- 1International Genome Center, Jiangsu University, Zhenjiang, China
- 2Institute of Immunology, Jiangsu University, Zhenjiang, China
- 3Children’s Hospital of Nanjing Medical University, Nanjing, China
- 4Department of Pathology and Laboratory Medicine, Western University, London, ON, Canada
Cardiac mast cells (CMCs) are multifarious immune cells with complex roles both in cardiac physiological and pathological conditions, especially in cardiac fibrosis. Little is known about the physiological importance of CMCs in cardiac homeostasis and inflammatory process. Therefore, the present review will summarize the recent progress of CMCs on origin, development and replenishment in the heart, including their effects on cardiac development, function and ageing under physiological conditions as well as the roles of CMCs in inflammatory progression and resolution. The present review will shed a light on scientists to understand cardioimmunology and to develop immune treatments targeting on CMCs following cardiac injury.
Introduction
Mast cells, innate non-circulating immune cells, exist in almost all tissues and play key roles in allergic disease and host defense, including the heart. Mast cells are highly heterogeneous, express a range of receptors on their surface and generate a variety of mediators to involve in extensive inflammation and immune regulation through degranulation. Therefore, mast cells are called “sentinels” in harmful conditions, with the ability to rapidly perceive invasion and initiate immune defense and different biochemical programs of homeostasis in time (1). Cardiac mast cells (CMCs) are present in the heart at a low density at homeostasis and is generally detected in the epicardium, endocardium, and myocardium of ventricle and atrium in mice, rats, and humans. Published data show that CMCs density is <1 cell/mm2 in mouse heart (2). Our unpublished data also demonstrate that CMCs account for <3% of CD45+ cells in mouse heart. Therefore, the present review will summarize the novels progress of CMCs on origin, development, replenishment, especially on cardiac development, function and ageing under physiological conditions as well as the roles of CMCs on inflammatory progression and resolution.
Origin, development and survival of CMCs
Mast cells have been always thought to originate from hematopoietic stem cells (HSCs) in bone marrow (3). However, recent data indicate that mast cells probably derive from three embryonic hematopoietic waves: early and late erythron-myeloid progenitors (EMPs) from yolk sac, and definitive HSCs from the aorta, gonads, and mesonephros region (4). Mast cells derived from different hematopoietic waves have different tissue preferences, for example, from the early EMPs distribute in adipose tissue, from late EMPs widely distribute in most connective tissues, and from fetal HSCs are the main cells group in mucosa. It is also suggested that bone marrow derived mast cells mainly replenish the mucosal mast cells (MMCs) after birth (4–6). Mast cells from the embryonic stage are thought to have reached peripheral tissues and matured into resident mast cells before birth, which possess tissue and function heterogeneity. After birth, mast cells precursors from bone marrow need to be released into the bloodstream and recruited by various mediators before entering the peripheral tissues. It is known that plenty of biologic agents, including growth factors, integrins, chemokines and adenosine nucleotides contribute to this recruitment process (7–10). Different mast cells subsets express different receptors which may contribute to their movement into specific tissues. For example, the recruitment to intestine requires α4β7 integrin and chemokine receptor CXCR2 expressed on mast cells progenitors (MCps), accompanying with mucosal addressin cellular adhesion molecule-1 and vascular cell adhesion molecule-1 on intestinal endothelium (11, 12). Furthermore, α4β7 and vascular cell adhesion molecule-1 are also required for the recruitment of mast cells precursors to the lungs (13). However, it is unclear that CMCs are derived from early and late EMPs, or maturation from MCp (14, 15). If it is the later, which factor can mediate the specific homing or recruitment of MCps to heart?
In both mouse and human, obtaining the cell surface and intracellular characteristics of fully differentiated mature mast cells requires a gradual process, that can be regulated by different cytokines, in which stem cell factor (SCF) and IL-3 may play a major role. SCF, the ligand of c-kit, not only facilitates cells migration, but also contributes to their development (16, 17). IL-3 can benefit the multiple hematopoietic lineage differentiation into mast cells in vitro (18, 19). However, IL-3 is not necessary for the generation of mast cells at homeostasis, it does benefit to increase the number (20). Like IL-3, the other cytokines, such as IL-4, IL-9, IL-10 and IL-13 can also synergistically promote mast cell proliferation and differentiation (21).
Then, how are CMCs maintained and renewed? Tissue mast cells are known to be long-lived cells and even after degranulation they can re-granulate and continue to survive, which is dependent on the local SCF levels (22). Because SCF can inactivate FOXO3a, a fork-head transcription factor, and down-regulate and phosphorylate its target Bim (a Bcl-2 homology 3-only proapoptotic protein) which promote mast cells survival (23). Bcl-2 family, well-known proteins, are critical for cells survival and death. Christine Moller and his colleagues are the first to directly demonstrate that both Bcl-x and Bcl-2 are essential for keeping mast cells survival during late development. Nevertheless, the upregulation of Bcl-XL and Bcl-2 by IgE is eliminated for bone marrow derived mast cells in IL-3–/–mice. IL-3 regulates pro-survival Bcl-2 family members (24, 25) and SCF suppresses pro-apoptotic Bim (23). The survival of mast cells depends on the ratio and interaction of anti-apoptotic and pro-apoptotic factors (26). Additionally, fibroblasts can also promote human mast cells survival (27). For example, it has been proved that mouse skin 3T3 fibroblasts can sustain the primary human lung mast cells for 13 days in the absence of exogenous growth factors (28). Although all the mast cells seemed not to have tight junctions and proliferation, these cells still maintained the general morphology, granule morphology and mediator content (29). Furthermore, the co-culture of gut-derived human mast cells with gut fibroblasts has the similar phenomena, and it is also indicated that human fibroblasts promote survival of human mast cells independent of SCF, IL-3, IL-4, and nerve growth factor (NGF). That’s because fibroblasts can release a soluble heat-sensitive molecule that downregulates apoptosis without promoting cell proliferation (30). In addition, an increase of mast cells is noted in the healing myocardium, and their progenitors are also found in the infarcted area, which is related to the activation and proliferation of fibroblasts following cardiac injury or not? The contribution of fibroblasts proliferation cannot be clarified, while the chemotaxis of circulating precursors to the heart may be the main mechanism leading to the accumulation of mast cells in ischemic heart (31). We at least know that fibroblasts contribute to mast cell survival. Other chemokines and cytokines involving in mast cells growth and survival need to be further confirmed in future (Figure 1) (32).
