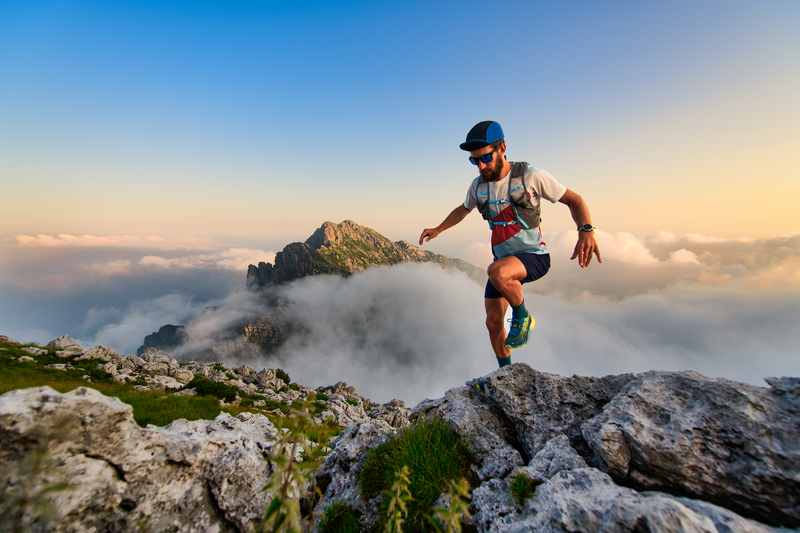
94% of researchers rate our articles as excellent or good
Learn more about the work of our research integrity team to safeguard the quality of each article we publish.
Find out more
REVIEW article
Front. Immunol. , 29 September 2022
Sec. Autoimmune and Autoinflammatory Disorders
Volume 13 - 2022 | https://doi.org/10.3389/fimmu.2022.978571
Kidney disease is a serious hazard to human health. Acute or chronic renal disease will have a significant negative impact on the body’s metabolism. The involvement of mitochondria in renal illness has received a lot of interest as research on kidney disease has advanced. Extracellular vesicles are gaining popularity as a means of intercellular communication in recent years. They have a close connection to both the nephropathy process and the intercellular transfer of mitochondria. The goal of this review is to present the extracellular vesicle transport mitochondria and its related biologically active molecules as new therapeutic options for the treatment of clinical kidney disease. This review focuses on the extracellular vesicles through the transfer of mitochondria and its related bioactive molecules, which affect mitochondrial energy metabolism, take part in immune regulation, and secrete outside the body.
The mitochondria are important cellular organelles, and their internal oxidation system delivers energy to cells via a series of metabolic events. In recent years, an increasing number of studies have revealed that mitochondria play a role not only in energy metabolism but also in immunological modulation and kidney disease by diverse pathways. Extracellular vesicles (EVs) have recently received considerable interest as a type of intercellular communication. Several studies have shown that EVs can transport mitochondria and its related bioactive molecule and create positive therapeutic effects. Hence, transporting technologies are likely novel therapeutic strategies for clinical kidney disease. This review will explore the role of the mitochondria in the histopathology of kidney disease and the therapeutic potential of EVs by regulating mitochondrial function. (Especially in kidney disease).
Mitochondria are double-membraned, elliptical organelles that consist of an outer membrane, inner membrane, stroma, and intermembrane space. The inner membranes are highly folded that form the “cristae.” These cristae contain respiratory chain-related enzyme protein complex, which is the main site of energy production via aerobic respiration in the mitochondria. As the key organelles of energy metabolism, mitochondria are constantly dividing and fusing. Mitochondrial division is mediated by the binding of cytoplasmic dynamicity-associated protein to the receptor mitochondrial fission protein 1 (Fis1) on the outer membrane of the mitochondria. Mitochondria reproduce by division or reduce their number by autophagy, which is how mitochondrial quality is controlled within the cell. Optic atrophy protein-1 (OPA1) on the inner membrane of mitochondria and mitochondrial fusion proteins (Mfn1 and Mfn2) on the outer membrane mediate mitochondrial fusion. Mitochondrial abnormalities can be weakened by fusing their contents, thereby allowing them to deal with greater energy demands during cellular stress (1). This process of continuous division and fusion is called mitochondrial dynamics, in which cells eliminate malfunctioning mitochondria, repair damaged mitochondria, relocate mitochondria to guarantee enough energy supply, and respond to environmental changes in a timely manner. Mitochondrial dynamics primarily manifests itself in cells by changing the morphology of the cristae to affect cell function—fused mitochondria have more cristae area and are more closely connected than divided mitochondria, thereby ensuring the patency of the electron transport chain and allowing for better use of the oxidative phosphorylation reaction. Divided mitochondria prefer to use glycolysis for energy metabolism. According to studies, preventing excessive mitochondrial division and boosting mitochondrial fusion improves cell stress tolerance (2).
