- Instituto de Medicina Molecular João Lobo Antunes, Faculdade de Medicina, Universidade de Lisboa, Lisboa, Portugal
In 1967, pioneering work by Ruth Nussenzweig demonstrated for the first time that irradiated sporozoites of the rodent malaria parasite Plasmodium berghei protected mice against a challenge with infectious parasites of the same species. This remarkable finding opened up entirely new prospects of effective vaccination against malaria using attenuated sporozoites as immunization agents. The potential for whole-sporozoite-based immunization in humans was established in a clinical study in 1973, when a volunteer exposed to X-irradiated P. falciparum sporozoites was found to be protected against malaria following challenge with a homologous strain of this parasite. Nearly five decades later, much has been achieved in the field of whole-sporozoite malaria vaccination, and multiple reports on the clinical evaluation of such candidates have emerged. However, this process has known different paces before and after the turn of the century. While only a few clinical studies were published in the 1970’s, 1980’s and 1990’s, remarkable progress was made in the 2000’s and beyond. This article reviews the history of the clinical assessment of whole-sporozoite malaria vaccines over the last forty-nine years, highlighting the impressive achievements made over the last few years, and discussing some of the challenges ahead.
Introduction
The 6 October 2021 will be forever engraved in the history of the fight against malaria as the date when RTS,S, the first vaccine against this devastating disease, was recommended by the World Health Organization (WHO) to be given to children living in regions with moderate-to-high transmission of Plasmodium falciparum (Pf) malaria. RTS,S, a subunit vaccine based on the Pf circumsporozoite protein (CSP), was initially developed by the Walter Reed Army Institute of Research (WRAIR) and GlaxoSmithKline (GSK), in 1987. A long path followed, during which the vaccine was evaluated in multiple clinical trials in malaria-endemic regions, leading to its eventual endorsement. Immunogenicity studies have indicated that RTS,S exerts its protective effect through antibodies against PfCSP and through CD4+ T cell responses, but no clear immune correlates of protection have been identified (1, 2). Results from a large Phase III clinical study have shown that 4 doses of the vaccine present relatively modest and rapidly waning 25.9% and 17.3% effectiveness against clinical and severe malaria, respectively, in newborns aged 6–12 weeks, and 36.3% and 32.2% efficiency against clinical and severe malaria, respectively, in children aged 5–17 months [(3) and reviewed in (4)]. A post-approval plan comprising 4 complementary Phase IV studies that will evaluate safety, effectiveness and impact of RTS,S in the context of its real-life implementation will support the ongoing evaluation of the vaccine’s benefit-risk and inform decision-making for its potential wider implementation across sub-Saharan Africa (5). Moreover, RTS,S is not expected to protect against the other human malaria parasites, namely P. vivax (Pv), P. ovale, P. malariae, and the zoonotic P. knowlesi (6). Thus, in spite of this landmark achievement, the licensing of RTS,S should not be viewed as the end of the road in the quest for a malaria vaccine. Rather, it should be seen as a stepping stone towards the WHO’s ambitious goals of, by 2030, licensing vaccines targeting Pf and Pv with protective efficacy of at least 75 percent against clinical malaria and that substantially reduce the incidence of human malaria infection (7).
Whole-sporozoite (WSp) vaccines (Figure 1) have emerged as a possible strategy to immunize against malaria since the demonstration that X-irradiated sporozoites of P. berghei (Pb) could induce protective immune responses against an intravenous challenge with fully infective Pb parasites (8). Interest in WSp vaccination increased following the initial demonstration by Clyde et al. that radiation-attenuated Pf sporozoites could also afford protective immunity against homologous Pf malaria (9). However, for a long time, WSp vaccination was considered impractical, and the barriers to the development of WSp vaccines seemed all but insurmountable (10). Nevertheless, research into this area gained momentum in the early 2000’s and, one by one, many of these barriers were overcome, through the efforts of several laboratories around the world and, pivotally, by the remarkable technological and scientific progress made by Stephen L. Hoffman’s team at Sanaria, Inc. and its network of collaborators.
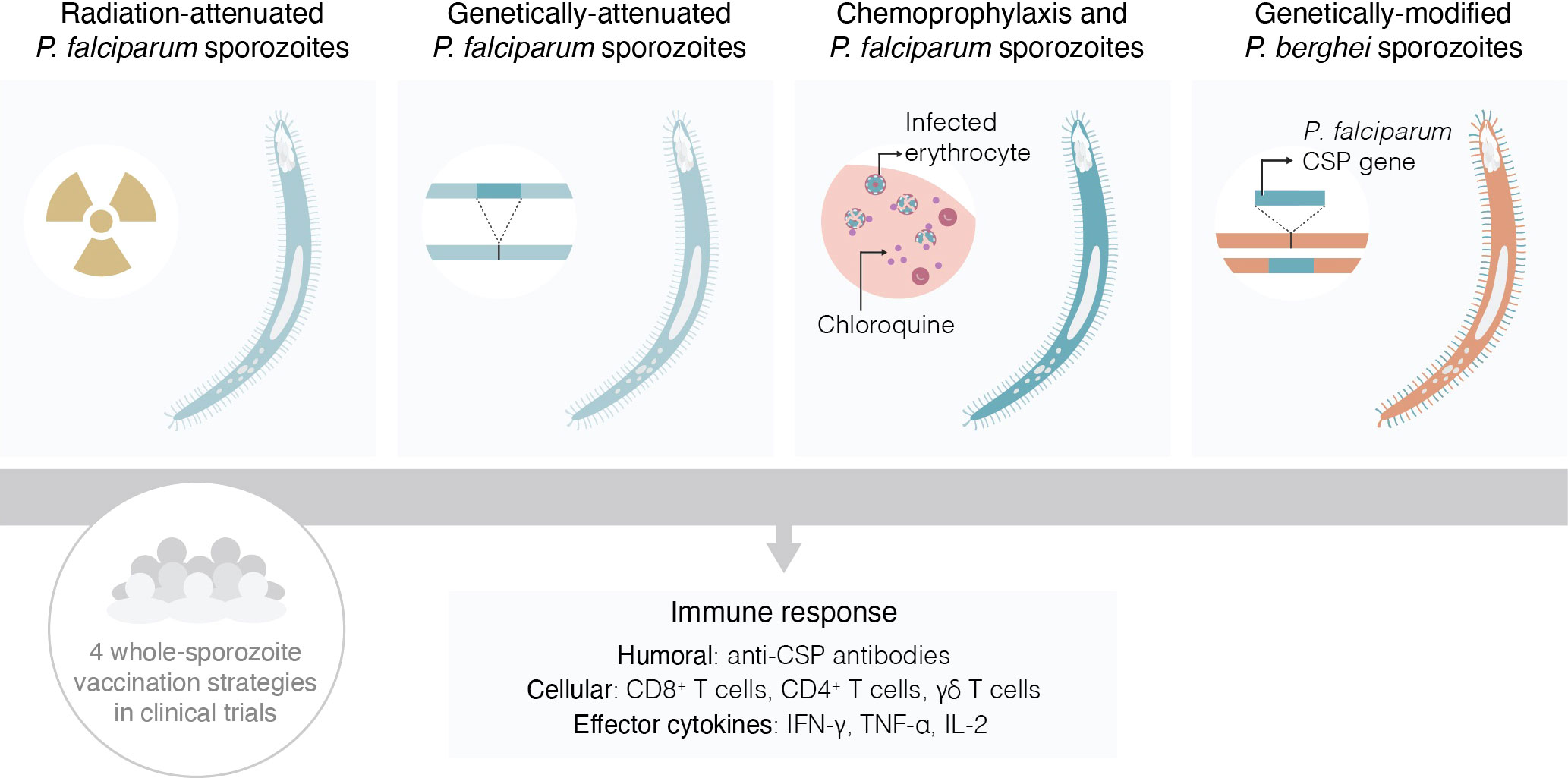
Figure 1 Schematic representation of the four types of whole-sporozoite vaccines against malaria assessed in clinical trials.
Nearly five decades have elapsed since the first clinical assessment of a WSp vaccine by Clyde et al., in 1973 (9). Whereas throughout the first 3 decades of this period such trials involved a total of only about two dozen human subjects (10, 11), this number has risen exponentially since then, generating an impressive amount of data on the immunogenicity and protective efficacy of WSp vaccines in humans (Figure 2). Here, we review the knowledge accumulated through these clinical studies, at a time when the prospect of WSp vaccines becoming a reality in a not-so-distant future seems more realistic than ever.
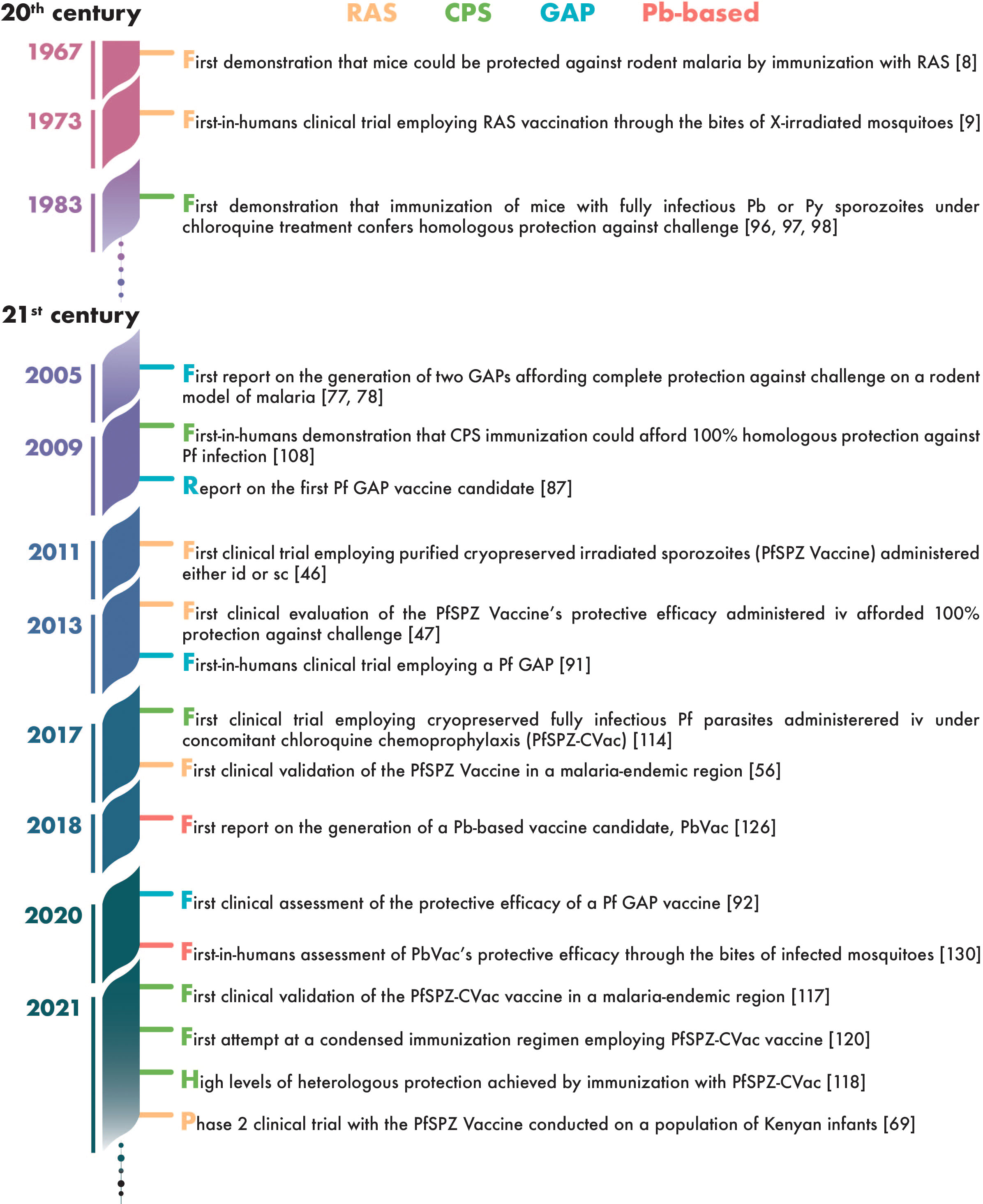
Figure 2 Timeline of landmark achievements in the development of whole-sporozoite vaccines against malaria.
Clinical evaluation of whole-sporozoite vaccines
Controlled human malaria infection
The widely used term Controlled Human Malaria Infection (CHMI) is technically incorrect, since, as McFadden eloquently states, “malaria is a disease, not an organism” (12). As such, describing infection by Plasmodium parasites as “malaria infections” is no more right than referring to HIV infections as “AIDS infections” or to SARS-CoV-2 infections as “COVID-19 infections”. However, the term CHMI appears to have been adopted by the community and, since it seems unlikely that it will be replaced by the more accurate “Controlled Human Infection by Malaria Parasites” (CHIMP) or “Controlled Human Plasmodium Infection” (CHPI), will be employed throughout this review.
CHMI is of paramount importance in the context of malaria vaccinology, as amply discussed in several reviews (13–20). Both early and recent studies aimed at assessing WSp vaccine candidates in the clinic have resorted to CHMI, employing the strictly controlled exposure of trial participants to the bites of laboratory-reared, Plasmodium-infected mosquitoes (21). CHMI by the bites of five mosquitoes consistently infects all malaria-naïve volunteers (22), although exposure to the bites of 3 aseptically-raised Pf-infected mosquitoes has also been proposed as a safe, effective procedure for CHMI in malaria-naïve adults (23). While the NF54 strain of Pf is most commonly employed for CHMI by mosquito bite, the 7G8, NF135.C10 and NF166.C8 Pf strains have also been reported as eligible for use in such studies (24). An alternative to mosquito bite-based CHMI lies in the use of Sanaria, Inc.’s PfSPZ Challenge, consisting of infectious, aseptic, purified, vialed, cryopreserved Pf sporozoites, which can be administered by needle and syringe (25). Dose-finding trials have shown that intravenous (iv) injection of 3200 PfSPZ Challenge leads to a geometric mean pre-patent period similar to that observed following the bites of 5 Pf-infected mosquitoes (26). Whether CHMI by mosquito bite is preferable to the iv route, or vice-versa, remains a matter of some controversy. While the former is the more natural route of infection, it does not allow the exact estimation of the number of inoculated sporozoites. Nevertheless, efforts have been made to standardize mosquito-administered CHMI (27), reducing the impact of this biological variability. On the other hand, PfSPZ Challenge enhances access to CHMI, including in malaria-endemic regions [(28, 29) and recently reviewed in (30)], which otherwise would be limited to the few research facilities with the capability to carry out Pf infections of mosquitoes for experimental purposes (25).
