- 1Department of Microbiology, School of Basic Medical Sciences, Anhui Medical University, Hefei, China
- 2Department of Cell Biology, School of Life Sciences, Anhui Medical University, Hefei, China
- 3Department of Immunology, School of Basic Medical Sciences, Anhui Medical University, Hefei, China
Vector-borne diseases have high morbidity and mortality and are major health threats worldwide. γδT cells represent a small but essential subpopulation of T cells. They reside in most human tissues and exert important functions in both natural and adaptive immune responses. Emerging evidence have shown that the activation and expansion of γδT cells invoked by pathogens play a diversified role in the regulation of host-pathogen interactions and disease progression. A better understanding of such a role for γδT cells may contribute significantly to developing novel preventative and therapeutic strategies. Herein, we summarize recent exciting findings in the field, with a focus on the role of γδT cells in the infection of vector-borne pathogens.
Introduction
Vector-borne emerging and re-emerging infectious diseases are major public health problems worldwide, accounting for more than one sixth of all infectious diseases (1, 2). They are caused by pathogens carried and transmitted by vectors, such as mosquitos, ticks, fleas, flies, lice, snails, and triatomine bugs (Figure 1).
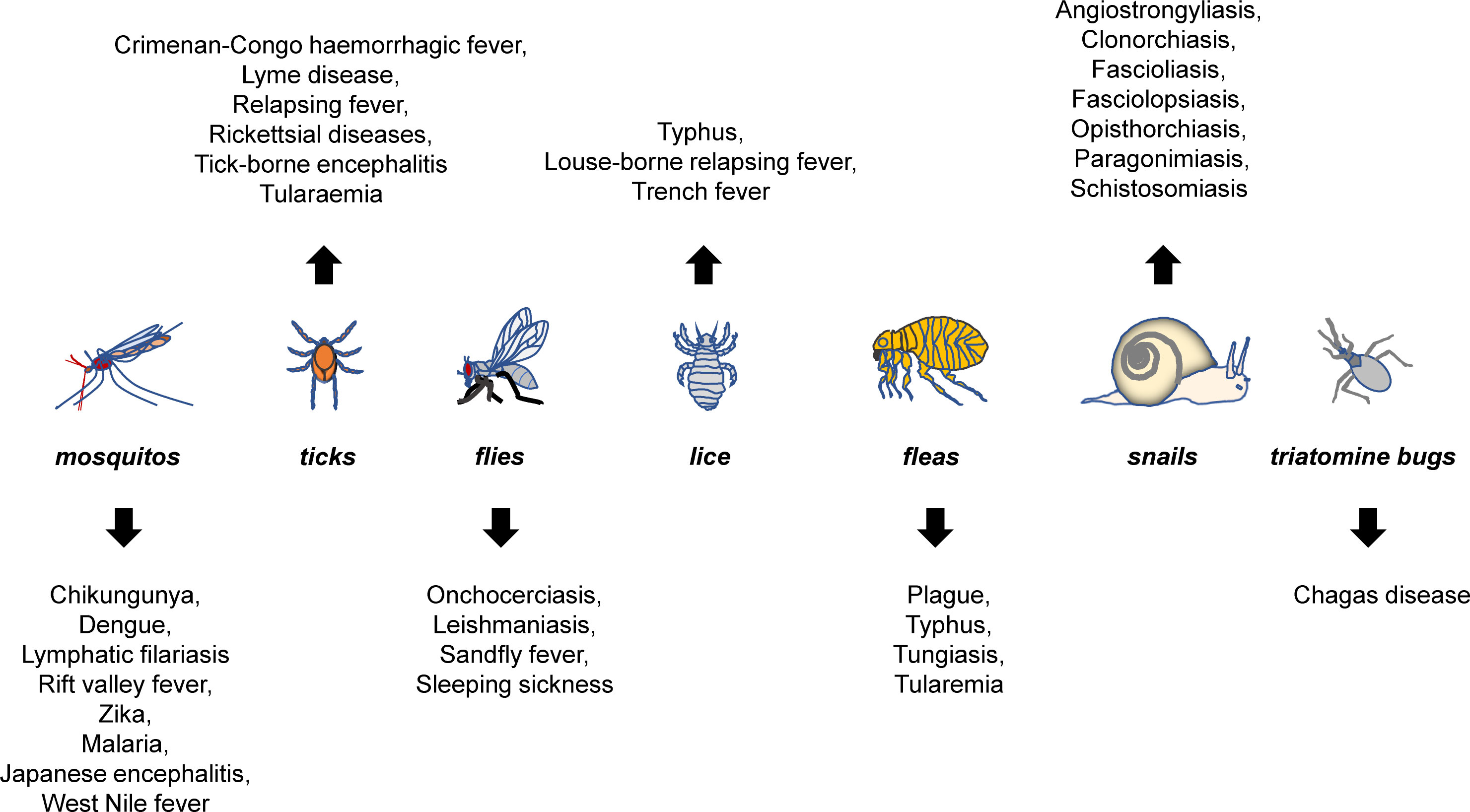
Figure 1 Major vector-borne diseases and their vectors. Mosquitos, ticks, fleas, flies, lice, snails, and triatomine bugs are best-characterized vectors that can carry pathogens for a variety of diseases. The listed are representative rather than a complete list of major vector-borne diseases that are known to be transmitted by each of the vectors.
WHO recently announced the spread of the vector-borne pathogens, primarily including parasites, viruses and bacteria (https://www.who.int/zh/news-room/fact-sheets/detail/vector-borne-diseases). Specifically, the parasites included were lymphatic filariasis (mosquito), schistosoma (aquatic snail), onchocodium filariasis (black fly) and trypanosoma (triatomine bug, tsetse fly) and etc; viruses include mosquito-borne chikungunya fever, dengue, lymphatic filariasis, Rift Valley fever, yellow fever, Zika, and tick-borne Crimean-Congo hemorrhagic fever virus, borrelia burgdorferi, tick-borne encephalitis virus, and etc.; and bacteria mainly include Typhoid, Coxella bainiensis, spot fever rickettsia, and etc. (Figure 1).
Vector-borne diseases may not be directly disseminated between humans. Under certain circumstances, they can be transmitted to different hosts through the bite by pathogen-infected vectors (3, 4). As emerging infectious diseases (including vector-borne diseases) have certain relationships with social and economic development (5), a better understanding of the emerging and recurrent infectious diseases, especially how these diseases are transmitted, has profound significance for both human health and social development.
In response to pathogen invasion, human immune system acts as an advanced structural and functional architecture, in which all components (e.g. immune organs and cells, inflammatory factors, humoral factors, cytokines and chemokines) are highly orchestrated towards eliminating the invaded pathogen (6). During the invasion, both innate and adaptive immune responses can be triggered. Compared to innate immunity, adaptive immunity utilizes antigen and antibody specificity to eliminate the pathogen, thereby maintaining a steady state of the host and creating immunological memory for combating potential re-invasion of the same pathogen.
Different leukocytes, for example B and T cells, are known to play differential roles during these processes (7). T cells can be categorized to conventional T cells (αβT cells) and unconventional T cells (γδT cells) according to the types of their cell surface antigen receptors (8). Although αβT and γδT originate (differentiate) from the same thymic precursors, there are huge differences of biological functions and structures between the two types of T cells. The αβT cell receptors are expressed by ~95% of the T cells in the spleen, lymph nodes, and circulation system, and by ~60-70% of T cells in the peripheral blood. They have α and β chains and exhibit MHC restriction during the recognition of antigens (9).
