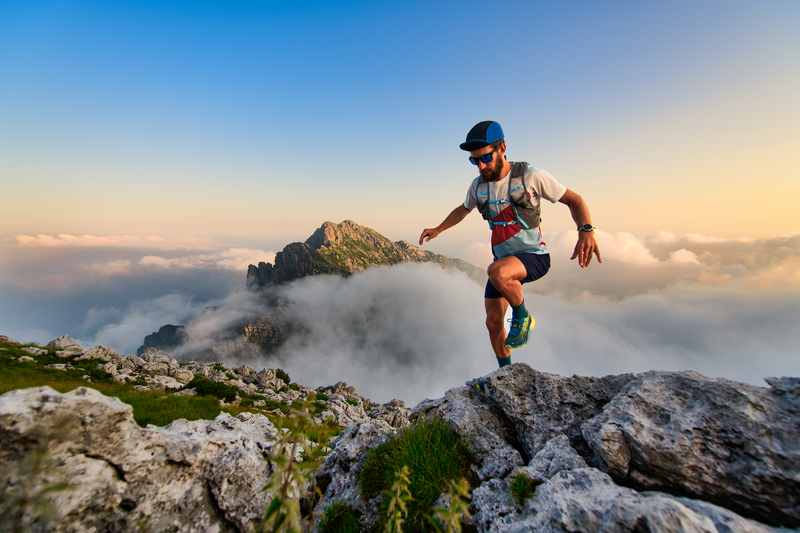
94% of researchers rate our articles as excellent or good
Learn more about the work of our research integrity team to safeguard the quality of each article we publish.
Find out more
REVIEW article
Front. Immunol. , 27 July 2022
Sec. Cancer Immunity and Immunotherapy
Volume 13 - 2022 | https://doi.org/10.3389/fimmu.2022.964898
This article is part of the Research Topic Immune Microenvironment and Immunotherapy in Malignant Brain Tumors View all 23 articles
Glioblastoma (GBM) is the most common and malignant primary brain tumor in adults. Currently, the standard treatment of glioblastoma includes surgery, radiotherapy, and chemotherapy. Despite aggressive treatment, the median survival is only 15 months. GBM progression and therapeutic resistance are the results of the complex interactions between tumor cells and tumor microenvironment (TME). TME consists of several different cell types, such as stromal cells, endothelial cells and immune cells. Although GBM has the immunologically “cold” characteristic with very little lymphocyte infiltration, the TME of GBM can contain more than 30% of tumor-associated microglia and macrophages (TAMs). TAMs can release cytokines and growth factors to promote tumor proliferation, survival and metastasis progression as well as inhibit the function of immune cells. Thus, TAMs are logical therapeutic targets for GBM. In this review, we discussed the characteristics and functions of the TAMs and evaluated the state of the art of TAMs-targeting strategies in GBM. This review helps to understand how TAMs promote GBM progression and summarizes the present therapeutic interventions to target TAMs. It will possibly pave the way for new immune therapeutic avenues for GBM patients.
Glioblastoma (GBM) is the most common and aggressive primary malignant brain tumor in adults with a dismal prognosis and poor quality of life (1). Despite aggressive treatment including surgical resection, targeted radiotherapy and high-dose chemotherapy, GBM patients still only have a median overall survival of 15 months and a 5-year survival rate of less than 3% (2). Some ongoing studies are evaluating the effects of immunotherapy for GBM, despite dramatic responses in some cases, the prognosis of GBM patients remains unfavorable (3). The immunologically “cold” tumor microenvironment (TME) has recently emerged as one of the crucial roles in GBM progression and therapeutic resistance (4). Thus, it may be an optimal strategy to disrupt the barrier of immunosuppression by targeting the genetically stable tumor stroma within the GBM TME rather than the constantly mutating tumor cells (4).
GBM is characterized by a lack of T cell infiltration but robust tumor-associated microglia and macrophages (TAMs) infiltration, which constitute more than 30% of infiltration cells in GBM (5). In addition, TAMs infiltration is associated with GBM progression. Compared with Foxp3+ regulatory T (Treg) cells, TAMs are a strong predictor of survival for patients with GBM (6). Mechanistically, TAMs could secret growth factors, cytokines and chemokines to build up and remodel the GBM TME, which enables the tumor cells to proliferate, survive and metastasize (7–9). Accordingly, targeting these tumor-supportive TAMs represents a novel promising treatment strategy to improve the prognosis of GBM patients (10).
In this review, we will discuss the current understanding of the origin, characteristics and functions of the TAMs and evaluate the state of the art of TAM-targeting strategies in GBM. This can help us understand how TAMs affect tumor progression and pave the way to enhance the efficacy of current therapies by targeting or harnessing TAMs for GBM patients.
In GBM, TAMs consist of resident microglia and peripheral macrophages recruiting to the tumor tissue (11). Both cell populations have the similar functions in the TME, so they are recognized as one cell cluster (12, 13). However, some studies have found that microglia and macrophages are different with various functions and different origins in GBM (Figure 1) (14, 15).
Figure 1 Distinct origins of Glioblastoma-associated Microglia and macrophages. Microglia derive from progenitors of the embryonic yolk sac, while peripheral macrophages are the monocytes deriving from the hematopoietic stem cells in bone marrow. Both cell populations enter the CNS and could be recruited by GBM cells, then referred to as tumor-associated microglia and macrophages (TAMs).
Brain-resident microglia are located in the brain parenchyma of the central nervous system and are derived from the primitive myeloid progenitors (15, 16). It is demonstrated that microglia derive from progenitors of the yolk sac and enter the CNS before embryonic day 8 in mice (15, 17). They are a long-living cell population and maintain the self-renewal capability without contribution from bone marrow-derived progenitors. Thus, microglia are identified as an ontogenically distinct population from the peripheral macrophage (18–21).
In healthy states, brain-resident microglia continuously scan their surroundings in order to maintain brain tissue homeostasis and immune defenses. However, microglia also have a pro-tumor activity in GBM patients (22).
In addition, TAMs also contain peripheral macrophages driven by inflammatory factors from GBM (23). Under the pathological stimulus of GBM, circulating bone marrow monocytes derived from hematopoietic stem cells can migrate to tumor tissue, where they differentiate into monocyte-derived macrophages and promote tumor progression (24, 25).
In a simplified model, TAMs are classified as M1 or M2 polarized cells that are relative with pro-inflammatory/anti-tumor or anti-inflammatory/pro-tumor property respectively. Interestingly, these two polarized states can convert from each other (26). Despite being oversimplified, this classification helps us to understand the polarization of TAMs.
This classification is based on some in vitro studies which observed the response to inflammations (27–31). However, the situation in vivo is more complex and intermediate phenotypes have been identified that do not belong to M1 or M2 polarized cells, suggesting that the transcriptional program of TAMs can be recognized as a dynamic spectrum. For example, different forms such as M2a, M2b and M2c have been proposed to describe the continuous phenotype of M2 polarized cells (32).
In terms of great diversity and plasticity of TAMs, they can acquire various phenotypes in different TME. It is reported that TAMs behave the M1 phenotype and exert anti-tumor activities in the early stage of tumor development (9, 33). The polarization of TAMs from M1 to M2 phenotype is associated with the tumor progression. Generally, TAMs mainly play a role in the initiation, malignant progression, angiogenesis and resistance to treatment in GBM. Thus, in this review, we focus on the pro-tumor effects of TAMs in GBM (5, 34–41).
TAMs constitute up to 30–40% of the bulk tumor mass and predominate the lymphocyte infiltration in GBM, making them an important consideration for their role in GBM initiation and progression. Several factors released by glioma cells can attract TAMs to the tumor sites (Figure 2). Then, TAMs could exert multiple pro-tumorigenic activities (Figure 3). To date, accumulating studies have demonstrated that factors released by TAMs play an important role in proliferation and invasion of GBM (42).
Figure 2 Glioblastoma-derived factors attracting TAMs. TAMs are recruited to the tumor sites by several glioblastoma-derived factors (CCL2, CSF-1, CX3CL1, MCP-1/3, GM-CSF, OPN, SDF-1, EGF).
