- Department of Hematology, Tianjin Medical University General Hospital, Tianjin, China
There are two figures and one table in this review, the review consists of 5823 words, without the description of figures and table, but including references.
Tumor cells escape anti-tumor immune responses in various ways, including functionally shaping the microenvironment through the secretion of various chemokines and, cytokines. Adenosine is a powerful immunosuppressive metabolite, that is frequently elevated in the extracellular tumor microenvironment (TME). Thus, it has recently been proposed as a novel antitumor immunoassay for targeting adenosine- generating enzymes, such as CD39, CD73, and adenosine receptors. In recent years, the discovery of the immune checkpoints, such as programmed cell death 1(PD-1) and cytotoxic T lymphocyte antigen 4 (CTLA-4), has also greatly changed treatment methods and ideas for malignant tumors. Malignant tumor immunotherapy has been developed from point-to-point therapy targeting immune checkpoints, combining different points of different pathways to create a therapy based on the macroscopic immune regulatory system network. This article reviews the theoretical basis of the adenosine energy axis and immune checkpoint combined therapy for malignant tumors and the latest advances in malignant tumors.
Adenosinergic axis and tumor immunology
Adenosine is an important regulator of metabolism and a key immune checkpoint regulator associated with tumors evading the host immune system (1–4). Extracellular adenosine (eADO) inhibits immune function. One of the major mechanisms of tumor immune evasion is the production of high eADO levels via the overexpression of ectonucleotidases (5–7). An effective immunosuppressive microenvironment is sustained when ADO functions synergistically or in combination with other immunosuppressive mechanisms (8). In 2006, high extracellular adenosine levels in tumors were discovered to play a key role in evading antitumor immune responses (9), and an environment rich in adenosine in the tumor may induce incompetent T cells (1, 10–12). The adenosine pathway is currently considered important for the effectiveness of immunotherapy and has become an important target for cancer therapy (1, 13).Endogenous ATP (eATP) can be released in large quantities through cell necrosis, apoptosis and mechanical damage (14), and can also be actively secreted by tumor cells, immunocytes, and other histocytes in the TME, triggered by various cell damage factors such as hypoxia, chronic inflammation, and cytotoxic drugs (2). The main source of eADO is the continuous degradation of eATP, which involves many different extracellular enzymes, including NTPDase1/CD39 and CD73 (2, 13, 14). CD39 is highly expressed in the tumor endothelium of the TME and on most immunocytes (including macrophages, myeloid cells, and FOXP3+ regulatory T cells (Treg) (3). The CD39 topological domain consists of two transmembrane domains, including short cytoplasmic N-and C-terminal segments and a large extracellular hydrophobic domain containing the active site (15).The extracellular domain contains five conserved propyrylase regions from ACR1 to ACR5, among which the amino acid sequences of ACR1 and ACR5 contain phosphate-binding motifs, which are believed to be critical for stabilizing the interaction between the enzyme and its nucleotide substrate during phosphate cleavage (4). CD39 can stabilize FOXP3+Tregs, contribute to their immunosuppressive function (16), promote type I Treg differentiation, produce IL-10, and restrict the activation of NLRP3 inflammatory bodies in dendritic cells (DCs) (2, 13, 14). CD73 can be found in different kinds of tissues, which includes the colon, liver, kidney, brain, lungs, and heart; leukocytes and endothelial cells of peripheral blood, lymph nodes, spleen and bone marrow (3). CD73 is now known as glycosyl phosphatidyl inositol (GPI)-anchored protein (17), which is a homodimeric disulfide linker protein of 548 amino acids, of which the N-terminus provides a binding site for two catalytic divalent metal ions and the C-terminus is a binding site for AMP (18). The expression and function of CD73 are elevated in the presence of hypoxia and inflammatory mediators (TGF- B, IFNs, TNF-α, IL-1B, PGE2, etc.), and the expression of CD73 is also increased in several tumor tissues, suggesting that CD73 is involved in tumor genesis and development (2, 13, 14, 19), eATP is decomposed into eADO through the sequence of CD39 and CD73, which bind to adenosine receptors on the cell membrane surface (2, 13, 14). Among several known adenosine receptors, adenosine receptor A2a (A2aR) is the predominant subtype and is mainly expressed in immunocytes (20). A2aR stimulation usually provides immunosuppressive signals that inhibit T cell proliferation, cytotoxicity, cytokine production, NK cell cytotoxicity, NKT cell cytokine production, CD40L upregulation, macrophage/DC antigen presentation, etc. (1, 19) (Figure 1). Based on the above background analysis, when we want to antagonize the immunosuppressive effect of eADO in the TME, we can start from the following three aspects: 1. It reduces the expression of CD39 in tumor cells and inhibits the conversion of eATP to AMP; 2. It reduces the expression of CD73 molecules on tumor cells and blocks the conversion of AMP into eADO, thereby reducing the binding of eADO to A2aR receptors on immune cells; 3. It reduces the expression of A2aR in immune cells, making them unable to combine with eADO to maintain their normal immune function.
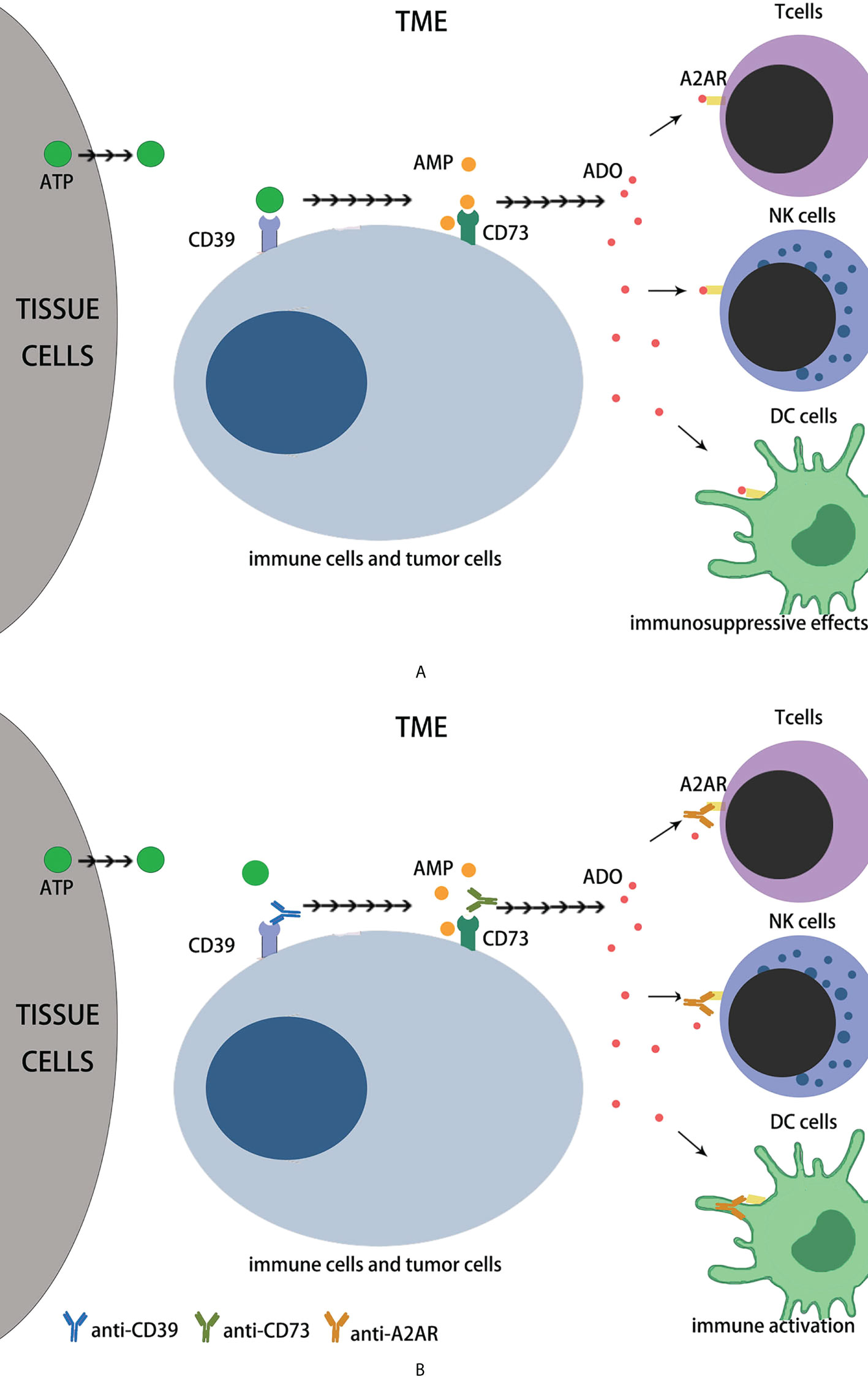
Figure 1 The immune effects of adenosine axis in TME. (A) The eATP can be released in large quantities during cell necrosis, apoptosis and mechanical damage, and can be actively secreted by tumor cells and other cells in the TME, which is triggered by hypoxia, chronic inflammation, nutrient deprivation, or cytotoxic drugs. Extracellular ATP is broken down by CD39 to AMP, then CD73 to adenosine. Extracellular adenosine binds to A2AR on immunocytes such as T cells, NK cells and DC cells, inhibiting their immune function. (B) Role of monoclonal antibodies in adenosine axis. CD39 mAb prevents eATP from binding to CD39 to reduce AMP production; CD73 mAb prevents AMP from binding to CD73 to reduce ADO production. A2AR mAb prevents ADO from binding to A2AR and inhibits its immunosuppressive effect.