On the contrary, the γδT cells express γ and δ chains of T cell receptors, account for ~5-15% T cells in the peripheral blood, and do not have MHC restriction during the recognition of antigens (10, 11). At the initial stage during the invasion of pathogens, γδT cells apparently exert innate immunity functions (6), so that they can rapidly respond by recognizing some common antigen components expressed by the invading pathogens, including glycolipids, glycoproteins, and mycobacterial derivatives (12). Although γδT cells have been known to act primarily in innate immunity, more and more findings have shown that these cells also exert fundamental functions in adaptive immune responses, for instance, by secreting cytokines and presenting antigens. Therefore, they have been considered as a bridge connecting innate immunity and adaptive immunity. However, the biological functions of γδT cells are not entirely dependent on HLA recognition mechanism.
γδT accounts for only a small part of the T cell population and is widely distributed in different parts of the human body, such as skin and intestinal tract (7). Human γδT cells are mainly categorized by the usage of δ chain, whereas mouse γδT cells are often categorized by the usage of γ chains. As such, human γδT cells can be divided into γδ1, γδ2, and γδ3 T cells (13), with their distribution and function varying from each other (14). γδ1 T cells are mainly distributed in the mucosal epithelium and play an important role in cell infection by listeria and cytomegalovirus. γδ2 T cells are relatively high in peripheral blood γδT cells and show strong immune response to mycobacterium and influenza virus (15, 16). They destroy pathogens or infected cells by interacting rapidly with them (17, 18). γδ3 T cells, which account for a small proportion of γδT cell and are abundant in the liver, act during chronic viral infection (19).
The common and more harmful vector-borne diseases include dengue virus, Japanese encephalitis, Lyme disease and malaria. γδT cells play a key role in the host immune responses to the invasion of arbo-borne pathogens. More and more studies have shown that γδT cells are critical for antiviral and immunomodulatory activities in the first stage of arbo-borne pathogen infection. They are activated and participate in innate immune responses by producing cytokines associated with appropriate T-assisted responses during the early stages of microbial infection, either intracellular or extracellular (20). In addition to directly fighting against invading pathogens, γδT cells can also respond by recruiting other natural immune cells such as neutrophils and macrophages (21).
Infectious diseases are caused mainly by pathogenic microorganisms such as bacteria, viruses and parasites. γδT cells play important roles in responding to the invasion of common pathogens. Zhao and colleagues have summarized the role played by γδT cells in host responses to mycobacterium tuberculosis, Listeria monocytogenes, influenza viruses, HIV, EBV, and HBV (13). However, little is known about the effects of vectors on host γδT cells. Emerging vector-borne infectious diseases are an important part of emerging infectious diseases and have been in an intensified form globally. Many social and natural factors, including environmental pollution and modern transportation and logistics, make it more convenient for vectors to transmit arboreal pathogens.
Traditionally, many vector-borne diseases can be treated by antibiotics-based therapeutics. However, at least partly due to the antibiotics abuse in clinic, a variety of pathogens have developed resistance to common antibiotics, leading to poor clinical outcomes when using antibiotics to treat infected patients (22). To address this problem, it is critical for developing novel therapeutic approaches. In the past decades, scientists and clinicians have focused on the roles of conventional T cells-mediated immune responses during the pathogen infection. Notably, more and more evidence has uncovered previously-unrecognized key roles of unconventional T cells in this process. Therefore, we feel it is important to summarize recent progresses in the field investigating the functions of T cells (Table 1), especially the unconventional γδT cells, during the host immune responses to vector-borne pathogens, such as plasmodium, borrelia burgdorferi, and dengue fever, with a hope to accelerate our efforts in developing novel and effective clinical therapeutic strategies.
Immune responses of the host γδT cells to mosquito-borne pathogens
Mosquito-borne viruses
Chikungunya
Chikungunya is caused by the infection of chikungunya fever virus. Its clinical manifestations include headache, fever, and serious joint pains (23–25). Vectors for chikungunya fever mainly include Aedes Aegypti and Aedes albopictus (also called Asian tiger mosquito) (75). Currently, there is no effective drugs or vaccines available for treating or preventing chikungunya (76). Different T cell family members play differential roles after the invasion of chikungunya fever. Rapidly accumulated CD8+ T cells have been detected in the joints of mice that are acutely infected by the virus to promote protective immunity, but the lack of CD8+ T seems to have no effect in preventing arthrophlogosis of the infected mice (77). On the contrary, the virus will not be able to induce joint diseases after the exhaustion of CD4+ T cells (77, 78). Activated CD4+ T cells have been shown to be implicated in the pathogenesis of arthrosis swell (77–79).
Unconventional γδT cells are also likely involved in promoting protective immunity in the host against chikungunya invasion. The numbers of γδT cells in the feet and lymph nodes are significantly increased after the mice are infected by chikungunya. Mice defective in γδT cells are more susceptible to chikungunya infection, exhibiting more severe foot swell and inflammation in the ankles, as well as increased oxidative damages, suggesting that γδT cells play critical roles in the protective immunity during the infection of chikungunya and subsequent inflammation and tissue damage (80).
Rift Valley Fever
Rift Valley Fever is a type of zoonosis caused by Rift Valley Fever virus, transmitted mainly by aedes and culex (26). Although most of infected patients only have minor fever, headache, and muscle pains, some patients may develop serious symptoms, including retinopathy, meningoencephalitis symptoms, and hemorrhagic fever (27). A possible role for γδT cells in Rift Valley Fever has been reported for infected sheep. Similar to other ruminants, the sheep’s γδT cells account for a major population of its peripheral blood mononuclear cells. When recombinant Rift Valley Fever vaccine has been injected into the sheep, the percentage of CD11b+ γδT in its peripheral blood mononuclear cells can be significantly increased, suggesting that these cells may be critical for the host responses in respond to the virus infection (28).
Yellow fever virus
Yellow fever virus belongs to the Flaviviridae, transmitted primarily by aedes and haemophilus mosquito. The symptoms for yellow fever commonly include fever, headache, jaundice, muscle pains, and emesia. Some patients may develop more serious symptoms and die quickly (29). So far, the YF-17D vaccine for yellow fever is probably one of the most effective vaccines. When it is inoculated into human hosts, γδ2T cells can respond quickly and produce IFN-γ within a week (28).
Dengue fever
Dengue virus is a mosquito-borne pathogen that is transmitted between hosts by mosquito bite (30). In clinic, most patients with slight infection will not have complications, and only a small population of patients will progress into severe disease states, exhibiting thrombocytopenia, end-organ damage, and other symptoms (31). critically ill patients commonly develop secondary infection, which is closely related with innate immunity (32, 33). In the host immunity, T cells are critical for eliminating pathogen invasion. In in vitro experiments, CD8+ αβT cells can respond to dengue virus, and many evidence have shown that these cells play important roles in the host responses (34, 35).
We know relatively less about the roles for γδ T cells in the dengue virus infection. Eleonora Cimini and colleagues analyzed peripheral blood mononuclear cells from 15 dengue fever patients, the results show a significant decrease of γδ2-T-cell frequency and an increase of failure markers. In addition, the ability of γδ2-T-cells to produce IFN-γ in response to the phosphor-antigen was limited (36). Interestingly, primary human γδT has been shown to be able to kill dengue virus in vitro, suggesting a potential role for these cells in the anti-dengue virus process. Further investigations by Chen-Yu Tsai and colleagues have shown that primary γδT cells serve as the early source of IFN-γ during dengue virus infection and promote the host immune responses by eliminating the virus-infected cells. Monocytes can act as helper cells to participate in the virus infection and enhance the immune reponses in an IL-18-dependent manner (37).