Figure 3 Contribution of glioblastoma-associated microglia and macrophages to tumor progression. TAMs have bimodal, yin and yang effects on immune responses. The yin and yang microglia/macrophage subtypes refer to M2- and M1-like respectively. M1-like TAMs are relative with the pro-inflammatory property while M2-like TAMs are relative with the anti-inflammatory property. The polarization of TAMs from M1 to M2 phenotype is associated with the tumor progression. TAMs are believed to promote GBM progression by several pro-tumorigenic activities including: 1) promoting GBM cells proliferation; 2) promoting GBM cells migration and invasion; 3) promoting angiogenesis in GBM; 4) facilitating extracellular matrix (ECM) degradation; 5) facilitating the immunosuppressive TME.
Microglia and microglia-conditioned medium promote the invasion of glioma in vitro, suggesting that substances released by TAMs mediated this effect. In contrast, oligodendroglia and endothelial cells only weakly stimulated glioma cell motility (43). However, this motility-promoting activity was decreased in glioma cells when the transforming growth factor (TGF)-β was knocked down, indicating the invasion of glioma cells is dependent on microglia-derived TGF-β (44). GBM invasion promoted by TGF-β is involved with the upregulation of integrin. TGF-β induces matrix metalloprotein (MMP)-2 expression and suppresses tissue inhibitor of metalloproteinases (TIMP)-2 expression, both of which accelerate the extracellular matrix (ECM) breakdown (45). MMP can not only mediate the degradation of ECM to facilitate the invasion of GBM into the brain parenchyma, but also facilitate the proliferation of GBM. In response to GBM released factors, membrane type 1 metalloprotease (MT1-MMP) is upregulated in TAMs, but not in tumor cells. Interestingly, microglia release TGF-β, which triggers the release of pro-MMP2 from GBM. Pro-MMP2 is then transformed into active MMP2 by TAMs-expressed MT1-MMP. After deletion of MyD88 or p38, the toll-like receptor (TLR) adapter protein, MT1-MMP expression and GBM proliferation is inhibited. Thus, the high expression of MT1-MMP and subsequently increased proliferation capacity is mediated by TAMs’ TLR and the p38 MAPK pathway (46, 47). Canonical NF-κB signaling has an anti-inflammatory role and is required for GBM proliferation (48).
Pleiotrophin (PTN)-PTPRZ1 paracrine signaling can support GBM malignant proliferation. TAMs secrete abundant PTN which binds to its receptor PTPRZ1 to stimulate GBM proliferation (49). In response to GBM secreted factors, TAMs can express high levels of ATX and LPA1 to support GBM proliferation and invasion (50). Co-chaperone stress-inducible protein 1 (STI1) secreted by TAMs promotes proliferation and invasion of GBM in vitro. In vivo, the STI1 expression is also measured in a GBM model. Interestingly, high expression of STI1 was observed in TAMs but not in peripheral blood monocytes and lymphocytes, suggesting that TAMs-derived STI1 is also modulated by the TME of GBM (51).
The CCL2/CCR2/IL-6 loop also has a role in promoting GBM invasion. Glioma-derived CCL2 acts on microglia and then triggers the production of IL-6 from microglia, which in turn promotes GBM invasion (52). TAMs highly express CCL8 which promotes the pseudopodia formation of GBM cells. CCL8 in the TME can bind to CCR1 and CCR5 on GBM cells and activate ERK1/2 phosphorylation, finally increasing the invasion of GBM (53). In addition, colony-stimulating factor-1 (CSF-1) secreted by GBM is a chemoattractant for TAMs and facilitates its M2-like activation in autochthonous models, while also demonstrating that CSF-1 overexpression induces GBM proliferation (54, 55). Furthermore, CSF-1 and epidermal growth factor (EGF) released by microglia also stimulate GBM invasion (56). Since both microglia and GBM express EGFR, EGF may serve as a paracrine factor to recruit TAMs to the tumor sites, and at the same time, it binds to EGFR of GBM to stimulate GBM invasion (57, 58).
Several studies have shown that GBM is characterized by abnormal angiogenesis (59–61). GBM resistance to anti-VEGF therapy is associated with the infiltration of macrophages (62). In GBM, there is an “inflammation-driven angiogenesis” mechanism. M2-like immunosuppressive macrophages promote angiogenesis, while M1-like pro-inflammatory macrophages suppress angiogenesis (63).
Depleting the TAMs in vivo reduces the GBM vessel density, suggesting that TAMs are of importance in tumor angiogenesis (64). Interestingly, selective depletion of resident microglia reduced tumoral vessels compared to ablation of the whole TAMs, indicating that resident microglia rather than peripheral macrophages are the crucial modulator to promote the angiogenesis of GBM (64).
TAMs isolated from GL261 glioma overexpress proangiogenic factors such as VEGF and CXCL2. VEGF is a well-known regulator of angiogenesis while CXCL2, a poorly described chemokine, displayed stronger angiogenic activity than VEGF in vitro (64). Interaction of the receptor for advanced glycation end products (RAGE) with its ligands can promote tumor angiogenesis. In GBM, the RAGE signaling in TAMs drives angiogenesis (65), during which the activation of RAGE can upregulate the IL-6 expression (66). Bevacizumab, a VEGF-targeting antibody, failed in a clinical trial evaluating the effect of the combination of bevacizumab to radiotherapy-temozolomide (TMZ) for the treatment of newly diagnosed GBM. Besides, the rate of adverse events was higher with bevacizumab than with placebo (67, 68). GBM-derived macrophage colony-stimulating factor (M-CSF) increases the IGFBP1 expression of microglial cells, which is an important effector to promote angiogenesis. Thus, IGFBP1 could be a potential alternate candidate for developing a targeted therapy for GBM (69).
GBM is characterized by the strong immunosuppressive TME. TAMs are important drivers of the local immunosuppressive TME and are relative to GBM progression and resistance to immunomodulating therapeutic strategies (70). M2-like TAMs contribute to the immunosuppressive TME by secreting immunosuppressive factors such as IL-6, TGF-β, IL-10 in GBM, while only low levels of M1-like pro-inflammatory cytokines such as IL-2, IL-12, TNF-α and IFN-γ are detected (52, 71, 72). This TAMs phenotype is modulated by GBM cells or their soluble factors in vitro, which in turn promotes the suppressive TME and contributes to immunoevasion of GBM (73).
The immune functions of TAMs isolated from GBM patients were analyzed. The results indicated that the expression of surface major histocompatibility complex class II (MHC class II) and costimulatory molecules CD40, CD80, and CD86 were reduced in TAMs, thus inhibiting antigen cross-presentation and T cells activation (74, 75). Similarly, in a rodent glioma model, the MHC II and B7 costimulatory molecules were significantly reduced when compared with the normal brain (76). mTOR-dependent regulation of STAT3 and NF-κB activity mediated the immunosuppressive function of TAMs. It plays a role in GBM immune evasion by suppressing the effector T cells infiltration, proliferation and immune function (77). In both in vitro and in vivo GBM mouse models, the activation of mTOR signaling was observed in the microglia but not in bone marrow-derived macrophages. The activation of mTOR signaling increased phosphorylation of STAT3 and inhibited the NF-κB pathway in microglia, thereby upregulating the expression of IL6 and IL10 (the anti-inflammatory M2-like cytokines) with a concomitant reduction in expression of IL12 (the pro-inflammatory M1-like cytokines) (77). The upregulation of STAT3 in TAMs of GBM has been previously reported (78, 79). It is associated with higher GBM grade and expression of anti-inflammatory cytokines (79). NF-κB is downregulated in TAMs, leading to reduced expression of inflammatory TLR, resulting in the impairment of anti-tumor immune responses in GBM (80). However, although another study found substantial expression of TLRs in TAMs, they were not stimulated to produce pro-inflammatory cytokines (75).