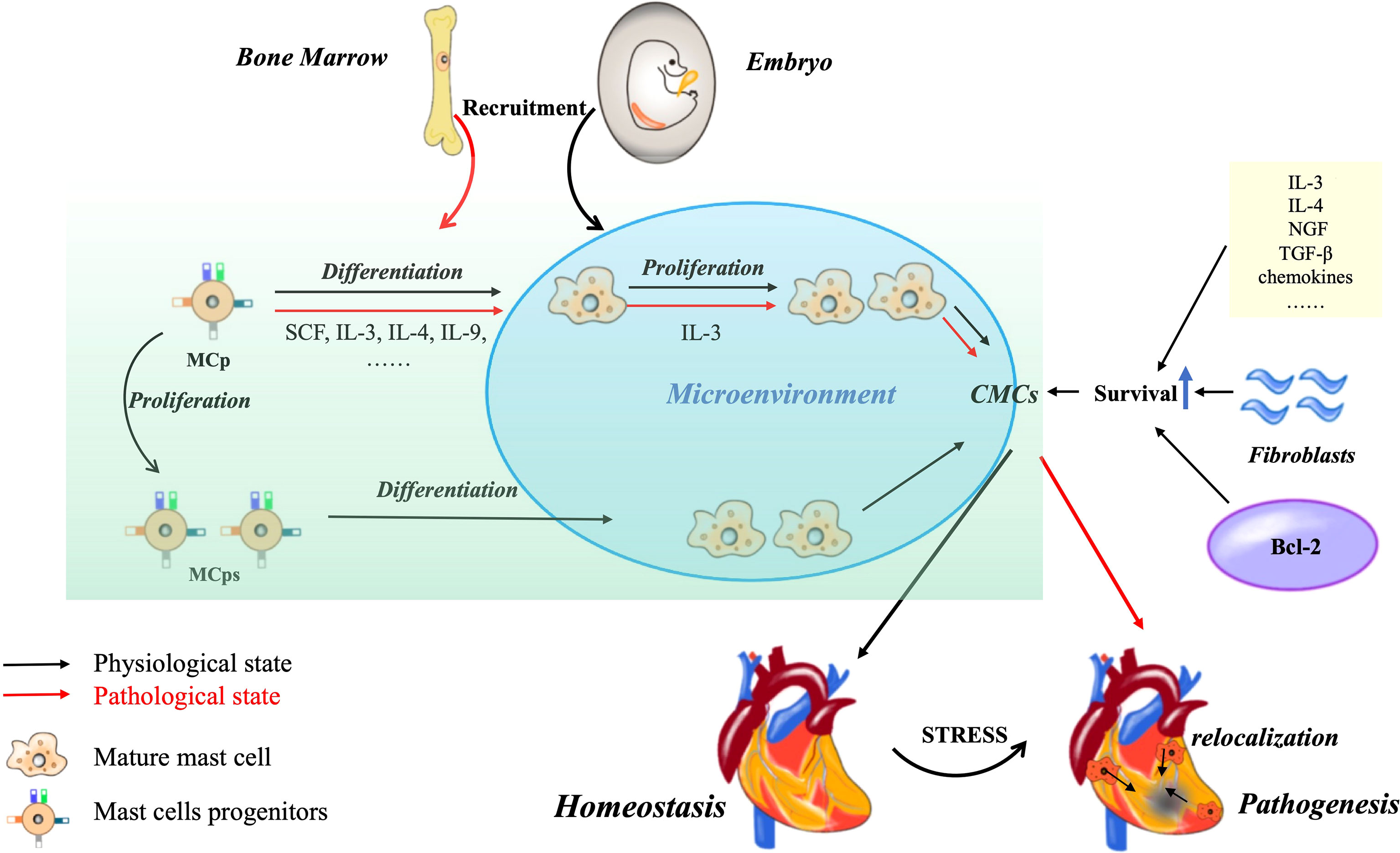
Figure 1 CMCs origin, development and survival. Most of CMCs in the physiological state of the heart come from embryonic stage, and only a small part come from bone marrow. The increase of CMCs can be differentiated from MCp or through self-proliferation. Under pathological conditions, MCps are recruited from bone marrow. The density can also be increased by the relocation of CMCs in non-injured sites. In addition, some cytokine chemokines, cardiac fibroblast derived growth factors and Bcl-2 family can promote the survival of CMCs.
Mast cells subsets
The classic classification of mast cells is based on their tissue distribution and granule content. According to the proteases they contained, mast cells are divided into mainly containing tryptase (MCTs) or chymase (MCCs) or both (MCTCs) (33). In human, almost 90% CMCs are MCTCs (34). MCTs is usually localized to mucosal surfaces and closely related to T cells, especially Th2-type. MCTCs, on the other hand, includes tryptase, chymase, carboxypeptidase and cathepsin G. It mainly exists in the gastrointestinal tract, skin, synovium and subcutaneous tissues. But the proportion and distribution of the two subsets may change in pathological states. For example, the number of MCTCs is increased in fibrotic diseases, but relatively unchanged in allergic or parasitic diseases. Therefore, MCTCs may be biased towards tissue remodeling and angiogenesis, and MCTs contribute to inflammation (35). Besides, mouse mast cells also can be divided into two lineages: inducible bone marrow–mucosal mast cells (MMCs) and constitutive embryonic-derived connective tissue mast cells (CTMCs) (36). Phenotypic differences between CTMCs and MMCs are acquired during the local tissue development, rather than determined by the genetic composition of their MCp or their different location in connective or mucosa tissue (37). In addition, like neutrophils (N1 and N2) and macrophages (M1 and M2), the complex biochemical environment of the tumor may promote mast cells differentiation into anti-tumor MC1 or pro-tumor MC2 (38). On the one hand, mast cells can generate excessive functionally active ROS which may induce cytotoxic effects that can promote tumor regression (39). On the other hand, large amounts of ROS exceed the capacity of cellular DNA repair systems, that may foster the occurrence of tumors. In addition, many other mast cell-derived mediators can also play distinct or even opposite roles in tumorigenesis (40).
The microenvironment promotes formation of specific mast cells phenotype
Heterogeneity is a major feature of mast cells, reflecting the complex interaction between different microenvironmental signals transmitted by tissues and the differentiation programs that determine their phenotypes (41). However, how mast cells form a highly heterogeneous phenotype affected by microenvironment in peripheral tissues has rarely been mentioned. Generally, cells in a given population show heterogeneity, which means that once they show a certain minimum level of variation in one or more characteristics (42). The preliminary studies demonstrate that mast cells at different anatomical positions have significant morphological differences (43). Other studies also show that in addition to differing in morphologic, rat and mouse MMCs and CTMCs appear to differ in many other aspects of biochemistry, histochemical characteristics, function and roles in inflammation and immunity (44–46). Similarly, human mast cells also differ in various aspects of their phenotype, just like morphologic characteristics, histochemistry, contents of proteases and sensitivity to stimulation by secretogogues (47–51). Notably, it has been suggested that the phenotype of mast cells, such as mediator contents or responsive abilities to specific stimuli, can be regulated, at least in some cases reversibly, by microenvironmental signals such as cytokines and growth factor (52). In fact, many potential variations in microenvironment may affect phenotype. The anatomical location is the first factor that affects the phenotype. For example, when cultured mast cells in vitro were transferred into different locations in vivo, which can give the chance to develop into CTMCs or MMCs, depending on local signals (53, 54). Secondly, inflammatory or immune processes may also cause transient changes of mast cells phenotype. For instance, the number of CMCs increase in cardiomyopathy compared to normal myocardium, and a second increase occurred after long-term mechanical support, but the phenotype is conversion from MCTCs into MCTs with the decrease of cardiac fibrosis (55). Furthermore, similar switch also exists in other specific conditions, for example, T cell-dependent response may contribute to mast cells proliferation or maturation/differentiation, high concentrations of eosinophils may benefit the switch of mast cells from MMCs to MCTCs (42). Finally, mast cells may also participate in the regulation of their numbers and phenotypes, especially during inflammation or disease, by autocrine or paracrine or other potential mechanisms (56). For instance, IL-4 possessing growth factor activity for mast cells in mice can promote phenotypic conversion into CTMCs with IL-3 (57). The more detailed mechanisms of the microenvironment on phenotype still need to be explored. Single cell RNA sequencing data of mast cells will provide further insights into heterogeneity as well as clear views of differences between and within different tissues.
CMCs distribution in heart and their functions
In mice, CMCs are mostly distributed in the epicardium (50%) or myocardium (45%), and a fraction is distributed in the endocardium (5%) (58). Similarly, the most CMCs of human are located in the interstitium and in the epicardium (59). Mast cells and their mediators are generally thought to participate in allergic diseases, however, increasing evidences suggest that mast cells may also play protective roles in several other pathological or physiological processes (59, 60). Single-Cell Sequencing shows that CMCs are existence in myocardium and epicardium, and activated and expanding in pressure overload-driven heart failure mouse model (61), furthermore, CMCs infiltration increase atrial fibrillation susceptibility following atrial burst stimulus (62). CMCs increase has been implicated in the chronic volume overload secondary to mitral regurgitation and aorto-caval fistula (63). Furthermore, mast cells in different site may possess the functional heterogeneity (60), for example, tryptase can activate protease-activated receptor 2 (PAR-2) located on cardiomyocytes, which may play a protective role during myocardial infarction (64). Moreover, PAR-2 on nerve fibers and myofibroblasts can also be activated by tryptase, which stimulates the release of substance P from sensory nerve fibers, which in turn activates MRGPRX2 receptors, a family of mas-related G-protein-coupled receptors, on human CMCs (1). The renin and chymase derived from the activated MRGPRX2 receptor, then respectively remove angiotensinogen and angiotensin I (Ang I) to form Ang II. The co-expression of renin and chymase by CMCs is very important for regulating the homeostasis of the cardiac renin-angiotensin system (59). Additionally, immunologic stimuli, bacterial and viral superantigens can activate primary human CMCs to release angiogenic (VEGF-A) and lymphangiogenic (VEGF-C) factors (1, 60, 65). Besides VEGF-A promoting angiogenesis, VEGF-C can also stablize blood pressure, promote lipid metabolism, and coronary artery development (60, 66–68).