Mitochondria play a role in immunological modulation through a variety of pathways in addition to energy metabolism.
(1) Immune modulation is aided by intermediates of the tricarboxylic acid cycle.
The tricarboxylic acid cycle is a vital physiological process for mitochondrial productivity, and it also plays a role in immune modulation. Citriconic acid, an intermediate product of the tricarboxylic acid cycle, has been shown to be able to manufacture fatty acids, nitric oxide, prostaglandin (3), and other compounds in M1-type macrophages (4), indicating that it can continue to synthesize anti-inflammatory chemicals in macrophages (5). Citriconic acid can also produce itaconic acid to inhibit isocitrate lyase and prevent the formation of tuberculosis bacilli and other microorganisms in the body (6).
(2) Immune function is influenced by mitochondrial dynamics.
Mitochondrial dynamics alter immune function by affecting mitochondrial location and consequently cellular energy supply. Microtubules and microfilaments hold mitochondria in place in cells, while motor driver proteins reside on the microfilaments, which can degrade the driver proteins in different directions to allow mitochondria to travel around in the cell (7). Mitochondria use this principle to travel to the synaptic location during T-cell activation, boosting the availability of ATP at the synapse and further controlling the active transport of calcium ions, causing T cells to become activated (8, 9). During activation, mitochondria in naive T cells tend to divide, causing the electron transport chain to become disjointed, increasing the formation of reactive oxygen species (ROS), which is beneficial to the activation of naive T cells (10). In effector T cells, mitochondria tend to divide for glycolysis, whereas memory T cells use mitochondrial fusion, which aids in memory T- cell long-term survival.
(3) Mitochondrial DNA plays a role in immunological regulation.
Because of its similarities to bacterial DNA, mitochondrial DNA (mtDNA) is also implicated in immunological control. In a variety of inflammatory models, mtDNA leakage has been detected. Although it is unclear how mtDNA is extracted from mitochondria, it is apparent that mtDNA plays a major role in immunity as damage-associated molecular patterns (DAMPs) (11). It is squeezed into the cytoplasm, where it is promptly identified by many DNA pattern receptors (including DAI, IFI16, DDX41, DNA-PK, and cGAS (12)) and causes a robust innate immune response (13). Furthermore, during apoptosis, mitochondria produce oxidized mitochondrial DNA, which binds to the NLRP3 inflammasome and enhances downstream apoptotic protease activity-1 activation and the generation of interleukin-1 (14). This way, mitochondrial DNA establishes a link between mitochondrial damage and apoptotic protease-1 (15).
(4) Mitochondria plays a vital role in the production of ROS. Mitochondrial ROS (mtROS) is mainly produced by electron transport chain (ETC) during the process of oxidative phosphorylation. The ETC contains four complexes, and some of it are H+ producing hydrotransmitters (complexes I, III, and IV). When oxygen radicals gain electrons before the expected reaction site (i.e., complex IV), they are converted into ROS (including peroxide, superoxide, and hydroxyl radical) (16). Therefore, complex I and complex III are the main ROS-producing sites (17). In addition, mitochondria generate ROS via pathways other than the respiratory chain (18). Uncoupling protein 2 (UCP2), for example, is a negative regulator of ROS in the mitochondria, and its mechanism needs to be investigated further (19). MtROS is also a key regulator of adaptive immunity. It has been discovered that mtROS can aid T-cell activation and contribute to the production of IL-2 (20). Furthermore, inhibiting T-cell glycerol-3-phosphate dehydrogenase-2 (GPD2), another enzyme that generates mtROS, reduces T-cell IL-2 production (21). According to the findings, mtROS may be directly or indirectly involved in the body’s immune process.
