- 1Department of Medical Oncology, The First Affiliated Hospital, Zhejiang University School of Medicine, and Key Laboratory of Cancer Prevention and Intervention, Ministry of Education, Hangzhou, China
- 2Department of Hematology, The First Affiliated Hospital, Zhejiang University School of Medicine, Hangzhou, China
Background: As the forefront of nanomedicine, bionic nanotechnology has been widely used for drug delivery in order to obtain better efficacy but less toxicity for cancer treatments. With the rise of immunotherapy, the combination of nanotechnology and immunotherapy will play a greater potential of anti-tumor therapy. Due to its advantage of homologous targeting and antigen library from source cells, cancer cell membrane (CCM)-wrapped nanoparticles (CCNPs) has become an emerging topic in the field of immunotherapy.
Key scientific concepts of review: CCNP strategies include targeting or modulating the tumor immune microenvironment and combination therapy with immune checkpoint inhibitors and cancer vaccines. This review summarizes the current developments in CCNPs for cancer immunotherapy and provides insight into the challenges of transferring this technology from the laboratory to the clinic as well as the potential future of this technology.
Conclusion: This review described CCNPs have enormous potential in cancer immunotherapy, but there are still challenges in terms of translating their effects in vitro to the clinical setting. We believe that these challenges can be addressed in the future with a focus on individualized treatment with CCNPs as well as CCNPs combined with other effective treatments.
Introduction to cancer immunotherapy and cancer cell membrane-wrapped nanoparticles
Cancer immunotherapy is known as the third technological revolution in cancer treatment. Compared with traditional anti-tumor therapies such as surgery, radiotherapy, and chemotherapy, immunotherapy is an innovative treatment that focuses on the use of the body’s own immune mechanism and has shown breakthrough efficacy for patients with recurrent and refractory malignant tumors (1). First of all, cellular immunotherapy, especially chimeric antigen receptor T-cell immunotherapy (CAR-T), is a representative breakthrough in cancer immunotherapy. CAR-T has accomplished remarkable results in the treatment of hematologic tumors, especially in patients with B-cell acute lymphoblastic leukemia, with an initial response rate of more than 90% (2). Hematologic tumors, however, are only a small part of a larger cancer population, with solid tumors accounting for about 90% of cancer cases, according to the latest cancer data published in 2022 (3). Despite the larger prevalence of solid tumors, the efficacy for solid tumors is not as expected due to the heterogeneity within solid tumors and tumor microenvironment (TME). No clinical application of CAR-T for the treatment of solid tumors has been approved by the US FDA. Targeting transforming growth factor (TGF)-β and oncolytic viruses to TME have been evaluated as therapeutic tools to increase the efficacy of CAR-T in metastatic castration-resistant prostate cancer (mCRPC) and solid tumor mouse models of melanoma and glioma (4, 5). Immune checkpoint inhibitors (ICIs) such as programmed death-ligand 1/programmed death-1 (PD-L1/PD-1) blockade and cytotoxic T-lymphocyte-associated protein 4 (CTLA-4) inhibitors are another representative breakthrough in cancer immunotherapy that has been successfully used in solid tumors (6). Ideal cancer vaccines could overcome the immune suppression in tumors and induce both humoral immunity and cellular immunity, which is considered a promising therapeutic strategy in the immunotherapy of solid tumors (7). Currently, most cancer vaccines are still in the stage of preclinical and clinical research except for Sipuleucel-T (Provenge), a US FDA-approved vaccine for mCRPC (8). To sum up, these three main cancer immunotherapies are still associated with numerous challenges.
In the pursuit of achieving the broader goal of “curing cancer,” apart from determining the dominant drivers of cancer immunity, optimizing long-term survival with multi-agent cancer immunotherapy combination regimens is an important line of research (9). With regard to the second goal, the development of “smart-designed” nanoparticles (NPs) opens up the possibility of therapeutic advancement. NPs can be classified as organic and inorganic. Polylactic glycolic acid (PLGA) and indocyanine green are the most commonly used organic NPs and the only near-infrared dyes approved by the US FDA, respectively (10). Liposomes and gelatin are other organic NPs, which also have the advantages of biocompatibility, biodegradability and non-toxicity (11). On the other hand, mesoporous silica (the US FDA-approved), Fe3O4, gold, upconversion NPs, etc., are commonly used inorganic NPs (12). The metal tunable features and electrical, optical, thermal and magnetic properties of inorganic NPs bring a rich combination to the design (13). “Smart-designed” NPs could be used for the delivery of single or multiple therapeutic cargos and could be integrated with photodynamic therapy (PDT), photothermal therapy (PTT) and acoustic dynamic therapy (SDT) to further increase the effects of drugs (14–16). However, NPs are exogenous substances that are recognized by the immune system and by renal and hepatic clearance. Biomimetic nanoengineering of NPs wrapped with cell membranes can make up for this drawback. Unlike most other membrane donor cells, cancer cells are easy to culture in large volumes in vitro for mass membrane collection, and more importantly, cancer cell membranes (CCMs) inherit the functions of homologous targeting and antigen library from source cells and have been used in cancer-targeted therapy and cellular immunotherapy (15, 17–22). Hence, CCMs are the ideal candidate for the engineering of NPs for cancer therapy (23). The first study on these membranes showed that the MDA-MB-435 tumor cell membrane coating enabled approximately 40-fold and 20-fold increases in uptake compared with red cell NPs and bare PLGA cores, respectively (22). Since this initial work, the use of CCM-wrapped NPs (CCNPs) has become an emerging topic in the field of anti-tumor therapy and immunotherapy today. In the following sections, we present an exploration of the application of CCNPs in diverse immunotherapy approaches and combination therapy. We also offer a constructive view on the challenges involved in transferring the progresses in this technology from the laboratory to the clinic (Figure 1).
