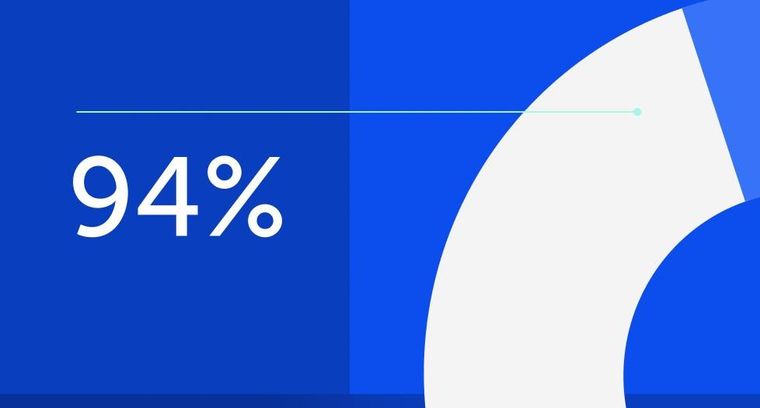
94% of researchers rate our articles as excellent or good
Learn more about the work of our research integrity team to safeguard the quality of each article we publish.
Find out more
SYSTEMATIC REVIEW article
Front. Immunol., 31 October 2022
Sec. Multiple Sclerosis and Neuroimmunology
Volume 13 - 2022 | https://doi.org/10.3389/fimmu.2022.975921
This article is part of the Research TopicImmune Response in Neurodegenerative Diseases, Cerebrovascular Disease, Intracranial and Skull Base TumorsView all 5 articles
The high morbidity, mortality, and disability rates associated with cerebrovascular disease (CeVD) pose a severe danger to human health. Gut bacteria significantly affect the onset, progression, and prognosis of CeVD. Gut microbes play a critical role in gut-brain interactions, and the gut-brain axis is essential for communication in CeVD. The reflection of changes in the gut and brain caused by gut bacteria makes it possible to investigate early warning biomarkers and potential treatment targets. We primarily discussed the following three levels of brain-gut interactions in a systematic review of the connections between gut microbiota and several cerebrovascular conditions, including ischemic stroke, intracerebral hemorrhage, intracranial aneurysm, cerebral small vessel disease, and cerebral cavernous hemangioma. First, we studied the gut microbes in conjunction with CeVD and examined alterations in the core microbiota. This enabled us to identify the focus of gut microbes and determine the focus for CeVD prevention and treatment. Second, we discussed the pathological mechanisms underlying the involvement of gut microbes in CeVD occurrence and development, including immune-mediated inflammatory responses, variations in intestinal barrier function, and reciprocal effects of microbial metabolites. Finally, based on the aforementioned proven mechanisms, we assessed the effectiveness and potential applications of the current therapies, such as dietary intervention, fecal bacterial transplantation, traditional Chinese medicine, and antibiotic therapy.
Given their high mortality and disability rates, cerebrovascular diseases (CeVDs) seriously threaten human health and place significant strain on healthcare systems, particularly in developing nations (1, 2). CeVDs include ischemic stroke (IS), intracerebral hemorrhage (ICH), cerebral cavernous malformation (CCM), intracranial aneurysm (IA), and cerebral small vessel disease (CSVD), among others. IS, which accounts for approximately 80% of CeVD occurrences, has the highest incidence and prevalence (3). Additionally, due to its high mortality, impairment rates (between 30% and 40%), and prevalence (between 10% and 15%), ICH is the second most common CeVD after IS (3). CCM and IA are significant risk factors for ICH (4, 5). They may bleed and rupture unexpectedly, causing critical neurological illnesses and a significant disease burden (4, 5).
Patients’ quality of life is significantly reduced by CSVD, which makes them prone to dementia, stroke, and cognitive dysfunction (6). In addition, pathophysiological changes, such as transient and permanent cessation of oxygen supply to brain tissues and the energy metabolism of brain cells, are the results of an IS. Furthermore, an IS leads to excitotoxicity, oxidative stress, neuroinflammation, apoptosis, amyloid production, tau protein dysfunction, and other diseases (7–10). In turn, these diseases cause neurological deficits, changes in memory and intelligence, movement disorders, tissue compression, and other systemic and local brain function damage (7–10). In addition, increased intracranial pressure, hematoma compression, and severe brain edema are all caused by ICH, which leads to neuroinflammation, apoptosis, membrane depolarization, mitochondrial dysfunction, and other negative effects (11–16). Finally, patients may have a poor prognosis with impaired motor function, cognitive impairment, seizure-like epilepsy, and impaired consciousness (11–16). Therefore, early diagnosis and treatment of CeVD have important clinical implications.
Diet has been identified as a major risk factor for CeVD, and improper diet and nutrition are strongly associated with the likelihood of a first stroke (17, 18). Rapid economic development has progressively altered people’s diets, and the consumption of foods high in cholesterol, such as red meat and egg yolks, has increased dramatically, resulting in a 26.6% increase in the stroke mortality rate (18, 19). Research on how diet affects CeVD has shown that gut microorganisms are primary regulators (18, 20). Intestinal microbes refer to the many microorganisms residing in the human gastrointestinal tract that rely on the human intestinal environment for survival and aid in the execution of immunological, metabolic, and endocrine activities (21).
Therefore, we extensively surveyed the literature on gut microbes and CeVD from 2012 to date (Figure 1). First, we summarized and analyzed the core microbes affecting CeVD among the various gut microbes and the microbial differences affecting various CeVDs. Second, we explored the interaction mechanisms between gut microbes and CeVD, including immunomodulation of the inflammatory response, gut barrier failure, and bidirectional effects of microbial metabolites. Finally, we explored the efficacy and applicability of existing CeVD therapies at the gut microbial level based on the pathways mentioned above. These therapies include flora transplantation, traditional Chinese medicine (TCM), and antibiotic therapy. Research on gut microbes and even the gut-brain axis ultimately aims to solve the problems associated with CeVD and minimize the threat of these diseases.
The evolution of the intestinal microbiota began with the colonization of parthenogenic anaerobic bacteria (Denatobacteria), followed by the growth of anaerobic bacteria (Lactobacillus and Bifidobacteria), and then evolved into diverse bacteria and various species of Bacteroidetes survived together (22, 23). With solid food consumption, intestinal microbes form a stable colony, which is then classified into three types according to their function. “Beneficial bacteria” help the body absorb nutrients, boost vitamin synthesis, resist the invasion of pathogenic microbes and toxic metabolites, and preserve the host’s homeostasis (22, 23). However, variations in beneficial bacteria can result in malnutrition, trace element insufficiency, systemic immunological problems, and pathological damage. The second category consists of “neutral bacteria,” which reside in the gut but are nonfunctional and have minimal health effects (22, 23). The third category is “dangerous bacteria,” which frequently impairs intestinal function, creates toxic compounds in the host, and weakens the body’s immune system (22, 23). Typically, these three types of bacteria coexist without causing any harm to their host’s health. The three groups of intestinal microorganisms are not mutually exclusive, though. The “beneficial bacteria,” “ neutral bacteria,” and “ dangerous bacteria” may transformation between states of the intestinal microbes especially in different diseases.
The dynamic equilibrium of the intestinal flora can be disrupted by immune system dysfunction or fluctuations in the intestinal environment. The amount of “beneficial bacteria” will decrease or exhibit abnormal functions, while the “dangerous microorganisms” reproduce rapidly (24–27). The human body absorbs numerous disease-causing chemicals through the digestive tract (24–27); this affects digestive function and the gut microbial balance. With intestinal ecological imbalance, intestinal mucosal immune function is disrupted, gastrointestinal permeability increases, massive bacterial translocation occurs, inflammatory factors spread, and intestinal immune dysfunction and pathogen resistance are diminished (24–27). Furthermore, changes in the dominant flora of gut microbes can also result in changes in gastrointestinal metabolites, such as a decrease in short-chain fatty acids (SCFAs) and an increase in trimethylamine-N-oxide (TMAO) (27–29). These metabolites are closely associated with neuroinflammation, post-stroke infection, and secondary brain injury. When intestinal microbial homeostasis is dysregulated, intestinal inflammatory factors such as T helper type (Th) 1, Th17, and interleukin IL-6 are released in large amounts. The release of these inflammatory factors results in intestinal permeability changes, barrier dysfunction, extravasation of inflammatory substances into peripheral blood, and transportation to the blood-brain barrier (BBB). Ultimately, they act on the cerebrovascular system and play a crucial role in CeVD occurrence, development, and prognosis (30–33). In CeVD research (34–37), the clinical efficacy of fecal bacterial transplantation (FMT), traditional Chinese medicine intervention, antibiotic intervention, and SCFA supplementation remains contentious.
Based on the above background, the specific composition of microbiota associated with susceptibility to CeVD is beneficial in understanding the disease pathogenesis. In a series of case-control and animal model studies (20, 24, 25, 27, 29, 38–61) a correlation between IS and altered intestinal microflora was found. Figures 2, 3 and Supplementary Table 1 show that the core florae of IS lesions are Actinobacteria, Lentisphaerae, Thickobacteria, and Bacteroides (20, 24, 25, 27, 29, 38–61). Proteobacteria and Firmicutes showed an overall increasing trend, while Bacteroidetes showed a decreasing trend, and there were some contradictions in the changes in Actinobacteria (20, 24, 25, 27, 29, 38–61). In addition, there were changes in Verrucomicrobia, Lentisphaerae, Candidatus, Parcubacteria, Deferribacteres, and other species of bacteria (20, 27, 41–44, 48, 54). However, there were some differences in the changes in different bacteria, different species of bacteria, and different lesion periods within each group.
Figure 2 Chart of intestinal flora changes in cerebrovascular diseases. The abscissa represents different bacteria, and the ordinate shows the number of changes in flora. (A) Changes of actinobacteria after ischemic stroke. (B) Changes of Firmicutes after ischemic stroke. (C) Changes of Bacteroidetes after ischemic stroke. (D) Changes of Proteobacteria after ischemic stroke. (E) Changes of other bacterias after ischemic stroke. (F) Changes of intestinal flora after intracerebral hemorrhage. (G) Changes of intestinal flora after cerebral cavernous hemangioma. (H) Changes of intestinal flora after intracranial aneurysm.
One study has reported that before a stroke occurs, the abundance of Enterobacteriaceae and Veillonellaceae species increased in the high-risk IS group, indicating that an increase in the abundance of opportunistic infections is related to an increased risk of IS (38). Using 16S rRNA V4 tags, Illumina sequencing revealed a significant difference between IS and asymptomatic patients. In IS, the incidence of opportunistic gut pathogens, such as Enterobacter, Megasphaera, Oscillibacter, and Desulfovibrio, increased. Simultaneously, symbiotic and beneficial bacteria such as Bacteroides, Prevotella, and Faecalibacterium were diminished (27, 62). This alteration is also confirmed in atherosclerotic stroke, characterized by overgrowth of gut pathogens (e.g., Desulfovibrio and Enterococcus), opportunistic pathogens (e.g., Eubacterium), and a few commensals or beneficial species (e.g., Bifidobacterium and Lactobacillus) (39). In addition, the traditional Chinese medicine Tanhuo decoction treatment for IS focuses on (i) promoting the growth of symbiotic flora and beneficial bacteria, (ii) competitively inhibiting the growth of opportunistic pathogenic bacteria, (iii) reducing aseptic inflammation and platelet aggregation, and (iv) enhancing the host’s gut metabolism and body immunity (63). In addition to directly impacting stroke, the accumulation of opportunistic infections is associated with the incidence and severity of post-stroke pneumonia (64). After gut microbiota dysbiosis, opportunistic pathogenic bacteria flourish, particularly Proteobacteria deformation (primarily Enterobacteriaceae), whose excessive proliferation is a sign of epithelial dysfunction and intestinal microbial structural instability, which can also exacerbate inflammation and invasion of exogenous pathogens (65, 66). Environmental or host factors (acute or chronic inflammation) play a selective role in altering homeostasis and causing dysbiosis in gut microbes (66). It is often accompanied by an outbreak of Proteobacteria deformation in the gut (66). The inability of the host to sustain commensal Proteobacteria deformation in low quantities or the weakened resistance of the colonizing microbiome can result in uncontrolled growth of Proteobacteria deformation, which can exacerbate the damage caused by inflammation and invasion by foreign pathogens (66). Consequently, cutting the malignant feedback loop could be a viable intervention method for preventing IS (66).