Acute kidney injury (AKI) is the sudden loss of renal function because of a variety of reasons. When AKI develops clinically, the glomerular filtration rate and urine volume both decline dramatically. Although the etiology of AKI caused by renal ischemia-reperfusion damage (IRI), nephrotoxic medications, sepsis, and other etiologies differs, they are all linked to oxidative stress, apoptosis, and the inflammatory response in renal tubular epithelial cells. AKI is also a key factor in the development of chronic renal disease (CKD) (22). As previously said, ROS is produced in mitochondria, and when a sufficient amount of ROS is produced that cannot be neutralized by the antioxidant system, oxidative stress occurs, and ROS can directly or indirectly damage nucleic acids, proteins, and lipids in cells. Excessive ROS products harm the biofilm, reducing its fluidity and increasing permeability, resulting in functional abnormalities in the biofilm (such as cell membrane rupture, mitochondrial membrane swelling and dissolution, and lysosomal membrane dissolution and rupture) as well as cell apoptosis (Figure 1). Hemodynamic alterations and renal fibrosis can be caused by oxidative stress that can exacerbate the onset and progression of kidney disease (23). The high oxidation activity of kidney mitochondria makes the organ vulnerable to oxidative stress damage, which can lead to kidney disease progression and renal failure (24). Excess ROS products serve as intracellular second messengers in the pathological process of AKI, releasing a variety of chemokines and inflammatory factors such as through multiple cellular inflammatory signal transduction pathways, causing the activation and proliferation of inflammatory cells, as well as migration to the injured site, thereby promoting the inflammatory response of the kidney, and ultimately leading to disease. In the mouse AKI model of sepsis induced by cecal ligation perforation, reduced oxidative stress is aided by inhibiting mitochondrial superoxide generation, which improves renal function and survival. Some researchers created a septic renal injury model in male Wistar rats and discovered that endotoxins and inflammatory mediators such tumor necrosis factor, interferon 7, and interleukin 1 produced in vivo can cause apoptosis and accelerate renal injury by increasing ROS levels (25). Furthermore, the ischemia-reperfusion mouse model revealed the opening of the mitochondrial permeability transition pore (mPTP) and subsequent cell death. Cyclophilin D (CypD) is a crucial element of mPTP, and the pp53 CypD complex has been linked to cell death. In vitro tests confirmed that the Pp53 CypD complex alters mitochondrial activity by mPTP opening, resulting in renal tubular cell death (10). This further highlights the importance of mitochondrial dysfunction in acute renal illness is the pathological process of AKI will continue to play a crucial part in chronic kidney injury. When AKI occurs, renal tubule shrinkage is observed, and the number of mitochondria in cells is considerably reduced, cristae are lost, autophagy-lysosomes are increased. At the same time, the oxidation-sensitive mitochondrial protein MPV17L was lost in rat kidney tissues, as was the expression of hypoxia markers and the content of glycolytic products like lactic acid and pyruvate, implying that mitochondrial depletion after AKI leads to irreversible metabolism, promoting the development of early fibrosis (26). In mice nephrectomy models, ROS has been shown to elevate inflammatory factors such as growth transforming factor (TGF-β), fibronectin (FN), and type IV collagen, resulting in chronic kidney injury and reduced renal function. Additionally, mitochondrial failure can activate the inflammasome, resulting in an inflammatory response (27). Damaged mitochondria release immune-related factors such as ROS, DNA, and cardiolipin, which further mediate the inflammatory activation of NLPR3 and the release of interleukin-18 (IL-18) and interleukin-1β (IL-1). It took a month after ischemia for these inflammatory factors to show a sustained increase in expression (28). Mitochondrial DNA interacts with endoplasmic reticulum molecules in a way known as organelle cross-talk, as a new inflammatory pathway, and it directly triggers tubulo-inflammatory responses and promotes the progression of CKD (29).
Figure 1 What happened to damaged mitochondria. mtDNA, mitochondria DNA; ROS, reactive oxygen species.
Mitochondria also play a role in inflammation. NETosis is a cell death mechanism that results in the formation of neutrophil extracellular traps (NETs), which are important in the host’s defense against bacterial infection (30). In recent years, it has been discovered that NETosis abnormalities and NETs clearance defects promote the production and release of type I interferon in systemic lupus erythematosus (SLE), based on the findings of related studies (31). It is linked to vascular complications as well as multiple organ injury (32). This research emphasizes the critical role of mitochondrial ROS in immune complex-mediated NETosis and the importance of mitochondria in the pathogenesis of SLE (33). At the same time, circulating cardiolipin of extracellular mitochondrial origin was discovered, which could explain why anticardiolipin autoantibodies are found in SLE or in the blood of patients with antiphospholipid syndrome (34).
Extracellular vesicles have been found to have anti-inflammatory, anti-apoptotic, angiogenesis, and anti-fibrosis effects on kidney disorders, according to a number of recent research. Studies have also revealed that extracellular vesicles transport mitochondria and that these bioactive molecules have beneficial therapeutic effects on a variety of disorders, including those of the liver, lungs, central nervous system, and other organs. The kidney is an extremely energy-dependent organ, and mitochondrial dysfunction is intimately associated with the development of many renal disorders. Therefore, by transporting mitochondria and their associated components, extracellular vesicles’ therapeutic effect on the kidney can be achieved.
According to related reports, vesicles have been proven to have significant therapeutic effects in kidney diseases.