Zika virus
Similar to dengue virus, Zica virus is primarily transmitted by infected aedes mosquitoes in tropical and subtropical regions. The infection of Zica virus can cause Guillain-Barre syndrome, neuropathy and myelitis. The infection during pregnancy may lead to the development of microcephaly and other congenital abnormalities in fetuses and newborns (38), and there has been a lack of clear treatment strategy. Previous reports have shown significantly increased Th1, Th2, Th9, and Th17 during acute Zica infection (39), suggesting that conventional T cells may dominate the host responses to Zica invasion. However, it should be noted that Eleonora Cimini and colleagues have also specifically detected γδ2TCR in Zika virus-infected patients and a significantly increased expression level of CD3+CD4−CD8− T cell subsets (40), implying a possible role for unconventional γδT cells.
West Nile virus
West Nile virus is a type of flavivirus, primarily transmitted by culex pipiens. Most West Nile virus-infected patients exhibit no significant symptoms (asymptomatic). However, the incidence of severe cases increases significantly in immunocompromised populations (41), and there is no targeted vaccine for such cases. γδT cells are thought to play an essential role in the early control of infection. They respond rapidly by producing large amounts of IFN-γ (42). In addition to serving as the main source of IFN-γ, γδT cells may also promote DC maturation and CD4+ T cell infiltration, as suggested by the observations that the expression of the dendric CD40, CD80, CD86 and MHC II molecules, as well as the expression of IL-12, are lower in γδT-deficient mice compared to those in wild-type mice. Furthermore, West Nile virus-induced activated γδT cells can promote the maturation of DC and the initiation and excitation of CD4+ cells (43) to combat against virus invasion.
Besides of above-mentioned roles, West Nile virus-activated γδT cells are also critical for limiting the invasion of the virus into the brain central vervous system, which is essential for protecting most infected-hosts from developing fatal encephalitis. Thomas Welte and colleagues have shown that, compared with young mice, older/aged mice are more susceptible to virus infection and have slower Vg1+ responses but more Vg4+ cells, which in turn produce TNF-α, a factor implicated in the destruction of the blood-brain barrier. On the other hand, low Vg4+ cells will allow the virus load in the brain, whereby reducing the mortality rate of virus-caused severe encephalitis (44). In the acquired immunity against West Nile virus infection, γδT cells also actively participate in the host defense process, and decreased memory responses of CD81+ T cells likely easily lead to secondary infection of the virus in γδT-deficient mice (45).
Mosquito-borne parasite
Malaria
Malaria is estimated to affect more than 200 million people each year (46). It is an arbo-borne disease transmitted by the bite of mosquito-borne plasmodium parasite. Despite more and more significant progresses in the control and reduction of malaria cases in the past decade, it still remains a major threat to global health (47). After invading into the host, malaria parasites parasitize their spores in the liver of the host, then start to grow and eventually form merozoites to invade red blood cells, leading to significant clinical symptoms and death (48, 49).
The invasion of plasmodium parasites can cause complicated immune responses, including humoral and cellular immunity responses. We have very limited knowledge about the nature of these responses, especially the cellular immunity responses. Previously, it has been reported that γδT cells can be activated by phosphor-antigen of the parasites (50), leading to a quick increase of γδT cells, especially the Vg9Vd2 subpopulation (51, 52) (Figure 2). Such activation and/or expansion of γδT cells appear to be persistent after the invasion of the plasmodium parasites and can occur during secondary infections (53–55). It is already known that the main cause of the high morbidity and mortality of malaria patients is the successful survival and exponential proliferation of plasmodium parasites within the host blood. In the supernatants from the co-culture of plasmodium parasites, γδT cells can be specifically expanded and promoted to acquire the parasite-lysing potential through the up-regulated expression of IFN-γ and other cytotoxic effector proteins.
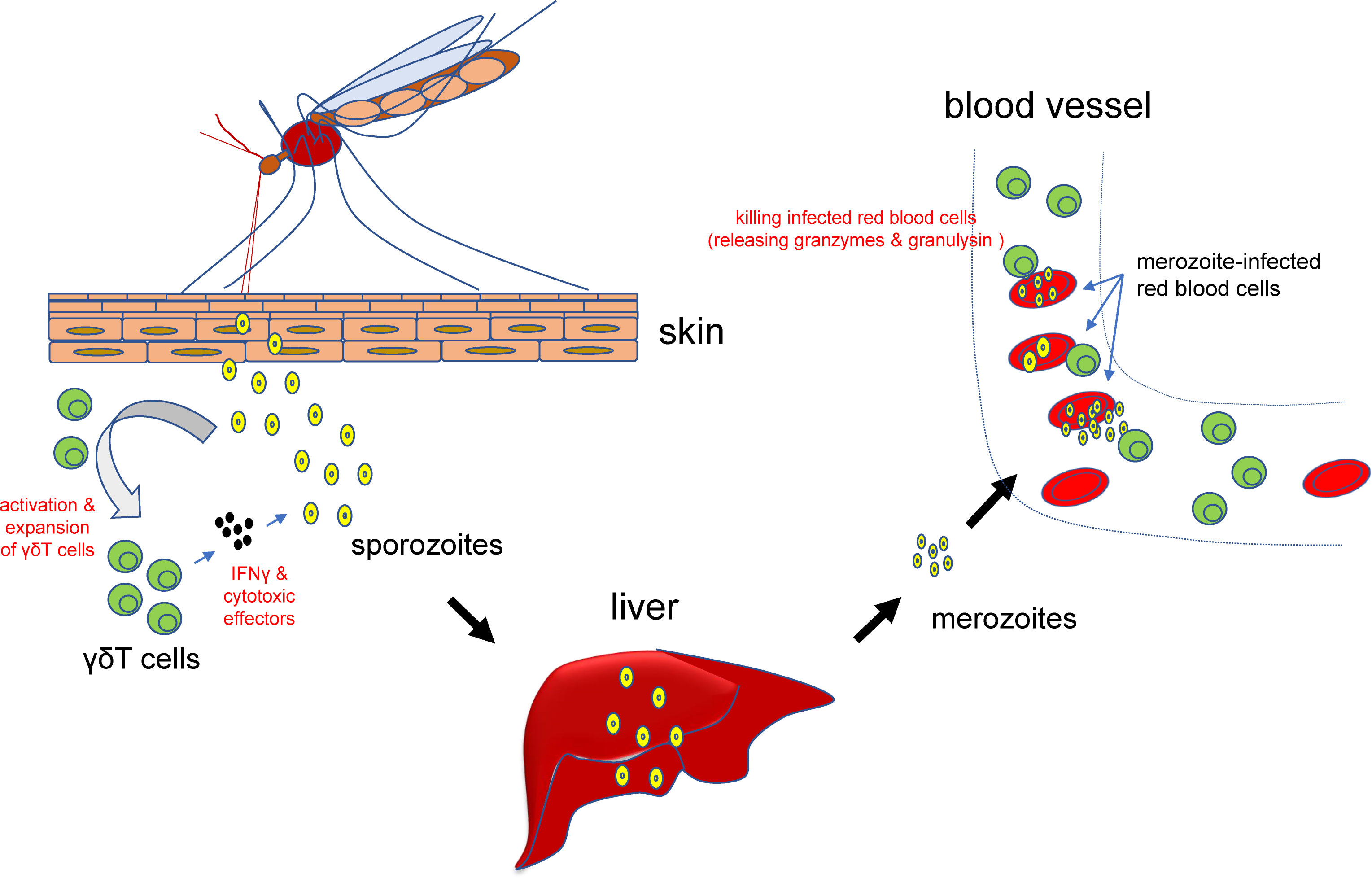
Figure 2 Roles for γδT cells in malaria parasites infection. At the early stage of infection, γδT cells can be activated and expanded and subsequently secret IFN-γ and other cytotoxic effectors to prevent or attenuate the infection. After the parasites have infected blood cells, activated γδT cells can also bind to the infected cells, release granzymes and granulysin, and kill the invaded plasmodium parasites and infected red blood cells.