FasL/Fas pathway plays an important role in immunosuppressive TME in GBM (81). T cells invading GBM express Fas receptor, which can directly contact with FasL-expressing GBM cells, and thus induce the apoptosis of T cells (82). Immunoblotting indicated that the membrane-bound FasL expressed nearly twice as much when murine G26 gliomas were implanted intracranially as compared to subcutaneously. Interestingly, microglia were absent in the subcutaneous tumors. Microglia are a major source of FasL expression in GBM and possibly contribute to the local immunosuppressive TME of GBM, which was mediated by the apoptosis of T cells via FasL/Fas interaction (81, 83). However, in TAMs isolated from GBM patients, the expression of FasL was low to absent, suggesting that apoptosis of T cells mediated through Fas/FasL may be a marginal immunosuppressive function by microglia (84). TAMs can express several chemokines such as CCL2, 5, 20 and 22 to enhance the recruitment of regulatory T cells (Tregs) (85–87). Tregs subsequently inhibit the activity of CD4+ and CD8+ effector T cells, natural killer (NK) cells, NKT cells, and antigen-presenting cells (APC) through a variety of mechanisms (85–87). It was recently described that TAMs overexpressed the enzymes indoleamine 2,3-dioxygenase 1 and tryptophan 2,3-dioxygenase 2 (IDO1/TDO2) to promote immunosuppression in GBM. L-Kynurenine (KYN) is a tryptophan-derived metabolite as a result of the enzymatic activity of IDO1/TDO2. KYN activates aryl hydrocarbon receptor (AHR) in TAMs to promote CCR2 expression, CD8+ T cell dysfunction and the generation of Tregs (88, 89).
Based on the multiple pro-tumor functions of TAMs, they are considered as an important potential therapeutic target. Possible approaches such as depleting TAMs, repolarizing M2-like to M1-like TAMs, enhancing phagocytic activity of TAMs and reducing recruitment of TAMs will be discussed in the following (Figure 4).
Figure 4 Strategies to target glioblastoma-associated microglia and macrophages. There are four general therapeutic strategies to target or utilize TAMs in GBM treatment including 1) directly depleting TAMs; 2) reprograming TAMs from an M2-like pro-tumoral phenotype to an M1-like anti-tumoral phenotype; 3) enhancing TAMs phagocytosis on tumor cells; 4) reducing TAMs recruitment to the tumor sites.
Due to the high infiltration of TAMs within GBM and thereby driving tumor progression, several methods to deplete TAMs have been investigated. For example, in the CD11b-HSVTK (herpes simplex virus thymidine kinase) mouse model, infusion of ganciclovir performs microglia depletion in vivo (90). Using this model, vessel density and tumor volume were decreased in GL261-bearing mice (46, 64). Interestingly, selective depletion of resident microglia induced similar results compared to the reduction of the whole myeloid cell population, suggesting that resident microglia rather than peripheral macrophages are more crucial in promoting vascularization in GBM (64). On the contrary, macrophages can also slow the progression of malignant gliomas. TAMs depletion by ganciclovir contributed to a 33% tumor increase in the GBM syngeneic GL261 mouse model (35). Liposome-encapsulated clodronate, which can selectively deplete microglia, reduced the invasiveness of GBM in GL261 cultured brain slices. Inoculation of exogenous microglia can restore the invasiveness behavior (91). However, administration of liposome-encapsulated clodronate into brain parenchyma can also cause severe damage to other brain cells and blood vessel integrity (92). Selectively limiting peripheral macrophage infiltration via genetic Ccl2 depletion prolonged the survival of tumor-bearing mice (93). A silico studies based on real patients parameters have found that depletion of TAMs may be beneficial only for the patients who received the therapy in the early-stage GBM (94).
A major limitation of these studies is that the depletion of TAMs was achieved before the gliomagenesis. Thus, these findings lack translatability because of the totally different situations occurring in GBM patients. Given the high plasticity and heterogenous of TAMs in the GBM microenvironment, depleting the total TAMs pool may not be the optimal strategy.
In addition to depleting TAMs, it may be more efficient to repolarize M2-like to M1-like phenotype and acquire anti-tumorigenic functions.
CSF-1 secreted by glioma cells is essential for the differentiation and survival of TAMs and facilitates M2 polarization of TAMs (55, 56). Therefore, blocking the CSF-1 or its receptor CSF-1R is another potential therapeutic strategy for GBM (55, 95). In a mouse proneural GBM model, CSF-1R blockade significantly inhibited GBM tumor growth and prolonged survival. At the same time, expression of M2 markers decreased in TAMs (55). However, although CSF-1R significantly prolonged overall survival of GBM-bearing mice, tumors eventually recurred in more than 50% of mice.
In recurrent GBM, the phosphatidylinositol 3-kinase (PI3K) pathway activity, driven by macrophage-secreted insulin-like growth factor-1 (IGF-1) and tumor cell IGF-1 receptor (IGF-1R), was increased. To break the resistance state to CSF-1R inhibitors, blocking PI3K and IGF-1R is combined with CSF-1R inhibition, resulting in significantly suppressed tumor growth and prolonged overall survival (96). In addition, transcription factor STAT3 is another target to repolarize the TAMs to the M1-like phenotype. Inhibition of STAT3 in tumor TAMs can activate M1-like phenotype and reverse cytokine expression profile to anti-tumorigenic function, resulting in GBM growth delay (38, 97, 98).
The nanoparticles delivering mRNAs encoding interferon regulatory factor-5 (IRF-5) and its activating kinase IKKβ were infused into glioma-bearing mice. These nanoreagents reversed the pro-tumor state of TAMs, repolarized them to an M1-like phenotype and promoted tumor regression (99). Recently, a study showed that IL-33 is expressed in both human GBM specimens and murine models. A positive correlation between IL-33 expression and M2-like phenotype markers was observed. Inhibiting IL-33 expression dramatically suppressed GBM growth and prolonged survival (100).
Through phagocytosis, APCs are able to capture and eliminate tumor cells and present the tumor-derived antigens to prime T cells. However, GBM cells can overexpress the anti-phagocytosis molecule (don’t eat me signals) such as CD47 which binds to signal regulatory protein alpha (SIRPα), an inhibitory receptor expressed on TAMs to inhibit phagocytosis by TAMs (101–103). Thus, inhibiting the CD47-SIRPα anti-phagocytic axis may be a promising strategy to enhance tumor phagocytosis and activate the adaptive immune system.
Hu5F9-G4, a humanized anti-CD47 antibody, can block the anti-phagocytic CD47-SIRPα interaction. It enhanced tumor phagocytosis and reduced tumor burden in preclinical xenografts of various human malignancies, including GBM (103). In addition, in an immunocompetent mouse glioma model, blocking the CD47-SIRPα axis by anti-CD47 antibody significantly increased phagocytosis of glioma cells and GSCs by macrophages, consequently inhibiting tumor growth and prolonging survival (104). By using orthotopically xenografted, immunodeficient and syngeneic mouse models with genetically color-coded macrophages (Ccr2RFP) and microglia (Cx3cr1GFP), it was demonstrated that, in addition to macrophages, resident microglia within the TAMs pool are also effector cells of GBM cells phagocytosis in response to anti-CD47 blockade. Additionally, microglia induced a less inflammatory response compared to peripheral macrophages, making them a promising target for clinical applications (102). CD47 knockout increased tumor-associated extracellular matrix protein tenascin C (TNC) in U87 intracranial xenografts. Its overexpression in GBM cells is mediated by the Notch pathway. Inhibition of TNC reduced the phagocytosis of CD47-/- tumor cells in cocultures and enhanced the growth of CD47-/- xenografts in vivo. This suggests that TNC can be a potential target to enhance phagocytosis mediated by CD47 blockade in GBM (105).
However, CD47 blockade alone is inefficient in stimulating glioma cells phagocytosis by TAMs and has limited anti-tumor effects. Combining TMZ with CD47 blockade enhances the glioma cells phagocytosis and increases antigen cross-presentation, leading to more efficient T cells priming and anti-tumor immune responses in vivo. This combo treatment also activates immune checkpoint which can be turned off by sequential administrations of an anti-PD1 antibody (101).
Due to the numerous chemoattractants existing in the TME of GBM, which can recruit TAMs to promote tumor progression, it is a promising therapy to reduce the recruitment of tumor-promoting TAMs.