In a mouse ischemia-reperfusion AKI model, stem-cell–derived vesicles reduced TLR2 and CX3CL1 expression in renal tubular cells and transferred related miRNAs to damaged renal tubular epithelial cells, reducing the infiltration of NK cells into renal damaged tissue and further reducing the inflammatory response (35).
To achieve an anti-apoptotic effect, stem cell-derived EVs obtained from urine can protect podocytes, inhibit renal tubular epithelial cell apoptosis, inhibit the overexpression of Caspase-3 (a terminal splicing enzyme in apoptosis), and promote the proliferation of glomerular endothelial cells in a rat model of type I diabetes. EVs also contain growth factors such as TGF-β1, angiopoietin, and bone morphogenetic protein 7, which can help kidney cells survive (36).
ApoA1 released by EVs has been shown to reduce renal ischemic injury, neutrophil activation, and aggregation by inhibiting the effects of ICAM-1 and P-selectin on endothelial cells, resulting in improved renal injury (37).
The release of mSC-derived, mirNA-LET7C-expressing EVs into the kidney inhibited TGF-1-induced expression of pro-fibrosis genes in target cells, alleviating renal fibrosis in a mouse model of unilateral ureteral occlusion (UUO) (38).
Compared to stem cell therapy, stem cell derived EVs for cell repair have low immunogenicity, are easy to preserve and transport, and reduce other risks associated with stem cell therapy. It has been reported that stem cells can transport mitochondria to other cells using microvesicles (MVs) ranging in diameter from 0.1 m to 1 µm (39) (Figure 2). Other mitochondrial components, such as soluble proteins, the mitochondrial inner membrane, and mtDNA, are transported by exosomes with smaller particle sizes. EVs transport mediated by arrestin domain containing protein 1 acquires suitable mitochondria in bone marrow mesenchymal stem cells, and these tiny vesicles are eventually engulfed by macrophages (40). MSC-derived mitochondria in the cytoplasm are packaged into vesicles containing the autophagy marker light chain 3, which then migrate to the cell’s periphery and merge with outgoing germinating vesicles in the plasma membrane during transport. In the central nervous system, this EV-dependent mitochondrial transport pathway has also been observed between astrocytes and neurons (41). Stem cells restore damaged cell functions by secreting extracellular vesicles that transport mitochondria and other components. This goal is primarily accomplished in two ways (42). One method is to process depolarized mtDNA through vesicles to complete mitochondrial quality control (43). The second is that healthy cells secrete mitochondria and other components to help damaged cells recover their bioenergetics (44). Although there is a lot of indirect evidence that EVs play a role in the intercellular transport of mitochondria and their components, more direct evidence about the biological activity of MV-transported mitochondria is needed.
Figure 2 The mechanism of EVs transporting mt into different cells. EVs, extracellular vesicles; mt, mitochondria; MVs, Microvesicles; IRI, ischemical reperfusion injury; ARDS, acute respiratry distress syndrome; AKI, acute kidney injury.
To begin with, EVs have unrivaled advantages in disease diagnosis. EVs can overcome the limitations of traditional sampling methods given their wide sources and availability in various body fluids, and many relevant studies have confirmed their diagnostic value. EVs containing mitochondria, for example, can be extracted from the blood of patients with systemic lupus erythematosus, and the concentration of these vesicles is linked to disease activity and inflammatory factor levels. Other research has found that EVs containing mitochondrial proteins can be extracted from the plasma of melanoma patients. These proteins are unique and distinguish this subpopulation of EVs from other cells, allowing for melanoma diagnosis with greater precision (45).
With the advancement of EVs research in recent years, EVs, particularly EVs derived from MSC, have been used in an increasing number of studies on diseases involving mitochondrial damage (1). MSC-EVS improved the effects of liver IRI in the ischemia-reperfusion model of hepatocytes by reducing intracellular ROS levels, alleviating hepatocyte apoptosis after ischemia-reperfusion, and preventing the formation of local Net by transferring functional mitochondria to intrahepatic neutrophils and restoring their mitochondrial function (46) (2).MSCs regulate human macrophages in the inflammatory environment of acute respiratory distress syndrome (ARDS) to reduce the production of pro-inflammatory cytokines, increase the expression of M2 phenotypic marker CD206, and improve macrophage phagocytosis. This effect is achieved by mitochondria-carrying MSC-EVS promoting macrophage oxidative phosphorylation to reveal a new mechanism of macrophage polarization regulation. Furthermore, this research suggests that mSC-EVS-induced changes in macrophages are sufficient to induce lung injury protection in vivo (47) (3). mSC-EVS has been shown to reduce mtDNA damage and inflammation after AKI in an ischemia-reperfusion–induced AKI model, which is dependent on the mitochondrial transcription factor A (TFAM) pathway. Transcription inhibition had no effect on the transfer of mitochondrial TFAMmRNA in recipient cells via EVs. As a result, MSC-EVS restored TFAM protein and TFAM-MTDNA complex (nucleoid) stability, reversing mtDNA deletion and mitochondrial oxidative phosphorylation (OXPHOS) defects in damaged renal tubular cells. As a result, MSC-EVS can effectively reduce mitochondrial damage and inflammation in cell and animal renal injury models by restoring TFAM expression and preventing mtDNA damage and cytoplasmic mtDNA leakage (48). In regenerative medicine, this study shows that MSC-EVS is a promising nanotherapy for attenuating various diseases characterized by mitochondrial damage (4). After a stroke, astrocyte-derived extracellular vesicles have been shown to transfer mitochondria to neurons via the CD83 signaling mechanism, allowing cells to survive in a state of relative hypoxia and glucose deficiency (41).
