiotic relationship with the host immune system to maintain the homeostasis of the host, and the breaking of this homeostasis can lead to chronic inflammation, autoimmune diseases, and even tumors. Our previous studies have revealed that gut microbiota may influence tumor progression, treatment and prognosis (20–22).
In recent years, cancer immunotherapy brought significant improvements for patients in terms of survival and quality of life. However, there are obvious individual differences in response to immunotherapy, and not all patients can obtain lasting benefits due to the occurrence of irAEs. Considering the close relationship between gut microbiota and the immune system, more and more studies have focused on the role of gut microbiota in regulating the efficacy of tumor immunotherapy and reducing side effects. Many studies have shown that the composition of gut microbiota can regulate the host response to ICIs, and higher microbial diversity is related to better treatment outcomes (23). The composition of gut microbiota is different between responders and non-responders to immunotherapy (24). Fecal microbiota transplantation (FMT) of responders showed improved response to ICIs (25). In conclusion, the efficacy of ICIs is related to the composition of gut microbiota, and specific bacteria can effectively improve the therapeutic response of ICIs.
Because of its complex effects on the human body, the gut microbiota has been regarded as a new “organ” of the human body and a new target for drug development. The gut microbiota is a very promising research field in reducing irAEs. Recently, several scientific studies on the impact of gut microbiota on IMC have revealed the relationship between the two, which may open up a new way to prevent and improve IMC. This review summarizes the related studies on the pathogenesis of IMC, especially the effect of gut microbiota. Furthermore, we also described the possible mechanism and clinical application of gut microbiota affecting IMC to explore the possibility of the gut microbiota as a biomarker and treatment of IMC.
At present, the most widely used immune checkpoint inhibitors are PD-1, PD-L1and CTLA-4 inhibitors. PD-1 presents in T cells, B cells, tumor-infiltrating lymphocytes, myeloid cells. PD-L1 exists on the surface of both antigen-presenting cells (APCs) and tumor cells, resulting in weakening of immune surveillance after binding with PD-1. CTLA-4 is typically expressed on T cells, B-cell subsets, and thymocytes, which is associated with the suppression of T-cell activity. T-cell activation results in enhancing immune response and CTLA-4 and PD-1 expression. CTLA-4 is constitutively located on the surface of Foxp3 CD4 regulatory T (Treg) cells, harbors higher affinity than CD28, contributing to competitively bind CD80/86, thus attenuates T cell activation (26). In addition, PD-1 with PD-L1 suppresses the T-cell receptor (TCR) downstream signaling, enhanced the inhibitory effect of Treg cells (27–29). Both CTLA-4 and PD-1/PD-L1 inhibitors can strengthen the activation and proliferation of effector T cell, restrain Treg cell function, induce inflammatory cytokines, boost antitumor immunity.
More and more studies have focused on the interaction between gut microbiota and ICIs, and the positive role of gut microbiota in enhancing response to ICIs has also been proved. Bacteroidales, Faecalibacterium play key roles in the immunostimulatory effects of CTLA-4 blockade (30). Key bacterial species belonging to various phyla, including Actinobacteria (Bifidobacteriaceae spp. and Coriobacteriaceae spp.) and Firmicutes (Ruminococcaceae spp. and Lachnospiraceae spp.), Verrucomicrobia (Akkermansia muciniphila) are associated with favorable response to PD-1 inhibitors by increasing the recruitment of T cells in cancer patients (31, 32). Bifidobacterium enhanced the effect of PD-L1 inhibitor, augmented dendritic cell function leading to enhanced CD8(+) T cell priming and accumulation in the tumor microenvironment (33).
IMC is a common irAE and mortality rate of about 5%which sometimes terminates the treatment process of cancer patients (2). Meta-analysis of 629 patients from 26 studies showed that ICIs was permanently discontinued in 52.4% of patients with IMC (34). Colitis accounts for 37% of deaths caused by irAEs (35). Death cases due to irAEs treated with anti-CTLA-4 are usually from IMC, while death cases of irAEs related to anti- PD-1/PD-L1 are usually from pneumonia. irAEs with the most common and highest mortality after combined immunotherapy are also IMC (2). Because IMC has no specific biomarkers, the preliminary diagnosis can be made only when symptoms and imaging support are presented. IMC can appear at any time during the treatment process, so it is difficult to accurately predict the onset time of IMC (36). The onset time of IMC ranges from 0 to 27 weeks and may vary by ICIs type. Colitis caused by CTLA-4 inhibitors appears to occur later than that caused by PD-1/PD-L1 inhibitors, with a median onset time of about 6 to 7 weeks (8). The onset time of colitis caused by PD-1/PD-L1 inhibitors ranged from 0.6 to 119.9 weeks after treatment. In addition, the onset time of IMC was significantly earlier in patients treated with combined ICIs (37). Studies have observed that the regression time of IMC is more than 18.5 weeks, which is more than 10 times the half-life of CTLA-4 inhibitors (8). This means that the impact of immunotherapy on the human immune system is long-term, so the occurrence of related symptoms should be monitored for a long time after the use of ICIs. The clinical symptoms of IMC include diarrhea, abdominal pain, bloody stool, fever, nausea, vomiting, and loss of appetite, among which diarrhea is the most common symptom. Severe IMC can also lead to intestinal perforation, toxic megacolon, and even life-threatening (38). The common CT findings of IMC are mesenteric vascular congestion, intestinal wall thickening and fluid-filled colon dilatation (39). IMC endoscopy usually shows mucosal ulcer, and sometimes nonulcerative inflammatory morphology such as erythema, exudation, erosion, loss of vascular pattern, edema, or granular mucosa (40). The pathological manifestation of IMC is that lymphocyte infiltration leads to intestinal lamina propria expansion, intraepithelial neutrophilia, and neutrophil crypt abscess (41). Generally, IMC is diagnosed after excluding infection, inflammatory bowel disease(IBD) and tumor metastasis. Endoscopic biopsy is considered the gold standard for IMC diagnosis (8). IMC is usually treated with corticosteroid, but many patients are not sensitive to corticosteroid. At the same time, due to the obvious side effects of corticosteroid on the whole body, they cannot be used in large quantities for a long time. Therefore, it is urgent to develop new treatments. Although increasing experience with IMC therapy has improved the clinical diagnosis and monitoring of IMC, it remains one of the main obstacles in immunotherapy.
irAEs are the result of the destruction of self-tolerance and the pathogenesis of irAEs involves many factors. As an organ-specific irAE, IMC is mainly induced by the recruitment of T cells and the production of inflammatory factors. However, there are many mechanisms different from irAEs in other organs. An in-depth understanding of these pathogenic mechanisms will help us to comprehensively understand IMC. Innate and adaptive immune abnormalities as well as changes in gut microbiota can affect the occurrence and development of IMC (Figure 1).
Figure 1 Mechanism of IMC. The pro-inflammatory pathways (CTL, Th17 cells, and neutrophils) enhance in IMC, while the anti-inflammatory pathways (Treg differentiation and IL-10 secretion) are inhibited. Macrophages recruit T cells through chemokines, and ILC3 plays an unknown role in IMC.
Neutrophils are the most abundant type of white blood cell in the human body. In the acute stage of inflammation, neutrophils are the first echelon to migrate to the inflammatory site. Pathological examination shows IMC has obvious neutrophil infiltration in the intestinal lamina propria. Analysis of the gene expression profile in whole blood samples from melanoma patients treated with ICIs showed that neutrophil activation markers CD177 and CEACAM1 were highly expressed in patients with gastrointestinal adverse reactions at baseline and continued to rise during the course of treatment (42). Group 3 innate immune cells (ILC3) are mainly concentrated in the intestinal tract, which produce immune responses to bacteria and regulate the balance of microbiota. The main homeostasis cytokine produced by ILC3 is interleukin-22(IL-22), which maintains intestinal homeostatic and promotes the proliferation of intestinal stem cells through IL-22 (43). On the contrary, ILC3 can also promote IL-17 production. The recent research has demonstrated that the severity of IMC is positively correlated with the number of ILC3 (44). Therefore, the current research shows that the role of ILC3 in IMC is contradictory and needs more detailed research to clarify. Another kind of innate immune cell is mucosa-associated invariant T(MAIT) cells. The number of MAIT cells increases in the intestinal tissues of patients with IMC, but there is no significant change in patients without IMC and patients with ulcerative colitis (45). T cells in IMC express high levels of IFN-γ、CXCR6 and CXCR3. CXCL16 is a ligand of CXCR6, which is expressed by myeloid cells in the colon and its expression is regulated by IFN-γ And TNF-α (46). Their binding also contributes to tumor cell metastasis, so they are potential targets to improve IMC (47). CXCR3 is a key surface molecule of Th1 cells, CXCL9 and CXCL10 as ligands of CXCR3 are induced by IFN, and its encoding genes are overexpressed in myeloid cells of IMC cases, especially macrophages, which help to recruit effector T cells to inflammatory sites (46). Studies have shown that CXCR3-deficient mice will not develop colitis induced by dextran sodium sulfate(DSS), confirming the influence of this pathway in the development of colitis (48).These studies suggest that myeloid cells, especially macrophages, play a significant role in T cell recruitment during the pathogenesis of IMC.
High levels of infiltration of CD4+T cells and CD8+T cells occurred in IMC cases. CD4+T cells are the main T cell subset in CTLA-4-induced colitis patients, while CD8+T cells are dominant in PD-1-induced colitis. TNF-α is highly expressed in intestinal mucosa with CTLA-4-induced colitis (49, 50). Single-cell analysis of IMC patients also confirmed that the number of cytotoxic T lymphocytes (CTL) and circulating T cells increased, and tissue resident memory T cells (Trm) decreased, while the colon Trm cells of ICIs-treated patients without IMC did not change. TCR sequences of T cells overlapped between CD8+ Trm cells and CTL, indicating the differentiation of CD8+ Trm cells into CTL. At the same time, the content of granzyme B and IFN-γ produced by CD8+ Trm cells increased. It can be speculated that high levels of CD8+ Trm cells in the colon are involved in the pathogenesis of IMC, and its cell activation promotes the rapid recruitment of CD8+ T cells and enhances the damage to the colon mucosa (46, 51). This also explains that IMC is more acute than other types of colitis. α4β7 and αEβ7 integrins are expressed on intestinal mucosal intraepithelial lymphocytes (IEL) and play a crucial part in the homing and localization of lymphocytes to the intestinal mucosa (52). Genes Coding αE、α4 and β7 (ITGAE 、ITGA4、ITGB7) are highly expressed in IMC-related T cell populations, especially CD8+ T cells, indicating that this may be a potential target for the treatment of IMC. And clinical studies have demonstrated the use of α4β7 integrin inhibitor (vedolizumab) in the treatment of hormone-resistant IMC patients (53).
Regulatory T cells are T cell subsets that control autoimmunity in vivo, which can maintain immune tolerance and inhibit inflammation. Treg cells overexpressed CTLA-4, so CTLA-4 inhibitors can induce the inactivation of related intratumor and intestinal CTLA-4+treg cells, and induce the activation of effector T cells, resulting in the development of IMC (54). Chaput et al. observed that IMC patients had a low baseline level of sCD25. CD25 is the α chain of IL-2 receptor and CD25 is important for IL-2 in promoting the development of Treg cells (55). In the single-cell analysis of IMC patients, the number of Treg cells did not decrease, but the Treg cell’s gene expression profile changed. It is speculated that IMC may not be caused by the consumption of CTLA-4+Tregs, but Treg cells may show a Th1-like pro-inflammatory effect under the action of IFN- γ (46).