The CX3CL1/CX3CR1 chemokine axis is crucial for the recruitment of TAMs. In response to activation of CX3CL1, CX3CR1-expressing TAMs can be recruited to promote GBM progression. In contrast, after blocking the CX3CL1/CX3CR1 system with neutralizing anti-CX3CL1 or CX3CR1 antibodies, recruitment of TAMs was significantly inhibited (106). Periostin secreted by GSCs can promote the recruitment of TAMs through the integrin αvβ3 in human GBM. Inhibiting periostin markedly decreased TAMs recruitment, suppressed tumor growth and prolonged survival of mice bearing GSC-derived xenografts (107). The activation of stromal cell-derived factor-1 (SDF-1/CXCL12) and its receptor, CXCR4, is essential for TAMs recruitment (108–110). Using U87-MG intracranial xenografts, inhibitors of CXCR4 signaling such as peptide R and Plerixafor modulated the TAMs towards an anti-tumorigenic phenotype and inhibited the growth of glioma cells in vitro (111). In addition, CCL2 (MCP-1) produced by the GBM microenvironment recruits microglial cells to GBM and promotes their growth in vivo (112).
OPN, secreted by GBM cells and TAMs, is another chemokine for recruiting TAMs to GBM and has the potential to be exploited. A positive correlation has been observed between OPN expression levels and glioma grades as well as the infiltration of TAMs. OPN blockade significantly impaired the ability of GBM to recruit TAMs, enhanced T cells effector activity and prolonged survival in GL261 glioma-bearing mice (113). Since the high expression levels of OPN are observed in TME, OPN can also be exploited as a homing molecule to the GBM TME (114, 115). For example, OPN aptamer triggered CD3+ T cells activation within the TME and prolonged the survival in mice bearing intracerebral GL261 tumors (113).
Three noncytotoxic drugs (an antibiotic - minocycline, an antihypertensive drug - telmisartan, and a bisphosphonate - zoledronic acid) have an inhibitory function on the MCP-1 synthesis and impede TAMs recruitment induced by MCP-1, thereby delaying GBM growth. Since these three older drugs have already been approved for treating infection, hypertension, and osteoporosis, respectively, they have the advantages of better safety and lower cost. They will be tested in a pilot clinical trial in primary glioblastoma patients (116). CSF-1 secreted by glioma cells can also support the recruitment of TAMs (55). Thus, CSF-1R inhibition has been receiving increasing attention (95, 117). Blockade of CSF-1R signaling by using the PLX3397 (a CSF-1R inhibitor) in glioma-bearing mice decreased the recruitment of TAMs and reduced the GBM invasion (56). However, these promising results was failed to translate into clinical application (118). No efficacy was observed in a phase II clinical trial (NCT01349036) in which PLX3397 was orally administered in recurrent GBM patients (118).
Based on the above preclinical studies, many clinical trials are being initiated by targeting TAMs for the treatment of GBM. Emactuzumab (RG7155), a therapeutic anti-CSF-1R antibody, has been combined with the programmed cell death-1 ligand (PD-L1)-blocking mAb atezolizumab in a phase I study (NCT02323191), in which a considerable ORR was particularly seen with a manageable safety profile. Another key target for CSF-1R blockage is the transcription factor STAT3. In a phase I clinical trial (NCT01904123), the side effects and best dose of STAT3 inhibitor WP1066 were evaluated in patients with recurrent malignant glioma. Macrophage migration inhibitory factor (MIF) is overexpressed in many tumors, including GBM. MIF induces angiogenesis, promotes cell cycle progression, and inhibits apoptosis (119). An ongoing phase I/II clinical trial (NCT03782415) is evaluating Ibudilast (a MIF inhibitor) and TMZ combo treatment in newly diagnosed and recurrent GBM. As mentioned before, the SDF-1/CXCL12 axis is essential for TAMs recruitment. Therefore, a pilot phase I/II trial (NCT01977677) studied the side effects and best dose of Plerixafor (a CXCR4 inhibitor) after radiation therapy plus TMZ to see how well it works in treating patients with newly diagnosed high-grade glioma. Table 1 summarizes the clinical trials of monotherapies or combinatorial approaches targeting TAMs in GBM.
Decades of investigations have been conducted to focus on the tumor cells themselves while less attention has been paid to the components of TME. With the accumulation of knowledge about the TME, TAMs have emerged as exciting targets for therapeutic intervention. Although many investigations have contributed substantially to our understanding of the origin, polarization and functional heterogeneity of TAMs, it is still unclear upon the complex interaction and dynamics between GBM and TAMs.
Many researches have demonstrated that macrophages and microglia within the TAMs pool in GBM show distinct morphological and transcriptional changes. In addition, they both have high plasticity and can be modulated by the different TME. Thus, it is crucial to differentiate these two cell populations and understand their different roles in GBM tumorigenesis and progression. In order to successfully target the immunosuppressive M2-like TAMs population for GBM treatment, we also need to fully understand the complex interplay between TAMs and other immune cells within the TME. Furthermore, a better understanding of the bridge-like role of TAMs between innate immune and adaptive immune system is indispensable for exploiting TAMs to activate an anti-tumor immune response.
Targeting TAMs has proven to be a promising strategy in preclinical trials. This strategy could not only suppress the germination of GBM “seeds” but also wreak the “fertile soil” of GBM, further destroying the immunosuppressive microenvironment, thus inhibiting the tumor growth. With the development of other immunotherapeutic strategies, novel synergistic combinations of TAMs-targeting therapeutics and other immunotherapies may ultimately support the eradication of GBM. Currently, extensive exploration and development of TAMs-targeting agents have been ongoing, among which some have been utilized in clinical trials. Hence, there is no doubt that TAMs-targeting strategies will benefit more GBM patients in the future.
All authors contributed to gathering of data, writing, editing, and revising of the manuscript.
This work was supported by the National Natural Science Foundation of China (81772693 and 82073404) and the Youth fund of National Natural Science Fund of China (82102898).
We thank our colleagues for stimulating discussions.
The authors declare that the research was conducted in the absence of any commercial or financial relationships that could be construed as a potential conflict of interest.
All claims expressed in this article are solely those of the authors and do not necessarily represent those of their affiliated organizations, or those of the publisher, the editors and the reviewers. Any product that may be evaluated in this article, or claim that may be made by its manufacturer, is not guaranteed or endorsed by the publisher.