Subsequently, γδT cells can directly kill plasmodium parasite-infected red blood cells to prevent or attenuate further infections (56) (Figure 2). These killer cells can bind to the infected red blood cells, release granzymes and granulysin to kill the invaded plasmodium parasites (46). Therefore, a decrease of the numbers of γδT cells may reversely facilitate the tolerance of plasmodium parasites. Accordingly, repeated plasmodium parasites infection may contribute to the development of clinical immunity in children living in plasmodium parasites-infested regions, which is characterized by decreased numbers of patients with clear symptoms, accompanied by increased numbers of asymptomatic patients (57, 58).
During the repeated plasmodium parasites infections, the numbers of γδ2+ γδT cells will be decreased in the peripheral blood, along with down-regulated production of cytokines and up-regulated immune-related factors. As such, repeated plasmodium parasites infections in the childhood will drive a progressive loss of the γδ2+ γδT cells, leading to increased immune tolerance of the patients to plasmodium parasites (59).
Notably, besides above-mentioned anti-pathogen roles, γδT cells may also have a paradoxical role in driving or participating in the pathogenesis of cerebral malaria, as the incidences of cerebral malaria complications is lower in infants with lower γδT reactivity. Julie Ribot and colleagues have shown that the γδT-deficient mice are more resistant to the development of cerebral malaria when infected with the plasmodium berghei ANKA sporozoa. Conversely, the presence of γδT cells can enhance the production of the plasmodium immune factors at the stage of liver infection and subsequently promote the inflammation reactions at the blood infection stage (60). Together, these findings demonstrate that γδT cells can promote the pathogenesis of IFN-γ-dependent plasmodium infection.
Immune responses of the host γδT cells to tick-borne pathogens
Borrelia burgdorferi
Compared to mosquito-borne pathogens, there have been less reports regarding the roles of γδT cells in the infection of tick-borne pathogens. The Lyme disease is the most frequently seen natural epidemic disease in the United States of America (61) and is transmitted through bites from different hosts by borrelia burgdorferi-carrying ticks (62). Initial symptoms after the pathogen invasion in most Lyme disease patients are characterized by chronic erythema migrans. Several weeks after the disease onset, some patients may develop neurological and cardiac problems. After several months, most patients will have recurrent symptoms such as joint pain or arthritis (63). Under the stimulation by borbora burgdorferi, an accumulation can be detected within the inflated joints of the patients (64), suggesting a possible role for these cells in the host immune responses to the pathogen. Similarly, proliferated γδT cells have also been detected in leukocytes from micewith Lyme disease and in human peripheral blood after tick bites (65). However, the responses of γδT cells may be indirectly through the actions of Toll-like receptors of DCs or monocytes, rather than through a direct mechanism by themselves (64). In addition, γδT cells may also act to activate the host acquired immunity during the infection of the pathogen (66).
Tularaemia
The pathogen for tularaemia is Francisella tularensis, a type of gram-negative bacterium, which can be transmitted by tick bites from different hosts and cause acute febrile disease (67). Increased numbers of γδT cells can be detected in the blood from tularaemia patients (67), possibly attributable to non-specific phosphor-molecules (68). Further investigations by M. KROCA and colleagues have revealed that the frequency of γδT cells can be increased and maintained for up tyo a year in the peripheral blood from tularaemia patients (69).
Immune responses of the host γδT cells to other vector-borne diseases
Leishmaniasis
Leishmaniasis is disease caused by the infection of leishmania and primarily transmitted by the bites from different hosts by leishmania-infected female diptera insect phlebotomus fly. Leishmaniasis can be categorized into three major subtypes, including visceral leishmaniasis, cutaneous leishmaniasis, and mucocutaneous leishmaniasis. Visceral leishmaniasis is also called kala-azar (black sickness) and is the most severe subtype of leishmaniasis. Visceral leishmaniasis leads to symptoms including irregular fever, weight loss, hepatosplenomegaly, and anamenia, and may eventually cause patient death. Cutaneous leishmaniasis is the most popular subtype and mainly causes skin ulcer. Mucocutaneous leishmaniasis mainly causes mucous membrane injury within the oral and nasal cavity. Leishmania belongs to parasites, and cellular immunity plays a central role in the host responses to its infection. An accumulation of γδT cells has been detected in the skin and blood from human hosts infected with leishmania (70, 71), suggesting a potential role for γδT cells in eliminating the infected parasites. Consistently, it has been reported that natural killer cells and γδT cells act through secreting INF-γ and TNF-α, respectively, to exert their functions in the host innate immunity against the leishmania invasion (72). Compared with healthy individuals, double negative T cells from about 75% of the cutaneous leishmaniasis patients express aβT cell receptors, and the rest of the double negative T cells express γδT cell receptors (73). In addition, dogs severely infected with leishmania may develop γδT cell lyphoma (71), suggesting that long-term stimulation by leishmania may lead to malignant transformation and lyphoma pathogenesis, but the underlying mechanism has been unclear.
South American trypanosomiasis
South American trypanosomiasis is also known as Chagas’ disease and mainly caused by direct contact with the excrement or urinate of Trypanosoma cruzi-infected trypanosoma triatoroae (stink bug). Currently there is no vaccine available for this disease. During the acute stage, the infected patients mainly exhibit symptoms including cyanosis swelling on one side of the skin or eyelid, headache, difficulty in breath, and muscle pains. At the chronic stage, the parasites parasitize in the intestine tracts and the heart. Years later after the infection, some patients may develop heart and digestive tract diseases. By utilizing γδT cell-deficient mice as a model, a recent report has shown that the γδT cells may not play a critical role in the elimination of the parasites at the acute stage of the disease but may contribute to tissue damage and pathogenesis. In cutaneous leishmaniasis patients, αβT cells and γδT cells secret inflammatory factors and IL-10, respectively to protect the hosts against the parasites invasion (74). Moreover, the frequency of IL-10 expression by γδT cells have been linked to an improvement of cardiac functions of cutaneous leishmaniasis patients, suggesting a potentially important role for γδT cells in the host responses (74).
Conclusions
It is estimated that vector-borne diseases lead to more than half million of global deaths each year, and some types of vector-borne diseases, such as chikungunya, leishmaniasis, and lymphatic filariasis may cause life-long diseases. Vaccines or other clinically effective drugs for many vector-borne diseases are still lacking, further worsening the life quality of the infected patients. As such, understanding better the host-pathogen interactions is critical for future developing novel and curative therapeutics.