Radiation-attenuated sporozoites
An appropriate dose of ionizing radiation (UV, X-ray and γ) can prevent replication of a pathogenic organism, while preserving metabolic activity (31). Radiation-attenuated Plasmodium sporozoites (RAS) retain their ability to infect liver cells but are unable to replicate and progress to form erythrocyte-infectious merozoites, likely as a result of extensive DNA damage, accompanied by downregulation of DNA repair genes (32). In 1967, Ruth Nussenzweig and colleagues reported for the first time that mice could be protected against rodent malaria by immunization with RAS (8). Publication of this report created hope that humans could be completely protected against malaria, inspiring others to explore the prospect of WSp immunization in the clinic (33). To this day, RAS remain the gold-standard of whole-organism vaccination against human malaria.
Early studies of RAS immunization in humans
Inspired by Ruth Nussenzweig’s pioneering report, in 1973, a team at the University of Maryland School of Medicine commenced trials to vaccinate human volunteers with Pf RAS, delivered by the bites of X-irradiated mosquitoes. In the first report of these studies, one of three volunteers fed on by 379 mosquitoes over the course of 84 days did not develop malaria following an infective challenge with sporozoites delivered by non-irradiated mosquitoes 15 days after the last immunization (9). This volunteer then underwent an additional 5 immunization sessions, during which he was exposed to a total of 819 irradiated mosquitoes, and remained protected against a second infectious sporozoite challenge 12 days after the last immunization (9). These observations constitute the first demonstration of the protective efficacy of RAS vaccination in the clinic. Interestingly, having remained malaria-free for 2 months after the second sporozoite challenge, the same volunteer was challenged by intravenous injection of Pf trophozoites, and developed parasitemia and clinical symptoms 5 days later (9). Although the authors may not have fully realized this at the time, this was also the first indication that the protection afforded by WSp vaccination is purely restricted to the pre-erythrocytic stage of Plasmodium infection. A subsequent report describes the first RAS immunization against both Pf and Pv through the bites of irradiated mosquitoes infected with either of these parasites. A single volunteer received immunizing doses of Pf or Pv on different days and at different intervals, and was subsequently challenged with infectious parasites of either species delivered by non-irradiated mosquitoes. The experimental setup employed might be considered less than appropriate nowadays, particularly considering the small number of study participants, the irregular immunization schedules, and the concomitant use of both human parasites. The subject underwent immunization with Pf sporozoites delivered by a total of 1806 irradiated mosquitoes, which protected him against a Pf but not a Pv challenge. Subsequent immunization by exposure to a total of 739 Pv-infected, irradiated mosquitoes conferred protection against Pv challenges for up to six months (34). Another volunteer immunized by the bites of 728 irradiated Pv-infected mosquitoes was reported to be unprotected against a Pv challenge one week after the last immunization, but was protected one week after the last inoculation of an additional series of 1251 bites (35). Finally, three volunteers immunized by the bites of 440-987 irradiated, Pf-infected mosquitoes were protected for 8 weeks against an infectious Pf challenge, but no protection was observed in volunteers exposed to 200 or fewer irradiated mosquitoes (36). Overall, of 11 volunteers who were immunized in the 1970s by the bites of irradiated, Pf-infected mosquitoes, five displayed species-specific (37) protection against a subsequent exposure to infective sporozoites of different Pf strains.
It would be more than a decade until the next clinical studies of WSp vaccines took place. In 1991, two groups of volunteers were vaccinated by repeated exposure to the bites of Pf-infected, X-irradiated mosquitoes. While two volunteers in group 1, exposed to 625 and 715 irradiated mosquitoes, were unprotected against an infectious Pf challenge delivered by mosquito bite, all three volunteers in group 2, who were exposed to a total of 1563-1681 immunizing bites, were fully protected against a Pf challenge three weeks after the last immunization (38). One of these subjects received a series of booster immunization bites approximately three months after that first challenge and was re-challenged nine months after that, remaining immune to virulent sporozoites (39). Between 1989 and 1999, another eleven volunteers were immunized at the Naval Medical Research Center and the Walter Reed Army Institute for Research. The results of these trials are summarized in a publication by Hoffman et al. in 2002, and show that ten of eleven volunteers immunized by the bites of 1001-2927 irradiated mosquitoes infected with Pf strain NF54 were protected against a homologous challenge two to nine weeks after the last immunization (11). Furthermore, four out of five protected subjects were also protected against a Pf re-challenge 23-42 weeks after a secondary immunization, and two volunteers were protected when re-challenged with the heterologous 7G8 strain of Pf (11). This report constitutes a landmark in WSp malaria vaccination, demonstrating not only that protective immunity elicited by Pf RAS is strain-transcendent, but also that it may persist for at least 10 months. These findings created a renewed interest in WSp vaccination against human malaria and paved the way for an entirely new era of research in this field.
WSp vaccination by injection: purification and cryopreservation of Pf sporozoites
The enthusiasm generated by the observations outlined above was curbed by the generally accepted conviction that a vaccine whose administration required the bites of more than 1000 mosquitoes was clinically impractical [reviewed in (40, 41)]. However, and contrary to what had successfully been done in rodent models, the injection of infected mosquito salivary gland material into humans posed unacceptable medical risks (41). This realization entailed several immediate concerns, arising from the (i) practical limitations in infecting mosquitoes with Pf, which depended on feeding on volunteers with circulating Pf gametocytes; (ii) relatively small numbers of sporozoites in the salivary glands of Pf-infected mosquitoes; and (iii) absolute necessity for adequate purification and preservation of Pf sporozoites intended for immunization. The first of these concerns had been overcome by the development of methods for in vitro culturing of Pf parasites in 1976 (42), and for gametocyte production from these cultures in 1982 (43). The challenges imposed by the other two concerns meant that, for the best part of the first decade of the 21st century, clinical trials employing RAS remained scarce (44). This situation changed dramatically thanks to the persistence of Stephen L. Hoffman and his team at Sanaria, Inc., who set out to develop methods to increase sporozoite yields in infected mosquitoes, as well as to purify and preserve these parasites (40). Their efforts culminated in the successful manufacture of the PfSPZ Vaccine, consisting of aseptically purified, metabolically active, non-replicating (irradiated), cryopreserved Pf sporozoites of the NF54 strain, suitable for clinical use (45) and GMP-compliant (31). This remarkable achievement completely changed the prospects for WSp vaccination, and prompted a surge of clinical trials to assess and optimize the immunogenicity and efficacy of RAS-based immunization.
Establishing the proof-of-principle of PfSPZ vaccination
In the first attempt at human vaccination with PfSPZ Vaccine, the vaccine was administered either by intradermal (id) or subcutaneous (sc) injection to a total of 80 volunteers, 44 of whom subsequently underwent homologous CHMI by mosquito bite, alongside 18 non-immunized controls. The results were nothing less than disappointing, with only two of the challenged vaccinees protected against infection, and none of the others displaying even a delay in time to detectable parasitemia (46). Unfazed by these results, the authors employed several animal models to dissect the immune responses elicited by injection of the vaccine through different routes. Their results provided unequivocal evidence that intravenous (iv) injection of PfSPZ Vaccine elicited significantly more potent immune responses than id and sc administration of the vaccine (46). These observations paved the way for the clinical evaluation of PfSPZ Vaccine’s protective efficacy when administered by iv injection and, in 2013, the Sanaria team reported for the first time that five doses of 1.35 x 105 iv-injected PfSPZ Vaccine (strain NF54) conferred 100% protection against an infectious challenge with PfNF54 parasites delivered by mosquito bite 3 weeks after the last immunization (47). This landmark study constituted the first demonstration that a WSp vaccine delivered by needle and syringe could confer high levels of protection against human malaria. Aided by the subsequent demonstration that PfSPZ vaccination could confer long-term protection against malaria (48), these findings laid the foundations for an ambitious plan to further the clinical development of PfSPZ Vaccine and other related products (49).
Protection against heterologous challenge
The demonstration that five doses of the PfSPZ Vaccine could induce high levels of protection against homologous challenge in trials conducted in the USA raised several questions, including whether it would be possible to reduce the number of vaccine doses employed, and if such protection would hold upon a heterologous challenge and/or in malaria-endemic regions. The issues of dose reduction and heterologous protection were addressed in several clinical trials reported from 2017 onwards. Heterologous protection studies commonly employ the South American Pf isolate 7G8 (50, 51), which is genetically diverse from the PfSPZ Vaccine’s PfNF54 strain (52). In fact, a recent analysis of the genome, proteome and CD8+ T cell epitopes of various Pf strains has shown that Pf7G8 is more distant from PfNF54 than any one of more than 700 African isolates investigated, suggesting that Pf7G8 constitutes a stringent surrogate for the vaccine’s field efficacy in Africa (53). In a report from 2017, 5 doses of 2.7 × 105 PfSPZ were shown to confer 92.3% and 80.0% protection against homologous (Pf3D7, a clone of PfNF54) and heterologous (Pf7G8) CHMI delivered by mosquito bite three weeks after the last immunization, respectively, but efficacy against the latter dropped dramatically to 10% twenty-four weeks after the final immunization (52). The same study also showed that a 3-dose regimen of 4.5 × 105 PfSPZ conferred 86.7% and 57.1% protection against homologous CHMI by mosquito bite three and twenty-four weeks after the last immunization, respectively (52). These results indicate that heterologous protection may be less pronounced and less durable than homologous protection, raising concerns about the vaccine’s efficacy in the field. Nevertheless, another study revealed 64% protection against homologous challenge 19 weeks after the last of three immunizations with 9.0 × 105 PfSPZ at 8-week intervals, and 83% of the protected subjects who underwent a repeat heterologous challenge with Pf7G8 parasites 33 weeks after the final immunization remained protected (54). Very recently, vaccination with 9 × 105 PfSPZ on days 1, 8, and 29 was found to be similarly protective against homologous (PfNF54, 77% overall efficacy) and heterologous (Pf7G8, 79% overall efficacy) CHMI delivered iv at 3 or 9-10 weeks after immunization (55).
Protective efficacy in malaria-endemic regions
The first clinical evaluation of the PfSPZ vaccine in a malaria-endemic region was conducted in healthy Malian adults, naturally exposed to malaria. Trial participants were exposed to five doses of iv-delivered 2.7 × 105 PfSPZ at days 0, 28, 56, 84, and 140 during the dry season, and were actively followed up for 24 weeks during the transmission season. The results of this trial were reported in 2017 and indicated a vaccine efficacy of 51.7% (56), which is markedly lower than observed in a previous CHMI trial in the USA with a similar vaccine dose and administration schedule (52). Shortly afterwards, an identical vaccination regimen was employed to administer PfSPZ to Tanzanian adults. Challenge by homologous iv CHMI three weeks after the last immunization revealed only 20% protection, and all protected individuals remained uninfected after a re-challenge at 24 weeks (57). Interestingly, antibody responses to PfCSP in these studies, as in a PfSPZ Vaccine immunogenicity study carried out in Equatorial Guinea (58), were lower than in the volunteers in the USA (57). These observations indicate that malaria-naïve individuals in the USA respond better to the vaccine than malaria-exposed individuals in Africa. This may result from the immune modulation caused by repeated exposure to malaria, and suggests that enhancing the vaccine’s immunogenicity and achieving sterile protection in endemic regions might require increasing the dose of PfSPZ and changing the interval between immunizations (49). In an attempt to increase vaccine efficacy in Tanzania, another trial was conducted where the PfSPZ dose was increased to 9 × 105 or 1.8 × 106, and the number of doses was reduced to 3, at 8-week intervals. Interestingly, and perhaps somewhat surprisingly, this study revealed an association between an increase in the dose and a decrease in vaccine efficacy. In fact, while 100% of the participants who received the 9 × 105 dose were protected against homologous (PfNF54) iv CHMI at 3 or 11 weeks, only 33% of those who received the 1.8 × 106 dose were protected against homologous (PfNF54) iv CHMI at 7.4 weeks (59). More recently, three doses of 1.8 × 106 PfSPZ at 1-, 13- and 19-week intervals afforded 51% efficacy against natural Pf transmission in Mali (60).
Multi-dose priming
The ability to elicit effective heterologous protection is an absolute requirement for a malaria vaccine to be deployed in the field, where multiple Pf strains likely coexist. Sanaria has therefore concentrated a large part of their recent efforts on improving PfSPZ’s heterologous protection. Hypothesizing that induction of liver-resident CD8+ T cells, which are pivotal for vaccine efficacy (61), could be enhanced by repeated priming with low PfSPZ vaccine doses, two multi-dose priming studies followed by CHMI were recently undertaken. In a clinical trial in the USA, 5 doses of 4.5 x 105 PfSPZ vaccine administered iv on days 1, 3, 5 and 7, and week 16 (referred to as multi-dose priming and delayed boosting) protected 40% of the subjects against heterologous challenge with Pf7G8 delivered by mosquito bite 12 weeks after the last immunization (62). Relevantly, in the same study, three immunizations with 9.0 × 105 PfSPZ at 8-week intervals (standard dose) afforded only 20% protection against heterologous Pf7G8 challenge by mosquito bite at 12 weeks, and three 8-weekly administration of 1.8 x 106 PfSPZ (escalated dose) afforded only 23% protection against heterologous Pf7G8 CHMI by mosquito bite at 24 weeks (62). More recently, the efficacy of multi-dose priming regimens of PfSPZ Vaccine against homologous (PfNF54) CHMI administered iv 6-7 weeks after the final immunization was evaluated in a clinical trial in Equatorial Guinea. In this study, four multi-dose priming regimens, with or without delayed boosting, were evaluated, all of which using doses of 9 x 105 PfSPZ delivered iv: days 1, 3, 5, 7 and 113; days 1, 3, 5 and 7; days 1, 3, 5, 7 and 29; and days 1, 8, and 29. A significant 51.3% protection was only observed for the regimen in which the vaccine was administered on a 4-week schedule, on days 1, 8, and 29 (63). The delayed boosting immunization schedule yielded a protective efficacy of ~40%, which is similar to that observed in the USA trial (62), but was not statistically significant (63). Perhaps surprisingly, protection afforded by the 2-dose multi-prime regimen (days 1, 8, and 29; 51.3%) was higher than that afforded by 4-dose multi-prime (days 1, 3, 5, 7 and 29; 10.7%), clearly a matter that demands additional investigation.