1. Omuro A, DeAngelis LM. Glioblastoma and other malignant gliomas: A clinical review. JAMA (2013) 310:1842–50. doi: 10.1001/jama.2013.280319
2. Stupp R, Taillibert S, Kanner A, Read W, Steinberg D, Lhermitte B, et al. Effect of tumor-treating fields plus maintenance temozolomide vs maintenance temozolomide alone on survival in patients with glioblastoma: A randomized clinical trial. JAMA (2017) 318:2306–16. doi: 10.1001/jama.2017.18718
3. Buerki RA, Chheda ZS, Okada H. Immunotherapy of primary brain tumors: Facts and hopes. Clin Cancer Res Off J Am Assoc Cancer Res (2018) 24:5198–205. doi: 10.1158/1078-0432.CCR-17-2769
4. Tomaszewski W, Sanchez-Perez L, Gajewski TF, Sampson JH. Brain tumor microenvironment and host state: Implications for immunotherapy. Clin Cancer Res Off J Am Assoc Cancer Res (2019) 25:4202–10. doi: 10.1158/1078-0432.CCR-18-1627
5. Hambardzumyan D, Gutmann DH, Kettenmann H. The role of microglia and macrophages in glioma maintenance and progression. Nat Neurosci (2016) 19:20–7. doi: 10.1038/nn.4185
6. Thomas AA, Fisher JL, Rahme GJ, Hampton TH, Baron U, Olek S, et al. Regulatory T cells are not a strong predictor of survival for patients with glioblastoma. Neuro Oncol (2015) 17:801–9. doi: 10.1093/neuonc/nou363
7. Noy R, Pollard JW. Tumor-associated macrophages: from mechanisms to therapy. Immunity (2014) 41:49–61. doi: 10.1016/j.immuni.2014.06.010
8. Gonzalez H, Hagerling C, Werb Z. Roles of the immune system in cancer: from tumor initiation to metastatic progression. Genes Dev (2018) 32:1267–84. doi: 10.1101/gad.314617.118
9. Mantovani A, Marchesi F, Malesci A, Laghi L, Allavena P. Tumour-associated macrophages as treatment targets in oncology. Nat Rev Clin Oncol (2017) 14:399–416. doi: 10.1038/nrclinonc.2016.217
10. Poon CC, Sarkar S, Yong VW, Kelly JJP. Glioblastoma-associated microglia and macrophages: targets for therapies to improve prognosis. Brain (2017) 140:1548–60. doi: 10.1093/brain/aww355
11. Ricard C, Tchoghandjian A, Luche H, Grenot P, Figarella-Branger D, Rougon G, et al. Phenotypic dynamics of microglial and monocyte-derived cells in glioblastoma-bearing mice. Sci Rep (2016) 6:26381. doi: 10.1038/srep26381
12. Dusoswa SA, Verhoeff J, Abels E, Méndez-Huergo SP, Croci DO, Kuijper LH, et al. Glioblastomas exploit truncated O-linked glycans for local and distant immune modulation via the macrophage galactose-type lectin. Proc Natl Acad Sci USA (2020) 117:3693–703. doi: 10.1073/pnas.1907921117
13. Sadahiro H, Kang K-D, Gibson JT, Minata M, Yu H, Shi J, et al. Activation of the receptor tyrosine kinase AXL regulates the immune microenvironment in glioblastoma. Cancer Res (2018) 78:3002–13. doi: 10.1158/0008-5472.CAN-17-2433
14. Prinz M, Mildner A. Microglia in the CNS: immigrants from another world. Glia (2011) 59:177–87. doi: 10.1002/glia.21104
15. Ginhoux F, Greter M, Leboeuf M, Nandi S, See P, Gokhan S, et al. Fate mapping analysis reveals that adult microglia derive from primitive macrophages. Science (2010) 330:841–5. doi: 10.1126/science.1194637
16. Goldmann T, Wieghofer P, Jordão MJC, Prutek F, Hagemeyer N, Frenzel K, et al. Origin, fate and dynamics of macrophages at central nervous system interfaces. Nat Immunol (2016) 17:797–805. doi: 10.1038/ni.3423
17. Gomez Perdiguero E, Klapproth K, Schulz C, Busch K, Azzoni E, Crozet L, et al. Tissue-resident macrophages originate from yolk-sac-derived erythro-myeloid progenitors. Nature (2015) 518:547–51. doi: 10.1038/nature13989
18. Crotti A, Ransohoff RM. Microglial physiology and pathophysiology: Insights from genome-wide transcriptional profiling. Immunity (2016) 44:505–15. doi: 10.1016/j.immuni.2016.02.013
19. Sousa C, Biber K, Michelucci A. Cellular and molecular characterization of microglia: A unique immune cell population. Front Immunol (2017) 8:198. doi: 10.3389/fimmu.2017.00198
20. Korin B, Ben-Shaanan TL, Schiller M, Dubovik T, Azulay-Debby H, Boshnak NT, et al. High-dimensional, single-cell characterization of the brain’s immune compartment. Nat Neurosci (2017) 20:1300–9. doi: 10.1038/nn.4610
21. Chinnery HR, Ruitenberg MJ, McMenamin PG. Novel characterization of monocyte-derived cell populations in the meninges and choroid plexus and their rates of replenishment in bone marrow chimeric mice. J Neuropathol Exp Neurol (2010) 69:896–909. doi: 10.1097/NEN.0b013e3181edbc1a
22. Lu-Emerson C, Snuderl M, Kirkpatrick ND, Goveia J, Davidson C, Huang Y, et al. Increase in tumor-associated macrophages after antiangiogenic therapy is associated with poor survival among patients with recurrent glioblastoma. Neuro Oncol (2013) 15:1079–87. doi: 10.1093/neuonc/not082
23. Gomez Perdiguero E, Schulz C, Geissmann F. Development and homeostasis of “resident” myeloid cells: the case of the microglia. Glia (2013) 61:112–20. doi: 10.1002/glia.22393
24. Qian B-Z, Li J, Zhang H, Kitamura T, Zhang J, Campion LR, et al. CCL2 recruits inflammatory monocytes to facilitate breast-tumour metastasis. Nature (2011) 475:222–5. doi: 10.1038/nature10138
25. Arwert EN, Harney AS, Entenberg D, Wang Y, Sahai E, Pollard JW, et al. A unidirectional transition from migratory to perivascular macrophage is required for tumor cell intravasation. Cell Rep (2018) 23:1239–48. doi: 10.1016/j.celrep.2018.04.007
26. Murray PJ, Allen JE, Biswas SK, Fisher EA, Gilroy DW, Goerdt S, et al. Macrophage activation and polarization: Nomenclature and experimental guidelines. Immunity (2014) 41:14–20. doi: 10.1016/j.immuni.2014.06.008
27. Mackaness GB. Cellular resistance to infection. J Exp Med (1962) 116:381–406. doi: 10.1084/jem.116.3.381
28. Michelucci A, Heurtaux T, Grandbarbe L, Morga E, Heuschling P. Characterization of the microglial phenotype under specific pro-inflammatory and anti-inflammatory conditions: Effects of oligomeric and fibrillar amyloid-beta. J Neuroimmunol (2009) 210:3–12. doi: 10.1016/j.jneuroim.2009.02.003
29. Gordon S. Alternative activation of macrophages. Nat Rev Immunol (2003) 3:23–35. doi: 10.1038/nri978
30. Stein M, Keshav S, Harris N, Gordon S. Interleukin 4 potently enhances murine macrophage mannose receptor activity: A marker of alternative immunologic macrophage activation. J Exp Med (1992) 176:287–92. doi: 10.1084/jem.176.1.287
31. Nathan CF, Murray HW, Wiebe ME, Rubin BY. Identification of interferon-gamma as the lymphokine that activates human macrophage oxidative metabolism and antimicrobial activity. J Exp Med (1983) 158:670–89. doi: 10.1084/jem.158.3.670
32. Mantovani A, Sica A, Sozzani S, Allavena P, Vecchi A, Locati M. The chemokine system in diverse forms of macrophage activation and polarization. Trends Immunol (2004) 25:677–86. doi: 10.1016/j.it.2004.09.015
33. Mantovani A, Bottazzi B, Colotta F, Sozzani S, Ruco L. The origin and function of tumor-associated macrophages. Immunol Today (1992) 13:265–70. doi: 10.1016/0167-5699(92)90008-U
34. Wei J, Gabrusiewicz K, Heimberger A. The controversial role of microglia in malignant gliomas. Clin Dev Immunol (2013) 2013:285246. doi: 10.1155/2013/285246
35. Galarneau H, Villeneuve J, Gowing G, Julien J-P, Vallières L. Increased glioma growth in mice depleted of macrophages. Cancer Res (2007) 67:8874–81. doi: 10.1158/0008-5472.CAN-07-0177
36. Rosales AA, Roque RS. Microglia-derived cytotoxic factors. part I: Inhibition of tumor cell growth in vitro. Brain Res (1997) 748:195–204. doi: 10.1016/s0006-8993(96)01301-7
37. Chicoine MR, Zahner M, Won EK, Kalra RR, Kitamura T, Perry A, et al. The in vivo antitumoral effects of lipopolysaccharide against glioblastoma multiforme are mediated in part by toll-like receptor 4. Neurosurgery (2007) 60:372–80. doi: 10.1227/01.NEU.0000249280.61761.2E
38. Zhang L, Alizadeh D, Van Handel M, Kortylewski M, Yu H, Badie B. Stat3 inhibition activates tumor macrophages and abrogates glioma growth in mice. Glia (2009) 57:1458–67. doi: 10.1002/glia.20863