A clinical trial analyzing gene expression in peripheral blood showed that CCL3, CCR3, IL-5, and IL-8, which are mainly involved in inflammatory immune response, are closely associated with immunotherapy-related diarrhea, especially grade 2-4 diarrhea (56). The expression of IL-8 is regulated by IL-17. IL-17 is a pro-inflammatory cytokine, mainly produced by Th17 cells, which participates in the pathogenesis of various autoimmune diseases and promotes tumor progression. The baseline level of IL-17 is related to severe immunotherapy-related diarrhea. More importantly, serum IL-17 level has been elevated throughout the course of IMC (57, 58). The pathogenicity of Th17 cells is more prominent when the Treg cell’s function is limited. IL-10 is an important cytokine for inhibiting colitis which is secreted by Treg cells. When the IL-10 receptor (IL-10R) gene in Treg cells in the mouse model is knocked out, it will develop into colitis under the action of Th17 cells, highlighting the indispensable role of IL-10 in maintaining the stability of the intestinal environment (59). In addition, IL-17 stimulates intestinal epithelial cells (IEC) to express chemokines CXCL1, CXCL2, CXCL5, CXCL8 and granulocyte-macrophage colony-stimulating factor(GM-CSF), which attract neutrophils and prevent their apoptosis cause neutrophil infiltration (60–62). Intraepithelial neutrophilia granulocytosis is also a typical pathological manifestation of IMC. Furthermore, it acts systemically by increasing serum amounts of granulocyte-colony-stimulating factor (G-CSF) and GM-CSF to increase the numbers and speed the development of neutrophils and macrophages. IL-17 also can facilitate the recruitment of large numbers of immune cells through the induction of matrix metalloproteinases (63). In conclusion, the Th17/IL-17 axis affects IMC in many ways.
In addition to the pathogenesis discussed above, the gut microbiota is also actively involved in the pathogenesis of IMC. Understanding the impact of gut microbiota on human immunity is crucial for the treatment and reestablishment of protective gut microbiota to prevent and improve IMC. The human gut microbiota is known to alter immune homeostasis and tolerance. The diversity and composition of microorganisms may affect IMC by changing the immune components in tumors and circulation (Table 1).
It was found that CTLA-4 inhibitor treatment in mice could lead to intestinal injuries, such as increased IEC death, imbalance of IEC and IEL homeostasis, and even colitis, and this effect was mediated by gut microbiota. Mice treated with B. fragilis and Burkholderia cepacia reduced symptoms of colitis caused by CTLA-4 inhibitors and enhanced the therapeutic effect (30). Dubin et al. studied melanoma patients treated with anti-CTLA-4 and found the fecal samples of patients without IMC had higher bacterial richness and more Bacteroidetes phylum. Furthermore, a model based on bacterial function in feces was proposed to predict the risk of IMC in patients with melanoma (64). Chaput’s study also showed that in melanoma patients receiving anti-CTLA-4 treatment, different gut microbiota composition was related to the occurrence and development of IMC and clinical efficacy of ICIs. The bacterial diversity in the intestines of patients with IMC is reduced, and Firmicutes content is rich in patients prone to IMC, while the proportion of Bacteroidetes in patients without IMC is higher (55). Other studies have shown that the use of Bifidobacterium can reduce IMC without damaging the anti-tumor function of CTLA-4 inhibitors (70).
The baseline fecal microbiome composition of lung cancer patients undergoing irAEs including IMC was significantly different from other lung cancer patients. Bifidobacterium and Desulfovibrio are significantly associated with a lower incidence of irAEs, and this observation is consistent across all severity levels of irAEs. Dynamic longitudinal monitoring of the gut microbiome in lung cancer patients showed the microbiome α-diversity decreased could be observed at the onset of irAEs. With the cessation of ICIs and hormone treatment, the symptoms of irAEs gradually reduced or even disappeared, and the microbiome α-diversity also showed decline slows down or completely reversed to the baseline (68). Analysis of baseline gut microbiota in advanced non-small cell lung cancer patients who received PD-1/PD-L1 inhibitors showed that intestinal microbiota was related to irAEs including IMC, among which Lactobacillaceae, Raoultella, and Akkermansia were related to low-grade irAEs, while Agathobacter was enriched in the feces of patients who experienced more severe irAEs (66). A stool analysis of patients with hepatobiliary carcinoma who developed IMC after receiving a PD-1/PD-L1 inhibitor showed that the Negativities class, Villonellaceae family, and Dialist genus of Firmicutes phylum were enriched in patients with mild IMC, while the dominant species in the feces of patients with severe IMC was Prevotellamassilia timonensis from the Bacteroidetes phylum (67). Interestingly, this was contrary to the previous research results. Although many studies have confirmed that the existence of Bacteroidetes can reduce the occurrence of irAEs, it seems that different species of Bacteroidetes play different roles in irAEs. Bacteroides dorei is significantly enriched in melanoma patients prone to irAEs, and Bacteroides vulgatus is associated with a lower incidence of irAEs (65). In addition, studies have found that Enterbacteriaceae is associated with clinical remission of IMC, and medium-grade IMC often indicates a better anti-tumor effect (69).
Combined immune checkpoint blocking (CICB) therapy targeting CTLA-4 and PD-1 is associated with high clinical benefits across tumor types, but unfortunately, the incidence of irAEs is also higher with the widespread use of CICB. Baseline Bacteroides intestinalis abundance was higher in gut microbiota samples from patients with IMC above grade 3 than below grade 3. The mouse model also showed similar results. The administration of CICB increased the content of Bacteroides intestinalis, and the mice with Bacteroides intestinalis suffered serious ileum damage (71).
Although immune system abnormality is the main cause of IMC, the pathogenesis of IMC is complex and diverse, and also involves nutrients, genetic susceptibility and other factors.
A retrospective study found that C-reactive protein (CRP) was elevated in patients with irAEs including IMC, but the elevated level of CRP had nothing to do with the severity of inflammation. Therefore, it was inferred that the elevated CRP could predict the occurrence of irAEs in the absence of infectious diseases (72). Elevated CRP baseline level is positively correlated with the infiltration of CD8+ T cells. It is known that activated effector T cells can activate a systemic inflammatory response, which may be the link between CRP and irAEs (73). The use of vitamin D before ICIs treatment significantly reduced the incidence of IMC, and the immune cells of vitamin D deficient mice showed IL-17 and IFN-γ increased secretion and destruction of epithelial barriers leading to IMC (74, 75). A higher baseline level of soluble CTLA-4 (sCTLA-4) is closely associated with irAEs in patients with melanoma, especially in the gastrointestinal tract. sCTLA-4 is mainly produced by Treg cells and participates in immune escape, but it is inconsistent with the above results. In conclusion, the feasibility of SCTLA-4 as a biomarker for predicting IMC needs further exploration (76). Genetic susceptibility is a key factor in autoimmune susceptibility. Human Leukocyte Antigen (HLA) haplotypes and polymorphisms of immunomodulatory genes such as CTLA-4 and CTLA-1 are one of the leading causes of various autoimmune diseases and may participate in the occurrence and development of irAEs. Through the analysis of HLA allele typing, it is determined that there is a significant correlation between HLA-DQB1*03:01 and IMC[50]. There may be a common antigen between tumor and colon tissue, which enables colon tissue to be recognized by antigen-specific T cells, resulting in colon injury (64, 77).
Innate and acquired immune complement each other in the human body and jointly maintain immune homeostasis. The application of ICIs will interfere with the balance between inflammation and tolerance, leading to abnormal immune system. Resulting in enhanced pro-inflammatory pathway and inhibited anti-inflammatory pathway in the intestine, which is manifested in active T cell recruitment mediated by myeloid cells, increased differentiation of pro-inflammatory cells and release of pro-inflammatory factors, and decreased differentiation of anti-inflammatory cells. These immune system disorders can induce the development of IMC. In addition, nutrients and genetic susceptibility are also the key factors of IMC.
With the subdivision of studies on different gut microbiota, it has been found that even bacteria from the same phylum have different compositions and physiological activities. Therefore, each kind of bacteria has a unique physiological function. By acting on different immune cells, they regulate the function and gene expression of immune cells, thus playing a complex and diverse role in immunity. Gut microbiota also interact with each other, and the interaction between microbiota is an important factor in determining the final metabolites of gut microbiota (78). Although it has been proved that gut microbiota can act on IMC, few studies explored the potential mechanism of gut microbiota on IMC, and there is no mainstream theory about the improvement of IMC by gut microbiota. We generally summarize the following:(1) change the diversity and composition of gut microbiota; (2) affect mucosal barrier function; (3) regulate mucosal and systemic immunity. Here are some common intestinal bacteria and their metabolites that may affect the molecular mechanism of IMC (Figure 2).
Figure 2 The mechanism of common gut bacteria in colon affecting IMC. (I) Bifidobacterium enhances Treg cells function and mitochondrial activity; (II) Bacteroides and Lactobacillus inhibit pro-inflammatory cells (Th1, Th17 cells, and neutrophils) and enhance anti-inflammatory pathways (Treg, Th2 cells differentiation, and IL-10 secretion); (III) Streptococcus promotes monocytes to produce Th1 and Th17 cells polarization factors; (IV) Prevotella stimulated Th17 cells by DC; (v) Akkermansia reduce the number of CTLs and Macs.
Actinobacteria play an important role in the breakdown of organic materials such as cellulose and chitin, thus in organic material turnover and carbon cycling. Actinobacteria are considered a treasure trove of secondary metabolites, including the Streptomyces, Actinomycetes, and so on. One of the most widely known is Bifidobacterium.
Bifidobacterium known as a probiotic is used to improve many types of colitis, including IBD. What is more, it can also enhance the efficacy of ICIs while attenuating the toxic effects. Sun et al. first explored the mechanism of Bifidobacterium improving IMC and found that Bifidobacterium treatment on IMC mice increased the relative abundance of Lactobacillus rhamnosum and promoted self-stimulating circulation of IL-10/IL-10Rα in the intestine, inhibited effector T cells by enhancing Tregs function and reduces damage to normal tissues (78). Bifidobacterium not only affects the function of immune cells but also may affect the energy utilization of immune cells. Bifidobacterium enhances mitochondrial function and activity while increasing mitochondrial volume and mitochondrial stress in Treg cells (78). Bifidobacterium adolescentis can also improve DSS-induced colitis by stimulating protective Treg, Th2 cells response, and reshaping gut microbiota (79).
At the same time treated with Bifidobacterium, compared with wild-type mice, IL-10 knockout mice and IL-22 antibody-treated mice lost more weight, had more leukocyte infiltration in the colon, had more severe damage to the intestinal structure, and also increased the levels of inflammatory cytokines in serum. This indicates that IL-22 and IL-10 play a crucial part in Bifidobacterium improvement of IMC.
Bacteroidetes are an important part of intestinal bacteria, including Bacteroides, Prevotella, etc. They participate in many important metabolic activities in the colon. Bacteroidales promote the presence of IEL in the colon and then produce IL-6 in myeloid differentiation primary response 88(MyD88) dependent way to maintain intestinal epithelial barrier homeostasis (80).