Extracellular vesicles are an important mode of communication between cells, and their transport mitochondrial bioactive molecule can play a role in immunomodulation either directly or indirectly. Platelets have been shown to secrete vesicles containing mitochondria, which are then broken down by phospholipase A2-IIA in neutrophils, releasing mtDNA, lysophospholipids, and fatty acids, which further activate neutrophils and promote their pro-inflammatory response (49). The ability of extracellular vesicles to participate in immune regulation is further demonstrated in this study.
The kidney is a highly energy-dependent organ due to the need for reabsorption, and mitochondria have high oxidative activity and are the main site of ROS production. Furthermore, it is linked to the occurrence and progression of several kidney diseases (50). Extracellular vesicles have been shown to have the function of transferring mitochondria and their associated components to repair damaged cells and participate in immune regulation (shown in Table 1), which is a novel concept with significant benefits. Kidney diseases are caused by wide range of factors, all of which are linked to immune function. As previously stated, mitochondria play a role in the occurrence and progression of kidney disease through a variety of mechanisms, including ROS production, immune regulation, and energy metabolism. Extracellular vesicles have been shown in recent studies to have anti-inflammatory, anti-apoptotic, angiogenesis-promoting, and anti-fibrosis effects in kidney diseases. Meanwhile, research has shown that mitochondrial extracellular vesicle transfer has a positive therapeutic effect on diseases of the liver, lungs, central nervous system, and other organs. Therefore, do mitochondrial associated EVs transfer play a role in kidney disease, and can it be used as a new kidney disease diagnosis and treatment strategy? We believe that if it is used in the diagnosis and treatment of varied kidney diseases, we can explore the possibility of improved disease diagnosis and treatment, which can benefit more patients with acute and chronic kidney disease. The mechanism of MSC-EVS transfer to mitochondria and related bioactive substances still have great therapeutic potential in kidney diseases, which is worthy of more peers to participate in the study. However, the majority of the evidence supporting MV transfer to mitochondria is indirect, and whether the transferred mitochondria have comparable biological activity is also worth discussing. To conclude, EVs transport of mitochondria and its related components is very promising, and it deserves more peer participation in this field.
JM: Preparing the table and figures. Drafting the manuscript. CW and JT: Drafting the manuscript. Reviewing and editing the figures and table. Conceptualization and revising. CL, ZS, and FW: Reviewing and editing the manuscript. XC: Preparing the figure and table. All authors contributed to the article and approved the submitted version.
This work was supported by the [National Natural Science Foundation of China #1] under Grant [number 82070758]; and [Hunan Provincial Key R&D Program Project#2] under Grant [number 2020SK2084].
The authors declare that the research was conducted in the absence of any commercial or financial relationships that could be construed as a potential conflict of interest.
All claims expressed in this article are solely those of the authors and do not necessarily represent those of their affiliated organizations, or those of the publisher, the editors and the reviewers. Any product that may be evaluated in this article, or claim that may be made by its manufacturer, is not guaranteed or endorsed by the publisher.