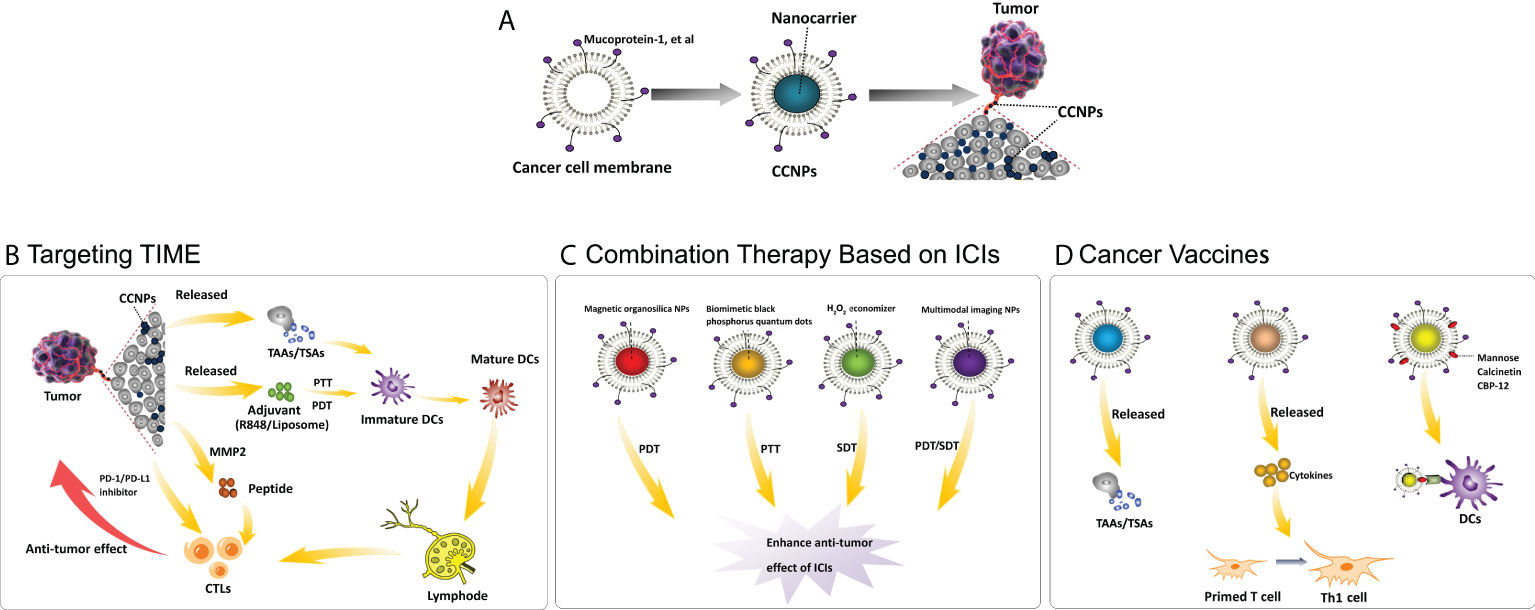
Figure 1 Application of cancer cell membrane-wrapped nanoparticles for cancer immunotherapy. (A) The structure of CCNPs; (B) Mechanism of CCNPs targeting on TME: CCNPs directly carry TSAs from cancer cell membranes or by different stimuli, such as PTT, then release TAAs. The released TSAs/TAAs, together with the immune adjuvant (R837/Liposome), drive the maturation of DCs. The CCNPs also used the tumor-specific enzyme metallomatrix protease 2 (MMP2) to dramatically extend the half-life of the peptides, e.g. PD-L1 inhibitory peptides, or combined with PD-1/PD-L1 inhibitors to further enhance the anti-tumor effect. The above processes promote the infiltration of CTLs for treating distant metastasis. (C) Combination therapy based on ICIs: A combination therapeutic strategy that includes PDT, PTT and SDT, particularly robust anti-cancer agents loaded on CCNPs, results in enhanced anti-tumor effect of PD-1/PD-L1 inhibitors. (D) Cancer vaccines: As tumor vaccines, CCNPs can directly release TSAs/TAAs, release cytokines to promote the transformation of functional immune cells or are added special molecules on the cancer cell membrane to increase the antigen presentation to DCs, thus further enhancing the anti-tumor immune effect. Abbreviations: CCNPs = cancer cell membrane-wrapped nanoparticles, TIME = tumor immune microenvironment, TAAs = tumor-associated antigens, TSAs = tumor-specific antigens, PTT = photothermal therapy, PDT = photodynamic therapy, MMP2 = metallomatrix protease 2, PD-L1/PD-1 = programmed death-ligand 1/programmed death-1, CTLs = cytotoxic T lymphocytes, DCs = dendritic cells, ICIs = immune checkpoint inhibitors, NPs = nanoparticles, SDT = sonodynamic therapy, PDT = photodynamic therapy, Th1 cell = T helper 1 cell, CBP-12 = 12-mer Clec9a binding peptide.
Applications of membrane-wrapped nanoparticles in cancer immunotherapy
Targeting or modulating the tumor immune microenvironment
The successful induction of anti-tumor adaptive immune response requires three elements: an antigen, an adjuvant, and a conducive immune microenvironment (24). To a great extent, the efficacy of cancer immunotherapy depends on the TME, especially the tumor immune microenvironment (TIME) (25). In CCMs, a wide range of molecules are retained on the surface of the source cancer cell, such as mucoprotein-1, epithelial adhesion moieties, lymphocyte-homing receptors, galectin-3, integrins, and cadherins, that could help CCNPs to escape the immune system (26–29). The use of CCMs introduces various characteristics of cancer cells to the nanocarriers, thus allowing the targeting of homotypic tumors and the development of personalized immunotherapy (30, 31). For example, Jin et al. demonstrated that MDA-MB-231-PLGA NPs disrupted the migration of cancer cells towards fibroblasts and increased the population of cytotoxic T lymphocytes (CTLs) in immune-competent mice (20). Further, Wu et al. showed that Fe3O4@SiO2 magnetic NPs bearing tumor-specific antigens (TSAs) on the surface effectively stimulated natural killer cells by enhancing the expression of surface-activating receptors and boosting anti-tumor function through the secretion of soluble cytotoxic effectors (32).
Combining CCNPs with adjuvant and/or tumor antigen technologies is a promising strategy to induce potent anti-tumor responses. Kim et al. developed a lipocomplex (4T1-liposome adjuvant-KillerRed) that has photocytotoxic and immunomodulatory properties, including dendritic cell (DC) maturation and production of CTLs following effective PDT (33). Similarly, Wang et al. reported an integrative photodynamic immunotherapy approach in which reactive oxygen species (ROS) triggered an anti-tumor immune cascade (34). In this approach, ovalbumin antigen was nano-packaged by establishing an intermolecular disulfide bond network between the antigens, which were employed as photosensitizer nanocarriers and subsequently coated with B16-OVA-CCMs (34).