Compared to unconventional γδT cells, the role for conventional αβT cells in the host responses to vector-borne pathogens has been more extensively and comprehensively studied, for instance Chikungunya virus (81–83), Rift Valley Fever virus (28), Yellow fever virus (84, 85), Zika virus (86, 87), West Nile virus (88), Malaria (89, 90), Borrelia burgdorferi (91), and Leishmaniasis (73, 92). However, as above-reviewed, our understanding of the role for γδT cells in these processes has been preliminary and incomplete.
It should be noted that most types of pathogens for vector-borne diseases are carried and disseminated by mosquitos and ticks, which transmitted the pathogens through the bites of host skin (93, 94). Given that γδT cells primarily reside in skin and mucosal tissues (95), these cells apparently are in the frontline to respond to pathogen invasion at the earliest stage. Therefore, it is important and urgent to gain a better understanding of the role for γδT cells during these precesses.
Previous studies have shown that αβT and γδT cells cooperate with each other and act synergistically towards eliminating pathogen invasion. As a bridge between innate and adaptive immunity, γδT cells have been known to play active roles during the first and secondary infections by pathogens and may serve as targets for clinical development. However, we still have limited knowledge about details of how they appropriately respond to vector-borne pathogen infection to facilitate the host immune responses.
During the early events of pathogen infection, activated γδT cells can secret multiple cytokines and inflammatory factors to induce the acquired immunity (96–98). Further studies in rodent models of infection of listeria, cytomegalovirus, and plasmodium parasites have revealed that γδT cells can strongly respond and quickly expand during secondary infections, suggesting that they have acquired certain levels of immune memory. These findings also suggest that the mode by which γδT cells respond to pathogen infections may be more complicated than previously appreciated.
Herein, we have reviewed recent findings related to the potential roles of γδT cells in response to several types of vector-borne pathogens, especially the mosquito- and tick-borne pathogens. We expect that these findings, together with those from more studies to analyze the interactions between γδT cells and vector-borne pathogens in the future, will provide useful information for developing clinically relevant targeted therapeutics.
Author contributions
CC and YY wrote the manuscript; AC and YY contributed to figures in this manuscript. All authors listed have made a substantial, direct, and intellectual contribution to the work and approved it for publication.
Acknowledgments
This work was partly supported by a junior faculty start-up fund from Anhui Medical University (to CC). We apologize for not being able to cite all important literatures in the field due to space limit.
Conflict of interest
The authors declare that the research was conducted in the absence of any commercial or financial relationships that could be construed as a potential conflict of interest.
Publisher’s note
All claims expressed in this article are solely those of the authors and do not necessarily represent those of their affiliated organizations, or those of the publisher, the editors and the reviewers. Any product that may be evaluated in this article, or claim that may be made by its manufacturer, is not guaranteed or endorsed by the publisher.
References
1. Endo A, Amarasekare P. Predicting the spread of vector-borne diseases in a warming world. Front Ecol Evol (2022) 10. doi: 10.3389/fevo.2022.758277
2. Lima AR, Dias LD, Garbuio M, Inada NM, Bagnato VS. A look at photodynamic inactivation as a tool for pests and vector-borne diseases control. Laser Phys Lett (2022) 19:025601. doi: 10.1088/1612-202X/ac4591
3. Chala B, Hamde F. Emerging and re-emerging vector-borne infectious diseases and the challenges for control: A review. Front Public Health (2021) 9:715759. doi: 10.3389/fpubh.2021.715759
4. Savic S, Vidic B, Grgic Z, Potkonjak A, Spasojevic L. Emerging vector-borne diseases - incidence through vectors. Front Public Health (2014) 2:267. doi: 10.3389/fpubh.2014.00267
5. Weiss RA, McMichael AJ. Social and environmental risk factors in the emergence of infectious diseases. Nat Med (2004) 10:S70–76. doi: 10.1038/nm1150
6. Chien YH, Meyer C, Bonneville M. Gammadelta T cells: first line of defense and beyond. Annu Rev Immunol (2014) 32:121–55. doi: 10.1146/annurev-immunol-032713-120216
7. Caccamo N, Dieli F, Wesch D, Jomaa H, Eberl M. Sex-specific phenotypical and functional differences in peripheral human Vgamma9/Vdelta2 T cells. J Leukoc Biol (2006) 79:663–6. doi: 10.1189/jlb.1105640
8. Morath A, Schamel WW. Alpha beta and gamma delta T cell receptors: Similar but different. J Leuk Biol (2020) 107:1045–55. doi: 10.1002/JLB.2MR1219-233R
9. Herrmann T, Karunakaran MM, Fichtner AS. A glance over the fence: Using phylogeny and species comparison for a better understanding of antigen recognition by human gamma delta T-cells. Immunol Rev (2020) 298:218–36. doi: 10.1111/imr.12919
10. Castro CD, Boughter CT, Broughton AE, Ramesh A, Adams EJ. Diversity in recognition and function of human gamma delta T cells. Immunol Rev (2020) 298:134–52. doi: 10.1111/imr.12930
11. Papotto PH, Yilmaz B, Silva-Santos B. Crosstalk between gamma delta T cells and the microbiota. Nat Microbiol (2021) 6:1110–7. doi: 10.1038/s41564-021-00948-2
12. Wu XX, Gu B, Yang H. The role of gamma delta T cells in the interaction between commensal and pathogenic bacteria in the intestinal mucosa. Int Rev Immunol (2022). doi: 10.1080/08830185.2022.2076846
13. Zhao Y, Lin L, Xiao Z, Li M, Wu X, Li W, et al. Protective role of gammadelta T cells in different pathogen infections and its potential clinical application. J Immunol Res (2018) 2018:5081634. doi: 10.1155/2018/5081634
14. Qu GY, Wang SL, Zhou ZL, Jiang DW, Liao AH, Luo J, et al. Comparing mouse and human tissue-resident gamma delta T cells. Front Immunol (2022) 13. doi: 10.3389/fimmu.2022.891687
15. Chen ZW. Protective immune responses of major Vgamma2Vdelta2 T-cell subset in m. Tuberculosis Infect Curr Opin Immunol (2016) 42:105–12. doi: 10.1016/j.coi.2016.06.005
16. Zhong H, Hu X, Janowski AB, Storch GA, Su L, Cao L, et al. Whole transcriptome profiling reveals major cell types in the cellular immune response against acute and chronic active Epstein-Barr virus infection. Sci Rep (2017) 7:17775. doi: 10.1038/s41598-017-18195-z
17. Cimini E, Sacchi A, Minicis S, De Bordoni V, Casetti R, Grassi G, et al. Vdelta2 T-cells kill ZIKV-infected cells by NKG2D-mediated cytotoxicity. Microorganisms (2019) 7:350. doi: 10.3390/microorganisms7090350
18. Agrati C, Castilletti C, Cimini E, Romanelli A, Lapa D, Quartu S, et al. Antiviral activity of human Vdelta2 T-cells against WNV includes both cytolytic and non-cytolytic mechanisms. New Microbiol (2016) 39:139–42.