Bacteroides thetaiotaomicron (B. thetaiotaomicron) can alleviate colitis by regulating tryptophan metabolism and T cell differentiation in inflammatory intestinal tissues. B. thetaiotaomicron stimulates antigen-specific CD4 + T cells of B. thetaiotaomicron to differentiate into Treg cells and effector T cells. Consumption of B. thetaiotaomicron-specific Treg cells can lead to colitis (81). B. thetaiotaomicron promotes the preferential differentiation of anti-inflammatory Treg and Th2 cells while inhibiting the differentiation of pro-inflammatory Th1 and Th17 cells, thereby reducing colitis. At the molecular level, B. thetaiotaomicron is related to CpG methylation within the Foxp3 promoter, which can enhance Tregs function. B. thetaiotaomicron also increases the levels of indoleacetic acid and indole propionic acid to increase Aromatic hydrocarbon Receptor (AhR) activation, which is connected with changes in the expression profile of transcription factors in T cells (82). Bacteroides fragilis(B. fragilis) has the immunomodulatory effect of limiting inflammation by stimulating Treg cells differentiation and increasing IL-10 level (83). Colonization of B. fragilis can reduce the infiltration of inflammatory cells (macrophages and neutrophils) in the colon mucosa (84). Polysaccharide A(PSA) is a macromolecular carbohydrate, as well as an immunogenic capsule component of non-toxigenic B. fragilis. It is an immunomodulatory factor that can promote mucosal immune development and inhibit colitis. B. fragilis colonization alone was sufficient to redress the imbalance of Th1/Th2 cells in the spleen of germ-free mice. Besides, PSA alleviates colitis by inhibiting pro-inflammatory cytokines relevant to the Th17 cells lineage (85). PSA can also promote the transformation of CD4+T cells into Foxp3+Treg cells and the production of IL-10 (83). Toll-like receptor 2(TLR2) is a transmembrane receptor that recognizes the pathogen-associated molecular patterns such as PSA, initiating a signaling cascade to promote immune response. PSA induces and preferentially binds to TLR2 on plasmacytoid DC (pDC), and then pDC specifically stimulates CD4+ T cells to secrete IL-10. Therefore, PSA can affect the inflammatory state by regulating innate and adaptive immunity (86). In addition to B. fragilis, Parabacteroides distasonis can also stimulate DC and then induce CD4 + T cells to differentiate into Treg cells, playing a protective role in the acute colitic mouse model (87).
Interestingly, not all Bacteroides have a positive effect on IMC, and some Bacteroides seem to be involved in the pathogenesis of IMC. For example, Enterotoxigenic B. fragilis is a strain of B. fragilis that secretes a 20-kDa heat-labile zinc-dependent metalloprotease toxin termed the B. fragilis toxin. It is closely related to IBD and colorectal cancer, and can rapidly induce IL-17-dependent colitis and tumorigenesis (88). Oral administration of Bacteroides ovatus and Bacteroides xylanisolvens induces the expression of pro-inflammatory factors CXCL9 and IFN-γ in the mouse model of lung cancer (89).
Prevotella induced the production and accumulation of Th17 cells in the colon and increased Th17 cells related cytokines in serum. The interaction between Prevotella and TLR2 on DC mediates the production of Th17-polarizing cytokines (IL-6 and IL-1β), indicating the potential pathogenic role of Prevotella in the gut (90).
Firmicutes are the largest bacterial group in the intestinal tract, including Bacillus, Enterococcus, Lactobacillus, Streptococcus, Clostridium, etc. They are the main bacterial group that produces short-chain fatty acids. Multiple studies have shown that the enrichment of Firmicutes is connected with IMC. Therefore, except for Lactobacillus, many bacteria from Firmicutes will promote the occurrence and development of IMC. The depletion of Treg cells in mice is accompanied by an increase in the relative abundance of Firmicutes and the occurrence of intestinal inflammation in mice, indicating that the effects of Treg cells and microbes are mutual (91). The preclinical study conducted by Chaput et al. showed that IMC may be related to the increased proportion of ICOS+CD4+T cells and ICOS+Treg cells induced by Firmicutes (55).
Lactobacillus is a kind of probiotic in firmicutes commonly used in fermented dairy products. Oral administration of Lacticaseibacillus paracasei significantly changes the microbial community and increases microbial diversity, helps to maintain intestinal homeostasis, and provides a complete intestinal barrier against IMC (92). Lactobacillus rhamnosus can reduce the severity of inflammation in the IMC mouse model by regulating Treg cells (93). Oral administration of Lactobacillus rhamnosus can activate the innate immunity of the host to enhance the immune response, regulate the ratio of Treg to Th1 population and the expression ratio of IL-10 and TNF-α to inhibit the host’s systemic inflammation (94). T-cells’ response can be indirectly manipulated by regulating intestinal DC. Lactobacillus casei Shirota can regulate DC in inflammatory intestinal tissue, which is manifested in the decrease of TLR2/4 expression, restoration of the ability to imprint homing molecules on T cells, and the stimulation of T cells to produce IL-22 (95). Lactobacillus acidophilus and Clostridium butyricum can reduce Th1-type colitis and Th2-type colitis, their combination can reduce neutrophil infiltration and improve colon ulcers (96). Lactobacillus reuteri mainly inhibits the occurrence and progression of IMC by reducing the number of ILC3 (44). Lactobacillus fermentum shows beneficial effects on colitic mice, which decreases levels of Th1, Th2 and Th17 cells related cytokines, and increases CD4+Foxp3+Treg cells in the colon and mesenteric lymph nodes (MLN). In addition, the administration of Lactobacillus fermentum reshapes and increases the diversity of gut microbiota, and increases the abundance of beneficial bacteria such as Lactobacillus and Akkermansia (97). Lactobacillus acidophilus treatment can also alleviate the severity of DSS-induced colitis and inhibit pro-inflammatory cytokines in the colon, such as IL-1β、 IL-6、IL-17, and TNF-α. In addition, Lactobacillus acidophilus directly induces the production of Treg cells and IL-10 (98).
Streptococcus spp. is known to be enriched in patients with irAEs (99). Streptococcus salivarius and Streptococcus agalactiae can effectively activate monocytes to secrete higher levels of IL-6, IL-12 and TNF, which are Th1 and Th17 cells skewing cytokines (100).
FMT can restore the balance of gut microbiota and alleviate colitis, especially up-regulate the abundance of Lactobacillus and down-regulate the abundance of Clostridium and Turicibacter. FMT can also selectively down-regulate the proportion of CD4+ and CD8+ T cells in the colon to maintain intestinal homeostasis. In addition, Clostridium is associated with inflammation-related genes CCL8 and IDO1 (101).
The mixture of Fusobacterium, Phascolarctobacterium, Eubacterium, and Ruminococcus of Firmicutes showed obvious IFN-γ+ CD8 +T cell induction ability (102). MAIT cells are activated during bacterial infection and exert antibacterial effects by producing IFN-γ, TNF-α, and IL-17 (103). The increase of MAIT cells in IMC patients proves the connection between microbiome and IMC. The antibacterial activity of MAIT cells may lead to the destruction of the intestinal barrier and the impairment of immunoregulation in IMC patients. SCFAs producing bacteria are positively correlated with the number of MAIT cells, such as Acidaminococcaceae of Firmicutes and Alistipes shahii and Alistipes inops of Bacteroidetes (104).
Verrucomicrobia is a newly classified group of bacteria that includes only a handful of recognized species. As a representative of verrucomicrobia found in feces, Akkermansia muciniphila makes up only about 3% of the intestine, but it plays multiple roles. Akkermansia muciniphila can improve colitis by reducing infiltrating macrophages and CD8 + CTLs in the colon and MLN (105).
The microbiota carries out complex and active metabolic activities in the human intestine, which not only provides energy and nutrition for growth but also produces a large number of metabolites such as short-chain fatty acids, secondary bile acids and indole derivatives into the human body. Short-chain fatty acids (SCFAs) are organic acids with 2-5 carbon atoms, which are mainly produced by gut microflora through fermentation and metabolism of oligosaccharides, polysaccharides, titanium, proteins, and glycoproteins (106). Bile acids (BAs) are cholesterol-derived molecules that participate in nutritional and energy-related physiological processes. Most of the BAs in the body are transported back to the liver through the enterohepatic circulation, but a small part of them are reabsorbed in the ileum and further transformed by bacteria in the colon, resulting in secondary BAs (107). About 4-6% of tryptophan moves along the gastrointestinal tract and is metabolized to indole and its derivatives by gut microflora (108). These metabolites affect intestinal homeostasis and inflammation by affecting immune cells and inflammatory factors (Figure 3).
Figure 3 Intestinal bacterial metabolites act on IMC. (I) Butyrate acts on dendritic cells, neutrophils, and macrophages through GPR to induce Treg cells differentiation, reduce the release of proinflammatory cytokines, and induce Treg cells differentiation by inhibiting HDAC; (II)Propionate reduces the release of pro-inflammatory chemokines and promote the secretion of IL-10 by Treg cells; (III) Secondary bile acids inhibit Th17 cells and induce Treg cells production through dendritic cells; (IV) Indole inhibit Th17 cells and macrophages, induce Treg cells production, and improve intestinal cell junction and barrier function. C3: Propionate, C4: Butyrate.
SCFAs mainly include acetic acid, propionic acid, isobutyric acid, butyrate, isovaleric acid, and valeric acid. Acetic acid is the main SCFA in the colon, and most Enterococcus can produce it; Propionic acid can be produced by Bacteroides and Clostridium; Butyrate mainly comes from Clostridium and Eubacterium (106). SCFAs have an immunosuppressive effect on both innate and adaptive immunity. G-coupled protein receptor 41 (GPR41), GPR43, and GPR109A are specific SCFAs receptors in humans. SCFAs reduce the production of TNF-α、IL-2, IL-6, and IL-8 by inhibiting histone deacetylase(HDAC) and activating GPR41 and GPR43 on neutrophils and macrophages. SCFAs can also inhibit the recruitment of macrophages and neutrophils, indicating their potential anti-inflammatory effect in vivo (109). Butyrate can regulate the function of intestinal macrophages. After treatment with butyrate, the proinflammatory mediators NO, IL-6 and IL-12 are down-regulated, maintaining the tolerance of macrophages to gut microbiota (110). Butyrate can also induce macrophages and DCs through GPR109A to regulate Treg cell amplification and increase the expression of IL-10 (111). Butyrate enhanced histone H3 acetylation in the promoter, and conserved non-coding sequence regions of the Foxp3 locus, induced the differentiation of Treg cells in the colon, and also improved the colitis caused by CD4 (+) T cells in mice (112). Similarly, some studies also found that butyrate increased the levels of Treg cells, IL-10 and IL-12 in peripheral blood of mice, decreased the levels of IL-17 in plasma and colon mucosa and thus improved colitis in mice (113). SCFAs promote the production of IL-22 by CD4 + T cells and ILC3 through inhibition of HDAC and GPR41 mediation (114). Ffar2 is the gene encoding GPR43. The binding of GPR43 and SCFAs on immune cells can mediate the resolution of inflammatory responses (115). Studies have confirmed that SCFAs relies on the expression of Ffar2 to prevent and improve colitis in mouse (109). In addition to Treg cells, SCFAs can also act on other cells to affect intestinal homeostasis. SCFAs promote the secretion of IL-10 by Th1 cells through GPR43 mediation, thereby weakening the pathogenic ability of gut microbiota antigen-specific Th1 cells to induce intestinal inflammation (116).