Vaccination of children and infants
Malaria exerts its heavier mortality burden on children and infants, with 77% of total malaria deaths in 2020 occurring under the age of 5 years-old (64). With this in mind, Sanaria, Inc. initiated an assessment of the safety and feasibility of iv administration of the PfSPZ Vaccine, aiming to conduct an efficacy trial on this age group. These assessments took place in Tanzania (65) and Kenya (66, 67), and were accompanied by a careful analysis of caregiver and community perceptions and experiences regarding participation in these studies (68). These efforts culminated in a recently reported phase 2 trial conducted in western Kenya on a population of 336 infants aged 5-12 months, naturally exposed to malaria. The vaccine was administered in three iv doses of 4.5 × 105, 9.0 × 105 or 1.8 × 106 PfSPZ spaced by 8 weeks, with a 12-month follow-up period. Although vaccine efficacy against clinical malaria was estimated at 45.8% in the highest-dose group at the study’s 3-months exploratory endpoint, significant protection against Pf infection was not observed in any dose group at the 6 months primary endpoint (69). These disappointing findings indicate that immune responses to the PfSPZ Vaccine are age-dependent, and may be explained by major differences between infants and older children and adults in the priming of PfSPZ-specific T cell responses (65, 69), and/or by the presence of low-level Pf parasitemia at the time of administration of the first vaccine dose (69, 70). In any case, these results clearly do not support the use of the PfSPZ Vaccine in infant populations, whose immune systems are immature, particularly for T-cell responses (71).
Genetically-attenuated parasites
Plasmodium parasites express several genes encoding pre-erythrocytic stage-specific proteins, some of which may be essential for the parasite’s intra-hepatic development (72). Genetically-attenuated parasites (GAP) have been engineered to abrogate the expression of one or more genes essential for completion of their developmental process in the liver. Targeted deletion of these genes results in parasites that are able to infect hepatocytes but arrest their liver-stage development at defined points, remaining unable to establish a symptomatic blood-stage infection in vivo (73). A potential advantage of GAP- over RAS-based immunization is that the former constitute a homogeneous population of parasites with defined genetic identity and attenuation phenotype, which may be designed to induce optimal protective immunity (74). It is usually accepted that the immunity induced by parasites whose liver development arrests later is superior to that induced by early-arresting parasites (75, 76). Therefore, the development of a late-arresting PfGAP that can elicit effective protective immunity against malaria remains an attractive objective to which much attention has been devoted.
GAPs: From mice to humans
Effective vaccination employing genetically attenuated Plasmodium parasites was first demonstrated in rodent models of malaria in the mid-2000’s. In 2005, Mueller at al. and van Dijk et al. showed that immunization of mice with Pb sporozoites deficient in the upregulated in infective sporozoites gene 3 (uis3) or in the p36p gene, respectively conferred complete protection against a challenge with infectious Pb sporozoites (77, 78). Over the next few years following these landmark studies, several reports emerged showing that highly effective protective immunity could be elicited by immunization by iv injection of other rodent parasite mutants, including p52-/p36-deficient P. yoelii (Py) (79), uis3-/uis4-deficient Pb (80) and Py (81), and purine nucleoside phosphorylase (pnp)-deficient Py (82), multidrug resistance-associated protein 2 (mrp2)-deficient Pb (83), and b9-/slarp-deficient Pb, followed by an iv challenge employing fully infective sporozoites of the same species (84).
Naturally, the success for GAP-based vaccination in rodents sparked an interest in the use of this approach to create vaccine candidates against human malaria. The genetic design of replication-competent vaccine strains holds the promise for a potent, broadly protective malaria vaccine (85). The development of appropriate genetic manipulation methods enabled the targeted deletion of genes in order to create Pf GAPs that arrest during hepatic development and that lack drug-resistance markers (86–88). Subsequent technical advances in genetic manipulation enhanced the efficiency and pace for generation of transgenic Plasmodium parasites (85). The first Pf GAP was reported in 2009 and consisted of a Pf parasite lacking the p52 and p36 genes, whose liver arrest was confirmed in vitro and in a liver-humanized mouse model (87). Since then, several other Pf GAPs have been reported in the literature, including Pfb9−/slarp− (84), Pfp52−/p36−/sap1− (89) and Pfmei2− (90). Several of these candidates have been, are currently, or will likely undergo evaluation in a clinical setting.
Clinical evaluation of Pf GAP vaccines
The number of Pf GAP candidates tested in humans is currently limited. The first report of such a clinical study dates from 2013, when Pfp52−/p36− sporozoites (87) were administered to six malaria-naïve volunteers by the bites of infected female Anopheles stephensi mosquitoes. Subjects were initially exposed to 5 bites/volunteer, which was followed by exposure to ~200 bites/volunteer one month later. Although all volunteers remained blood stage-negative after the low dose exposure, one volunteer developed parasitemia after exposure to 263 bites, activating a Stopping Rule in the study (91). Genotyping analysis confirmed that the parasite in the peripheral circulation of this volunteer was Pfp52−/p36−, showing that a breakthrough infection, rather than a reversion to wild-type Pf, had occurred (91). This observation highlights the need to identify gene deletions, or a combination thereof, that ensure the parasite’s complete arrest in the liver of the immunized subjects. In an attempt to achieve this, an additional deletion was included to generate the Pfp52−/p36−/sap1− parasite, termed PfGAP3KO (89). To confirm immunization safety, PfGAP3KO was administered to 10 subjects by a single exposure to the bites of 150 to 200 bites per volunteer. All participants in this study remained blood stage-negative, indicating complete attenuation of PfGAP3KO in humans, and paving the way for the evaluation of its protective efficacy in the clinic (89).
The first Pf GAP to have undergone an evaluation of its protective efficacy in humans is Pfb9−/slarp− (84). Aseptic, purified, and cryopreserved Pfb9−/slarp− sporozoites were manufactured by Sanaria, Inc., creating the PfSPZ-GA1 Vaccine. No breakthrough infections were observed following the iv administration of three doses of 4.5 × 105 or 9.0 × 105 PfSPZ-GA1 Vaccine at 8-week intervals (92). Subjects were then challenged by mosquito bite CHMI with PfNF54 parasites 3 weeks after the last immunization. Although all vaccine groups showed a significant increase in pre-patency time, only 1 of 12 volunteers in the 4.5 × 105 PfSPZ-GA1 group and 2 of 13 volunteers in the 9.0 × 105 PfSPZ-GA1 group were sterily protected (92). Even though these results may appear somewhat disappointing, this is a landmark trial in that it constitutes the first clinical assessment of the protective efficacy of a Pf GAP vaccine. Furthermore, it should be noted that all volunteers from a Pf RAS control group, immunized with three doses of 4.5 × 105 PfSPZ Vaccine, developed parasitemia (92), which may reflect a particularly high stringency of the PfNF54 mosquito bite challenge employed in this study.
The clinical evaluation of PfGAP3KO’s immunogenicity and protective efficacy was reported very recently. In this trial, the vaccine was delivered by three (with 4 weeks between the first and second vaccinations and the 8 weeks between the second and third vaccinations) or five (with 4 weeks between the first four vaccinations and 8 weeks between the fourth and fifth vaccinations) immunizations, with ~200 PfGAP3KO-infected mosquito bites per immunization. CHMI was carried out by the bites of PfNF54-infected mosquitoes either 4 weeks after the last immunization of the 6 volunteers in each of study arms 1 and 2, or 26 weeks after the first CHMI for study participants in both study arms who did not have any detectable Pf infection after the first CHMI. The vaccine protected 50% of the volunteers in either study arm after the first CHMI, and protected 1 of the 6 volunteers who undertook the second CHMI (93).
The road ahead for Pf GAP vaccination remains wide open, with efforts ongoing towards the identification of late-arresting replication-competent Pf parasites that are completely attenuated and highly immunogenic. Moreover, existing mutants, such as Pfmei2−, are already undergoing clinical evaluation, and several others are likely to follow. Finally, the possibility of iteratively improving these parasites through the expression of additional antigens or immunomodulatory elements offers the prospect of a rationale for the creation of increasingly efficacious and versatile Pf GAP candidates (85).
Chemoprophylaxis and sporozoites
Depending on their molecular target and mode of action, antiplasmodial drugs may act either on multiple or only on specific stages of the parasite’s life cycle. Immunization by ChemoProphylaxis and Sporozoites (CPS) relies on the ability of an antiplasmodial compound to provide a prophylactic cover against the symptomatic stage of Plasmodium infection following the administration of non-attenuated sporozoites. Thus, the inoculated, replication-competent, parasites are able to infect, develop and egress from hepatic cells unencumbered, but are eliminated prior to egress or following merozoite release into the blood stream, during the first wave of invasion of red blood cells (94). Liver infection elicits potent pre-erythrocytic immune responses, while the appearance of disease symptoms is prevented by the presence of the circulating drug. Unrestricted liver stage growth expands parasite biomass and antigenic repertoire to a greater extent than what occurs with RAS and GAP, potentially enhancing immunogenicity and decreasing the dose of immunizing parasites required for protection. In addition, the presence of an abortive blood-stage infection may elicit humoral immune responses against blood-stage Plasmodium antigens (95). Early pre-clinical studies showed that immunization of mice with fully infectious Pb (96, 97) or Py (98) sporozoites under chloroquine treatment, a drug that specifically targets blood stage parasites (99), conferred significant protection against a sporozoite challenge with the same parasite species. Since then, similar results have been obtained employing other antiplasmodial drugs, such as primaquine (100), mefloquine (101), pyrimethamine (102), piperaquine (103), artesunate (104), clindamycin (105), azithromycin (105) and arteether (106). More recently, CPS employing P. knowlesi (Pk) sporozoites and chloroquine was also shown to confer significant protection against Pk infection in a non-human primate model (107). Collectively, these pre-clinical observations paved the way to a wide array of studies aimed at assessing the potential of CPS immunization for vaccination against human malaria.
CPS immunization by mosquito bite
The first-in-humans demonstration that CPS immunization could afford high levels of sterile protection against Pf infection was provided by a landmark study in 2009, carried out at Nijmegen’s Radboud University Medical Centre. In this seminal study, ten volunteers were exposed to the bites of 12 to 15 PfNF54-infected mosquitoes in three immunization sessions at 1-month intervals, whilst under the cover of a prophylactic chloroquine regimen. Five control subjects received an equivalent number of non-infected mosquito bites and were subjected to a similar chloroquine regimen. Both groups of volunteers were challenged by homologous CHMI delivered by mosquito bite 8 weeks after the last immunization dose (4 weeks after the discontinuation of chloroquine prophylaxis). Whereas all control subjects developed PfNF54 parasitemia, all immunized volunteers were protected against infection, indicating a striking 100% homologous protective efficacy of this immunization method (108). Importantly, a homologous re-challenge of six protected volunteers 2.5 years after the original study revealed that four of them remained sterilely protected, while the remaining two showed prolonged prepatent periods (109).
In a trial aimed at discerning the contributions of pre-erythrocytic and erythrocytic immunity for the protection afforded by Pf CPS vaccination, 4 out of 5 subjects (80%) taking chloroquine prophylaxis and immunized by 3 exposures to the bites of 15 PfNF54-infected mosquitoes at 1-month intervals were fully protected against a homologous CHMI by mosquito bite (110). In another group of 9 similarly immunized volunteers, none was protected against a blood-stage challenge by iv administration of asexual PfNF54 parasites, showing that protection against malaria CPS immunization is entirely mediated by pre-erythrocytic immunity (110). In a subsequent trial, 60 and 70% homologous protection was observed for volunteers under either chloroquine or mefloquine prophylaxis, respectively, who were exposed 3 times to 8 PfNF54-infected mosquitoes at monthly intervals (111).
The enthusiasm generated by the high protective efficacy observed in these homologous CHMI trials led to the assessment of the protection conferred by CPS immunization against heterologous parasite strains. Thus, in a follow-up study, 16 volunteers previously immunized by CPS employing PfNF54 parasites delivered by mosquito bite and homologously challenged with the same parasite strain were re-challenged 14 months after the last immunization with Pf strain NF135.C10. Only 2 out of 13 volunteers that were previously fully protected against PfNF54 were also fully protected against Pf NF135.C10, while the remaining 11 displayed an increased pre-patent period (112). These somewhat disappointing results were made even more so by the results of a subsequent clinical trial. There, CPS immunization with PfNF54 protected 5 out of 5 volunteers against a PfNF54 challenge 14 weeks after the last immunization, but sterilely protected only 2 out of 10 and 1 out of 9 volunteers against CHMI with Pf strains NF135.C10 and NF166.C8, respectively, all delivered by mosquito bite (113). These findings raise important questions regarding the potency of the immune responses required for effective heterologous protection following CPS immunization and the optimization thereof. This may involve the use of an immunizing Pf strain with intrinsically higher liver stage infectivity, an increase in the immunization dose or an alteration of the immunization regimen (113). Some of these challenges can at least be partially addressed by resorting to iv administration of the immunizing parasites, as discussed below.
Enter Sanaria’s PfSPZ-CVac
In view of the promising results of early CPS vaccine trials in the clinic, the team at Sanaria, Inc. and its collaborators posited that PfSPZ Challenge could serve as a replacement for mosquito bite delivery of immunizing Pf parasites, hence giving rise to a CPS vaccine approach termed PfSPZ- Chemoprophylaxis Vaccine (PfSPZ-CVac) (49). In the first clinical trial with PfSPZ-CVac, 3-4 id administrations of 7.5 x 104 PfSPZ employing chloroquine as the drug partner induced no sterile protection against homologous CHMI with PfSPZ Challenge (114). With the benefit of hindsight, it is now clear that this is not a surprising result, given the poor immunogenicity of vaccine administration by the id route, as observed in PfSPZ vaccine studies ongoing at the time (46, 47). Thus, in a subsequent landmark trial carried out at the University of Tübingen, PfSPZ-CVac was administered iv, with chloroquine as the partner drug. A dose-dependent protective effect of the vaccine was observed, with 100% of the volunteers immunized by three doses of 5.12 × 104 sporozoites at 28-day intervals being protected against homologous iv CHMI with PfSPZ Challenge (PfNF54) 10 weeks after the last immunization (115). Remarkably, not only was this the first time that complete sterile immunity by PfSPZ-CVac was observed in the clinic, but also this was achieved with sporozoite doses 1-2 orders of magnitude lower than those required by RAS immunization with PfSPZ Vaccine, as outlined above. These results confirmed the high immunogenicity of the PfSPZ-CVac immunization approach, opening the door for further optimization of the immunization regimen and its assessment against heterologous CHMI or in the field (116).
The first assessment of PfSPZ-CVac in a malaria-endemic region took place in Equatorial Guinea and was reported in 2021. In this clinical trial, 3 doses of 2.7 × 106 PfSPZ Vaccine or 1.0 × 105 PfSPZ-CVac were administered at 8- or 4-week intervals, respectively, to different groups of volunteers. Immunized subjects underwent homologous CHMI by iv administration of PfSPZ Challenge (PfNF54) at a median of 14 weeks after the last immunization. Vaccine efficacies were 27 and 55% for PfSPZ Vaccine and PfSPZ-CVac, respectively, and were not statistically different from each other (117). Pre-patency as assessed by thick blood smear was significantly longer for PfSPZ Vaccine, but not for PfSPZ-CVac recipients, than controls (117). This trial constitutes the first head-to-head comparison of PfSPZ Vaccine and PfSPZ-CVac efficacies. It should be noted that the efficacy of both immunizations was lower than that observed in homologous CHMI studies in malaria-naïve volunteers employing lower vaccination doses (54, 115), once again indicating that immunization regimens in the field require further optimization.