CCNPs loaded with multiple drugs, such as chemotherapy drugs and ICIs, will have synergistic effects when used in combination therapy. For example, Cheng et al. showed that the intelligent biomimetic nanoplatform AM@DLMSN@CuS/R848 has a strong homogeneous targeting ability to mediate triple-negative breast cancer (TNBC)-targeted delivery of the immune adjuvant R848 and the PD-1/PD-L1 inhibitor AUNP-12 (35). In addition, AM@DLMSN@CuS/R848 could possess high photothermal efficiency that can ablate the primary tumors of TNBC and trigger the rapid release of R848 to produce vaccine-like functions against TNBC recurrence and metastasis (35). In another such study, Chen et al. reported a cocktail therapy based on “nano-targeted cells” (36). In this therapy, PTT was triggered by laser irradiation and helped the release of tumor-associated antigens (TAAs), thereby enhancing docetaxel-mediated immune effects (36). The released TAAs, together with the immune adjuvant R837, drove the maturation of DCs and secretion of cytokines, including TNF-α, IL-6, and IL-12 (36). Furthermore, docetaxel polarized protumoral M2-phenotype tumor-associated macrophages (TAMs) to tumoricidal M1-phenotype TAMs; this alleviated immunosuppression in the TME and was accompanied by a decrease in IL-10 (36). The above processes promoted the infiltration of CTLs for the treatment of distant metastasis (36). Fang et al. developed a multifunctional 2D ultrathin FePSe3@APP@CCM nanosystem with multimodal imaging ability, photothermal features, and PD-1-inhibiting capability (37). In terms of cancer immunotherapy, the nanosystem could trigger DC maturation and subsequent cytokine secretion to activate T cell-related immune responses following PTT (37). At the same time, the nanosystem could achieve immunotherapy with an anti-PD-1 checkpoint inhibitor strategy by blocking the PD-1/PD-L1 pathway (37). Wu et al. reported that biomimetic S-CM-HPAD NPs could not only modulate tumor immune escape by inhibiting myeloid-derived suppressor cell recruitment in tumors but also alter the TME by improving T-cell proliferation and stimulating the secretion of cytokines (38).
The low immunogenicity and TIME of tumors are negative for initiating and forwarding immune cycle, respectively (39, 40). Meng et al. developed an effective delivery system for peptides that target the TIME (41). The system used the tumor-specific enzyme metallomatrix protease 2 (MMP2) to dramatically extend the half-life of the peptides (60 times longer than the control group) (41). For example, prolongation of the half-life of the PD-L1 inhibitory peptide could maintain the reactivation capacity of T cells and inhibit tumor growth under both in vitro and in vivo conditions (41). In a similar strategy, Alsaiari et al. loaded nivolumab in biomimetic metal-organic frameworks (zeolitic imidazolate frameworks or ZIFs) that are known to enrich nivolumab within TIME, enhance the sensitivity of TIME to anti–PD-1 immunotherapy, and systemically activate a specific anti-tumor immune response enabled by the local inhibition of the regulatory T-cells (42). In a noteworthy innovative contribution by Yang et al., they proposed a potential anti-tumor strategy in which a smart biomimetic nanoplatform targets both tumor metabolism and immunity based on ROS-ferroptosis-glycolysis regulation (43).
Hybrid membranes can combine the properties of various cell membranes and have optimized function (44). Hao et al. developed chemoimmunotherapy delivery vehicles based on C6 cell membranes and DC membranes (DCm) to create hybrid membrane-coated docetaxel nanosuspensions (45). For cancer immunotherapy, this CNNP presents antigens to the immune system for efficient downstream immune activation based on the antigen presentation characteristic of DCm (46). Xiong et al. fused a murine-derived ID8 cancer cell-red blood cell hybrid membrane coated indocyanine green-loaded Fe3O4 magnetic NPs to treat ovarian cancer via synergistic photothermal-immunotherapy (46). In this study, the CCNP was characterized by a prolonged circulation lifetime in blood (46). This CCNP, in response to PTT, could induce tumor necrosis and release whole-cell tumor antigens, activate CD8+ cytotoxic T cells and reduce regulatory Foxp3+ T cells, and further enhance antitumor immunotherapy (46).
Combination therapy with immune checkpoint inhibitors, photothermal and sono-photodynamic technologies
In recent years, immunotherapy with ICIs (commonly used anti-CTLA-4 antibodies in the clinic such as ipilimumab; anti-PD-1 antibodies such as nivolumab, pembrolizumab, camrelizumab, and sintilimab; and anti-PD-L1 antibodies such as atezolizumab and durvalumab) has shown promise for long-term survival in a fraction of patients with advanced or metastatic cancer, but off-target effects and drug resistance remain inevitable (47, 48). The reasons for the failure of ICI treatment include a lack of TAAs on the surface of tumor cells that can be recognized by immune cells, a lack of tumor-infiltrating lymphocytes (TILs), an immunosuppressive TME, and the presence of other suppressive immune checkpoints and suppressive cytokines (49, 50). These regimens combining ICIs with other technologies can eliminate negative regulators, overcome the immunosuppressive TME, increase T-cell survival, drive T-cell memory, and activate new immunity in patients without an existing strong immune response (9). Therefore, a combination therapeutic strategy that includes chemotherapy, PDT, PTT, SDT, and others, particularly robust anti-cancer agents loaded on CCNPs, may have immense potential.
As early as 2019, Xie et al. designed the CCNP method, in which mesoporous silica NPs (MSNs) are loaded with glucose oxidase and then wrapped with B16-F10 CCMs to realize starvation therapy together with anti-PD-1 antibody therapy and improved anti-cancer effects (51). In the same year, Wang et al. developed bullet-like Janus magnetic mesoporous organosilica NPs loaded with chlorine e6 and realized the combined application of PDT mediated with immunogenic NPs and magnetic hyperthermia to synergically enhance the anti-metastatic efficacy of anti-CTLA-4 antibody immunotherapy (52). Subsequently, Shao et al. and Zhao et al. reported that the combined treatment strategy of “doxorubicin-loaded or dacarbazine-loaded MONs coated with CCMs and anti-PD-1/PD-L1 antibody” has significant potential as a superior chemo-immunotherapy method (53, 54). Zhao’s team also developed a combined photothermal immunotherapy strategy based on CCM-encapsulated biomimetic black phosphorus quantum dots for tumor-targeted PTT and anti-PD-L1 antibody immunotherapy (55). Apart from PTT and hyperthermia, SDT is another promising site-specific tumor cell killing strategy that has the characteristics of being non-invasive and having high tissue penetration depth (56). Jiang et al. showed that the H2O2 economizer notably alleviates hypoxia-associated limitations in TME and that on-demand oxygen production-assisted sonodynamic immunotherapy can significantly inhibit 4T1 tumors (57). However, due to the complex and dynamic features of cancer occurrence and development, treatment with just one or two strategies may not be sufficient to meet the clinical needs (58). Therefore, combined anti-tumor therapy strategies exploring different therapeutic approaches and related mechanisms of action have broad clinical applications (59). It is worth noting that the serious side effects of combination therapy should not be ignored. Lin et al. designed and constructed a novel multimodal imaging nanoprobe fused with CNNPs loaded with superparamagnetic iron oxide and hematoporphyrin monomethylether, which augmented triple therapy with PTT, SDT, and ICI under the precise guidance of magnetic resonance, photoacoustic imaging, and photothermal imaging (60). In a recent innovative research, the use of cascade cell membrane (CCM and DCm) camouflaging to prepare biomimetic NPs was found to resolve the cross-priming of T cells, and it was applied in immunotherapy with a clinical anti-PD-1 antibody to induce powerful antigen-specific anti-tumor immunity in multiple mouse models of tumors, including B16-OVA, TC-1, and Hepa 1–6 tumors (61).