IL-17A producing γδ T cells are tissue resident cells involved in tissue defense and intestinal inflammation regulation. Microbiota-derived propionate inhibits IL-17 production by intestinal γδ T cells in HDAC-dependent manner and has been successfully verified in IBD patients (117). In colitic mice fed with propionate, the number of Foxp3+Treg cells and the level of IL-10 in the colon lamina propria of increased, the weight of mice increased, the disease score decreased, and the levels of colon proinflammatory cytokines decreased (109). Butyrate and propionate regulate gene expression and lymphocyte recruitment in DC since SCFAs strongly reduce the release of several pro-inflammatory chemokines (118).
Endogenous bile acids in the intestinal cavity can promote the renewal and regeneration of intestinal stem cells in response to injury (119). Firmicutes, Actinomycetes, and Bacteroides can convert secondary bile acids into 3-oxolitholic acid(3-oxoLCA) and isolithocholic acid (isoLCA), and then inhibit the differentiation of Th17 cells. The contents of 3-oxoLCA and isoLCA in IBD patients are also greatly reduced, indicating that this may be one of the mechanisms leading to intestinal inflammation (120). Secondary bile acid 3β-Hydrohydroxycholic (isoDCA) can increase the induction of Foxp3+Treg cells by reducing the immunostimulatory properties of DC. The microbiota communities containing engineered Bacteroides are designed by using synthetic biological methods, which can produce isoDCA and promote the generation of Treg cells in vivo (121).
Indole and its derivatives bind to AhR, enhance barrier function and tight connection, maintain intestinal homeostasis, regulate intestinal immune tolerance and weaken inflammatory response, maintain the integrity of apical junction complex and related actin regulatory proteins to reduce intestinal permeability (122–124). Indole-3-acetic acid (I3A) and indole-3-methanol (I3C) produced by Bacteroides ovatus can promote immune cells to produce IL-22 and prevent microbial imbalance caused by colitis, thus have a beneficial impact on colitis (125). I3C can also increase butyrate production, inhibit Th17 cells and induce Tregs production to alleviate colitis (126). Indole reduces pro-inflammatory signals (IL-8 and NF-κB) and promotes the expression of anti-inflammatory ingredients including IL-10 (127). Both tryptamine and I3A reduce the production of proinflammatory cytokines in colon macrophages (128).
Intestinal adaptive immunity plays a crucial part in maintaining immune tolerance and the integrity of the intestinal barrier, and the innate immune system can regulate the adaptive immune response. Gut bacteria can not only directly affect the adaptive immune system, but also act on the innate immune system and thus affect adaptive immunity. Due to the variety of gut microbiota, each bacterium has different effects on IMC. Gut microbiota metabolites are indispensable physiological substances in the human body. With the deepening of its research, it is found that these metabolites also play a key role in inhibiting intestinal inflammation and maintaining intestinal homeostasis.
Recently, a number of large-scale clinical trials for the application of gut microbiota and related products in IMC have been established to research the potential biomarkers in the microbiome and their safety and effectiveness in IMC treatment (Table 2). These different microbial components can be used to develop clinical biomarkers to divide patients into susceptible and non-susceptible groups to IMC before ICIs treatment, to balance the potential risks and benefits of immunotherapy for patients. It can also be used to alleviate and treat IMC, especially in hormone-insensitive cases.
Antibiotics can change the composition and content of gut microbiota, eradicate a variety of pathogenic bacteria including Helicobacter pylori, and then prevent the occurrence and development of tumors. However, some studies have confirmed that antibiotics can reduce the efficacy of tumor immunotherapy, and the cumulative incidence rate of IMC is significantly higher in antibiotic-exposed patients. Therefore, antibiotics are considered a double-edged sword in tumor treatment (129). Antibiotic treatment for anaerobes will lead to the occurrence and progress of IMC, and the application of antibiotics after ICIs treatment has a higher incidence rate of IMC than that before ICIs treatment (130). Component analysis of the intestinal microbiome of melanoma mice showed that vancomycin aggravated the symptoms of IMC by killing Lactobacillus. Oral administration of Lactobacillus reuteri can inhibit the occurrence and progression of colitis, thereby improving the weight loss and inflammatory state caused by ICIs treatment (44). The use of antibiotics still needs further discussion, but antibiotics should certainly be used cautiously during immunotherapy.
FMT uses the feces of healthy donors from another individual or the same individual to balance or restore the intestinal microbial composition through oral gavage or direct delivery to the gastrointestinal tract. It is a safe and low incidence of adverse events of procedure, which holds great promise in the treatment of recurrent Clostridium difficile infection and ulcerative colitis (131). The potential of IMC insensitive to corticosteroids by changing the composition of gut microbiota has been reported. An IMC patient whose symptoms were not relieved after corticosteroids and infliximab treatment completely disappeared and never relapsed after FMT (132). Wang et al. reported two patients with glucocorticoid-resistant IMC whose symptoms were completely relieved after FMT treatment (133). In the future, if the efficacy of FMT is proved to be more beneficial than the current first-line treatment, FMT may be used as the first-line treatment of IMC. Nevertheless, because FMT is an invasive procedure and has not been used as a routine method for clinical treatment, investigational new drug applications should be submitted before FMT. So FMT used as the treatment of IMC has a long way to go. Researches are still needed to determine microbiota kinds, the frequency of FMT as well as the risks associated with FMT and other protocols and specifications (134). Currently, many clinical trials on the application of FMT are underway to investigate its safety and efficacy in the treatment of IMC(NCT04163289, NCT03819296, NCT04038619, see Table 2 for details).
Prebiotics are various fibers that can be digested by microorganisms. Bacteria can metabolize prebiotics to produce secondary metabolites such as SCFAs. In prebiotic supplemented mice, the genus relative abundance of Ruminococcus and Bifidobacteria increased, which was related to significantly reducing tumorigenesis, increasing the concentration of SCFAs in cecum and feces, and reducing the expression of inflammatory markers (135). Feeding 2-Fucosyllactose to mice with colitis can decrease IL-1β and IL-6 expression, increase TGF-β and occludin expression and propionate concentration, accompanied by the expansion of Ruminococcus, and the most important thing is to effectively alleviate inflammation (136).Clinical trials on the application of prebiotics have been established to investigate its safety and feasibility in the treatment of IMC(NCT04552418, see Table 2 for details).
Engineered microbiomes are new microorganisms that be engineered using modern bioengineering techniques such as genome editing. They have a variety of advantages, including site-specific drug delivery, sustainable release and convenient control, and a large number of studies have fully demonstrated their broad prospects in the treatment of metabolic diseases and cancer. Researchers constructed an engineered butyrate-producing strain, BsS-RS06551, using B. subtilis SCK6 as cellular chassis, could produce high levels of butyrate and increase the abundance of beneficial bacteria, such as Bifidobacterium, Lactobacillus, and Akkermansia (137). Researchers engineered Escherichia coli Nissle 1917 (ECN) to express IL-10, produce curly fiber matrix, ketone body (R)-3-hydroxybutyrate (3HB), and Schistosoma immunomodulatory protein Sj16 and other substances. These substances can protect the intestinal mucosa by promoting the growth of probiotics, promoting intestinal epithelial integrity and repair, and downregulating inflammatory response-related cells or cytokines, so as to improve DSS-induced colitis (138–141).
IMC is the most common irAEs, and its incidence rate varies with different types of ICIs. How to prevent and treat IMC is a difficult problem in clinical practice. In this review, we first introduce the onset cycle and clinical characteristics of IMC. Next, we discuss the pathogenesis of IMC, especially the abnormalities of the innate and adaptive immune systems. Then we summarize the latest progress in animal research and human clinical trials and demonstrate that the diversity and composition change of gut microbiota is an important factor of IMC. In addition, we focus on how some common gut bacteria affect IMC. Gut microbiota not only affects local immunity but also affects the systemic immune system. Each kind of bacteria has unique physiological activities, which can trigger anti-inflammatory or pro-inflammatory pathways by affecting the function and metabolism of different immune cells. Gut microbiota metabolites are also an important part of balancing intestinal homeostasis and inflammation. Finally, we summarize the clinical trials on the intervention of intestinal bacteria and related products in IMC patients, to explore the possibility of using gut microbiota as a biomarker of tumor immunotherapy toxicity. As well as exploring the effectiveness and safety of using FMT, prebiotics, probiotics, metabolites, and engineered microbiomes to avoid immunotherapy intestinal toxicity.
irAEs are caused by the excessive immune response attacking normal tissues, which usually means excessive activation of the anti-tumor immune response. Many studies have confirmed that anti-tumour response is higher in patients with IMC and other irAEs (142). Therefore, the methods used for the treatment of IMC may reduce the response to ICIs at the same time, which is not desirable. Many bacteria in the gut microbiota, such as Bifidobacterium, Lactobacillus, and Bacteroides fragilis, play an active role in enhancing the antitumor efficacy of ICIs and improving IMC. If the targeted microbiota modulation is performed before or during the treatment of ICIs, the antitumor effect of ICIs can be maximized and IMC can be minimized. However, there is still a lack of representative clinical trials to promote the transformation of research results into clinical practice. In the future, more detailed research is needed to determine the timing, duration, target bacteria, and specific methods of microbiota regulation. With the deepening study of gut microbiota, it may be possible to directly or indirectly regulate gut microbiota and its metabolites in the future, to achieve targeted regulation of immunotherapy, enhance therapeutic efficacy and reduce adverse reactions including IMC. This will enable more patients to benefit from immunotherapy in the long term and prolong the survival of tumor patients.
XH was mainly responsible for writing conception, literature searching, and drafting of the manuscript. DZ provided professional revision for the article. DL participated in the collection of literature and revision of the article. JC participated in the conception and revision of the article. All authors contributed to the article and approved the submitted version.
This work was supported by the Chinese Society of Clinical Oncology (CSCO) Research Foundation (Nos. Y-JS2019-034)and “1+X ”program for Clinical Competency enhancement–Clinical Research Incubation Project, The Second Hospital of Dalian Medical University(2022LCYJYB01)
The authors are grateful to the directors (Prof. Jun Chen) and team members of the Department of Oncology, The Second Hospital of Dalian Medical University for the guidance and wonderful mentoring that led to the writing of this paper.
The authors declare that the research was conducted in the absence of any commercial or financial relationships that could be construed as a potential conflict of interest.
All claims expressed in this article are solely those of the authors and do not necessarily represent those of their affiliated organizations, or those of the publisher, the editors and the reviewers. Any product that may be evaluated in this article, or claim that may be made by its manufacturer, is not guaranteed or endorsed by the publisher.
