- 1Unidad de Bioquímica, Instituto Nacional de Ciencias Médicas y Nutrición Salvador Zubirán, Mexico City, Mexico
- 2Facultad de Ciencias, Universidad Nacional Autónoma de México (UNAM), Mexico City, Mexico
- 3Área de matemáticas, preparatoria agrícola, Universidad Autónoma Chapingo, Texcoco, Mexico
- 4Departamento de Investigación, Hospital Regional ISSSTE, Puebla, Mexico
- 5Immunology Center, Georgetown University, Washington, DC, United States
- 6Subdirección de Investigación Biomédica, Instituto Nacional de Enfermedades Respiratorias Ismael Cosío Villegas, Mexico City, Mexico
Antimicrobial resistance (AMR) is a global health problem that causes more than 1.27 million deaths annually; therefore, it is urgent to focus efforts on solving or reducing this problem. The major causes of AMR are the misuse of antibiotics and antimicrobials in agriculture, veterinary medicine, and human medicine, which favors the selection of drug-resistant microbes. One of the strategies proposed to overcome the problem of AMR is to use polyvalent human immunoglobulin or IVIG. The main advantage of this classic form of passive immunization is its capacity to enhance natural immunity mechanisms to eliminate bacteria, viruses, or fungi safely and physiologically. Experimental data suggest that, for some infections, local administration of IVIG may produce better results with a lower dose than intravenous application. This review presents evidence supporting the use of polyvalent human immunoglobulin in AMR, and the potential and challenges associated with its proposed usage.
Introduction
With the discovery of penicillin by Fleming, antibiotics first appeared as a potential “magic bullet” that could specifically target disease-causing microorganisms without affecting the host; thus constituting a revolution in medicine (1). Soon after, due to the countless lives saved, research advancements led to the discovery of other novel antibiotic classes; thus, the 1950s to 1970s period was considered “the golden era for antibiotics” (2). Many other antimicrobials, for example those directed at fungal and parasitic agents, were also developed during the latter part of the 20th century (3).
Unfortunately, time and experience showed that several microorganisms developed resistance to almost all of the antibiotics discovered during that period, and many of these drugs became obsolete over the past decades (4). The Nobel Prize in Physiology or Medicine in 1945 was awarded jointly to Sir Alexander Fleming, Ernst Boris Chain, and Sir Howard Walter Florey “for the discovery of penicillin and its curative effect in various infectious diseases.” Ironically, during his Nobel Award acceptance lecture, Fleming made the following prophetic statement: “The time may come when penicillin can be bought by anyone in the shops. Then, there is the danger that the ignorant man may easily underdose himself and, by exposing his microbes to non-lethal quantities of the drug, make them resistant.”
Antimicrobial resistance (AMR) occurs in nature, independent of human activity, as an evolutionary process caused by environmental pressures introduced by changing conditions. AMR also appears rapidly due to the selective pressure from the overuse of antimicrobial drugs for human medical treatment and animal husbandry to combat or prevent infectious diseases.
Currently, AMR constitutes a global health problem in medicine for both community- and hospital-acquired infections, causing an increase in disease severity and mortality, and leading to a reduction in therapeutic options. The main bacterial species presenting the highest frequency of AMR of medical interest include the following members of the ESKAPE group: Enterococcus faecium, Staphylococcus aureus, Klebsiella pneumoniae, Acinetobacter baumannii, Pseudomonas aeruginosa, and Enterobacteria spp. (E. coli, Salmonella spp., and Shigella spp.) (5). Other bacteria with an increased frequency of AMR are gonococci and Mycobacterium tuberculosis (see Table 1). In 2017, the World Health Organization called upon the global community of nations to direct its attention to the international challenge of AMR, particularly gram-negative bacteria, and to take action against this problem (16). Other microbes of medical importance that cause infections with high resistance to antimicrobials and high mortality associated with AMR include the fungi Aspergillus and Candida (e.g., Candida auris) (17–20).
In addition, microorganisms in the environment and in clinics may develop resistance to multiple classes of antimicrobials, becoming microbes known as “superbugs”. This is not just a laboratory concern, but has become a global health peril. Infections caused by superbugs are associated with high death tolls and increased economic costs, making it imperative to seek alternative therapies to treat those infections (21, 22). This review investigates the potential use of human intravenous polyvalent immunoglobulin (IVIG) as an alternative or adjuvant therapy to avoid AMR in human infectious diseases.
Alternative strategies for infection control
In a recent study on the global burden of AMR, based on data from 204 countries and territories, there were an estimated 4.95 million deaths worldwide associated with AMR, including 1.27 million deaths directly attributable to bacterial AMR (23). Therefore, there is a need to reduce the effects of AMR while simultaneously permitting the adequate control of infectious diseases in humans, and the performance of productive activities, such as agriculture and livestock, in a balanced way. Table 2 summarizes the main approaches to avoid or reduce the impact of AMR.
Alternative strategies to control antimicrobial drug resistance include: a) a reduction in the use of antimicrobials, b) the development of new AM drugs, and c) the implementation of novel non-antibiotic therapies for infections. Reducing the use of antibiotics is a simple and inexpensive measure for lowering AMR, but it requires a global effort to educate both health care providers and the public. This would dramatically reduce the indiscriminate prescription and demand for antimicrobials (24). A second approach is the development of new antimicrobials, which is time-consuming and costly, from drug discovery to laboratory tests and clinical trials, before a new antimicrobial is licensed for general use in humans (24, 25). The third strategy to find drugs to combat infections, distinct from the existing antimicrobials, includes several options presented below.
Many plant-derived chemical compounds with antimicrobial properties, primarily polyphenols, terpenes, and flavonoids, have shown good results in vitro to eliminate diverse bacteria with AMR, as tested alone or in combination with known antibiotics (26). However, controlled clinical trials are warranted. Alternatively, the development of new antimicrobials by chemically modifying existing drugs is relatively faster and less expensive than the development of new AM drugs, but the rapid emergence of strains resistant to the new drugs is very likely. Another strategy to find new antimicrobial drugs is drug repurposing, which allows for the identification of new effects of existing pharmacological drugs for treating humans with a known safety profile but without the investment of cost and time. The discovery of unexpected secondary beneficial effects of some drugs was found by serendipity, but today there are systematic repurposing strategies based on experimental or in silico approaches. There are many databases of drugs and their pharmacological effects intended for repository use in cancer, autoimmune diseases, and infectious diseases (27, 28).
Phage therapy is an alternative tool to fight human bacterial infections, distinct from antibiotics, and it consists of the delivery of bacterial-specific lytic viruses (bacteriophages, i.e., literally ‘bacterial eaters’, or simply phages). Phages infect bacteria through specific receptors, and then they inject their nucleic acids, followed by the replication of the virus, assembly inside the bacterial cell body and finally the lysis of the bacteria in a host-specific way. The approach is hypothetically ideal because phages are ubiquitous, harmless to all their surroundings and can be administered orally (29). An advantage of phage therapy is that it can leave the beneficial commensal microbiota intact, targeting only harmful bacteria, and phages can be auto renewed while there is a bacterial host available. Phage therapy for infections has been reported in clinical case reports (30, 31) and clinical trials (32), showing variable efficacy against infections (33), and it is currently considered primarily experimental. Additional work is required to establish which are the best infections to treat and the optimal methods to administer potential phage therapies. Another promising non-antibiotic therapy relies on antimicrobial peptides (AMPs), which are antimicrobial agents produced by all living organisms (21). AMPs are amphipathic peptides that can be inserted into the cell wall and cellular membranes of microbes, causing destabilization and disruption (34). Additionally, vaccines constitute a weapon to prevent infectious diseases and antibiotic resistance. The prophylactic use of vaccines allows the host to build an immune response before encountering the pathogen. Thus, following vaccination, infections caused by the occurrence of drug-resistant microbes and superbugs may be less likely to occur (35).
Unfortunately, antibiotic resistance has dramatically increased worldwide in recent decades, with no signs of receding. It adds to the burden on health care and can potentially contribute to the end of the “antibiotic era” (36). Non-antibiotic therapies are promising alternative therapies to combat drug-resistant microbes and superbugs. The discovery of novel classes of antibiotics or alternative therapies requires urgent attention as we move toward a post-antibiotic era (22).
Mechanisms of action of IVIG and potential use as adjuvant treatment for infections
The use of polyvalent intravenous immunoglobulin (IVIG) constitutes an alternative or complementary therapy to treat infections caused by drug-resistant microbes and superbugs. Commercially available IVIG products are derived from pooled plasma from thousands of healthy donors. These products consist primarily of IgG, and traces of IgA and IgM antibodies (37), which are able to recognize millions of molecules in thousands of microbes (viruses, bacteria, protozoa, fungi, and helminths) due to natural infections or vaccine stimulation in donors (38).