Heterologous protection by PfSPZ-CVac vaccination
The issue of vaccination dose began to be assessed in a trial reported in 2021, where PfSPZ-CVac was used in combination with either chloroquine or pyrimethamine at a dose of 2 × 105 sporozoites, a 4-fold increase relative to that employed in the Mordmuller et al. study (115). In this study, subjects received 3 monthly immunizations with either partner drug, and underwent CHMI by iv administration of PfSPZ Challenge 3 months after the last immunization. The data revealed 100% heterologous (Pf7G8) protection in the chloroquine group, whereas 87.5 and ~78% protective efficacy was observed against homologous (PfNF54) and heterologous (Pf7G8) challenge, respectively, in the pyrimethamine group (118). These remarkable results constitute the first demonstration that high levels of heterologous protection can be achieved for at least 3 months through PfSPZ-CVac vaccination, which is significantly higher than what had been observed for RAS immunization with 9 × 105 PfSPZ Vaccine (62). However, in a very recent study in Mali, 3 doses of 2 × 105 PfSPZ-CVac (chloroquine) administered at 0, 4 and 8 weeks afforded only an estimated, non-statistically significant, protective efficacy of ~33% against naturally transmitted Pf infection over a 48-week surveillance period spanning wet and dry seasons (119).
Condensed PfSPZ-CVac immunization regimens
Also in 2021, a condensed immunization regimen employing PfSPZ-CVac and chloroquine was attempted for the first time. Inoculation of 1.1 × 105 sporozoites, twice the dose employed in the Mordmuller et al. study (115), on days 1, 6 and 29, yielded 77% protection against heterologous (Pf7G8) iv CHMI with PfSPZ Challenge 12 weeks after the last immunization (120). The importance of this study lies not only on the high protective efficacy observed, but also on the fact that in the immunization regimen employed chloroquine was administered only on the days of vaccine inoculation, limiting to three the number of visits to complete vaccination (120). In yet another study from 2021, two condensed regimens of three administrations of 5.12x104 PfSPZ-CVac seven days apart and of 1.024x105 PfSPZ-CVac five days apart, using chloroquine as the partner drug, were assessed in the clinic. The two regimens gave very different protections against homologous CHMI with PfSPZ Challenge (PfNF54), with the 7-day group showing 0% protective efficacy, and the higher-dose, 5-day group displaying 75% protective efficacy (121). It should be noted that vaccine administration to the former group coincided with patent parasitemia, suggesting that this may be associated with the observed lack of sterile immunity (121). Finally, in a very recent assessment of accelerated PfSPZ-CVac vaccination regimens, volunteers underwent three-dose immunization regimens at days 0/14/28 or at days 0/5/10, employing 5.12 × 104 sporozoites/dose and chloroquine as the partner drug. Homologous CHMI was performed by iv administration of PfSPZ Challenge (PfNF54) 10 weeks after the last immunization. The two immunization regimens yielded similar protective efficacies of 67 and 63% for 28- and 10-day vaccination schedules, respectively, but the latter resulted in more pronounced cellular and humoral immune responses than the former (122). Collectively, these results pave the way for further development of an effective condensed regimen of PfSPZ-CVac immunization, capable of eliciting protective immunity in the field.
P. berghei-based vaccination against human malaria
Rodent Plasmodium parasites are the most widely employed models of malaria research, particularly in what concerns the investigation of the pre-erythrocytic stages of infection (123). In recent years, rodent malaria parasites have also emerged as potential candidates for WSp immunization against human malaria. The idea draws from the origins of vaccination, when Edward Jenner unknowingly established the notion of cross-species protective immunity, by successfully using cowpox to vaccinate humans against smallpox (124). The notion that a similar principle may apply to Pb and human malaria parasites is supported by the presence of cross-species epitopes in different malaria parasites (125), and is strengthened by the high percentage of predicted T cell epitopes shared between the former and the latter (126). Besides, Pb’s high amenability to genetic modification, solidified by years of experience in this area, enables the insertion of selected human Plasmodium antigens into neutral loci of its genome, effectively turning the rodent parasite into a unique platform for expression of heterologous Plasmodium antigens (127). Immunization with such chimeric Pb sporozoites is therefore expected to elicit not only cross-species immune responses, but also targeted immunity against human malaria parasites arising from those heterologous immunogens (128).
Pre-clinical validation of Pb-based WSp vaccination
The concept of Pb-based WSp vaccination was validated in 2018 through the generation of PbVac, a Pb parasite that expresses PfCSP under the control of the strictly pre-erythrocytic Pbuis4 promoter (126). Pre-clinical characterization of PbVac showed that it expresses both the endogenous PbCSP and the heterologous PfCSP at the surface of sporozoites and liver stages, and that it displays wild-type Pb-like mosquito and hepatic infectivity levels (126). Employing liver- and blood-humanized mouse models, PbVac was also shown to invade and develop inside human hepatocytes and to be unable to replicate inside human erythrocytes. Moreover, and crucially, PbVac was found to infect human primary hepatocytes with significantly higher efficacy than Pf, which may potentially entail high levels of human liver infectivity (126). Immunization of rabbits by the bites of PbVac-infected mosquitoes elicited cross-species cellular immune responses, as well as PfCSP-specific antibody responses that functionally inhibit infection of human hepatocytes by Pf, both in vitro and in liver-humanized mice (126). Collectively, these data unequivocally demonstrated PbVac’s potential for immunization against Pf malaria, warranting its evaluation in the clinic. However, this posed a significant challenge, not only because there was no previous history of experimental administration of rodent malaria parasites to humans, but also due to the fact that PbVac is a genetically modified organism, and that sporozoites of this parasite can only be generated in mosquitoes infected by feeding on the blood of infected mice. Thus, several additional studies were performed to ensure the safety of PbVac for human use, including the creation of a Master Cell Bank, whole-genome sequencing of the transgenic parasite, a complete set of microbiological analyses, and tissue distribution and drug-sensitivity studies (129). The complete set of pre-clinical data gathered in these studies (126, 129) paved the way for its assessment in humans.
Clinical assessment of PbVac
The first-in-humans assessment of PbVac was reported in 2020 and consisted of a phase 1/2a clinical trial, in which PbVac sporozoites were administered to volunteers by the bites of infected female A. stephensi mosquitoes. Safety was assessed in a phase 1 dose-escalation study, in which groups of volunteers were exposed to the bites of 5, 25 and 75 PbVac-infected mosquitoes, with no breakthrough infections or serious adverse events recorded (130). In phase 2a of the study, 12 volunteers were immunized by four exposures to the bites of 75 PbVac-infected mosquitoes, spaced by 4 (between the first and second and between the second and third immunizations) or 8 (between the third and fourth immunizations) weeks, and were challenged 3 weeks after the last immunization by PfNF54-infected mosquito bites. A significant delay in blood stage patency and a significantly lower parasite density at first detection in the blood was observed in immunized volunteers, corresponding to an estimated 95% decrease in PfNF54 liver load for vaccinated subjects relative to non-immunized controls (130). It should be noted that the 4 x 75 PbVac-infected mosquito bites employed in this study corresponds to a much lower vaccine dose than that delivered by the more than 1000 mosquito bites previously used for immunization with Pf RAS (11). Thus, although no sterile protection was observed in the PbVac study, the marked reduction in liver parasite load triggered by immunization with the clearly sub-optimal dose employed, alongside the dose-dependent humoral and cellular immune responses observed (130), support further exploration of Pb-based vaccination against malaria. To this end, the production of aseptically purified, vialed, cryopreserved PbVac sporozoites that can be administered by parenteral injection at defined doses is currently ongoing in collaboration with Sanaria, Inc. Furthermore, the possibility of inserting multiple heterologous genes in the Pb genome (131) under the control of suitable pre-erythrocytic promoters (132) enables the generation of transgenic Pb parasites that express genes from different human Plasmodium parasites and from different stages of their life cycle. This possibility is particularly appealing in the case of Pv, for which an in vitro culture system is yet to be achieved (133), which severely limits the development of a WSp vaccine. Thus, transgenic Pb parasites expressing suitable Pv antigens may serve as unique surrogates for WSp vaccination against this human malaria parasite.
Immune responses elicited by whole-sporozoite vaccination
WSp vaccines primarily aim at boosting the host’s immunity through the generation of effective and long-lasting immune responses that control and/or eliminate the parasite during the pre-erythrocytic stage of its life cycle. The investigation of these immunological mechanisms and their correlation with protection have been the focus of multiple studies that led to a thorough, yet still incomplete, picture of the immunity that ensues following vaccination, as recently reviewed (134–136). Although several studies have suggested a relation between some immune parameters and protection, a definitive immune correlate of protective efficacy of WSp vaccination remains to be clearly identified. Studies in mice and non-human primates have provided robust evidence that a large part of the pre-erythrocytic immune response that leads to protection is cell-mediated in the liver [reviewed in (61, 137)]. However, the fact that, in humans, immune parameters can only be analyzed in the peripheral circulation constitutes a limitation to the assessment of the global WSp-associated immunological landscape in the clinic. Moreover, it is likely that different WSp vaccines may produce distinct humoral and cellular response signatures that define protective immunity. In this chapter we will outline the main humoral and cellular immune responses identified during the clinical assessment of a variety of WSp vaccine candidates.
Antibody-mediated responses
Vaccines commonly act by inducing an antibody-mediated response against specific microorganisms or their constituents. The humoral responses induced by WSp vaccines are largely directed at pre-erythrocytic antigens, with CSP, the most abundant protein on the surface of sporozoites, representing the hallmark parasite target [reviewed in (134, 136, 138, 139)]. Sporozoite- or CSP-specific antibodies are consistently induced in response to WSp vaccination of malaria-naïve individuals, and some studies have reported a correlation of antibody titers with RAS (47, 69), CPS (115), GAP (92) or PbVac (130) immunization doses, or with PfSPZ-induced protection (48, 92). Importantly, pre-exposure has been identified as a limiting factor for the magnitude of the humoral responses elicited by RAS (57, 58, 140) and PfSPZ-CVac (117) immunizations. Whether a similar effect is observed following immunization with other types of WSp vaccines remains to be addressed.
In addition to the magnitude of the humoral response, it is also important to assess the functionality of the antibodies generated by vaccination. Antibodies against sporozoites or their antigens may limit the infection in several ways, including by decreasing their motility (141), inhibiting hepatocyte invasion and parasite development (142), or mediating their destruction through mechanisms such as antibody-dependent cytotoxicity or phagocytosis upon opsonization (143, 144). The functionality of the circulating antibodies induced by WSp vaccination can be assessed by a variety of in vitro assays or in vivo studies, as recently reviewed (145). An important role for antibodies in pre-erythrocytic immunity was initially established through the observation that patency following administration of Pb sporozoites to naïve mice was delayed by passive transfer of serum from RAS-immunized mice (146). A functional role for antibodies elicited by PfSPZ (48), CPS (147) and Pf GAP3KO (89) immunization has been demonstrated in vivo using liver-humanized mouse models.
Different WSp vaccine approaches lead to distinct extents of parasite development in the liver, hence differing in the breadth of Plasmodium antigens presented to the host. Accordingly, antibodies to asexual and sexual erythrocytic antigens were low to undetectable following PfSPZ Vaccine (47) and early-arresting GAP (91) immunizations, while humoral responses against both pre-erythrocytic and cross-stage Plasmodium antigens are induced by CPS vaccination (148). Functional antibodies against the immunodominant CSP, which is common across WSp vaccine strategies, are prevalent in all WSp immunization approaches [reviewed in (134); see also (92, 130, 149–151)]. Nonetheless, antibodies against non-CSP proteins from CPS-immunized volunteers were shown to block Pf parasite development in hepatocytes in vitro and in vivo (152). In fact, several other antigens besides CSP currently constitute promising vaccine candidates, including thrombospondin-related adhesion protein (TRAP) (153) and cell-traversal protein for Plasmodium ookinetes and sporozoites (CelTOS) (154). Excitingly, Pb-based WSp vaccination (126, 130) offers a platform that may be used as a backbone for insertion of multiple genes, to elicit tailored humoral immune responses that enhance and/or synergize with those induced against CSP. This strategy may trigger humoral immunity against multiple Pf strains, as well as against other Plasmodium species, such as Pv, to overcome current limitations of the existing WSp vaccination approaches.
Cellular immunity
Cellular immunity is critical for the protection elicited by RAS immunization in rodent and non-human primate models, and most pre-clinical data indicate a central role for CD8+ T cells and interferon-γ (IFN-γ) in protection by this vaccination approach (46, 155, 156). In addition, other cell populations, including CD4+ T cells, γδ T cells and natural killer (NK) cells, can also play a role in mediating protection [reviewed in (135, 137)].
CD8+ T cells recognize pathogen-derived peptides bound to MHC class I molecules on the surface of antigen presenting cells or infected cells, and can eliminate liver stage Plasmodium parasites either directly, such as through perforin-mediated lysis (157), or indirectly, through cytokine (e.g. IFN-γ, TNF-α) production [reviewed in (135, 137)]. Sterile immunity induced by RAS vaccination in mice is abolished upon depletion of CD8+ T cells or IFN-γ (155, 158), and IFN-γ directly impairs Plasmodium development in human hepatocytes in culture (159). In general, immunizations of humans by RAS (48, 65, 69) and CPS (92, 115) do not consistently nor robustly induce Pf-specific CD8+ T cells in the blood of vaccinated subjects. Nevertheless, some studies reported the detection and dose-dependent increase in the frequency of those cells after vaccination by RAS (47), GAP (91, 92) or PbVac (130), although this did not correlate with protection or patency. Moreover, increased granzyme B expression by CD8+ T cells was associated with protection following CPS vaccination (111). The overall suboptimal detection of parasite-specific CD8+ T cells in the blood is likely associated with their predominant tissue residency. Indeed, Pf-specific IFN-γ-producing CD8+ T cells produced upon RAS immunization of non-human primates are mainly localized to the liver, where they can be present at up to 100 times higher frequencies than in the blood (46, 48). These studies have highlighted the importance of vaccine administration route (iv>>id or sc), dose and schedule on the formation of tissue-resident CD8+ T cell responses, which likely extends to the other WSp immunization strategies.