ICIs, Immune Checkpoint Inhibitors; irAEs, Immune-Related Adverse Events; IMC, Immune Checkpoint Inhibitor-Mediated Colitis; CTLA-4, Cytotoxic T Lymphocyte Antigen-4; PD-1/PD-L1, Programmed Death-1/Programmed Death Ligand-1; FMT, Fecal microbiota transplantation; APCs, Antigen-presenting cells; Treg, Regulatory T; TCR, T-cell receptor; IBD, Inflammatory Bowel Disease; ILC3, Group 3 innate immune cells; IL, interleukin; MAIT, mucosa-associated invariant T; DSS, Dextran Sodium Sulfate; CTL, Cytotoxic T lymphocytes; Trm, Tissue resident memory T cells; CXCL, CXC motif chemokine ligand; IEC, Intestinal epithelial cells; Intraepithelial lymphocytes; GM-CSF, Granulocyte-macrophage colony-stimulating factor; CRP, C-reactive protein; sCTLA-4, Soluble CTLA-4; HLA, Human Leukocyte Antigen; CICB, Combined immune checkpoint blocking; MyD88, Myeloid differentiation primary response 88; PSA, Polysaccharide A; TLR2, Toll-like receptor 2; pDC, Plasmacytoid DC; MLN, Mesenteric lymph nodes; IDO, indoleamine2,3-dioxygenase; CCL, CC motif chemokine ligand; SCFAs, Short-chain fatty acids; Bas, Bile acids; GPR, G-coupled protein receptor; HDAC, Histone deacetylase; Th, T helper cell; Mac, macrophage; NE, neutrophil; DC, dendritic cell; MNC, monocyte; TNF, tumor necrosis factor; IFN, interferon.
1. Hargadon KM, Johnson CE, Williams CJ. Immune checkpoint blockade therapy for cancer: An overview of fda-approved immune checkpoint inhibitors. Int Immunopharmacol (2018) 62:29–39. doi: 10.1016/j.intimp.2018.06.001
2. Wang DY, Salem JE, Cohen JV, Chandra S, Menzer C, Ye F, et al. Fatal toxic effects associated with immune checkpoint inhibitors: A systematic review and meta-analysis. JAMA Oncol (2018) 4(12):1721–28. doi: 10.1001/jamaoncol
3. Michot JM, Bigenwald C, Champiat S, Collins M, Carbonnel F, Postel-Vinay S, et al. Immune-related adverse events with immune checkpoint blockade: A comprehensive review. Eur J Cancer (2016) 54:139–48. doi: 10.1016/j.ejca.2015.11.016
4. Inno A, Metro G, Bironzo P, Grimaldi AM, Grego E, Di Nunno V, et al. Pathogenesis, clinical manifestations and management of immune checkpoint inhibitors toxicity. Tumori (2017) 103(5):405–21. doi: 10.5301/tj.5000625
5. Khoja L, Day D, Wei-Wu Chen T, Siu LL, Hansen AR. Tumour- and class-specific patterns of immune-related adverse events of immune checkpoint inhibitors: A systematic review. Ann Oncol (2017) 28(10):2377–85. doi: 10.1093/annonc/mdx286
6. Abu-Sbeih H, Fs A, Luo W, Qiao W, Raju GS, Wang Y, et al. Importance of endoscopic and histological evaluation in the management of immune checkpoint inhibitor-induced colitis. J Immunother Cancer (2018) 6(1):018–0411. doi: 10.1186/s40425-1
7. Soularue E, Lepage P, Colombel JF, Coutzac C, Faleck D, Marthey L, et al. Enterocolitis due to immune checkpoint inhibitors: A systematic review. Gut (2018) 67(11):2056–67. doi: 10.1136/gutjnl-2018-316948
8. Tang L, Wang J, Lin N, Zhou Y, He W, Liu J, et al. Immune checkpoint inhibitor-associated colitis: From mechanism to management. Front Immunol (2021) 12:800879. doi: 10.3389/fimmu.2021.800879
9. Tandon P, Bourassa-Blanchette S, Bishay K, Parlow S, Laurie SA, Mccurdy JD. The risk of diarrhea and colitis in patients with advanced melanoma undergoing immune checkpoint inhibitor therapy: A systematic review and meta-analysis. J Immunother (2018) 41(3):101–08. doi: 10.1097/CJI.0000000000000213
10. Geukes Foppen MH, Rozeman EA, Van Wilpe S, Postma C, Snaebjornsson P, van Thienen JV, et al. Immune checkpoint inhibition-related colitis: Symptoms, endoscopic features, histology and response to management. ESMO Open (2018) 3(1):2017–000278. doi: 10.1136/esmoopen-2017-000278
11. Rice JB, White AG, Scarpati LM, Wan G, Nelson WW. Long-term systemic corticosteroid exposure: A systematic literature review. Clin Ther (2017) 39(11):2216–29. doi: 10.1016/j.clinthera.2017.09.011
12. Sørup S, Darvalics B, Russo L, Oksen D, Lamy FX, Verpillat P, et al. High-dose corticosteroid use and risk of hospitalization for infection in patients treated with immune checkpoint inhibitors–a nationwide register-based cohort study. Cancer Med (2021) 10(14):4957–63. doi: 10.1002/cam4.4040
13. Aldea M, Orillard E, Mansi L, Marabelle A, Scotte F, Lambotte O, et al. How to manage patients with corticosteroids in oncology in the era of immunotherapy? Eur J Cancer (2020) 141:239–51. doi: 10.1016/j.ejca.2020.09.032
14. Verheijden RJ, May AM, Blank CU, Aarts MJB, Van Den Berkmortel FWPJ, van den Eertwegh AJM, et al. Association of anti-tnf with decreased survival in steroid refractory ipilimumab and anti-Pd1-Treated patients in the Dutch melanoma treatment registry. Clin Cancer Res (2020) 26(9):2268–74. doi: 10.1158/1078-0432.CCR-19-3322
15. Amoroso C, Perillo F, Strati F, Fantini MC, Caprioli F, Facciotti F, et al. The role of gut microbiota biomodulators on mucosal immunity and intestinal inflammation. Cells (2020) 9(5):1234. doi: 10.3760/cma.j.cn112137-20200520-01598
16. Agus A, Clément K, Sokol H. Gut microbiota-derived metabolites as central regulators in metabolic disorders. Gut (2021) 70(6):1174–82. doi: 10.1136/gutjnl-2020-323071
17. Shi N, Li N, Duan X, Niu H. Interaction between the gut microbiome and mucosal immune system. Mil Med Res (2017) 4(14):017–0122. doi: 10.1186/s40779-9
18. Qin J, Li R, Raes J, Arumugam M, Burgdorf KS, Manichanh C, et al. A human gut microbial gene catalogue established by metagenomic sequencing. Nature (2010) 464(7285):59–65. doi: 10.1038/nature08821
19. Miele L, Giorgio V, Alberelli MA, De Candia E, Gasbarrini A, Grieco A. Impact of gut microbiota on obesity, diabetes, and cardiovascular disease risk. Curr Cardiol Rep (2015) 17(12):015–0671. doi: 10.1007/s11886-z
20. Liu X, Cheng Y, Zang D, Zhang M, Li X, Liu D, et al. The role of gut microbiota in lung cancer: From carcinogenesis to immunotherapy. Front Oncol (2021) 11:720842(720842). doi: 10.3389/fonc.2021.720842
21. Zhang M, Zhou H, Xu S, Liu D, Cheng Y, Gao B, et al. The gut microbiome can be used to predict the gastrointestinal response and efficacy of lung cancer patients undergoing chemotherapy. Ann Palliat Med (2020) 9(6):4211–27. doi: 10.21037/apm-20-2183
22. Zhang M, Liu D, Zhou H, Liu X, Li X, Cheng YE, et al. Intestinal flora characteristics of advanced non-small cell lung cancer in China and their role in chemotherapy based on metagenomics: A prospective exploratory cohort study. Thorac Cancer (2021) 12(24):3293–303. doi: 10.1111/1759-7714.14199
23. Salgia NJ, Bergerot PG, Maia MC, Dizman N, Hsu J, Gillece JD, et al. Stool microbiome profiling of patients with metastatic renal cell carcinoma receiving anti-Pd-1 immune checkpoint inhibitors. Eur Urol (2020) 78(4):498–502. doi: 10.1016/j.eururo.2020.07.011
24. Matson V, Fessler J, Bao R, Chongsuwat T, Zha Y, Alegre ML, et al. The commensal microbiome is associated with anti-Pd-1 efficacy in metastatic melanoma patients. Science (2018) 359(6371):104–08. doi: 10.1126/science.aao3290
25. Baruch EN, Youngster I, Ben-Betzalel G, Ortenberg R, Lahat A, Katz L, et al. Fecal microbiota transplant promotes response in immunotherapy-refractory melanoma patients. Science (2021) 371(6529):602–09. doi: 10.1126/science.abb5920
26. Wing K, Onishi Y, Prieto-Martin P, Yamaguchi T, Miyara M, Fehervari Z, et al. Ctla-4 control over Foxp3+ regulatory T cell function. Science (2008) 322(5899):271–5. doi: 10.1126/science.1160062
27. Miller PL, Carson TL. Mechanisms and microbial influences on ctla-4 and pd-1-Based immunotherapy in the treatment of cancer: A narrative review. Gut Pathog (2020) 12(43):020–00381. doi: 10.1186/s13099-020-00381-6
28. Qureshi OS, Zheng Y, Nakamura K, Attridge K, Manzotti C, Schmidt EM, et al. Trans-endocytosis of Cd80 and Cd86: A molecular basis for the cell-extrinsic function of ctla-4. Science (2011) 332(6029):600–3. doi: 10.1126/science.1202947
29. Li Y, Li F, Jiang F, Lv X, Zhang R, Lu A, et al. A mini-review for cancer immunotherapy: Molecular understanding of pd-1/Pd-L1 pathway & translational blockade of immune checkpoints. Int J Mol Sci (2016) 17(7):1151. doi: 10.3390/ijms17071151
30. Vétizou M, Pitt JM, Daillère R, Lepage P, Waldschmitt N, Flament C, et al. Anticancer immunotherapy by ctla-4 blockade relies on the gut microbiota. Science (2015) 350(6264):1079–84. doi: 10.1126/science.aad1329
31. Gopalakrishnan V, Spencer CN, Nezi L, Reuben A, Andrews MC, Karpinets TV, et al. Gut microbiome modulates response to anti-Pd-1 immunotherapy in melanoma patients. Science (2018) 359(6371):97–103. doi: 10.1126/science.aan4236
32. Routy B, Le Chatelier E, Derosa L, Duong CPM, Alou MT, Daillère R, et al. Gut microbiome influences efficacy of pd-1-Based immunotherapy against epithelial tumors. Science (2018) 359(6371):91–7. doi: 10.1126/science.aan3706
33. Sivan A, Corrales L, Hubert N, Williams JB, Aquino-Michaels K, Earley ZM, et al. Commensal bifidobacterium promotes antitumor immunity and facilitates anti-Pd-L1 efficacy. Science (2015) 350(6264):1084–9. doi: 10.1126/science.aac4255
34. Tran AN, Wang M, Hundt M, Chugh R, Ohm J, Grimshaw A, et al. Immune checkpoint inhibitor-associated diarrhea and colitis: A systematic review and meta-analysis of observational studies. J Immunother (2021) 44(8):325–34. doi: 10.1097/CJI.0000000000000383