Firmicutes and Bacteroidetes are two of the main gut microbial species. Both are obligate anaerobic bacteria that can adapt to the anaerobic environment of the intestines and ferment compounds, such as butyrate, which impact the human body (40, 41, 67, 68). The Firmicutes/Bacteroidetes ratio was originally discovered in obese people, demonstrating an increase in Firmicutes and a decrease in Bacteroidetes (69, 70). Furthermore, there was a substantial correlation between the ratio of Firmicutes to Bacteroidetes and the BMI of adults (69, 70). Changes in the ratio of Firmicutes to Bacteroidetes have also been reported in IS (40). The Firmicutes/Bacteroidetes ratio increased significantly in elderly mice with IS with intestinal ecological imbalance. Force-feeding young mice with an increased Firmicutes/Bacteroidetes ratio increased their mortality after IS. In addition, reducing the intestinal Firmicutes/Bacteroidetes ratio of elderly mice increased their survival rate and facilitated functional recovery (40). An increased Firmicutes/Bacteroidetes ratio was also observed in the intestinal flora of mice with IS, including reproductively aged animals with IS (41, 67, 68). Figures 2A–E) depicts the microbiological alterations in IS. Firmicutes exhibited a generally increasing trend, and the ratio of Firmicutes to Bacteroidetes increased (40, 41, 67, 68). A reduced Firmicutes/Bacteroidetes ratio is connected with a slim phenotype, youth, cardiovascular health, and a well-balanced immune system, all of which are advantageous for the human body (71–73). The increase in Firmicutes/Bacteroidetes flora decreases the population of SCFA-producing bacteria and increases the population of lactic acid-producing bacteria (74–76). SCFAs are the energy source for approximately 60–70% of the colon epithelium, which is strongly associated with intestinal immune function and intestinal barrier function, which may be a key reason why the imbalance of Firmicutes/Bacteroidetes flora impacts IS (74–76).
Akkermansia colonizes the intestinal mucosa and regulates basal metabolism by generating mucin-degrading enzymes and utilizing mucin as a source of nitrogen and carbon in the epithelial mucous layer (42). Akkermansia and Lactobacillus growth is frequently observed in intestinal microbiota abnormalities following an IS (40–44). Both are beneficial bacteria that can increase the growth of other beneficial bacteria and create beneficial neuroactive chemicals, which may be related to a compensatory response secondary to the massive reduction of SCFA-producing bacteria, especially butyrate-producing bacteria (41, 77). Akkermansia may use mucin to produce high quantities of acetate, which the Ruminococcus family that produces butyrate can stimulate butyrate production (38, 42, 43). Butyrate can improve wound healing, reinforce intestinal epithelial cell integrity in mice with IS, and inhibit bacterial migration (38, 42, 43). In addition, butyrate can stimulate mucus production and Reg3γ expression in the colon, leading to microbiome reorganization (43, 78). The blood inflammatory markers IL-2, interferon-gamma (IFN-γ), IL-12P40, and monocyte chemoattractant protein-1 (MCP-1) can be reduced by artificially increasing the abundance of Akkermansia bacteria in the colon (79). In addition, it can improve intestinal tight junction proteins (claudin 3, block occluder, and cannabinoid receptor 1), reverse monooxygenase 3 containing linolenin in the liver, and promote the conversion of trimethylamine (TMA) to TMAO (79). These effects contribute to the stable state of the basal metabolism of the host and stimulate the generation of additional beneficial bacteria via diverse signaling pathways (79).
IS is associated with bacteria with poor SCFA production (41). In contrast, another study found that SCFA-producing bacteria (Odoribacter, Akkermansia, Ruminococcaceae UCG-005, and Victivallis) were abundant in late IS. ChristensenellaceAE R-7 group, norank f Ruminococcaceae, and Enterobacter levels are associated with stroke severity (43). The amount of ChristensenellaceAE R-7 is positively associated with the outcome of patients with IS (43). Changes in the bacterial abundance of SCFAs may be associated with the severity and duration of stroke lesions. Furthermore, the relative abundance of SCFA-producing bacteria decreases in patients with stroke-induced cognitive impairment and depression (40, 80). SCFA supplementation can improve post-stroke recovery, cortical reconfiguration, and synaptic plasticity (81). Butyrate is an essential SCFA; its bacterial abundance corresponds to stroke severity (33, 43, 45). Roseburia was considerably enriched in butyrate in mild stroke patients (NIHSS score <4); conversely, Erysipelotrichaceae incertae sedis was abundant in non-mild stroke patients (43, 45). In addition, isobutyric acid, butyric acid, and 2-methyl butyric acid were negatively associated with the percentage change in NIHSS scores. Furthermore, isobutyric acid and 2-methyl butyric acid were favorably linked with NIHSS discharge (33). The abundance of butyrate-producing bacteria was also associated with post-stroke infection (29). Butyrate-producing bacteria were also associated with fasting blood glucose, and severe stroke was positively associated with stress hyperglycemia (fasting blood glucose/glycosylated hemoglobin ratio) (45). It has also been demonstrated that artificially increasing butyrate (sodium butyrate) reduces BBB damage and infarct size in diabetic mice with IS (40, 46).
Studies have reported that Escherichia/Shigella, Peptoniphilus, Ezakiella, and Enterococcus are more abundant in the bacterial flora after IS and ICH (29). ICH is primarily characterized by increased Actinomycetes, Bacteroidetes, and Firmicutes and minor alterations in other bacteria (25, 40, 48). Moreover, gut microorganisms change over time. After ICH, Proteobacteria deformation (Escherichia coli) grew significantly on the first day, whereas Firmicutes (Romboutsia and Peptostreptococcaceae) increased significantly on the third day and then continued to be dominated by an increase in Firmicutes (25). Intracerebral aneurysms are the primary cause of CeVD, including subarachnoid and intracranial hemorrhage (ICH). The decrease in Hungatella hathewayi (35) and the increase in Campylobacter (82) are the predominant microorganism changes in the intestines that influence the onset, progression, and prognosis of IA. These bacteria have not been found in other CeVD for the time being (35, 82). An increase in Bacteroidetes may play a role in the incidence of dementia after CSVD (83). In CCM, Actinomycetes (Bifidobacterium) and Firmicutes (Faecalibacterium) declined, whereas Bacteroides (Odoribacter and Bacteroidales S24-7) increased in the gut microbiome (49, 84).
The gastrointestinal tract is an important immune organ with the largest immune cell repertoire. This collection includes almost 70% of the immune system of the body. As mentioned before, CeVD is characterized by alterations in the different flora of intestinal microorganisms. Inflammatory responses and immune modulations follow various degrees of damage to the gut and brain. They lead to alterations in the intestinal barrier’s function and the impact of microbial metabolites on the brain through this barrier, which may be a way to combat CeVD in the future. Therefore, understanding the mechanisms underlying the lesions is the most effective way to identify targets for intervention (Figure 4).
Figure 4 Pathways of cerebrovascular injury due to intestinal microbial imbalance. TMAO, trimethylamine-N-oxide; SCFAs, short-chain fatty acids; LPS, lipopolysaccharide; TLR4, toll-like receptor 4; CCL, chemokines. By Figdraw and BioRender.com.
During IS, the gut microbiota varies rapidly (minutes to hours). Multiple signals of intestinal homeostasis disorder, vagal nerve activation, and cerebral inflammatory signals stimulate intestinal myeloid cells to activate receptor 1. This worsens damage to the intestinal mucosal layer, modifies intestinal permeability, and permits intestinal cells to enter the bloodstream from the intestines (85, 86). When LPS and bacteria are translocated to the peripheral area, normal immune homeostatic processes in the gut can be damaged by the extravasation of immune cells into the brain tissue, which accelerates brain damage after stroke (87). After IS, bone marrow cells from peripheral triggering receptor expressed on myeloid cells-1(TREM1) induce a fast innate immune response that inhibits brain recovery processes, including glutathione metabolism, anti-inflammatory TREM2 signaling, and lysosomal degradation (86). LPS produced by Gram-negative bacteria translocate from the intestine to the systemic circulation after intestinal imbalances and increased intestinal permeability. Moreover, after IS, the BBB is damaged, allowing LPS to enter the brain and bind to inflammatory cytokines and TLR4 receptors on microglia (84, 87–90). Consequently, microglia and macrophages are hyperactivated, resulting in (i) exacerbated neuroinflammation and large amounts of inflammatory factors such as TNF-α, IL-1β, IL-6, TNF, and iNOS release, (ii) increased production of chemokines in the brain parenchyma, and (iii) subsequent infiltration of cytotoxic immune cells, including neutrophils, inducing neuronal apoptosis and brain injury (84, 87–90). The gut microbes can also be affected. There is a negative correlation between Bifidobacterium and IL-1, IL-2, IL-6, and hs-CRP levels (47). The elevated expression of the inflammatory cytokines IL-2 and IL-6 in the intestine and brain tissues of patients with post-stroke depression decreased following exercise intervention, which may be related to the enhanced expression of Lactobacillus and Bifidobacteria (91). Besides, the ratio of Firmicutes to Bacteroidetes was higher in older mice than in younger mice, and systemic proinflammatory cytokine levels were elevated (40). When Bifidobacterium longum, commensal Clostridia, Eubacterium faecalis, and Lactobacillus fermentum were transplanted into old mice, neuroinflammation and neurological impairments following stroke were mitigated to varying degrees (37). Except the direct effect, metabolites of dysbiosis, also promote the release of inflammatory factors, and the indirect effect of IS cannot be ignored. SCFAs have been associated with elevated levels of systemic proinflammatory markers IL-6, TNF-α, vascular cell adhesion molecule-1(VCAM-1), IL-17, and MCP-1 after stroke, which was associated with a high disease burden at discharge and the duration of inpatient recovery (33). Moreover, butyrate-producing bacterial abundance is an independent predictor of post-stroke infection (29).
IA rupture is associated with gut microbiota affecting the influx of macrophages within the aneurysm, matrix metalloproteinase (MMP) production, and the imbalance between pro-inflammatory and anti-inflammatory cells M1 and M2 (92, 93). After antibiotic inhibition of intestinal microorganisms, inflammation in cerebral arteries during aneurysm formation is suppressed, the number of macrophages is reduced, mRNA expression of inflammatory factors IL-1β, IL-6 and iNOS is decreased, and MCP-1 is reduced (93). In addition, the expression of the pro-inflammatory cytokines IL-6 and TNF-α was increased in animals transplanted with feces from IA patients (35). The increased gene expression of intestinal proinflammatory proteins IL-1, (toll-like receptor) TLR-2, and IL-17 in CSVD-affected animals facilitated the buildup of bacterial toxins and bacteria, triggered the progressive progression of gut inflammation into systemic inflammation, and consequently exacerbated BBB damage (30). However, in CCM, the cell wall component of gram-negative bacterial lipopolysaccharides, in conjunction with the intracellular adaptor protein complex defect resulting from the loss of function mutations of Krev interaction trapped protein 1, CCM2, and programmed cell death 10 genes, activates TLR4 in brain endothelial cells through TLR4-Mekk3-KLF1/4 signal transduction (84, 88). CCM production is accelerated by activating innate immunity and co-mediating inflammation reactions with lipopolysaccharide (LPS) (84, 88). ICH significantly increases the intestinal inflammatory response by increasing the release of the inflammatory cytokines IL-1β, IL-6, and TNF-α, activating intracellular adhesion molecule-1 (ICAM-1) and other chemokines, including MCP-1 and CCL-5, MPO activity, and IL-1β concentration in the peripheral circulation, initiating the infiltration of inflamed cells into the intestine, leading to delayed small bowel motility and bowel obstruction (94–97). One study reports that intestinal inflammatory factors and T lymphocytes gradually infiltrate the ICH hematoma, and the number of CD4 T cells, CD8 T cells, and regulatory T lymphocytes (Tregs) increases (98, 99). Additionally, IFN-γ and IL-17 proinflammatory cytokine expression increases (99). Fourteen days after ICH, the mRNA levels of inducible nitric oxide synthase, tumor necrosis factor, and other proinflammatory indicators were significantly elevated (99).