19. Mangan BA, Dunne MR, O'Reilly VP, Dunne PJ, Exley MA, O'Shea D, et al. Cutting edge: CD1d restriction and Th1/Th2/Th17 cytokine secretion by human Vdelta3 T cells. J Immunol (2013) 191:30–4. doi: 10.4049/jimmunol.1300121
20. Ferrick DA, Schrenzel MD, Mulvania T, Hsieh B, Ferlin WG, Lepper H. Differential production of interferon-γ and interleukin-4 in response to Th1- and Th2-stimulating pathogens by γδ T cells in vivo. Nature (1995) 373:255–7. doi: 10.1038/373255a0
21. Lawand M, Dechanet-Merville J, Dieu-Nosjean MC. Key features of gamma-delta T-cell subsets in human diseases and their immunotherapeutic implications. Front Immunol (2017) 8. doi: 10.3389/fimmu.2017.00761
22. Thabit AK, Crandon JL, Nicolau DP. Antimicrobial resistance: impact on clinical and economic outcomes and the need for new antimicrobials. Expert Opin Pharmacother (2015) 16:159–77. doi: 10.1517/14656566.2015.993381
23. Burt FJ, Chen WQ, Miner JJ, Lenschow DJ, Merits A, Schnettler E, et al. Chikungunya virus: an update on the biology and pathogenesis of this emerging pathogen. Lancet Infect Dis (2017) 17:E107–17. doi: 10.1016/S1473-3099(16)30385-1
24. Cunha RVD, Trinta KS. Chikungunya virus: clinical aspects and treatment - a review. Mem Inst Oswaldo Cruz (2017) 112:523–31. doi: 10.1590/0074-02760170044
25. van Aalst M, Nelen CM, Goorhuis A, Stijnis C, Grobusch MP. Long-term sequelae of chikungunya virus disease: A systematic review. Travel Med Infect Di (2017) 15:8–22. doi: 10.1016/j.tmaid.2017.01.004
26. Linthicum KJ, Britch SC, Anyamba A. Rift valley fever: An emerging mosquito-borne disease. Annu Rev Entomol (2016) 61:395. doi: 10.1146/annurev-ento-010715-023819
27. Hise AG, Traylor Z, Hall NB, Sutherland LJ, Dahir S, Ermler ME, et al. Association of symptoms and severity of rift valley fever with genetic polymorphisms in human innate immune pathways. PloS Negl Trop D (2015) 9:e0003584. doi: 10.1371/journal.pntd.0003584
28. Lebedev M, Faburay B, Richt JA, Young A. Myeloid-like gammadelta T cell subset in the immune response to an experimental rift valley fever vaccine in sheep. Vet Immunol Immunopathol (2021) 233:110184. doi: 10.1016/j.vetimm.2021.110184
29. Litvoc MN, Novaes CTG, Lopes MIBF. Yellow fever. Rev Assoc Med Bras (2018) 64:106–13. doi: 10.1590/1806-9282.64.02.106
32. Jayachandran B, Chanda K, Balamurali MM. Overview of pathogenesis, diagnostics, and therapeutics of infectious diseases: Dengue and zika. ACS Omega (2021) 6:22487–96. doi: 10.1021/acsomega.1c03536
33. Guzman MG, Gubler DJ, Izquierdo A, Martinez E, Halstead SB. Dengue infection. Nat Rev Dis Primers (2016) 2:16055. doi: 10.1038/nrdp.2016.55
34. Bukowski JF, Kurane I, Lai CJ, Bray M, Falgout B, Ennis FA. Dengue virus-specific cross-reactive Cd8+ human cyto-toxic T-lymphocytes. J Virol (1989) 63:5086–91. doi: 10.1128/jvi.63.12.5086-5091.1989
35. Weiskopf D, Angelo MA, Azeredo EL, de Sidney J, Greenbaum JA, Fernando AN, et al. Comprehensive analysis of dengue virus-specific responses supports an HLA-linked protective role for CD8(+) T cells. P Natl Acad Sci USA (2013) 110:E2046–53. doi: 10.1073/pnas.1305227110
36. Cimini E, Grassi G, Beccacece A, Casetti R, Castilletti C, Capobianchi MR, et al. In acute dengue infection, high TIM-3 expression may contribute to the impairment of IFNgamma production by circulating Vdelta2 T cells. Viruses (2022) 14:130. doi: 10.3390/v14010130
37. Tsai CY, Liong KH, Gunalan MG, Li N, Lim DS, Fisher DA, et al. Type I IFNs and IL-18 regulate the antiviral response of primary human gammadelta T cells against dendritic cells infected with dengue virus. J Immunol (2015) 194:3890–900. doi: 10.4049/jimmunol.1303343
38. Wang ZY, Wang PG, An J. Zika virus and zika fever. Virol Sin (2016) 31:103–9. doi: 10.1007/s12250-016-3780-y
39. Tappe D, Perez-Giron JV, Zammarchi L, Rissland J, Ferreira DF, Jaenisch T, et al. Cytokine kinetics of zika virus-infected patients from acute to reconvalescent phase. Med Microbiol Immun (2016) 205:269–73. doi: 10.1007/s00430-015-0445-7
40. Cimini E, Castilletti C, Sacchi A, Casetti R, Bordoni V, Romanelli A, et al. Human zika infection induces a reduction of IFN-gamma producing CD4 T-cells and a parallel expansion of effector Vdelta2 T-cells. Sci Rep (2017) 7:6313. doi: 10.1038/s41598-017-06536-x
41. Campbell GL, Marfin AA, Lanciotti RS, Gubler DJ. West Nile Virus. Lancet Infect Dis (2002) 2:519–29. doi: 10.1016/S1473-3099(02)00368-7
42. Wang T, Scully E, Yin ZN, Kim J, Yan J, Mamula M, et al. IFN-gamma-producing gammadelta T cells help control murine West Nile virus infection. FASEB J (2004) 18:A806–7. doi: 10.4049/jimmunol
43. Fang H, Welte T, Zheng X, Chang GJ, Holbrook MR, Soong L, et al. Gammadelta T cells promote the maturation of dendritic cells during West Nile virus infection. FEMS Immunol Med Microbiol (2010) 59:71–80. doi: 10.1111/j.1574-695X.2010.00663.x
44. Welte T, Lamb J, Anderson JF, Born WK, O'Brien RL, Wang T. Role of two distinct gammadelta T cell subsets during West Nile virus infection. FEMS Immunol Med Microbiol (2008) 53:275–83. doi: 10.1111/j.1574-695X.2008.00430.x
45. Wang T, Gao Y, Scully E, Davis CT, Anderson JF, Welte T, et al. Gamma delta T cells facilitate adaptive immunity against West Nile virus infection in mice. J Immunol (2006) 177:1825–32. doi: 10.4049/jimmunol.177.3.1825
46. Hernandez-Castaneda MA, Happ K, Cattalani F, Wallimann A, Blanchard M, Fellay I, et al. Gammadelta T cells kill plasmodium falciparum in a granzyme- and granulysin-dependent mechanism during the late blood stage. J Immunol (2020) 204:1798–809. doi: 10.4049/jimmunol.1900725
47. Dantzler KW, Jagannathan P. Gammadelta T cells in antimalarial immunity: New insights into their diverse functions in protection and tolerance. Front Immunol (2018) 9:2445. doi: 10.3389/fimmu.2018.02445
48. Sturm A, Amino R, Sand C, van de Regen T, Retzlaff S, Rennenberg A, et al. Manipulation of host hepatocytes by the malaria parasite for delivery into liver sinusoids. Science (2006) 313:1287–90. doi: 10.1126/science.1129720