CMCs on cardiac development, senescence and function
Most reviews focus on the roles of mast cells in pathological conditions, the present review focuses on the physiological roles. A few studies have suggested that mast cells may participate in the morphogenesis of some mouse organs, such as the mammary glands (69) and corneal (70). The published data demonstrated that the density and number of CMCs are dynamically changed with age in rat (71). Our unpublished data also demonstrate that CMCs exist at embryonic stage in mouse heart. Therefore, we speculate that the CMCs may contribute to cardiac development. Similarly, CMCs density in children was low under the two years old, but the number of CMCs firstly increases and then decreases continuously with age (72). The rapid increase of CMCs density in the early postnatal period accompanies angiogenesis. Furthermore, the corneal mast cells promote corneal angiogenesis (73). All these data suggest that CMCs may play physiological roles on cardiac growth and development. In addition, immune-activated human CMCs can also produce VEGF-A and VEGF-C to induce the formation of new blood vessels and lymphatics, while the similar function in physiological state has not been confirmed (60, 68). CMCs exist not only around the cardiac vessels of neonatal mouse, but also around the nerve fibers. So CMCs might also have a positive effect on nerve development in heart (74, 75).
Multiple evidences indicate that mast cells may be involved in the development of the heart, so whether it has an impact on the aging of the heart? Although there is no direct evidence that mast cells are involved in heart aging, we can speculate from the effect of mast cells on the aging of other tissues and organs. Firstly, the number of mast cells in the mesenteric lymphatic vessels is 27% higher and in the mesentery is 400% higher of the older rats (24 months) compared with the younger rats (9 months) (76). In healthy elderly (≥ 75 years old), the mast cells in the skin increased by 40% compared with the biopsy of young people (≤30 years old) (77). Furthermore, although the liver has only a slight aging process compared to other organs, mast cells also play an important role in this process (32). One study has demonstrated that inhibiting SCF/c-Kit signaling pathway can reduce biliary senescence, with decrease mast cells activation and hepatic damage (78). In conclusion, the increase of mast cells can be detected in a variety of aging organs, so we suspect that they may play a role in the process of organ aging. The effect of mast cells on cardiac senescence can be reflected from two aspects: structure and function (79). It is normally assumed that the damage and apoptosis caused by mast cells to cardiomyocytes will eventually lead to cardiac dysfunction, a manifestation of cardiac aging. Co-culture of mast cells with cardiomyocytes promotes significant cardiomyocytes apoptosis for possibly the exposure to mast cell granules (80). It has been suggested that chymase derived from CMCs may induce myosin degradation in cardiomyocytes (81). Furthermore, activation of CMCs is pro-inflammatory and not only induces apoptosis, but also leads to extracellular matrix degradation, which may lead to eventual myocardial dysfunction (Figure 2) (82). These data suggest that CMCs can induce heart aging, but a more detailed mechanism remains to be explored.
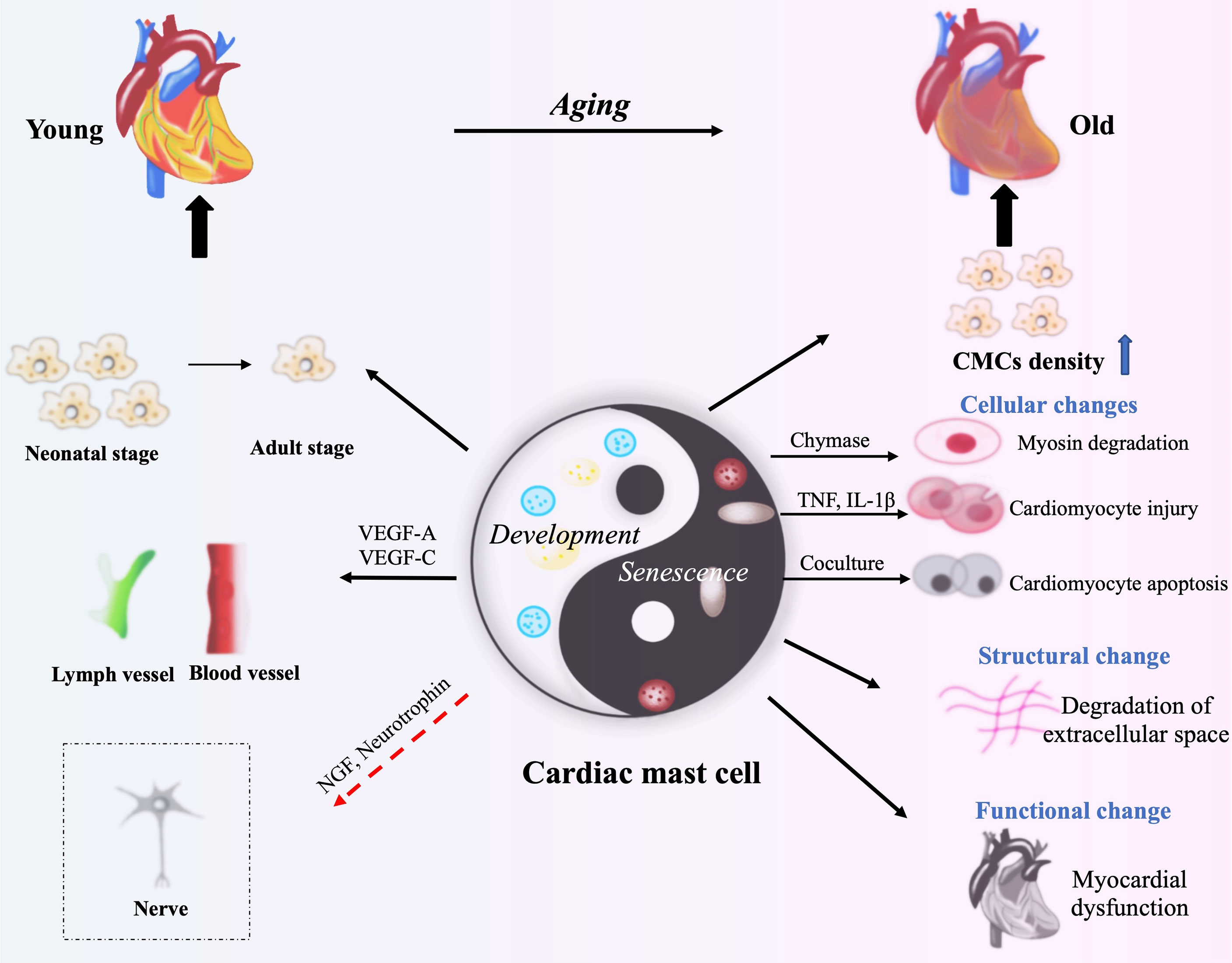
Figure 2 The roles of CMCs in cardiac development and aging. VEGF-A, VEGF-C, NGF and neurotrophin from CMCs contribute to cardiac development through benefiting the formation of blood vessels and lymphatic vessels, and the development of cardiac nerves (neonatal stage). CMCs produce Chymase, TNF and IL-1β to degrade myosin or to damage cardiomyocytes. CMCs’ activation following cardiac injury causes inflammatory response, and then lead to the structural damage and cardiac dysfunction (Old Stage). The red arrow means speculation.
The trigger of CMCs activation and degranulation
Degranulation is considered to be the main way of mast cells playing physiological and pathological roles with IgE as the main trigger. As well known, mast cells express a large number of FcϵRI receptors, once IgE receptor cross-linking and calcium influx lead to mast cell degranulation (83). Currently, there are at least three ways in which mast cells release intracellular mediators, namely kiss-and-run, piecemeal, and compound exocytosis (84). In IgE-mediated allergic reactions, almost all vesicles are released from mast cells within minutes to hours. However, IgE is not the only trigger that stimulates mast cell degranulation, and activation induced by different components also leads to release of different mediators. There are numerous stimulants such as IgG, neuropeptides, cytokines, chemokines, TLR ligands, complements and other inflammatory products, that can directly cause mast cells to degranulate and selectively release mediators to stimulate proliferation, differentiation and migration (85). Mast cells subsets functions are different, not only because of the mediators produced, but also because of different sensitivities to stimulus. In addition to endogenous stimulus, some exogenous molecules can also directly activate mast cells, manifested as drug side effects or aggravating individual allergic state (86). It is worth mentioning that the process of mast cell degranulation in fibrosis is different from that in allergic reactions, and the release of mast cell vesicles may be more frequent and accompany with more subtle symptoms. It can occur by a slow process called piecemeal degranulation, and the vesicles can travel through the lymphatic vessels across the interstitial space to distant lymph nodes. Additionally, less discussed mechanism is the direct penetration of mast cell vesicles into another cell via intercellular contact, known as the transgranulation (83).