After separating the external intra-abdominal environment from the inside milieu of the gastrointestinal tract, the gastrointestinal barrier measures approximately 400 m2. This barrier serves a dual purpose: it permits the absorption of nutrients, water, and electrolytes and limits the host’s exposure to harmful lumen antigens (100). The mucosal, epithelial, and immunological layers constitute the intestinal barrier. CeVD in the three-layer barrier of the gut appeared to be damaged to varying degrees. It is also possible to track barrier disturbances during the early stages of CeVD in an is also possible to track barrier disturbances. The intestinal mucosa in the MCAO group showed cell edema, thickness, and shortening of the villi 3 hours postoperatively, which increased over time. In the early stages of IS, necrosis develops at the top of the villi and damages the intestinal mucosa within 24 hours (44, 50, 101). This results in altered gastrointestinal dynamics owing to villous shrinkage, crypt distortion, mucosal edema, and significant inflammatory cell infiltration.
Similarly, gastrointestinal motility is reduced to varying degrees (44, 50, 101). At the histomorphological level, substantial intestinal epithelial cell destruction, thickening and shortening of villi, the fusion of adjacent villi, and exposure of the lamina propria were noted following ICH (102). At the ultrastructural level, intestinal secretions increase, and epithelial cells are unevenly arranged, sparse, and fragmented at the surface of the villi (102). In addition, the intestinal mucus barrier was compromised in mice with CCM, and E-cadherin (CDH1) was reduced. However, the continuity of the epithelial cell adhesion molecule connection increased, and neither f-actin nor zonula occludens-1 (ZO-1) changed (103).
The morphological changes were external manifestations, while the internal disruption of barrier function was associated with (i) the lipid polysaccharide of gram-negative bacteria, (ii) metabolites of dominant flora such as SCFAs and TMAO, and (iii) intestinal inflammatory factors including TNF-α, IL-1, IL-6, TNF, and iNOS (32, 50, 98, 99, 104). The influx of LPS from gut extravasation into the bloodstream and systemic circulation may be linked to the disruption of the gut mucosal barrier. In addition, studies have shown that the interaction between LPS and TLR induces an inflammatory response in the host, exacerbating damage to gut barrier function (84, 87–90). One study reported that the plasma levels of LPS, D-lactate, zonulin, TNF-α, IFN-γ, and IL-6 progressively increased over time in macaques with IS compared to healthy macaques (50). ICH in mice causes significant intestinal mucosal injury, barrier dysfunction, delay of slight intestinal movement, an inflammatory reaction characterized by white blood cells infiltrating intestinal tissue, and elevated levels of inflammatory cytokines in intestinal tissue and serum.
Furthermore, oxidative stress is distinguished by indirect reactive oxygen species and antioxidant gene levels (32). The cytotoxic effect of inflammatory substances causes intestinal microvilli injury, which results in the breakdown of tight junction structures between cells, a decrease in the tight junction proteins occludin and claudin-1, and an increase in the gut barrier dysfunction (32, 98, 99, 104). After CeVD, dysbiosis of the gut microbiota, intestinal mucosal injury, and chronic systemic inflammation persist and may impair immunological homeostasis.
SCFAs are essential in maintaining gut bacterial balance, gut epithelial functional integrity, gut immunology, and inflammation. They induce the development of the intestinal barrier by suppressing the NOD-like receptor protein 3 (NLRP3) inflammasome and autophagy and protect the intestinal barrier against LPS damage. SCFAs also function as an energy source to protect the intestinal barrier and suppress autophagy and as a histone deacetylase (HDAC) inhibitor to inhibit the NLRP3 inflammasome (105). The interaction between the NLRP3 inflammasome and autophagy affects the intestinal barrier function; however, SCFAs can restore barrier function (105). A reduction in SCFAs following IS has been confirmed. Butyrate is a frequently investigated SCFA metabolite whose supplementation can reduce the poor prognosis associated with IS and improve intestinal barrier dysfunction. In addition to lowering inflammation and damage in the gut, butyrate also affects tight junction proteins (28). ZO-1, occludin, and claudin-4 are upregulated to preserve the integrity of the gut mucosa (46). In addition, butyrate supplementation influences epithelial oxygen consumption and preserves the stability of the hypoxia-inducible factor, a transcription factor that regulates intestinal barrier protection (106).
Altered intestinal permeability, impairment of intestinal barrier function, and the passage of intestinal flora metabolites through the intestinal barrier affect CeVD. The relationship between intestinal bacteria and CeVD may be directly related to the actions of intestinal metabolites, such as TMAO, SCFAs, taurine, and other medications. In patients with IS, plasma TMAO levels were dose-dependently linked to worse functional outcomes (107, 108). TMAO upregulates the expression of scavenger receptors (CD36 and SR-A1) in macrophages. This results in increased cholesterol buildup in macrophages and the production of foam cells in atherosclerotic lesions (109), hence increasing the risk of cerebral thrombosis. In contrast, TMAO can induce excessive vascular inflammation and oxidative stress, reducing the bioavailability of eNOS-derived NO and decreasing endothelial function in elderly rats (108, 110). In contrast, endothelial dysfunction is induced by excess generation of reactive oxygen species, and inflammation is the major mechanism of cerebrovascular injury (108, 110). Moreover, the cascade of mitogen-activated protein kinase activation, extracellular signal-associated kinase, and nuclear factor-B signaling in endothelial cells can induce vascular inflammation (111). Increased proinflammatory intermediate CD14 and CD16 also promote increased serum TMAO concentrations, which enhances the risk of recurrent stroke after IS and cardiovascular events (112). This is done to prevent aseptic inflammation and platelet aggregation in the body and lower the risk of recurrent stroke following stroke (63). Chronic low-dose TMAO influences inflammatory variables, such as TNF and IL-1. However, it reverses LPS-induced BBB damage and memory impairment, prevents the degradation of cell structural arrangement, lowers oxidative stress, and preserves the integrity of cerebrovascular endothelial cells (113). Nonetheless, in ICH, a high blood concentration of TMAO may exacerbate cellular inflammation by activating microglia and astrocytes (114). Furthermore, it does not affect the expression of inflammatory factors around the hematoma in the acute phase of ICH. In addition, it does not affect lesion volume in the acute phase of ICH, brain swelling and brain water content, and later brain atrophy, white matter damage, and nerve function (114). Therefore, TMAO is not a therapeutic target for ICH (114). One of the most significant metabolites of intestinal microbes, SCFAs can affect oxidative stress, intestinal barrier dysfunction, and inflammatory response, and their production of bacterial decrease is associated with IS and ICH (41, 74, 80). The quantity of SCFA-producing bacteria in the gut is susceptible to aging and is related to the severity of IS and poor prognosis conditions such post-stroke depression and cognitive impairment (40, 42, 80, 83). SCFA supplementation can promote post-stroke recovery and cortical reorganization, modulate post-stroke synaptic plasticity, and decrease microglial activation and immune cell production (81). Transplantation of SCFA-producing bacteria can (i) reduce IL-17 T cells in the brain, (ii) alleviate neurological deficits and inflammation following stroke, and (iii) increase the concentration of SCFA in the gut and plasma of elderly stroke mice (37). Moreover, it can increase the expression of the mucin genes Muc2 and Muc4 in the intestinal epithelium. It can also increase the protective mucous layer, modify the T lymphocyte population, modulate antibody secretion, and might penetrate the systemic circulation to regulate cytokine release (37, 115). The transplantation of butyric acid-producing bacteria-rich bacterial flora can minimize nerve damage and cerebral infarction volume following IS (51). Additionally, it can alleviate cerebral edema, lower blood cholesterol levels, inhibit histone deacetylase HDAC, activate PPAR, reduce inflammation, and alter the gut flora (51). This results in an increase in helpful lactic acid bacteria and intestinal barrier repair (51). In conclusion, SCFAs influence CeVD primarily by modulating inflammatory and immunological pathways and intestinal barrier function but not by directly affecting brain tissues. Taurine, a gut microbial metabolite, is closely associated with lesions in IA and IS (35, 116, 117). A rtificial taurine supplementation can reverse the formation and rupture of IA, which is correlated with a decrease in host inflammatory markers, thus weakening the inflammatory process. Reducing the activation of MMPs, particularly MMP-9, inhibits extracellular matrix remodeling, increases collagen IV and laminin in the structure of the cerebral artery, and preserves cerebral artery integrity (35). Moreover, taurine canreverse the activation of PARP and NF-B in the ischemic core penumbra, drastically reducing TNF-α, IL-1β, inducible nitric oxide synthase, and ICAM-1, and decreasing myeloperoxidase activity. Reduced neutrophil infiltration within the penumbra and center (116, 117). Exogenous injection of adequate taurine may restrict intracellular taurine release and increase extracellular taurine levels, thereby promoting intracellular homeostasis, clearing HOCl, reducing Tau-NCl2 synthesis, elevating tau-NHCl levels, and inhibiting inflammation. This eventually leads to a decrease in ischemic brain injury (117). Therefore, taurine reduces the inflammatory response and may protect against secondary injury after CeVD, thus decreasing IA occurrence. Intestinal bacteria metabolize bodily components to produce intestinal metabolites, such as tryptamine, indole, indole propionic acid, indoleacetic acid, indole aldehyde, bile acid, and phenylacetylglutamine. These metabolites can regulate intestinal permeability, gastrointestinal motility, and microbial composition (118–124).
In addition to the processes mentioned above, another putative mechanism for cerebrovascular injury is the reciprocal connection between gut microorganisms and the brain. The intestine can influence the brain in various ways, including the neuroendocrine pathway, anatomical pathways, neurotransmitters, and many other mechanisms (125, 126). Anatomically, the vagus nerve in the spinal cord and autonomic nervous system allows the intestine to communicate directly with the brain. The enteric neural system in the stomach and autonomic nervous system, and the vagus nerve in the spinal cord can both contribute to bidirectional communication between the gut and brain (125, 127–129). Accordingly, gut microorganisms can directly stimulate afferent neurons of the enteric nervous system and vagal signals of the gut to trigger inflammatory reactions and affect the brain (130). The neuroendocrine-hypothalamic-pituitary-adrenal axis is a pathway by which gut microorganisms influence the brain (125, 129). Gut microbes also play crucial neuroendocrine roles. Changes in the dominant microbiota affect the expression and release of the hypothalamic adrenocorticotropin-releasing hormone, which affects adrenal gland function (125, 129). Bifidobacteria in feces can influence the stress response in GF mice (131).
Additionally, being under a lot of stress causes the amount of Bacillus mimicus to drop and the amount of Clostridium perfringens to increase (132). The brain is also affected by neurotransmitters, neuromodulators, and other chemicals produced by gut microbes (125, 133, 134). For instance, chemicals produced by gut microorganisms and intestinal bacteria, such as gamma amino acids, butyric acid, 5-hydroxytryptamine (5-HT), dopamine, and SCFAs, can ultimately affect the brain through numerous exchanges and interactions (135). For example, the fish gut bacterium Edwardsiella tarda consumes tryptophan from food and breaks it down to produce indole, which activates Trpa1 channels in enteroendocrine cells and causes the production and secretion of 5-HT (136, 137). Likewise, serotonin stimulates intestinal neurons, improves intestinal dynamics, and stimulates vagal activity, all of which affect brain activity. Additionally, gut microbes control how tryptophan precursors produced by 5-HT are synthesized, influencing how neurons that release pentraxins in the brain communicate and, ultimately, how neurological and psychiatric illnesses develop (138, 139). To ascertain whether these possible pathways are pathophysiological mechanisms of cerebrovascular injury caused by gut bacteria, further in-depth research into each pathway may be worthwhile.