CD4+ T cells can have a multiplicity of roles in mediating protective immunity in malaria, including aiding the survival and differentiation of CD8+ T cells (160, 161), the development of efficient B cell responses (162, 163), or by acting directly through pro-inflammatory cytokine (eg. IFN-γ, TNF-α, IL-2) production (reviewed in (135, 137, 164). Many studies have reported the presence of Pf-specific CD4+ T cells, and particularly of polyfunctional memory Th1 cells (producing IFN-γ, TNF-α and/or IL-2), in the blood of volunteers immunized with RAS (47, 48, 54, 57, 65), CPS (92, 115), GAP (91, 92) and PbVac (130), but they were only associated with protective immunity following CPS vaccination (108, 109, 115). In addition, the increased expression of the degranulation marker CD107a on CD4+ T cells has also been associated with protection against homologous (111) but not heterologous (113) challenge following CPS immunization. Importantly, Pf-specific polyfunctional memory CD4+ T cell responses were low to undetectable in PfSPZ-vaccinated infants in Tanzania (65) and Western Kenya (69), raising concerns regarding the implementation of the PfSPZ vaccination strategy in this immunologically immature population.
γδ T cells, which represent 2-5% of total T cells in humans, are unconventional T cells that are not restricted by classical MHC-mediated antigen presentation. The major subset of γδ T cells in the blood, Vγ9+Vδ2+, recognizes stress or pathogen-related phosphoantigens that specifically and robustly activate them to proliferate, secrete cytokines (such as IFN-γ and TNF-α), and display cytotoxic behavior [reviewed in (165, 166)]. Accordingly, human γδ T cells are innate responders to Plasmodium parasites in vitro (167) and are able to directly kill merozoites (168). Data from animal studies have provided evidence that γδ T cells can inhibit Plasmodium hepatic development (169), and are necessary for the generation of protective CD8+ T cell responses and for sterile protection following RAS vaccination (170), among other functions (reviewed in (135, 137, 171). In RAS vaccine clinical trials, γδ T cells expanded upon immunization of malaria-naïve and pre-exposed volunteers (47, 48, 54, 170), and the frequency of Vδ2+ γδ T cells was found to be predictive of protection, both at baseline and prior to CHMI (48). Vδ2+ γδ T cell expansion was further reported in some studies following CPS (115, 118) and PbVac (130) immunization. Hence, γδ T cells, and specifically the Vδ2+ subset, represent a potential correlate of protection that warrants further exploration (136).
NK and NKT cells are important innate and innate-like effector cells that are abundant in the liver, and have been implicated in cell-mediated immunity to liver stage Plasmodium infection [reviewed in (172, 173)]. Although not extensively analyzed in the context of WSp vaccination, NK and NKT cells were shown to contribute to the increase in IFNγ production by lymphocytes responding to Pf following CHMI (174), and NK cells were found to upregulate activation and proliferation markers during CPS immunization (94). Importantly, an increase in NK and NKT cell frequencies was found following PbVac immunization, which, for the latter population, correlated the prepatent period of vaccinated individuals (130). Future vaccine development studies should further investigate in depth these and other innate and innate-like populations, as well as related pathways, in light of recent data on their involvement in immune signatures that potentially correlate with protection (175).
Final remarks: Lessons from the past, challenges for the future
Looking back to the history of research on WSp vaccines against malaria, it is clear that much has been achieved, particularly during this last decade. While until the early 2010’s progress was relatively slow, and only a handful of clinical trials had been performed, this number has risen dramatically since then. During this period, Sanaria Inc.’s achievements have revolutionized the field, transforming an attractive, yet unpractical, immunization strategy into a family of injectable products suitable for vaccination and CHMI. The PfSPZ Vaccine alone has now been administered to more than 1700 volunteers in over 20 clinical trials, PfSPZ-CVac has been assessed in a large array of clinical studies and immunization regimens, and PfSPZ Challenge has been used for CHMI of several dozen subjects (31). It was also during this period that Pf GAP vaccination was first evaluated in the clinic, as was a novel WSp immunization strategy based on the use of genetically modified Pb parasites. We presently understand the elicitation of immunity by WSp vaccines better than ever before, and major technical hurdles that once seemed unsurmountable have now been overcome. And yet, the road travelled so far was not without pitfalls, and many important challenges still lay on the path ahead. Despite progress in the automation of mosquito dissections (176), an effective system for in vitro production of Pf sporozoites remains unavailable. Nevertheless, Sanaria, Inc. have publicly announced that major achievements have been made in this regard, and it is very likely that these findings will be published in the near future. Although much has been learned from the immunological analyses of clinical samples from participants in multiple trials (145), immune correlates of malaria vaccine efficacy remain largely undefined (136). On the other hand, the disappointing results of the only clinical trial of a WSp vaccine in infants raises justified concerns about the effectiveness of this immunization approach in that age group (69). Moreover, the higher protective efficacy in malaria naïve volunteers when compared to malaria pre-exposed volunteers (47, 52, 56, 59), as well as the variable levels of protection afforded by different regimens of PfSPZ-CVac vaccination (115, 118, 120, 121), suggest that additional optimization of immunization regimens with these vaccines is required. Finally, the relatively low clinical efficacy of the PfSPZ-GA1 and PbVac candidates (92, 130) demands additional development of these promising, yet still suboptimal, vaccination approaches. Several of these issues will more than likely be addressed in future clinical trials, either planned or ongoing. According to clinicaltrials.gov, there are currently several active, recruiting, or not yet recruiting trials of WSp malaria vaccines, including studies aimed at assessing PfSPZ Vaccine efficacy in Malian women of childbearing age (NCT03989102) and in Malian children (NCT04940130), as well as against heterologous CHMI in malaria-naïve USA adults (NCT04966871), and a head-to-head comparison between an early-arresting [GA1: Pfb9−/slarp− (84)] and a late-arresting [GA2: Pfmei2− (90)] GAP is currently ongoing at the Leiden University Medical Center (NCT04577066). Plans are also being made for the clinical evaluation of the safety and protective efficacy of parentally injected PbVac. Moving forward, these and other studies will continue to compound our accumulated knowledge on human immunization with WSp malaria vaccines, bringing their use for preventing disease and, ultimately, contributing to its elimination, ever closer to reality.
Author contributions
All authors listed have made a substantial, direct, and intellectual contribution to the work and approved it for publication.
Funding
MP acknowledges the “la Caixa” Foundation for Grant HR21-848, the GSK OpenLab Foundation for grant TC269, and Fundação para a Ciência e Tecnologia (FCT) for grant PTDC-SAU-INF-29550-2017. HN-C acknowledges funding from FCT (reference DL57/2016/CP1451/CT0011).
Acknowledgments
Helena Pinheiro is gratefully acknowledged for designing Figure 1 of the manuscript.
Conflict of interest
The authors declare that the research was conducted in the absence of any commercial or financial relationships that could be construed as a potential conflict of interest.
Publisher’s note
All claims expressed in this article are solely those of the authors and do not necessarily represent those of their affiliated organizations, or those of the publisher, the editors and the reviewers. Any product that may be evaluated in this article, or claim that may be made by its manufacturer, is not guaranteed or endorsed by the publisher.
References
1. White MT, Verity R, Griffin JT, Asante KP, Owusu-Agyei S, Greenwood B, et al. Immunogenicity of the Rts,S/As01 malaria vaccine and implications for duration of vaccine efficacy: Secondary analysis of data from a phase 3 randomised controlled trial. Lancet Infect Dis (2015) 15(12):1450–8. doi: 10.1016/S1473-3099(15)00239-X
2. Kazmin D, Nakaya HI, Lee EK, Johnson MJ, van der Most R, van den Berg RA, et al. Systems analysis of protective immune responses to Rts,S malaria vaccination in humans. Proc Natl Acad Sci U.S.A. (2017) 114(9):2425–30. doi: 10.1073/pnas.1621489114
3. Rts SCTP. Efficacy and safety of the Rts,S/As01 malaria vaccine during 18 months after vaccination: A phase 3 randomized, controlled trial in children and young infants at 11 African sites. PloS Med (2014) 11(7):e1001685. doi: 10.1371/journal.pmed.1001685
4. Nadeem AY, Shehzad A, Islam SU, Al-Suhaimi EA, Lee YS. Mosquirix rts, S/As01 vaccine development, immunogenicity, and efficacy. Vaccines (Basel) (2022) 10(5):1–15. doi: 10.3390/vaccines10050713
5. Praet N, Asante KP, Bozonnat MC, Akite EJ, Ansah PO, Baril L, et al. Assessing the safety, impact and effectiveness of Rts,S/As01e malaria vaccine following its introduction in three Sub-Saharan African countries: Methodological approaches and study set-up. Malar J (2022) 21(1):132. doi: 10.1186/s12936-022-04144-3
6. Venkatesan P. The future of malaria control in light of Rts,S. Lancet Microbe (2022) 3(4):e251. doi: 10.1016/S2666-5247(22)00070-2
8. Nussenzweig RS, Vanderberg J, Most H, Orton C. Protective immunity produced by the injection of X-irradiated sporozoites of plasmodium berghei. Nature (1967) 216(5111):160–2. doi: 10.1038/216160a0
9. Clyde DF, Most H, McCarthy VC, Vanderberg JP. Immunization of man against sporozite-induced falciparum malaria. Am J Med Sci (1973) 266(3):169–77. doi: 10.1097/00000441-197309000-00002
10. Druilhe P, Barnwell JW. Pre-erythrocytic stage malaria vaccines: Time for a change in path. Curr Opin Microbiol (2007) 10(4):371–8. doi: 10.1016/j.mib.2007.07.009
11. Hoffman SL, Goh LM, Luke TC, Schneider I, Le TP, Doolan DL, et al. Protection of humans against malaria by immunization with radiation-attenuated plasmodium falciparum sporozoites. J Infect Dis (2002) 185(8):1155–64. doi: 10.1086/339409
12. McFadden GI. Plasmodium: More don’ts. Trends Parasitol (2019) 35(1):4–6. doi: 10.1016/j.pt.2018.10.002
13. Sauerwein RW, Roestenberg M, Moorthy VS. Experimental human challenge infections can accelerate clinical malaria vaccine development. Nat Rev Immunol (2011) 11(1):57–64. doi: 10.1038/nri2902
14. Chattopadhyay R, Pratt D. Role of controlled human malaria infection (Chmi) in malaria vaccine development: A U.S. food & drug administration (Fda) perspective. Vaccine (2017) 35(21):2767–9. doi: 10.1016/j.vaccine.2017.03.072
15. Stanisic DI, McCarthy JS, Good MF. Controlled human malaria infection: Applications, advances, and challenges. Infect Immun (2018) 86(1):1–17. doi: 10.1128/IAI.00479-17
16. Roestenberg M, Kamerling IMC, de Visser SJ. Controlled human infections as a tool to reduce uncertainty in clinical vaccine development. Front Med (Lausanne) (2018) 5:297. doi: 10.3389/fmed.2018.00297
17. Friedman-Klabanoff DJ, Laurens MB, Berry AA, Travassos MA, Adams M, Strauss KA, et al. The controlled human malaria infection experience at the university of Maryland. Am J Trop Med Hyg (2019) 100(3):556–65. doi: 10.4269/ajtmh.18-0476
18. Hoffman SL. Experimental challenge of volunteers with malaria. Ann Intern Med (1997) 127(3):233–5. doi: 10.7326/0003-4819-127-3-199708010-00010
19. Epstein JE, Rao S, Williams F, Freilich D, Luke T, Sedegah M, et al. Safety and clinical outcome of experimental challenge of human volunteers with plasmodium falciparum-infected mosquitoes: An update. J Infect Dis (2007) 196(1):145–54. doi: 10.1086/518510
20. Matuschewski K, Borrmann S. Controlled human malaria infection (Chmi) studies: Over 100 years of experience with parasite injections. In: Ariey F, Gay F, Ménard R, editors. Malaria control and elimination. New York, NY: Springer New York (2019). p. 91–101.
21. Chulay JD, Schneider I, Cosgriff TM, Hoffman SL, Ballou WR, Quakyi IA, et al. Malaria transmitted to humans by mosquitoes infected from cultured plasmodium falciparum. Am J Trop Med Hyg (1986) 35(1):66–8. doi: 10.4269/ajtmh.1986.35.66
22. Spring M, Polhemus M, Ockenhouse C. Controlled human malaria infection. J Infect Dis (2014) 209 Suppl 2:S40–5. doi: 10.1093/infdis/jiu063
23. Laurens MB, Billingsley P, Richman A, Eappen AG, Adams M, Li T, et al. Successful human infection with p. falciparum using three aseptic anopheles stephensi mosquitoes: A new model for controlled human malaria infection. PloS One (2013) 8(7):e68969. doi: 10.1371/journal.pone.0068969
24. Langenberg MCC, Wammes LJ, McCall MBB, Bijker EM, van Gemert GJ, Graumans W, et al. Controlled human malaria infection with graded numbers of plasmodium falciparum Nf135.C10- or Nf166.C8-infected mosquitoes. Am J Trop Med Hyg (2018) 99(3):709–12. doi: 10.4269/ajtmh.18-0194
25. Gomez-Perez GP, Legarda A, Munoz J, Sim BK, Ballester MR, Dobano C, et al. Controlled human malaria infection by intramuscular and direct venous inoculation of cryopreserved plasmodium falciparum sporozoites in malaria-naive volunteers: Effect of injection volume and dose on infectivity rates. Malar J (2015) 14:306. doi: 10.1186/s12936-015-0817-x
26. Mordmuller B, Supan C, Sim KL, Gomez-Perez GP, Ospina Salazar CL, Held J, et al. Direct venous inoculation of plasmodium falciparum sporozoites for controlled human malaria infection: A dose-finding trial in two centres. Malar J (2015) 14:117. doi: 10.1186/s12936-015-0628-0
27. Laurens MB, Duncan CJ, Epstein JE, Hill AV, Komisar JL, Lyke KE, et al. A consultation on the optimization of controlled human malaria infection by mosquito bite for evaluation of candidate malaria vaccines. Vaccine (2012) 30(36):5302–4. doi: 10.1016/j.vaccine.2012.04.088
28. Shekalaghe S, Rutaihwa M, Billingsley PF, Chemba M, Daubenberger CA, James ER, et al. Controlled human malaria infection of tanzanians by intradermal injection of aseptic, purified, cryopreserved plasmodium falciparum sporozoites. Am J Trop Med Hyg (2014) 91(3):471–80. doi: 10.4269/ajtmh.14-0119
29. Hodgson SH, Juma E, Salim A, Magiri C, Kimani D, Njenga D, et al. Evaluating controlled human malaria infection in Kenyan adults with varying degrees of prior exposure to plasmodium falciparum using sporozoites administered by intramuscular injection. Front Microbiol (2014) 5:686. doi: 10.3389/fmicb.2014.00686
30. Kibwana E, Kapulu M, Bejon P. Controlled human malaria infection studies in Africa Past, present, and future. Berlin, Heidelberg: Springer Berlin Heidelberg (2022) p. 1–29.