CMCs in cardiac inflammation and functional remodeling following injury
The growing evidence shows that CMCs plays an important role in the occurrence and development of cardiovascular diseases (87). After myocardial infarction, CMCs density increase, rapidly degranulate, release a large number of bioactive mediators and initiate a cascade of cytokines to promote early inflammatory healing (88). CMCs play an undeniable role in the cardiac inflammation initiation and resolution. Because optimal healing requires inhibition of chemokine and cytokine synthesis, this leads to regression of inflammation and collagen deposition (31). However, the influence of CMCs on fibrosis remains a focus. CMCs produce a variety of growth factors, angiogenic factors and extracellular matrix regulators. All the products can affect matrix remodeling, promote granulation and scar formation, and have an important role on cardiac remodeling.
Inflammatory Development and Resolution
The association of inflammation with myocardial infarction has been perceived for more than a century and inflammation is properly considered part of the healing process. The involvement of mast cells in inflammation has traditionally been thought to be only one aspect of the allergic response, but this does not seem to be the case. Following cardiac injury, the internal and external factors mentioned above can induce CMCs degranulation, and their derived histamine and TNF activate microvascular endothelium, up-regulate P and E-selectin, respectively, as well as adhesion molecules such as ICAM-1, which affect vascular tension and permeability. Eventually it mediates the infiltration of inflammatory cells, such as neutrophils, basophils, monocytes/macrophages, lymphocytes, etc (85) (89).
Inflammation benefits to cardiac repair, but this effect does not last (88). The release of cytokines and inflammatory cells infiltration directly or indirectly induced by CMCs are significant events in the progression of myocardial infarction, which play a key role in phagocytosis and clearance of dead cells and debris. Nevertheless, this acute inflammatory response is transient and then disappears (31, 90). This may be related to some anti-inflammatory mediators secreted by CMCs, such as IL-10 and IL-13, which can limit the expansion of inflammatory response and protect non-infarcted cardiomyocytes. IL-10 restrains the inflammatory response by inhibiting the production of IL-1α, IL-1β, TNF-α, IL-6, and IL-8 through lipopolysaccharide-activated monocytes (91). This can be demonstrated by the obvious inflammatory response of IL-10 knockout mice after myocardial infarction, which is characterized by increased neutrophil infiltration and elevated blood TNF-α levels (92). The importance of IL-13 on CMCs needs to be further investigated as it is not only derived from CMCs, but also secreted by many other cells in the microenvironment. In addition, mast cells can also exert anti-inflammatory or immunosuppressive effects by releasing mediators that degrade proinflammatory molecules (52). Mast cell proteinase 4 has been shown to degrade mast cell-derived TNF in mice in vitro, and it also can reduce TNF levels in vivo and limit inflammation (93). Besides, IL-37 is an important regulatory cytokine that inhibits inflammation, and mast cells can modulate the anti-inflammatory activity of IL-37 by trypsin-like action, resulting in the more biologically active form of IL-37 (94). Notably, VEGF-C is a major lymphangiogenic factor produced by human CMCs (95, 96), which has a potential cardioprotective effect, as cardiac lymphatic activation contributes to inflammation resolution and plays a crucial role in fighting myocardial edema (60, 97). Furthermore, mast cells can also inhibit inflammation through activation of PAR-2 on cardiomyocytes (64). Timely suppression of the inflammatory mediators such as chemokines and cytokines in healing infarction is critical to the repair process and can inhibit the continuous recruitment of inflammatory cells (31). More detailed anti-inflammatory mechanisms of mast cells remain to be studied. If we can find out the specific mechanism of the occurrence and resolution of cardiac inflammation regulated by CMCs, and then identify clinically appropriate targets, it may bring great improvement to the treatment of cardiovascular disease.
CMCs: Pro-Fibrosis and Anti-Fibrosis
Although the obvious inflammation-related properties of CMCs, its main function in cardiac remodeling is related to the regulation of fibrous tissue metabolism. Cardiac fibrosis is actually an accumulation of the extracellular matrix, such as collagen (89). However, current studies have found that CMCs are double-edged sword in inducing cardiac remodeling, which can not only stimulate collagen synthesis and lead to fibrosis, but also induce matrix metalloproteinase activation and collagen degradation, with ultimately ventricular dilation (87).
Firstly, fibrosis is necessary for proper wound healing which can restores function to damaged tissue after myocardial injury, such as myocardial infarction or hypertension-induced stretch injury. Chymase and tryptase in CMCs have pro-fibrotic properties which are well-known fibroblast activity promoters, can mediate the activation of TGF-β and Ang II. However, fibrotic deposits are essential to restore normal heart function, but excessive remodeling can reduce contractile force and heart function, resulting in chronic heart failure (15). Additionally, CMCs can also secrete some anti-fibrotic mediators, such as IL-10, IL-13, CXCL-10 and VEGF, which have their own anti-fibrotic pathways, respectively (98). For example, IL-10 can reduce fibrotic remodeling by decreasing IL-1β and TNF levels, as well as MMP-9 expression and activity, and by increasing capillary density (99). CMCs-derived IL-13 can induce macrophages with an M2c phenotype, which is associated with reduced fibrosis. Moreover, VEGF-A can increase capillary density in damaged tissues and promote proper repair of cardiac fibrosis (93). At last, CXCL10 has been proved that it can inhibit the migration of fibroblasts to myocardium and delay their phenotypic differentiation into fibrogenic myofibroblasts. (Figure 3) (15). It cannot be ignored that CMCs have significant pro-fibrotic and anti-fibrotic effects, several studies have drawn controversial conclusions and described possible implications for this phenomenon, including harmful, neutral, or protective effects in cardiac remodeling (100). These conflicting conclusions are attributed to the failure to ensure a strictly correct clinical environment and the selection of appropriate animal models (27). Different culture systems, primary cell sources and even the initial cell number used in the experiment are also critical, and subtle differences may lead to different or even contradictory conclusions. To clarify these contradictory results, it is significant to correctly understand the characteristics of each in vitro and in vivo system used to culture mast cells, which can help us understand the real function of CMCs in the heart (21).
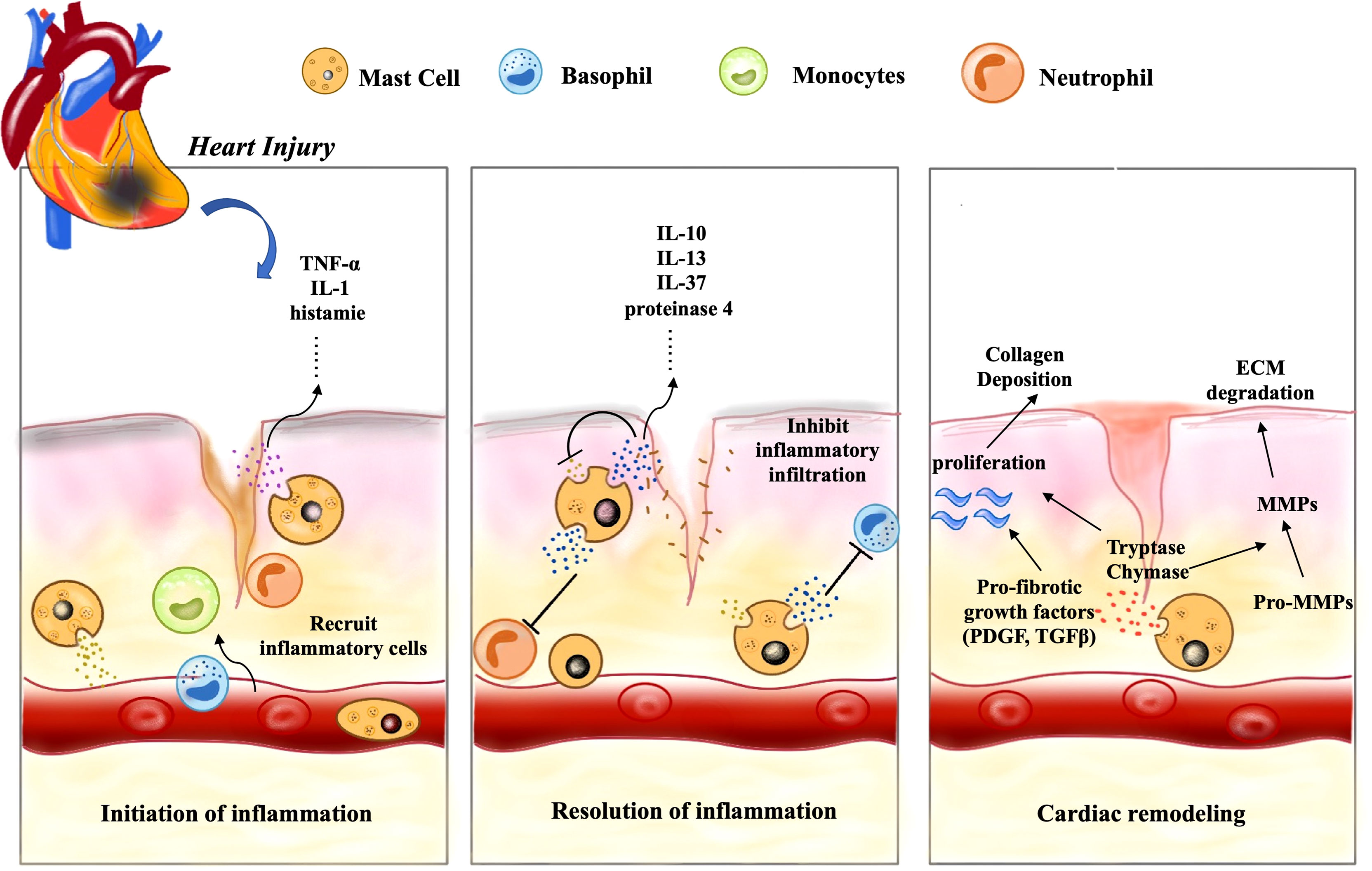
Figure 3 CMCs play an important role in cardiac remodeling following injury. Following cardiac injury, firstly, CMCs are activated, release a large number of inflammatory mediators, such as histamine, TNF-α and IL-1 to change vascular permeability, recruit a lot of inflammatory cells (basophils, monocytes, neutrophils, etc.), and induce early inflammation. Secondly, CMCs also mediate the inflammatory resolution by secreting some anti-inflammatory factors such as IL-10, IL-13 and IL-37. Finally, a series of pro-fibrotic and anti-fibrotic effects coexist and lead to cardiac remodeling.