Diet is one of the direct drivers of gut microbial dysbiosis, affecting the course of cardiovascular disease and CeVD, as indicated in Table 1 and Figure 5. In addition, numerous scientific studies have indicated the importance of diet and nutrition in reducing cardiovascular diseases and CeVD (140, 146). Diets rich in fat and carnitine, such as meat and eggs, increase the abundance of TMAO-producing gut bacteria, which are believed to promote TMAO-induced platelet activation and increase the risk of thrombotic events (147). Furthermore, dietary choline can boost the activation of microglia and astrocytes in the vicinity of the hematoma in ICH, causing inflammation (114); when TMAO levels in the blood surpass a quarter of the average, the risk of stroke and cardiovascular disease increases by 2.5 times (148, 149). In addition, the dominant flora following an intestinal microbiome disorder metabolizes amino acids and produces harmful metabolites such as para-cresol sulfate, horse uric acid, indolyl sulfate, para-cresol glucosidic acid, phenylacetylglutamine, and phenyl sulfate. These metabolites cause secondary renal damage when excreted by the kidney (18, 150). Therefore, those at risk of stroke should minimize meat and egg yolk consumption (18).
Table 1 Interventions, influence mechanisms and efficacy of gut microbes in cerebrovascular disease.
Figure 5 Therapeutic modalities for modulating the risk of cerebrovascular disease through intervention with gut microbes. By Figdraw and BioRender.com.
In antiepileptic mice, a ketogenic diet was observed to decrease gut bacterial diversity and increase the relative abundance of Akkermansia muciniphila (151). In addition, the short-term gut microbiota of mice with multiple sclerosis is dysregulated, and a long-term ketogenic diet leads to a progressive decrease in Akkermansia bacteria (152). The amount and variety of gut flora were restored after 12–24 weeks of a ketogenic diet (152). By reducing excitotoxicity, oxidative stress, and apoptotic processes, a ketogenic diet can improve post-stroke motor function and increase the neuronal repair of the ischemic penumbra in patients with IS (142, 143). Further research is necessary to determine whether a ketogenic diet can prevent and treat CeVD by modulating gut bacteria.
In addition, one study reported that a diet high in sugar calories significantly impacted CeVD. In the barley diet group, the ratio of Bacteroidetes to Firmicutes in intestinal microorganisms was significantly lower than that in the rice diet group. In contrast, the cholesterol level of medium and small particle sizes of high-density lipoprotein (HDL) was significantly increased, which may decrease the risk of stroke (144). Intestinal microbiota abnormalities also result in the loss of SCFAs, whereas a high-fiber diet can increase butyrate levels in the circulation (145). In mice fed a high-fiber diet, inflammation generated by endotoxins and lipopolysaccharides increased IL-1RA, whereas IL-1 and tumor necrosis factor-alpha (TNF-α) were reduced. By polarizing Th2 in mice and increasing the substitution activation of macrophages, endotoxin- and lipopolysaccharide-induced illness damage is reduced, which helps enhance brain immunity (141, 153). The incidence and risk of stroke are negatively correlated with dietary fiber intake (154).
Flora transplantation restores the original dominant intestinal flora and intestinal homeostasis by transferring functional bacteria from the normal population feces to the gastrointestinal tract of dysbiosis, thus achieving the objective of treating the pathological state in the host (155). Currently, CeVD animal models have been used to investigate the therapeutic effects of this method (29, 35, 37, 46, 63, 68). Intestinal T cells develop protective activity following transplantation of feces from a young population into mice with IS. Treg cells and IL-17 T cells contribute to decreased inflammation, neurological deficits, and impairment of intestinal barrier function following stroke (29, 37, 63). Transplantation of adult rat bacterial flora into reproductively aged rats significantly improved the area of cerebral infarction, lowered the production of IL-17, and exacerbated behavioral impairment after stroke (68). H. hathewayi is positively associated with taurine levels, decreases cerebral artery inflammation, and restores extracellular matrix integrity to avoid IA (35). In mice with ICH, transplantation of bacterial flora can affect T cells in the brain, reduce neuroinflammation following bleeding, and restore the average fluorescence intensity of the tight junction proteins occludin and claudin-1, thereby restoring intestinal barrier function (46). Flora transplantation plays an effective role in the prevention and treatment of CeVD in animal models. However, there are difficulties in implementing simple flora transplantation applied clinically to feces collected from healthy donors or the recipients themselves (self-FMT) and then administered to the intestines of patients with the disease or associated ecological disorders (124).
Therefore, it is of major application value to test medications with comparable efficacy or generate treatment-specific microbiota. Metformin possesses potent anti-inflammatory and immunosuppressive properties. In ICH mice, metformin effectively ameliorates neurological dysfunction and lowers neuroinflammation by suppressing the proinflammatory polarization of microglia/macrophages and has a neuroprotective effect similar to that of microflora transplantation (48). The most widely studied florae are prebiotics and probiotics. Probiotics are non-digestible compounds that regulate the composition and/or activity of the intestinal microbiota through their metabolism by microorganisms in the gut, thereby conferring beneficial physiological effects to the host (156).
In contrast, probiotics are live, giveable microorganisms capable of conferring health benefits to the host (157). After intake, probiotics and prebiotics can be transported straight to the gastrointestinal system, inhibiting the action of pathogenic gut microorganisms and restoring the balance of “beneficial bacteria,” “neutral bacteria,” and “bad bacteria” to prevent and treat disease (158, 159). For example, probiotics administration restored the intestinal microecological balance, intestinal TMAO production decreased, SCFA production increased, tight junction protein damage decreased, and adaptive and innate immune activation decreased. In addition, inflammatory damage following stroke decreased (82, 160, 161). In addition, it has been proven to treat mood disorders following a stroke, including anxiety and depression, 3 months after the event (28).
Chinese medicine is also an important intervention for gut microbes and CeVD. Tanhuo decoction can control gut microorganisms, enhance gut bacteria, compete with harmful bacteria to decrease LPS and TMAO synthesis, and decrease aseptic inflammation and platelet aggregation in blood vessels and the neurological system (63). Anthraquinone glycoside rhubarb is an efficient treatment for cerebral ischemic injury and can regulate the disturbance of gut flora induced by cerebral ischemia-reperfusion injury (82). Consequently, there is an imbalance between the release and degradation of neurotransmitters such as 5-HT and 5-hydroxyindoleacetic acid, which is caused by disturbances in the intestinal flora (82). The classic TCM formula Tongqiao Huoxue Decoction (TQHXD) can (i) control the changes in flora after IS, (ii) reduce the excessive increase in Bacteroides, (iii) control the abnormal changes in the abundance of some flora, (iv) improve the inflammatory response caused by T cell imbalance, and (v) restore the function of the gut barrier (18). In addition, decoctions, including Pueraria root and rhizome, ameliorated dyslipidemia, elevated blood viscosity, and a high risk of thrombosis after stroke and repaired the ecological imbalance of the gut microbiome and the integrity of the brain-gut barrier (162). NaoMaiTong corrected the dismal prognosis following stroke by restoring gut flora (44). Formulations of Xingu Chengqi stimulate the release of anti-inflammatory factors, such as IL-10, and downregulate proinflammatory factors, such as TNF-α, IL-17A, and IL-22 (163). Increasing SCFA levels also enhance neurological function, alleviates cerebral infarction, and reduce neuronal death (163). Resveratrol mitigates ischemic brain injury by modulating T lymphocytes to minimize BBB injury and neuroinflammation caused by proinflammatory factors (31). Shengli Sansheng Pulvis improves the endothelial connection complex by increasing vasoactive intestinal peptides and receptors and decreasing claudin-5, ZO-1, occludin, VE-calcitonin, and MMP 2/9 expression. Moreover, it increases trans-endothelial resistance in microvascular endothelial cells of the brain following ischemia (164). In conclusion, modulation of intestinal bacteria by TCM has a substantial influence on the treatment of CeVD and has a substantial therapeutic effect.
Antibiotic intervention can alter the makeup and function of the intestinal microbiota and microbiome homeostasis. The use of antibiotics to consume gut flora, which produces inflammatory factors such as IL-1, IL-6, and iNOS, decreases IA formation (93). In IS, gut microbial changes generated by antibiotic intervention can induce dendritic cell modifications that affect gut immune homeostasis, resulting in increased regulatory T cells, decreased IL-17 T cells, and reduced ischemic brain injury in mice (87). In contrast, depletion of gut microbes by broad-spectrum antibiotics does not affect the area of brain damage 1 day after stroke but suppresses systemic immunity and reduces survival from IS and severe secondary colitis (34). In addition, broad-spectrum antibiotic treatment for 3 weeks reduces eNOS activity, leading to (i) brain endothelial cell dysfunction, (ii) a significant increase in spontaneous tone in the middle cerebral arteries (MCAs), (iii) significant blunting of L-NAME-induced vasoconstriction, (iv) reduction in intraluminal ATP-mediated dilation, and (v) significantly increased risk of stroke and IA (165). Therefore, antibiotic administration to prevent and cure strokes caused by gut bacteria remains controversial. However, applying antibiotics with a limited spectrum that target specific bacteria may result in more precise CeVD prevention and treatment.
Exercise can increase learning, enhance memory, and alleviate despair, anxiety, and other negative feelings. In addition, exercise training can block the proinflammatory factors IL-2 and IL-6, accelerate the transformation of microglia into M2, regulate gut flora, and lower the incidence of depression following stroke (91). It can also increase the decline in BAC303, EREC482, and LAB158, which are inflammatory microbiota. This alleviates behavioral impairment after stroke, reduces infarct size, and improves infarct cell survival rate (166). In addition, ghrelin reduces ICH-induced intestinal barrier dysfunction by reducing intestinal mucosal damage, lowering intestinal permeability, enhancing tight-junction molecules, inhibiting ICAM-1 expression, and suppressing endotoxin translocation (102).
There are still many unanswered questions regarding the gut microbiota and pathogenesis of CeVD. First, the ultimate goal of scientific research is to cure clinical disorders. However, the prevention and therapy strategies for intestinal bacteria in CeVD are not specific, and targeted flora transplantation has not been effectively utilized in medical practice. In the future, evaluation of the efficiency of interventions against specific pathogenic bacteria may be of greater clinical relevance. Second, numerous studies have demonstrated an association between gut microbial diseases and CeVD. However, further research is needed to confirm the role of gut microbes in the occurrence of CeVD. Prevention and therapy can be enhanced if this causal relationship can be confirmed early. In addition, the primary mechanisms of action of intestinal microorganisms in CeVD include immunological problems, inflammatory reactions, gut barrier dysfunction, and the impact of gut metabolites. However, the specific molecules, locations, and mechanisms of gut bacteria acting on CeVD after imbalance have not been explored. If these constraints can be overcome, the mechanism and potential treatment of intestinal bacteria that cause CeVD can be developed, and the therapeutic significance of this research can be significantly enhanced.
Gut bacteria provide novel ideas for the effective prevention and treatment of CeVD, thus reversing the conventional understanding of vascular diseases and neuroinflammation. In the link between gut bacteria and CeVD, metabolites of dysbiosis of the gut flora, such as TMAO, SCFAs, and taurine, contribute significantly to cerebrovascular injury and increase disease progression. The food consumed may affect these metabolites; hence, dietary changes may be related to an increased risk of CeVD. In managing gut microorganisms and CeVD, dietary treatments, FMT, herbal medication, and antibiotics have specific protective effects on the regulation of gut microbes and the prevention of CeVD. However, research on the association between gut microorganisms and ICH, IA, CSVD, and CCM is still in its infancy. Future studies examining gut-brain axis-based CeVD risk reduction and therapeutic targets are of great clinical significance.
The original contributions presented in the study are included in the article/Supplementary Material. Further inquiries can be directed to the corresponding authors.
LZ and XZ designed the research and determined the structure of the manuscript. XZ, LX, LW selected the references and contributed to the writing. LZ and SW contributed to the revision and finalization of the review. All authors contributed to the article and approved the submitted version.