Application in cancer vaccine therapy
Cancer vaccine therapy, together with ICI therapy and adoptive cellular therapy, are currently three of the most widely used cancer immunotherapy approaches (62–64). Sipuleucel-T (Provenge), a US FDA-approved vaccine for mCRPC in 2010, is the first therapeutic vaccine to be approved for any cancer (65). Cancer vaccines, especially cancer nanovaccines, have demonstrated effective cancer immunotherapy effects by active immune amplification of the tumor-specific T-cell response through the targeted and coded delivery of an antigen and adjuvant (66, 67). CCMs containing TAAs or TSAs have been widely used in the manufacture of cancer vaccines. To a large extent, the camouflage strategy with vaccines can ensure the targeting and effectiveness of tumor antigen presentation. Fontana et al. developed novel porous siliconbased nanovaccines that could enhance the secretion of IFNγ in peripheral blood mononuclear cells but did not induce the secretion of IL4; this oriented the polarization of the newly primed Tcells toward a Th1 cellmediated response (68). Likewise, Cheng et al. produced a cancer vaccine by reconstructing the CCM and using monophosphoryl lipid A as a Toll-like receptor 4 agonist along with egg phosphatidylcholine; this vaccine could significantly enhance immune response and establish immune memory against a 4T1 challenge (69). The above two studies have provided promising candidates for the clinical translation of cancer vaccines.
As combination therapy is known to synergistically enhance anti-tumor effects, several researchers have also explored combination therapy with cancer vaccine. For example, Ye et al. integrated CCM-coated Black phosphorus quantum dots (BPQDs), granulocyte-macrophage colony-stimulating factor (GM-CSF), and lipopolysaccharide into a thermosensitive hydrogel that was subcutaneously injected for sustained delivery of the vaccine; this strategy as found to dramatically improve the survival rate of 4T1 or B16F10 tumor-bearing mice after surgery when it was combined with anti-PD-1 antibody immunotherapy and near-infrared irradiation (70). In terms of combined chemotherapy and PDT, Ni et al. explored a vaccine (a CCM coated with calcium carbonate NPs loaded with low-dose doxorubicin hydrochloride and chlorins e6) that could elicit TAAs and DC recruitment and trigger a subsequent immune response cascade (71). To further improve the efficacy of such nanovaccines, another promising strategy is to modify the surface of such CCNPs to afford them the ability to target DCs. Due to the specific binding of mannose and its receptors on DCs, mannose modification could promote the binding and cellular uptake of NPs by DCs and, consequently, enhance lymph node retention of the cancer vaccine for more effective in vivo vaccination (72). Calcinetin, as a representative of damage-associated molecular patterns, is expressed on the surface of the cancer vaccine; this could induce active uptake by DCs while activating immune memory cells to provide long-term protection (73). One study has shown that a 12-mer Clec9a-binding peptide (CBP-12) that specifically targets Clec9a can enhance antigen uptake and CD8+ T cell responses even in the absence of adjuvants (74). In another such study, Gou et al. developed an engineered CBP-12-expressed CCM-coated cancer vaccine to deliver a stimulator of interferon gene agonists and tumor antigens to Clec9a+ DCs (73). This cancer vaccine exhibited robust anti-tumor effects in both anti-PD-1-responsive and anti-PD-1-resistant tumor models, and when combined with radiotherapy, it had significant synergistic anti-tumor effects (75).
A much simpler and more interesting approach would be to directly modify the atypical design of immune adjuvants and/or aptamers to coat them on CCMs, rather than loading them inside the NPs and then coating those NPs with CCMs. Accordingly, Liu et al. designed a relatively simple strategy for equipping CCMs with functional DNA (including a CpG oligonucleotide, a Toll-like receptor 9 agonist as an immune adjuvant, and a DC-specific intercellular adhesion molecule-3-grabbing nonintegrin-targeted aptamer) as a targeted cancer vaccine that could induce powerful anti-tumor immune responses (76). Further, when this vaccine is combined with anti-PD-1 antibody immunotherapy, it could achieve the optimal therapeutic outcome in combating existing tumors and preventing tumor recurrence via an immune memory effect (76).
Challenges and perspectives
With the rapid development of nanotechnology, synthetic nanocarriers play an increasingly important role in cancer immunotherapy. Stimuli-responsive nanocarriers enable the precise control of drug release through exposure to specific stimuli and exhibit excellent specificity in response to various stimuli. In particular, stimuli-responsive nanocarriers have evolved rapidly from single stimuli-responsive systems to multistimuli-responsive systems (77). However, one of the key challenges is that the size of most nanomaterials ranges from tens to hundreds of nanometers due to the limitations of the preparation methods. Nanocarriers with large size (≥200 nm) are primarily accumulated in extracellular spaces while nanocarriers with small sizes (≤10 nm) can easily be filtered out through the kidneys (78). Even more crucial is that the immune system recognizes and eliminates the majority of nanocarriers as foreign substances. Hence these disadvantages make it difficult for nanocarriers to meet clinical standards. CCNPs possess beneficial characteristics, such as prolonged drug delivery, immunological evasion, homotypic targeting, longer blood circulation, and specific ligand/receptor recognition, which can overcome major barriers in cancer immunotherapy (79).
In the future, the emphasis should be on the recovery of specific immunosuppressive pathways in anti-tumor processes, rather than merely boosting the broad and untargeted systemic immune response (1). Specifically, the following research pathways would be beneficial: determining whether TIME is caused by the tumor, focusing on immunosuppression of the TME, and identifying new targets in the major functional pathways (1). Existing experimental CCNPs can simulate part of the TME, but cannot fully adapt to the complex and changeable TIME, especially in the human body. This is one of the key reasons for primary and secondary drug resistance in various types of immunotherapy and even in immunotherapy combination regimens. Hybrid membrane nanocarriers may have some advantages because they can combine the properties of a variety of cell membranes (e.g., RBCs, WBCs, and platelets including DCs and T cells) and optimize their function. Of particular concern, however, is that more complex CCNPs imply greater immunogenicity and toxicity, which may cause unwanted immune responses. In addition, the concern about injecting cancer cell-derived substances into the body is valid, and healthy people may be reluctant to accept this technology as a preventive vaccine in particular. In addition to providing more medical education to the public, technical optimizations and rigorous procedures must be developed to ensure the purity of the membrane wrapping, that is, to ensure that it does not contain any molecules that may promote cancer growth. Another important dilemma to consider for clinical use is that this approach may be appropriate only for patients already diagnosed with cancers that have been surgically removed, as the required amount of cancer cells can be obtained from the resected tissue (80). Some other challenges in isolating primary cancer cells from biopsy samples are the procedures for eliminating the extracellular matrix and the conditions required to grow it in a way that prevents significant changes in the antigen profiles (81, 82). In addition, the need for large-scale cell culture and industrialization are also key challenges that may require further breakthroughs in biomedical technology.