35. Ramos-Casals M, Brahmer JR, Callahan MK, Flores-Chávez A, Keegan N, Khamashta MA, et al. Immune-related adverse events of checkpoint inhibitors. Nat Rev Dis Primers (2020) 6(1):020–0160. doi: 10.1038/s41572-6
36. Durrechou Q, Domblides C, Sionneau B, Lefort F, Quivy A, Ravaud A, et al. Management of immune checkpoint inhibitor toxicities. Cancer Manag Res (2020) 12:9139–58. doi: 10.2147/CMAR.S218756
37. Wang DY, Mooradian MJ, Kim D, Shah NJ, Fenton SE, Conry RM, et al. Clinical characterization of colitis arising from anti-Pd-1 based therapy. Oncoimmunology (2018) 8(1):1524695. doi: 10.1080/2162402X.2018
38. Martins F, Sofiya L, Gp S, Lamine F, Maillard M, Fraga M, et al. Adverse effects of immune-checkpoint inhibitors: Epidemiology, management and surveillance. Nat Rev Clin Oncol (2019) 16(9):563–80. doi: 10.1007/s00432-019-03002-1
39. Gupta A, De Felice KM, Loftus EV Jr., Khanna S. Systematic review: Colitis associated with anti-Ctla-4 therapy. Aliment Pharmacol Ther (2015) 42(4):406–17. doi: 10.1111/apt.13281
40. Nishida T, Iijima H, Adachi S. Immune checkpoint inhibitor-induced Diarrhea/Colitis: Endoscopic and pathologic findings. World J Gastrointest Pathophysiol (2019) 10(2):17–28. doi: 10.4291/wjgp.v10.i2.17
41. Oble DA, Mino-Kenudson M, Goldsmith J, Hodi FS, Seliem RM, Dranoff G, et al. Alpha-Ctla-4 mab-associated panenteritis: A histologic and immunohistochemical analysis. Am J Surg Pathol (2008) 32(8):1130–7. doi: 10.1097/PAS.0b013e31817150e3
42. Shahabi V, Berman D, Chasalow SD, Wang L, Tsuchihashi Z, Hu B, et al. Gene expression profiling of whole blood in ipilimumab-treated patients for identification of potential biomarkers of immune-related gastrointestinal adverse events. J Transl Med (2013) 11(75):1479–5876. doi: 10.1186/11-75
43. Saez A, Gomez-Bris R, Herrero-Fernandez B, Mingorance C, Rius C, Gonzalez-Granado JM. Innate lymphoid cells in intestinal homeostasis and inflammatory bowel disease. Int J Mol Sci (2021) 22(14):7618. doi: 10.3390/ijms22147618
44. Wang T, Zheng N, Luo Q, Jiang L, He B, Yuan X, et al. Probiotics lactobacillus reuteri abrogates immune checkpoint blockade-associated colitis by inhibiting group 3 innate lymphoid cells. Front Immunol (2019) 10:1235(1235). doi: 10.3389/fimmu.2019.01235
45. Sasson SC, Zaunders JJ, Nahar K, Munier CML, Fairfax BP, Olsson-Brown A, et al. Mucosal-associated invariant T (Mait) cells are activated in the gastrointestinal tissue of patients with combination ipilimumab and nivolumab therapy-related colitis in a pathology distinct from ulcerative colitis. Clin Exp Immunol (2020) 202(3):335–52. doi: 10.1111/cei.13502
46. Luoma AM, Suo S, Williams HL, Sharova T, Sullivan K, Manos M, et al. Molecular pathways of colon inflammation induced by cancer immunotherapy. Cell (2020) 182(3):655–71. doi: 10.1016/j.cell.2020.06.001
47. Xiao G, Wang X, Wang J, Zu L, Cheng G, Hao M, et al. Cxcl16/Cxcr6 chemokine signaling mediates breast cancer progression by Perk1/2-dependent mechanisms. Oncotarget (2015) 6(16):14165–78. doi: 10.18632/oncotarget.3690
48. Chami B, Yeung AW, Van Vreden C, King NJ, Bao S. The role of Cxcr3 in dss-induced colitis. PloS One (2014) 9(7):e101622. doi: 10.1371/journal.pone.0101622
49. Coutzac C, Adam J, Soularue E, Collins M, Racine A, Mussini C, et al. Colon immune-related adverse events: Anti-Ctla-4 and anti-Pd-1 blockade induce distinct immunopathological entities. J Crohns Colitis (2017) 11(10):1238–46. doi: 10.1093/ecco-jcc/jjx081
50. Hone Lopez S, Kats-Ugurlu G, Renken RJ, Buikema HJ, de Groot MR, Visschedijk MC, et al. Immune checkpoint inhibitor treatment induces colitis with heavy infiltration of Cd8 + T cells and an infiltration pattern that resembles ulcerative colitis. Virchows Arch (2021) 479(6):1119–29. doi: 10.1007/s00428-021-03170-x
51. Sasson SC, Slevin SM, Cheung VTF, Nassiri I, Olsson-Brown A, Fryer E, et al. Interferon-Gamma-Producing Cd8+ tissue resident memory T cells are a targetable hallmark of immune checkpoint inhibitor-colitis. Gastroenterology (2021) 161(4):1229–44. doi: 10.1053/j.gastro.2021.06.025
52. Johansson-Lindbom B, Agace WW. Generation of gut-homing T cells and their localization to the small intestinal mucosa. Immunol Rev (2007) 215:226–42. doi: 10.1111/j.1600-065X.2006.00482.x
53. Abu-Sbeih H, Ali FS, Alsaadi D, Jennings J, Luo W, Gong Z, et al. Outcomes of vedolizumab therapy in patients with immune checkpoint inhibitor-induced colitis: A multi-center study. J Immunother Cancer (2018) 6(1):018–0461. doi: 10.1186/s40425-4
54. Romano E, Kusio-Kobialka M, Foukas PG, Baumgaertner P, Meyer C, Ballabeni P, et al. Ipilimumab-dependent cell-mediated cytotoxicity of regulatory T cells ex vivo by nonclassical monocytes in melanoma patients. Proc Natl Acad Sci U.S.A. (2015) 112(19):6140–5. doi: 10.1073/pnas.1417320112
55. Chaput N, Lepage P, Coutzac C, Soularue E, Le Roux K, Monot C, et al. Baseline gut microbiota predicts clinical response and colitis in metastatic melanoma patients treated with ipilimumab. Ann Oncol (2017) 28(6):1368–79. doi: 10.1093/annonc/mdx108
56. Friedlander P, Wood K, Wassmann K, Christenfeld AM, Bhardwaj N, Oh WK. A whole-blood rna transcript-based gene signature is associated with the development of ctla-4 blockade-related diarrhea in patients with advanced melanoma treated with the checkpoint inhibitor tremelimumab. J Immunother Cancer (2018) 6(1):018–0408. doi: 10.1186/s40425-9
57. Nardinocchi L, Sonego G, Passarelli F, Avitabile S, Scarponi C, Failla CM, et al. Interleukin-17 and interleukin-22 promote tumor progression in human nonmelanoma skin cancer. Eur J Immunol (2015) 45(3):922–31. doi: 10.1002/eji.201445052
58. Tarhini AA, Zahoor H, Lin Y, Malhotra U, Sander C, Butterfield LH, et al. Baseline circulating il-17 predicts toxicity while tgf-B1 and il-10 are prognostic of relapse in ipilimumab neoadjuvant therapy of melanoma. J Immunother Cancer (2015) 3(39):015–0081. doi: 10.1186/s40425-1
59. Chaudhry A, Samstein RM, Treuting P, Liang Y, Pils MC, Heinrich JM, et al. Interleukin-10 signaling in regulatory T cells is required for suppression of Th17 cell-mediated inflammation. Immunity (2011) 34(4):566–78. doi: 10.1016/j.immuni.2011.03.018
60. Blaschitz C, Raffatellu M. Th17 cytokines and the gut mucosal barrier. J Clin Immunol (2010) 30(2):196–203. doi: 10.1007/s10875-010-9368-7
61. Griffin GK, Newton G, Tarrio ML, Bu DX, Maganto-Garcia E, Azcutia V, et al. Il-17 and tnf-A sustain neutrophil recruitment during inflammation through synergistic effects on endothelial activation. J Immunol (2012) 188(12):6287–99. doi: 10.4049/jimmunol.1200385
62. Metzemaekers M, Vandendriessche S, Berghmans N, Gouwy M, Proost P. Truncation of Cxcl8 to Cxcl8(9-77) enhances actin polymerization and in vivo migration of neutrophils. J Leukoc Biol (2020) 107(6):1167–73. doi: 10.1002/JLB.3AB0220-470R
63. Veldhoen M. Interleukin 17 is a Chief orchestrator of immunity. Nat Immunol (2017) 18(6):612–21. doi: 10.1038/ni.3742
64. Dubin K, Callahan MK, Ren B, Khanin R, Viale A, Ling L, et al. Intestinal microbiome analyses identify melanoma patients at risk for checkpoint-Blockade-Induced colitis. Nat Commun (2016) 7:10391. doi: 10.1038/ncomms10391
65. Usyk M, Pandey A, Hayes RB, Moran U, Pavlick A, Osman I, et al. Bacteroides vulgatus and bacteroides dorei predict immune-related adverse events in immune checkpoint blockade treatment of metastatic melanoma. Genome Med (2021) 13(1):021–00974. doi: 10.1186/s13073-z
66. Hakozaki T, Richard C, Elkrief A, Hosomi Y, Benlaïfaoui M, Mimpen I, et al. The gut microbiome associates with immune checkpoint inhibition outcomes in patients with advanced non-small cell lung cancer. Cancer Immunol Res (2020) 8(10):1243–50. doi: 10.1158/2326-6066.CIR-20-0196
67. Mao J, Wang D, Long J, Yang X, Lin J, Song Y, et al. Gut microbiome is associated with the clinical response to anti-Pd-1 based immunotherapy in hepatobiliary cancers. J Immunother Cancer (2021) 9(12):2021–003334. doi: 10.1136/jitc
68. Chau J, Yadav M, Liu B, Furqan M, Dai Q, Shahi S, et al. Prospective correlation between the patient microbiome with response to and development of immune-mediated adverse effects to immunotherapy in lung cancer. BMC Cancer (2021) 21(1):021–08530. doi: 10.1186/s12885-z
69. Sakurai T, De Velasco MA, Sakai K, Nagai T, Nishiyama H, Hashimoto K, et al. Integrative analysis of gut microbiome and host transcriptomes reveals associations between treatment outcomes and immunotherapy-induced colitis. Mol Oncol (2022) 16(7):1493–507. doi: 10.1002/1878-0261.13062
70. Wang F, Yin Q, Chen L, Davis MM. Bifidobacterium can mitigate intestinal immunopathology in the context of ctla-4 blockade. Proc Natl Acad Sci U.S.A. (2018) 115(1):157–61. doi: 10.1073/pnas
71. Andrews MC, Duong CPM, Gopalakrishnan V, Iebba V, Chen WS, Derosa L, et al. Gut microbiota signatures are associated with toxicity to combined ctla-4 and PD-1 blockade. Nat Med (2021) 27(8):1432–41. doi: 10.1038/s41591-021-01406-6
72. Abolhassani AR, Schuler G, Kirchberger MC, Heinzerling L. C-reactive protein as an early marker of immune-related adverse events. J Cancer Res Clin Oncol (2019) 145(10):2625–31. doi: 10.1007/s00432-019-03002-1
73. Nakayama T, Saito K, Kumagai J, Nakajima Y, Kijima T, Yoshida S, et al. Higher serum c-reactive protein level represents the immunosuppressive tumor microenvironment in patients with clear cell renal cell carcinoma. Clin Genitourin Cancer (2018) 16(6):e1151–e58. doi: 10.1016/j.clgc.2018.07.027