This study was supported by the National Science & Technology Fundamental Resources Investigation Program of China to LZ (No.2018FY100900), The Hunan Provincial Natural Science Foundation of China Grant to YZ (No.2021JJ30923), The Provincial Science and Technology Innovation Leading Talents Project to LZ (No.2021RC4014), National Clinical Research Center for Geriatric Disorders (XiangYa Hospital).
We thank all research on gut microbes and cerebrovascular diseases included in this study.
The authors declare that the research was conducted in the absence of any commercial or financial relationships that could be construed as a potential conflict of interest.
All claims expressed in this article are solely those of the authors and do not necessarily represent those of their affiliated organizations, or those of the publisher, the editors and the reviewers. Any product that may be evaluated in this article, or claim that may be made by its manufacturer, is not guaranteed or endorsed by the publisher.
The Supplementary Material for this article can be found online at: https://www.frontiersin.org/articles/10.3389/fimmu.2022.975921/full#supplementary-material
1. GBD 2016 Causes of Death Collaborators. Global, regional, and national age-sex specific mortality for 264 causes of death, 1980-2016: a systematic analysis for the global burden of disease study 2016Lancet (2017) 390(10100):1151–210 doi: 10.1016/S0140-6736
2. Feigin VL, Norrving B, Mensah GA. Global burden of stroke. Circ Res (2017) 120(3):439–48. doi: 10.1161/CIRCRESAHA.116.308413
3. An SJ, Kim TJ, Yoon BW. Epidemiology, risk factors, and clinical features of intracerebral hemorrhage: An update. J Stroke. (2017) 19(1):3–10. doi: 10.5853/jos.2016.00864
4. Tawk RG, Hasan TF, D’Souza CE, Peel JB, Freeman WD. Diagnosis and treatment of unruptured intracranial aneurysms and aneurysmal subarachnoid hemorrhage. Mayo Clin Proc (2021) 96(7):1970–2000. doi: 10.1016/j.mayocp.2021.01.005
5. Snellings DA, Hong CC, Ren AA, Lopez-Ramirez MA, Girard R, Srinath A, et al. Cerebral cavernous malformation: From mechanism to therapy. Circ Res (2021) 129(1):195–215. doi: 10.1161/CIRCRESAHA.121.318174
6. Chojdak-Łukasiewicz J, Dziadkowiak E, Zimny A, Paradowski B. Cerebral small vessel disease: A review. Adv Clin Exp Med (2021) 30(3):349–56. doi: 10.17219/acem/131216
7. Hossmann KA. Pathophysiology and therapy of experimental stroke. Cell Mol Neurobiol (2006) 26(7-8):1057–83. doi: 10.1007/s10571-006-9008-1
8. Radenovic L, Nenadic M, Ułamek-Kozioł M, Januszewski S, Czuczwar SJ, Andjus PR, et al. Heterogeneity in brain distribution of activated microglia and astrocytes in a rat ischemic model of alzheimer’s disease after 2 years of survival. Aging (2020) 12(12):12251–67. doi: 10.18632/aging.103411
9. Pluta R. The role of apolipoprotein e in the deposition of beta-amyloid peptide during ischemia-reperfusion brain injury. a model of early alzheimer’s disease. Ann N Y Acad Sci (2000) 903:324–34. doi: 10.1111/j.1749-6632
10. Sekeljic V, Bataveljic D, Stamenkovic S, Ułamek M, Jabłoński M, Radenovic L, et al. Cellular markers of neuroinflammation and neurogenesis after ischemic brain injury in the long-term survival rat model. Brain Struct Funct (2012) 217(2):411–20. doi: 10.1007/s00429-011-0336-7
11. Qureshi AI, Ali Z, Suri MF, Shuaib A, Baker G, Todd K, et al. Extracellular glutamate and other amino acids in experimental intracerebral hemorrhage: an in vivo microdialysis study. Crit Care Med (2003) 31(5):1482–9. doi: 10.1097/01.CCM.0000063047.63862.99
12. Lusardi TA, Wolf JA, Putt ME, Smith DH, Meaney DF. Effect of acute calcium influx after mechanical stretch injury in vitro on the viability of hippocampal neurons. J Neurotrauma (2004) 21(1):61–72. doi: 10.1089/089771504772695959
13. Nakamura T, Xi G, Park JW, Hua Y, Hoff JT, Keep RF. Holo-transferrin and thrombin can interact to cause brain damage. Stroke. (2005) 36(2):348–52. doi: 10.1161/01.STR.0000153044.60858.1b
14. Zhu H, Wang Z, Yu J, Yang X, He F, Liu Z, et al. Role and mechanisms of cytokines in the secondary brain injury after intracerebral hemorrhage. Prog Neurobiol (2019) 178:101610. doi: 10.1016/j.pneurobio.2019.03.003
15. Vespa PM, O’Phelan K, Shah M, Mirabelli J, Starkman S, Kidwell C, et al. Acute seizures after intracerebral hemorrhage: a factor in progressive midline shift and outcome. Neurology. (2003) 60(9):1441–6. doi: 10.1212/01.wnl.0000063316
16. Yoshimura M, Yamanouchi H, Kuzuhara S, Mori H, Sugiura S, Mizutani T, et al. Dementia in cerebral amyloid angiopathy: a clinicopathological study. J Neurol (1992) 239(8):441–50. doi: 10.1007/BF00856809
17. English C, MacDonald-Wicks L, Patterson A, Attia J, Hankey GJ. The role of diet in secondary stroke prevention. Lancet Neurol (2021) 20(2):150–60. doi: 10.1016/S1474-4422(20)30433-6
18. Spence JD. Diet for stroke prevention. Stroke Vasc Neurol (2018) 3(2):44–50. doi: 10.1136/svn-2017-000130
19. Weiwei C, Runlin G, Lisheng L, Manlu Z, Wen W, Yongjun W, et al. Outline of the report on cardiovascular diseases in China, 2014. Eur Heart J Suppl (2016) 18(Suppl F):F2–F11. doi: 10.1093/eurheartj/suw030
20. Nam HS. Gut microbiota and ischemic stroke: The role of trimethylamine n-oxide. J Stroke. (2019) 21(2):151–9. doi: 10.5853/jos.2019.00472
21. Sampson TR, Mazmanian SK. Control of brain development, function, and behavior by the microbiome. Cell Host Microbe (2015) 17(5):565–76. doi: 10.1016/j.chom.2015.04.011
22. Rodríguez JM, Murphy K, Stanton C, Ross RP, Kober OI, Juge N, et al. The composition of the gut microbiota throughout life, with an emphasis on early life. Microb Ecol Health Dis (2015) 26:26050. doi: 10.3402/mehd
23. Yang T, Richards EM, Pepine CJ, Raizada MK. The gut microbiota and the brain-gut-kidney axis in hypertension and chronic kidney disease. Nat Rev Nephrol (2018) 14(7):442–56. doi: 10.1038/s41581-018-0018-2
24. Singh V, Roth S, Llovera G, Sadler R, Garzetti D, Stecher B, et al. Microbiota dysbiosis controls the neuroinflammatory response after stroke. J Neurosci (2016) 36(28):7428–40. doi: 10.1523/JNEUROSCI.1114-16.2016
25. Zhang H, Huang Y, Li X, Han X, Hu J, Wang B, et al. Dynamic process of secondary pulmonary infection in mice with intracerebral hemorrhage. Front Immunol (2021) 12:767155. doi: 10.3389/fimmu.2021.767155
26. Huang Q, Xia J. Influence of the gut microbiome on inflammatory and immune response after stroke. Neurol Sci (2021) 42(12):4937–51. doi: 10.1007/s10072-021-05603-6
27. Yin J, Liao SX, He Y, Wang S, Xia GH, Liu FT, et al. Dysbiosis of gut microbiota with reduced trimethylamine-N-Oxide level in patients with Large-artery atherosclerotic stroke or transient ischemic attack. J Am Heart Assoc (2015) 4(11):e002699. doi: 10.1161/JAHA.115.002699
28. Liu Y, Kong C, Gong L, Zhang X, Zhu Y, Wang H, et al. The association of post-stroke cognitive impairment and gut microbiota and its corresponding metabolites. J Alzheimers Dis (2020) 73(4):1455–66. doi: 10.3233/JAD-191066
29. Haak BW, Westendorp WF, van Engelen TSR, Brands X, Brouwer MC, Vermeij JD, et al. Disruptions of anaerobic gut bacteria are associated with stroke and post-stroke infection: a prospective case-control study. Transl Stroke Res (2021) 12(4):581–92. doi: 10.1007/s12975-020-00863-4
30. Nelson JW, Phillips SC, Ganesh BP, Petrosino JF, Durgan DJ, Bryan RM. The gut microbiome contributes to blood-brain barrier disruption in spontaneously hypertensive stroke prone rats. FASEB J (2021) 35(2):e21201. doi: 10.1096/fj.202001117R
31. Dou Z, Rong X, Zhao E, Zhang L, Lv Y. Neuroprotection of resveratrol against focal cerebral Ischemia/Reperfusion injury in mice through a mechanism targeting gut-brain axis. Cell Mol Neurobiol (2019) 39(6):883–98. doi: 10.1007/s10571-019-00687-3
32. Cheng Y, Zan J, Song Y, Yang G, Shang H, Zhao W. Evaluation of intestinal injury, inflammatory response and oxidative stress following intracerebral hemorrhage in mice. Int J Mol Med (2018) 42(4):2120–8. doi: 10.3892/ijmm.2018.3755
33. Henry N, Frank J, McLouth C, Trout AL, Morris A, Chen J, et al. Short chain fatty acids taken at time of thrombectomy in acute ischemic stroke patients are independent of stroke severity but associated with inflammatory markers and worse symptoms at discharge. Front Immunol (2022) 12:797302. doi: 10.3389/fimmu.2021.797302
34. Winek K, Engel O, Koduah P, Heimesaat MM, Fischer A, Bereswill S, et al. Depletion of cultivatable gut microbiota by broad-spectrum antibiotic pretreatment worsens outcome after murine stroke. Stroke. (2016) 47(5):1354–63. doi: 10.1161/STROKEAHA.115.011800
35. Li H, Xu H, Li Y, Jiang Y, Hu Y, Liu T, et al. Alterations of gut microbiota contribute to the progression of unruptured intracranial aneurysms. Nat Commun (2020) 11(1):3218. doi: 10.1038/s41467-020-16990-3
36. Zhang F, Zhai M, Wu Q, Jia X, Wang Y, Wang N. Protective effect of tong-Qiao-Huo-Xue decoction on inflammatory injury caused by intestinal microbial disorders in stroke rats. Biol Pharm Bull (2020) 43(5):788–800. doi: 10.1248/bpb
37. Lee J, d’Aigle J, Atadja L, Quaicoe V, Honarpisheh P, Ganesh BP, et al. Gut microbiota-derived short-chain fatty acids promote poststroke recovery in aged mice. Circ Res (2020) 127(4):453–65. doi: 10.1161/CIRCRESAHA.119.316448
38. Zeng X, Gao X, Peng Y, Wu Q, Zhu J, Tan C, et al. Higher risk of stroke is correlated with increased opportunistic pathogen load and reduced levels of butyrate-producing bacteria in the gut. Front Cell Infect Microbiol (2019) 9:4. doi: 10.3389/fcimb.2019.00004
39. Xu DJ, Wang KC, Yuan LB, Li HF, Xu YY, Wei LY, et al. Compositional and functional alterations of gut microbiota in patients with stroke. Nutr Metab Cardiovasc Dis (2021) 31(12):3434–48. doi: 10.1016/j.numecd.2021.08.045
40. Spychala MS, Venna VR, Jandzinski M, Doran SJ, Durgan DJ, Ganesh BP, et al. Age-related changes in the gut microbiota influence systemic inflammation and stroke outcome. Ann Neurol (2018) 84(1):23–36. doi: 10.1002/ana.25250