Future perspective
Like macrophages and dendritic cells, CMCs are highly heterogeneous population of innate immune cells, with different morphological functions, mediator contents and surface receptors. The origin and differentiation of the different subsets remain unclear. CMCs are strategically located in close proximity to cardiomyocytes, coronary microvessels, nerves, and lymphatic vessels. Understanding the specific roles of CMCs in different sites of the heart in pathological and physiological processes will lead to a breakthrough in the treatment of cardiovascular diseases. Although they are distributed in small numbers and proportions within the steady-state heart, we reasonably suspect they are linked to the cardiac development and function, even the aging process. In a word, CMCs are a double-edged sword that may have potentially beneficial or harmful effects. The detailed roles of CMCs in cardiac development and injury remain controversial and contradictory, thus, several key questions about them remain unanswered. For example, the mechanisms about migration and differentiation of CMCs remain to be confirmed: whether CMCs precursors are regulated by specific mediators during migration to heart, whether CMCs proliferate and renew according to the pathway we mentioned above in both presence and absence of pathological injury, and whether cardiac-resident and recruited mast cells play divergent roles during homeostasis. Do different CMCs subsets have the same origin and developmental process, and whether their different phenotypes are changed by their microenvironment or driven by their designated progenitor cells? Specific mechanisms of CMCs on the development and function of the heart remain in the speculative stage. Its effect on the aging of the heart is only inferred from the performance of other organs. Some direct evidence is still lacking. With the growing understanding to CMCs, the other function may be demonstrated in future except for pro-inflammation and pro-fibrosis in cardiac injury. However, the dispute as to whether they perform harmful, neutral or protective activities has also not been resolved.
Author contributions
JJ and YJ collected the material and draw Figures. JJ and ZS wrote the draft. ZS provides ideas and grant. SC revised the manuscript. All authors contributed to the article and approved the submitted version.
Funding
This work was supported by National Natural Science Foundation of China (Grant No. 81871244), Primary Research & Development Plan of Jiangsu Province (BE2019700), Jiangsu Province “333” project (BRA2018016), Six talent peaks project in Jiangsu Province (2019-WSN-122), Projects of International Cooperation from Jiangsu (BX2019100), and international cooperation and exchange from Zhenjiang (GJ2020010).
Conflict of Interest
The authors declare that the research was conducted in the absence of any commercial or financial relationships that could be construed as a potential conflict of interest.
Publisher’s Note
All claims expressed in this article are solely those of the authors and do not necessarily represent those of their affiliated organizations, or those of the publisher, the editors and the reviewers. Any product that may be evaluated in this article, or claim that may be made by its manufacturer, is not guaranteed or endorsed by the publisher.
References
1. Varricchi G, Pecoraro A, Loffredo S, Poto R, Rivellese F, Genovese A, et al. Heterogeneity of Human Mast Cells With Respect to MRGPRX2 Receptor Expression and Function. Front Cell Neurosci (2019) 13:299. doi: 10.3389/fncel.2019.00299
2. Gersch C, Dewald O, Zoerlein M, Michael LH, Entman ML, Frangogiannis NG. Mast Cells and Macrophages in Normal C57/BL/6 Mice. Histochem Cell Biol (2002) 118(1):41–9. doi: 10.1007/s00418-002-0425-z
3. Valent P, Akin C, Hartmann K, Nilsson G, Reiter A, Hermine O, et al. Mast Cells as a Unique Hematopoietic Lineage and Cell System: From Paul Ehrlich's Visions to Precision Medicine Concepts. Theranostics (2020) 10(23):10743–68. doi: 10.7150/thno.46719
4. Li Z, Liu S, Xu J, Zhang X, Han D, Liu J, et al. Adult Connective Tissue-Resident Mast Cells Originate From Late Erythro-Myeloid Progenitors. Immunity (2018) 49(4):640–53.e5. doi: 10.1016/j.immuni.2018.09.023
5. Kitamura Y, Shimada M, Hatanaka K, Miyano Y. Development of Mast Cells From Grafted Bone Marrow Cells in Irradiated Mice. Nature (1977) 268(5619):442–3. doi: 10.1038/268442a0
6. Dahlin JS, Maurer M, Metcalfe DD, Pejler G, Sagi-Eisenberg R, Nilsson G. The Ingenious Mast Cell: Contemporary Insights Into Mast Cell Behavior and Function. Allergy. (2022) 77(1):83–99. doi: 10.1111/all.14881
7. Liu C, Liu Z, Li Z, Wu Y. Molecular Regulation of Mast Cell Development and Maturation. Mol Biol Rep (2010) 37(4):1993–2001. doi: 10.1007/s11033-009-9650-z
8. Meininger CJ, Yano H, Rottapel R, Bernstein A, Zsebo KM, Zetter BR. The C-Kit Receptor Ligand Functions as a Mast Cell Chemoattractant. Blood (1992) 79(4):958–63. doi: 10.1182/blood.V79.4.958.bloodjournal794958
9. Gruber BL, Marchese MJ, Kew R. Angiogenic Factors Stimulate Mast-Cell Migration. Blood. (1995) 86(7):2488–93. doi: 10.1182/blood.V86.7.2488.2488
10. Taub D, Dastych J, Inamura N, Upton J, Kelvin D, Metcalfe D, et al. Bone Marrow-Derived Murine Mast Cells Migrate, But Do Not Degranulate, in Response to Chemokines. J Immunol (1995) 154(5):2393–402.
11. Hallgren J, Gurish MF. Mast Cell Progenitor Trafficking and Maturation. Adv Exp Med Biol (2011) 716:14–28. doi: 10.1007/978-1-4419-9533-9_2
12. Gurish MF, Tao H, Abonia JP, Arya A, Friend DS, Parker CM, et al. Intestinal Mast Cell Progenitors Require CD49dbeta7 (Alpha4beta7 Integrin) for Tissue-Specific Homing. J Exp Med (2001) 194(9):1243–52. doi: 10.1084/jem.194.9.1243
13. Abonia JP, Hallgren J, Jones T, Shi T, Xu Y, Koni P, et al. Alpha-4 Integrins and VCAM-1, But Not MAdCAM-1, are Essential for Recruitment of Mast Cell Progenitors to the Inflamed Lung. Blood (2006) 108(5):1588–94. doi: 10.1182/blood-2005-12-012781
14. Dahlin JS, Hallgren J. Mast Cell Progenitors: Origin, Development and Migration to Tissues. Mol Immunol (2015) 63(1):9–17. doi: 10.1016/j.molimm.2014.01.018
15. Legere SA, Haidl ID, Legare JF, Marshall JS. Mast Cells in Cardiac Fibrosis: New Insights Suggest Opportunities for Intervention. Front Immunol (2019) 10:580. doi: 10.3389/fimmu.2019.00580
16. Tsai M, Takeishi T, Thompson H, Langley KE, Zsebo KM, Metcalfe DD, et al. Induction of Mast Cell Proliferation, Maturation, and Heparin Synthesis by the Rat C-Kit Ligand, Stem Cell Factor. Proc Natl Acad Sci USA (1991) 88(14):6382–6. doi: 10.1073/pnas.88.14.6382
17. Cho KA, Park M, Kim YH, Woo SY. Th17 Cell-Mediated Immune Responses Promote Mast Cell Proliferation by Triggering Stem Cell Factor in Keratinocytes. Biochem Biophys Res Commun (2017) 487(4):856–61. doi: 10.1016/j.bbrc.2017.04.141
18. Ihle JN, Keller J, Oroszlan S, Henderson LE, Copeland TD, Fitch F, et al. Biologic Properties of Homogeneous Interleukin 3. I. Demonstration of WEHI-3 Growth Factor Activity, Mast Cell Growth Factor Activity, P Cell-Stimulating Factor Activity, Colony-Stimulating Factor Activity, and Histamine-Producing Cell-Stimulating Factor Activity. J Immunol (1983) 131(1):282–7.