74. Grover S, Dougan M, Tyan K, Giobbie-Hurder A, Blum SM, Ishizuka J, et al. Vitamin d intake is associated with decreased risk of immune checkpoint inhibitor-induced colitis. Cancer (2020) 126(16):3758–67. doi: 10.1002/cncr.32966
75. Fletcher J, Cooper SC, Ghosh S, Hewison M. The role of vitamin d in inflammatory bowel disease: Mechanism to management. Nutrients (2019) 11(5):1019. doi: 10.3390/nu11051019
76. Pistillo MP, Fontana V, Morabito A, Dozin B, Laurent S, Carosio R, et al. Soluble ctla-4 as a favorable predictive biomarker in metastatic melanoma patients treated with ipilimumab: An Italian melanoma intergroup study. Cancer Immunol Immunother (2019) 68(1):97–107. doi: 10.1007/s00262-018-2258-1
77. Hasan Ali O, Berner F, Bomze D, Fässler M, Diem S, Cozzio A, et al. Human leukocyte antigen variation is associated with adverse events of checkpoint inhibitors. Eur J Cancer (2019) 107:8–14. doi: 10.1016/j.ejca.2018.11.009
78. Sun S, Luo L, Liang W, Yin Q, Guo J, Rush AM, et al. Bifidobacterium alters the gut microbiota and modulates the functional metabolism of T regulatory cells in the context of immune checkpoint blockade. Proc Natl Acad Sci U.S.A. (2020) 117(44):27509–15. doi: 10.1073/pnas.1921223117
79. Fan L, Qi Y, Qu S, Chen X, Li A, Hendi M, et al. B. adolescentis ameliorates chronic colitis by regulating Treg/Th2 response and gut microbiota remodeling. Gut Microbes (2021) 13(1):1–17. doi: 10.1080/19490976.2020.1826746
80. Kuhn KA, Schulz HM, Regner EH, Severs EL, Hendrickson JD, Mehta G, et al. Bacteroidales recruit il-6-Producing intraepithelial lymphocytes in the colon to promote barrier integrity. Mucosal Immunol (2018) 11(2):357–68. doi: 10.1038/mi.2017.55
81. Wegorzewska MM, Glowacki RWP, Hsieh SA, Donermeyer DL, Hickey CA, Horvath SC, et al. Diet modulates colonic T cell responses by regulating the expression of a bacteroides thetaiotaomicron antigen. Sci Immunol (2019) 4(32):eaau9079. doi: 10.1126/sciimmunol.aau9079
82. Li K, Hao Z, Du J, Gao Y, Yang S, Zhou Y. Bacteroides thetaiotaomicron relieves colon inflammation by activating aryl hydrocarbon receptor and modulating Cd4 + T cell homeostasis. Int Immunopharmacol (2021) 90:107183. doi: 10.1016/j.intimp.2020.107183
83. Round JL, Mazmanian SK. Inducible Foxp3+ regulatory T-cell development by a commensal bacterium of the intestinal microbiota. Proc Natl Acad Sci U.S.A. (2010) 107(27):12204–9. doi: 10.1073/pnas.0909122107
84. Chiu CC, Ching YH, Wang YC, Liu JY, Li YP, Huang YT, et al. Monocolonization of germ-free mice with bacteroides fragilis protects against dextran sulfate sodium-induced acute colitis. BioMed Res Int (2014) 675786(10):29. doi: 10.1155/2014/675786
85. Troy EB, Kasper DL. Beneficial effects of bacteroides fragilis polysaccharides on the immune system. Front Biosci (2010) 15(1):25–34. doi: 10.2741/3603
86. Dasgupta S, Erturk-Hasdemir D, Ochoa-Reparaz J, Reinecker HC, Kasper DL. Plasmacytoid dendritic cells mediate anti-inflammatory responses to a gut commensal molecule Via both innate and adaptive mechanisms. Cell Host Microbe (2014) 15(4):413–23. doi: 10.1016/j.chom.2014.03.006
87. Cuffaro B, Assohoun ALW, Boutillier D, Súkeníková L, Desramaut J, Boudebbouze S, et al. In vitro characterization of gut microbiota-derived commensal strains: Selection of parabacteroides distasonis strains alleviating tnbs-induced colitis in mice. Cells (2020) 9(9):2104. doi: 10.3390/cells9092104
88. Chan JL, Wu S, Geis AL, Chan GV, Gomes TAM, Beck SE, et al. Non-toxigenic bacteroides fragilis (Ntbf) administration reduces bacteria-driven chronic colitis and tumor development independent of polysaccharide a. Mucosal Immunol (2019) 12(1):164–77. doi: 10.1038/s41385-018-0085-5
89. Heshiki Y, Vazquez-Uribe R, Li J, Ni Y, Quainoo S, Imamovic L, et al. Predictable modulation of cancer treatment outcomes by the gut microbiota. Microbiome (2020) 8(1):020–00811. doi: 10.1186/s40168-2
90. Huang Y, Tang J, Cai Z, Zhou K, Chang L, Bai Y, et al. Prevotella induces the production of Th17 cells in the colon of mice. J Immunol Res (2020) 1:9607328. doi: 10.1155/2020/9607328
91. Kehrmann J, Effenberg L, Wilk C, Schoemer D, Ngo Thi Phuong N, Adamczyk A, et al. Depletion of Foxp3 + regulatory T cells is accompanied by an increase in the relative abundance of firmicutes in the murine gut microbiome. Immunology (2020) 159(3):344–53. doi: 10.1111/imm.13158
92. Zhang SL, Han B, Mao YQ, Zhang ZY, Li ZM, Kong CY, et al. Lacticaseibacillus paracasei Sh2020 induced antitumor immunity and synergized with anti-programmed cell death 1 to reduce tumor burden in mice. Gut Microbes (2022) 14(1):2046246. doi: 10.1080/19490976.2022
93. Tan B, Tang H, Xu Y, Chen MJ, Wang MZ, Qian JM. Protective effect and mechanism of lactobacillus rhamnosus on immune checkpoint inhibitors related colitis in mice. Zhonghua Yi Xue Za Zhi (2020) 100(42):3332–37. doi: 10.3760/cma.j.cn112137-20200520-01598
94. Han SK, Shin YJ, Lee DY, Kim KM, Yang SJ, Kim DS, et al. Lactobacillus rhamnosus Hdb1258 modulates gut microbiota-mediated immune response in mice with or without lipopolysaccharide-induced systemic inflammation. BMC Microbiol (2021) 21(1):021–02192. doi: 10.1186/s12866-4
95. Mann ER, Bernardo D, Ng SC, Rigby RJ, Al-Hassi HO, Landy J, et al. Human gut dendritic cells drive aberrant gut-specific T-cell responses in ulcerative colitis, characterized by increased il-4 production and loss of il-22 and ifnγ. Inflammation Bowel Dis (2014) 20(12):2299–307. doi: 10.1097/MIB.0000000000000223
96. Wang Y, Gu Y, Fang K, Mao K, Dou J, Fan H, et al. Lactobacillus acidophilus and clostridium butyricum ameliorate colitis in murine by strengthening the gut barrier function and decreasing inflammatory factors. Benef Microbes (2018) 9(5):775–87. doi: 10.3920/BM2017.0035
97. Jang YJ, Kim WK, Han DH, Lee K, Ko G. Lactobacillus fermentum species ameliorate dextran sulfate sodium-induced colitis by regulating the immune response and altering gut microbiota. Gut Microbes (2019) 10(6):696–711. doi: 10.1080/19490976.2019.1589281
98. Park JS, Choi JW, Jhun J, Kwon JY, Lee BI, Yang CW, et al. Lactobacillus acidophilus improves intestinal inflammation in an acute colitis mouse model by regulation of Th17 and treg cell balance and fibrosis development. J Med Food (2018) 21(3):215–24. doi: 10.1089/jmf.2017.3990
99. Mcculloch JA, Davar D, Rodrigues RR, Badger JH, Fang JR, Cole AM, et al. Intestinal microbiota signatures of clinical response and immune-related adverse events in melanoma patients treated with anti-Pd-1. Nat Med (2022) 28(3):545–56. doi: 10.1038/s41591-022-01698-2
100. Ma QY, Huang DY, Zhang HJ, Wang S, Chen XF. Upregulation of bacterial-specific Th1 and Th17 responses that are enriched in Cxcr5 + Cd4 + T cells in non-small cell lung cancer. Int Immunopharmacol (2017) 52:305–09. doi: 10.1016/j.intimp.2017.09.024
101. Wen X, Wang HG, Zhang MN, Zhang MH, Wang H, Yang XZ. Fecal microbiota transplantation ameliorates experimental colitis Via gut microbiota and T-cell modulation. World J Gastroenterol (2021) 27(21):2834–49. doi: 10.3748/wjg.v27.i21.2834
102. Tanoue T, Morita S, Plichta DR, Skelly AN, Suda W, Sugiura Y, et al. A defined commensal consortium elicits Cd8 T cells and anti-cancer immunity. Nature (2019) 565(7741):600–05. doi: 10.1038/s41586-019-0878-z
103. Ioannidis M, Cerundolo V, Salio M. The immune modulating properties of mucosal-associated invariant T cells. Front Immunol (2020) 11:1556. doi: 10.3389/fimmu.2020.01556
104. Maifeld A, Bartolomaeus H, Löber U, Avery EG, Steckhan N, Markó L, et al. Fasting alters the gut microbiome reducing blood pressure and body weight in metabolic syndrome patients. Nat Commun (2021) 12(1):021–22097. doi: 10.1038/s41467-0
105. Wang L, Tang L, Feng Y, Zhao S, Han M, Zhang C, et al. A purified membrane protein from akkermansia muciniphila or the pasteurised bacterium blunts colitis associated tumourigenesis by modulation of Cd8 + T cells in mice. Gut (2020) 69(11):1988–97. doi: 10.1136/gutjnl-2019-320105
106. Wong JM, De Souza R, Kendall CW, Emam A, Jenkins DJ. Colonic health: Fermentation and short chain fatty acids. J Clin Gastroenterol (2006) 40(3):235–43. doi: 10.1097/00004836-200603000-00015
107. Wahlström A, Sayin SI, Marschall HU, Bäckhed F. Intestinal crosstalk between bile acids and microbiota and its impact on host metabolism. Cell Metab (2016) 24(1):41–50. doi: 10.1016/j.cmet.2016.05.005
108. Roager HM, Licht TR. Microbial tryptophan catabolites in health and disease. Nat Commun (2018) 9(1):018–05470. doi: 10.1038/s41467-4
109. Smith PM, Howitt MR, Panikov N, Michaud M, Gallini CA, Bohlooly-Y M, et al. The microbial metabolites, short-chain fatty acids, regulate colonic treg cell homeostasis. Science (2013) 341(6145):569–73. doi: 10.1126/science.1241165
110. Chang PV, Hao L, Offermanns S, Medzhitov R. The microbial metabolite butyrate regulates intestinal macrophage function Via histone deacetylase inhibition. Proc Natl Acad Sci U.S.A. (2014) 111(6):2247–52. doi: 10.1073/pnas.1322269111
111. Singh N, Gurav A, Sivaprakasam S, Brady E, Padia R, Shi H, et al. Activation of Gpr109a, receptor for niacin and the commensal metabolite butyrate, suppresses colonic inflammation and carcinogenesis. Immunity (2014) 40(1):128–39. doi: 10.1016/j.immuni.2013.12.007