41. Tan C, Wu Q, Wang H, Gao X, Xu R, Cui Z, et al. Dysbiosis of gut microbiota and short-chain fatty acids in acute ischemic stroke and the subsequent risk for poor functional outcomes. JPEN J Parenter Enteral Nutr (2021) 45(3):518–29. doi: 10.1002/jpen.1861
42. Stanley D, Moore RJ, Wong CHY. An insight into intestinal mucosal microbiota disruption after stroke. Sci Rep (2018) 8(1):568. doi: 10.1038/s41598-017-18904-8
43. Li N, Wang X, Sun C, Wu X, Lu M, Si Y, et al. Change of intestinal microbiota in cerebral ischemic stroke patients. BMC Microbiol (2019) 19(1):191. doi: 10.1186/s12866-019-1552-1
44. Lin H, Chen S, Shen L, Hu T, Cai J, Zhan S, et al. Integrated analysis of the cecal microbiome and plasma metabolomics to explore NaoMaiTong and its potential role in changing the intestinal flora and their metabolites in ischemic stroke. Front Pharmacol (2022) 12:773722. doi: 10.3389/fphar.2021.773722
45. Gu M, Chen N, Sun H, Li Z, Chen X, Zhou J, et al. Roseburia abundance associates with severity, evolution and outcome of acute ischemic stroke. Front Cell Infect Microbiol (2021) 11:669322. doi: 10.3389/fcimb.2021.669322
46. Wang H, Song W, Wu Q, Gao X, Li J, Tan C, et al. Fecal transplantation from db/db mice treated with sodium butyrate attenuates ischemic stroke injury. Microbiol Spectr (2021) 9(2):e0004221. doi: 10.1128/Spectrum.00042-21
47. Kang Y, Yang Y, Wang J, Ma Y, Cheng H, Wan D. Correlation between intestinal flora and serum inflammatory factors in post-stroke depression in ischemic stroke. J Coll Physicians Surg Pak (2021) 31(10):1224–7. doi: 10.29271/jcpsp.2021.10.1224
48. Yu X, Fu X, Wu X, Tang W, Xu L, Hu L, et al. Metformin alleviates neuroinflammation following intracerebral hemorrhage in mice by regulating Microglia/Macrophage phenotype in a gut microbiota-dependent manner. Front Cell Neurosci (2022) 15:789471. doi: 10.3389/fncel.2021.789471
49. Polster SP, Sharma A, Tanes C, Tang AT, Mericko P, Cao Y, et al. Permissive microbiome characterizes human subjects with a neurovascular disease cavernous angioma. Nat Commun (2020) 11(1):2659. doi: 10.1038/s41467-020-16436-w
50. Chen Y, Liang J, Ouyang F, Chen X, Lu T, Jiang Z, et al. Persistence of gut microbiota dysbiosis and chronic systemic inflammation after cerebral infarction in cynomolgus monkeys. Front Neurol (2019) 10:661. doi: 10.3389/fneur.2019.00661
51. Chen R, Xu Y, Wu P, Zhou H, Lasanajak Y, Fang Y, et al. Transplantation of fecal microbiota rich in short chain fatty acids and butyric acid treat cerebral ischemic stroke by regulating gut microbiota. Pharmacol Res (2019) 148:104403. doi: 10.1016/j.phrs.2019.104403
52. Li Q, Guo Y, Yu X, Liu W, Zhou L. Protective mechanism of rhubarb anthraquinone glycosides in rats with cerebral ischaemia-reperfusion injury: interactions between medicine and intestinal flora. Chin Med (2020) 15:60. doi: 10.1186/s13020-020-00341-x
53. Xu N, Kan P, Yao X, Yang P, Wang J, Xiang L, et al. Astragaloside IV reversed the autophagy and oxidative stress induced by the intestinal microbiota of AIS in mice. J Microbiol (2018) 56(11):838–46. doi: 10.1007/s12275-018-8327-5
54. Ji W, Zhu Y, Kan P, Cai Y, Wang Z, Wu Z, et al. Analysis of intestinal microbial communities of cerebral infarction and ischemia patients based on high throughput sequencing technology and glucose and lipid metabolism. Mol Med Rep (2017) 16(4):5413–7. doi: 10.3892/mmr.2017.7227
55. Yamashiro K, Tanaka R, Urabe T, Ueno Y, Yamashiro Y, Nomoto K, et al. Gut dysbiosis is associated with metabolism and systemic inflammation in patients with ischemic stroke. PloS One (2017) 12(2):e0171521. doi: 10.1371/journal.pone.0171521
56. Stanley D, Mason LJ, Mackin KE, Srikhanta YN, Lyras D, Prakash MD, et al. Translocation and dissemination of commensal bacteria in post-stroke infection. Nat Med (2016) 22(11):1277–84. doi: 10.1038/nm.4194
57. Wang W, Li X, Yao X, Cheng X, Zhu Y. The characteristics analysis of intestinal microecology on cerebral infarction patients and its correlation with apolipoprotein e. Medicine (2018) 97(41):e12805. doi: 10.1097/MD.0000000000012805
58. Huang L, Wang T, Wu Q, Dong X, Shen F, Liu D, et al. Analysis of microbiota in elderly patients with acute cerebral infarction. PeerJ. (2019) 7:e6928. doi: 10.7717/peerj.6928
59. Houlden A, Goldrick M, Brough D, Vizi ES, Lénárt N, Martinecz B, et al. Brain injury induces specific changes in the caecal microbiota of mice via altered autonomic activity and mucoprotein production. Brain Behav Immun (2016) 57:10–20. doi: 10.1016/j.bbi.2016.04.003
60. Wu W, Sun Y, Luo N, Cheng C, Jiang C, Yu Q, et al. Integrated 16S rRNA gene sequencing and LC-MS analysis revealed the interplay between gut microbiota and plasma metabolites in rats with ischemic stroke. J Mol Neurosci (2021) 71(10):2095–106. doi: 10.1007/s12031-021-01828-4
61. Kawabata S, Takagaki M, Nakamura H, Oki H, Motooka D, Nakamura S, et al. Dysbiosis of gut microbiome is associated with rupture of cerebral aneurysms. Stroke. (2022) 53(3):895–903. doi: 10.1161/STROKEAHA.121.034792
62. Wang Z, Xu K, Zhou H. [Characteristics of gut virome and microbiome in patients with stroke]. Nan Fang Yi Ke Da Xue Xue Bao (2021) 41(6):862–9. doi: 10.12122/j.issn.1673-4254.2021.06.08
63. Guo Q, Jiang X, Ni C, Li L, Chen L, Wang Y, et al. Gut microbiota-related effects of tanhuo decoction in acute ischemic stroke. Oxid Med Cell Longev (2021) 2021:5596924. doi: 10.1155/2021/5596924
64. Xia GH, Zhang MS, Wu QH, Wang HD, Zhou HW, He Y, et al. Dysbiosis of gut microbiota is an independent risk factor of stroke-associated pneumonia: A Chinese pilot study. Front Cell Infect Microbiol (2021) 11:715475. doi: 10.3389/fcimb.2021.715475
65. Shin NR, Whon TW, Bae JW. Proteobacteria: microbial signature of dysbiosis in gut microbiota. Trends Biotechnol (2015) 33(9):496–503. doi: 10.1016/j.tibtech.2015.06.011
66. Litvak Y, Byndloss MX, Tsolis RM, Bäumler AJ. Dysbiotic proteobacteria expansion: a microbial signature of epithelial dysfunction. Curr Opin Microbiol (2017) 39:1–6. doi: 10.1016/j.mib.2017.07.003
67. Huang Q, Di L, Yu F, Feng X, Liu Z, Wei M, et al. Alterations in the gut microbiome with hemorrhagic transformation in experimental stroke. CNS Neurosci Ther (2022) 28(1):77–91. doi: 10.1111/cns.13736
68. Park MJ, Pilla R, Panta A, Pandey S, Sarawichitr B, Suchodolski J, et al. Reproductive senescence and ischemic stroke remodel the gut microbiome and modulate the effects of estrogen treatment in female rats. Transl Stroke Res (2020) 11(4):812–30. doi: 10.1007/s12975-019-00760-5
69. Ley RE, Turnbaugh PJ, Klein S, Gordon JI. Microbial ecology: human gut microbes associated with obesity. Nature. (2006) 444(7122):1022–3. doi: 10.1038/4441022a
70. Koliada A, Syzenko G, Moseiko V, Budovska L, Puchkov K, Perederiy V, et al. Association between body mass index and Firmicutes/Bacteroidetes ratio in an adult Ukrainian population. BMC Microbiol (2017) 17(1):120. doi: 10.1186/s12866-017-1027-1
71. Nicholson JK, Holmes E, Kinross J, Burcelin R, Gibson G, Jia W, et al. Host-gut microbiota metabolic interactions. Science. (2012) 336(6086):1262–7. doi: 10.1126/science.1223813
72. Tang WH, Kitai T, Hazen SL. Gut microbiota in cardiovascular health and disease. Circ Res (2017) 120(7):1183–96. doi: 10.1161/CIRCRESAHA.117.309715
73. Peter J, Fournier C, Keip B, Rittershaus N, Stephanou-Rieser N, Durdevic M, et al. Intestinal microbiome in irritable bowel syndrome before and after gut-directed hypnotherapy. Int J Mol Sci (2018) 19(11):3619. doi: 10.3390/ijms19113619
74. Liu P, Wang Y, Yang G, Zhang Q, Meng L, Xin Y, et al. The role of short-chain fatty acids in intestinal barrier function, inflammation, oxidative stress, and colonic carcinogenesis. Pharmacol Res (2021) 165:105420. doi: 10.1016/j.phrs.2021.105420
75. Scheppach W. Effects of short chain fatty acids on gut morphology and function. Gut (1994) 35(1 Suppl):S35–8. doi: 10.1136/gut.35.1_suppl.s35
76. Yang T, Santisteban MM, Rodriguez V, Li E, Ahmari N, Carvajal JM, et al. Gut dysbiosis is linked to hypertension. Hypertension. (2015) 65(6):1331–40. doi: 10.1161/HYPERTENSIONAHA.115.05315
77. Xiong Z, Peng K, Song S, Zhu Y, Gu J, Huang C, et al. Cerebral intraparenchymal hemorrhage changes patients’ gut bacteria composition and function. Front Cell Infect Microbiol (2022) 12:829491. doi: 10.3389/fcimb.2022.829491
78. Chen XL, Li Q, Huang WS, Lin YS, Xue J, Wang B, et al. Serum YKL-40, a prognostic marker in patients with large-artery atherosclerotic stroke. Acta Neurol Scand (2017) 136(2):97–102. doi: 10.1111/ane.12688
79. Xu Y, Wang N, Tan HY, Li S, Zhang C, Feng Y. Function of akkermansia muciniphila in obesity: Interactions with lipid metabolism, immune response and gut systems. Front Microbiol (2020) 11:219. doi: 10.3389/fmicb.2020.00219
80. Ling Y, Gu Q, Zhang J, Gong T, Weng X, Liu J, et al. Structural change of gut microbiota in patients with post-stroke comorbid cognitive impairment and depression and its correlation with clinical features. J Alzheimers Dis (2020) 77(4):1595–608. doi: 10.3233/JAD-200315
81. Sadler R, Cramer JV, Heindl S, Kostidis S, Betz D, Zuurbier KR, et al. Short-chain fatty acids improve poststroke recovery via immunological mechanisms. J Neurosci (2020) 40(5):1162–73. doi: 10.1523/JNEUROSCI.1359-19.2019
82. Guo Y, Li Q, Yu X, Liang Y. Rhubarb anthraquinone glycosides protect against cerebral ischemia-reperfusion injury in rats by regulating brain-gut neurotransmitters. BioMed Chromatogr (2021) 35(5):e5058. doi: 10.1002/bmc.5058
83. Saji N, Murotani K, Hisada T, Tsuduki T, Sugimoto T, Kimura A, et al. The association between cerebral small vessel disease and the gut microbiome: A cross-sectional analysis. J Stroke Cerebrovasc Dis (2021) 30(3):105568. doi: 10.1016/j.jstrokecerebrovasdis.2020.105568