Immune checkpoint
The immune system consists of innate and acquired immunity, which, once activated, clears infectious pathogens and tumor cells. Inhibitory pathways in antimicrobial or antitumor immune responses normally maintain auto-tolerance to avoid excessive damage and limit associated tissue damage (21, 22). This receptor and ligand inhibitory pathways are known as “Immune Checkpoint” and are used by tumor cells to avoid Immune attack. The development of monoclonal antibodies to inhibit these checkpoints, thereby removing the Inhibition of immunocytes and enabling them to recognize and kill tumor cells, is called “Immune Checkpoint Inhibition”. These drugs are called “ immune checkpoint inhibitors” (ICI) (23, 24). FDA-approved anti-CTLA-4 and anti-PD-1 antibodies for cancer treatment, which led to the belief that immunotherapy for cancer was realistic and further encouraged the development of other new ICIs (25–27). Immunotherapy is becoming an important treatment for cancer patients (1, 13). Immune checkpoint blocking (ICB) based on monoclonal antibody (mAb) has also proven to be a safe and effective treatment for hematologic malignancies in the past decade (22, 27). In oncology, checkpoints currently targeted by inhibitors to amplify the reactivity of T cells, NK cells and bone marrow cells include CTLA-4 (28), PD-1, PD-L1 (PD1 ligand 1/CD274),LAG-3 (CD223), TIM3 (T cell immunoglobulin-3), TIGIT(T cell immunoglobulin and ITIM domain) (29), VISTA (V-domain immunoglobulin suppressor of T cell activation) (30), B7/H3(CD276), KIR (killer cell immunoglobulin-like receptors),NKG2A, A2AR, CD39, CD73, CSF1R, and CD47 (22, 31, 32) (Figure 2).
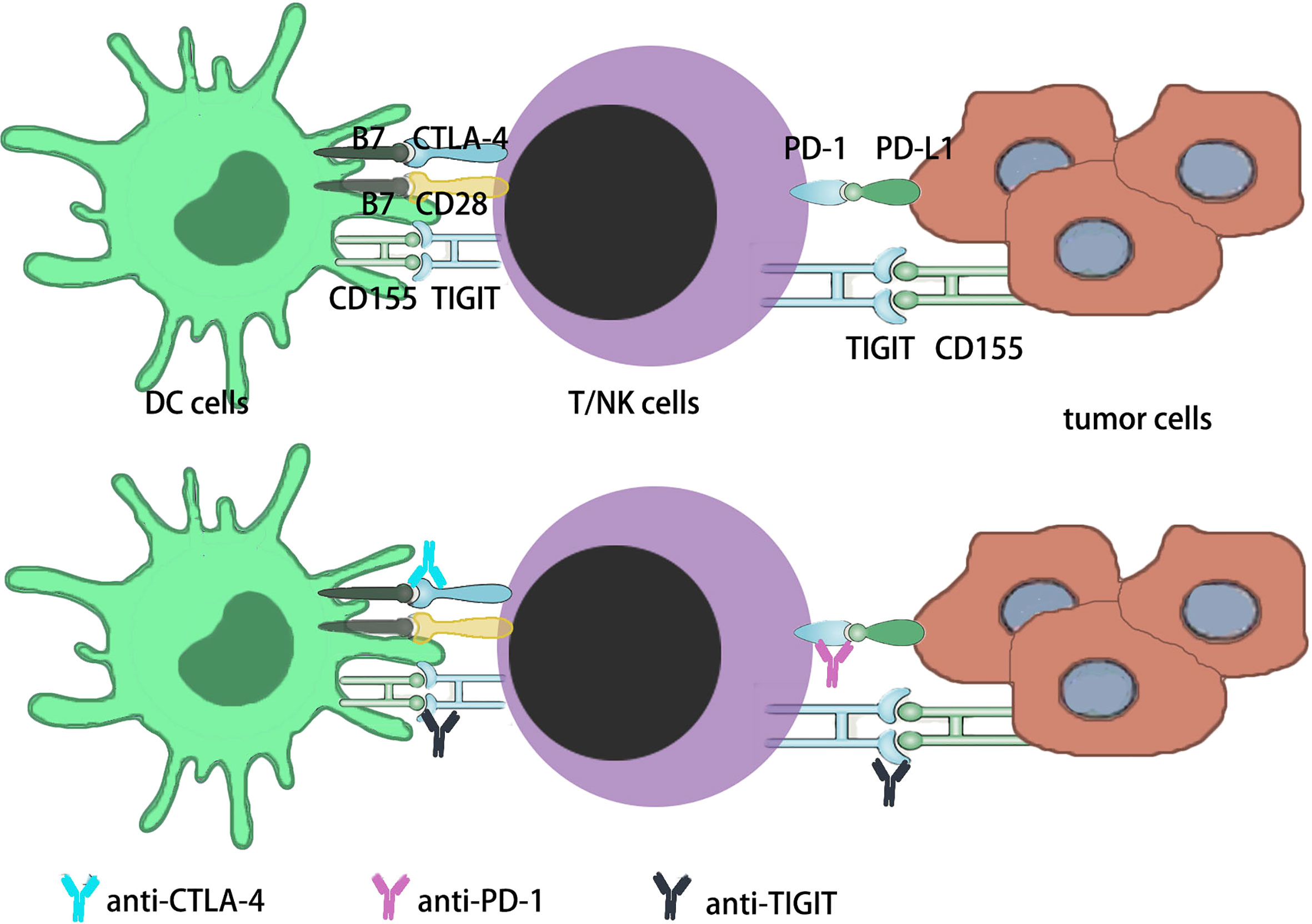
Figure 2 Immune checkpoints in current studies. i) The PD-1 is expressed on activated T cells in the early-stage lymph node as well as late-stage tumor tissues in the TME. In the early stage and late stage, PD-1 sustains immune homeostasis by decreasing activated T cells function. Tumors might become resistant to this suppression signaling, increasing the survival potential of the tumor cells. ii) The CTLA-4 is expressed on T cells that are activated by DCs in the lymph node. By MHC interaction with T cell receptor and B7 signal interaction with CD28 on T cells. In order to sustain immune homeostasis, CTLA-4 downregulates the function of activated T cells through the interaction of B7 signaling with CTLA-4 on T cells. Tumors may develop toleration to this inhibitory signal, thus improving the survival potential of tumor cells. iii) TIGIT is expressed both on NK cells and T cells, which includes CD4+ T cells, CD8+ T cells and Tregs. TIGIT has three ligands, CD155, CD112 and CD113, and the main ligand for TIGIT is CD155. The main effect of TIGIT is downregulating the function of NK cells and T cells.
Each member of the adenosine signaling pathway constitutes a different drug target, meaning that it is possible for combined therapy with more than one drug to target this or complementary signaling pathway (33). Many of these combinations are currently in preclinical and clinical trials, such as anti-CD73 and anti-A2aR combinations, anti-CD73 and anti-PD-1 combinations, and anti-A2aR and anti-TIGIT antibody combinations (21, 31, 34–36) (Table 1).