31. James ER, Matheny S, Overby J, Sim BKL, Eappen AG, Li T, et al. A first for human vaccinology: Gmp compliant radiation attenuation of plasmodium falciparum sporozoites for production of a vaccine against malaria. Front Immunol (2022) 13:851028. doi: 10.3389/fimmu.2022.851028
32. Hoffman BU, Chattopadhyay R. Plasmodium falciparum: Effect of radiation on levels of gene transcripts in sporozoites. Exp Parasitol (2008) 118(2):247–52. doi: 10.1016/j.exppara.2007.08.014
33. Hoffman SL. Ruth Nussenzweig (1928-2018) malaria vaccine and immunology pioneer. Am J Trop Med Hyg (2018) 99(2):253–4. doi: 10.4269/ajtmh.18-1928
34. Clyde DF. Immunization of man against falciparum and vivax malaria by use of attenuated sporozoites. Am J Trop Med Hyg (1975) 24(3):397–401. doi: 10.4269/ajtmh.1975.24.397
35. Clyde DF. Immunity to falciparum and vivax malaria induced by irradiated sporozoites: A review of the university of Maryland studies, 1971-75. Bull World Health Organ (1990) 68 Suppl:9–12.
36. Rieckmann KH, Beaudoin RL, Cassells JS, Sell KW. Use of attenuated sporozoites in the immunization of human volunteers against falciparum malaria. Bull World Health Organ (1979) 57 Suppl 1:261–5.
37. Clyde DF, McCarthy VC, Miller RM, Hornick RB. Specificity of protection of man immunized against sporozoite-induced falciparum malaria. Am J Med Sci (1973) 266(6):398–403. doi: 10.1097/00000441-197312000-00001
38. Herrington D, Davis J, Nardin E, Beier M, Cortese J, Eddy H, et al. Successful immunization of humans with irradiated malaria sporozoites: Humoral and cellular responses of the protected individuals. Am J Trop Med Hyg (1991) 45(5):539–47. doi: 10.4269/ajtmh.1991.45.539
39. Edelman R, Hoffman SL, Davis JR, Beier M, Sztein MB, Losonsky G, et al. Long-term persistence of sterile immunity in a volunteer immunized with X-irradiated plasmodium falciparum sporozoites. J Infect Dis (1993) 168(4):1066–70. doi: 10.1093/infdis/168.4.1066
40. Luke TC, Hoffman SL. Rationale and plans for developing a non-replicating, metabolically active, radiation-attenuated plasmodium falciparum sporozoite vaccine. J Exp Biol (2003) 206(Pt 21):3803–8. doi: 10.1242/jeb.00644
41. Vanderberg JP. Reflections on early malaria vaccine studies, the first successful human malaria vaccination, and beyond. Vaccine (2009) 27(1):2–9. doi: 10.1016/j.vaccine.2008.10.028
42. Trager W, Jensen JB. Human malaria parasites in continuous culture. Science (1976) 193(4254):673–5. doi: 10.1126/science.781840
43. Campbell CC, Collins WE, Nguyen-Dinh P, Barber A, Broderson JR. Plasmodium falciparum gametocytes from culture in vitro develop to sporozoites that are infectious to primates. Science (1982) 217(4564):1048–50. doi: 10.1126/science.7051285
44. Hickey BW, Lumsden JM, Reyes S, Sedegah M, Hollingdale MR, Freilich DA, et al. Mosquito bite immunization with radiation-attenuated plasmodium falciparum sporozoites: Safety, tolerability, protective efficacy and humoral immunogenicity. Malar J (2016) 15(1):377. doi: 10.1186/s12936-016-1435-y
45. Hoffman SL, Billingsley PF, James E, Richman A, Loyevsky M, Li T, et al. Development of a metabolically active, non-replicating sporozoite vaccine to prevent plasmodium falciparum malaria. Hum Vaccin (2010) 6(1):97–106. doi: 10.4161/hv.6.1.10396
46. Epstein JE, Tewari K, Lyke KE, Sim BK, Billingsley PF, Laurens MB, et al. Live attenuated malaria vaccine designed to protect through hepatic Cd8(+) T cell immunity. Science (2011) 334(6055):475–80. doi: 10.1126/science.1211548
47. Seder RA, Chang LJ, Enama ME, Zephir KL, Sarwar UN, Gordon IJ, et al. Protection against malaria by intravenous immunization with a nonreplicating sporozoite vaccine. Science (2013) 341(6152):1359–65. doi: 10.1126/science.1241800
48. Ishizuka AS, Lyke KE, DeZure A, Berry AA, Richie TL, Mendoza FH, et al. Protection against malaria at 1 year and immune correlates following pfspz vaccination. Nat Med (2016) 22(6):614–23. doi: 10.1038/nm.4110
49. Richie TL, Billingsley PF, Sim BK, James ER, Chakravarty S, Epstein JE, et al. Progress with plasmodium falciparum sporozoite (Pfspz)-based malaria vaccines. Vaccine (2015) 33(52):7452–61. doi: 10.1016/j.vaccine.2015.09.096
50. Burkot TR, Williams JL, Schneider I. Infectivity to mosquitoes of plasmodium falciparum clones grown in vitro from the same isolate. Trans R Soc Trop Med Hyg (1984) 78(3):339–41. doi: 10.1016/0035-9203(84)90114-7
51. Laurens MB, Berry AA, Travassos MA, Strauss K, Adams M, Shrestha B, et al. Dose-dependent infectivity of aseptic, purified, cryopreserved plasmodium falciparum 7g8 sporozoites in malaria-naive adults. J Infect Dis (2019) 220(12):1962–6. doi: 10.1093/infdis/jiz410
52. Epstein JE, Paolino KM, Richie TL, Sedegah M, Singer A, Ruben AJ, et al. Protection against plasmodium falciparum malaria by pfspz vaccine. JCI Insight (2017) 2(1):e89154. doi: 10.1172/jci.insight.89154
53. Silva JC, Dwivedi A, Moser KA, Sissoko MS, Epstein JE, Healy SA, et al. Plasmodium falciparum 7g8 challenge provides conservative prediction of efficacy of Pfnf54-based pfspz vaccine in Africa. Nat Commun (2022) 13(1):3390. doi: 10.1038/s41467-022-30882-8
54. Lyke KE, Ishizuka AS, Berry AA, Chakravarty S, DeZure A, Enama ME, et al. Attenuated pfspz vaccine induces strain-transcending T cells and durable protection against heterologous controlled human malaria infection. Proc Natl Acad Sci U.S.A. (2017) 114(10):2711–6. doi: 10.1073/pnas.1615324114
55. Mordmuller B, Sulyok Z, Sulyok M, Molnar Z, Lalremruata A, Calle CL, et al. A pfspz vaccine immunization regimen equally protective against homologous and heterologous controlled human malaria infection. NPJ Vaccines (2022) 7(1):100. doi: 10.1038/s41541-022-00510-z
56. Sissoko MS, Healy SA, Katile A, Omaswa F, Zaidi I, Gabriel EE, et al. Safety and efficacy of pfspz vaccine against plasmodium falciparum Via direct venous inoculation in healthy malaria-exposed adults in Mali: A randomised, double-blind phase 1 trial. Lancet Infect Dis (2017) 17(5):498–509. doi: 10.1016/S1473-3099(17)30104-4
57. Jongo SA, Shekalaghe SA, Church LWP, Ruben AJ, Schindler T, Zenklusen I, et al. Safety, immunogenicity, and protective efficacy against controlled human malaria infection of plasmodium falciparum sporozoite vaccine in Tanzanian adults. Am J Trop Med Hyg (2018) 99(2):338–49. doi: 10.4269/ajtmh.17-1014
58. Olotu A, Urbano V, Hamad A, Eka M, Chemba M, Nyakarungu E, et al. Advancing global health through development and clinical trials partnerships: A randomized, placebo-controlled, double-blind assessment of safety, tolerability, and immunogenicity of pfspz vaccine for malaria in healthy equatoguinean men. Am J Trop Med Hyg (2018) 98(1):308–18. doi: 10.4269/ajtmh.17-0449
59. Jongo SA, Church LWP, Mtoro AT, Schindler T, Chakravarty S, Ruben AJ, et al. Increase of dose associated with decrease in protection against controlled human malaria infection by pfspz vaccine in Tanzanian adults. Clin Infect Dis (2020) 71(11):2849–57. doi: 10.1093/cid/ciz1152
60. Sissoko MS, Healy SA, Katile A, Zaidi I, Hu Z, Kamate B, et al. Safety and efficacy of a three-dose regimen of plasmodium falciparum sporozoite vaccine in adults during an intense malaria transmission season in Mali: A randomised, controlled phase 1 trial. Lancet Infect Dis (2022) 22(3):377–89. doi: 10.1016/S1473-3099(21)00332-7
61. Fernandez-Ruiz D, de Menezes MN, Holz LE, Ghilas S, Heath WR, Beattie L. Harnessing liver-resident memory T cells for protection against malaria. Expert Rev Vaccines (2021) 20(2):127–41. doi: 10.1080/14760584.2021.1881485
62. Lyke KE, Singer A, Berry AA, Reyes S, Chakravarty S, James ER, et al. Multidose priming and delayed boosting improve plasmodium falciparum sporozoite vaccine efficacy against heterologous p. falciparum controlled human malaria infection. Clin Infect Dis (2021) 73(7):e2424–e35. doi: 10.1093/cid/ciaa1294
63. Jongo SA, Church LWP, Nchama V, Hamad A, Chuquiyauri R, Kassim KR, et al. Multi-dose priming regimens of pfspz vaccine: Safety and efficacy against controlled human malaria infection in equatoguinean adults. Am J Trop Med Hyg (2022) 106(4):1215–26. doi: 10.4269/ajtmh.21-0942
64. WHO. World malaria report. Geneva: World Health Organization (2021). Licence: Cc by-Nc-Sa 3.0 Igo.
65. Jongo SA, Church LWP, Mtoro AT, Chakravarty S, Ruben AJ, Swanson PA, et al. Safety and differential antibody and T-cell responses to the plasmodium falciparum sporozoite malaria vaccine, pfspz vaccine, by age in Tanzanian adults, adolescents, children, and infants. Am J Trop Med Hyg (2019) 100(6):1433–44. doi: 10.4269/ajtmh.18-0835
66. Oneko M, Cherop YR, Sang T, Gutman JR, Wiegand R, Nyang’au EM, et al. Feasibility of direct venous inoculation of the radiation-attenuated plasmodium falciparum whole sporozoite vaccine in children and infants in siaya, Western Kenya. Vaccine (2020) 38(29):4592–600. doi: 10.1016/j.vaccine.2020.05.008
67. Steinhardt LC, Richie TL, Yego R, Akach D, Hamel MJ, Gutman JR, et al. Safety, tolerability, and immunogenicity of plasmodium falciparum sporozoite vaccine administered by direct venous inoculation to infants and young children: Findings from an age de-escalation, dose-escalation, double-blind, randomized controlled study in Western Kenya. Clin Infect Dis (2020) 71(4):1063–71. doi: 10.1093/cid/ciz925
68. Achieng F, Rosen JG, Cherop RY, Kariuki S, Hoffman SL, Seder R, et al. Caregiver and community perceptions and experiences participating in an infant malaria prevention trial of pfspz vaccine administered by direct venous inoculation: A qualitative study in siaya county, Western Kenya. Malar J (2020) 19(1):226. doi: 10.1186/s12936-020-03293-7
69. Oneko M, Steinhardt LC, Yego R, Wiegand RE, Swanson PA, Kc N, et al. Safety, immunogenicity and efficacy of pfspz vaccine against malaria in infants in Western Kenya: A double-blind, randomized, placebo-controlled phase 2 trial. Nat Med (2021) 27(9):1636–45. doi: 10.1038/s41591-021-01470-y
70. Greenwood BM, Bradley-Moore AM, Bryceson AD, Palit A. Immunosuppression in children with malaria. Lancet (1972) 1(7743):169–72. doi: 10.1016/s0140-6736(72)90569-7
71. Simon AK, Hollander GA, McMichael A. Evolution of the immune system in humans from infancy to old age. Proc Biol Sci (2015) 282(1821):20143085. doi: 10.1098/rspb.2014.3085
72. Kaiser K, Matuschewski K, Camargo N, Ross J, Kappe SH. Differential transcriptome profiling identifies plasmodium genes encoding pre-erythrocytic stage-specific proteins. Mol Microbiol (2004) 51(5):1221–32. doi: 10.1046/j.1365-2958.2003.03909.x
73. Kappe SH, Vaughan AM, Boddey JA, Cowman AF. That was then but this is now: Malaria research in the time of an eradication agenda. Science (2010) 328(5980):862–6. doi: 10.1126/science.1184785
74. Khan SM, Janse CJ, Kappe SH, Mikolajczak SA. Genetic engineering of attenuated malaria parasites for vaccination. Curr Opin Biotechnol (2012) 23(6):908–16. doi: 10.1016/j.copbio.2012.04.003
75. Butler NS, Schmidt NW, Vaughan AM, Aly AS, Kappe SH, Harty JT. Superior antimalarial immunity after vaccination with late liver stage-arresting genetically attenuated parasites. Cell Host Microbe (2011) 9(6):451–62. doi: 10.1016/j.chom.2011.05.008
76. Borrmann S, Matuschewski K. Targeting plasmodium liver stages: Better late than never. Trends Mol Med (2011) 17(9):527–36. doi: 10.1016/j.molmed.2011.05.008
77. Mueller AK, Labaied M, Kappe SH, Matuschewski K. Genetically modified plasmodium parasites as a protective experimental malaria vaccine. Nature (2005) 433(7022):164–7. doi: 10.1038/nature03188
78. van Dijk MR, Douradinha B, Franke-Fayard B, Heussler V, van Dooren MW, van Schaijk B, et al. Genetically attenuated, P36p-deficient malarial sporozoites induce protective immunity and apoptosis of infected liver cells. Proc Natl Acad Sci U.S.A. (2005) 102(34):12194–9. doi: 10.1073/pnas.0500925102
79. Labaied M, Harupa A, Dumpit RF, Coppens I, Mikolajczak SA, Kappe SH. Plasmodium yoelii sporozoites with simultaneous deletion of P52 and P36 are completely attenuated and confer sterile immunity against infection. Infect Immun (2007) 75(8):3758–68. doi: 10.1128/IAI.00225-07