There are two uses of immunoglobulin therapy: replacement therapy, and immunomodulatory therapy. IVIG was produced initially as a replacement therapy for children with primary immunodeficiency (PID) and low levels of IgG (agammaglobulinemia or in severe combined immunodeficiency), and it helped to prevent infections in those children (39). IVIG is usually administered to patients with PID intravenously (IV), between 200 and 600 mg/kg of body weight for restitution therapy every 3-4 weeks. Alternative subcutaneous (SC) administration was performed at a weekly dose of 100 mg/kg of body weight. Most of these therapies are prophylactic. However, the dose may be adjusted depending on the clinical characteristics of the patients: e.g., the frequency and intensity of infections, the level of hypogammaglobulinemia, and the prophylactic use of antibiotics (40). The second use of immunoglobulin is based on its immunomodulatory properties when used in a higher concentration in the treatment of several autoimmune diseases, such as idiopathic thrombocytopenic purpura (ITP), rheumatoid arthritis, systemic lupus erythematosus (SLE), and myelin neurodegenerative autoimmune diseases, such as multiple sclerosis. Higher concentrations of IVIG are required to treat these autoimmune disorders, usually 1 or 2 g/kg every 4 weeks (41–44). However, the great diversity of primary immunodeficiencies, spanning more than 350 diseases caused by mutations in more than 480 genes, is related not only to infection susceptibility but also to autoimmunity, allergy, inflammation, and malignancy (45). Thus, in clinical practice, there is variability in the IVIG dose administered to patients with PID and infections, depending on their pathogenesis and on their clinical manifestations. Although some meta-analysis studies have shown that a higher monthly dose of IVIG is related to a lower risk of infections (46, 47), another meta-analysis study with subcutaneous IgG did not find a significant clinical advantage linked to increasing doses. Therefore, the IVIG dose administered to distinct patients may be individually tailored, with a balance between reducing infections and side effects caused by treatment (48).
There are several mechanisms by which IVIG mediates its antimicrobial effects and modulates the immune response. First, gamma globulin can bind directly to the cell surface membrane or to one of its appendages, e.g., pili or flagella, extirpating the functional activity of the microbe. The mechanisms underlying the beneficial effects of immunoglobulins in infections are thought to involve toxin and superantigen neutralization, bacterial opsonization enhancing phagocytosis, and intracellular killing (49–53). During viral infections, IVIG may neutralize viruses before they infect host cells; in addition, immunoglobulins also recognize viral antigens expressed on the membrane of infected cells and may induce antibody-dependent cell-mediated cytotoxicity by NK cells, which eliminate virus-infected cells (54). Moreover, IVIG also has several immunomodulatory properties. For example, in dermatomyositis, an inflammatory disease, IVIG modulates complement activation mediated by the Fc fraction, binding C3b and C4b fragments, and inhibiting the formation and endothelial deposition of the C5-C9 membrane attack complex (MAC), which causes tissue damage in the microvasculature (55). IVIG also binds and neutralizes the complement anaphylatoxins C3a and C5a via the F(ab)´2 fraction, inhibiting the production of histamine and thromboxane by diverse cells; IVIG also protects pigs from the lethal effects of C5a anaphylatoxin (56). In addition, during its manufacture, IVIG is subjected to physicochemical changes, solvents, detergents, and stabilizers to assure sterility and to prevent the formation of IgG aggregates that might activate complement or activate innate immune cells via FcγR (57, 58). Other immunomodulator properties of IVIG include the inhibition of autoantibodies by idiotype networks, the saturation of the FcRn receptor (neonatal Fc receptor) aiding in the destruction of autoantibodies, the functional blockade of Fc receptors modulating the activation of leukocytes and cytokine production, and also the influence on the maturation and activation of dendritic cells, macrophages, NK, and other cell populations (59–62). Some of the effector properties of IVIG are modulated by the Fc fraction, while others are modulated by the F(ab)´2 fraction (63). Most of the mechanisms underlying the immunomodulatory effects of IVIG have been studied, primarily, in autoimmune or inflammatory diseases, but they also participate in the inflammation associated with infections. It is beyond the scope of this review to cover all the mechanisms of action of IVIG, so readers interested in a more detailed description of those mechanisms are advised to consult the excellent reviews of the topic (61, 63, 64).
Interestingly, many pathogenic gram-negative bacteria, such as Pseudomonas, Yersinia, Salmonella or Shigella, have a special toxin secretory apparatus, Type III, which these bacteria use to actively inject toxins into the cytoplasm of the host cells. This toxin-release mechanism avoids extracellular recognition by antibodies. Antigen V was discovered in Yersinia pestis and is a component of the secretion apparatus, which is located on the needle-shaped tip of the bacterial secretion system. IVIG contains antibodies able to recognize and neutralize the antigen PcrV (a homolog of antigen V) from Pseudomonas aeruginosa, increasing the survival of Pseudomonas-infected mice (65). Therefore, IVIG may have a potential fifth mechanism for bacterial neutralization, which interferes with the type III toxin-secretory apparatus. This fifth IVIG bacterial neutralization mechanism may be functional even for bacteria with AMR (66). Figure 1 shows the main mechanisms attributed to IVIG for aiding in the control of infections.
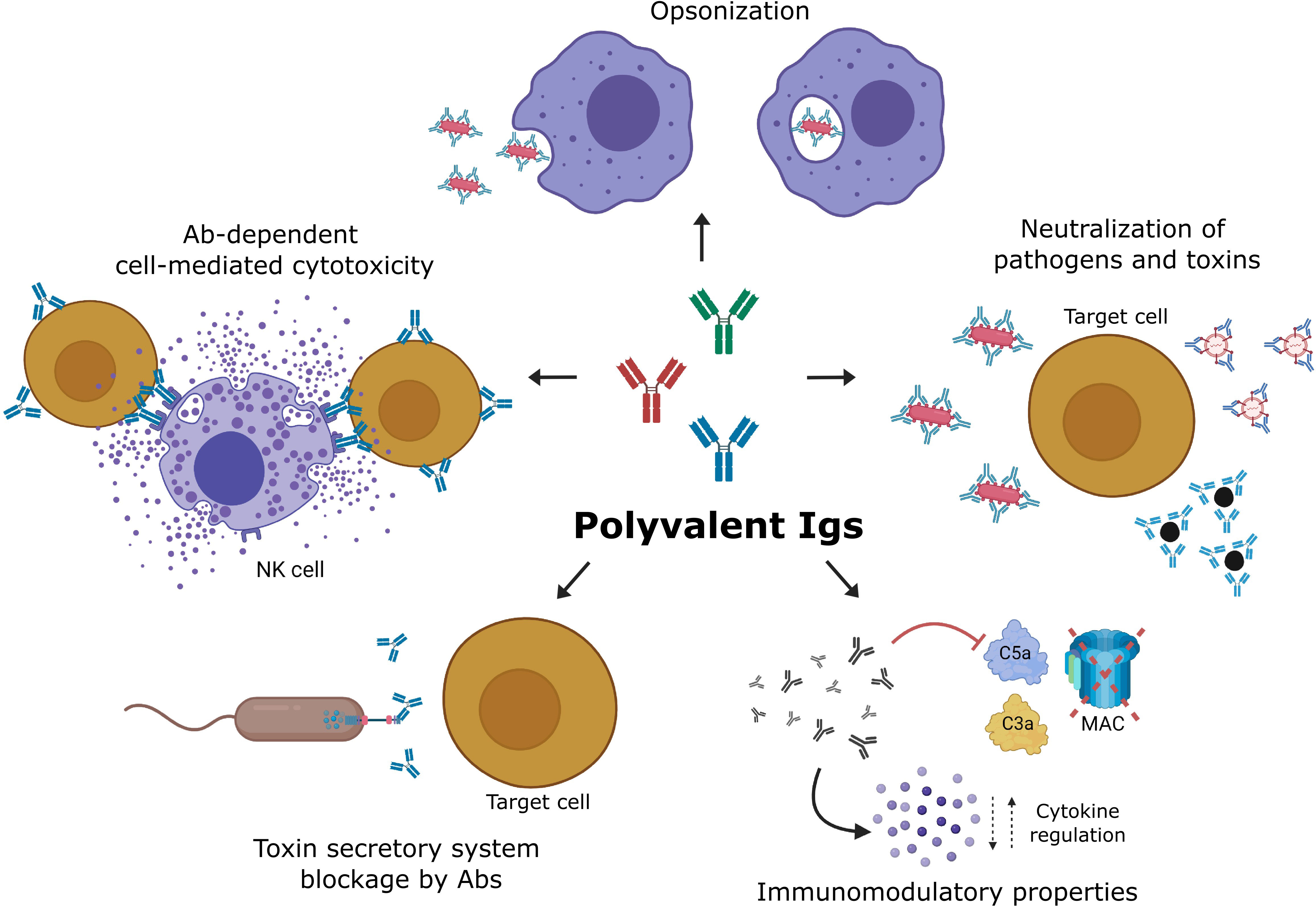
Figure 1 Mechanisms of action of IVIG during the treatment of infections. The neutralization of microbes, toxins, and superantigens blocks the interaction with receptors, preventing their internalization and effects. The opsonization of distinct microbes, such as viruses, bacteria, and fungi, facilitates their phagocytosis and intracellular killing. Antibody-dependent cell-mediated cytotoxicity (ADCC) allows NK cells to kill virus-infected cells that express viral antigens on their membranes. Antibodies of IVIG may also recognize and block components of the Type III toxin secretory system of bacteria, inhibiting toxin injection into host cells. Immunoglobulin molecules in IVIG preparations scavenge activated complement fragments and, thereby, prevent indiscriminate lysis of host cells, immune damage mediated by enhanced opsonophagocytosis, and exaggerated inflammatory reaction. Created with BioRender.com.
The antibodies contained in IVIG promote mechanisms for killing microbes that depend on the enhancement of the normal immune response, even if microbes present AMR. For example, Ono and colleagues (67) experimentally tested the in vitro phagocytosis of sensitive or antibiotic-resistant bacteria, including vancomycin-resistant Staphylococcus aureus (VRSA), methicillin-resistant S. aureus (MRSA), penicillin-resistant Streptococcus pneumoniae (PRSP), and extended spectrum beta-lactamase (ESBL) producers E. coli and K. pneumoniae, by human polymorphonuclear cells (PMN). Opsonization of those bacteria with IVIG increased phagocytosis, and the opsonic indices were quite similar between drug-resistant and drug-sensitive strains. Therefore, the potential of IVIG to control or eliminate microbes that present AMR and superbugs is enormous, making it an attractive treatment option in the post-antibiotic era.