1. Xiao X, Hu Y, Quiros PM, Wei Q, Lopez-Otin C, Dong Z. OMA1 mediates OPA1 proteolysis and mitochondrial fragmentation in experimental models of ischemic kidney injury. Am J Physiology-Renal Physiol (2014) 306(11):F1318–26. doi: 10.1152/ajprenal.00036.2014
2. Xu Y, Shen J, Ran Z. Emerging views of mitophagy in immunity and autoimmune diseases. Autophagy (2020) 16(1):3–17. doi: 10.1080/15548627.2019.1603547
3. Tannahill GM, Curtis AM, Adamik J, Palsson-McDermott EM, McGettrick AF, Goel G, et al. Succinate is an inflammatory signal that induces IL-1 beta through HIF-1 alpha. Nature (2013) 496(7444):238. doi: 10.1038/nature11986
4. Angajala A, Lim S, Phillips JB, Kim J-H, Yates C, You Z, et al. Diverse roles of mitochondria in immune responses: Novel insights into immuno-metabolism. Front Immunol (2018) 9. doi: 10.3389/fimmu.2018.01605
5. Michelucci A, Cordes T, Ghelfi J, Pailot A, Reiling N, Goldmann O, et al. Immune-responsive gene 1 protein links metabolism to immunity by catalyzing itaconic acid production. Proc Natl Acad Sci USA (2013) 110(19):7820–5. doi: 10.1073/pnas.1218599110
6. O'Neill LAJ, Kishton RJ, Rathmell J. A guide to immunometabolism for immunologists. Nat Rev Immunol (2016) 16(9):553–65. doi: 10.1038/nri.2016.70
7. Wu D, Xu Y, Ding T, Zu Y, Yang C, Yu L. Pairing of integrins with ECM proteins determines migrasome formation. Cell Res (2017) 27(11):1397–400. doi: 10.1038/cr.2017.108
8. Desdin-Mico G, Soto-Heredero G, Mittelbrunn M. Mitochondrial activity in T cells. Mitochondrion (2018) 41:51–7. doi: 10.1016/j.mito.2017.10.006
9. Quintana A, Hoth M. Mitochondrial dynamics and their impact on T cell function. Cell Calcium (2012) 52(1):57–63. doi: 10.1016/j.ceca.2012.02.005
10. Mills EL, Kelly B, Logan A, Costa ASH, Varma M, Bryant CE, et al. Succinate dehydrogenase supports metabolic repurposing of mitochondria to drive inflammatory macrophages. Cell (2016) 167(2):457. doi: 10.1016/j.cell.2016.08.064
11. Banoth B, Cassel SL. Mitochondria in innate immune signaling. Trans Res (2018) 202:52–68. doi: 10.1016/j.trsl.2018.07.014
12. Riley JS, Tait SWG. Mitochondrial DNA in inflammation and immunity. EMBO Rep (2020) 21(4):e49799. doi: 10.15252/embr.201949799
13. Youle RJ, van der Bliek AM. Mitochondrial fission, fusion, and stress. Science (2012) 337(6098):1062–5. doi: 10.1126/science.1219855
14. Shimada K, Crother TR, Karlin J, Dagvadorj J, Chiba N, Chen S, et al. Oxidized mitochondrial DNA activates the NLRP3 inflammasome during apoptosis. Immunity (2012) 36(3):401–14. doi: 10.1016/j.immuni.2012.01.009
15. Nakahira K, Haspel JA, Rathinam VAK, Lee S-J, Dolinay T, Lam HC, et al. Autophagy proteins regulate innate immune responses by inhibiting the release of mitochondrial DNA mediated by the NALP3 inflammasome. Nat Immunol (2011) 12(3):222–U57. doi: 10.1038/ni.1980
16. Tsushima K, Bugger H, Wende AR, Soto J, Jenson GA, Tor AR, et al. Mitochondrial reactive oxygen species in lipotoxic hearts induce post-translational modifications of AKAP121, DRP1, and OPA1 that promote mitochondrial fission. Circ Res (2018) 122(1):58–73. doi: 10.1161/CIRCRESAHA.117.311307
17. Zorov DB, Juhaszova M, Sollott SJ. MITOCHONDRIAL REACTIVE OXYGEN SPECIES (ROS) AND ROS-INDUCED ROS RELEASE. Physiol Rev (2014) 94(3):909–50. doi: 10.1152/physrev.00026.2013
18. Sena LA, Chandel NS. Physiological roles of mitochondrial reactive oxygen species. Mol Cell (2012) 48(2):158–67. doi: 10.1016/j.molcel.2012.09.025
19. Rousset S, Emre Y, Join-Lambert O, Hurtaud C, Ricquier D, Cassard-Doulcier A-M. The uncoupling protein 2 modulates the cytokine balance in innate immunity. Cytokine (2006) 35(3-4):135–42. doi: 10.1016/j.cyto.2006.07.012
20. Sena LA, Li S, Jairaman A, Prakriya M, Ezponda T, Hildeman DA, et al. Mitochondria are required for antigen-specific T cell activation through reactive oxygen species signaling. Immunity (2013) 38(2):225–36. doi: 10.1016/j.immuni.2012.10.020