19. Rennick DM, Lee FD, Yokota T, Arai KI, Cantor H, Nabel GJ. A Cloned MCGF cDNA Encodes a Multilineage Hematopoietic Growth Factor: Multiple Activities of Interleukin 3. J Immunol (1985) 134(2):910–4.
20. Lantz CS, Boesiger J, Song CH, Mach N, Kobayashi T, Mulligan RC, et al. Role for Interleukin-3 in Mast-Cell and Basophil Development and in Immunity to Parasites. Nature. (1998) 392(6671):90–3. doi: 10.1038/32190
21. Hu ZQ, Zhao WH, Shimamura T. Regulation of Mast Cell Development by Inflammatory Factors. Curr Med Chem (2007) 14(28):3044–50. doi: 10.2174/092986707782793998
22. Mekori YA, Oh CK, Metcalfe DD. The Role of C-Kit and its Ligand, Stem Cell Factor, in Mast Cell Apoptosis. Int Arch Allergy Immunol (1995) 107(1-3):136–8. doi: 10.1159/000236955
23. Möller C, Alfredsson J, Engström M, Wootz H, Xiang Z, Lennartsson J, et al. Stem Cell Factor Promotes Mast Cell Survival via Inactivation of FOXO3a-Mediated Transcriptional Induction and MEK-Regulated Phosphorylation of the Proapoptotic Protein Bim. Blood (2005) 106(4):1330–6. doi: 10.1182/blood-2004-12-4792
24. Kohno M, Yamasaki S, Tybulewicz VL, Saito T. Rapid and Large Amount of Autocrine IL-3 Production is Responsible for Mast Cell Survival by IgE in the Absence of Antigen. Blood (2005) 105(5):2059–65. doi: 10.1182/blood-2004-07-2639
25. Karlsson R, Engström M, Jönsson M, Karlberg P, Pronk CJ, Richter J, et al. Phosphatidylinositol 3-Kinase is Essential for Kit Ligand-Mediated Survival, Whereas Interleukin-3 and Flt3 Ligand Induce Expression of Antiapoptotic Bcl-2 Family Genes. J Leukoc Biol (2003) 74(5):923–31. doi: 10.1189/jlb.0403142
26. Moller C, Karlberg M, Abrink M, Nakayama KI, Motoyama N, Nilsson G. Bcl-2 and Bcl-XL are Indispensable for the Late Phase of Mast Cell Development From Mouse Embryonic Stem Cells. Exp Hematol (2007) 35(3):385–93. doi: 10.1016/j.exphem.2006.11.008
27. Bradding P, Pejler G. The Controversial Role of Mast Cells in Fibrosis. Immunol Rev (2018) 282(1):198–231. doi: 10.1111/imr.12626
28. Levi-Schaffer F, Austen KF, Caulfield JP, Hein A, Gravallese PM, Stevens RL. Co-Culture of Human Lung-Derived Mast Cells With Mouse 3T3 Fibroblasts: Morphology and IgE-Mediated Release of Histamine, Prostaglandin D2, and Leukotrienes. J Immunol (1987) 139(2):494–500.
29. Levi-Schaffer F, Kelav-Appelbaum R, Rubinchik E. Human Foreskin Mast Cell Viability and Functional Activity is Maintained Ex Vivo by Coculture With Fibroblasts. Cell Immunol (1995) 162(2):211–6. doi: 10.1006/cimm.1995.1071
30. Sellge G, Lorentz A, Gebhardt T, Levi-Schaffer F, Bektas H, Manns MP, et al. Human Intestinal Fibroblasts Prevent Apoptosis in Human Intestinal Mast Cells by a Mechanism Independent of Stem Cell Factor, IL-3, IL-4, and Nerve Growth Factor. J Immunol (2004) 172(1):260–7. doi: 10.4049/jimmunol.172.1.260
31. Frangogiannis NG. The Mechanistic Basis of Infarct Healing. Antioxid Redox Signal (2006) 8(11-12):1907–39. doi: 10.1089/ars.2006.8.1907
32. Jarido V, Kennedy L, Hargrove L, Demieville J, Thomson J, Stephenson K, et al. The Emerging Role of Mast Cells in Liver Disease. Am J Physiol Gastrointest Liver Physiol (2017) 313(2):G89–101. doi: 10.1152/ajpgi.00333.2016
33. Balakumar P, Singh AP, Ganti SS, Krishan P, Ramasamy S, Singh M. Resident Cardiac Mast Cells: Are They the Major Culprit in the Pathogenesis of Cardiac Hypertrophy? Basic Clin Pharmacol Toxicol (2008) 102(1):5–9. doi: 10.1111/j.1742-7843.2007.00147.x
34. Sperr WR, Bankl HC, Mundigler G, Klappacher G, Grossschmidt K, Agis H, et al. The Human Cardiac Mast Cell: Localization, Isolation, Phenotype, and Functional Characterization. Blood (1994) 84(11):3876–84. doi: 10.1182/blood.V84.11.3876.bloodjournal84113876
35. Krishnaswamy G, Kelley J, Johnson D, Youngberg G, Stone W, Huang SK, et al. The Human Mast Cell: Functions in Physiology and Disease. Front Biosci (2001) 6:D1109–27. doi: 10.2741/krishnas
36. Derakhshan T, Samuchiwal SK, Hallen N, Bankova LG, Boyce JA, Barrett NA, et al. Lineage-Specific Regulation of Inducible and Constitutive Mast Cells in Allergic Airway Inflammation. J Exp Med (2021) 218(1):e20200321. doi: 10.1084/jem.20200321
37. Gurish MF, Austen KF. Developmental Origin and Functional Specialization of Mast Cell Subsets. Immunity (2012) 37(1):25–33. doi: 10.1016/j.immuni.2012.07.003
38. Varricchi G, de Paulis A, Marone G, Galli SJ. Future Needs in Mast Cell Biology. Int J Mol Sci (2019) 20(18):4397. doi: 10.3390/ijms20184397
39. Swindle EJ, Metcalfe DD, Coleman JW. Rodent and Human Mast Cells Produce Functionally Significant Intracellular Reactive Oxygen Species But Not Nitric Oxide. J Biol Chem (2004) 279(47):48751–9. doi: 10.1074/jbc.M409738200
40. Kessenbrock K, Plaks V, Werb Z. Matrix Metalloproteinases: Regulators of the Tumor Microenvironment. Cell (2010) 141(1):52–67. doi: 10.1016/j.cell.2010.03.015
41. Frossi B, Mion F, Sibilano R, Danelli L, Pucillo CEM. Is it Time for a New Classification of Mast Cells? What do We Know About Mast Cell Heterogeneity? Immunol Rev (2018) 282(1):35–46. doi: 10.1111/imr.12636
42. Galli SJ. New Insights Into "the Riddle of the Mast Cells": Microenvironmental Regulation of Mast Cell Development and Phenotypic Heterogeneity. Lab Invest (1990) 62(1):5–33. doi: 10.1007/978-1-4612-0485-5_5
43. Hardy WB, Wesbrook FF. The Wandering Cells of the Alimentary Canal. J Physiol (1895) 18(5-6):490–i3. doi: 10.1113/jphysiol.1895.sp000582
44. Mayrhofer G, Fisher R. Mast Cells in Severely T-Cell Depleted Rats and the Response to Infestation With Nippostrongylus Brasiliensis. Immunology (1979) 37(1):145–55.