49. Miller LH, Ackerman HC, Su XZ, Wellems TE. Malaria biology and disease pathogenesis: insights for new treatments. Nat Med (2013) 19:156–67. doi: 10.1038/nm.3073
50. Eberl M. What you, too, should know about BTN2A1 antigen recognition by human gamma delta T cells: one step closer to knowing. Immunol Cell Biol (2020) 98:351–4. doi: 10.1111/imcb.12334
51. Deroost K, Langhorne J. Gamma/Delta T cells and their role in protection against malaria. Front Immunol (2018) 9. doi: 10.3389/fimmu.2018.02973
52. Costa G, Loizon S, Guenot M, Mocan I, Halary F, de Saint-Basile G, et al. Control of plasmodium falciparum erythrocytic cycle: gammadelta T cells target the red blood cell-invasive merozoites. Blood (2011) 118:6952–62. doi: 10.1182/blood-2011-08-376111
53. Galley HF, Lowes DA, Thompson K, Wilson ND, Wallace CA, Webster NR. Characterisation of gamma delta (gammadelta) T cell populations in patients with sepsis. Cell Biol Int (2015) 39:210–6. doi: 10.1002/cbin.10361
54. Matsushima A, Ogura H, Fujita K, Koh T, Tanaka H, Sumi Y, et al. Early activation of gammadelta T lymphocytes in patients with severe systemic inflammatory response syndrome. Shock (2004) 22:11–5. doi: 10.1097/01.shk.0000129203.84330.b3
55. Mamedov MR, Scholzen A, Nair RV, Cumnock K, Kenkel JA, Oliveira JHM, et al. A macrophage colony-Stimulating-Factor-Producing gammadelta T cell subset prevents malarial parasitemic recurrence. Immunity (2018) 48:350–363 e357. doi: 10.1016/j.immuni.2018.01.009
56. Junqueira C, Polidoro RB, Castro G, Absalon S, Liang Z, Sen Santara S, et al. Gamma delta T cells suppress plasmodium falciparum blood-stage infection by direct killing and phagocytosis. Nat Immunol (2021) 22:347–57. doi: 10.1038/s41590-020-00847-4
57. Schofield L, Grau GE. Immunological processes in malaria pathogenesis. Nat Rev Immunol (2005) 5:722–35. doi: 10.1038/nri1686
58. Portugal S, Moebius J, Skinner J, Doumbo S, Doumtabe D, Kone Y, et al. Exposure-dependent control of malaria-induced inflammation in children. PloS Pathog (2014) 10:e1004079. doi: 10.1371/journal.ppat.1004079
59. Jagannathan P, Kim CC, Greenhouse B, Nankya F, Bowen K, Eccles-James I, et al. Loss and dysfunction of Vdelta2(+) gammadelta T cells are associated with clinical tolerance to malaria. Sci Transl Med (2014) 6:251ra117. doi: 10.1126/scitranslmed.3009793
60. Ribot JC, Neres R, Zuzarte-Luis V, Gomes AQ, Mancio-Silva L, Mensurado S, et al. Gammadelta-T cells promote IFN-gamma-dependent plasmodium pathogenesis upon liver-stage infection. Proc Natl Acad Sci USA (2019) 116:9979–88.
61. Burgdorfer W, Barbour AG, Hayes SF, Benach JL, Grunwaldt E, Davis JP, et al. Lyme-Disease - a tick-borne spirochetosis. Science (1982) 216:1317–9. doi: 10.1126/science.7043737
63. Rahn DW. Lyme-Disease - clinical manifestations, diagnosis, and treatment. Semin Arthritis Rheu (1991) 20:201–18. doi: 10.1016/0049-0172(91)90017-T
64. Collins C, Shi C, Russell JQ, Fortner KA, Budd RC. Activation of gamma delta T cells by borrelia burgdorferi is indirect via a TLR- and caspase-dependent pathway. J Immunol (2008) 181:2392–8. doi: 10.4049/jimmunol.181.4.2392
65. Seth M B. Martin, * Richard flowers, MD,† Arturo p. saavedra, MD, PhD, MBA,†, m. and alejandro a. gru, a reactive peripheral gamma-delta T-cell lymphoid. Am J Dermatopathol (2019) 41:e73–5. doi: 10.1097/DAD.0000000000001352
66. Shi CX, Sahay B, Russell JQ, Fortner KA, Hardin N, Sellati TJ, et al. Reduced immune response to borrelia burgdorferi in the absence of gamma delta T cells. Infect Immun (2011) 79:3940–6. doi: 10.1128/IAI.00148-11
67. Sumida T, Maeda T, Takahashi H, Yoshida S, Yonaha F, Sakamoto A, et al. Predominant expansion of V gamma 9/V delta 2 T cells in a tularemia patient. Infect Immun (1992) 60:2554–8. doi: 10.1128/iai.60.6.2554-2558.1992
68. Poquet Y, Kroca M, Halary F, Stenmark S, Peyrat MA, Bonneville M, et al. Expansion of Vγ9Vδ2 T cells is triggered byFrancisella tularensis-derived phosphoantigens in tularemia but not after tularemia vaccination. Infect Immun (1998) 66(5):2107–14. doi: 10.1128/IAI.66.5.2107-2114.1998
69. Kroca M, Tärnvik A, Sjöstedt A. The proportion of circulating gd T cells increases after the first week of onset of. Clin Exp Immunol (2000) 120:280–4. doi: 10.1046/j.1365-2249.2000.01215.x
70. Modlin RL, Pirmez C, Hofman FM, Torigian V, Uyemura K, Rea TH, et al. Lymphocytes bearing antigen-specific gamma delta T-cell receptors accumulate in human infectious disease lesions. Nature (1989) 339:544–8. doi: 10.1038/339544a0
71. Welte T, Lamb J, Anderson JF, Born WK, O'Brien RL, Wang T, et al. Extranodal gammadelta-t-cell lymphoma in a dog with leishmaniasis. Vet Clin Pathol (2008) 37:298–301. doi: 10.1111/j.1939-165X.2008.00048.x
72. Lagler H, Willheim M, Traunmüller F, Wahl K, Winkler H, Ramharter M, et al. Cellular profile of cytokine production in a patient with visceral leishmaniasis: gammadelta+ T cells express both type 1 cytokines and interleukin-10. Scand J Immunol (2003) 57:291–5. doi: 10.1046/j.1365-3083.2003.01223.x
73. Antonelli LRV, Dutra WO, Oliveira RR, Torres KC, Guimarães LH, Bacellar O, et al. Disparate immunoregulatory potentials for double-negative (CD4(-) CD8(-)) alpha beta and gamma delta T cells from human patients with cutaneous leishmaniasis. Infect Immun (2006) 74:6317–23. doi: 10.1128/IAI.00890-06
74. Villani FN, Rocha MO, Nunes Mdo C, Antonelli LR, Magalhães LM, dos Santos JS, et al. Trypanosoma cruzi-induced activation of functionally distinct alphabeta and gammadelta CD4- CD8- T cells in individuals with polar forms of chagas' disease. Infect Immun (2010) 78:4421–30. doi: 10.1128/IAI.00179-10
75. van den Hurk AF, Hall-Mendelin S, Pyke AT, Frentiu FD, McElroy K, Day A, et al. Impact of wolbachia on infection with chikungunya and yellow fever viruses in the mosquito vector aedes aegypti. PloS Negl Trop D (2012) 6:e1892. doi: 10.1371/journal.pntd.0001892
76. Weaver SC, Lecuit M. Chikungunya virus and the global spread of a mosquito-borne disease. N Engl J Med (2015) 372:1231–9. doi: 10.1056/NEJMra1406035