Laboratory experimental evidence for the use of IVIG against infections, including AMR
The results from in vitro studies suggested that IVIG should be considered a viable option to treat diseases caused by bacteria. Human PMNs obtained from patients with septicemia caused by gram-negative bacteria showed an initial reduction in their phagocytic and ROS (reactive oxygen species) production capacity compared to healthy controls. Following in vitro opsonization of E. coli with IVIG and IgM-enriched IVIG using PMNs from these patients, there was a significant increase in both phagocytosis and ROS production (68). Itoh and colleagues (69) demonstrated that phagocytic activity and killing by human neutrophils against drug-resistant E. coli and P. aeruginosa was increased in the presence of IVIG and was associated with autophagy. Matsuo and colleagues (70) found that in vitro IVIG increases the ROS production and killing of multidrug resistant bacteria by neutrophils isolated from patients receiving immunosuppressive drugs after hematopoietic stem cell transplantation (HSCT).
In vivo studies using mouse models have also assessed the efficacy of IVIG against several bacterial infections. For example, Ramisse and colleagues (71) demonstrated that immunosuppressed mice that were challenged intranasally with S. pneumoniae and treated with human complete IVIG or with F(ab)´2 were protected against infection. The protective effect was reached at a 50-fold lower concentration of IVIG in mice treated intranasally with whole IVIG or F(ab)´2fragments compared with intravenous administration. In addition, another study showed that intranasal IVIG or F(ab)´2 fragments protected 90% of the mice from lethal nasal influenza (72). In another study, Farag and colleagues (73) infected mice with clinical isolates of MRSA Staphylococcus aureus via intraperitoneal injection, and the mice were treated with human IVIG. The mice that received intraperitoneal IVIG had an 80% survival rate, while the rate of survival was only 25% in mice that received intravenous IVIG. Therefore, taken together, these studies suggested that IVIG can be used at lower concentrations, and can be more effective when it is targeted more directly at the infection site, instead of by intravenous administration.
In humans, there is a risk of bacteremia during lung infection and intervention with mechanical ventilation. In murine models of mechanical ventilation and bacterial infection, animals that received human IVIG before Klebsiella pneumoniae (74) or Pseudomonas aeruginosa (65) challenge had significantly reduced lung infections and bacteremia. These studies suggest the potential use of IVIG to prevent bacteremia in humans during mechanical ventilation.
Other laboratory studies on distinct bacteria have shown the benefits of using only IVIG. Roy and colleagues (75) infected mice intraperitoneally (i.p.) with Mycobacterium tuberculosis, and then administered a high i.p. dose of human IVIG to the mice. The animals treated with IVIG had a lower bacterial load in the spleen and lungs than controls that received human serum albumin. Combination therapy using IVIG and antibiotics is another strategy to combat infections caused by drug-resistant bacteria and superbugs. In a murine model, mice were infected intranasally with Streptococcus pneumoniae, and were then treated with suboptimal concentrations of ampicillin plus suboptimal IVIG. Mice that received the combined treatment had a significantly higher survival rate and reduction in the bacterial CFU in their lungs and blood compared to those that received individual treatments (76). However, other studies with mice using antibiotics plus human IVIG did not find enhanced bacterial elimination of Staphylococcus aureus (77).
In a preclinical study, rabbits were infected with different isolates of Staphylococcus aureus that presented AMR and were treated with a 200 mg/kg dose of human IVIG, vancomycin or linezolid, or a combination of IVIG+linezolid or IVIG+vancomycin (78). Rabbits treated with IVIG+linezolid or IVIG+vancomycin had a higher survival rate and lower CFU in their lungs than animals that received individual treatments. The authors of the study demonstrated and concluded that human IVIG contains antibodies that neutralize the S. aureus toxins α-hemolysin (HIa) and Panton-Valentine leukocydin (PVL), which are responsible for causing necrotizing pneumonia, and may be used as an adjuvant therapy for human necrotizing pneumonia caused by MRSA.
Studies in humans using IVIG for microbial infections and infections with AMR
IVIG is used to provide antibodies to patients with PID to prevent infections (79, 80). IVIG has been shown to prevent infections in patients with transient or secondary immunodeficiency, such as transplant patients or cancer patients in radiation treatment, and with infectious diseases that do not respond to conventional therapy, including infections with AMR. Table 3 presents references for studies using IVIG as an adjuvant treatment in difficult-to-eliminate infections in animal models and humans.
Streptococcus pyogenes or Group A Streptococcus (GAS) is a gram-positive bacterium that causes infections, from benign ones, such as pharyngitis, to severe infections with bacteremia, necrosis and STSS (streptococcal toxin septic syndrome), and that may present with antibiotic resistance (97). In a case report, a 55-year-old man with a leg infection with Streptococcus pyogenes did not respond to antibiotics, developed STSS, and his leg was amputated because of severe necrosis. Immediately after surgery, the patient was treated with IVIG for 5 days, with fast improvement and negative Streptococcus culture from his blood. He was discharged from the hospital in good condition 25 days after his admission (84).
Patients hospitalized with GAS suffer from severe infections. Clindamycin improves the outcome, but fatal cases are frequent. In a prospective observational study with 84 patients with invasive and severe GAS infections (iGAS), the patients who received clindamycin had a death rate of 15%, while mortality was 39% in patients who did not receive clindamycin. The addition of IVIG to clindamycin treatment reduced the mortality rate to 7%, suggesting an additional benefit of using IVIG (91). In another observational cohort study, of 67 selected patients hospitalized with STSS, 23 received a combination of IVIG + clindamycin and/or penicillin, while 44 received only antibiotics (92). Mortality after 28 days was 7/23 (13%) in the IVIG group, contrasting with 22/44 (50%) in the control group, or conversely, 28-day survival was 87% vs. 50% for the IVIG and control groups, respectively. Accordingly, IVIG increases the probability of surviving STSS. GAS produces a variety of toxins that subvert the innate immune response (97), and IVIG may neutralize those toxins, enabling the normal immune response to eliminate bacteria.
Infections by Clostridium difficile produce colitis or diarrhea; they are frequently hospital-acquired and are related to the use of antibiotics. C. difficile produces toxins A and B, which cause severe inflammation, namely fluid secretion, and increased mucosal permeability, enteritis and colitis, and these toxins can be neutralized by antibodies contained in IVIG (80). IVIG improved the clinical outcome of child or adult patients with C. difficile infection who did not respond to antibiotics, eliminating diarrhea and bacterial toxins in stool (82, 85, 98–100). Therefore, patients suffering GAS or C. difficile infections who do not respond to antibiotics can benefit from IVIG treatment, possibly through the neutralization of toxins produced by these bacteria.
The polyvalent nature of IVIG permits the antibody recognition of different microbe families, and it can also be used in fungal or viral infections. Candida albicans is an opportunistic fungus that causes infections in patients with primary or secondary immunodeficiency and is sometimes difficult to eliminate. Two girls with primary immunodeficiency affecting IFN-γ and IL-17 production, and with mutations in their IL12RB1 and STAT1 genes, were treated for oral candidiasis with IVIG mouthwash. This topical IVIG treatment reduced infection in 12 days at a rate of greater than 95 and 70%, respectively; the patient with the STAT1 mutation had had chronic mucocutaneous candidiasis since she was 8 months old and severe oral candidiasis resistant to voriconazole. Fungal infections in patients were eliminated after complementary treatments with nystatin and caspofungin (86). Therefore, in that paper, we proposed that C. albicans is opsonized in the mouth by IVIG in the mouthwash, which may facilitate the phagocytosis of yeasts by mouth mucosal phagocytes; then, the killing of the fungi occurs by ROS-mediated mechanisms, which circumvents AMR.
IVIG has been tested in clinical trials on hospitalized patients under immunosuppressive interventions to prevent infections or as an adjuvant for infection treatment of sepsis and septic shock. In a comparative study of 150 patients with multiple traumas and mechanical ventilation, 76 patients received IVIG, and 74 received only the usual care. Pneumonia frequency and antibiotic use were lower in the IVIG group than in the control group (28 vs. 43%, p=0.06), but sepsis and mortality were similar in both groups (87). In another study with multiple trauma patients, 21 received IVIG plus penicillin, and 18 received only penicillin. The frequency of pneumonia- and non-catheter related infections was significantly lower in the IVIG group; however, hospital stay and infection-related mortality were similar in both groups (101). IVIG has also been used to prevent cytomegalovirus (CMV) infections in high-risk transplant patients, primarily in kidney transplantation. While some studies reported favorable results both in vitro (102) and in vivo (103), a meta-analysis study reviewing 18 clinical trials of treatments with IVIG to prevent CMV infections in transplant patients did not find protective evidence (96).