21. Kaminski MM, Sauer SW, Kaminski M, Opp S, Ruppert T, Grigaravicius P, et al. T Cell activation is driven by an ADP-dependent glucokinase linking enhanced glycolysis with mitochondrial reactive oxygen species generation. Cell Rep (2012) 2(5):1300–15. doi: 10.1016/j.celrep.2012.10.009
22. Coca SG, Singanamala S, Parikh CR. Chronic kidney disease after acute kidney injury: a systematic review and meta-analysis. Kidney Int (2012) 81(5):442–8. doi: 10.1038/ki.2011.379
23. Granger DN, Kvietys PR. Reperfusion injury and reactive oxygen species: The evolution of a concept. Redox Biol (2015) 6:524–51. doi: 10.1016/j.redox.2015.08.020
24. Galvan DL, Badal SS, Long J, Chang BH, Schumacker PT, Overbeek PA, et al. Real-time in vivo mitochondrial redox assessment confirms enhanced mitochondrial reactive oxygen species in diabetic nephropathy. Kidney Int (2017) 92(5):1282–7. doi: 10.1016/j.kint.2017.05.015
25. Zhang Q, Raoof M, Chen Y, Sumi Y, Sursal T, Junger W, et al. Circulating mitochondrial DAMPs cause inflammatory responses to injury. Nature (2010) 464(7285):104–U15. doi: 10.1038/nature08780
26. Tirichen H, Yaigoub H, Xu W, Wu C, Li R, Li Y. Mitochondrial reactive oxygen species and their contribution in chronic kidney disease progression through oxidative stress. Front Physiol (2021) 12. doi: 10.3389/fphys.2021.627837
27. Basile DP, Anderson MD, Sutton TA. Pathophysiology of acute kidney injury. Compr Physiol (2012) 2(2):1303–53. doi: 10.1002/cphy.c110041
28. Sander LE, Garaude J. The mitochondrial respiratory chain: A metabolic rheostat of innate immune cell-mediated antibacterial responses. Mitochondrion. (2018) 41:28–36. doi: 10.1016/j.mito.2017.10.008
29. Mills E, O'Neill LAJ. Succinate: a metabolic signal inflammation. Trends Cell Biol (2014) 24(5):313–20. doi: 10.1016/j.tcb.2013.11.008
30. Phillipson M, Kubes P. The neutrophil in vascular inflammation. Nat Med (2011) 17(11):1381–90. doi: 10.1038/nm.2514
31. Kaplan MJ. Neutrophils in the pathogenesis and manifestations of SLE. Nat Rev Rheumatol (2011) 7(12):691–9. doi: 10.1038/nrrheum.2011.132
32. Villanueva E, Yalavarthi S, Berthier CC, Hodgin JB, Khandpur R, Lin AM, et al. Netting neutrophils induce endothelial damage, infiltrate tissues, and expose immunostimulatory molecules in systemic lupus erythematosus. J Immunol (2011) 187(1):538–52. doi: 10.4049/jimmunol.1100450
33. Lood C, Blanco LP, Purmalek MM, Carmona-Rivera C, De Ravin SS, Smith CK, et al. Neutrophil extracellular traps enriched in oxidized mitochondrial DNA are interferogenic and contribute to lupus-like disease. Nat Med (2016) 22(2):146–53. doi: 10.1038/nm.4027
34. Boilard E, Fortin PR. Mitochondria drive NETosis and inflammation in SLE. Nat Rev Rheumatol (2016) 12(4):195–6. doi: 10.1038/nrrheum.2016.24
35. Zou X, Gu D, Zhang G, Zhong L, Cheng Z, Liu G, et al. NK cell regulatory property is involved in the protective role of MSC-derived extracellular vesicles in renal ischemic reperfusion injury. Hum Gene Ther (2016) 27(11):926–35. doi: 10.1089/hum.2016.057
36. Jiang Z-Z, Liu Y-M, Niu X, Yin J-Y, Hu B, Guo S-C, et al. Exosomes secreted by human urine-derived stem cells could prevent kidney complications from type I diabetes in rats. Stem Cell Res Ther (2016) 7:24. doi: 10.1186/s13287-016-0287-2
37. Shi N, Wu M-P. Apolipoprotein a-I attenuates renal ischemia/reperfusion injury in rats. J Biomed Science (2008) 15(5):577–83. doi: 10.1007/s11373-008-9258-7
38. Wang B, Yao K, Huuskes BM, Shen H-H, Zhuang J, Godson C, et al. Mesenchymal stem cells deliver exogenous MicroRNA-let7c via exosomes to attenuate renal fibrosis. Mol Ther (2016) 24(7):1290–301. doi: 10.1038/mt.2016.90
39. Wang J, Li H, Yao Y, Zhao T, Chen Y-Y, Shen Y-L, et al. Stem cell-derived mitochondria transplantation: a novel strategy and the challenges for the treatment of tissue injury. Stem Cell Res Ther (2018) 9:106. doi: 10.1186/s13287-018-0832-2