In due course, to translate this technology to the clinic, the following points may be urgently needed breakthroughs. First of all, the complexity of various synthetic nanocarriers hinder their large-scale uses, we should always consider simplifying in the design and then screening several nanocarriers with more promising clinical application and apply them to clinical trials to verify their effectiveness and safety. Secondly, the powerful advantage of “mimicking nature” is that cell membrane materials have the advantage of biologically active vesicles. A similar and standardized manufacturing process will be required in order to develop standardized or quantified bioactive membrane-coated nanocarriers. Finally, aside from the technical difficulties, the concept of prioritizing treatment for cancer patients in well-designed clinical trials should be widely understood and practiced throughout society. In the future of translational medicine, we hope that it will be possible to obtain CCMs from primary or metastatic tumor lesions and develop personalized CCNPs for cancer immunotherapy.
Conclusions
This review has highlighted the current major developments in CCNPs for cancer immunotherapy. CCNPs have enormous potential as powerful vehicles to enhance cancer therapy, but there are immense challenges in translating their effects in vitro to the clinical setting. The future of this field lies in solving this issue and providing tailormade, personalized treatment to individual patients based on CCNPs and CCNPs combined with other effective therapies. While this objective is challenge, it will continue to drive research around CCNPs.
Author contributions
PS and JR contributed to the conception and design this study. QJ, RC, FY, CY, QL, SX and WW conducted the initial literature review and selected the studies for inclusion. QJ and MX wrote the initial draft of the manuscript. YJ, PS, and JR revised the draft and approved the final submission of the study. All authors contributed to the article and approved the submitted version.
Funding
This work was supported by fund from the National Natural Science Foundation of China (No.82100161 and No.81874173) and the Natural Science Foundation of Zhejiang Province (No. LQ22H080004 and No. LY22H160019).
Conflict of interest
The authors declare that the research was conducted in the absence of any commercial or financial relationships that could be construed as a potential conflict of interest.
Publisher’s note
All claims expressed in this article are solely those of the authors and do not necessarily represent those of their affiliated organizations, or those of the publisher, the editors and the reviewers. Any product that may be evaluated in this article, or claim that may be made by its manufacturer, is not guaranteed or endorsed by the publisher.
Abbreviations
CAR-T, chimeric antigen receptor T-cell immunotherapy; TME, tumor microenvironment; TGF, transforming growth factor; mCRPC, metastatic castration-resistant prostate cancer; ICIs, immune checkpoint inhibitors; PD-L1/PD-1, programmed death-ligand 1/programmed death-1; CTLA-4, cytotoxic T-lymphocyte-associated protein 4; NPs, nanoparticles; PLGA, polylactic glycolic acid; PDT, photodynamic therapy; PTT, photothermal therapy; SDT, sonodynamic therapy; CCMs, cancer cell membranes; CCNPs, cancer cell membrane-wrapped nanoparticles; TIME, tumor immune microenvironment; CTLs, cytotoxic T lymphocytes; TSAs, tumor-specific antigens; DC, dendritic cell; ROS, reactive oxygen species; TNBC, triple-negative breast cancer; TAAs, tumor-associated antigens; TAMs, tumor-associated macrophages; MMP2, metallomatrix protease 2; DCm, dendritic cell membrane; TILs, tumor-infiltrating lymphocytes; MSNs, mesoporous silica NPs; BPQDs, Black phosphorus quantum dots; GM-CSF, granulocyte macrophage colony-stimulating factor; CBP-12, 12-mer Clec9a-binding peptide.
References
1. Tan S, Li D, Zhu X. Cancer immunotherapy: Pros, cons and beyond. BioMed Pharmacother (2020) 124:109821. doi: 10.1016/j.biopha.2020.109821
2. Myers RM, Li Y, Barz LA, Barrett DM, Teachey DT, Callahan C, et al. Humanized CD19-targeted chimeric antigen receptor (CAR) T cells in CAR-naive and CAR-exposed children and young adults with relapsed or refractory acute lymphoblastic leukemia. J Clin Oncol (2021) 39(27):3044–55. doi: 10.1200/JCO.20.03458
3. Xia C, Dong X, Li H, Cao M, Sun D, He S, et al. Cancer statistics in China and united states, 2022: profiles, trends, and determinants. Chin Med J (Engl) (2022) 135(5):584–90. doi: 10.1097/CM9.0000000000002108
4. Narayan V, Barber-Rotenberg JS, Jung IY, Lacey SF, Rech AJ, Davis MM, et al. PSMA-targeting TGFβ-insensitive armored CAR t cells in metastatic castration-resistant prostate cancer: a phase 1 trial. Nat Med (2022) 28(4):724–34. doi: 10.1038/s41591-022-01726-1
5. Evgin L, Kottke T, Tonne J, Thompson J, Huff AL, van Vloten J, et al. Oncolytic virus-mediated expansion of dual-specific CAR T cells improves efficacy against solid tumors in mice. Sci Transl Med (2022) 14(640):eabn2231. doi: 10.1126/scitranslmed.abn2231
6. Upadhaya S, Neftelinov ST, Hodge J, Campbell J. Challenges and opportunities in the PD1/PDL1 inhibitor clinical trial landscape. Nat Rev Drug Discovery (2022) 21(7):482–3. doi: 10.1038/d41573-022-00030-4
7. Liu J, Fu M, Wang M, Wan D, Wei Y, Wei X. Cancer vaccines as promising immuno-therapeutics: platforms and current progress. J Hematol Oncol (2022) 15(1):28. doi: 10.1186/s13045-022-01247-x
8. Saxena M, van der Burg SH, Melief CJM, Bhardwaj N. Therapeutic cancer vaccines. Nat Rev Cancer (2021) 21(6):360–78. doi: 10.1038/s41568-021-00346-0
9. Hegde PS, Chen DS. Top 10 challenges in cancer immunotherapy. Immunity (2020) 52(1):17–35. doi: 10.1016/j.immuni.2019.12.011