Standard commercial IVIG preparations contain mostly IgG and some traces of IgM and IgA; however, normal human plasma contains IgM and IgA in higher proportions, and IgM is the first isotype of the antibody synthesized during the natural immune response against microorganisms. IgM is a pentameric immunoglobulin that has a high capacity to neutralize toxins, including superantigens, and for agglutination and may also be associated with higher antimicrobial activity (104). The commercial preparation of IgM-enriched IVIG (M-IVIG), which is called pentaglobin, contains 38 g/L IgG, 6 g/L IgM and 6 g/L IgA, while standard IVIG contains more than 96% monomeric IgG, a low percentage of dimeric IgG and traces of IgM and IgA (93). Some studies on sepsis in humans have used M-IVIG instead of standard IVIG. In a one-center study on patients with gram-negative septic shock, 27 were treated with antibiotics plus M-IVIG, and 28 were treated only with antibiotics; the clinical evolution and overall lower mortality (1/27) at 80 days were more favorable for patients in the M-IVIG group than for those in the control group, in which mortality was 9/28 (88). Another multicenter randomized clinical trial (90) on patients with abdominal sepsis also found lower mortality (27.5%) in patients treated with antibiotics plus M-IVIG compared to the control group (48.1%) that received only antibiotics. A meta-analysis by Norrby-Teglund and colleagues on 10 clinical trials of adults with sepsis found favorable results with a mortality reduction in patients treated with the adjuvant use of M-IVIG compared to the usual treatment (O.R. = 0.35), and the results were better in patients with gram-negative sepsis (93). However, a meta-analysis that included 14 clinical trials with patients with severe sepsis or septic shock did not identify a mortality reduction in patients who received IVIG (94).
Alejandria and colleagues (95) published an extensive meta-analysis on studies using polyvalent IVIG to reduce mortality in neonate and adult patients with sepsis and septic shock. The analysis showed that in adults, the use of IVIG (n=1430) or M-IVIG (n=528) reduced mortality compared to non-intervention or a placebo. In contrast, there was no reduction in mortality for neonates with sepsis (n= 3667) treated with IVIG compared to the controls, and better results were observed in studies with M-IVIG, although with fewer patients (3 trials, n=164). The clinical trials on patients with sepsis who received anti-endotoxin antibodies (n=4671) or anti-cytokine monoclonal antibodies did not show significant beneficial effects. The authors highlighted the heterogeneity of the studies (clinical disease, interventions, outcome assessment, and quality of the studies). The sub-analysis of high-quality studies showed a reduction in the beneficial results of IVIG observed in the global analysis. Another disadvantage of using IVIG in sepsis or septic shock is that it must be used during the first stage of infection, and the utility of IVIG is more limited during advanced stages of infection.
In summary, studies on humans with infectious diseases using IVIG as only treatment or as an adjuvant, reported diverse and sometimes contrasting results. Because there is heterogeneity in distinct studies with patients, there is a need to identify the infections and conditions in which the use of IVIG is more promising, as an alternative or as a complementary treatment to avoid AMR.
Advantages and limitations of IVIG for infectious diseases: The option of immune immunoglobulins and the importance of topical treatments
The first and main advantage of using IVIG to treat infection is that the transfer of immunity is instantaneous. The second is the diversity of antibodies contained in IVIG, with millions of specificities able to neutralize quite diverse toxins, superantigens or to opsonize multiple microorganisms, and provide immunomodulatory properties. The third advantage is to avoid AMR. Thus, the transference of immunity by antibody treatments provides the host with premade effector antimicrobial weapons able to prevent infections or to help eliminate ongoing infections, even if they are caused by microbes with AMR.
Nevertheless, there is an intrinsic lot-to-lot variability in the antibodies present in IVIG that depends, in turn, on the microbes that the plasma donors face during natural infections or vaccination (105); consequently, this variability in the antibody specificities depends on the regions from which donors are selected (106–109). Therefore, a potential constraint for the specific use of IVIG may be that a specific preparation of IVIG does not contain enough titers of antibodies against the target microbe. To overcome this deficiency, the alternative is to obtain or produce immune or specific IgG, which contains high titers of the antibodies of interest. For example, the benefits of immune plasma therapy tested during the beginning of the COVID-19 pandemic were due to high titers of specific antibodies against SARS-CoV-2 coming from subjects who recently recovered from COVID-19 (110, 111). There are also some immune IgGs commercially available for specific needs, such as anti-tetanus, anti-B hepatitis, anti-rabies, anti-RSV or anti-RH (112). Immune immunoglobulins are also obtained through the immunization of human volunteers with specific antigens. Bhakdi and colleagues immunized human volunteers with S. aureus α-toxoid and obtained sera from donors to purify and pool the immune IgG. The immune IgG specific for the α-toxin of S. aureus showed protection in vitro against toxic effects on platelets and monocytes and protected cynomolgus monkeys from the toxic and lethal effects of α-toxin, while normal IVIG did not (113).
During the last century, at the Gamaleya Institute of Moscow, Dr. Simon Skurkovich developed extensive work using human anti-Staphylococcus plasma (ASP) and anti-Staphylococcus immunoglobulin (ASIG) for treating Staphylococcus infections. Dr. Skurkovich immunized human volunteers with S. aureus toxoid to obtain ASP and ASIG. The specific immunoglobulin ASIG was used in the USSR and some countries in Western Europe to treat distinct forms of staphylococcal disease, such as meningoencephalitis, pneumonia, osteomyelitis, and post-surgery infections, showing favorable results and a significant reduction in mortality (114). This approach may be clinically useful since staphylococcal infections cause high morbidity and mortality in humans, are frequently present in AMR, and there is no available vaccine for Staphylococcus (115). Unfortunately, most of the work by S. Skurkovich and his colleagues was published in Russian and was not known or followed in eastern countries. However, the favorable results obtained in the Soviet Union with ASIG need to be reproduced in clinical controlled studies.
Recently, Emile Jacque and colleagues (116) developed a novel strategy for the enrichment of specific anti-respiratory syncytial virus (RSV) antibodies obtained from human generic IVIG, that is, normal, non-immune sera. Specific antibodies for RSV were purified and enriched by column affinity using protein F from the virus capsid. The enriched anti-RSV Ig efficiently neutralized the virus in an in vitro assay with Hep-2 cells. Moreover, anti-RSV Ig given nasally to BALB/c mice inhibited the in vivo RSV infection of mice in the lungs and was three times more efficient at protection than Synagis®, a monoclonal antibody against RSV (116). Thus, specific anti-RSV Ig antibodies used locally and intranasally protected mice efficiently from infection. Other authors obtained enriched specific anti-Staphylococcus aureus, anti-Streptococcus pyogenes and anti-Enterococcus, using vancomycin-resistant strains, from normal IVIG, and the enriched antibodies were able to neutralize bacteria two to four times more efficiently than normal IVIG and increase phagocytosis by neutrophils (117).
Lastly, giving antibody treatments locally instead of systemically as intravenous injections made the antibodies available in situ for the infections, and this approach reduced the concentration of antibodies necessary to give an effector function and to control an infection. The concept of local or topical use of IVIG or specific antibodies has been experimentally tested for infections associated with burns (118–121), peritonitis (122, 123), oral (86), and respiratory infections (71, 72, 76, 124), including SARS-CoV-2 neutralization with an inhaled single-dose of chain fragment variable (small human antibodies) to prevent lung infection (125). In general, the results of the cited papers showed that local delivery of IVIG or specific IgG prevented dissemination of infections to distinct organs and reduced mortality in animal models. The additional benefit of giving IgG at the site of infection or near the infected tissue or organ was the lower concentration of antibodies needed to reach a biological and therapeutic effect. This concept was well illustrated in the experiments by Ramisse and colleagues: the control of Streptococcus pneumoniae nasal infection in mice was reached with 50 lower doses of human IVIG administered nasally compared with IVIG given intraperitoneally (71).
Concluding remarks
The use of IVIG in experimental models and in some human diseases has shown that the antibodies contained in these pools of IgG have the potential to aid in controlling and/or eliminating infections, even when the causal agent exhibited AMR. This potential of IVIG relies on its ability to facilitate, enhance, and modulate mechanisms of the immune response, eliminating microbes and controlling inflammation.
The challenge in optimizing the use of IVIG to fight global AMR consists, firstly, in identifying infections that are more likely to respond to these therapies. Secondly, in using antibodies that are more specific for the infectious agent, and the use of antibodies combined with antibiotics that will increase its efficacy. Finally, finding mechanisms to apply and deliver IVIG at the site of infection has the potential to increase the probability of therapy success and to reduce the amount of IVIG needed to achieve its beneficial effects.
Author contributions
SP-S: contribution for idea, literature review and draft and edit writing. AC-G: data curation, literature review and draft writing. OP-R, data curation, draft writing and statistical assistance. JG-R, literature review and contribution on clinical aspects; JB, data curation, literature analysis, and manuscript writing and editing; MT, original idea, data curation, resources, supervision, writing and final edits. All authors contributed to the article and approved the submitted version.
Funding
This work was supported by the normal budget from the Unit of Biochemistry at the Instituto Nacional de Ciencias Médicas y Nutrición Salvador Zubirán, INCMNSZ and the Instituto Nacional de Enfermedades Respiratorias Ismael Cosío Villegas, INER.
Acknowledgments
We thank Dr. Alberto Huberman from Instituto Nacional de Ciencias Médicas y Nutrición Salvador Zubirán for his critical reading of the manuscript and for his valuable suggestions.
Conflict of interest
The authors declare that the research was conducted in the absence of any commercial or financial relationships that could be construed as a potential conflict of interest.
Publisher’s note
All claims expressed in this article are solely those of the authors and do not necessarily represent those of their affiliated organizations, or those of the publisher, the editors and the reviewers. Any product that may be evaluated in this article, or claim that may be made by its manufacturer, is not guaranteed or endorsed by the publisher.