45. Jarrett EE, Haig DM. Mucosal Mast Cells In Vivo and In Vitro. Immunol Today (1984) 5(4):115–9. doi: 10.1016/0167-5699(84)90046-X
46. Befus AD, Pearce FL, Gauldie J, Horsewood P, Bienenstock J. Mucosal Mast Cells. I. Isolation and Functional Characteristics of Rat Intestinal Mast Cells. J Immunol (1982) 128(6):2475–80.
47. Razin E, Stevens RL, Akiyama F, Schmid K, Austen KF. Culture From Mouse Bone Marrow of a Subclass of Mast Cells Possessing a Distinct Chondroitin Sulfate Proteoglycan With Glycosaminoglycans Rich in N-Acetylgalactosamine-4,6-Disulfate. J Biol Chem (1982) 257(12):7229–36. doi: 10.1016/S0021-9258(18)34561-7
48. Kawanami O, Ferrans VJ, Fulmer JD, Crystal RG. Ultrastructure of Pulmonary Mast Cells in Patients With Fibrotic Lung Disorders. Lab Invest (1979) 40(6):717–34.
49. Ruitenberg EJ, Gustowska L, Elgersma A, Ruitenberg HM. Effect of Fixation on the Light Microscopical Visualization of Mast Cells in the Mucosa and Connective Tissue of the Human Duodenum. Int Arch Allergy Appl Immunol (1982) 67(3):233–8. doi: 10.1159/000233024
50. Leung KB, Flint KC, Brostoff J, Hudspith BN, Johnson NM, Lau HY, et al. Effects of Sodium Cromoglycate and Nedocromil Sodium on Histamine Secretion From Human Lung Mast Cells. Thorax (1988) 43(10):756–61. doi: 10.1136/thx.43.10.756
51. Lawrence ID, Warner JA, Cohan VL, Hubbard WC, Kagey-Sobotka A, Lichtenstein LM. Purification and Characterization of Human Skin Mast Cells. Evidence for Human Mast Cell Heterogeneity. J Immunol (1987) 139(9):3062–9.
52. Galli SJ, Kalesnikoff J, Grimbaldeston MA, Piliponsky AM, Williams CM, Tsai M. Mast Cells as "Tunable" Effector and Immunoregulatory Cells: Recent Advances. Annu Rev Immunol (2005) 23:749–86. doi: 10.1146/annurev.immunol.21.120601.141025
53. Nakano T, Sonoda T, Hayashi C, Yamatodani A, Kanayama Y, Yamamura T, et al. Fate of Bone Marrow-Derived Cultured Mast Cells After Intracutaneous, Intraperitoneal, and Intravenous Transfer Into Genetically Mast Cell-Deficient W/Wv Mice. Evidence That Cultured Mast Cells can Give Rise to Both Connective Tissue Type and Mucosal Mast Cells. J Exp Med (1985) 162(3):1025–43. doi: 10.1084/jem.162.3.1025
54. Otsu K, Nakano T, Kanakura Y, Asai H, Katz HR, Austen KF, et al. Phenotypic Changes of Bone Marrow-Derived Mast Cells After Intraperitoneal Transfer Into W/Wv Mice That are Genetically Deficient in Mast Cells. J Exp Med (1987) 165(3):615–27. doi: 10.1084/jem.165.3.615
55. Akgul A, Skrabal CA, Thompson LO, Loebe M, Lafuente JA, Noon GP, et al. Role of Mast Cells and Their Mediators in Failing Myocardium Under Mechanical Ventricular Support. J Heart Lung Transplant (2004) 23(6):709–15. doi: 10.1016/j.healun.2003.06.006
56. Galli SJ. Mast Cells and Basophils. Curr Opin Hematol (2000) 7(1):32–9. doi: 10.1097/00062752-200001000-00007
57. Hamaguchi Y, Kanakura Y, Fujita J, Takeda S, Nakano T, Tarui S, et al. Interleukin 4 as an Essential Factor for In Vitro Clonal Growth of Murine Connective Tissue-Type Mast Cells. J Exp Med (1987) 165(1):268–73. doi: 10.1084/jem.165.1.268
58. Ingason AB, Mechmet F, Atacho DAM, Steingrímsson E, Petersen PH. Distribution of Mast Cells Within the Mouse Heart and its Dependency on Mitf. Mol Immunol (2019) 105:9–15. doi: 10.1016/j.molimm.2018.11.009
59. Varricchi G, Marone G, Kovanen PT. Cardiac Mast Cells: Underappreciated Immune Cells in Cardiovascular Homeostasis and Disease. Trends Immunol (2020) 41(8):734–46. doi: 10.1016/j.it.2020.06.006
60. Varricchi G, Rossi FW, Galdiero MR, Granata F, Criscuolo G, Spadaro G, et al. Physiological Roles of Mast Cells: Collegium Internationale Allergologicum Update 2019. Int Arch Allergy Immunol (2019) 179(4):247–61. doi: 10.1159/000500088
61. Martini E, Kunderfranco P, Peano C, Carullo P, Cremonesi M, Schorn T, et al. Single-Cell Sequencing of Mouse Heart Immune Infiltrate in Pressure Overload-Driven Heart Failure Reveals Extent of Immune Activation. Circulation (2019) 140(25):2089–107. doi: 10.1161/CIRCULATIONAHA.119.041694
62. Liao CH, Akazawa H, Tamagawa M, Ito K, Yasuda N, Kudo Y, et al. Cardiac Mast Cells Cause Atrial Fibrillation Through PDGF-A-Mediated Fibrosis in Pressure-Overloaded Mouse Hearts. J Clin Invest (2010) 120(1):242–53. doi: 10.1172/JCI39942
63. Janicki JS, Brower GL, Gardner JD, Forman MF, Stewart JA Jr., Murray DB, et al. Cardiac Mast Cell Regulation of Matrix Metalloproteinase-Related Ventricular Remodeling in Chronic Pressure or Volume Overload. Cardiovasc Res (2006) 69(3):657–65. doi: 10.1016/j.cardiores.2005.10.020
64. Ngkelo A, Richart A, Kirk JA, Bonnin P, Vilar J, Lemitre M, et al. Mast Cells Regulate Myofilament Calcium Sensitization and Heart Function After Myocardial Infarction. J Exp Med (2016) 213(7):1353–74. doi: 10.1084/jem.20160081
65. Varricchi G, Loffredo S, Borriello F, Pecoraro A, Rivellese F, Genovese A, et al. Superantigenic Activation of Human Cardiac Mast Cells. Int J Mol Sci (2019) 20(8):1828. doi: 10.3390/ijms20081828
66. Machnik A, Neuhofer W, Jantsch J, Dahlmann A, Tammela T, Machura K, et al. Macrophages Regulate Salt-Dependent Volume and Blood Pressure by a Vascular Endothelial Growth Factor-C-Dependent Buffering Mechanism. Nat Med (2009) 15(5):545–52. doi: 10.1038/nm.1960
67. Martel C, Li W, Fulp B, Platt AM, Gautier EL, Westerterp M, et al. Lymphatic Vasculature Mediates Macrophage Reverse Cholesterol Transport in Mice. J Clin Invest (2013) 123(4):1571–9. doi: 10.1172/JCI63685
68. Chen HI, Poduri A, Numi H, Kivela R, Saharinen P, McKay AS, et al. VEGF-C and Aortic Cardiomyocytes Guide Coronary Artery Stem Development. J Clin Invest (2014) 124(11):4899–914. doi: 10.1172/JCI77483
69. Lilla JN, Werb Z. Mast Cells Contribute to the Stromal Microenvironment in Mammary Gland Branching Morphogenesis. Dev Biol (2010) 337(1):124–33. doi: 10.1016/j.ydbio.2009.10.021
70. Liu J, Fu T, Song F, Xue Y, Xia C, Liu P, et al. Mast Cells Participate in Corneal Development in Mice. Sci Rep (2015) 5:17569. doi: 10.1038/srep17569
71. Yong LC, Watkins SG, Boland JE. The Mast Cell: III. Distribution and Maturation in Various Organs of the Young Rat. Pathology (1979) 11(3):427–45. doi: 10.3109/00313027909059020
72. Hellstrom B, Holmgren H. Numerical Distribution of Mast Cells in the Human Skin and Heart. Acta Anat (Basel) (1950) 10(1-2):81–107. doi: 10.1159/000140456
73. Rakusan K, Sarkar K, Turek Z, Wicker P. Mast Cells in the Rat Heart During Normal Growth and in Cardiac Hypertrophy. Circ Res (1990) 66(2):511–6. doi: 10.1161/01.RES.66.2.511
74. Blennerhassett MG. Nerve and Mast Cell Interaction: Cell Conflict or Information Exchange? Prog Clin Biol Res (1994) 390:225–41.