10. Vijayan V, Uthaman S, Park IK. Cell membrane coated nanoparticles: An emerging biomimetic nanoplatform for targeted bioimaging and therapy. Adv Exp Med Biol (2018) 1064:45–59. doi: 10.1007/978-981-13-0445-3_3
11. Virlan MJR, Miricescu D, Radulescu R, Sabliov CM, Totan A, Calenic B, et al. Organic nanomaterials and their applications in the treatment of oral diseases. Molecules (2016) 21:207. doi: 10.3390/molecules21020207
12. Lei W, Yang C, Wu Y, Ru G, He X, Tong X, et al. Nanocarriers surface engineered with cell membranes for cancer targeted chemotherapy. J Nanobiotechnol (2022) 20(1):45. doi: 10.1186/s12951-022-01251-w
13. Anselmo AC. Mitragotri s. A Rev Clin Transl Inorg Nanopart AAPS J (2015) 17:1041–54. doi: 10.1208/s12248-015-9780-2
14. Fan W, Yung B, Huang P, Chen X. Nanotechnology for multimodal synergistic cancer therapy. Chem Rev (2017) 117(22):13566–638. doi: 10.1021/acs.chemrev.7b00258
15. Vijayan V, Uthaman S, Park IK. Cell membrane-camouflaged nanoparticles: A promising biomimetic strategy for cancer theragnostics. Polym (Basel) (2018) 10(9):983. doi: 10.3390/polym10090983
16. Harris JC, Scully MA, Day ES. Cancer cell membrane-coated nanoparticles for cancer management. Cancers (Basel) (2019) 11(12):1836. doi: 10.3390/cancers11121836
17. Shi J, Kantoff PW, Wooster R, Farokhzad OC. Cancer nanomedicine: progress, challenges and opportunities. Nat Rev Cancer (2017) 17(1):20–37. doi: 10.1038/nrc.2016.108
18. Sun H, Su J, Meng Q, Yin Q, Chen L, Gu W, et al. Cancer-Cell-Biomimetic nanoparticles for targeted therapy of homotypic tumors. Adv Mater (2016) 28(43):9581–8. doi: 10.1002/adma.201602173
19. Sun H, Su J, Meng Q, Yin Q, Chen L, Gu W, et al. Cancer cell membrane-coated gold nanocages with hyperthermia-triggered drug release and homotypic target inhibit growth and metastasis of breast cancer. Adv Funct Mater (2017) 27(3):1604300. doi: 10.1002/adfm.201604300
20. Jin J, Krishnamachary B, Barnett JD, Chatterjee S, Chang D, Mironchik Y, et al. Human cancer cell membrane-coated biomimetic nanoparticles reduce fibroblast-mediated invasion and metastasis and induce T-cells. ACS Appl Mater Interfaces (2019) 11(8):7850–61. doi: 10.1021/acsami.8b22309
21. Zhu JY, Zheng DW, Zhang MK, Yu WY, Qiu WX, Hu JJ, et al. Preferential cancer cell self-recognition and tumor self-targeting by coating nanoparticles with homotypic cancer cell membranes. Nano Lett (2016) 16(9):5895–901. doi: 10.1021/acs.nanolett.6b02786
22. Fang RH, Hu CM, Luk BT, Gao W, Copp JA, Tai Y, et al. Cancer cell membrane-coated nanoparticles for anticancer vaccination and drug delivery. Nano Lett (2014) 14(4):2181–8. doi: 10.1021/nl500618u
23. Fang RH, Kroll AV, Gao W, Zhang L. Cell membrane coating nanotechnology. Adv Mater (2018) 30(23):e1706759. doi: 10.1002/adma.201706759
24. Jiang J, Mei J, Yi S, Feng C, Ma Y, Liu Y, et al. Tumor associated macrophage and microbe: The potential targets of tumor vaccine delivery. Adv Drug Delivery Rev (2022) 180:114046. doi: 10.1016/j.addr.2021.114046
25. Ma J, Huang L, Hu D, Zeng S, Han Y, Shen H. The role of the tumor microbe microenvironment in the tumor immune microenvironment: bystander, activator, or inhibitor? J Exp Clin Cancer Res (2021) 40(1):327. doi: 10.1186/s13046-021-02128-w
26. Nath S, Mukherjee P. MUC1: a multifaceted oncoprotein with a key role in cancer progression. Trends Mol Med (2014) 20(6):332–42. doi: 10.1016/j.molmed.2014.02.007
27. Gordon-Alonso M, Hirsch T, Wildmann C, van der Bruggen P. Galectin-3 captures interferon-gamma in the tumor matrix reducing chemokine gradient production and T-cell tumor infiltration. Nat Commun (2017) 8(1):793. doi: 10.1038/s41467-017-00925-6
28. Bose RJ, Paulmurugan R, Moon J, Lee SH, Park H. Cell membrane-coated nanocarriers: the emerging targeted delivery system for cancer theranostics. Drug Discovery Today (2018) 23(4):891–9. doi: 10.1016/j.drudis.2018.02.001
29. Sökeland G, Schumacher U. The functional role of integrins during intra- and extravasation within the metastatic cascade. Mol Cancer (2019) 18(1):12. doi: 10.1186/s12943-018-0937-3
30. Nie D, Dai Z, Li J, Yang Y, Xi Z, Wang J, et al. Cancer-Cell-Membrane-Coated nanoparticles with a yolk-shell structure augment cancer chemotherapy. Nano Lett (2020) 20(2):936–46. doi: 10.1021/acs.nanolett.9b03817
31. Rao L, Yu G, Meng Q, Bu L, Tian R, Lin L, et al. Cancer cell membrane-coated nanoparticles for personalized therapy in patient-derived xenograft models. Adv Funct Mater (2019) 29(51):1905671. doi: 10.1002/adfm.201905671
32. Wu D, Shou X, Zhang Y, Li Z, Wu G, Wu D, et al. Cell membrane-encapsulated magnetic nanoparticles for enhancing natural killer cell-mediated cancer immunotherapy. Nanomedicine (2021) 32:102333. doi: 10.1016/j.nano.2020.102333
33. Kim HY, Kang M, Choo YW, Go SH, Kwon SP, Song SY, et al. Immunomodulatory lipocomplex functionalized with photosensitizer-embedded cancer cell membrane inhibits tumor growth and metastasis. Nano Lett (2019) 19(8):5185–93. doi: 10.1021/acs.nanolett.9b01571
34. Wang H, Wang K, He L, Liu Y, Dong H, Li Y. Engineering antigen as photosensitiser nanocarrier to facilitate ROS triggered immune cascade for photodynamic immunotherapy. Biomaterials (2020) 244:119964. doi: 10.1016/j.biomaterials.2020.119964
35. Cheng Y, Chen Q, Guo Z, Li M, Yang X, Wan G, et al. An intelligent biomimetic nanoplatform for holistic treatment of metastatic triple-negative breast cancer via photothermal ablation and immune remodeling. ACS Nano (2020) 14(11):15161–81. doi: 10.1021/acsnano.0c05392
36. Chen Q, Zhang L, Li L, Tan M, Liu W, Liu S, et al. Cancer cell membrane-coated nanoparticles for bimodal imaging-guided photothermal therapy and docetaxel-enhanced immunotherapy against cancer. J Nanobiotechnol (2021) 19(1):449. doi: 10.1186/s12951-021-01202-x