Abbreviations
AM, Antimicrobial; AMR, AM resistance; AMP, Antimicrobial peptides; ASIG, Anti-Staphylococcus immunoglobulin; ASP, Anti-Staphylococcus plasma CFU, colony forming units; COVID-19, Coronavirus disease 2019; GAS, group A Streptococcus; ESBL, Extended spectrum beta lactamase; IVIG, Polyvalent human immunoglobulin G; M-IVIG, IgM-enriched human immunoglobulin; i.p., Intraperitoneal; i.v., Intravenous; i.n., Intranasal; ITP, idiopathic thrombocytopenic purpura; MAC, membrane attack complex; MNC, Mononuclear leukocytes; MRSA, Methicillin-resistant Staphylococcus aureus; NK, Natural Killer cells; O.R., Odds ratio; PMN, Polymorphonuclear leukocytes; PID, primary immunodeficiency; RCT, Randomized clinical trial; ROS, reactive oxygen species; SC, subcutaneous; SLE, systemic lupus erythematosus; TSSS, Toxoid-specific Staphylococcus shock; VRSA, Vancomycin-resistant Staphylococcus aureus.
References
1. Tan SY, Tatsumura Y. Alexander Fleming (1881-1955): Discoverer of penicillin. Singapore Med J (2015) 56(7):366–7. doi: 10.11622/smedj.2015105
2. Aminov RI. A brief history of the antibiotic era: lessons learned and challenges for the future. Front Microbiol (2010) 134:134. doi: 10.3389/fmicb.2010.00134
3. Robbins N, Wright GD, Cowen LE. Antifungal drugs: The current armamentarium and development of new agents. Microbiol Spectr (2016) 4(5):1–20. doi: 10.1128/microbiolspec.FUNK-0002-2016
4. Zaman SB, Hussain MA, Nye R, Mehta V, Mamun KT, Hossain N. A review on antibiotic resistance: Alarm bells are ringing. Cureus (2017) 9(6):e1403. doi: 10.7759/cureus.1403
5. De Oliveira DM, Forde BM, Kidd TJ, Harris PN, Schembri MA, Beatson SA, et al. Antimicrobial resistance in ESKAPE pathogens. Clin Microbiol Rev (2020) 33(3):e00181–19. doi: 10.1128/CMR.00181-19
6. Doi Y. Treatment options for carbapenem-resistant gram-negative bacterial infections. Clin Infect Dis (2019) 69(Suppl 7):S565–75. doi: 10.1093/cid/ciz830
7. Cherazard R, Epstein M, Doan TL, Salim T, Bharti S, Smith MA. Antimicrobial resistant streptococcus pneumoniae: Prevalence, mechanisms, and clinical implications. Am J Ther (2017) 24(3):e361–9. doi: 10.1097/MJT.0000000000000551
8. Hakenbeck R, Bruckner R, Denapaite D, Maurer P. Molecular mechanisms of beta-lactam resistance in streptococcus pneumoniae. Future Microbiol (2012) 7(3):395–410. doi: 10.2217/fmb.12.2
9. Gagliotti C, Balode A, Baquero F, Degener J, Grundmann H, Gur D, et al. Participants: Escherichia coli and staphylococcus aureus: bad news and good news from the European antimicrobial resistance surveillance network (EARS-net, formerly EARSS), 2002 to 2009. Euro Surveill (2011) 16(11):1–5. doi: 10.2807/ese.16.11.19819-en
10. Lee CR, Lee JH, Park KS, Jeon JH, Kim YB, Cha CJ, et al. Antimicrobial resistance of hypervirulent klebsiella pneumoniae: Epidemiology, hypervirulence-associated determinants, and resistance mechanisms. Front Cell Infect Microbiol (2017) 7:483. doi: 10.3389/fcimb.2017.00483
11. Bassetti M, Righi E, Carnelutti A, Graziano E, Russo A. Multidrug-resistant klebsiella pneumoniae: challenges for treatment, prevention and infection control. Expert Rev Anti Infect Ther (2018) 16(10):749–61. doi: 10.1080/14787210.2018.1522249
12. Hawser SP, Bouchillon SK, Lascols C, Hackel M, Hoban DJ, Badal RE, et al. Susceptibility of European escherichia coli clinical isolates from intra-abdominal infections, extended-spectrum beta-lactamase occurrence, resistance distribution, and molecular characterization of ertapenem-resistant isolates (SMART 2008-2009). Clin Microbiol Infect (2012) 18(3):253–9. doi: 10.1111/j.1469-0691.2011.03550.x
13. Banawas SS. Clostridium difficile infections: A global overview of drug sensitivity and resistance mechanisms. BioMed Res Int (2018) 2018:8414257. doi: 10.1155/2018/8414257
14. Lange C, Chesov D, Heyckendorf J, Leung CC, Udwadia Z, Dheda K. Drug-resistant tuberculosis: An update on disease burden, diagnosis and treatment. Respirology (2018) 23(7):656–73. doi: 10.1111/resp.13304
15. Lone SA, Ahmad A. Candida auris-the growing menace to global health. Mycoses (2019) 62(8):620–37. doi: 10.1111/myc.12904
16. World Health Organization. WHO publishes list of bacteria for which new antibiotics are urgently needed. (2017) WHO.Available at: https://www.who.int/news/item/27-02-2017-who-publishes-list-of-bacteria-for-which-new-antibiotics-are-urgently-needed.
17. Lockhart SR, Etienne KA, Vallabhaneni S, Farooqi J, Chowdhary A, Govender NP, et al. Simultaneous emergence of multidrug-resistant candida auris on 3 continents confirmed by whole-genome sequencing and epidemiological analyses. Clin Infect Dis (2017) 64(2):134–40. doi: 10.1093/cid/ciw691
18. Rudramurthy SM, Chakrabarti A, Paul RA, Sood P, Kaur H, Capoor MR, et al. Candida auris candidaemia in Indian ICUs: analysis of risk factors. J Antimicrob Chemother (2017) 72(6):1794–801. doi: 10.1093/jac/dkx034
19. Schelenz S, Hagen F, Rhodes JL, Abdolrasouli A, Chowdhary A, Hall A, et al. First hospital outbreak of the globally emerging candida auris in a European hospital. Antimicrob Resist Infect Control (2016) 5:1–7. doi: 10.1186/s13756-016-0132-5
20. Perlin DS, Rautemaa-Richardson R, Alastruey-Izquierdo A. The global problem of antifungal resistance: prevalence, mechanisms, and management. Lancet Infect Dis (2017) 17(12):e383–92. doi: 10.1016/S1473-3099(17)30316-X
21. Aslam B, Wang W, Arshad MI, Khurshid M, Muzammil S, Rasool MH, et al. Antibiotic resistance: a rundown of a global crisis. Infect Drug Resist (2018) 11:1645–58. doi: 10.2147/IDR.S173867
22. Kumar M, Sarma DK, Shubham S, Kumawat M, Verma V, Nina PB, et al. Futuristic non-antibiotic therapies to combat antibiotic resistance: A review. Front Microbiol (2021) 12:609459. doi: 10.3389/fmicb.2021.609459
23. Antimicrobial Resistance C. Global burden of bacterial antimicrobial resistance in 2019: a systematic analysis. Lancet (2022) 399(10325):629–55. doi: 10.1016/S0140-6736(21)02724-0
24. Carlet J, Jarlier V, Harbarth S, Voss A, Goossens H, Pittet D, et al. Participants of the 3rd world healthcare-associated infections: Ready for a world without antibiotics? the pensieres antibiotic resistance call to action. Antimicrob Resist Infect Control (2012) 1(1):11. doi: 10.1186/2047-2994-1-11
25. Gajdacs M. The concept of an ideal antibiotic: Implications for drug design. Molecules (2019) 24(5):1–16. doi: 10.3390/molecules24050892
26. Alvarez-Martinez FJ, Barrajon-Catalan E, Herranz-Lopez M, Micol V. Antibacterial plant compounds, extracts and essential oils: An updated review on their effects and putative mechanisms of action. Phytomedicine (2021) 90:153626. doi: 10.1016/j.phymed.2021.153626
27. Cha Y, Erez T, Reynolds IJ, Kumar D, Ross J, Koytiger G, et al. Drug repurposing from the perspective of pharmaceutical companies. Br J Pharmacol (2018) 175(2):168–80. doi: 10.1111/bph.13798
28. Challa AP, Lavieri RR, Lewis JT, Zaleski NM, Shirey-Rice JK, Harris PA, et al. Systematically prioritizing candidates in genome-based drug repurposing. Assay Drug Dev Technol (2019) 17(8):352–63. doi: 10.1089/adt.2019.950
29. Wittebole X, De Roock S, Opal SM. A historical overview of bacteriophage therapy as an alternative to antibiotics for the treatment of bacterial pathogens. Virulence (2014) 5(1):226–35. doi: 10.4161/viru.25991
30. Chan BK, Turner PE, Kim S, Mojibian HR, Elefteriades JA, Narayan D. Phage treatment of an aortic graft infected with pseudomonas aeruginosa. Evol Med Public Health (2018) 2018(1):60–6. doi: 10.1093/emph/eoy005
31. Duplessis C, Biswas B, Hanisch B, Perkins M, Henry M, Quinones J, et al. Refractory pseudomonas bacteremia in a 2-Year-Old sterilized by bacteriophage therapy. J Pediatr Infect Dis Soc (2018) 7(3):253–6. doi: 10.1093/jpids/pix056
32. Sarker SA, Sultana S, Reuteler G, Moine D, Descombes P, Charton F, et al. Oral phage therapy of acute bacterial diarrhea with two coliphage preparations: A randomized trial in children from Bangladesh. EBioMedicine (2016) 4:124–37. doi: 10.1016/j.ebiom.2015.12.023
33. Kortright KE, Chan BK, Koff JL, Turner PE. Phage therapy: A renewed approach to combat antibiotic-resistant bacteria. Cell Host Microbe (2019) 25(2):219–32. doi: 10.1016/j.chom.2019.01.014
34. Lei J, Sun L, Huang S, Zhu C, Li P, He J, et al. The antimicrobial peptides and their potential clinical applications. Am J Transl Res (2019) 11(7):3919–31.