80. Jobe O, Lumsden J, Mueller AK, Williams J, Silva-Rivera H, Kappe SH, et al. Genetically attenuated plasmodium berghei liver stages induce sterile protracted protection that is mediated by major histocompatibility complex class I-dependent interferon-Gamma-Producing Cd8+ T cells. J Infect Dis (2007) 196(4):599–607. doi: 10.1086/519743
81. Tarun AS, Dumpit RF, Camargo N, Labaied M, Liu P, Takagi A, et al. Protracted sterile protection with plasmodium yoelii pre-erythrocytic genetically attenuated parasite malaria vaccines is independent of significant liver-stage persistence and is mediated by Cd8+ T cells. J Infect Dis (2007) 196(4):608–16. doi: 10.1086/519742
82. Ting LM, Gissot M, Coppi A, Sinnis P, Kim K. Attenuated plasmodium yoelii lacking purine nucleoside phosphorylase confer protective immunity. Nat Med (2008) 14(9):954–8. doi: 10.1038/nm.1867
83. van der Velden M, Rijpma SR, Verweij V, van Gemert GJ, Chevalley-Maurel S, van de Vegte-Bolmer M, et al. Protective efficacy induced by genetically attenuated mid-to-Late liver-stage arresting plasmodium berghei Deltamrp2 parasites. Am J Trop Med Hyg (2016) 95(2):378–82. doi: 10.4269/ajtmh.16-0226
84. van Schaijk BC, Ploemen IH, Annoura T, Vos MW, Foquet L, van Gemert GJ, et al. A genetically attenuated malaria vaccine candidate based on p. falciparum B9/Slarp gene-deficient sporozoites. Elife (2014) 3:1–18. doi: 10.7554/eLife.03582
85. Goswami D, Minkah NK, Kappe SHI. Designer parasites: Genetically engineered plasmodium as vaccines to prevent malaria infection. J Immunol (2019) 202(1):20–8. doi: 10.4049/jimmunol.1800727
86. van Schaijk BC, Vos MW, Janse CJ, Sauerwein RW, Khan SM. Removal of heterologous sequences from plasmodium falciparum mutants using flpe-recombinase. PloS One (2010) 5(11):e15121. doi: 10.1371/journal.pone.0015121
87. VanBuskirk KM, O’Neill MT, de la Vega P, Maier AG, Krzych U, Williams J, et al. Preerythrocytic, live-attenuated plasmodium falciparum vaccine candidates by design. Proc Natl Acad Sci U.S.A. (2009) 106(31):13004–9. doi: 10.1073/pnas.0906387106
88. O’Neill MT, Phuong T, Healer J, Richard D, Cowman AF. Gene deletion from plasmodium falciparum using flp and cre recombinases: Implications for applied site-specific recombination. Int J Parasitol (2011) 41(1):117–23. doi: 10.1016/j.ijpara.2010.08.001
89. Kublin JG, Mikolajczak SA, Sack BK, Fishbaugher ME, Seilie A, Shelton L, et al. Complete attenuation of genetically engineered plasmodium falciparum sporozoites in human subjects. Sci Transl Med (2017) 9(371):1–11. doi: 10.1126/scitranslmed.aad9099
90. Goswami D, Betz W, Locham NK, Parthiban C, Brager C, Schafer C, et al. A replication-competent late liver stage-attenuated human malaria parasite. JCI Insight (2020) 5(13):1–19. doi: 10.1172/jci.insight.135589
91. Spring M, Murphy J, Nielsen R, Dowler M, Bennett JW, Zarling S, et al. First-in-Human evaluation of genetically attenuated plasmodium falciparum sporozoites administered by bite of anopheles mosquitoes to adult volunteers. Vaccine (2013) 31(43):4975–83. doi: 10.1016/j.vaccine.2013.08.007
92. Roestenberg M, Walk J, van der Boor SC, Langenberg MCC, Hoogerwerf MA, Janse JJ, et al. A double-blind, placebo-controlled phase 1/2a trial of the genetically attenuated malaria vaccine pfspz-Ga1. Sci Transl Med (2020) 12(544):1–11. doi: 10.1126/scitranslmed.aaz5629
93. Murphy SC, Vaughan AM, Kublin JG, Fishbauger M, Seilie AM, Cruz KP, et al. A genetically engineered plasmodium falciparum parasite vaccine provides protection from controlled human malaria infection. Sci Transl Med (2022) 14(659):eabn9709. doi: 10.1126/scitranslmed.abn9709
94. Bijker EM, Borrmann S, Kappe SH, Mordmuller B, Sack BK, Khan SM. Novel approaches to whole sporozoite vaccination against malaria. Vaccine (2015) 33(52):7462–8. doi: 10.1016/j.vaccine.2015.09.095
95. Marques-da-Silva C, Peissig K, Kurup SP. Pre-erythrocytic vaccines against malaria. Vaccines (Basel) (2020) 8(3):1–15. doi: 10.3390/vaccines8030400
96. Orjih AU, Cochrane AH, Nussenzweig RS. Comparative studies on the immunogenicity of infective and attenuated sporozoites of plasmodium berghei. Trans R Soc Trop Med Hyg (1982) 76(1):57–61. doi: 10.1016/0035-9203(82)90019-0
97. Beaudoin RL, Strome CP, Mitchell F, Tubergen TA. Plasmodium berghei: Immunization of mice against the anka strain using the unaltered sporozoite as an antigen. Exp Parasitol (1977) 42(1):1–5. doi: 10.1016/0014-4894(77)90054-6
98. Belnoue E, Costa FT, Frankenberg T, Vigario AM, Voza T, Leroy N, et al. Protective T cell immunity against malaria liver stage after vaccination with live sporozoites under chloroquine treatment. J Immunol (2004) 172(4):2487–95. doi: 10.4049/jimmunol.172.4.2487
99. Yayon A, Vande Waa JA, Yayon M, Geary TG, Jensen JB. Stage-dependent effects of chloroquine on plasmodium falciparum in vitro. J Protozool (1983) 30(4):642–7. doi: 10.1111/j.1550-7408.1983.tb05336.x
100. Putrianti ED, Silvie O, Kordes M, Borrmann S, Matuschewski K. Vaccine-like immunity against malaria by repeated causal-prophylactic treatment of liver-stage plasmodium parasites. J Infect Dis (2009) 199(6):899–903. doi: 10.1086/597121
101. Culleton RL, Inoue M, Reece SE, Cheesman S, Carter R. Strain-specific immunity induced by immunization with pre-erythrocytic stages of plasmodium chabaudi. Parasit Immunol (2011) 33(1):73–8. doi: 10.1111/j.1365-3024.2010.01251.x
102. Friesen J, Borrmann S, Matuschewski K. Induction of antimalaria immunity by pyrimethamine prophylaxis during exposure to sporozoites is curtailed by parasite resistance. Antimicrob Agents Chemother (2011) 55(6):2760–7. doi: 10.1128/AAC.01717-10
103. Pfeil J, Sepp KJ, Heiss K, Meister M, Mueller AK, Borrmann S. Protection against malaria by immunization with non-attenuated sporozoites under single-dose piperaquine-tetraphosphate chemoprophylaxis. Vaccine (2014) 32(45):6005–11. doi: 10.1016/j.vaccine.2014.07.112
104. Peng X, Keitany GJ, Vignali M, Chen L, Gibson C, Choi K, et al. Artesunate versus chloroquine infection-Treatment-Vaccination defines stage-specific immune responses associated with prolonged sterile protection against both pre-erythrocytic and erythrocytic plasmodium yoelii infection. J Immunol (2014) 193(3):1268–77. doi: 10.4049/jimmunol.1400296
105. Friesen J, Silvie O, Putrianti ED, Hafalla JC, Matuschewski K, Borrmann S. Natural immunization against malaria: Causal prophylaxis with antibiotics. Sci Transl Med (2010) 2(40):40ra9. doi: 10.1126/scitranslmed.3001058
106. Bhardwaj J, Siddiqui AJ, Goyal M, Prakash K, Soni A, Puri SK. Repetitive live sporozoites inoculation under arteether chemoprophylaxis confers protection against subsequent sporozoite challenge in rodent malaria model. Acta Trop (2016) 158:130–8. doi: 10.1016/j.actatropica.2016.02.016
107. Pichyangkul S, Spring MD, Yongvanitchit K, Kum-Arb U, Limsalakpetch A, Im-Erbsin R, et al. Chemoprophylaxis with sporozoite immunization in p. knowlesi rhesus monkeys confers protection and elicits sporozoite-specific memory T cells in the liver. PloS One (2017) 12(2):e0171826. doi: 10.1371/journal.pone.0171826
108. Roestenberg M, McCall M, Hopman J, Wiersma J, Luty AJ, van Gemert GJ, et al. Protection against a malaria challenge by sporozoite inoculation. N Engl J Med (2009) 361(5):468–77. doi: 10.1056/NEJMoa0805832
109. Roestenberg M, Teirlinck AC, McCall MB, Teelen K, Makamdop KN, Wiersma J, et al. Long-term protection against malaria after experimental sporozoite inoculation: An open-label follow-up study. Lancet (2011) 377(9779):1770–6. doi: 10.1016/S0140-6736(11)60360-7
110. Bijker EM, Bastiaens GJ, Teirlinck AC, van Gemert GJ, Graumans W, van de Vegte-Bolmer M, et al. Protection against malaria after immunization by chloroquine prophylaxis and sporozoites is mediated by preerythrocytic immunity. Proc Natl Acad Sci U.S.A. (2013) 110(19):7862–7. doi: 10.1073/pnas.1220360110
111. Bijker EM, Schats R, Obiero JM, Behet MC, van Gemert GJ, van de Vegte-Bolmer M, et al. Sporozoite immunization of human volunteers under mefloquine prophylaxis is safe, immunogenic and protective: A double-blind randomized controlled clinical trial. PloS One (2014) 9(11):e112910. doi: 10.1371/journal.pone.0112910
112. Schats R, Bijker EM, van Gemert GJ, Graumans W, van de Vegte-Bolmer M, van Lieshout L, et al. Heterologous protection against malaria after immunization with plasmodium falciparum sporozoites. PloS One (2015) 10(5):e0124243. doi: 10.1371/journal.pone.0124243
113. Walk J, Reuling IJ, Behet MC, Meerstein-Kessel L, Graumans W, van Gemert GJ, et al. Modest heterologous protection after plasmodium falciparum sporozoite immunization: A double-blind randomized controlled clinical trial. BMC Med (2017) 15(1):168. doi: 10.1186/s12916-017-0923-4
114. Bastiaens GJH, van Meer MPA, Scholzen A, Obiero JM, Vatanshenassan M, van Grinsven T, et al. Safety, immunogenicity, and protective efficacy of intradermal immunization with aseptic, purified, cryopreserved plasmodium falciparum sporozoites in volunteers under chloroquine prophylaxis: A randomized controlled trial. Am J Trop Med Hyg (2016) 94(3):663–73. doi: 10.4269/ajtmh.15-0621
115. Mordmuller B, Surat G, Lagler H, Chakravarty S, Ishizuka AS, Lalremruata A, et al. Sterile protection against human malaria by chemoattenuated pfspz vaccine. Nature (2017) 542(7642):445–9. doi: 10.1038/nature21060
116. Draper SJ, Sack BK, King CR, Nielsen CM, Rayner JC, Higgins MK, et al. Malaria vaccines: Recent advances and new horizons. Cell Host Microbe (2018) 24(1):43–56. doi: 10.1016/j.chom.2018.06.008
117. Jongo SA, Urbano V, Church LWP, Olotu A, Manock SR, Schindler T, et al. Immunogenicity and protective efficacy of radiation-attenuated and chemo-attenuated pfspz vaccines in equatoguinean adults. Am J Trop Med Hyg (2021) 104(1):283–93. doi: 10.4269/ajtmh.20-0435
118. Mwakingwe-Omari A, Healy SA, Lane J, Cook DM, Kalhori S, Wyatt C, et al. Two chemoattenuated pfspz malaria vaccines induce sterile hepatic immunity. Nature (2021) 595(7866):289–94. doi: 10.1038/s41586-021-03684-z
119. Coulibaly D, Kone AK, Traore K, Niangaly A, Kouriba B, Arama C, et al. Pfspz-cvac malaria vaccine demonstrates safety among malaria-experienced adults: A randomized, controlled phase 1 trial. EClinicalMedicine (2022) 52:101579. doi: 10.1016/j.eclinm.2022.101579
120. Sulyok Z, Fendel R, Eder B, Lorenz FR, Kc N, Karnahl M, et al. Heterologous protection against malaria by a simple chemoattenuated pfspz vaccine regimen in a randomized trial. Nat Commun (2021) 12(1):2518. doi: 10.1038/s41467-021-22740-w
121. Murphy SC, Deye GA, Sim BKL, Galbiati S, Kennedy JK, Cohen KW, et al. Pfspz-cvac efficacy against malaria increases from 0% to 75% when administered in the absence of erythrocyte stage parasitemia: A randomized, placebo-controlled trial with controlled human malaria infection. PloS Pathog (2021) 17(5):e1009594. doi: 10.1371/journal.ppat.1009594
122. Ibanez J, Fendel R, Lorenz FR, Granados-Bayon P, Bruckner S, Esen M, et al. Efficacy, T cell activation and antibody responses in accelerated plasmodium falciparum sporozoite chemoprophylaxis vaccine regimens. NPJ Vaccines (2022) 7(1):59. doi: 10.1038/s41541-022-00473-1
123. Prudencio M, Mota MM, Mendes AM. A toolbox to study liver stage malaria. Trends Parasitol (2011) 27(12):565–74. doi: 10.1016/j.pt.2011.09.004
124. Plotkin S. History of vaccination. Proc Natl Acad Sci U.S.A. (2014) 111(34):12283–7. doi: 10.1073/pnas.1400472111
125. Mitran CJ, Yanow SK. The case for exploiting cross-species epitopes in malaria vaccine design. Front Immunol (2020) 11:335. doi: 10.3389/fimmu.2020.00335
126. Mendes AM, Machado M, Goncalves-Rosa N, Reuling IJ, Foquet L, Marques C, et al. A plasmodium berghei sporozoite-based vaccination platform against human malaria. NPJ Vaccines (2018) 3:33. doi: 10.1038/s41541-018-0068-2
127. Lin JW, Annoura T, Sajid M, Chevalley-Maurel S, Ramesar J, Klop O, et al. A novel ‘Gene Insertion/Marker out’ (Gimo) method for transgene expression and gene complementation in rodent malaria parasites. PloS One (2011) 6(12):e29289. doi: 10.1371/journal.pone.0029289
128. Mendes AM, Scholzen A, Mueller A-K, Khan SM, Sauerwein RW, Prudêncio M. Whole-sporozoite malaria vaccines. In: Mota MM, Rodriguez A, editors. Malaria. Cham, Switzerland: Springer (2017).