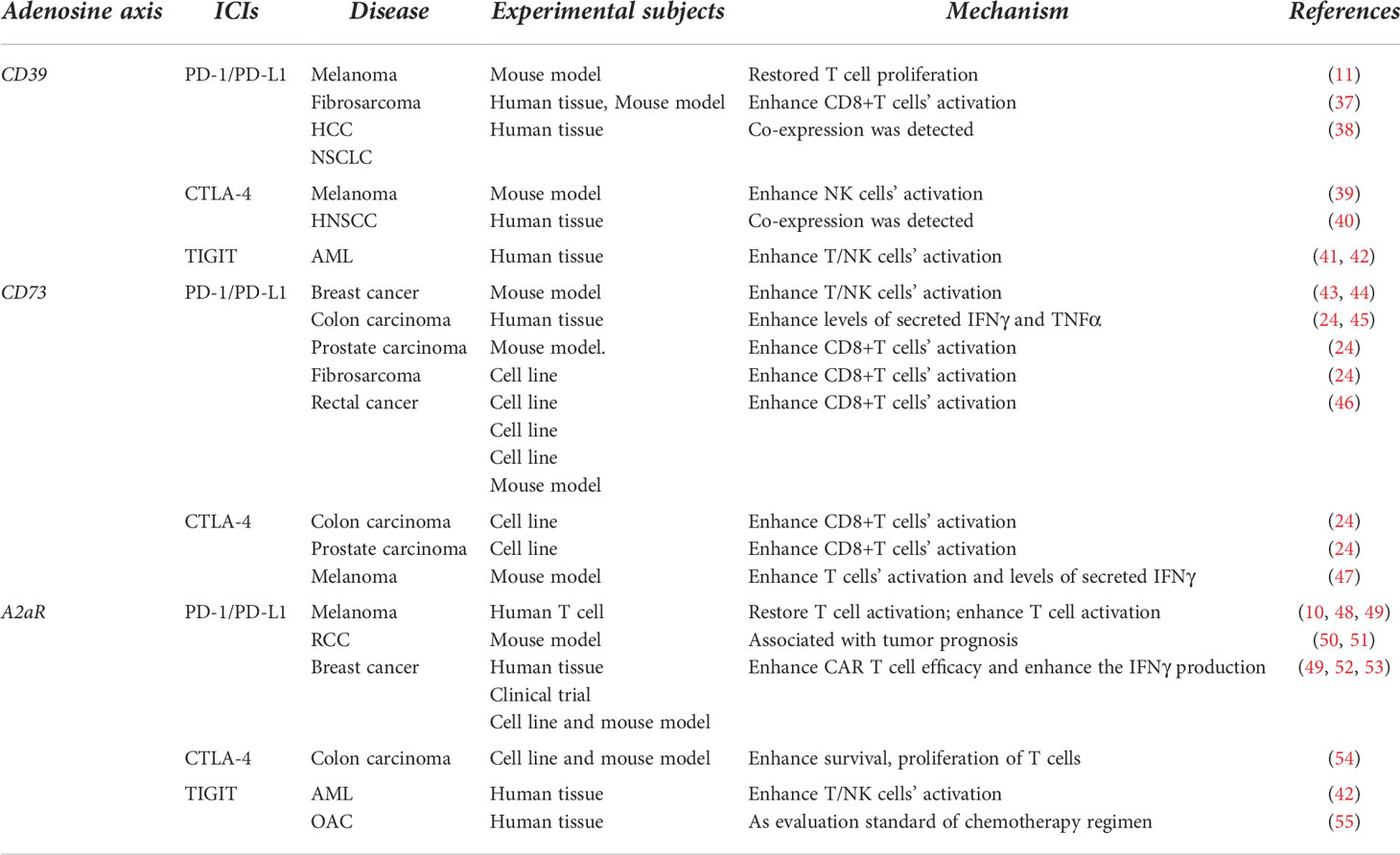
Table 1 Application of different targets on adenosinergic axis combined with various immune checkpoints in tumor therapy.
Combination of CD39 with immune checkpoints
The rapid development of flow cytometry in recent years has further confirmed the expression of CD39 in tumor cells, particularly in melanoma, lymphoma, and chronic lymphocytic leukemia (CLL) cell lines (13, 14). In melanoma B16F10 mouse model and colorectal cancer Mc-38 mouse model, CD39-defective mice were resistant to tumor metastasis (31, 56). It has been documented that all cells expressing CD39 exhibit strong ATPase activity, which can be counterbalanced by CD39 inhibitors, such as ARL-67156 and POM-1, by measuring the degradation of eATP or releasing free phosphate from the cell culture supernatant. Treatment with BY40, a CD39 blocking antibody currently under preclinical development, reduces the inhibition of CD4+ and CD8+T cell proliferation, which is induced by tumor tissue and increases cytotoxicity mediated by cytotoxic T lymphocytes (CTL) and NK cells (16). At present, many studies have shown that human CD39+CD8+ T cells exhibited draining dysfunction or phenotype gene signature of T cells, including highly expressed inhibitory receptors, PD-1 and CTLA-4 (57). Thus targeted therapy of CD39 combined with other immune binding sites has great significance in the therapy of tumors. Currently, the main CD39 mAb used in clinical research is IPH5201, which blocks the hydrolysis of ATP by a membrane and soluble CD39, thus promoting DC maturation and macrophage activation (11); BY40 has been reported to block membrane-associated, but insoluble, human CD39 enzyme activity, but its clinical efficacy has not been evaluated (11, 58); POM1 is mainly used for experimental studies on mice and cell lines (11).
CD39 mAb combined with PD-1 mAb
PD-1 encodes immunoglobulin superfamily proteins and is focused on sustaining immune tolerance to autoantigens and preventing autoimmune diseases. PD-L1 is a PD-1 ligand blocking the interaction between the tumor cells expressing PD-L1 and tumor-specific T cells expressing PD-1 using PD-1 or PD-L1 antibodies enhance the cytolytic activity of T cells (25). It has strong therapeutic value and significance in solid tumors and hematologic malignancies (33, 59). However, during immunotherapy, many tumors show resistance to PD-1/PD-L1. The exhibition of resistance by patients might be due to the immunosuppressive TME, where ROS or nitrogen oxides (NO) released by bone marrow-derived suppressor cells (MDSC) tire T cells and no longer recognize tumor cells. PD1 resistance and poor prognosis in hepatocellular carcinoma (HCC) patients are associated with the upregulation of CD39 expression in macrophages, and CD39 can be used as a marker of unfavorable prognosis in HCC patients (37). This significantly improved through combination therapy with CD39 mAb. It has been reported that the therapy combining anti-CD39 and anti-PD1 mAbs can further slow tumor growth and that the inhibition of CD39 enzyme function can make the tumor model with inherent drug resistance sensitive to PD1 antibody (11, 38).This may be because CD39 mAb and PD-1 mAb can recover the ability of CD8+T cells to produce cytokines. CD39 mAb combined with PD-1 mAb has become one of the targets of many tumor therapies (37).
CD39 mAb combined with CTLA-4 mAb
CTLA-4 is a molecule belonging to the immunoglobulin superfamily. It was first discovered in the cDNA libraries of CTLs and expressed in activated T cells, Tregs, and acute myeloid leukemia cells (28, 33). Although CTLA-4 and its homologue CD28 bind to ligand B7 on B cells and APCs, stimulation of CTLA-4 does not result in T cell activation, but rather in T-cell-mediated antibodies that inhibit and prevent allograft rejection (23, 25). Blocking the CTLA-4-B7 interaction with anti-CTLA-4 mAb results in an enhanced alloantigen response that inhibits negative signaling to T cells (32). However, anti-CTLA-4 is rarely effective as a single drug for highly oncogenic and immunogenic tumors. Targeting CD39 with POM-1 has a synergistic effect on anti-CTLA-4 checkpoint blockade. Specifically, blocking CD39 with POM-1 significantly increased the antitumor activation of CTLA-4 mAb in a mouse model of lung metastasis, and showed better efficacy in a CD39-deficient mouse model of tumor transplanted with B16F10 (39). Recent research also showed that the expression of CTLA-4 and CD39 may be potential target molecules that inhibit Treg activity in situ (40). Although there is limited literature on the combination of CD39 mAb with CTLA-4 mAb, according to the current study, the combination of the two mAbs has great potential in tumor therapy, especially in the treatment of tumor metastasis.
CD39 mAb combined with TIGIT mAb
TIGIT is an inhibitory receptor expressed on lymphocytes that has recently attracted attention as the latest target for tumor immunotherapy. This shows the interplay between TIGHT and CD155, which is expressed on APCs or tumor cells, reducing T and NK cell function. TIGIT, a significant inhibitor of antitumor responses, blocks the tumor immune cycle in multiple steps (60–62). Several studies have shown that blocking TIGIT can prevent various solid and hematologic malignancies. In AML, inhibition of CD39 combined with TIGIT can increase AML cell lysis in 2/3 cell lines, and the combined inhibition of TIGIT and CD39 significantly improved NK cell killing activity in vitro, thus further enhancing the NK cell killing effect on AML cells (41, 42, 63). Owing to the difference in the expression of the TIGIT/PVRIG axis and CD39 in different NK cell subsets, joint blocking of these pathways may enhance the cytotoxic function of different NK cell subsets in vivo. In addition, it has been preliminarily reported that RORγ agonists can simultaneously reduce the expression of CD39, TIGIT, and other immune checkpoints on lymphocytes, and integrate multiple antitumor mechanisms into one therapy. This enhances immune activity and reduces immunosuppression, thus effectively inhibiting tumor growth (64).