35. Rosini R, Nicchi S, Pizza M, Rappuoli R. Vaccines against antimicrobial resistance. Front Immunol (2020) 11:1048. doi: 10.3389/fimmu.2020.01048
36. Hrvatin V. Combating antibiotic resistance: New drugs or alternative therapies? CMAJ (2017) 189(37):E1199. doi: 10.1503/cmaj.109-5469
37. Cherin P, Cabane J. Relevant criteria for selecting an intravenous immunoglobulin preparation for clinical use. BioDrugs (2010) 24(4):211–23. doi: 10.2165/11537660-000000000-00000
38. Spath PJ, Schneider C, von Gunten S. Clinical use and therapeutic potential of IVIG/SCIG, plasma-derived IgA or IgM, and other alternative immunoglobulin preparations. Arch Immunol Ther Exp (Warsz) (2017) 65(3):215–31. doi: 10.1007/s00005-016-0422-x
39. Ballow M. Safety of IGIV therapy and infusion-related adverse events. Immunol Res (2007) 38(1-3), 122–32. doi: 10.1007/s12026-007-0003-5
40. Bellanti JA, Galvez Romero J. [Clinical directions for human immunoglobulin in primmary immunodeficiencies] indicaciones clínicas de la inmunoglobulina humana en inmunodeficiencias primarias. In: B JA, Méndez-León JI, Leyva-Rendón A, Majluf-Cruz A, editors. Inmunoglobulina humana. tratamiento en inmunodeficiencias, autoinmunidad, inflamación y COVID-19. (Ergon, Mexico) (2022).
41. Levy Y, Sherer Y, Ahmed A, Langevitz P, George J, Fabbrizzi F, et al. A study of 20 SLE patients with intravenous immunoglobulin–clinical and serologic response. Lupus (1999) 8(9):705–12. doi: 10.1191/096120399678841007
42. Nacci F, Righi A, Conforti ML, Miniati I, Fiori G, Martinovic D, et al. Intravenous immunoglobulins improve the function and ameliorate joint involvement in systemic sclerosis: a pilot study. Ann Rheum Dis (2007) 66(7):977–9. doi: 10.1136/ard.2006.060111
43. Saito E, Koike T, Hashimoto H, Miyasaka N, Ikeda Y, Hara M, et al. Efficacy of high-dose intravenous immunoglobulin therapy in Japanese patients with steroid-resistant polymyositis and dermatomyositis. Mod Rheumatol (2008) 18(1):34–44. doi: 10.1007/s10165-007-0013-0
44. Wong PH, White KM. Impact of immunoglobulin therapy in pediatric disease: a review of immune mechanisms. Clin Rev Allergy Immunol (2016) 51(3):303–14. doi: 10.1007/s12016-015-8499-2
45. Bousfiha A, Jeddane L, Picard C, Al-Herz W, Ailal F, Chatila T, et al. Human inborn errors of immunity: 2019 update of the IUIS phenotypical classification. J Clin Immunol (2020) 40(1):66–81. doi: 10.1007/s10875-020-00758-x
46. Lucas M, Lee M, Lortan J, Lopez-Granados E, Misbah S, Chapel H. Infection outcomes in patients with common variable immunodeficiency disorders: relationship to immunoglobulin therapy over 22 years. J Allergy Clin Immunol (2010) 125(6):1354–1360 e4. doi: 10.1016/j.jaci.2010.02.040
47. Orange JS, Grossman WJ, Navickis RJ, Wilkes MM. Impact of trough IgG on pneumonia incidence in primary immunodeficiency: A meta-analysis of clinical studies. Clin Immunol (2010) 137(1):21–30. doi: 10.1016/j.clim.2010.06.012
48. Shapiro RS, Wasserman RL, Bonagura V, Gupta S. Emerging paradigm of primary immunodeficiency disease: Individualizing immunoglobulin dose and delivery to enhance outcomes. J Clin Immunol (2017) 37(2):190–6. doi: 10.1007/s10875-014-9990-x
49. Jarczak D, Kluge S, Nierhaus A. Use of intravenous immunoglobulins in sepsis therapy-a clinical view. Int J Mol Sci (2020) 21(15):1–17. doi: 10.3390/ijms21155543
50. Norrby-Teglund A, Ihendyane N, Kansal R, Basma H, Kotb M, Andersson J, et al. Relative neutralizing activity in polyspecific IgM, IgA, and IgG preparations against group a streptococcal superantigens. Clin Infect Dis (2000) 31(5):1175–82. doi: 10.1086/317423
51. Winter LE, Barenkamp SJ. Human antibodies specific for the high-molecular-weight adhesion proteins of nontypeable haemophilus influenzae mediate opsonophagocytic activity. Infect Immun (2003) 71(12):6884–91. doi: 10.1128/IAI.71.12.6884-6891.2003
52. Bayry J, Lacroix-Desmazes S, Kazatchkine MD, Kaveri SV. Intravenous immunoglobulin for infectious diseases: back to the pre-antibiotic and passive prophylaxis era? Trends Pharmacol Sci (2004) 25(6):306–10. doi: 10.1016/j.tips.2004.04.002
53. Yanagisawa C, Hanaki H, Natae T, Sunakawa K. Neutralization of staphylococcal exotoxins in vitro by human-origin intravenous immunoglobulin. J Infect Chemother (2007) 13(6):368–72. doi: 10.1007/s10156-007-0551-6
54. Yajima M, Shiraki A, Daikoku T, Oyama Y, Yoshida Y, Shiraki K. Functional differences between antiviral activities of sulfonated and intact intravenous immunoglobulin preparations toward varicella-zoster virus and cytomegalovirus. J Infect Chemother (2015) 21(6):427–33. doi: 10.1016/j.jiac.2015.01.012
55. Basta M, Dalakas MC. High-dose intravenous immunoglobulin exerts its beneficial effect in patients with dermatomyositis by blocking endomysial deposition of activated complement fragments. J Clin Invest (1994) 94(5):1729–35. doi: 10.1172/JCI117520
56. Basta M, Van Goor F, Luccioli S, Billings EM, Vortmeyer AO, Baranyi L, et al. F(ab)'2-mediated neutralization of C3a and C5a anaphylatoxins: a novel effector function of immunoglobulins. Nat Med (2003) 9(4):431–8. doi: 10.1038/nm836
57. Barahona Afonso AF, Joao CM. The production processes and biological effects of intravenous immunoglobulin. Biomolecules (2016) 6(1):15. doi: 10.3390/biom6010015
58. Wasserman RL, Garcia D, Greener BN, Kestenberg K, Pinkert A, Mond J, et al. Manufacturing process optimization of ADMA biologics' intravenous immunoglobulin products, BIVIGAM((R)) and ASCENIV. Immunotherapy (2019) 11(16):1423–33. doi: 10.2217/imt-2019-0157
59. Galeotti C, Kaveri SV, Bayry J. IVIG-mediated effector functions in autoimmune and inflammatory diseases. Int Immunol (2017) 29(11):491–8. doi: 10.1093/intimm/dxx039
60. Rojas M, Rodriguez Y, Monsalve DM, Acosta-Ampudia Y, Camacho B, Gallo JE, et al. Convalescent plasma in covid-19: Possible mechanisms of action. Autoimmun Rev (2020) 19(7):102554. doi: 10.1016/j.autrev.2020.102554
61. Shock A, Humphreys D, Nimmerjahn F. Dissecting the mechanism of action of intravenous immunoglobulin in human autoimmune disease: Lessons from therapeutic modalities targeting fcgamma receptors. J Allergy Clin Immunol (2020) 146(3):492–500. doi: 10.1016/j.jaci.2020.06.036
62. Tha-In T, Bayry J, Metselaar HJ, Kaveri SV, Kwekkeboom J. Modulation of the cellular immune system by intravenous immunoglobulin. Trends Immunol (2008) 29(12):608–15. doi: 10.1016/j.it.2008.08.004
63. Lu LL, Suscovich TJ, Fortune SM, Alter G. Beyond binding: antibody effector functions in infectious diseases. Nat Rev Immunol (2018) 18(1):46–61. doi: 10.1038/nri.2017.106
64. Schwab I, Nimmerjahn F. Intravenous immunoglobulin therapy: how does IgG modulate the immune system? Nat Rev Immunol (2013) 13(3):176–89. doi: 10.1038/nri3401
65. Katoh H, Yasumoto H, Shimizu M, Hamaoka S, Kinoshita M, Akiyama K, et al. IV immunoglobulin for acute lung injury and bacteremia in pseudomonas aeruginosa pneumonia. Crit Care Med (2016) 44(1):e12–24. doi: 10.1097/CCM.0000000000001271
66. Sawa T, Kinoshita M, Inoue K, Ohara J, Moriyama K. Immunoglobulin for treating bacterial infections: One more mechanism of action. Antibodies (Basel) (2019) 8(4):1–13. doi: 10.3390/antib8040052