84. Tang AT, Choi JP, Kotzin JJ, Yang Y, Hong CC, Hobson N, et al. Endothelial TLR4 and the microbiome drive cerebral cavernous malformations. Nature. (2017) 545(7654):305–10. doi: 10.1038/nature22075
85. El-Hakim Y, Mani KK, Eldouh A, Pandey S, Grimaldo MT, Dabney A, et al. Sex differences in stroke outcome correspond to rapid and severe changes in gut permeability in adult sprague-dawley rats. Biol Sex Differ (2021) 12(1):14. doi: 10.1186/s13293-020-00352-1
86. Liu Q, Johnson EM, Lam RK, Wang Q, Bo Ye H, Wilson EN, et al. Peripheral TREM1 responses to brain and intestinal immunogens amplify stroke severity. Nat Immunol (2019) 20(8):1023–34. doi: 10.1038/s41590-019-0421-2
87. Benakis C, Brea D, Caballero S, Faraco G, Moore J, Murphy M, et al. Commensal microbiota affects ischemic stroke outcome by regulating intestinal γδ T cells. Nat Med (2016) 22(5):516–23. doi: 10.1038/nm.4068
88. Kurita N, Yamashiro K, Kuroki T, Tanaka R, Urabe T, Ueno Y, et al. Metabolic endotoxemia promotes neuroinflammation after focal cerebral ischemia. J Cereb Blood Flow Metab (2020) 40(12):2505–20. doi: 10.1177/0271678X19899577
89. Klimiec E, Pasinska P, Kowalska K, Pera J, Slowik A, Dziedzic T. The association between plasma endotoxin, endotoxin pathway proteins and outcome after ischemic stroke. Atherosclerosis. (2018) 269:138–43. doi: 10.1016/j.atherosclerosis.2017.12.034
90. Lambertsen KL, Biber K, Finsen B. Inflammatory cytokines in experimental and human stroke. J Cereb Blood Flow Metab (2012) 32(9):1677–98. doi: 10.1038/jcbfm.2012.88
91. Yang J, Zhou G, Ou Z, Jia N, Wang D. The effect and mechanism of exercise training on rats with poststroke depression based on the intestinal flora. Comput Math Methods Med (2021) 2021:3567447. doi: 10.1155/2021/3567447
92. Chalouhi N, Hoh BL, Hasan D. Review of cerebral aneurysm formation, growth, and rupture. Stroke. (2013) 44(12):3613–22. doi: 10.1161/STROKEAHA.113.002390
93. Shikata F, Shimada K, Sato H, Ikedo T, Kuwabara A, Furukawa H, et al. Potential influences of gut microbiota on the formation of intracranial aneurysm. Hypertension. (2019) 73(2):491–6. doi: 10.1161/HYPERTENSIONAHA.118.11804
94. Kalff JC, Schraut WH, Billiar TR, Simmons RL, Bauer AJ. Role of inducible nitric oxide synthase in postoperative intestinal smooth muscle dysfunction in rodents. Gastroenterology. (2000) 118(2):316–27. doi: 10.1016/s0016-5085(00)70214-9
95. Wehner S, Vilz TO, Stoffels B, Kalff JC. Immune mediators of postoperative ileus. Langenbecks Arch Surg (2012) 397(4):591–601. doi: 10.1007/s00423-012-0915-y
96. The FO, de Jonge WJ, Bennink RJ, van den Wijngaard RM, Boeckxstaens GE. The ICAM-1 antisense oligonucleotide ISIS-3082 prevents the development of postoperative ileus in mice. Br J Pharmacol (2005) 146(2):252–8. doi: 10.1038/sj.bjp.0706303
97. Wehner S, Schwarz NT, Hundsdoerfer R, Hierholzer C, Tweardy DJ, Billiar TR, et al. Induction of IL-6 within the rodent intestinal muscularis after intestinal surgical stress. Surgery. (2005) 137(4):436–46. doi: 10.1016/j.surg.2004.11.003
98. Turner JR. ‘Putting the squeeze’ on the tight junction: understanding cytoskeletal regulation. Semin Cell Dev Biol (2000) 11(4):301–8. doi: 10.1006/scdb.2000.0180
99. Yu X, Zhou G, Shao B, Zhou H, Xu C, Yan F, et al. Gut microbiota dysbiosis induced by intracerebral hemorrhage aggravates neuroinflammation in mice. Front Microbiol (2021) 12:647304. doi: 10.3389/fmicb.2021.647304
100. Van Spaendonk H, Ceuleers H, Witters L, Patteet E, Joossens J, Augustyns K, et al. Regulation of intestinal permeability: The role of proteases. World J Gastroenterol (2017) 23(12):2106–23. doi: 10.3748/wjg
101. Xu X, Zhu Y, Chuai J. Changes in serum ghrelin and small intestinal motility in rats with ischemic stroke. Anat Rec (2012) 295(2):307–12. doi: 10.1002/ar.21490
102. Cheng Y, Wei Y, Yang W, Cai Y, Chen B, Yang G, et al. Ghrelin attenuates intestinal barrier dysfunction following intracerebral hemorrhage in mice. Int J Mol Sci (2016) 17(12):2032. doi: 10.3390/ijms17122032
103. Tang AT, Sullivan KR, Hong CC, Goddard LM, Mahadevan A, Ren A, et al. Distinct cellular roles for PDCD10 define a gut-brain axis in cerebral cavernous malformation. Sci Transl Med (2019) 27:11(520):eaaw3521. doi: 10.1126/scitranslmed
104. Chen R, Xu Y, Wu P, Zhou H, Lasanajak Y, Fang Y, et al. Transplantation of fecal microbiota rich in short chain fatty acids and butyric acid treat cerebral ischemic stroke by regulating gut microbiota. Pharmacol Res 148:104403. doi: 10.1016/j.phrs.2019.104403
105. Feng Y, Wang Y, Wang P, Huang Y, Wang F. Short-chain fatty acids manifest stimulative and protective effects on intestinal barrier function through the inhibition of NLRP3 inflammasome and autophagy. Cell Physiol Biochem (2018) 49(1):190–205. doi: 10.1159/000492853
106. Kelly CJ, Zheng L, Campbell EL, Saeedi B, Scholz CC, Bayless AJ, et al. Crosstalk between microbiota-derived short-chain fatty acids and intestinal epithelial HIF augments tissue barrier function. Cell Host Microbe (2015) 17(5):662–71. doi: 10.1016/j.chom.2015.03.005
107. He S, Jiang H, Zhuo C, Jiang W. Trimethylamine/Trimethylamine-N-Oxide as a key between diet and cardiovascular diseases. Cardiovasc Toxicol (2021) 21(8):593–604. doi: 10.1007/s12012-021-09656-z
108. Zhang J, Wang L, Cai J, Lei A, Liu C, Lin R, et al. Gut microbial metabolite TMAO portends prognosis in acute ischemic stroke. J Neuroimmunol (2021) 354:577526. doi: 10.1016/j.jneuroim.2021.577526
109. Wang Z, Klipfell E, Bennett BJ, Koeth R, Levison BS, Dugar B, et al. Gut flora metabolism of phosphatidylcholine promotes cardiovascular disease. Nature. (2011) 472(7341):57–63. doi: 10.1038/nature09922
110. Li T, Chen Y, Gua C, Li X. Elevated circulating trimethylamine n-oxide levels contribute to endothelial dysfunction in aged rats through vascular inflammation and oxidative stress. Front Physiol (2017) 8:350. doi: 10.3389/fphys.2017.00350
111. Seldin MM, Meng Y, Qi H, Zhu W, Wang Z, Hazen SL, et al. Trimethylamine n-oxide promotes vascular inflammation through signaling of mitogen-activated protein kinase and nuclear factor-κB. J Am Heart Assoc (2016) 5(2):e002767. doi: 10.1161/JAHA.115.002767
112. Haghikia A, Li XS, Liman TG, Bledau N, Schmidt D, Zimmermann F, et al. Gut microbiota-dependent trimethylamine n-oxide predicts risk of cardiovascular events in patients with stroke and is related to proinflammatory monocytes. Arterioscler Thromb Vasc Biol (2018) 38(9):2225–35. doi: 10.1161/ATVBAHA.118.311023
113. Hoyles L, Pontifex MG, Rodriguez-Ramiro I, Anis-Alavi MA, Jelane KS, Snelling T, et al. Regulation of blood-brain barrier integrity by microbiome-associated methylamines and cognition by trimethylamine n-oxide. Microbiome. (2021) 9(1):235. doi: 10.1186/s40168-021-01181-z
114. Li C, Zhu L, Dai Y, Zhang Z, Huang L, Wang TJ, et al. Diet-induced high serum levels of trimethylamine-n-oxide enhance the cellular inflammatory response without exacerbating acute intracerebral hemorrhage injury in mice. Oxid Med Cell Longev (2022) 2022:1599747. doi: 10.1155/2022/1599747
115. Koh A, De Vadder F, Kovatcheva-Datchary P, Bäckhed F. From dietary fiber to host physiology: Short-chain fatty acids as key bacterial metabolites. Cell. (2016) 165(6):1332–45. doi: 10.1016/j.cell.2016.05.041
116. Sun M, Zhao YM, Gu Y, Xu C. Therapeutic window of taurine against experimental stroke in rats. Transl Res (2012) 160(3):223–9. doi: 10.1016/j.trsl.2012.02.007
117. Sun M, Zhao Y, Gu Y, Xu C. Anti-inflammatory mechanism of taurine against ischemic stroke is related to down-regulation of PARP and NF-κB. Amino Acids (2012) 42(5):1735–47. doi: 10.1007/s00726-011-0885-3
118. Bian KY, Jin HF, Sun W, Sun YJ. DCA can improve the ACI-induced neurological impairment through negative regulation of Nrf2 signaling pathway. Eur Rev Med Pharmacol Sci (2019) 23(1):343–51. doi: 10.26355/eurrev_201901_16782
119. Rodrigues CM, Sola S, Nan Z, Castro RE, Ribeiro PS, Low WC, et al. Tauroursodeoxycholic acid reduces apoptosis and protects against neurological injury after acute hemorrhagic stroke in rats. Proc Natl Acad Sci U S A (2003) 100(10):6087–92. doi: 10.1073/pnas.1031632100
120. Nemet I, Saha PP, Gupta N, Zhu W, Romano KA, Skye SM, et al. A cardiovascular disease-linked gut microbial metabolite acts via adrenergic receptors. Cell. (2020) 180(5):862–877.e22. doi: 10.1016/j.cell.2020.02.016
121. Yu F, Feng X, Li X, Luo Y, Wei M, Zhao T, et al. Gut-derived metabolite phenylacetylglutamine and white matter hyperintensities in patients with acute ischemic stroke. Front Aging Neurosci (2021) 13:675158. doi: 10.3389/fnagi.2021.675158
122. Krishnan S, Ding Y, Saedi N, Choi M, Sridharan GV, Sherr DH, et al. Gut microbiota-derived tryptophan metabolites modulate inflammatory response in hepatocytes and macrophages. Cell Rep (2018) 23(4):1099–111. doi: 10.1016/j.celrep.2018.03.109
123. Darlington LG, Mackay GM, Forrest CM, Stoy N, George C, Stone TW. Altered kynurenine metabolism correlates with infarct volume in stroke. Eur J Neurosci (2007) 26(8):2211–21. doi: 10.1111/j.1460-9568.2007.05838
124. Kim M, Huda MN, Bennett BJ. Sequence meets function-microbiota and cardiovascular disease. Cardiovasc Res (2022) 118(2):399–412. doi: 10.1093/cvr/cvab030
125. Wang HX, Wang YP. Gut microbiota-brain axis. Chin Med J (2016) 129(19):2373–80. doi: 10.4103/0366-6999.190667
126. Galland L. The gut microbiome and the brain. J Med Food (2014) 17(12):1261–72. doi: 10.1089/jmf.2014.7000
127. Mulak A, Bonaz B. Brain-gut-microbiota axis in parkinson’s disease. World J Gastroenterol (2015) 21(37):10609–20. doi: 10.3748/wjg.v21.i37.10609
128. Foster JA, McVey Neufeld KA. Gut-brain axis: how the microbiome influences anxiety and depression. Trends Neurosci (2013) 36(5):305–12. doi: 10.1016/j.tins.2013.01.005