Association of CD73 with immune checkpoints
CD73 is expressed in various types of cancer and is known to promote tumor growth, metastasis, and drug tolerance in glioblastoma, melanoma, leukemia, colon, breast, ovarian, and bladder cancers (13, 19, 65). In human breast cancer cells, high expression of CD73 is related to low response and high resistance to anthracyclines (44, 46, 66). High levels of CD73 are associated with immunosuppression and tumor progression. The overexpression of CD73 in tumors not only leads to metastasis of tumor cells and anthracycline resistance but also leads to immune escape because of excess adenosine production (67, 68). Therefore, inhibitors of CD73 are currently used in combination with existing cancer therapies for cancer immunotherapy, including anti-PD-1/PD-L1 and anti-CTLA-4 therapies (44, 46, 67). Although blocking CD73 alone does not result in a cure, the inhibition of CD73 increases the antitumor effect of immune checkpoint therapies, including anti-CTLA-4 and anti-PD-1 (19, 24, 67). The synergistic effects of combined CTLA-4 mAb with CD73 mAb and combined PD-1 mAb with CD73 mAb immunotherapy have been observed in preclinical models of both breast cancer and colon cancer (24, 45, 68). Currently, MEDI9447(AstrazenecaMedimmune), a human IgG1CD73 mAb (46), can selectively inhibit the activity of CD73ECN and cross-react with mouse and human CD73. MEDI9447 internalized the desetting of CD73 from the cell surface, thereby inhibiting the conversion of AMP to adenosine and removing the inhibition of T cell proliferation mediated by AMP. In an immunoactive mouse tumor model, MEDI9447 reduces immunosuppressive effects and promotes antineoplastic function (45); BMS986179, a high affinity antibody, inhibits the activity of CD73 and mediates the internalization of CD73 (19, 69); CPI-006 (also known as CPX006) acts mainly by inhibiting CD73 activity and/or inducing CD73 downregulation; IPH5301, which blocks AMP from degrading to the immunosuppressant adenosine. At present, these antibodies are undergoing early-stage clinical trials (11, 70).
CD73 mAb combined with PD-1/PD-L1 mAb
As mentioned above, CD73-derived adenosine strongly mediates tumor immune status and metastasis, and weak patient response to PD-1 antibodies may also be associated with elevated intratomatous adenosine levels. In this context, the combination of CD73, mAb, and PD-1 mAb may be particularly effective in tumor immunotherapy. Currently, various studies have focused on the clinical effects of combining CD73 inhibition with PD-1 blockade. In melanoma, breast cancer, colon cancer, non-small cell lung cancer (NSCLC), prostate cancer, and other malignant tumors (24, 43, 45), combining the CD73 mAb with the PD-1 mAb has shown a more significant effect than these drugs alone. The high expression of CD73 on the surface of tumor cells shows a weaker effect of immunotherapy with a PD-1 antibody, and the combined use of PD-1 mAb and CD73 mAb prominently inhibited tumor growth (46), and increased gene expression related to inflammation and T cell function, causing an increase in the number and activity of tumor-infiltrating CD8+T cells and the production of IFN-γ and TNF-α (20, 46). It has also been reported that MEDI9447 when combined with anti-PD-1 antibodies, can produce a better antitumor effect, which is supported by multiple phases I/II trials based on MEDI9447. Preliminary phase I data for MEDI9447(NCT02503774) have recently been reported (24).The safety of MEDI9447 and duvacizumAb (anti-PD-L1) treatment is controllable, and PD-1 is consistent with its mechanism of function. BMS-986179 was also found to enhance the antineoplastic activity of anti-PD-1mAb in preclinical animal models (19, 71). Notably, the combination of A2aR antagonist and PD-1 antibody also showed an antimetastatic effect. However, the combination therapy with A2aR antagonists was effective only when tumors expressed high CD73 levels, suggesting that CD73 can also be used as a potential tumor indicator to assess the benefit of combination therapy (13, 19, 44).
CD73 mAb combined with CTLA-4 mAb
The combination of CD73 mAb and PD-1 mAb was more effective against both subcutaneous and metastatic tumors than that of CD73 mAb and CTLA-4 mAb (24). This may be due to the stronger antineoplastic activity of PD-1 mAb itself than that of CTLA-4 mAb or the synergistic effect of CD73 mAb and PD-1 mAb on Tregs. However, CD73 mAb combined with CTLA-4 mAb still has clinical significance and cannot be ignored. CD73 mAb combined with CTLA-4 mAb significantly improved the median survival in a tumor metastasis mouse model (71). In melanoma, the efficacy of anti-CTLA-4 therapy can be enhanced by targeting various immunosuppressive mechanisms in tumor tissues, including CD73. CD73 antibody combined with CTLA-4 mAb significantly inhibited melanoma growth. In a mouse melanoma model, the percentage of infiltrated CD8+T and CD4+T cells significantly increased after the combination of the two antibodies, and the proportion of Tregs was also increased compared with that of the two antibodies alone, which may be due to the increase in CD4+T cells after the combination of the two monoclonal antibodies. At the same time, IFN-γ levels increased in melanoma tissues of mice treated with CD73 antibody in combination with CTLA-4 antibody (47). CTLA-4 mAb is also of great clinical significance in hematologic malignancies such as AML and MDS (22) and has great potential in combination with CD73 antibody in the treatment of malignant diseases of the blood.
Association of A2AR with immune checkpoints
The A2a receptor (A2aR) in the adenosinergic pathway is an important immune checkpoint. Adenosine levels in the extracellular fluid are increased in the TME because of the special metabolism of tumor cells, which contributes to tumor immune escape. Therefore, A2aR inhibitors are being investigated to enhance the effect of immunotherapy (2, 13). Several A2aR antagonists have been developed and tested in multiple preclinical studies. At least four drugs, CPI-444 (10) PBF-509 (Novartis/Pablobiofarma), MK-3814 (Merck), and AZD4635 (AstraZeneca/Heptares), are currently in phase I clinical trials (13). CPI-444 intensifies antineoplastic immunity and enhances anti-PD-L1 mAb activity in mice. CPI-444 has also been shown to intensify the antitumor effect of adoptive metastases of HER2-specific CD8+T cells in tumor-bearing mice treated with cyclophosphamide and a novel gene-expressed whole-cell vaccine (GVAX) (10). Vipatant (REDOX/Juno therapy) and Etradine (Kyowa Hakko Kirin) are other promising oral A2a antagonists that have previously gone through clinical trials for Parkinson’s disease and may be effective in cancer patients. Recent research has shown that A2aR inhibits T cell proliferation and cytokine secretion and increases the expression of PD-1 and CTLA-4 on the surface (72, 73). Currently, combination therapy with A2aR and other immune checkpoints is also attracting attention (10, 13).
A2aR mAb combined with PD-1/PD-L1 mAb
As the expression of A2a is increased in antigen-activated T cells and PD-1 is involved in inhibiting T cell function, the combination of targeted blocking of these two molecules is considered a new direction in tumor therapy. Recent clinical studies have shown that in renal cell cancer (RCC) patients, A2aR and PD-L1 expression in the primary tumors may foresee the consequences of therapy with anti-VEGF agents and ICIs (51), and the A2aR antagonist ciforadenant showed monotherapy activity in patients who were resistant to or intractable to previous anti-PD-L1 therapy. Although this trial did not deliberately compare the effects of monotherapy with those of combination therapy, treatment with an A2aR antagonist plus anti-PD-L1 appeared to improve efficacy (50). Studies have shown that blocking A2aR with CPI-444 reduces the expression of checkpoints of various pathways in T-effs and Tregs, including PD-1 and LAG-3. By reducing the expression of immune checkpoints on these T cells, the threshold for anti-PD-1 treatment is lowered. In other words, there is a synergistic reaction with the combination of CPI-444 and PD-1 mAb (10, 48). Moreover, A2aR blockers significantly reduced the expression of PD-1 and LAG-3 in the draining lymph nodes of tumor-bearing mice (53). Another group successfully combined A2aR blockers with anti-PD-1 inhibitors in an anti-tumor regimen in a mouse model (35). Mittal et al. (49) also reported that combining SCH58261, the A2aR inhibitor, with anti-PD-1 therapy significantly reduced the burden of metastasis compared with either monotherapy alone. Uniting therapy with PD-1 mAbs and CPI-444 showed significant improvement in tumor regression and survival in CT26 and MC38 tumor models (more significant in CT26 tumor models) (48). In NSCLC mouse models, A2a receptor inhibition overcomes the resistance of tumor cells to PD-1/PD-L1 blocking treatment. Meanwhile, A2aR and CD73 were upregulated in mice treated with PD-1 or PD-L1 mAbs (52, 53). In mouse models of breast, colon, and hepatocellular carcinomas, drug resistance of tumor cells to PD-1/PD-L1 can be prevented through dual blocking of PD-1 and A2aR. Blocking A2aR after a viral attack also reduced the expression of PD-1, LAG-3, and TIM-3 on CD8+T cells and Tregs. These abundant in vivo and in vitro experiments suggest that the combination of A2aR blockers and PD-1/PD-L1 antibodies is of great significance in the clinical treatment of tumors (34, 71).