39. Mora R, Abschuetz A, Kees T, Dokic I, Joschko N, Kleber S, et al. TNF-alpha- and TRAIL-resistant glioma cells undergo autophagy-dependent cell death induced by activated microglia. Glia (2009) 57:561–81. doi: 10.1002/glia.20785
40. Hwang S-Y, Yoo B-C, Jung J, Oh E-S, Hwang J-S, Shin J-A, et al. Induction of glioma apoptosis by microglia-secreted molecules: The role of nitric oxide and cathepsin b. Biochim Biophys Acta (2009) 1793:1656–68. doi: 10.1016/j.bbamcr.2009.08.011
41. Brantley EC, Guo L, Zhang C, Lin Q, Yokoi K, Langley RR, et al. Nitric oxide-mediated tumoricidal activity of murine microglial cells. Transl Oncol (2010) 3:380–8. doi: 10.1593/tlo.10208
42. Quail DF, Joyce JA. The microenvironmental landscape of brain tumors. Cancer Cell (2017) 31:326–41. doi: 10.1016/j.ccell.2017.02.009
43. Bettinger I, Thanos S, Paulus W. Microglia promote glioma migration. Acta Neuropathol (2002) 103:351–5. doi: 10.1007/s00401-001-0472-x
44. Wesolowska A, Kwiatkowska A, Slomnicki L, Dembinski M, Master A, Sliwa M, et al. Microglia-derived TGF-beta as an important regulator of glioblastoma invasion–an inhibition of TGF-beta-dependent effects by shRNA against human TGF-beta type II receptor. Oncogene (2008) 27:918–30. doi: 10.1038/sj.onc.1210683
45. Wick W, Platten M, Weller M. Glioma cell invasion: regulation of metalloproteinase activity by TGF-beta. J Neurooncol (2001) 53:177–85. doi: 10.1023/a:1012209518843
46. Markovic DS, Vinnakota K, Chirasani S, Synowitz M, Raguet H, Stock K, et al. Gliomas induce and exploit microglial MT1-MMP expression for tumor expansion. Proc Natl Acad Sci USA (2009) 106:12530–5. doi: 10.1073/pnas.0804273106
47. Vinnakota K, Hu F, Ku M-C, Georgieva PB, Szulzewsky F, Pohlmann A, et al. Toll-like receptor 2 mediates microglia/brain macrophage MT1-MMP expression and glioma expansion. Neuro Oncol (2013) 15:1457–68. doi: 10.1093/neuonc/not115
48. Achyut BR, Angara K, Jain M, Borin TF, Rashid MH, Iskander ASM, et al. Canonical NFκB signaling in myeloid cells is required for the glioblastoma growth. Sci Rep (2017) 7:13754. doi: 10.1038/s41598-017-14079-4
49. Shi Y, Ping Y-F, Zhou W, He Z-C, Chen C, Bian B-S-J, et al. Tumour-associated macrophages secrete pleiotrophin to promote PTPRZ1 signalling in glioblastoma stem cells for tumour growth. Nat Commun (2017) 8:15080. doi: 10.1038/ncomms15080
50. Amaral RF, Geraldo LHM, Einicker-Lamas M, E Spohr TCL de S, Mendes F, Lima FRS. Microglial lysophosphatidic acid promotes glioblastoma proliferation and migration via LPA(1) receptor. J Neurochem (2021) 156:499–512. doi: 10.1111/jnc.15097
51. Carvalho da Fonseca AC, Wang H, Fan H, Chen X, Zhang I, Zhang L, et al. Increased expression of stress inducible protein 1 in glioma-associated microglia/macrophages. J Neuroimmunol (2014) 274:71–7. doi: 10.1016/j.jneuroim.2014.06.021
52. Zhang J, Sarkar S, Cua R, Zhou Y, Hader W, Yong VW. A dialog between glioma and microglia that promotes tumor invasiveness through the CCL2/CCR2/interleukin-6 axis. Carcinogenesis (2012) 33:312–9. doi: 10.1093/carcin/bgr289
53. Zhang X, Chen L, Dang W-Q, Cao M-F, Xiao J-F, Lv S-Q, et al. CCL8 secreted by tumor-associated macrophages promotes invasion and stemness of glioblastoma cells via ERK1/2 signaling. Lab Invest (2020) 100:619–29. doi: 10.1038/s41374-019-0345-3
54. De I, Steffen MD, Clark PA, Patros CJ, Sokn E, Bishop SM, et al. CSF1 overexpression promotes high-grade glioma formation without impacting the polarization status of glioma-associated microglia and macrophages. Cancer Res (2016) 76:2552–60. doi: 10.1158/0008-5472.CAN-15-2386
55. Pyonteck SM, Akkari L, Schuhmacher AJ, Bowman RL, Sevenich L, Quail DF, et al. CSF-1R inhibition alters macrophage polarization and blocks glioma progression. Nat Med (2013) 19:1264–72. doi: 10.1038/nm.3337
56. Coniglio SJ, Eugenin E, Dobrenis K, Stanley ER, West BL, Symons MH, et al. Microglial stimulation of glioblastoma invasion involves epidermal growth factor receptor (EGFR) and colony stimulating factor 1 receptor (CSF-1R) signaling. Mol Med (2012) 18:519–27. doi: 10.2119/molmed.2011.00217
57. Nolte C, Kirchhoff F, Kettenmann H. Epidermal growth factor is a motility factor for microglial cells in vitro: Evidence for EGF receptor expression. Eur J Neurosci (1997) 9:1690–8. doi: 10.1111/j.1460-9568.1997.tb01526.x
58. Huang PH, Xu AM, White FM. Oncogenic EGFR signaling networks in glioma. Sci Signal (2009) 2:re6. doi: 10.1126/scisignal.287re6
59. El Hallani S, Boisselier B, Peglion F, Rousseau A, Colin C, Idbaih A, et al. A new alternative mechanism in glioblastoma vascularization: Tubular vasculogenic mimicry. Brain (2010) 133:973–82. doi: 10.1093/brain/awq044
60. Weller M. Angiogenesis in glioblastoma: just another moving target? Brain (2010) 133:955–6. doi: 10.1093/brain/awq063
61. Takano S, Yamashita T, Ohneda O. Molecular therapeutic targets for glioma angiogenesis. J Oncol (2010) 2010:351908. doi: 10.1155/2010/351908
62. Piao Y, Liang J, Holmes L, Zurita AJ, Henry V, Heymach JV, et al. Glioblastoma resistance to anti-VEGF therapy is associated with myeloid cell infiltration, stem cell accumulation, and a mesenchymal phenotype. Neuro Oncol (2012) 14:1379–92. doi: 10.1093/neuonc/nos158
63. Cui X, Morales R-TT, Qian W, Wang H, Gagner J-P, Dolgalev I, et al. Hacking macrophage-associated immunosuppression for regulating glioblastoma angiogenesis. Biomaterials (2018) 161:164–78. doi: 10.1016/j.biomaterials.2018.01.053
64. Brandenburg S, Müller A, Turkowski K, Radev YT, Rot S, Schmidt C, et al. Resident microglia rather than peripheral macrophages promote vascularization in brain tumors and are source of alternative pro-angiogenic factors. Acta Neuropathol (2016) 131:365–78. doi: 10.1007/s00401-015-1529-6
65. Chen X, Zhang L, Zhang IY, Liang J, Wang H, Ouyang M, et al. RAGE expression in tumor-associated macrophages promotes angiogenesis in glioma. Cancer Res (2014) 74:7285–97. doi: 10.1158/0008-5472.CAN-14-1240
66. Kierdorf K, Fritz G. RAGE regulation and signaling in inflammation and beyond. J Leukoc Biol (2013) 94:55–68. doi: 10.1189/jlb.1012519
67. Chinot OL, Wick W, Mason W, Henriksson R, Saran F, Nishikawa R, et al. Bevacizumab plus radiotherapy-temozolomide for newly diagnosed glioblastoma. N Engl J Med (2014) 370:709–22. doi: 10.1056/NEJMoa1308345
68. Gilbert MR, Dignam JJ, Armstrong TS, Wefel JS, Blumenthal DT, Vogelbaum MA, et al. A randomized trial of bevacizumab for newly diagnosed glioblastoma. N Engl J Med (2014) 370:699–708. doi: 10.1056/NEJMoa1308573
69. Nijaguna MB, Patil V, Urbach S, Shwetha SD, Sravani K, Hegde AS, et al. Glioblastoma-derived macrophage colony-stimulating factor (MCSF) induces microglial release of insulin-like growth factor-binding protein 1 (IGFBP1) to promote angiogenesis. J Biol Chem (2015) 290:23401–15. doi: 10.1074/jbc.M115.664037
70. Locarno CV, Simonelli M, Carenza C, Capucetti A, Stanzani E, Lorenzi E, et al. Role of myeloid cells in the immunosuppressive microenvironment in gliomas. Immunobiology (2020) 225:151853. doi: 10.1016/j.imbio.2019.10.002
71. Perng P, Lim M. Immunosuppressive mechanisms of malignant gliomas: Parallels at non-CNS sites. Front Oncol (2015) 5:153. doi: 10.3389/fonc.2015.00153
72. Paulus W, Baur I, Huettner C, Schmausser B, Roggendorf W, Schlingensiepen KH, et al. Effects of transforming growth factor-beta 1 on collagen synthesis, integrin expression, adhesion and invasion of glioma cells. J Neuropathol Exp Neurol (1995) 54:236–44. doi: 10.1097/00005072-199503000-00010
73. Kostianovsky AM, Maier LM, Anderson RC, Bruce JN, Anderson DE. Astrocytic regulation of human monocytic/microglial activation. J Immunol (2008) 181:5425–32. doi: 10.4049/jimmunol.181.8.5425
74. Suzumura A, Sawada M, Yamamoto H, Marunouchi T. Transforming growth factor-beta suppresses activation and proliferation of microglia in vitro. J Immunol (1993) 151:2150–8.