67. Ono Y, Ito T, Watanabe T, Koshio O, Tansho S, Ikeda T, et al. Opsonic activity assessment of human intravenous immunoglobulin preparations against drug-resistant bacteria. J Infect Chemother (2004) 10(4):234–8. doi: 10.1007/s10156-004-0325-3
68. Wenisch C, Parschalk B, Patruta S, Brustbauer R, Graninger W. Effect of polyclonal immunoglobulins on neutrophil phagocytic capacity and reactive oxygen production in patients with gram-negative septicemia. Infection (1999) 27(3):183–6. doi: 10.1007/BF02561525
69. Itoh H, Matsuo H, Kitamura N, Yamamoto S, Higuchi T, Takematsu H, et al. Enhancement of neutrophil autophagy by an IVIG preparation against multidrug-resistant bacteria as well as drug-sensitive strains. J Leukoc Biol (2015) 98(1):107–17. doi: 10.1189/jlb.4A0813-422RRR
70. Matsuo H, Itoh H, Kitamura N, Kamikubo Y, Higuchi T, Shiga S, et al. Intravenous immunoglobulin enhances the killing activity and autophagy of neutrophils isolated from immunocompromised patients against multidrug-resistant bacteria. Biochem Biophys Res Commun (2015) 464(1):94–9. doi: 10.1016/j.bbrc.2015.06.004
71. Ramisse F, Binder P, Szatanik M, Alonso JM. Passive and active immunotherapy for experimental pneumococcal pneumonia by polyvalent human immunoglobulin or F(ab')2 fragments administered intranasally. J Infect Dis (1996) 173(5):1123–8. doi: 10.1093/infdis/173.5.1123
72. Ramisse F, Deramoudt FX, Szatanik M, Bianchi A, Binder P, Hannoun C, et al. Effective prophylaxis of influenza a virus pneumonia in mice by topical passive immunotherapy with polyvalent human immunoglobulins or F(ab')2 fragments. Clin Exp Immunol (1998) 111(3):583–7. doi: 10.1046/j.1365-2249.1998.00538.x
73. Farag N, Mahran L, Abou-Aisha K, El-Azizi M. Assessment of the efficacy of polyclonal intravenous immunoglobulin G (IVIG) against the infectivity of clinical isolates of methicillin-resistant staphylococcus aureus (MRSA) in vitro and in vivo. Eur J Clin Microbiol Infect Dis (2013) 32(9):1149–60. doi: 10.1007/s10096-013-1861-5
74. Lachmann RA, van Kaam AH, Haitsma JJ, Verbrugge SJ, Delreu F, Lachmann B. Immunoglobulin m-enriched intravenous polyclonal immunoglobulins reduce bacteremia following klebsiella pneumoniae infection in an acute respiratory distress syndrome rat model. Exp Lung Res (2004) 30(4):251–60. doi: 10.1080/01902140490439013
75. Roy E, Stavropoulos E, Brennan J, Coade S, Grigorieva E, Walker B, et al. Therapeutic efficacy of high-dose intravenous immunoglobulin in mycobacterium tuberculosis infection in mice. Infect Immun (2005) 73(9):6101–9. doi: 10.1128/IAI.73.9.6101-6109.2005
76. De Hennezel L, Ramisse F, Binder P, Marchal G, Alonso JM. Effective combination therapy for invasive pneumococcal pneumonia with ampicillin and intravenous immunoglobulins in a mouse model. Antimicrob Agents Chemother (2001) 45(1):316–8. doi: 10.1128/AAC.45.1.316-318.2001
77. Sallam MM, Abou-Aisha K, El-Azizi M. A novel combination approach of human polyclonal IVIG and antibiotics against multidrug-resistant gram-positive bacteria. Infect Drug Resist (2016) 9:301–11. doi: 10.2147/IDR.S120227
78. Diep BA, Le VT, Badiou C, Le HN, Pinheiro MG, Duong AH, et al. IVIG-mediated protection against necrotizing pneumonia caused by MRSA. Sci Transl Med (2016) 8(357):357ra124. doi: 10.1126/scitranslmed.aag1153
79. Jolles S, Sewell WA, Misbah SA. Clinical uses of intravenous immunoglobulin. Clin Exp Immunol (2005) 142(1):1–11. doi: 10.1111/j.1365-2249.2005.02834.x
80. Shah PJ, Vakil N, Kabakov A. Role of intravenous immune globulin in streptococcal toxic shock syndrome and clostridium difficile infection. Am J Health Syst Pharm (2015) 72(12):1013–9. doi: 10.2146/ajhp140359
81. Rockman S, Lowther S, Camuglia S, Vandenberg K, Taylor S, Fabri L, et al. Intravenous immunoglobulin protects against severe pandemic influenza infection. EBioMedicine (2017) 19:119–27. doi: 10.1016/j.ebiom.2017.04.010
82. Hassoun A, Ibrahim F. Use of intravenous immunoglobulin for the treatment of severe clostridium difficile colitis. Am J Geriatr Pharmacother (2007) 5(1):48–51. doi: 10.1016/j.amjopharm.2007.03.001
83. Arkilo D, Pierce B, Ritter F, Doescher JS, Frost M. Diverse seizure presentation of acute mycoplasma pneumoniae encephalitis resolving with immunotherapy. J Child Neurol (2014) 29(4):564–6. doi: 10.1177/0883073813480242
84. Lamothe F, D'Amico P, Ghosn P, Tremblay C, Braidy J, Patenaude JV. Clinical usefulness of intravenous human immunoglobulins in invasive group a streptococcal infections: case report and review. Clin Infect Dis (1995) 21(6):1469–70. doi: 10.1093/clinids/21.6.1469
85. Murphy C, Vernon M, Cullen M. Intravenous immunoglobulin for resistant clostridium difficile infection. Age Ageing (2006) 35(1):85–6. doi: 10.1093/ageing/afi212
86. Pedraza-Sanchez S, Mendez-Leon JI, Gonzalez Y, Ventura-Ayala ML, Herrera MT, Lezana-Fernandez JL, et al. Oral administration of human polyvalent IgG by mouthwash as an adjunctive treatment of chronic oral candidiasis. Front Immunol (2018) 9:2956. doi: 10.3389/fimmu.2018.02956
87. Glinz W, Grob PJ, Nydegger UE, Ricklin T, Stamm F, Stoffel D, et al. Polyvalent immunoglobulins for prophylaxis of bacterial infections in patients following multiple trauma. A randomized placebo-controlled study. Intensive Care Med (1985) 11(6):288–94. doi: 10.1007/BF00273538
88. Schedel I, Dreikhausen U, Nentwig B, Hockenschnieder M, Rauthmann D, Balikcioglu S, et al. Treatment of gram-negative septic shock with an immunoglobulin preparation: a prospective, randomized clinical trial. Crit Care Med (1991) 19(9):1104–13. doi: 10.1097/00003246-199109000-00003
89. Darenberg J, Ihendyane N, Sjolin J, Aufwerber E, Haidl S, Follin P, et al. Intravenous immunoglobulin G therapy in streptococcal toxic shock syndrome: a European randomized, double-blind, placebo-controlled trial. Clin Infect Dis (2003) 37(3):333–40. doi: 10.1086/376630
90. Rodriguez A, Rello J, Neira J, Maskin B, Ceraso D, Vasta L, et al. Effects of high-dose of intravenous immunoglobulin and antibiotics on survival for severe sepsis undergoing surgery. Shock (2005) 23(4):298–304. doi: 10.1097/01.shk.0000157302.69125.f8
91. Carapetis JR, Jacoby P, Carville K, Ang SJ, Curtis N, Andrews R. Effectiveness of clindamycin and intravenous immunoglobulin, and risk of disease in contacts, in invasive group a streptococcal infections. Clin Infect Dis (2014) 59(3):358–65. doi: 10.1093/cid/ciu304
92. Linner A, Darenberg J, Sjolin J, Henriques-Normark B, Norrby-Teglund A. Clinical efficacy of polyspecific intravenous immunoglobulin therapy in patients with streptococcal toxic shock syndrome: a comparative observational study. Clin Infect Dis (2014) 59(6):851–7. doi: 10.1093/cid/ciu449
93. Norrby-Teglund A, Haque KN, Hammarstrom L. Intravenous polyclonal IgM-enriched immunoglobulin therapy in sepsis: a review of clinical efficacy in relation to microbiological aetiology and severity of sepsis. J Intern Med (2006) 260(6):509–16. doi: 10.1111/j.1365-2796.2006.01726.x
94. Laupland KB, Kirkpatrick AW, Delaney A. Polyclonal intravenous immunoglobulin for the treatment of severe sepsis and septic shock in critically ill adults: a systematic review and meta-analysis. Crit Care Med (2007) 35(12):2686–92.