A2aR mAb combined with CTLA-4 mAb
Combining A2aR mAb CPI-444 with anti-CTLA-4 therapy eliminated tumors in up to 90% of the treated mice, including restoring an immune response in a model against an incomplete response to CTLA-4 monotherapy. Moreover, tumor cells remained suppressed after re-inoculation of mice with tumor cells, suggesting that CPI-444 induces systemic antineoplastic immune memory and that the combination of CPI-444 with CTLA-4 mAb increases the presence of CD8+T cells and IFNγ and Gzm B levels in tumors (71). In a mouse melanoma model, inhibition of both CD73 and A2aR increased CTLA-4 the therapeutic effect. Blocking A2aR plays an important role in regulating T-cell function and significantly reduces melanoma growth (47). Most importantly, the combination of A2aR antagonists and anti-CTLA-4 therapy significantly restricts tumor growth and enhances the antitumor immune response (10, 71). Additionally, other studies have shown that the concomitant blocking of A2aR and CTLA-4 in T cells can synergistically enhance the antitumor response by downregulating PKA, SHP2, and PP2Aα signaling pathways, providing a theoretical basis for A2aR mAb combined with CTLA-4 mAb as a new treatment regimen for tumors (54).
A2aR mAb combined with TIGIT mAb
The frequency of TIGIT + NK cells in the blood of patients was negatively correlated with AML prognosis. Compared with healthy subjects, AML patients had abnormal NK cell populations in the peripheral blood (PB) and bone marrow (BM), which showed an increased frequency of TIGIT+, PVRIG+, CD39+, and CD69+NK cells. Thus, TIGIT is a potential target for AML treatment (42). The purinergic pathway also regulates NK cell functions. Proliferation and hypoxia of tumor cells increase the utilization of ATP and activate cancer-related CD39 and CD73, which catalyze the continuous dephosphorylation of ATP to AMP and then to eADO. Extracellular adenosine accumulation interacts with adenosine receptors expressed on the surface of NK cells and inhibits signaling through A2aR; therefore, A2aR antibodies are also important targets for tumor therapy (55). It has been demonstrated that the combined blocking of TIGIT and A2aR enhances NK-92 cell-mediated cytotoxicity in AML (42). In other tumors, the combination of TIGIT and A2aR mAbs is still being explored (55).
Comparison of the efficacy of CD39, CD73, A2AR and immune checkpoint inhibitors in clinical treatment
Studies have shown that A2AR mAb, Ciforadenant (CPI-144) has a good effect on RCC, and its combination with atezolizumab has a better effect than Ciforadenant alone (74). Taminadenant, another A2AR antagonist, has shown promising efficacy in NSCLC, either alone or in combination with the PD-1 mAb, Spartalizumab (75). There does not appear to be a significant difference in the efficacy of A2aR antagonists alone or in combination with immune checkpoint inhibitors for malignancy, but the number of clinical trials in this area is small, and the results of these two trials are not representative. The combination of A2aR antagonists and immune checkpoint inhibitors has been seriously considered in clinical practice and needs to be explored and validated in more clinical trials.
Although there are no cases of CD39 mAb used alone in clinical treatment, clinical studies have shown that the expression of CD39 in chronic lymphocytic leukemia patients is closely related to the stage of disease, the time of first treatment, and the prognosis of patients (76, 77). Similar results have been found in immune-related diseases, such as Crohn’s disease and multiple sclerosis (78, 79). Due to various limitations, treatment with CD39 mAb combined with immune checkpoints such as PD-1, CTLA-4, and TIGIT has been limited to mouse models, cell lines, and human tissues. However, this combination therapy has been proved to have high clinical potential and value by a large number of in vitro experiments (11, 37–42).
CD73 is currently mainly used as a prognostic indicator for clinical tumors, including breast and rectal cancers (23, 24, 43–47). Few trials have applied the CD73 mAb in the clinical treatment of tumors. Recent in vitro experiments have confirmed that the CD73 mAb can enhance the efficacy of immune checkpoint inhibitors and reduce the formation of resistance. Its combination with immune checkpoint inhibitors is far more effective than either of them alone (23, 24, 43–47).
The efficacy of antagonists of CD39 and CD73, A2aR targets on the adenosinergic axis alone and in combination has been discussed in a study on multiple myeloma (80). In vitro experiments confirmed that the inhibitory effect of the three target antagonists alone on myeloma was not as good as that of the two target antagonists combined, while the combined use of three target antagonists, CD39, CD73, and A2aR, had the best inhibitory effect on myeloma.
Conclusion
In recent years, therapeutic advances in cancer immunotherapy (CIT) have emerged rapidly, reflecting the importance of human immune system interactions with cancer, as well as the complex and highly regulated nature of the immune system (81, 82). In the context of complex immune networks, point-to-point therapy has been unable to achieve satisfactory tumor treatment effects; therefore, combining various targeting axes or immune checkpoints will become a new direction of tumor treatment.
The role of the adenosine axis in the tumor microenvironment is mainly induced by hypoxia; therefore, some studies have also called this the hypoxia–adenosine axis. Extracellular adenosine increases under hypoxic conditions, and simultaneously, the expression of CD39 and CD73 improves (8). Antihypoxia-adenosine therapy is synergistic with other immune checkpoint inhibitors such as CTLA-4 and PD-1 mAbs. The combination of anti-hypoxia-adenosine strategies may enhance the clinical response to other immunotherapies, chemotherapy, and radiotherapy (71). With advances in the treatment of tumors with immune checkpoint blockers such as CTLA-4 and PD-1/PDL1, more therapeutic targets have been sought, including but not limited to the immune targets in the adenosine energy axis mentioned above, to overcome the problems of incomplete tumor regression or recurrence after treatment (3). Immune checkpoint suppressor molecules are emerging as new potential targets for tumor therapy. In the TME, these molecular mechanisms may operate and may be supplementary to approved immunotherapies (26, 83, 84). When the immune escape of tumor cells makes the control of tumors difficult (85), the use of immune checkpoint inhibitors can offset the immune escape of tumor cells to a certain extent and further improve the response rate. Without increasing or even decreasing the adverse events related to excessive tissue damage, autoimmunity, and other immune-associated side reactions associated with the use of immune checkpoint inhibitors alone (22, 86–88). In the future, the treatment trend of malignant tumors will be developed from point-to-point therapy targeting individual immune checkpoints to a combination of immune networks composed of various signaling pathways, such as the adenosine axis. Non-traditional immunotherapies can induce or enhance antitumor immunity. Consequently, they may force tumors to upregulate immune checkpoints, which can be blocked as part of a combined strategy (87, 89–92).
Author contributions
ZL: Conceptualization, Writing - Review and Editing, Project administration. XL: Writing - Original Draft, Visualization, Resources. XX: Resources. HS: Resources. XZ: Resources. RF: Supervision, Funding acquisition, Project administration. All authors contributed to the article and approved the submitted version.
Funding
This work was supported by the National Natural Science Foundation of China Youth Project (grant no. 81900131,82000219), the National Natural Science Foundation of China Youth Project (grant no. 8210010129), the Tianjin Municipal Natural Science Foundation (grant no. 18JCQNJC80400, 19JCZDJC32900), the Tianjin Education Commission Research Project (grant no. 2018KJ043,20140118), the Tianjin Education Commission Research Project (grant no. 2018KJ045), and the Tianjin Science and Technology Planning Project (no. 20YFZCSY00060).Tianjin Municipal Health Commission Youth Project (grant no. TJWJ2021QN001);Medjaden Academy & Research Foundation for Young Scientists (Grant No. MJR20221011);Tianjin Key Medical Discipline(Specialty) Construction project(Grant TJYXZDXK-028A).
Conflict of interest
The authors declare that the research was conducted in the absence of any commercial or financial relationships that could be construed as a potential conflict of interest.