75. Hussain SF, Yang D, Suki D, Aldape K, Grimm E, Heimberger AB. The role of human glioma-infiltrating microglia/macrophages in mediating antitumor immune responses. Neuro Oncol (2006) 8:261–79. doi: 10.1215/15228517-2006-008
76. Badie B, Bartley B, Schartner J. Differential expression of MHC class II and B7 costimulatory molecules by microglia in rodent gliomas. J Neuroimmunol (2002) 133:39–45. doi: 10.1016/s0165-5728(02)00350-8
77. Dumas AA, Pomella N, Rosser G, Guglielmi L, Vinel C, Millner TO, et al. Microglia promote glioblastoma via mTOR-mediated immunosuppression of the tumour microenvironment. EMBO J (2020) 39:e103790. doi: 10.15252/embj.2019103790
78. West AJ, Tsui V, Stylli SS, Nguyen HPT, Morokoff AP, Kaye AH, et al. The role of interleukin-6-STAT3 signalling in glioblastoma. Oncol Lett (2018) 16:4095–104. doi: 10.3892/ol.2018.9227
79. Wu A, Wei J, Kong L-Y, Wang Y, Priebe W, Qiao W, et al. Glioma cancer stem cells induce immunosuppressive macrophages/microglia. Neuro Oncol (2010) 12:1113–25. doi: 10.1093/neuonc/noq082
80. Mieczkowski J, Kocyk M, Nauman P, Gabrusiewicz K, Sielska M, Przanowski P, et al. Down-regulation of IKKβ expression in glioma-infiltrating microglia/macrophages is associated with defective inflammatory/immune gene responses in glioblastoma. Oncotarget (2015) 6:33077–90. doi: 10.18632/oncotarget.5310
81. Badie B, Schartner J, Prabakaran S, Paul J, Vorpahl J. Expression of fas ligand by microglia: possible role in glioma immune evasion. J Neuroimmunol (2001) 120:19–24. doi: 10.1016/s0165-5728(01)00361-7
82. Didenko VV, Ngo HN, Minchew C, Baskin DS. Apoptosis of T lymphocytes invading glioblastomas multiforme: A possible tumor defense mechanism. J Neurosurg (2002) 96:580–4. doi: 10.3171/jns.2002.96.3.0580
83. Ford AL, Foulcher E, Lemckert FA, Sedgwick JD. Microglia induce CD4 T lymphocyte final effector function and death. J Exp Med (1996) 184:1737–45. doi: 10.1084/jem.184.5.1737
84. Hussain SF, Yang D, Suki D, Grimm E, Heimberger AB. Innate immune functions of microglia isolated from human glioma patients. J Transl Med (2006) 4:15. doi: 10.1186/1479-5876-4-15
85. Ebner F, Brandt C, Thiele P, Richter D, Schliesser U, Siffrin V, et al. Microglial activation milieu controls regulatory T cell responses. J Immunol (2013) 191:5594–602. doi: 10.4049/jimmunol.1203331
86. Wang D, Yang L, Yue D, Cao L, Li L, Wang D, et al. Macrophage-derived CCL22 promotes an immunosuppressive tumor microenvironment via IL-8 in malignant pleural effusion. Cancer Lett (2019) 452:244–53. doi: 10.1016/j.canlet.2019.03.040
87. Korbecki J, Kojder K, Simińska D, Bohatyrewicz R, Gutowska I, Chlubek D, et al. CC chemokines in a tumor: A review of pro-cancer and anti-cancer properties of the ligands of receptors CCR1, CCR2, CCR3, and CCR4. Int J Mol Sci (2020) 21:8412. doi: 10.3390/ijms21218412
88. Campesato LF, Budhu S, Tchaicha J, Weng C-H, Gigoux M, Cohen IJ, et al. Blockade of the AHR restricts a treg-macrophage suppressive axis induced by l-kynurenine. Nat Commun (2020) 11:4011. doi: 10.1038/s41467-020-17750-z
89. Takenaka MC, Gabriely G, Rothhammer V, Mascanfroni ID, Wheeler MA, Chao C-C, et al. Control of tumor-associated macrophages and T cells in glioblastoma via AHR and CD39. Nat Neurosci (2019) 22:729–40. doi: 10.1038/s41593-019-0370-y
90. Heppner FL, Greter M, Marino D, Falsig J, Raivich G, Hövelmeyer N, et al. Experimental autoimmune encephalomyelitis repressed by microglial paralysis. Nat Med (2005) 11:146–52. doi: 10.1038/nm1177
91. Markovic DS, Glass R, Synowitz M, van Rooijen N, Kettenmann H. Microglia stimulate the invasiveness of glioma cells by increasing the activity of metalloprotease-2. J Neuropathol Exp Neurol (2005) 64:754–62. doi: 10.1097/01.jnen.0000178445.33972.a9
92. Han X, Li Q, Lan X, El-Mufti L, Ren H, Wang J. Microglial depletion with clodronate liposomes increases proinflammatory cytokine levels, induces astrocyte activation, and damages blood vessel integrity. Mol Neurobiol (2019) 56:6184–96. doi: 10.1007/s12035-019-1502-9
93. Chen Z, Feng X, Herting CJ, Garcia VA, Nie K, Pong WW, et al. Cellular and molecular identity of tumor-associated macrophages in glioblastoma. Cancer Res (2017) 77:2266–78. doi: 10.1158/0008-5472.CAN-16-2310
94. Wu Y, Lu Y, Chen W, Fu J, Fan R. In silico experimentation of glioma microenvironment development and anti-tumor therapy. PloS Comput Biol (2012) 8:e1002355. doi: 10.1371/journal.pcbi.1002355
95. Cannarile MA, Weisser M, Jacob W, Jegg A-M, Ries CH, Rüttinger D. Colony-stimulating factor 1 receptor (CSF1R) inhibitors in cancer therapy. J Immunother Cancer (2017) 5:53. doi: 10.1186/s40425-017-0257-y
96. Quail DF, Bowman RL, Akkari L, Quick ML, Schuhmacher AJ, Huse JT, et al. The tumor microenvironment underlies acquired resistance to CSF-1R inhibition in gliomas. Science (2016) 352:aad3018. doi: 10.1126/science.aad3018
97. Fujiwara Y, Komohara Y, Kudo R, Tsurushima K, Ohnishi K, Ikeda T, et al. Oleanolic acid inhibits macrophage differentiation into the M2 phenotype and glioblastoma cell proliferation by suppressing the activation of STAT3. Oncol Rep (2011) 26:1533–7. doi: 10.3892/or.2011.1454
98. Fujiwara Y, Komohara Y, Ikeda T, Takeya M. Corosolic acid inhibits glioblastoma cell proliferation by suppressing the activation of signal transducer and activator of transcription-3 and nuclear factor-kappa b in tumor cells and tumor-associated macrophages. Cancer Sci (2011) 102:206–11. doi: 10.1111/j.1349-7006.2010.01772.x
99. Zhang F, Parayath NN, Ene CI, Stephan SB, Koehne AL, Coon ME, et al. Genetic programming of macrophages to perform anti-tumor functions using targeted mRNA nanocarriers. Nat Commun (2019) 10:3974. doi: 10.1038/s41467-019-11911-5
100. De Boeck A, Ahn BY, D’Mello C, Lun X, Menon SV, Alshehri MM, et al. Glioma-derived IL-33 orchestrates an inflammatory brain tumor microenvironment that accelerates glioma progression. Nat Commun (2020) 11:4997. doi: 10.1038/s41467-020-18569-4