95. Alejandria MM, Lansang MA, Dans LF, Mantaring JB. 3rd: Intravenous immunoglobulin for treating sepsis, severe sepsis and septic shock. Cochrane Database Syst Rev (2013) 9):CD001090. doi: 10.1002/14651858.CD001090.pub2
96. Bourassa-Blanchette S, Patel V, Knoll GA, Hutton B, Fergusson N, Bennett A, et al. Clinical outcomes of polyvalent immunoglobulin use in solid organ transplant recipients: A systematic review and meta-analysis - part II: Non-kidney transplant. Clin Transplant (2019) 33(7):e13625. doi: 10.1111/ctr.13625
97. Walker MJ, Barnett TC, McArthur JD, Cole JN, Gillen CM, Henningham A, et al. Disease manifestations and pathogenic mechanisms of group a streptococcus. Clin Microbiol Rev (2014) 27(2):264–301. doi: 10.1128/CMR.00101-13
98. Leung DY, Kelly CP, Boguniewicz M, Pothoulakis C, LaMont JT, Flores A. Treatment with intravenously administered gamma globulin of chronic relapsing colitis induced by clostridium difficile toxin. J Pediatr (1991) 118(4 Pt 1):633–7. doi: 10.1016/s0022-3476(05)83393-1
99. Abdulaziz S, Abou-Shala N, Al-Tarifi A, Amin R. Salvage immunotherapy for fulminant pseudomembranous colitis. BMJ Case Rep (2013) 2013:1–2. doi: 10.1136/bcr-2013-008786
100. Beales IL. Intravenous immunoglobulin for recurrent clostridium difficile diarrhoea. Gut (2002) 51(3):456. doi: 10.1136/gut.51.3.456
101. Douzinas EE, Pitaridis MT, Louris G, Andrianakis I, Katsouyanni K, Karmpaliotis D, et al. Prevention of infection in multiple trauma patients by high-dose intravenous immunoglobulins. Crit Care Med (2000) 28(1):8–15. doi: 10.1097/00003246-200001000-00002
102. Frenzel K, Ganepola S, Michel D, Thiel E, Kruger DH, Uharek L, et al. Antiviral function and efficacy of polyvalent immunoglobulin products against CMV isolates in different human cell lines. Med Microbiol Immunol (2012) 201(3):277–86. doi: 10.1007/s00430-012-0229-2
103. Leroy F, Sechet A, Abou Ayache R, Thierry A, Belmouaz S, Desport E, et al. Cytomegalovirus prophylaxis with intravenous polyvalent immunoglobulin in high-risk renal transplant recipients. Transplant Proc (2006) 38(7):2324–6. doi: 10.1016/j.transproceed.2006.07.001
104. Haque KN, Zaidi MH, Bahakim H. IgM-enriched intravenous immunoglobulin therapy in neonatal sepsis. Am J Dis Child (1988) 142(12):1293–6. doi: 10.1001/archpedi.1988.02150120047038
105. Serra A, Marzo N, Pons B, Maduell P, Lopez M, Grancha S. Characterization of antibodies in human immunoglobulin products from different regions worldwide. Int J Infect Dis (2021) 104:610–6. doi: 10.1016/j.ijid.2021.01.034
106. Audet S, Virata-Theimer ML, Beeler JA, Scott DE, Frazier DJ, Mikolajczyk MG, et al. Measles-virus-neutralizing antibodies in intravenous immunoglobulins. J Infect Dis (2006) 194(6):781–9. doi: 10.1086/506363
107. Goldacker S, Witte T, Huzly D, Schlesier M, Peter HH, Warnatz K. Analysis of specific IgG titers against tick-borne encephalitis in patients with primary antibody deficiency under immunoglobulin substitution therapy: impact of plasma donor origin. Front Immunol (2014) 5:675. doi: 10.3389/fimmu.2014.00675
108. Planitzer CB, Farcet MR, Schiff RI, Ochs HD, Kreil TR. Neutralization of different echovirus serotypes by individual lots of intravenous immunoglobulin. J Med Virol (2011) 83(2):305–10. doi: 10.1002/jmv.21980
109. Rabel PO, Planitzer CB, Farcet MR, Kreil TR. Tick-borne encephalitis virus-neutralizing antibodies in different immunoglobulin preparations. Clin Vaccine Immunol (2012) 19(4):623–5. doi: 10.1128/CVI.05705-11
110. Libster R, Perez Marc G, Wappner D, Coviello S, Bianchi A, Braem V, et al. Early high-titer plasma therapy to prevent severe covid-19 in older adults. N Engl J Med (2021) 384(7):610–8. doi: 10.1056/NEJMoa2033700
111. Wenjing L, Yuanzheng F, Li JY, Tang LV, Yu H. Safety and efficacy of convalescent plasma therapy in severely and critically ill patients with COVID-19: a systematic review with meta-analysis. Aging (Albany NY) (2020) 13(1):1498–509. doi: 10.18632/aging.202195
112. Bernal C, Joda R, Montoro J. Bruno: [An update in hemoderivatives] hemoderivados: actualización (article in Spanish). (Universidad de Barcelona) (2002). http://www.ub.edu/legmh/curs.htm
113. Bhakdi S, Mannhardt U, Muhly M, Hugo F, Ronneberger H, Hungerer KD. Human hyperimmune globulin protects against the cytotoxic action of staphylococcal alpha-toxin in vitro and in vivo. Infect Immun (1989) 57(10):3214–20. doi: 10.1128/iai.57.10.3214-3220.1989
114. Kelly J. Immunotherapy against antibiotic-resistant bacteria: the Russian experience with an antistaphylococcal hyperimmune plasmaand immunoglobulin. Microbes Infect (2000) 2(11):1383–92. doi: 10.1016/s1286-4579(00)01292-2
115. Cheung GYC, Bae JS, Otto M. Pathogenicity and virulence of staphylococcus aureus. Virulence (2021) 12(1):547–69. doi: 10.1080/21505594.2021.1878688
116. Jacque E, Chottin C, Laubreton D, Nogre M, Ferret C, de Marcos S, et al. Hyper-enriched anti-RSV immunoglobulins nasally administered: A promising approach for respiratory syncytial virus prophylaxis. Front Immunol (2021) 12:683902. doi: 10.3389/fimmu.2021.683902
117. Reglinski M, Sriskandan S. Treatment potential of pathogen-reactive antibodies sequentially purified from pooled human immunoglobulin. BMC Res Notes (2019) 12(1):228. doi: 10.1186/s13104-019-4262-8
118. Barnea Y, Carmeli Y, Gur E, Kuzmenko B, Gat A, Neville LF, et al. Efficacy of antibodies against the n-terminal of pseudomonas aeruginosa flagellin for treating infections in a murine burn wound model. Plast Reconstr Surg (2006) 117(7):2284–91. doi: 10.1097/01.prs.0000218768.34429.e0
119. Felts AG, Giridhar G, Grainger DW, Slunt JB. Efficacy of locally delivered polyclonal immunoglobulin against pseudomonas aeruginosa infection in a murine burn wound model. Burns (1999) 25(5):415–23. doi: 10.1016/s0305-4179(99)00017-0
120. Felts AG, Grainger DW, Slunt JB. Locally delivered antibodies combined with systemic antibiotics confer synergistic protection against antibiotic-resistant burn wound infection. J Trauma (2000) 49(5):873–8. doi: 10.1097/00005373-200011000-00014
121. Mousavi M, Behrouz B, Irajian G, Mahdavi M, Korpi F, Motamedifar M. Passive immunization against pseudomonas aeruginosa recombinant PilA in a murine burn wound model. Microb Pathog (2016) 101:83–8. doi: 10.1016/j.micpath.2016.10.027
122. Barekzi NA, Felts AG, Poelstra KA, Slunt JB, Grainger DW. Locally delivered polyclonal antibodies potentiate intravenous antibiotic efficacy against gram-negative infections. Pharm Res (2002) 19(12):1801–7. doi: 10.1023/a:1021481122011
123. Barekzi NA, Poelstra KA, Felts AG, Rojas IA, Slunt JB, Grainger DW. Efficacy of locally delivered polyclonal immunoglobulin against pseudomonas aeruginosa peritonitis in a murine model. Antimicrob Agents Chemother (1999) 43(7):1609–15. doi: 10.1128/AAC.43.7.1609
124. Bruss JB, Siber GR. Protective effects of pertussis immunoglobulin (P-IGIV) in the aerosol challenge model. Clin Diagn Lab Immunol (1999) 6(4):464–70. doi: 10.1128/CDLI.6.4.464-470.1999
Keywords: antimicrobial resistance, antibiotic stewardship, public health, misuse of antibiotics, human immunoglobulin, infection control, infectious diseases
Citation: Pedraza-Sánchez S, Cruz-González A, Palmeros-Rojas O, Gálvez-Romero JL, Bellanti JA and Torres M (2023) Polyvalent human immunoglobulin for infectious diseases: Potential to circumvent antimicrobial resistance. Front. Immunol. 13:987231. doi: 10.3389/fimmu.2022.987231
Received: 05 July 2022; Accepted: 05 December 2022;
Published: 09 January 2023.
Edited by:
Cristina Toscano Fonseca, Grupo de Pesquisas em Biologia e Imunologia de Doenças Infecciosas e Parasitárias (FIOCRUZ), BrazilReviewed by:
Khaled Mohamed Mahmoud Abou-Aisha, German University in Cairo, EgyptMilan Basta, Biovisions, Inc, United States
Copyright © 2023 Pedraza-Sánchez, Cruz-González, Palmeros-Rojas, Gálvez-Romero, Bellanti and Torres. This is an open-access article distributed under the terms of the Creative Commons Attribution License (CC BY). The use, distribution or reproduction in other forums is permitted, provided the original author(s) and the copyright owner(s) are credited and that the original publication in this journal is cited, in accordance with accepted academic practice. No use, distribution or reproduction is permitted which does not comply with these terms.
*Correspondence: Martha Torres, bWFydGhhdG9ycmVzOThAeWFob28uY29t; Sigifredo Pedraza-Sánchez, c2lnaWZyZWRvLnBlZHJhemFzQGluY21uc3oubXg=