37. Fang X, Wu X, Li Z, Jiang L, Lo WS, Chen G, et al. Biomimetic anti-PD-1 peptide-loaded 2D FePSe(3) nanosheets for efficient photothermal and enhanced immune therapy with multimodal MR/PA/Thermal imaging. Adv Sci (Weinh) (2021) 8(2):2003041. doi: 10.1002/advs.202003041
38. Wu M, Liu X, Bai H, Lai L, Chen Q, Huang G, et al. Surface-layer protein-enhanced immunotherapy based on cell membrane-coated nanoparticles for the effective inhibition of tumor growth and metastasis. ACS Appl Mater Interfaces (2019) 11(10):9850–9. doi: 10.1021/acsami.9b00294
39. O’Donnell JS, Teng M, Smyth MJ. Cancer immunoediting and resistance to T cell-based immunotherapy. Nat Rev Clin Oncol (2019) 16(3):151–67. doi: 10.1038/s41571-018-0142-8
40. Anderson KG, Stromnes IM, Greenberg PD. Obstacles posed by the tumor microenvironment to T cell activity: A case for synergistic therapies. Cancer Cell (2017) 31(3):311–25. doi: 10.1016/j.ccell.2017.02.008
41. Meng X, Wang J, Zhou J, Tian Q, Qie B, Zhou G, et al. Tumor cell membrane-based peptide delivery system targeting the tumor microenvironment for cancer immunotherapy and diagnosis. Acta Biomater (2021) 127:266–75. doi: 10.1016/j.actbio.2021.03.056
42. Alsaiari SK, Qutub SS, Sun S, Baslyman W, Aldehaiman M, Alyami M, et al. Sustained and targeted delivery of checkpoint inhibitors by metal-organic frameworks for cancer immunotherapy. Sci Adv (2021) 7(4):eabe7174. doi: 10.1126/sciadv.abe7174
43. Yang J, Ma S, Xu R, Wei Y, Zhang J, Zuo T, et al. Smart biomimetic metal organic frameworks based on ROS-ferroptosis-glycolysis regulation for enhanced tumor chemo-immunotherapy. J Control Release (2021) 334:21–33. doi: 10.1016/j.jconrel.2021.04.013
44. Ren X, Zheng R, Fang X, Wang X, Zhang X, Yang W, et al. Red blood cell membrane camouflaged magnetic nanoclusters for imaging-guided photothermal therapy. Biomaterials (2016) 92:13–24. doi: 10.1016/j.biomaterials.2016.03.026
45. Hao W, Cui Y, Fan Y, Chen M, Yang G, Wang Y, et al. Hybrid membrane-coated nanosuspensions for multi-modal anti-glioma therapy via drug and antigen delivery. J Nanobiotechnol (2021) 19(1):378. doi: 10.1186/s12951-021-01110-0
46. Xiong J, Wu M, Chen J, Liu Y, Chen Y, Fan G, et al. Cancer-erythrocyte hybrid membrane-camouflaged magnetic nanoparticles with enhanced photothermal-immunotherapy for ovarian cancer. ACS Nano (2021) 15(12):19756–70. doi: 10.1021/acsnano.1c07180
47. Sharma P, Allison JP. The future of immune checkpoint therapy. Science (2015) 348(6230):56–61. doi: 10.1126/science.aaa8172
48. Sharma P, Allison JP. Immune checkpoint targeting in cancer therapy: toward combination strategies with curative potential. Cell (2015) 161(2):205–14. doi: 10.1016/j.cell.2015.03.030
49. Bates JP, Derakhshandeh R, Jones L, Webb TJ. Mechanisms of immune evasion in breast cancer. BMC Cancer (2018) 18(1):556. doi: 10.1186/s12885-018-4441-3
50. Liu Z, Li M, Jiang Z. Wang X. A Compr Immunol Portrait Triple-Negative Breast Cancer Transl Oncol (2018) 11(2):311–29. doi: 10.1016/j.tranon.2018.01.011
51. Xie W, Deng WW, Zan M, Rao L, Yu GT, Zhu DM, et al. Cancer cell membrane camouflaged nanoparticles to realize starvation therapy together with checkpoint blockades for enhancing cancer therapy. ACS Nano (2019) 13(3):2849–57. doi: 10.1021/acsnano.8b03788
52. Wang Z, Zhang F, Shao D, Chang Z, Wang L, Hu H, et al. Janus nanobullets combine photodynamic therapy and magnetic hyperthermia to potentiate synergetic anti-metastatic immunotherapy. Adv Sci (Weinh) (2019) 6(22):1901690. doi: 10.1002/advs.201901690
53. Shao D, Zhang F, Chen F, Zheng X, Hu H, Yang C, et al. Biomimetic diselenide-bridged mesoporous organosilica nanoparticles as an X-ray-Responsive biodegradable carrier for chemo-immunotherapy. Adv Mater (2020) 32(50):e2004385. doi: 10.1002/adma.202004385
54. Zhao P, Qiu L, Zhou S, Li L, Qian Z, Zhang H. Cancer cell membrane camouflaged mesoporous silica nanoparticles combined with immune checkpoint blockade for regulating tumor microenvironment and enhancing antitumor therapy. Int J Nanomed (2021) 16:2107–21. doi: 10.2147/IJN.S295565
55. Zhao P, Xu Y, Ji W, Zhou S, Li L, Qiu L, et al. Biomimetic black phosphorus quantum dots-based photothermal therapy combined with anti-PD-L1 treatment inhibits recurrence and metastasis in triple-negative breast cancer. J Nanobiotechnol (2021) 19(1):181. doi: 10.1186/s12951-021-00932-2
56. Yue W, Chen L, Yu L, Zhou B, Yin H, Ren W, et al. Checkpoint blockade and nanosonosensitizer-augmented noninvasive sonodynamic therapy combination reduces tumour growth and metastases in mice. Nat Commun (2019) 10(1):2025. doi: 10.1038/s41467-019-09760-3
57. Jiang Q, Qiao B, Lin X, Cao J, Zhang N, Guo H, et al. A hydrogen peroxide economizer for on-demand oxygen production-assisted robust sonodynamic immunotherapy. Theranostics (2022) 12(1):59–75. doi: 10.7150/thno.64862
58. Hu D, Chen L, Qu Y, Peng J, Chu B, Shi K, et al. Oxygen-generating hybrid polymeric nanoparticles with encapsulated doxorubicin and chlorin e6 for trimodal imaging-guided combined chemo-photodynamic therapy. Theranostics (2018) 8(6):1558–74. doi: 10.7150/thno.22989
59. Feng Q, Zhang Y, Zhang W, Shan X, Yuan Y, Zhang H, et al. Tumor-targeted and multi-stimuli responsive drug delivery system for near-infrared light induced chemo-phototherapy and photoacoustic tomography. Acta Biomater (2016) 38:129–42. doi: 10.1016/j.actbio.2016.04.024
60. Lin X, He T, Tang R, Li Q, Wu N, Zhou Y, et al. Biomimetic nanoprobe-augmented triple therapy with photothermal, sonodynamic and checkpoint blockade inhibits tumor growth and metastasis. J Nanobiotechnol (2022) 20(1):80. doi: 10.1186/s12951-022-01287-y