129. Al Omran Y, Aziz Q. The brain-gut axis in health and disease. Adv Exp Med Biol (2014) 817:135–53. doi: 10.1007/978-1-4939-0897-4_6
130. Borre YE, O’Keeffe GW, Clarke G, Stanton C, Dinan TG, Cryan JF. Microbiota and neurodevelopmental windows: implications for brain disorders. Trends Mol Med (2014) 20(9):509–18. doi: 10.1016/j.molmed.2014.05.002
131. Sudo N, Chida Y, Aiba Y, Sonoda J, Oyama N, Yu XN, et al. Postnatal microbial colonization programs the hypothalamic-pituitary-adrenal system for stress response in mice. J Physiol (2004) 558(Pt 1):263–75. doi: 10.1113/jphysiol.2004.063388
132. Bailey MT, Dowd SE, Galley JD, Hufnagle AR, Allen RG, Lyte M. Exposure to a social stressor alters the structure of the intestinal microbiota: implications for stressor-induced immunomodulation. Brain Behav Immun (2011) 25(3):397–407. doi: 10.1016/j.bbi.2010.10.023
133. Dissanayake D, Hall H, Berg-Brown N, Elford AR, Hamilton SR, Murakami K, et al. Nuclear factor-κB1 controls the functional maturation of dendritic cells and prevents the activation of autoreactive T cells. Nat Med (2011) 17(12):1663–7. doi: 10.1038/nm.2556
134. O’Hara AM, Shanahan F. The gut flora as a forgotten organ. EMBO Rep (2006) 7(7):688–93. doi: 10.1038/sj.embor.7400731
135. Forsythe P, Bienenstock J, Kunze WA. Vagal pathways for microbiome-brain-gut axis communication. Adv Exp Med Biol (2014) 817:115–33. doi: 10.1007/978-1-4939-0897-4_5
136. Ye L, Bae M, Cassilly CD, Jabba SV, Thorpe DW, Martin AM, et al. Enteroendocrine cells sense bacterial tryptophan catabolites to activate enteric and vagal neuronal pathways. Cell Host Microbe (2021) 29(2):179–96. doi: 10.1016/j.chom.2020.11.011
137. Benech N, Rolhion N, Sokol H. Tryptophan metabolites get the gut moving. Cell Host Microbe (2021) 29(2):145–7. doi: 10.1016/j.chom.2021.01.009
138. Wurtman RJ. Synapse formation and cognitive brain development: effect of docosahexaenoic acid and other dietary constituents. Metabolism (2008) 57 Suppl 2(Suppl 2):S6–10. doi: 10.1016/j.metabol.2008.07.007
139. Gostner JM, Geisler S, Stonig M, Mair L, Sperner-Unterweger B, Fuchs D. Tryptophan metabolism and related pathways in psychoneuroimmunology: The impact of nutrition and lifestyle. Neuropsychobiology. (2020) 79(1):89–99. doi: 10.1159/000496293
140. Baden MY, Shan Z, Wang F, Li Y, Manson JE, Rimm EB, et al. Quality of plant-based diet and risk of total, ischemic, and hemorrhagic stroke. Neurology. (2021) 96(15):e1940–53. doi: 10.1212/WNL.0000000000011713
141. Bourassa MW, Alim I, Bultman SJ, Ratan RR. Butyrate, neuroepigenetics and the gut microbiome: Can a high fiber diet improve brain health? Neurosci Lett (2016) 625:56–63. doi: 10.1016/j.neulet.2016.02.009
142. Shaafi S, Sharifi-Bonab M, Ghaemian N, Mokhtarkhani M, Akbari H. Early motor-behavioral outcome of ischemic stroke with ketogenic diet preconditioning: Interventional animal study. J Stroke Cerebrovasc Dis (2019) 28(4):1032–9. doi: 10.1016/j.jstrokecerebrovasdis.2018.12.024
143. Shaafi S, Mahmoudi J, Pashapour A, Farhoudi M, Sadigh-Eteghad S, Akbari H. Ketogenic diet provides neuroprotective effects against ischemic stroke neuronal damages. Adv Pharm Bull (2014) 4(Suppl 2):479–81. doi: 10.5681/apb.2014.071
144. Shimizu C, Wakita Y, Kihara M, Kobayashi N, Tsuchiya Y, Nabeshima T. Association of lifelong intake of barley diet with healthy aging: Changes in physical and cognitive functions and intestinal microbiome in senescence-accelerated mouse-prone 8 (SAMP8). Nutrients. (2019) 11(8):1770. doi: 10.3390/nu11081770
145. Priebe MG, Wang H, Weening D, Schepers M, Preston T, Vonk RJ. Factors related to colonic fermentation of nondigestible carbohydrates of a previous evening meal increase tissue glucose uptake and moderate glucose-associated inflammation. Am J Clin Nutr (2010) 91(1):90–7. doi: 10.3945/ajcn.2009.28521
147. Zhu W, Gregory JC, Org E, Buffa JA, Gupta N, Wang Z, et al. Gut microbial metabolite TMAO enhances platelet hyperreactivity and thrombosis risk. Cell. (2016) 165(1):111–24. doi: 10.1016/j.cell.2016.02.011
148. Tang WH, Wang Z, Levison BS, Koeth RA, Britt EB, Fu X, et al. Intestinal microbial metabolism of phosphatidylcholine and cardiovascular risk. N Engl J Med (2013) 368(17):1575–84. doi: 10.1056/NEJMoa1109400
149. Zhu W, Romano KA, Li L, Buffa JA, Sangwan N, Prakash P, et al. Gut microbes impact stroke severity via the trimethylamine n-oxide pathway. Cell Host Microbe (2021) 29(7):1199–208. doi: 10.1016/j.chom.2021.05.002
150. Gansevoort RT, Correa-Rotter R, Hemmelgarn BR, Jafar TH, Heerspink HJ, Mann JF, et al. Chronic kidney disease and cardiovascular risk: epidemiology, mechanisms, and prevention. Lancet 2013 Jul (9889) 27:382. doi: 10.1016/S0140-6736(13)60595-4
151. Olson CA, Vuong HE, Yano JM, Liang QY, Nusbaum DJ, Hsiao EY. The gut microbiota mediates the anti-seizure effects of the ketogenic diet. Cell. (2018) 173(7):1728–1741.e13. doi: 10.1016/j.cell.2018.04.027
152. Swidsinski A, Dörffel Y, Loening-Baucke V, Gille C, Göktas Ö, Reißhauer A, et al. Reduced mass and diversity of the colonic microbiome in patients with multiple sclerosis and their improvement with ketogenic diet. Front Microbiol (2017) 8:1141. doi: 10.3389/fmicb.2017.01141
153. Sherry CL, Kim SS, Dilger RN, Bauer LL, Moon ML, Tapping RI, et al. Sickness behavior induced by endotoxin can be mitigated by the dietary soluble fiber, pectin, through up-regulation of IL-4 and Th2 polarization. Brain Behav Immun (2010) 24(4):631–40. doi: 10.1016/j.bbi.2010.01.015
154. Casiglia E, Tikhonoff V, Caffi S, Boschetti G, Grasselli C, Saugo M, et al. High dietary fiber intake prevents stroke at a population level. Clin Nutr (2013) 32(5):811–8. doi: 10.1016/j.clnu.2012.11.025
155. Liu T, Yang Z, Zhang X, Han N, Yuan J, Cheng Y. 16S rDNA analysis of the effect of fecal microbiota transplantation on pulmonary and intestinal flora. 3 Biotech (2017) 7(6):370. doi: 10.1007/s13205-017-0997-x
156. Bindels LB, Delzenne NM, Cani PD, Walter J. Towards a more comprehensive concept for prebiotics. Nat Rev Gastroenterol Hepatol (2015) 12(5):303–10. doi: 10.1038/nrgastro.2015.47
157. Hill C, Guarner F, Reid G, Gibson GR, Merenstein DJ, Pot B, et al. Expert consensus document. the international scientific association for probiotics and prebiotics consensus statement on the scope and appropriate use of the term probiotic. Nat Rev Gastroenterol Hepatol (2014) 11(8):506–14. doi: 10.1038/nrgastro.2014.66
158. Abraham BP, Quigley EMM. Probiotics in inflammatory bowel disease. Gastroenterol Clin North Am (2017) 46(4):769–82. doi: 10.1016/j.gtc.2017.08.003
159. Wu H, Chiou J. Potential benefits of probiotics and prebiotics for coronary heart disease and stroke. Nutrients. (2021) 13(8):2878. doi: 10.3390/nu13082878
160. McLoughlin RF, Berthon BS, Jensen ME, Baines KJ, Wood LG. Short-chain fatty acids, prebiotics, synbiotics, and systemic inflammation: a systematic review and meta-analysis. Am J Clin Nutr (2017) 106(3):930–45. doi: 10.3945/ajcn.117.156265
161. Zhong DY, Li L, Ma RM, Deng YH. The effect of probiotics in stroke treatment. Evid Based Complement Alternat Med (2021) 2021:4877311. doi: 10.1155/2021/4877311
162. Chen R, Wu P, Cai Z, Fang Y, Zhou H, Lasanajak Y, et al. Puerariae lobatae radix with chuanxiong rhizoma for treatment of cerebral ischemic stroke by remodeling gut microbiota to regulate the brain-gut barriers. J Nutr Biochem (2019) 65:101–14. doi: 10.1016/j.jnutbio.2018.12.004
163. Gao Q, Han ZY, Tian DF, Liu GL, Wang ZY, Lin JF, et al. Xinglou chengqi decoction improves neurological function in experimental stroke mice as evidenced by gut microbiota analysis and network pharmacology. Chin J Nat Med (2021) 19(12):881–99. doi: 10.1016/S1875-5364(21)60079-1
164. Xia ZY, Luo C, Liu BW, Bian XQ, Li Y, Pang AM, et al. Shengui sansheng pulvis maintains blood-brain barrier integrity by vasoactive intestinal peptide after ischemic stroke. Phytomedicine. (2020) 67:153158. doi: 10.1016/j.phymed.2019.153158
165. Rustia AJ, Paterson JS, Best G, Sokoya EM. Microbial disruption in the gut promotes cerebral endothelial dysfunction. Physiol Rep (2021) 9(21):e15100. doi: 10.14814/phy2.15100
Keywords: cerebrovascular diseases, ischemic stroke, intestinal microbiome, fecal bacteria transplantation, inflammation, immunology
Citation: Zou X, Wang L, Xiao L, Wang S and Zhang L (2022) Gut microbes in cerebrovascular diseases: Gut flora imbalance, potential impact mechanisms and promising treatment strategies. Front. Immunol. 13:975921. doi: 10.3389/fimmu.2022.975921
Received: 22 June 2022; Accepted: 12 October 2022;
Published: 31 October 2022.
Edited by:
John Zhang, Loma Linda University, United StatesReviewed by:
Yingkun He, Henan Provincial People’s Hospital, ChinaCopyright © 2022 Zou, Wang, Xiao, Wang and Zhang. This is an open-access article distributed under the terms of the Creative Commons Attribution License (CC BY). The use, distribution or reproduction in other forums is permitted, provided the original author(s) and the copyright owner(s) are credited and that the original publication in this journal is cited, in accordance with accepted academic practice. No use, distribution or reproduction is permitted which does not comply with these terms.
*Correspondence: Le Zhang, emx6ZHpsemRAY3N1LmVkdS5jbg==; Sai Wang, MTU1MDI3NzM1MUBxcS5jb20=
†These authors have contributed equally to this work
Disclaimer: All claims expressed in this article are solely those of the authors and do not necessarily represent those of their affiliated organizations, or those of the publisher, the editors and the reviewers. Any product that may be evaluated in this article or claim that may be made by its manufacturer is not guaranteed or endorsed by the publisher.
Research integrity at Frontiers
Learn more about the work of our research integrity team to safeguard the quality of each article we publish.