101. von Roemeling CA, Wang Y, Qie Y, Yuan H, Zhao H, Liu X, et al. Therapeutic modulation of phagocytosis in glioblastoma can activate both innate and adaptive antitumour immunity. Nat Commun (2020) 11:1508. doi: 10.1038/s41467-020-15129-8
102. Hutter G, Theruvath J, Graef CM, Zhang M, Schoen MK, Manz EM, et al. Microglia are effector cells of CD47-SIRPα antiphagocytic axis disruption against glioblastoma. Proc Natl Acad Sci USA (2019) 116:997–1006. doi: 10.1073/pnas.1721434116
103. Gholamin S, Mitra SS, Feroze AH, Liu J, Kahn SA, Zhang M, et al. Disrupting the CD47-SIRPα anti-phagocytic axis by a humanized anti-CD47 antibody is an efficacious treatment for malignant pediatric brain tumors. Sci Transl Med (2017) 9:eaaf2968. doi: 10.1126/scitranslmed.aaf2968
104. Li F, Lv B, Liu Y, Hua T, Han J, Sun C, et al. Blocking the CD47-SIRPα axis by delivery of anti-CD47 antibody induces antitumor effects in glioma and glioma stem cells. Oncoimmunology (2018) 7:e1391973. doi: 10.1080/2162402X.2017.1391973
105. Ma D, Liu S, Lal B, Wei S, Wang S, Zhan D, et al. Extracellular matrix protein tenascin c increases phagocytosis mediated by CD47 loss of function in glioblastoma. Cancer Res (2019) 79:2697–708. doi: 10.1158/0008-5472.CAN-18-3125
106. Held-Feindt J, Hattermann K, Müerköster SS, Wedderkopp H, Knerlich-Lukoschus F, Ungefroren H, et al. CX3CR1 promotes recruitment of human glioma-infiltrating microglia/macrophages (GIMs). Exp Cell Res (2010) 316:1553–66. doi: 10.1016/j.yexcr.2010.02.018
107. Zhou W, Ke SQ, Huang Z, Flavahan W, Fang X, Paul J, et al. Periostin secreted by glioblastoma stem cells recruits M2 tumour-associated macrophages and promotes malignant growth. Nat Cell Biol (2015) 17:170–82. doi: 10.1038/ncb3090
108. Wang S-C, Yu C-F, Hong J-H, Tsai C-S, Chiang C-S. Radiation therapy-induced tumor invasiveness is associated with SDF-1-regulated macrophage mobilization and vasculogenesis. PloS One (2013) 8:e69182. doi: 10.1371/journal.pone.0069182
109. Wang S-C, Hong J-H, Hsueh C, Chiang C-S. Tumor-secreted SDF-1 promotes glioma invasiveness and TAM tropism toward hypoxia in a murine astrocytoma model. Lab Invest (2012) 92:151–62. doi: 10.1038/labinvest.2011.128
110. Kioi M, Vogel H, Schultz G, Hoffman RM, Harsh GR, Brown JM. Inhibition of vasculogenesis, but not angiogenesis, prevents the recurrence of glioblastoma after irradiation in mice. J Clin Invest (2010) 120:694–705. doi: 10.1172/JCI40283
111. Mercurio L, Ajmone-Cat MA, Cecchetti S, Ricci A, Bozzuto G, Molinari A, et al. Targeting CXCR4 by a selective peptide antagonist modulates tumor microenvironment and microglia reactivity in a human glioblastoma model. J Exp Clin Cancer Res (2016) 35:55. doi: 10.1186/s13046-016-0326-y
112. Platten M, Kretz A, Naumann U, Aulwurm S, Egashira K, Isenmann S, et al. Monocyte chemoattractant protein-1 increases microglial infiltration and aggressiveness of gliomas. Ann Neurol (2003) 54:388–92. doi: 10.1002/ana.10679
113. Wei J, Marisetty A, Schrand B, Gabrusiewicz K, Hashimoto Y, Ott M, et al. Osteopontin mediates glioblastoma-associated macrophage infiltration and is a potential therapeutic target. J Clin Invest (2019) 129:137–49. doi: 10.1172/JCI121266
114. Mi Z, Guo H, Russell MB, Liu Y, Sullenger BA, Kuo PC. RNA Aptamer blockade of osteopontin inhibits growth and metastasis of MDA-MB231 breast cancer cells. Mol Ther (2009) 17:153–61. doi: 10.1038/mt.2008.235
115. Talbot LJ, Mi Z, Bhattacharya SD, Kim V, Guo H, Kuo PC. Pharmacokinetic characterization of an RNA aptamer against osteopontin and demonstration of in vivo efficacy in reversing growth of human breast cancer cells. Surgery (2011) 150:224–30. doi: 10.1016/j.surg.2011.05.015
116. Salacz ME, Kast RE, Saki N, Brüning A, Karpel-Massler G, Halatsch M-E. Toward a noncytotoxic glioblastoma therapy: blocking MCP-1 with the MTZ regimen. Onco Targets Ther (2016) 9:2535–45. doi: 10.2147/OTT.S100407
117. DeNardo DG, Ruffell B. Macrophages as regulators of tumour immunity and immunotherapy. Nat Rev Immunol (2019) 19:369–82. doi: 10.1038/s41577-019-0127-6
118. Butowski N, Colman H, De Groot JF, Omuro AM, Nayak L, Wen PY, et al. Orally administered colony stimulating factor 1 receptor inhibitor PLX3397 in recurrent glioblastoma: An ivy foundation early phase clinical trials consortium phase II study. Neuro Oncol (2016) 18:557–64. doi: 10.1093/neuonc/nov245
Keywords: glioblastoma, glioma, macrophages, microglia, tumor-associated microglia/macrophages (TAMs), immunotherapy
Citation: Wang G, Zhong K, Wang Z, Zhang Z, Tang X, Tong A and Zhou L (2022) Tumor-associated microglia and macrophages in glioblastoma: From basic insights to therapeutic opportunities. Front. Immunol. 13:964898. doi: 10.3389/fimmu.2022.964898
Received: 09 June 2022; Accepted: 05 July 2022;
Published: 27 July 2022.
Edited by:
Tiziana Schioppa, University of Brescia, ItalyReviewed by:
Dingkang Xu, Chinese Academy of Medical Sciences and Peking Union Medical College, ChinaCopyright © 2022 Wang, Zhong, Wang, Zhang, Tang, Tong and Zhou. This is an open-access article distributed under the terms of the Creative Commons Attribution License (CC BY). The use, distribution or reproduction in other forums is permitted, provided the original author(s) and the copyright owner(s) are credited and that the original publication in this journal is cited, in accordance with accepted academic practice. No use, distribution or reproduction is permitted which does not comply with these terms.
*Correspondence: Aiping Tong, YWlwaW5ndG9uZ0BzY3UuZWR1LmNu; Liangxue Zhou, bGlhbmd4dWVfemhvdUAxMjYuY29t
†These authors have contributed equally to this work
Disclaimer: All claims expressed in this article are solely those of the authors and do not necessarily represent those of their affiliated organizations, or those of the publisher, the editors and the reviewers. Any product that may be evaluated in this article or claim that may be made by its manufacturer is not guaranteed or endorsed by the publisher.
Research integrity at Frontiers
Learn more about the work of our research integrity team to safeguard the quality of each article we publish.