77. Teo TH, Lum FM, Claser C, Lulla V, Lulla A, Merits A, et al. A pathogenic role for CD4(+) T cells during chikungunya virus infection in mice. J Immunol (2013) 190:259–69. doi: 10.4049/jimmunol.1202177
78. Poh CM, Chan YH, Ng LFP. Role of T cells in chikungunya virus infection and utilizing their potential in anti-viral immunity. Front Immunol (2020) 11:287. doi: 10.3389/fimmu.2020.00287
79. Hoarau JJ, Jaffar Bandjee MC, Krejbich Trotot P, Das T, Li-Pat-Yuen G, Dassa B, et al. Persistent chronic inflammation and infection by chikungunya arthritogenic alphavirus in spite of a robust host immune response. J Immunol (2010) 184:5914–27. doi: 10.4049/jimmunol.0900255
80. Long KM, Ferris MT, Whitmore AC, Montgomery SA, Thurlow LR, McGee CE, et al. Gammadelta T cells play a protective role in chikungunya virus-induced disease. J Virol (2016) 90:433–43. doi: 10.1128/JVI.02159-15
81. Wauquier N, Becquart P, Nkoghe D, Padilla C, Ndjoyi-Mbiguino A, Leroy EM. The acute phase of chikungunya virus infection in humans is associated with strong innate immunity and T CD8 cell activation. J Infect Dis (2011) 204:115–23. doi: 10.1093/infdis/jiq006
82. Poo YS, Rudd PA, Gardner J, Wilson JA, Larcher T, Colle MA, et al. Multiple immune factors are involved in controlling acute and chronic chikungunya virus infection. PloS Negl Trop D (2014) 8:e3354. doi: 10.1371/journal.pntd.0003354
83. Ninla-aesong P, Mitarnun W, Noipha K. Proinflammatory cytokines and chemokines as biomarkers of persistent arthralgia and severe disease after chikungunya virus infection: A 5-year follow-up study in southern Thailand. Viral Immunol (2019) 32:442–52. doi: 10.1089/vim.2019.0064
84. Douam F, Soto Albrecht YE, Hrebikova G, Sadimin E, Davidson C, Kotenko SV, et al. Type III interferon-mediated signaling is critical for controlling live attenuated yellow fever virus infection in vivo. Mbio (2017) 8(4):e00819–17. doi: 10.1128/mBio.00819-17
85. Akondy RS, Monson ND, Miller JD, Edupuganti S, Teuwen D, Wu H, et al. The yellow fever virus vaccine induces a broad and polyfunctional human memory CD8(+) T cell response. J Immunol (2009) 183:7919–30. doi: 10.4049/jimmunol.0803903
86. Wen JS, Tang WW, Sheets N, Ellison J, Sette A, Kim K, et al. Identification of zika virus epitopes reveals immunodominant and protective roles for dengue virus cross-reactive CD8(+) T cells. Nat Microbiol (2017) 2:17036. doi: 10.1038/nmicrobiol.2017.36
87. Bullard BL, Corder BN, Gorman MJ, Diamond MS, Weaver EA. Efficacy of a T cell-biased adenovirus vector as a zika virus vaccine. Sci Rep-Uk (2018) 8:18017. doi: 10.1038/s41598-018-35755-z
88. Kim S, Pinto AK, Myers NB, Hawkins O, Doll K, Kaabinejadian S, et al. A novel T-cell receptor mimic defines dendritic cells that present an immunodominant West Nile virus epitope in mice. Eur J Immunol (2014) 44:1936–46. doi: 10.1002/eji.201444450
89. Hassan R, Franco SA, Stefanoff CG, Romano SO, Diamond HR, Franco LG, et al. Hepatosplenic gamma delta T-cell lymphoma following seven malaria infections. Pathol Int (2006) 56:668–73. doi: 10.1111/j.1440-1827.2006.02027.x
90. Teirlinck AC, McCall MB, Roestenberg M, Scholzen A, Woestenenk R, de Mast Q, et al. Longevity and composition of cellular immune responses following experimental plasmodium falciparum malaria infection in humans. PloS Pathog (2011) 7:e1002389. doi: 10.1371/journal.ppat.1002389
91. Belperron AA, Dailey CM, Booth CJ, Bockenstedt LK. Marginal zone b-cell depletion impairs murine host defense against borrelia burgdorferi infection. Infect Immun (2007) 75:3354–60. doi: 10.1128/IAI.00422-07
92. Antonelli LR, Dutra WO, Oliveira RR, Torres KC, Guimarães LH, Bacellar O, et al. Disparate immunoregulatory potentials for CD4-CD8-alpha/beta and gamma/delta T cells from cutaneous leishmaniasis patients. Am J Trop Med Hyg (2007) 77:88–9. doi: 10.1128/IAI.00890-06
93. Cheng G, Liu Y, Wang PH, Xiao XP. Mosquito defense strategies against viral infection. Trends Parasitol (2016) 32:177–86. doi: 10.1016/j.pt.2015.09.009
94. Markowicz M, Schötta AM, Höss D, Kundi M, Schray C, Stockinger H, et al. Infections with tickborne pathogens after tick bite, Austria, 2015-2018. Emerg Infect Dis (2021) 27:1048–56. doi: 10.3201/eid2704.203366
95. Nielsen MM, Witherden DA, Havran WL. Gamma delta T cells in homeostasis and host defence of epithelial barrier tissues. Nat Rev Immunol (2017) 17:733–45. doi: 10.1038/nri.2017.101
96. Wang KQ, Hou YQ, Li QH, Zhao DP, Duan YC, Ran ZS, et al. Inhibitory effect of LY294002 on CD3mAb-activated T cells and mtb-ag-activated gamma delta T cells via TCR signal transduction pathway. Int J Clin Exp Patho (2017) 10:5538–44.
97. Wang KQ, Hou Y, Gu C, Zhao D, Duan Y, Ran Z, et al. Inhibitory effect of the mitogen activated protein kinase specific inhibitor PD98059 on mtb-ag-activated gamma delta T cells. Int J Clin Exp Patho (2017) 10:9644–8.
Keywords: γδT cells, vector-borne diseases (VBDs), host immune response, infection, pathogens
Citation: Chen C, Chen A and Yang Y (2022) A diversified role for γδT cells in vector-borne diseases. Front. Immunol. 13:965503. doi: 10.3389/fimmu.2022.965503
Received: 09 June 2022; Accepted: 28 July 2022;
Published: 16 August 2022.
Edited by:
Yuekang Xu, Anhui Normal University, ChinaReviewed by:
Hui Chen, Chinese Academy of Medical Sciences and Peking Union Medical College, ChinaTakeshi Nitta, The University of Tokyo, Japan
Copyright © 2022 Chen, Chen and Yang. This is an open-access article distributed under the terms of the Creative Commons Attribution License (CC BY). The use, distribution or reproduction in other forums is permitted, provided the original author(s) and the copyright owner(s) are credited and that the original publication in this journal is cited, in accordance with accepted academic practice. No use, distribution or reproduction is permitted which does not comply with these terms.
*Correspondence: Chen Chen, Y2hlbmNoZW5AYWhtdS5lZHUuY24=; Yanan Yang, eWFuZy55YW5hbkBhaG11LmVkdS5jbg==