112. Furusawa Y, Obata Y, Fukuda S, Endo TA, Nakato G, Takahashi D, et al. Commensal microbe-derived butyrate induces the differentiation of colonic regulatory T cells. Nature (2013) 504(7480):446–50. doi: 10.1038/nature12721
113. Zhang M, Zhou Q, Dorfman RG, Huang X, Fan T, Zhang H, et al. Butyrate inhibits interleukin-17 and generates tregs to ameliorate colorectal colitis in rats. BMC Gastroenterol (2016) 16(1):016–0500. doi: 10.1186/s12876-x
114. Yang W, Yu T, Huang X, Bilotta AJ, Xu L, Lu Y, et al. Intestinal microbiota-derived short-chain fatty acids regulation of immune cell il-22 production and gut immunity. Nat Commun (2020) 11(1):020–18262. doi: 10.1038/s41467-6
115. Maslowski KM, Vieira AT, Ng A, Kranich J, Sierro F, Yu D, et al. Regulation of inflammatory responses by gut microbiota and chemoattractant receptor Gpr43. Nature (2009) 461(7268):1282–6. doi: 10.1038/nature08530
116. Sun M, Wu W, Chen L, Yang W, Huang X, Ma C, et al. Microbiota-derived short-chain fatty acids promote Th1 cell il-10 production to maintain intestinal homeostasis. Nat Commun (2018) 9(1):018–05901. doi: 10.1038/s41467-2
117. Dupraz L, Magniez A, Rolhion N, Richard ML, Da Costa G, Touch S, et al. Gut microbiota-derived short-chain fatty acids regulate il-17 production by mouse and human intestinal Γδ T cells. Cell Rep (2021) 36(1):109332. doi: 10.1016/j.celrep.2021
118. Nastasi C, Candela M, Bonefeld CM, Geisler C, Hansen M, Krejsgaard T, et al. The effect of short-chain fatty acids on human monocyte-derived dendritic cells. Sci Rep (2015) 5:16148. doi: 10.1038/srep16148
119. Sorrentino G, Perino A, Yildiz E, El Alam G, Bou Sleiman M, Gioiello A, et al. Bile acids signal Via Tgr5 to activate intestinal stem cells and epithelial regeneration. Gastroenterology (2020) 159(3):956–68. doi: 10.1053/j.gastro.2020.05.067
120. Paik D, Yao L, Zhang Y, Bae S, D’Agostino GD, Zhang M, et al. Human gut bacteria produce T(H)17-modulating bile acid metabolites. Nature (2022) 603(7903):907–12. doi: 10.1038/s41586-022-04480-z
121. Campbell C, Mckenney PT, Konstantinovsky D, Isaeva OI, Schizas M, Verter J, et al. Bacterial metabolism of bile acids promotes generation of peripheral regulatory T cells. Nature (2020) 581(7809):475–79. doi: 10.1038/s41586-020-2193-0
122. Jennis M, Cavanaugh CR, Leo GC, Mabus JR, Lenhard J, Hornby PJ, et al. Microbiota-derived tryptophan indoles increase after gastric bypass surgery and reduce intestinal permeability in vitro and in vivo. Neurogastroenterol Motil (2018) 30(2):e13178. doi: 10.1111/nmo.13178
123. Zhang J, Zhu S, Ma N, Johnston LJ, Wu C, Ma X. Metabolites of microbiota response to tryptophan and intestinal mucosal immunity: A therapeutic target to control intestinal inflammationophan and intestinal mucosal immunity. Med Res Rev (2021) 41(2):1061–88. doi: 10.1002/med.21752
124. Scott SA, Fu J, Chang PV. Microbial tryptophan metabolites regulate gut barrier function Via the aryl hydrocarbon receptor. Proc Natl Acad Sci U.S.A. (2020) 117(32):19376–87. doi: 10.1073/pnas.2000047117
125. Ihekweazu FD, Engevik MA, Ruan W, Shi Z, Fultz R, Engevik KA, et al. Bacteroides ovatus promotes il-22 production and reduces trinitrobenzene sulfonic acid-driven colonic inflammation. Am J Pathol (2021) 191(4):704–19. doi: 10.1016/j.ajpath.2021.01.009
126. Busbee PB, Menzel L, Alrafas HR, Dopkins N, Becker W, Miranda K, et al. Indole-3-Carbinol prevents colitis and associated microbial dysbiosis in an il-22-Dependent manner. JCI Insight (2020) 5(1):127551. doi: 10.1172/jci.insight
127. Powell DN, Swimm A, Sonowal R, Bretin A, Gewirtz AT, Jones RM, et al. Indoles from the commensal microbiota act Via the ahr and il-10 to tune the cellular composition of the colonic epithelium during aging. Proc Natl Acad Sci U.S.A. (2020) 117(35):21519–26. doi: 10.1073/pnas.2003004117
128. Krishnan S, Ding Y, Saedi N, Choi M, Sridharan GV, Sherr DH, et al. Gut microbiota-derived tryptophan metabolites modulate inflammatory response in hepatocytes and macrophages. Cell Rep (2018) 23(4):1099–111. doi: 10.1016/j.celrep.2018.03.109
129. Mohiuddin JJ, Chu B, Facciabene A, Poirier K, Wang X, Doucette A, et al. Association of antibiotic exposure with survival and toxicity in patients with melanoma receiving immunotherapy and toxicity in patients with. J Natl Cancer Inst (2021) 113(2):162–70. doi: 10.1093/jnci/djaa057
130. Abu-Sbeih H, Herrera LN, Tang T, Altan M, Chaftari AP, Okhuysen PC, et al. Impact of antibiotic therapy on the development and response to treatment of immune checkpoint inhibitor-mediated diarrhea and colitis. J Immunother Cancer (2019) 7(1):019–0714. doi: 10.1186/s40425-x
131. Khanna S, Vazquez-Baeza Y, González A, Weiss S, Schmidt B, Muñiz-Pedrogo DA, et al. Changes in microbial ecology after fecal microbiota transplantation for recurrent c. difficile infection affected by underlying inflammatory bowel disease. Microbiome (2017) 5(1):017–0269. doi: 10.1186/s40168-3
132. Fasanello MK, Robillard KT, Boland PM, Bain AJ, Kanehira K. Use of fecal microbial transplantation for immune checkpoint inhibitor colitis. ACG Case Rep J (2020) 7(4):0000000000000360. doi: 10.14309/crj
133. Wang Y, Wiesnoski DH, Helmink BA, Gopalakrishnan V, Choi K, DuPont HL, et al. Fecal microbiota transplantation for refractory immune checkpoint inhibitor-associated colitis. Nat Med (2018) 24(12):1804–08. doi: 10.1038/s41591-018-0238-9
134. Sunkara T, Rawla P, Ofosu A, Gaduputi V. Fecal microbiota transplant - a new frontier in inflammatory bowel disease. J Inflammation Res (2018) 11:321–28. doi: 10.2147/JIR.S176190
135. Hu Y, Le Leu RK, Christophersen CT, Somashekar R, Conlon MA, Meng XQ, et al. Manipulation of the gut microbiota using resistant starch is associated with protection against colitis-associated colorectal cancer in rats. Carcinogenesis (2016) 37(4):366–75. doi: 10.1093/carcin/bgw019
136. Grabinger T, Glaus Garzon JF, Hausmann M, Geirnaert A, Lacroix C, Hennet T. Alleviation of intestinal inflammation by oral supplementation with 2-fucosyllactose in mice. Front Microbiol (2019) 10:1385. doi: 10.3389/fmicb.2019.01385
137. Wang L, Cheng X, Bai L, Gao M, Kang G, Cao X, et al. Positive interventional effect of engineered butyrate-producing bacteria on metabolic disorders and intestinal flora disruption in obese mice. Microbiol Spectr (2022) 10(2):01147–21. doi: 10.1128/spectrum
138. Yan X, Liu XY, Zhang D, Zhang YD, Li ZH, Liu X, et al. Construction of a sustainable 3-Hydroxybutyrate-Producing probiotic escherichia coli for treatment of colitis. Cell Mol Immunol (2021) 18(10):2344–57. doi: 10.1038/s41423-021-00760-2
139. Wang L, Liao Y, Yang R, Zhu Z, Zhang L, Wu Z, et al. An engineered probiotic secreting Sj16 ameliorates colitis Via Ruminococcaceae/Butyrate/Retinoic acid axis. Bioeng Transl Med (2021) 6(3):e10219. doi: 10.1002/btm2.10219
140. Cui M, Pang G, Zhang T, Sun T, Zhang L, Kang R, et al. Optotheranostic nanosystem with phone visual diagnosis and optogenetic microbial therapy for ulcerative colitis at-home care. ACS Nano (2021) 15(4):7040–52. doi: 10.1021/acsnano.1c00135
141. Praveschotinunt P, Duraj-Thatte AM, Gelfat I, Bahl F, Chou DB, Joshi NS, et al. Engineered e. coli nissle 1917 for the delivery of matrix-tethered therapeutic domains to the gut. Nat Commun (2019) 10(1):019–13336. doi: 10.1038/s41467-6
142. Akamatsu H, Murakami E, Oyanagi J, Shibaki R, Kaki T, Takase E. Immune-related adverse events by immune checkpoint inhibitors significantly predict durable efficacy even in responders with advanced non-small cell lung cancer. Oncologist (2020) 25(4):e679–e83. doi: 10.1634/theoncologist.2019-0299
Keywords: gut microbiota, immune-related adverse events, colitis, immune checkpoint inhibitor, immunity, mechanism, clinical application
Citation: Han X, Zang D, Liu D and Chen J (2022) The multifaceted roles of common gut microbiota in immune checkpoint inhibitor-mediated colitis: From mechanism to clinical application. Front. Immunol. 13:988849. doi: 10.3389/fimmu.2022.988849
Received: 07 July 2022; Accepted: 26 August 2022;
Published: 14 September 2022.
Edited by:
Stephanie Dorta-Estremera, University of Puerto Rico, Puerto RicoReviewed by:
Wang Ma, First Affiliated Hospital of Zhengzhou University, ChinaCopyright © 2022 Han, Zang, Liu and Chen. This is an open-access article distributed under the terms of the Creative Commons Attribution License (CC BY). The use, distribution or reproduction in other forums is permitted, provided the original author(s) and the copyright owner(s) are credited and that the original publication in this journal is cited, in accordance with accepted academic practice. No use, distribution or reproduction is permitted which does not comply with these terms.
*Correspondence: Jun Chen, Y2hlbmp1bmRsQHZpcC5zaW5hLmNvbQ==
†These authors have contributed equally to this work
Disclaimer: All claims expressed in this article are solely those of the authors and do not necessarily represent those of their affiliated organizations, or those of the publisher, the editors and the reviewers. Any product that may be evaluated in this article or claim that may be made by its manufacturer is not guaranteed or endorsed by the publisher.
Research integrity at Frontiers
Learn more about the work of our research integrity team to safeguard the quality of each article we publish.