40. Phinney DG, Di Giuseppe M, Njah J, Sala E, Shiva S, St Croix CM, et al. Mesenchymal stem cells use extracellular vesicles to outsource mitophagy and shuttle microRNAs. Nat Commun (2015) 6:8472. doi: 10.1038/ncomms9472
41. Hayakawa K, Esposito E, Wang X, Terasaki Y, Liu Y, Xing C, et al. Transfer of mitochondria from astrocytes to neurons after stroke. Nature (2016) 535(7613):551. doi: 10.1038/nature18928
42. Paliwal S, Chaudhuri R, Agrawal A, Mohanty S. Regenerative abilities of mesenchymal stem cells through mitochondrial transfer. J Biomed Sci (2018) 25:31. doi: 10.1186/s12929-018-0429-1
43. McLelland G-L, Soubannier V, Chen CX, McBride HM, Fon EA. Parkin and PINK1 function in a vesicular trafficking pathway regulating mitochondrial quality control. EMBO J (2014) 33(4):282–95. doi: 10.1002/embj.201385902
44. Sinha P, Islam MN, Bhattacharya S, Bhattacharya J. Intercellular mitochondrial transfer: bioenergetic crosstalk between cells. Curr Opin Genet Dev (2016) 38:97–101. doi: 10.1016/j.gde.2016.05.002
45. Jang SC, Crescitelli R, Cvjetkovic A, Belgrano V, Bagge RO, Sundfeldt K, et al. Mitochondrial protein enriched extracellular vesicles discovered in human melanoma tissues can be detected in patient plasma. J Extracellular Vesicles (2019) 8(1):1635420. doi: 10.1080/20013078.2019.1635420
46. Hsu M-J, Karkossa I, Schaefer I, Christ M, Kuehne H, Schubert K, et al. Mitochondrial transfer by human mesenchymal stromal cells ameliorates hepatocyte lipid load in a mouse model of NASH. Biomedicines (2020) 8(9):350. doi: 10.3390/biomedicines8090350
47. Morrison TJ, Jackson MV, Cunningham EK, Kissenpfennig A, McAuley DF, O'Kane CM, et al. Mesenchymal stromal cells modulate macrophages in clinically relevant lung injury models by extracellular vesicle mitochondrial transfer. Am J Respir Crit Care Med (2017) 196(10):1275–86. doi: 10.1164/rccm.201701-0170OC
48. Zhao M, Liu S, Wang C, Wang Y, Wan M, Liu F, et al. Mesenchymal stem cell-derived extracellular vesicles attenuate mitochondrial damage and inflammation by stabilizing mitochondrial DNA. ACS Nano (2021) 15(1):1519–38. doi: 10.1021/acsnano.0c08947
49. Boudreau LH, Duchez A-C, Cloutier N, Soulet D, Martin N, Bollinger J, et al. Platelets release mitochondria serving as substrate for bactericidal group IIA-secreted phospholipase A(2) to promote inflammation. Blood (2014) 124(14):2173–83. doi: 10.1182/blood-2014-05-573543
Keywords: mitochondria, renal diseases, treatment, extracellular vesicle (EV), immune
Citation: Mao J, Li C, Wu F, She Z, Luo S, Chen X, Wen C and Tian J (2022) MSC-EVs transferring mitochondria and related components: A new hope for the treatment of kidney disease. Front. Immunol. 13:978571. doi: 10.3389/fimmu.2022.978571
Received: 26 June 2022; Accepted: 13 September 2022;
Published: 29 September 2022.
Edited by:
Egor Plotnikov, Lomonosov Moscow State University, RussiaReviewed by:
Mujib Ullah, Stanford University, United StatesCopyright © 2022 Mao, Li, Wu, She, Luo, Chen, Wen and Tian. This is an open-access article distributed under the terms of the Creative Commons Attribution License (CC BY). The use, distribution or reproduction in other forums is permitted, provided the original author(s) and the copyright owner(s) are credited and that the original publication in this journal is cited, in accordance with accepted academic practice. No use, distribution or reproduction is permitted which does not comply with these terms.
*Correspondence: Chuan Wen, Y2h1YW53ZW5AY3N1LmVkdS5jbg==; Jidong Tian, dGlhbmppZG9uZ0Bjc3UuZWR1LmNu
Disclaimer: All claims expressed in this article are solely those of the authors and do not necessarily represent those of their affiliated organizations, or those of the publisher, the editors and the reviewers. Any product that may be evaluated in this article or claim that may be made by its manufacturer is not guaranteed or endorsed by the publisher.
Research integrity at Frontiers
Learn more about the work of our research integrity team to safeguard the quality of each article we publish.