75. Kritas SK, Caraffa A, Antinolfi P, Saggini A, Pantalone A, Rosati M, et al. Nerve Growth Factor Interactions With Mast Cells. Int J Immunopathol Pharmacol (2014) 27(1):15–9. doi: 10.1177/039463201402700103
76. Chatterjee V, Gashev AA. Aging-Associated Shifts in Functional Status of Mast Cells Located by Adult and Aged Mesenteric Lymphatic Vessels. Am J Physiol Heart Circ Physiol (2012) 303(6):H693–702. doi: 10.1152/ajpheart.00378.2012
77. Pilkington SM, Barron MJ, Watson REB, Griffiths CEM, Bulfone-Paus S. Aged Human Skin Accumulates Mast Cells With Altered Functionality That Localize to Macrophages and Vasoactive Intestinal Peptide-Positive Nerve Fibres. Br J Dermatol (2019) 180(4):849–58. doi: 10.1111/bjd.17268
78. Meadows V, Kennedy L, Hargrove L, Demieville J, Meng F, Virani S, et al. Downregulation of Hepatic Stem Cell Factor by Vivo-Morpholino Treatment Inhibits Mast Cell Migration and Decreases Biliary Damage/Senescence and Liver Fibrosis in Mdr2-/- Mice. Biochim Biophys Acta Mol Basis Dis (2019) 1865(12):165557. doi: 10.1016/j.bbadis.2019.165557
79. Steenman M, Lande G. Cardiac Aging and Heart Disease in Humans. Biophys Rev (2017) 9(2):131–7. doi: 10.1007/s12551-017-0255-9
80. Hara M, Matsumori A, Ono K, Kido H, Hwang MW, Miyamoto T, et al. Mast Cells Cause Apoptosis of Cardiomyocytes and Proliferation of Other Intramyocardial Cells In Vitro. Circulation (1999) 100(13):1443–9. doi: 10.1161/01.CIR.100.13.1443
81. Powell PC, Wei CC, Fu L, Pat B, Bradley WE, Collawn JF, et al. Chymase Uptake by Cardiomyocytes Results in Myosin Degradation in Cardiac Volume Overload. Heliyon (2019) 5(4):e01397. doi: 10.1016/j.heliyon.2019.e01397
82. Kritikou E, Kuiper J, Kovanen PT, Bot I. The Impact of Mast Cells on Cardiovascular Diseases. Eur J Pharmacol (2016) 778:103–15. doi: 10.1016/j.ejphar.2015.04.050
83. Hugle T. Beyond Allergy: The Role of Mast Cells in Fibrosis. Swiss Med Wkly (2014) 144:w13999. doi: 10.4414/smw.2014.13999
84. Sahid MNA, Kiyoi T. Mast Cell Activation Markers for In Vitro Study. J Immunoassay Immunochem (2020) 41(4):778–816. doi: 10.1080/15321819.2020.1769129
85. McNeil HP, Gotis-Graham I. Human Mast Cell Subsets–Distinct Functions in Inflammation? Inflammation Res (2000) 49(1):3–7. doi: 10.1007/PL00012386
86. Yu Y, Blokhuis BR, Garssen J, Redegeld FA. Non-IgE Mediated Mast Cell Activation. Eur J Pharmacol (2016) 778:33–43. doi: 10.1016/j.ejphar.2015.07.017
87. Janicki JS, Brower GL, Levick SP. The Emerging Prominence of the Cardiac Mast Cell as a Potent Mediator of Adverse Myocardial Remodeling. Methods Mol Biol (2015) 1220:121–39. doi: 10.1007/978-1-4939-1568-2_8
88. Shao Z, Nazari M, Guo L, Li SH, Sun J, Liu SM, et al. The Cardiac Repair Benefits of Inflammation do Not Persist: Evidence From Mast Cell Implantation. J Cell Mol Med (2015) 19(12):2751–62. doi: 10.1111/jcmm.12703
89. Legere SA, Haidl ID, Castonguay MC, Brunt KR, Legare JF, Marshall JS, et al. Increased Mast Cell Density is Associated With Decreased Fibrosis in Human Atrial Tissue. J Mol Cell Cardiol (2020) 149:15–26. doi: 10.1016/j.yjmcc.2020.09.001
90. Dewald O, Ren G, Duerr GD, Zoerlein M, Klemm C, Gersch C, et al. Of Mice and Dogs: Species-Specific Differences in the Inflammatory Response Following Myocardial Infarction. Am J Pathol (2004) 164(2):665–77. doi: 10.1016/S0002-9440(10)63154-9
91. Pereira Beserra F, Fernando Sérgio Gushiken L, Fernanda Hussni M, Helena Pellizzon C. Regulatory Mechanisms and Chemical Signaling of Mediators Involved in the Inflammatory Phase of Cutaneous Wound Healing. Wound Healing - Curr Perspect (2019) 5–22. doi: 10.5772/intechopen.81731
92. Yang Z, Zingarelli B, Szabó C. Crucial Role of Endogenous Interleukin-10 Production in Myocardial Ischemia/Reperfusion Injury. Circulation. (2000) 101(9):1019–26. doi: 10.1161/01.CIR.101.9.1019
93. Mukai K, Tsai M, Saito H, Galli SJ. Mast Cells as Sources of Cytokines, Chemokines, and Growth Factors. Immunol Rev (2018) 282(1):121–50. doi: 10.1111/imr.12634
94. Theoharides TC, Tsilioni I, Conti P. Mast Cells May Regulate The Anti-Inflammatory Activity of IL-37. Int J Mol Sci (2019) 20(15):3701. doi: 10.3390/ijms20153701
95. Dieterich LC, Detmar M. Tumor Lymphangiogenesis and New Drug Development. Adv Drug Delivery Rev (2016) 99(Pt B):148–60. doi: 10.1016/j.addr.2015.12.011
96. Karaman S, Detmar M. Mechanisms of Lymphatic Metastasis. J Clin Invest (2014) 124(3):922–8. doi: 10.1172/JCI71606
97. Vieira JM, Norman S, Villa Del Campo C, Cahill TJ, Barnette DN, Gunadasa-Rohling M, et al. The Cardiac Lymphatic System Stimulates Resolution of Inflammation Following Myocardial Infarction. J Clin Invest (2018) 128(8):3402–12. doi: 10.1172/JCI97192
98. Kologrivova I, Shtatolkina M, Suslova T, Ryabov V. Cells of the Immune System in Cardiac Remodeling: Main Players in Resolution of Inflammation and Repair After Myocardial Infarction. Front Immunol (2021) 12:664457. doi: 10.3389/fimmu.2021.664457
99. Verma SK, Garikipati VNS, Krishnamurthy P, Schumacher SM, Grisanti LA, Cimini M, et al. Interleukin-10 Inhibits Bone Marrow Fibroblast Progenitor Cell-Mediated Cardiac Fibrosis in Pressure-Overloaded Myocardium. Circulation (2017) 136(10):940–53. doi: 10.1161/CIRCULATIONAHA.117.027889
Keywords: cardiac mast cells, cardiac development, cardiac aging, cardiac injury, inflammation
Citation: Jin J, Jiang Y, Chakrabarti S and Su Z (2022) Cardiac Mast Cells: A Two-Head Regulator in Cardiac Homeostasis and Pathogenesis Following Injury. Front. Immunol. 13:963444. doi: 10.3389/fimmu.2022.963444
Received: 07 June 2022; Accepted: 24 June 2022;
Published: 15 July 2022.
Edited by:
Guo-Chang Fan, University of Cincinnati, United StatesReviewed by:
Kun Yang, East Tennessee State University, United StatesYonggang Ma, University of South Florida, United States
Lu Wang, Renmin Hospital of Wuhan University, China
Copyright © 2022 Jin, Jiang, Chakrabarti and Su. This is an open-access article distributed under the terms of the Creative Commons Attribution License (CC BY). The use, distribution or reproduction in other forums is permitted, provided the original author(s) and the copyright owner(s) are credited and that the original publication in this journal is cited, in accordance with accepted academic practice. No use, distribution or reproduction is permitted which does not comply with these terms.
*Correspondence: Zhaoliang Su, c3psMzBAdWpzLmVkdS5jbg==
†These authors have contributed equally to this work