61. Chen F, Geng Z, Wang L, Zhou Y, Liu J. Biomimetic nanoparticles enabled by cascade cell membrane coating for direct cross-priming of T cells. Small (2022) 18(3):e2104402. doi: 10.1002/smll.202104402
62. Restifo NP, Dudley ME, Rosenberg SA. Adoptive immunotherapy for cancer: harnessing the T cell response. Nat Rev Immunol (2012) 12(4):269–81. doi: 10.1038/nri3191
63. Byun DJ, Wolchok JD, Rosenberg LM, Girotra M. Cancer immunotherapy - immune checkpoint blockade and associated endocrinopathies. Nat Rev Endocrinol (2017) 13(4):195–207. doi: 10.1038/nrendo.2016.205
64. Hu Z, Ott PA, Wu CJ. Towards personalized, tumour-specific, therapeutic vaccines for cancer. Nat Rev Immunol (2018) 18(3):168–82. doi: 10.1038/nri.2017.131
65. Handy CE, Antonarakis ES. Sipuleucel-T for the treatment of prostate cancer: novel insights and future directions. Future Oncol (2018) 14(10):907–17. doi: 10.2217/fon-2017-0531
66. Wculek SK, Cueto FJ, Mujal AM, Melero I, Krummel MF, Sancho D. Dendritic cells in cancer immunology and immunotherapy. Nat Rev Immunol (2020) 20(1):7–24. doi: 10.1038/s41577-019-0210-z
67. Irvine DJ, Dane EL. Enhancing cancer immunotherapy with nanomedicine. Nat Rev Immunol (2020) 20(5):321–34. doi: 10.1038/s41577-019-0269-6
68. Fontana F, Shahbazi MA, Liu D, Zhang H, Mäkilä E, Salonen J, et al. Multistaged nanovaccines based on porous Silicon@Acetalated Dextran@Cancer cell membrane for cancer immunotherapy. Adv Mater (2017) 29(7). doi: 10.1002/adma.201603239
69. Cheng R, Fontana F, Xiao J, Liu Z, Figueiredo P, Shahbazi MA, et al. Recombination monophosphoryl lipid a-derived vacosome for the development of preventive cancer vaccines. ACS Appl Mater Interfaces (2020) 12(40):44554–62. doi: 10.1021/acsami.0c15057
70. Ye X, Liang X, Chen Q, Miao Q, Chen X, Zhang X, et al. Surgical tumor-derived personalized photothermal vaccine formulation for cancer immunotherapy. ACS Nano (2019) 13(3):2956–68. doi: 10.1021/acsnano.8b07371
71. Ni J, Song J, Wang B, Hua H, Zhu H, Guo X, et al. Dendritic cell vaccine for the effective immunotherapy of breast cancer. BioMed Pharmacother (2020) 126:110046. doi: 10.1016/j.biopha.2020.110046
72. Yang R, Xu J, Xu L, Sun X, Chen Q, Zhao Y, et al. Cancer cell membrane-coated adjuvant nanoparticles with mannose modification for effective anticancer vaccination. ACS Nano (2018) 12(6):5121–9. doi: 10.1021/acsnano.7b09041
73. Xiong X, Zhao J, Pan J, Liu C, Guo X, Zhou S. Personalized nanovaccine coated with calcinetin-expressed cancer cell membrane antigen for cancer immunotherapy. Nano Lett (2021) 21(19):8418–25. doi: 10.1021/acs.nanolett.1c03004
74. Gou S, Wang S, Liu W, Chen G, Zhang D, Du J, et al. Adjuvant-free peptide vaccine targeting Clec9a on dendritic cells can induce robust antitumor immune response through Syk/IL-21 axis. Theranostics (2021) 11(15):7308–21. doi: 10.7150/thno.56406
75. Gou S, Liu W, Wang S, Chen G, Chen Z, Qiu L, et al. Engineered nanovaccine targeting Clec9a(+) dendritic cells remarkably enhances the cancer immunotherapy effects of STING agonist. Nano Lett (2021) 21(23):9939–50. doi: 10.1021/acs.nanolett.1c03243
76. Liu B, Yang Y, Chao Y, Xiao Z, Xu J, Wang C, et al. Equipping cancer cell membrane vesicles with functional DNA as a targeted vaccine for cancer immunotherapy. Nano Lett (2021) 21(22):9410–8. doi: 10.1021/acs.nanolett.1c02582
77. Cheng H, Fan X, Ye E, Chen H, Yang J, Ke L, et al. Dual tumor microenvironment remodeling by glucose-contained radical copolymer for MRI-guided photoimmunotherapy. Adv Mater (2022) 34(25):e2107674. doi: 10.1002/adma.202107674
78. Tian M, Xin X, Wu R, Guan W, Zhou W. Advances in intelligent-responsive nanocarriers for cancer therapy. Pharmacol Res (2022) 178:106184. doi: 10.1016/j.phrs.2022.106184
79. von Roemeling C, Jiang W, Chan CK, Weissman IL, Kim BYS. Breaking down the barriers to precision cancer nanomedicine. Trends Biotechnol (2017) 35(2):159–71. doi: 10.1016/j.tibtech.2016.07.006
80. González FE, Gleisner A, Falcón-Beas F, Osorio F, López MN, Salazar-Onfray F. Tumor cell lysates as immunogenic sources for cancer vaccine design. Hum Vaccin Immunother (2014) 10(11):3261–9. doi: 10.4161/21645515.2014.982996
81. Mitra A, Mishra L, Li S. Technologies for deriving primary tumor cells for use in personalized cancer therapy. Trends Biotechnol (2013) 31(6):347–54. doi: 10.1016/j.tibtech.2013.03.006
Keywords: cancer cell membrane, membrane-wrapped, nanoparticle, drug delivery, immunotherapy, nanovaccine
Citation: Jiang Q, Xie M, Chen R, Yan F, Ye C, Li Q, Xu S, Wu W, Jia Y, Shen P and Ruan J (2022) Cancer cell membrane-wrapped nanoparticles for cancer immunotherapy: A review of current developments. Front. Immunol. 13:973601. doi: 10.3389/fimmu.2022.973601
Received: 20 June 2022; Accepted: 11 August 2022;
Published: 29 August 2022.
Edited by:
Cheryl Lai-Lai Chiang, Centre Hospitalier Universitaire Vaudois (CHUV), SwitzerlandReviewed by:
Hongwei Cheng, Xiamen University, ChinaXiaojun Xia, Sun Yat-sen University Cancer Center (SYSUCC), China
Copyright © 2022 Jiang, Xie, Chen, Yan, Ye, Li, Xu, Wu, Jia, Shen and Ruan. This is an open-access article distributed under the terms of the Creative Commons Attribution License (CC BY). The use, distribution or reproduction in other forums is permitted, provided the original author(s) and the copyright owner(s) are credited and that the original publication in this journal is cited, in accordance with accepted academic practice. No use, distribution or reproduction is permitted which does not comply with these terms.
*Correspondence: Yunlu Jia, jiayunlu@zju.edu.cn; Peng Shen, shenp@zju.edu.cn; Jian Ruan, software233@zju.edu.cn
†These authors have contributed equally to this work and share first authorship