129. Mendes AM, Reuling IJ, Andrade CM, Otto TD, Machado M, Teixeira F, et al. Pre-clinical evaluation of a p. berghei-based whole-sporozoite malaria vaccine candidate. NPJ Vaccines (2018) 3:54. doi: 10.1038/s41541-018-0091-3
130. Reuling IJ, Mendes AM, de Jong GM, Fabra-Garcia A, Nunes-Cabaco H, van Gemert GJ, et al. An open-label phase 1/2a trial of a genetically modified rodent malaria parasite for immunization against plasmodium falciparum malaria. Sci Transl Med (2020) 12(544):1–12. doi: 10.1126/scitranslmed.aay2578
131. Salman AM, Mogollon CM, Lin JW, van Pul FJ, Janse CJ, Khan SM. Generation of transgenic rodent malaria parasites expressing human malaria parasite proteins. Methods Mol Biol (2015) 1325:257–86. doi: 10.1007/978-1-4939-2815-6_21
132. Caldelari R, Dogga S, Schmid MW, Franke-Fayard B, Janse CJ, Soldati-Favre D, et al. Transcriptome analysis of plasmodium berghei during exo-erythrocytic development. Malar J (2019) 18(1):330. doi: 10.1186/s12936-019-2968-7
133. Bermudez M, Moreno-Perez DA, Arevalo-Pinzon G, Curtidor H, Patarroyo MA. Plasmodium vivax in vitro continuous culture: The spoke in the wheel. Malar J (2018) 17(1):301. doi: 10.1186/s12936-018-2456-5
134. Cockburn IA, Seder RA. Malaria prevention: From immunological concepts to effective vaccines and protective antibodies. Nat Immunol (2018) 19(11):1199–211. doi: 10.1038/s41590-018-0228-6
135. Goh YS, McGuire D, Renia L. Vaccination with sporozoites: Models and correlates of protection. Front Immunol (2019) 10:1227. doi: 10.3389/fimmu.2019.01227
136. Stanisic DI, McCall MBB. Correlates of malaria vaccine efficacy. Expert Rev Vaccines (2021) 20(2):143–61. doi: 10.1080/14760584.2021.1882309
137. Kurup SP, Butler NS, Harty JT. T Cell-mediated immunity to malaria. Nat Rev Immunol (2019) 19(7):457–71. doi: 10.1038/s41577-019-0158-z
138. Mendes AM, Scholzen A, Mueller AK, Khan SM, Sauerwein RW, Prudencio M. Whole-sporozoite malaria vaccines. In: Mota MM, Rodriguez A, editors. Malaria: Immune response to infection and vaccination. Cham: Springer International Publishing (2017). p. 99–137.
139. Tan J, Piccoli L, Lanzavecchia A. The antibody response to plasmodium falciparum: Cues for vaccine design and the discovery of receptor-based antibodies. Annu Rev Immunol (2019) 37:225–46. doi: 10.1146/annurev-immunol-042617-053301
140. Camponovo F, Campo JJ, Le TQ, Oberai A, Hung C, Pablo JV, et al. Proteome-wide analysis of a malaria vaccine study reveals personalized humoral immune profiles in Tanzanian adults. Elife (2020) 9:1–21. doi: 10.7554/eLife.53080
141. Vanderberg JP, Frevert U. Intravital microscopy demonstrating antibody-mediated immobilisation of plasmodium berghei sporozoites injected into skin by mosquitoes. Int J Parasitol (2004) 34(9):991–6. doi: 10.1016/j.ijpara.2004.05.005
142. Nudelman S, Renia L, Charoenvit Y, Yuan L, Miltgen F, Beaudoin RL, et al. Dual action of anti-sporozoite antibodies in vitro. J Immunol (1989) 143(3):996–1000.
143. Renia L, Mattei D, Goma J, Pied S, Dubois P, Miltgen F, et al. A malaria heat-Shock-Like determinant expressed on the infected hepatocyte surface is the target of antibody-dependent cell-mediated cytotoxic mechanisms by nonparenchymal liver cells. Eur J Immunol (1990) 20(7):1445–9. doi: 10.1002/eji.1830200706
144. Aliprandini E, Tavares J, Panatieri RH, Thiberge S, Yamamoto MM, Silvie O, et al. Cytotoxic anti-circumsporozoite antibodies target malaria sporozoites in the host skin. Nat Microbiol (2018) 3(11):1224–33. doi: 10.1038/s41564-018-0254-z
145. Moita D, Nunes-Cabaco H, Mendes AM, Prudencio M. A guide to investigating immune responses elicited by whole-sporozoite pre-erythrocytic vaccines against malaria. FEBS J (2022) 289(12):3335–59. doi: 10.1111/febs.16016
146. Nussenzweig RS, Vanderberg JP, Sanabria Y, Most H. Plasmodium berghei: Accelerated clearance of sporozoites from blood as part of immune-mechanism in mice. Exp Parasitol (1972) 31(1):88–97. doi: 10.1016/0014-4894(72)90051-3
147. Behet MC, Foquet L, van Gemert GJ, Bijker EM, Meuleman P, Leroux-Roels G, et al. Sporozoite immunization of human volunteers under chemoprophylaxis induces functional antibodies against pre-erythrocytic stages of plasmodium falciparum. Malar J (2014) 13:136. doi: 10.1186/1475-2875-13-136
148. Nahrendorf W, Scholzen A, Bijker EM, Teirlinck AC, Bastiaens GJ, Schats R, et al. Memory b-cell and antibody responses induced by plasmodium falciparum sporozoite immunization. J Infect Dis (2014) 210(12):1981–90. doi: 10.1093/infdis/jiu354
149. Tan J, Sack BK, Oyen D, Zenklusen I, Piccoli L, Barbieri S, et al. A public antibody lineage that potently inhibits malaria infection through dual binding to the circumsporozoite protein. Nat Med (2018) 24(4):401–7. doi: 10.1038/nm.4513
150. Zenklusen I, Jongo S, Abdulla S, Ramadhani K, Lee Sim BK, Cardamone H, et al. Immunization of malaria-preexposed volunteers with pfspz vaccine elicits long-lived igm invasion-inhibitory and complement-fixing antibodies. J Infect Dis (2018) 217(10):1569–78. doi: 10.1093/infdis/jiy080
151. Kisalu NK, Idris AH, Weidle C, Flores-Garcia Y, Flynn BJ, Sack BK, et al. A human monoclonal antibody prevents malaria infection by targeting a new site of vulnerability on the parasite. Nat Med (2018) 24(4):408–16. doi: 10.1038/nm.4512
152. Fabra-Garcia A, Yang AS, Behet MC, Yap Z, van Waardenburg Y, Kaviraj S, et al. Human antibodies against noncircumsporozoite proteins block plasmodium falciparum parasite development in hepatocytes. JCI Insight (2022) 7(6):1–12. doi: 10.1172/jci.insight.153524
153. Ogwang C, Kimani D, Edwards NJ, Roberts R, Mwacharo J, Bowyer G, et al. Prime-boost vaccination with chimpanzee adenovirus and modified vaccinia Ankara encoding trap provides partial protection against plasmodium falciparum infection in Kenyan adults. Sci Transl Med (2015) 7(286):286re5. doi: 10.1126/scitranslmed.aaa2373
154. Espinosa DA, Vega-Rodriguez J, Flores-Garcia Y, Noe AR, Munoz C, Coleman R, et al. The plasmodium falciparum cell-traversal protein for ookinetes and sporozoites as a candidate for preerythrocytic and transmission-blocking vaccines. Infect Immun (2017) 85(2):1–12. doi: 10.1128/IAI.00498-16
155. Schofield L, Villaquiran J, Ferreira A, Schellekens H, Nussenzweig R, Nussenzweig V. Gamma interferon, Cd8+ T cells and antibodies required for immunity to malaria sporozoites. Nature (1987) 330(6149):664–6. doi: 10.1038/330664a0
156. Weiss WR, Jiang CG. Protective Cd8+ T lymphocytes in primates immunized with malaria sporozoites. PloS One (2012) 7(2):e31247. doi: 10.1371/journal.pone.0031247
157. Butler NS, Schmidt NW, Harty JT. Differential effector pathways regulate memory Cd8 T cell immunity against plasmodium berghei versus p. yoelii sporozoites. J Immunol (2010) 184(5):2528–38. doi: 10.4049/jimmunol.0903529
158. Weiss WR, Sedegah M, Beaudoin RL, Miller LH, Good MF. Cd8+ T cells (Cytotoxic/Suppressors) are required for protection in mice immunized with malaria sporozoites. Proc Natl Acad Sci U.S.A. (1988) 85(2):573–6. doi: 10.1073/pnas.85.2.573
159. Mellouk S, Lunel F, Sedegah M, Beaudoin RL, Druilhe P. Protection against malaria induced by irradiated sporozoites. Lancet (1990) 335(8691):721. doi: 10.1016/0140-6736(90)90832-p
160. Carvalho LH, Sano G, Hafalla JC, Morrot A, Curotto de Lafaille MA, Zavala F. Il-4-Secreting Cd4+ T cells are crucial to the development of Cd8+ T-cell responses against malaria liver stages. Nat Med (2002) 8(2):166–70. doi: 10.1038/nm0202-166
161. Overstreet MG, Chen YC, Cockburn IA, Tse SW, Zavala F. Cd4+ T cells modulate expansion and survival but not functional properties of effector and memory Cd8+ T cells induced by malaria sporozoites. PloS One (2011) 6(1):e15948. doi: 10.1371/journal.pone.0015948
162. Obeng-Adjei N, Portugal S, Tran TM, Yazew TB, Skinner J, Li S, et al. Circulating Th1-Cell-Type tfh cells that exhibit impaired b cell help are preferentially activated during acute malaria in children. Cell Rep (2015) 13(2):425–39. doi: 10.1016/j.celrep.2015.09.004
163. Ryg-Cornejo V, Ioannidis LJ, Ly A, Chiu CY, Tellier J, Hill DL, et al. Severe malaria infections impair germinal center responses by inhibiting T follicular helper cell differentiation. Cell Rep (2016) 14(1):68–81. doi: 10.1016/j.celrep.2015.12.006
164. Oliveira GA, Kumar KA, Calvo-Calle JM, Othoro C, Altszuler D, Nussenzweig V, et al. Class ii-restricted protective immunity induced by malaria sporozoites. Infect Immun (2008) 76(3):1200–6. doi: 10.1128/IAI.00566-07
165. Lawand M, Dechanet-Merville J, Dieu-Nosjean MC. Key features of gamma-delta T-cell subsets in human diseases and their immunotherapeutic implications. Front Immunol (2017) 8:761. doi: 10.3389/fimmu.2017.00761
166. Kumar A, Singh B, Tiwari V, Singh VK, Sundar SS, Kumar R. Emerging role of Γδ T cells in protozoan infection and their potential clinical application. Infect Genet Evol (2022) 98(105210):1–7. doi: 10.1016/j.meegid.2022.105210
167. D’Ombrain MC, Robinson LJ, Stanisic DI, Taraika J, Bernard N, Michon P, et al. Association of early interferon-gamma production with immunity to clinical malaria: A longitudinal study among Papua new guinean children. Clin Infect Dis (2008) 47(11):1380–7. doi: 10.1086/592971
168. Costa G, Loizon S, Guenot M, Mocan I, Halary F, de Saint-Basile G, et al. Control of plasmodium falciparum erythrocytic cycle: Gammadelta T cells target the red blood cell-invasive merozoites. Blood (2011) 118(26):6952–62. doi: 10.1182/blood-2011-08-376111
169. Tsuji M, Mombaerts P, Lefrancois L, Nussenzweig RS, Zavala F, Tonegawa S. Gamma delta T cells contribute to immunity against the liver stages of malaria in alpha beta T-Cell-Deficient mice. Proc Natl Acad Sci U.S.A. (1994) 91(1):345–9. doi: 10.1073/pnas.91.1.345
170. Zaidi I, Diallo H, Conteh S, Robbins Y, Kolasny J, Orr-Gonzalez S, et al. Gammadelta T cells are required for the induction of sterile immunity during irradiated sporozoite vaccinations. J Immunol (2017) 199(11):3781–8. doi: 10.4049/jimmunol.1700314
171. Deroost K, Langhorne J. Gamma/Delta T cells and their role in protection against malaria. Front Immunol (2018) 9:2973. doi: 10.3389/fimmu.2018.02973
172. Burrack KS, Hart GT, Hamilton SE. Contributions of natural killer cells to the immune response against plasmodium. Malar J (2019) 18(1):321. doi: 10.1186/s12936-019-2953-1
173. Vasan S, Tsuji M. A double-edged sword: The role of nkt cells in malaria and hiv infection and immunity. Semin Immunol (2010) 22(2):87–96. doi: 10.1016/j.smim.2009.11.001
174. Teirlinck AC, McCall MB, Roestenberg M, Scholzen A, Woestenenk R, de Mast Q, et al. Longevity and composition of cellular immune responses following experimental plasmodium falciparum malaria infection in humans. PloS Pathog (2011) 7(12):e1002389. doi: 10.1371/journal.ppat.1002389
175. Moncunill G, Scholzen A, Mpina M, Nhabomba A, Hounkpatin AB, Osaba L, et al. Antigen-stimulated pbmc transcriptional protective signatures for malaria immunization. Sci Transl Med (2020) 12(543):1–17. doi: 10.1126/scitranslmed.aay8924
Keywords: vaccine, plasmodium, sporozoite, clinical trial, protective efficacy, immunogenicity
Citation: Nunes-Cabaço H, Moita D and Prudêncio M (2022) Five decades of clinical assessment of whole-sporozoite malaria vaccines. Front. Immunol. 13:977472. doi: 10.3389/fimmu.2022.977472
Received: 24 June 2022; Accepted: 17 August 2022;
Published: 08 September 2022.
Edited by:
Benjamin Mordmüller, Radboud University Medical Centre, NetherlandsReviewed by:
Ashley Vaughan, Seattle Children’s Research Institute, United StatesClaudia Andrea Daubenberger, Swiss Tropical and Public Health Institute (Swiss TPH), Switzerland
Annie Shu-Ping Yang, Radboud University Medical Centre, Netherlands
Copyright © 2022 Nunes-Cabaço, Moita and Prudêncio. This is an open-access article distributed under the terms of the Creative Commons Attribution License (CC BY). The use, distribution or reproduction in other forums is permitted, provided the original author(s) and the copyright owner(s) are credited and that the original publication in this journal is cited, in accordance with accepted academic practice. No use, distribution or reproduction is permitted which does not comply with these terms.
*Correspondence: Miguel Prudêncio, bXBydWRlbmNpb0BtZWRpY2luYS51bGlzYm9hLnB0