Publisher’s note
All claims expressed in this article are solely those of the authors and do not necessarily represent those of their affiliated organizations, or those of the publisher, the editors and the reviewers. Any product that may be evaluated in this article, or claim that may be made by its manufacturer, is not guaranteed or endorsed by the publisher.
References
1. Ohta A. A metabolic immune checkpoint: Adenosine in tumor microenvironment. Front Immunol (2016) 7:109. doi: 10.3389/fimmu.2016.00109
2. Boison D, Yegutkin GG. Adenosine metabolism: Emerging concepts for cancer therapy. Cancer Cell (2019) 36(6):582–96. doi: 10.1016/j.ccell.2019.10.007
3. Allard B, Allard D, Buisseret L, Stagg J. The adenosine pathway in immuno-oncology. Nat Rev Clin Oncol (2020) 17(10):611–29. doi: 10.1038/s41571-020-0382-2
4. Guo S, Han F, Zhu W. CD39 - a bright target for cancer immunotherapy. BioMed Pharmacother (2022) 151:113066. doi: 10.1016/j.biopha.2022.113066
5. Antonioli L, Blandizzi C, Pacher P, Haskó G. Immunity, inflammation and cancer: a leading role for adenosine. Nat Rev Cancer (2013) 13(12):842–57. doi: 10.1038/nrc3613
6. Stagg J, Smyth MJ. Extracellular adenosine triphosphate and adenosine in cancer. Oncogene (2010) 29(39):5346–58. doi: 10.1038/onc.2010.292
7. Beavis PA, Stagg J, Darcy PK, Smyth MJ. CD73: A potent suppressor of antitumor immune responses. Trends Immunol (2012) 33(5):231–7. doi: 10.1016/j.it.2012.02.009
8. Scheffel TB, Grave N, Vargas P, Diz FM, Rockenbach L, Morrone FB. Immunosuppression in gliomas via PD-1/PD-L1 axis and adenosine pathway. Front Oncol (2020) 10:617385. doi: 10.3389/fonc.2020.617385
9. Ohta A, Gorelik E, Prasad SJ, Ronchese F, Lukashev D, Wong MKK, et al. A2A adenosine receptor protects tumors from antitumor T cells. Proc Natl Acad Sci USA (2006) 103(35):13132–7. doi: 10.1073/pnas.0605251103
10. Willingham SB, Ho PY, Hotson A, Hill C, Piccione EC, Hsieh J, et al. A2AR antagonism with CPI-444 induces antitumor responses and augments efficacy to anti-PD-(L)1 and anti-CTLA-4 in preclinical models. Cancer Immunol Res (2018) 6(10):1136–49. doi: 10.1158/2326-6066.CIR-18-0056
11. Perrot I, Michaud H-A, Giraudon-Paoli M, Augier S, Docquier A, Gros L, et al. Blocking antibodies targeting the CD39/CD73 immunosuppressive pathway unleash immune responses in combination cancer therapies. Cell Rep (2019) 27(8):2411–2425.e9. doi: 10.1016/j.celrep.2019.04.091
12. Chew V, Toh HC, Abastado JP. Immune microenvironment in tumor progression: Characteristics and challenges for therapy. J Oncol 2012 (2012) p:608406. doi: 10.1155/2012/608406
13. Leone RD, Emens LA. Targeting adenosine for cancer immunotherapy. J Immunother Cancer (2018) 6(1):57. doi: 10.1186/s40425-018-0360-8
14. Antonioli L, Pacher P, Vizi ES, Haskó G. CD39 and CD73 in immunity and inflammation. Trends Mol Med (2013) 19(6):355–67. doi: 10.1016/j.molmed.2013.03.005
15. Maliszewski CR, Delespesse GJ, Schoenborn MA, Armitage RJ, Fanslow WC, Nakajima T, et al. The CD39 lymphoid cell activation antigen. Molecular cloning and structural characterization. J Immunol (1994) 153(8):3574–83.
16. Bonnefoy N, Bastid J, Alberici G, Bensussan A, Eliaou J-F. CD39: A complementary target to immune checkpoints to counteract tumor-mediated immunosuppression. Oncoimmunology (2015) 4(5):e1003015. doi: 10.1080/2162402X.2014.1003015
17. Solyom A, Trams EG. Enzyme markers in characterization of isolated plasma membranes. Enzyme (1972) 13(5-6):329–72. doi: 10.1159/000459682
18. Strater N. Ecto-5’-nucleotidase: Structure function relationships. Purinergic Signal (2006) 2(2):343–50. doi: 10.1007/s11302-006-9000-8
19. Chen S, Wainwright DA, Wu JD, Wan Y, Matei DE, Zhang Y, et al. CD73: An emerging checkpoint for cancer immunotherapy. Immunotherapy (2019) 11(11):983–97. doi: 10.2217/imt-2018-0200
20. Ghisoni G, Genta S, Scotto G, Tuninetti V, Turinetto M, et al. Immuno-metabolism and microenvironment in cancer: Key players for immunotherapy. Int J Mol Sci (2020) 21(12). doi: 10.3390/ijms21124414
21. Baghbani E, Noorolyai S, Shanehbandi D, Mokhtarzadeh A, Aghebati-Maleki L, Shahgoli VK, et al. Regulation of immune responses through CD39 and CD73 in cancer: Novel checkpoints. Life Sci (2021) 282:119826. doi: 10.1016/j.lfs.2021.119826
22. Kantarjian H, Garcia-Manero G, Allison J, Sharma P, Daver N. The emerging role of immune checkpoint based approaches in AML and MDS. Leuk Lymphoma (2018) 59(4):790–802. doi: 10.1080/10428194.2017.1344905
23. Antonia SJ, Vansteenkiste JF, Moon E. Immunotherapy: Beyond anti-PD-1 and anti-PD-L1 therapies. Am Soc Clin Oncol Educ Book (2016) 35:e450–8. doi: 10.1200/EDBK_158712
24. Allard B, Pommey S, Smyth MJ, Stagg J. Targeting CD73 enhances the antitumor activity of anti-PD-1 and anti-CTLA-4 mAbs. Clin Cancer Res (2013) 19(20):5626–35. doi: 10.1158/1078-0432.CCR-13-0545
25. Khair DO, Bax HJ, Mele S, Crescioli S, Pellizzari G, Khiabany A, et al. Combining immune checkpoint inhibitors: Established and emerging targets and strategies to improve outcomes in melanoma. Front Immunol (2019) 10:453. doi: 10.3389/fimmu.2019.00453
26. Li B, Chan HL, Chen P. Immune checkpoint inhibitors: Basics and challenges. Curr Med Chem (2019) 26(17):3009–25. doi: 10.2174/0929867324666170804143706
27. DePeaux K, Delgoffe GM. Metabolic barriers to cancer immunotherapy. Nat Rev Immunol (2021) 21(12):785–97. doi: 10.1038/s41577-021-00541-y
28. Rowshanravan B, Halliday N, Sansom DM. CTLA-4: a moving target in immunotherapy. Blood (2018) 131(1):58–67. doi: 10.1182/blood-2017-06-741033
29. Joller N, Kuchroo VK. Tim-3, lag-3, and TIGIT. Curr Top Microbiol Immunol (2017) 410:127–56. doi: 10.1007/82_2017_62
30. Zhang X, Li E, Zhang G, Wang X, Tang X, et al. VISTA: an immune regulatory protein checking tumor and immune cells in cancer immunotherapy. J Hematol Oncol (2020) 13(1):83. doi: 10.1186/s13045-020-00917-y
31. Allard D, Allard B, Stagg J. On the mechanism of anti-CD39 immune checkpoint therapy. J Immunother Cancer (2020) 8(1). doi: 10.1136/jitc-2019-000186
32. Salik B, Smyth MJ, Nakamura K. Targeting immune checkpoints in hematological malignancies. J Hematol Oncol (2020) 13(1):111. doi: 10.1186/s13045-020-00947-6
33. Wang H, Kaur G, Sankin AI, Chen F, Guan F, Zang X, et al. Immune checkpoint blockade and CAR-T cell therapy in hematologic malignancies. J Hematol Oncol (2019) 12(1):59. doi: 10.1186/s13045-019-0746-1
34. Helms RS, Powell JD. Rethinking the adenosine-A2AR checkpoint: implications for enhancing anti-tumor immunotherapy. Curr Opin Pharmacol (2020) 53:77–83. doi: 10.1016/j.coph.2020.07.003
35. Allard D, Turcotte M, Stagg J. Targeting A2 adenosine receptors in cancer. Immunol Cell Biol (2017) 95(4):333–9. doi: 10.1038/icb.2017.8
36. Vijayan D, Young A, Teng MWL, Smyth MJ. Targeting immunosuppressive adenosine in cancer. Nat Rev Cancer (2017) 17(12):709–24. doi: 10.1038/nrc.2017.86
37. Lu J, Zhang P, Huang X, Guo X, Gao C, Zeng H, et al. Amplification of spatially isolated adenosine pathway by tumor-macrophage interaction induces anti-PD1 resistance in hepatocellular carcinoma. J Hematol Oncol (2021) 14(1):200. doi: 10.1186/s13045-021-01207-x
38. Tøndell A, Wahl SGF, Sponaas A-M, Sørhaug S, Børset M, Haug M, et al. Ectonucleotidase CD39 and checkpoint signalling receptor programmed death 1 are highly elevated in intratumoral immune cells in non-small-cell lung cancer. Transl Oncol (2020) 13(1):17–24. doi: 10.1016/j.tranon.2019.09.003
39. Zhang H, Vijayan D, Li X-Y, Robson SC, Geetha N, Teng MWL. The role of NK cells and CD39 in the immunological control of tumor metastases. Oncoimmunology (2019) 8(6):e1593809. doi: 10.1080/2162402X.2019.1593809
40. Jie H-B, Gildener-Leapman N, Li J, Srivastava RM, Gibson SP, Whiteside TL, et al. Intratumoral regulatory T cells upregulate immunosuppressive molecules in head and neck cancer patients. Br J Cancer (2013) 109(10):2629–35. doi: 10.1038/bjc.2013.645
41. Brauneck F, Haag F, Woost R, Wildner N, Tolosa E, Rissiek A, et al. Increased frequency of TIGIT(+)CD73-CD8(+) T cells with a TOX(+) TCF-1low profile in patients with newly diagnosed and relapsed AML. Oncoimmunology (2021) 10(1):1930391. doi: 10.1080/2162402X.2021.1930391
42. Brauneck F, Seubert E, Wellbrock J, Schulze Zur Wiesch J, Duan Y, Magnus T, et al. Combined blockade of TIGIT and CD39 or A2AR enhances NK-92 cell-mediated cytotoxicity in AML. Int J Mol Sci (2021) 22(23). doi: 10.3390/ijms222312919
43. Liu S, Liu D, Wang H, Horecny I, Shen R, Zhang R, et al. A novel CD73 inhibitor SHR170008 suppresses adenosine in tumor and enhances anti-tumor activity with PD-1 blockade in a mouse model of breast cancer. Onco Targets Ther (2021) 14:4561–74. doi: 10.2147/OTT.S326178
44. Neo SY, Yang Y, Record J, Ma R, Chen X, Chen Z, et al. CD73 immune checkpoint defines regulatory NK cells within the tumor microenvironment. J Clin Invest (2020) 130(3):1185–98. doi: 10.1172/JCI128895
45. Hay CM, Sult E, Huang Q, Mulgrew K, Fuhrmann SR, McGlinchey KA. Targeting CD73 in the tumor microenvironment with MEDI9447. Oncoimmunology (2016) 5(8):e1208875. doi: 10.1080/2162402X.2016.1208875
46. Wurm M, Schaaf O, Reutner K, Ganesan R, Mostböck S, Pelster C, et al. A novel antagonistic CD73 antibody for inhibition of the immunosuppressive adenosine pathway. Mol Cancer Ther (2021) 20(11):2250–61. doi: 10.1158/1535-7163.MCT-21-0107
47. Iannone R, Miele L, Maiolino P, Pinto A, Morello S. Adenosine limits the therapeutic effectiveness of anti-CTLA4 mAb in a mouse melanoma model. Am J Cancer Res (2014) 4(2):172–81.
48. Leone RD, Sun I-M, Oh MH, Sun IH, Wen J, Englert J, et al. Inhibition of the adenosine A2a receptor modulates expression of T cell coinhibitory receptors and improves effector function for enhanced checkpoint blockade and ACT in murine cancer models. Cancer Immunol Immunother (2018) 67(8):1271–84. doi: 10.1007/s00262-018-2186-0
49. Mittal D, Young A, Stannard K, Yong M, Teng MWL, Allard B, et al. Antimetastatic effects of blocking PD-1 and the adenosine A2A receptor. Cancer Res (2014) 74(14):3652–8. doi: 10.1158/0008-5472.CAN-14-0957
50. Fong L, Hotson A, Powderly JD, Sznol M, Heist RS, Choueiri TK, et al. Adenosine 2A receptor blockade as an immunotherapy for treatment-refractory renal cell cancer. Cancer Discov (2020) 10(1):40–53. doi: 10.1158/2159-8290.CD-19-0980
51. Kamai T, Kijima T, Tsuzuki T, Nukui A, Abe H, Arai K. Increased expression of adenosine 2A receptors in metastatic renal cell carcinoma is associated with poorer response to anti-vascular endothelial growth factor agents and anti-PD-1/Anti-CTLA4 antibodies and shorter survival. Cancer Immunol Immunother (2021) 70(7):2009–21. doi: 10.1007/s00262-020-02843-x
52. Beavis PA, Henderson MA, Giuffrida L, Mills JK, Sek K, Cross RS, et al. Targeting the adenosine 2A receptor enhances chimeric antigen receptor T cell efficacy. J Clin Invest (2017) 127(3):929–41. doi: 10.1172/JCI89455
53. Beavis PA, Milenkovski N, Henderson MA, John LB, Allard B, Loi S, et al. Adenosine receptor 2A blockade increases the efficacy of anti-PD-1 through enhanced antitumor T-cell responses. Cancer Immunol Res (2015) 3(5):506–17. doi: 10.1158/2326-6066.CIR-14-0211
54. Ghasemi-Chaleshtari M, Kiaie SH, Irandoust M, Karami H, Afjadi MN, Ghani S, et al. Concomitant blockade of A2AR and CTLA-4 by siRNA-loaded polyethylene glycol-chitosan-alginate nanoparticles synergistically enhances antitumor T-cell responses. J Cell Physiol (2020) 235(12):10068–80. doi: 10.1002/jcp.29822
55. Davern M, Donlon NE, Sheppard A, O'Connell F, Hayes C, Bhardwaj A. Chemotherapy regimens induce inhibitory immune checkpoint protein expression on stem-like and senescent-like oesophageal adenocarcinoma cells. Transl Oncol (2021) 14(6):101062. doi: 10.1016/j.tranon.2021.101062
56. Sun X, Wu Y, Gao W, Enjyoji K, Muller CE, Murakami T. CD39/ENTPD1 expression by CD4+Foxp3+ regulatory T cells promotes hepatic metastatic tumor growth in mice. Gastroenterology (2010) 139(3):1030–40. doi: 10.1053/j.gastro.2010.05.007
57. Gupta PK, Godec J, Wolski D, Adland E, Yates K, Pauken KE, et al. CD39 expression identifies terminally exhausted CD8+ T cells. PloS Pathog (2015) 11(10):e1005177. doi: 10.1371/journal.ppat.1005177
58. Nikolova M, Carriere M, Jenabian M-A, Limou S, Younas M, Kok A. CD39/adenosine pathway is involved in AIDS progression. PloS Pathog (2011) 7(7):e1002110. doi: 10.1371/journal.ppat.1002110
59. Jalali S, Price-Troska T, Paludo J, Villasboas J, Kim Yang H-J Z-Z. Soluble PD-1 ligands regulate T-cell function in waldenstrom macroglobulinemia. Blood Adv (2018) 2(15):1985–97. doi: 10.1182/bloodadvances.2018021113
60. Anderson AC, Joller N, Kuchroo VK. Lag-3, Tim-3, and TIGIT: Co-inhibitory receptors with specialized functions in immune regulation. Immunity (2016) 44(5):989–1004. doi: 10.1016/j.immuni.2016.05.001
61. Johnston RJ, Comps-Agrar L, Hackney J, Yu X, Huseni M, Yang Y, et al. The immunoreceptor TIGIT regulates antitumor and antiviral CD8(+) T cell effector function. Cancer Cell (2014) 26(6):923–37. doi: 10.1016/j.ccell.2014.10.018
62. Dougall WC, Kurtulus S, Smyth MJ, Anderson AC. TIGIT and CD96: new checkpoint receptor targets for cancer immunotherapy. Immunol Rev (2017) 276(1):112–20. doi: 10.1111/imr.12518
63. Harjunpaa H, Guillerey C. TIGIT as an emerging immune checkpoint. Clin Exp Immunol (2020) 200(2):108–19. doi: 10.1111/cei.13407
64. Hu X, Liu X, Moisan J, Wang Y, Lesch CA, Spooner C, et al. Synthetic RORgamma agonists regulate multiple pathways to enhance antitumor immunity. Oncoimmunology (2016) 5(12):e1254854. doi: 10.1080/2162402X.2016.1254854.
65. Kong Y, Jia B, Zhao C, Claxton DF, Sharma A, Annageldiyev C, et al. Downregulation of CD73 associates with T cell exhaustion in AML patients. J Hematol Oncol (2019) 12(1):40. doi: 10.1186/s13045-019-0728-3
66. Loi S, Pommey S, Haibe-Kains B, Beavis PA, Darcy PK, Smyth MJ, et al. CD73 promotes anthracycline resistance and poor prognosis in triple negative breast cancer. Proc Natl Acad Sci USA (2013) 110(27):11091–6. doi: 10.1073/pnas.1222251110
67. Antonioli L, Yegutkin GG, Pacher P, Blandizzi C, Haskó G. Anti-CD73 in cancer immunotherapy: Awakening new opportunities. Trends Cancer (2016) 2(2):95–109. doi: 10.1016/j.trecan.2016.01.003
68. Roh M, Wainwright DA, Wu JD, Wan Y, Zhang B. Targeting CD73 to augment cancer immunotherapy. Curr Opin Pharmacol (2020) 53:66–76. doi: 10.1016/j.coph.2020.07.001
69. Siu LL, Burris H, Le DT, Hollebecque A, Steeghs N, Delord JP, et al. Preliminary phase 1 profile of BMS-986179, an anti-CD73 antibody, in combination with nivolumab in patients with advanced solid tumors. Cancer Res (2018) 78(13). doi: 10.1158/1538-7445
70. Barnhart BC, Sega E, Yamniuk A, Hatcher S, Lei M, Ghermazien H, et al. A therapeutic antibody that inhibits CD73 activity by dual mechanisms. Cancer Res (2016) 76. doi: 10.1158/1538-7445.Am2016-1476.
71. Vigano S, Alatzoglou D, Irving M, Ménétrier-Caux C, Caux C, Romero P, et al. Targeting adenosine in cancer immunotherapy to enhance T-cell function. Front Immunol (2019) 10:925. doi: 10.3389/fimmu.2019.00925
72. Ohta A, Ohta Akiko, Madasu M, Kini R, Subramanian M, Goel N, et al. A2A adenosine receptor may allow expansion of T cells lacking effector functions in extracellular adenosine-rich microenvironments. J Immunol (2009) 183(9):5487–93. doi: 10.4049/jimmunol.0901247
73. Sevigny CP, Li L, Awad AS, Huang L, McDuffie M, Linden J, et al. Activation of adenosine 2A receptors attenuates allograft rejection and alloantigen recognition. J Immunol (2007) 178(7):4240–9. doi: 10.4049/jimmunol.178.7.4240
74. Fong L, Hotson A, Powderly JD, Sznol M, Heist RS, Choueiri TK, et al. Adenosine 2A receptor blockade as an immunotherapy for treatment-refractory renal cell cancer. Cancer Discov (2020) 10(1):40–53. doi: 10.1158/2159-8290.CD-19-0980
75. Chiappori AA, Creelan B, Tanvetyanon T, Gray JE, Haura EB, Thapa R, et al. Phase I study of taminadenant (PBF509/NIR178), an adenosine 2A receptor antagonist, with or without spartalizumab (PDR001), in patients with advanced non-small cell lung cancer. Clin Cancer Res (2022) 28(11):2313–20. doi: 10.1158/1078-0432.CCR-21-2742
76. Zaki EM, Zahran AM, Metwaly AA, Hafez R, Hussein S, Mohammed AE, et al. Impact of CD39 expression on CD4+ T lymphocytes and 6q deletion on outcome of patients with chronic lymphocytic leukemia. Hematol Oncol Stem Cell Ther (2019) 12(1):26–31. doi: 10.1016/j.hemonc.2018.09.002
77. Perry C, Hazan-Halevy I, Kay S, Cipok M, Grisaru D, Deutsch V, et al. Increased CD39 expression on CD4(+) T lymphocytes has clinical and prognostic significance in chronic lymphocytic leukemia. Ann Hematol (2012) 91(8):1271–9. doi: 10.1007/s00277-012-1425-2
78. Bai A, Moss A, Kokkotou E, Usheva A, Sun X, Cheifetz A, et al. CD39 and CD161 modulate Th17 responses in crohn’s disease. J Immunol (2014) 193(7):3366–77. doi: 10.4049/jimmunol.1400346
79. Visweswaran M, Hendrawan K, Massey JC, Khoo ML, Ford CD, Zaunders JJ, et al. Sustained immunotolerance in multiple sclerosis after stem cell transplant. Ann Clin Transl Neurol (2022) 9(2):206–20. doi: 10.1002/acn3.51510
80. Yang R, Elsaadi S, Misund K, Abdollahi P, Vandsemb EN, Moen SH, et al. Conversion of ATP to adenosine by CD39 and CD73 in multiple myeloma can be successfully targeted together with adenosine receptor A2A blockade. J Immunother Cancer (2020) 8(1). doi: 10.1136/jitc-2020-000610
81. Hegde PS, Chen DS. Top 10 challenges in cancer immunotherapy. Immunity (2020) 52(1):17–35. doi: 10.1016/j.immuni.2019.12.011
82. Galon J, Bruni D. Approaches to treat immune hot, altered and cold tumours with combination immunotherapies. Nat Rev Drug Discov (2019) 18(3):197–218. doi: 10.1038/s41573-018-0007-y
83. Johnson DE, O’Keefe RA, Grandis JR. Targeting the IL-6/JAK/STAT3 signalling axis in cancer. Nat Rev Clin Oncol (2018) 15(4):234–48. doi: 10.1038/nrclinonc.2018.8
84. Llovet JM, Montal R, Sia D, Finn RS. Molecular therapies and precision medicine for hepatocellular carcinoma. Nat Rev Clin Oncol (2018) 15(10):599–616. doi: 10.1038/s41571-018-0073-4
85. Hinshaw DC, Shevde LA. The tumor microenvironment innately modulates cancer progression. Cancer Res (2019) 79(18):4557–66. doi: 10.1158/0008-5472.CAN-18-3962
86. Zhang Y, Zheng J. Functions of immune checkpoint molecules beyond immune evasion. Adv Exp Med Biol 2020 (1248) p:201–26.
87. Yap TA, Parkes EE, Peng W, Moyers JT, Curran MA, Tawbi HA, et al. Development of immunotherapy combination strategies in cancer. Cancer Discov (2021) 11(6):1368–97. doi: 10.1158/2159-8290.CD-20-1209
88. Eschweiler S, Clarke J, Ramírez-Suástegui C, Panwar B, Madrigal A, Chee SJ, et al. Intratumoral follicular regulatory T cells curtail anti-PD-1 treatment efficacy. Nat Immunol (2021) 22(8):1052–63. doi: 10.1038/s41590-021-00958-6
89. Pardoll DM. The blockade of immune checkpoints in cancer immunotherapy. Nat Rev Cancer (2012) 12(4):252–64. doi: 10.1038/nrc3239
90. Bassez A, Vos H, Dyck LV, Floris G, Arijs I, Desmedt C, et al. A single-cell map of intratumoral changes during anti-PD1 treatment of patients with breast cancer. Nat Med (2021) 27(5):820–32. doi: 10.1038/s41591-021-01323-8
91. Simon B, Harrer DC, Thirion C, Schuler-Thurner B, Schuler G, Uslu U, et al. Enhancing lentiviral transduction to generate melanoma-specific human T cells for cancer immunotherapy. J Immunol Methods (2019) 472:55–64. doi: 10.1016/j.jim.2019.06.015
Keywords: adenosine, CD39, CD73, adenosine receptor 2A, PD-1, CTLA- 4
Citation: Liu Z, Liu X, Shen H, Xu X, Zhao X and Fu R (2022) Adenosinergic axis and immune checkpoint combination therapy in tumor: A new perspective for immunotherapy strategy. Front. Immunol. 13:978377. doi: 10.3389/fimmu.2022.978377
Received: 26 June 2022; Accepted: 23 August 2022;
Published: 08 September 2022.
Edited by:
Xuyao Zhang, Fudan University, ChinaCopyright © 2022 Liu, Liu, Shen, Xu, Zhao and Fu. This is an open-access article distributed under the terms of the Creative Commons Attribution License (CC BY). The use, distribution or reproduction in other forums is permitted, provided the original author(s) and the copyright owner(s) are credited and that the original publication in this journal is cited, in accordance with accepted academic practice. No use, distribution or reproduction is permitted which does not comply with these terms.
*Correspondence: Rong Fu, ZnVyb25nODM2OUB0bXUuZWR1LmNu
†These authors have contributed equally to this work